- 1College of Chinese Materia Medica and Yunnan Key Laboratory of Southern Medicinal Utilization, Yunnan University of Chinese Medicine, Kunming, China
- 2Center for Life Sciences, School of Life Sciences, Yunnan University, Kunming, China
With the improvement of living conditions and the popularity of unhealthy eating and living habits, obesity is becoming a global epidemic. Obesity is now recognized as a disease that not only increases the risk of metabolic diseases such as type 2 diabetes (T2D), non-alcoholic fatty liver disease (NAFLD), cardiovascular disease (CVD), and cancer but also negatively affects longevity and the quality of life. The traditional Chinese medicines (TCMs) are highly enriched in bioactive compounds and have been used for the treatment of obesity and obesity-related metabolic diseases over a long period of time. In this review, we selected the most commonly used anti-obesity or anti-hyperlipidemia TCMs and, where known, their major bioactive compounds. We then summarized their multi-target molecular mechanisms, specifically focusing on lipid metabolism, including the modulation of lipid absorption, reduction of lipid synthesis, and increase of lipid decomposition and lipid transportation, as well as the regulation of appetite. This review produces a current and comprehensive understanding of integrative and systematic mechanisms for the use of TCMs for anti-obesity. We also advocate taking advantage of TCMs as another therapy for interventions on obesity-related diseases, as well as stressing the fact that more is needed to be done, scientifically, to determine the active compounds and modes of action of the TCMs.
Introduction
The state of being overweight and obesity are defined as the abnormal or excessive accumulation of fat, mostly in the form of triacylglycerols (TAGs) and cholesterol esters (CEs) in adipose and non-adipose tissues or organs. The World Health Organization (WHO) classification uses body mass index (BMI) to define overweight as being 25–29.9 kg/m2 and obesity as being ≥30 kg/m2 (https://www.who.int/topics/obesity/zh/). Along with an increasing accessibility to food and the popularity of unhealthy lifestyles, obesity is becoming a global epidemic and its metabolic consequences are currently among the most pressing public health challenges (Hossain et al., 2007). The primary consequence of obesity is associated with an increased mortality and a susceptibility to comorbidities, with few viable therapeutic interventions being available. Today, obesity is increasingly gaining attention due to its intimate association with a growing list of diseases beyond T2D, NAFLD, cancers (Stoll, 1998; Arem and Irwin, 2013; Seo et al., 2015; Incio et al., 2018), and CVD (Lazo and Clark, 2008; Zhao et al., 2019), such as atherosclerosis (AS) (Rocha and Libby, 2009; Aboonabi et al., 2019). Meanwhile, obesity also has a substantial impact on the quality of life. Obesity is usually associated with a lower health-related quality of life than those possessing a normal weight (Pinhas-Hamiel et al., 2006). In children and adolescents, obesity is usually associated with sedentary lifestyles, lower levels of self-esteem, social exclusion, poor educational achievements, and so on (de Beer et al., 2007; Wille et al., 2008). In addition, global health costs associated with obesity and its complications are estimated to be ∼US$2 trillion (Dobbs et al., 2014). If the prevalence of obesity continues on its rising trend, almost half of the world’s adult population could be overweight or obese by 2030, imposing even greater personal, social, and economic costs (https://www.mckinsey.com/industries/healthcare-systems-and-services).
Effectively combating obesity around the world may require a comprehensive strategy involving multiple interventions (Dobbs et al., 2014). Management of obesity is aimed at weight loss, which improves the quality of life. Studies have shown that weight loss after treatment was associated with varying degrees of improvement in obesity-related psychosocial problems, physical functioning, physical role functioning, bodily pain, general health, mental health, and vitality (Kaukua et al., 2003; Pearl et al., 2018). To lose weight, lifestyle interventions, dietary changes, and physical activity are the first-line approaches, followed by medical treatment and bariatric surgery. Thus far, several drugs have been approved for weight loss, such as orlistat, liraglutide, lorcaserin, and diethylpropion (Solas et al., 2016; Gadde et al., 2018). However, in addition to the considerable financial cost of these drugs, numerous side effects have been increasingly reported, such as headache, dizziness, fatigue, nausea, dry mouth, insomnia, anxiety, and constipation (Smith et al., 2010; Aronne et al., 2013; Pi-Sunyer et al., 2015; Nissen et al., 2016; Solas et al., 2016; Gadde et al., 2018).
The pathogenesis of obesity is complex and determined by the interaction of genetic, environmental, and psychosocial factors acting through several physiological mediators (González-Muniesa et al., 2017). Many studies have reported that lipid metabolic pathways are a potential therapeutic target to prevent or delay the occurrence and progression of obesity and obesity-related metabolic diseases, as the major physiological factor in these diseases is the disturbance of lipid metabolism, such as a dysfunction in lipid absorption, lipid synthesis, lipid decomposition, and/or lipid transportation (Meikle and Summers, 2017). Compared with the modern drugs mentioned above, TCMs have been widely used to treat obesity for a very long time. This fact alone suggests that TCMs may be used as a vast resource for the development of natural anti-obesity drugs possessing fewer side effects (Li et al., 2017; Martel et al., 2017; Zhang Y. et al., 2018; Zhang et al., 2020; Ji-Ping et al., 2021). While some of the anti-obesity effects and mechanisms of TCMs have been studied in the past decade, most of these studies only focused on single/several genes or signaling pathways involved in lipid metabolism. Our goal in this review is to collate these data and give a systematic and comprehensive overview of the anti-obesity effects and mechanisms of TCMs and their major ingredients by targeting lipid metabolism.
The Traditional Chinese Medicines With Anti-Obesity Effects
The diverse evolution of plants represents a near inexhaustible source of biologically active compounds. The importance of these natural products for medicine and health has been immense (Courdavault et al., 2020). For many years, scientists have been researching and applying natural, plant-derived preparations as medicine in clinical treatment. Through these actions, active compounds such as strychnine and brucine (Langley, 1918), quinine (Dickson, 1823), colchicine (1890), caffeine (Bennett, 1873), and artemisinin (Ma et al., 2020) have been discovered. Thus, natural products provide important clues to the identification and development of synergistic drugs.
TCM refers to substances used for the prevention, diagnosis, and treatment of diseases, as well as for rehabilitation and health care under the guidance of TCM theories. It may be the best resource for the use of natural products, and it represents the accumulated experiences of thousands of years of medical practice. The written records of TCM date back at least 2,000 years to Shen Nong’s Classic of Materia Medica. In the long history of China, TCM has made an indelible contribution to the health of the Chinese people. Moreover, based on 2,000 years of experience in the use of TCMs and modern scientific research, the eleventh edition of the Pharmacopoeia of the People’s Republic of China (ChP) was promulgated and implemented in 2020. In this volume, the ChP stipulated the processing, usage, dosage, and compatibility of TCMs and included many classic prescriptions (Chinese Pharmacopoeia Commission).
Notably, in recent decades, there has been a growing interest in TCM. Since 1973, there have been 2,104 articles related to “traditional Chinese medicine” AND “lipid metabolism” in PubMed, while in 2020 alone, there were 389 articles (PubMed, https://pubmed.ncbi.nlm.nih.gov, last accessed on March 31, 2021). And 35 individual prescriptions from the ChP (2020 edition) were identified, each of which is clearly indicated as being anti-obesity and anti-hyperlipidemia (Table 1). Based on the formulation of these 35 prescriptions, we searched the Latin name of each single TCM for “anti-obesity” OR “anti-hyperlipidemia” in PubMed and CNKI in recent decades. Five TCMs including Crataegus pinnatifida Bunge, Salvia miltiorrhiza Bunge, Polygonum multiflorum Thunb., Alisma plantago-aquatica L., and Panax notoginseng (Burkill) F.H. Chen were identified. Each of these plants was implicated not only to function in anti-obesity and anti-hyperlipidemia but also to be mechanistically associated with these processes. Also, based on the widespread use of edible TCMs among people, some medicinal plants appear in diets. We also reviewed commonly used edible TCMs possessing the effects of anti-obesity and anti-hyperlipidemia including Scutellaria baicalensis Georgi, Curcuma longa L., pu-erh tea, green tea, Tripterygium wilfordii Hook. f., chilli peppers, and grape and the main bioactive compounds from them, such as baicalin, curcumin, epigallocatechin gallate, green tea polyphenol, triptolide and celastrol, capsaicin, and resveratrol (Sham et al., 2014; Wang S., et al., 2014; Martel et al., 2017).
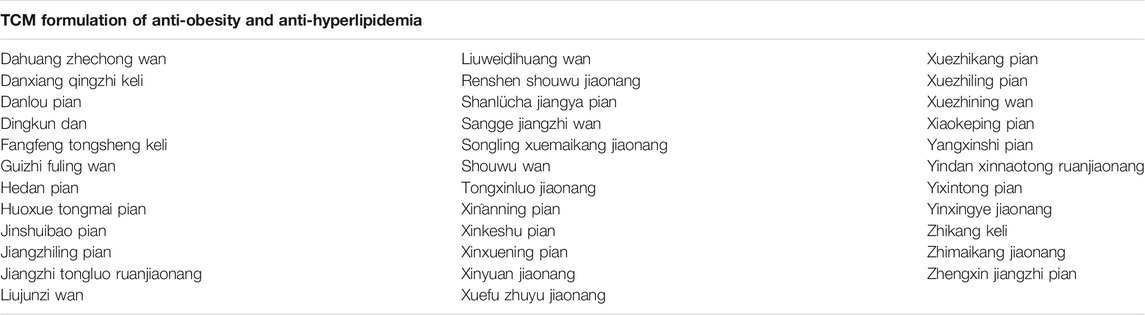
TABLE 1. TCM anti-obesity formulations from the Pharmacopoeia of the People’s Republic of China (ChP) (2020 edition).
Targeting Lipid Metabolism With Bioactive Compounds From TCMs With Anti-Obesity Effects
Over many years of observation and research, it has been found that TCMs can regulate all steps of lipid metabolism, targeting multiple pathways, including the modulation of lipid absorption, reduction of lipid synthesis, and increase of lipid decomposition and lipid transportation, as well as the physiological process of appetite regulation (Figure 1). The following is a systematic and comprehensive review of the anti-obesity effect of TCMs by targeting the above-mentioned lipid metabolism.
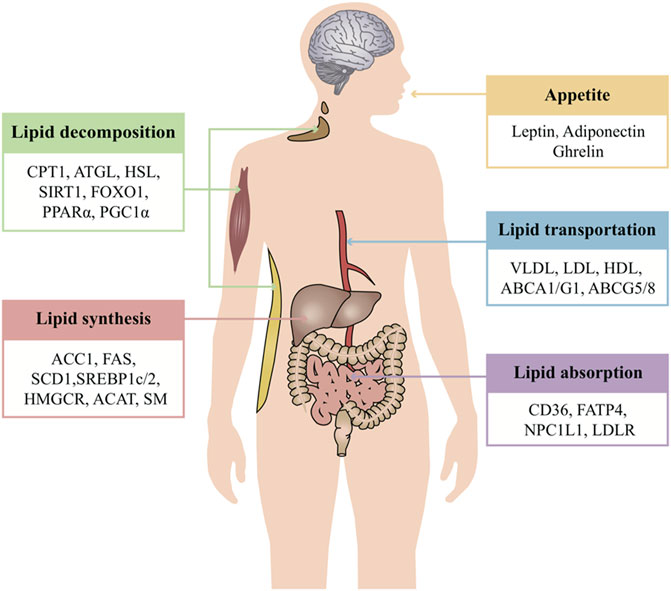
FIGURE 1. Overview of systematic regulated lipid metabolism of TCMs. TCM treatment of obesity mainly regulates lipid metabolism from five aspects: appetite, lipid absorption, lipid synthesis, lipid decomposition, and lipid transportation. Each link intersects and regulates each other to maintain the stability of the internal environment. One of the advantages of TCM is that it can act on multiple aspects and targets at the same time and systematically regulate life activities. Appetite is regulated by the level of leptin, adiponectin, ghrelin, and so on. These hormones serve as a critical signal to regulate food intake. The small intestine absorbs lipids derived exogenously from the diet. Dietary fat comprises a variety of lipids, while lipids synthesized in the liver are packaged in very low–density lipoproteins and delivered to adipose tissue for storage. CD36, FATP, NPC1L1, and LDLR are the classic regulators of lipid absorption. Apart from lipid absorption, excessive lipid synthesis is another cause of obesity. Some enzymes of lipid synthesis are the key to disease treatment, such as ACC, FAS, SCD, HMGCR, SM, and ACAT. These enzymes may also be regulated by the transcription factors SREBP and LXR. Cytoplasmic lipolysis and lysosomal-mediated autophagy (lipophagy) are two pathways that are known to break down TAGs and CEs in lipid droplets. In this process, ATGL, HSL, and MGL break down TAGs into free fatty acids which then undergo oxidative decomposition in the mitochondria via CPT1 to provide energy. The activity of these enzymes may also be controlled by SIRT1, FOXO1, and PGC1α. Lipoproteins are the major carriers of lipids in circulation. The major forms of lipoproteins are chylomicrons, VLDL, IDL, LDL, and HDL. The lipoproteins are responsible for transportation of FAs and cholesterol. Furthermore, the transportation of cholesterol to the extracellular environment is controlled by ABCA1, ABCG1, and ABCG5/8.
Regulation of Appetite
Feelings of hunger and satiety are regulated by complex neural and endocrine interactions among the gut, brain, adipose tissues, and other organs. As early as the 1960s, leptin was identified as a hormone linked to obesity. Leptin is secreted by adipose tissue and regulates appetite through inhibiting orexigenic neurons while stimulating anorexigenic pro-opiomelanocortin neurons (Friedman, 1997; Elias et al., 1999). Another hormone, ghrelin, which is released by the gastrointestinal tract when the stomach is empty, induces hunger by acting on hypothalamic brain cells in the central nervous system (CNS) (Ahima and Antwi, 2008). Moreover, the protein hormone adiponectin is secreted by adipocytes and circulates in the plasma. In contrast to leptin, adiponectin is reduced in obesity and increased in response to fasting. Adiponectin deficiency induces insulin resistance (IR) and hyperlipidemia and is associated with increased susceptibility toward vascular injury and atherosclerosis (Kadowaki et al., 2008).
Discovery of leptin brought hopes for treatment of obesity. Data from both humans and animals have established that the leptin level increases and is positively correlated with fat mass, thereby suppressing appetite. Conversely, weight loss leads to a decrease in the leptin level and produces a consequent increase in food intake (Campfield et al., 1995; Halaas et al., 1995; Pelleymounter et al., 1995; Montague et al., 1997; Licinio et al., 2004; Farooqi et al., 2007). Adiponectin levels decrease in obesity. Adiponectin enhances AMPK activity in the arcuate hypothalamus (ARH) via its receptor AdipoR1 to stimulate food intake; this stimulation of appetite by adiponectin is attenuated by the dominant-negative AMPK expression in the ARH (Kubota et al., 2007). Ghrelin levels increase during food deprivation in animals and prior to meals in humans and may serve as a critical signal to induce hunger during fasting (Ahima and Antwi, 2008).
As mentioned, TCMs can affect multiple steps in these hormone signaling pathways. Salvia miltiorrhiza Bunge can significantly inhibit the appetite and body weight by increasing the sensitivity to leptin and inhibiting ghrelin activity (Xin-Min et al., 2010; Tung et al., 2017). A high-fat diet (HFD) can increase the serum levels of leptin, insulin, and glucose. Polygonum multiflorum Thunb. could reverse these changes (Choi et al., 2018). Administration of Panax notoginseng (Burkill) F.H. Chen saponins (PNSs) for 30 days resulted in a significant decrease in serum insulin, leptin, body weight, food intake, and serum triglyceride (TG) levels compared with a diabetic control group (Yang et al., 2010). Curcuma longa L. may contribute to decreasing body weight and regulating leptin secretion in animals (Song and Choi, 2016) and humans (Navekar et al., 2017). Baicalin, a flavonoid of the herbal medicine Scutellaria baicalensis Georgi, also increased the plasma leptin level vs. the diabetic control (Waisundara et al., 2009). Finally, rats fed with fructose/green tea and fructose/pu-erh tea showed the greatest reduction in serum TG, cholesterol, insulin, and leptin levels (Huang and Lin, 2012). Consistent with obesity induction, adiponectin levels were reduced in HFD-fed mice and adiponectin levels were restored after green tea treatment in the wild type (WT) (Bolin et al., 2020). The green tea polyphenols also have the same curative effect (Tian et al., 2013).
Capsaicin is the molecule that is responsible for the pungency of hot peppers. It functions by stimulating the sympathoadrenal system that mediates the thermogenic and anorexigenic effects of capsaicinoids. Capsaicin treatment in mice fed on an HFD for 10 weeks lowered obesity, fasting glucose, insulin, leptin, and hepatic TGs while increasing adiponectin mRNA/protein in the adipose tissue. Furthermore, capsaicin increased GLP1 and decreased ghrelin secretion, indicating a possible interaction between transient receptor potential cation channel subfamily V member 1 (TrpV1) and GLP1 (Smeets and Westerterp-Plantenga, 2009; Kang et al., 2010). Also, leptin levels in the plasma were significantly lower in resveratrol-treated animals (Jimoh et al., 2018; Yu et al., 2019) and humans (Timmers et al., 2011). Celastrol, a compound of Tripterygium wilfordii Hook. f., is a leptin sensitizer (Xu et al., 2021). It can suppress appetite, block the reduction of energy expenditures, and lead up to a 45% weight loss in hyperleptinemic diet–induced obese mice by increasing leptin sensitivity (Liu et al., 2015). Celastrol, an NF-κB inhibitor, reduced IR and lipid abnormalities and led to higher plasma adiponectin levels in the db/db mice with celastrol treatment for 2 months (Kim et al., 2013).
Regulation of Lipid Uptake From the Intestine
The small intestine absorbs lipids derived exogenously from the diet including non-polar lipids, predominantly TAGs and CEs, and polar PLs. Dietary lipids such as TAGs, CEs, and PLs along with endogenous lipids from the bile are completely digested by pancreatic enzymes in the intestinal lumen, producing fatty acids (FAs), monoacylglycerols (MAGs), cholesterol, and lysophospholipids (Ko et al., 2020). The uptake of FAs and MAGs can be driven by the concentration gradient or facilitated by other proteins such as cluster of differentiation 36 (CD36) and fatty acid transport protein 4 (FATP4). Cholesterol uptake is mediated by Niemann–Pick C1-like 1 (NPC1L1). The TCMs can regulate lipid(s) uptake from the intestine (Figure 2).
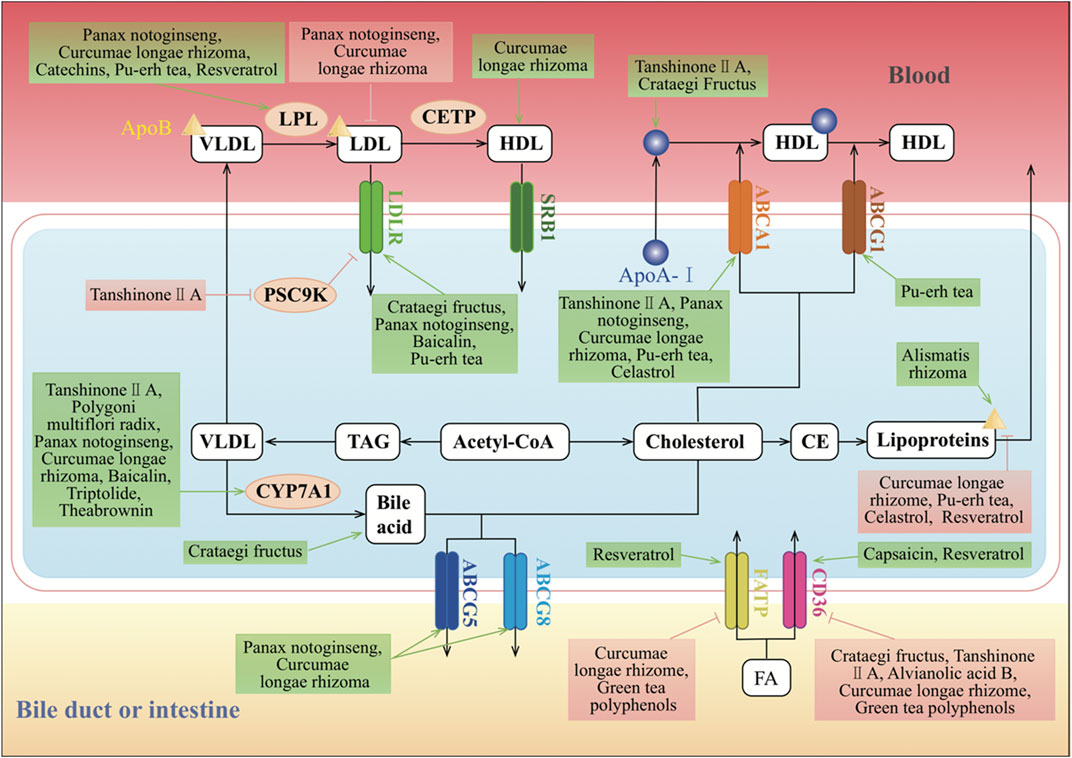
FIGURE 2. Molecular mechanisms of TCMs in lipid absorption and transportation. CD36, FATP, and LDLR are the classic regulators of lipid absorption. CD36 and FATP mediate the absorption of FA, and LDLR mediates the absorption of LDL. SR-BI can mediate the selective absorption of CE, which plays an important role in HDL metabolism and cholesterol “reversal.” Cholesterol is synthesized from acetyl-CoA. Excess cholesterol in hepatic cells is exported to the blood by ABCA1 or the homodimer of ABCG1, or to the intestinal lumen and bile ducts by the ABCG5 and ABCG8 heterodimers. Cholesterol can also be converted to CE by ACAT for storage in lipid droplets or for secretion as lipoproteins. The major forms of lipoproteins are chylomicrons, VLDL, IDL, LDL, and HDL, and they differ in their size, density, composition, and functions. CYP7A1 converts cholesterol into bile acids in the reverse cholesterol transport pathway. In the endogenous pathway, the liver produces VLDL, which interacts with LPL in the circulation to form IDL, with the release of TG and FAs. IDL is rapidly removed by the liver via the interaction of its apolipoprotein E component with LDLR. Furthermore, IDL forms LDL upon removal of TG by hepatic lipase. CETP induces LDL to generate HDL, which is an anti-atherogenic lipoprotein or “good cholesterol,” as it captures the cholesterol from peripheral tissues or other lipoproteins and transports it back to the liver by the third pathway, which is termed reverse cholesterol transport. ↑/⊥ depicts the positive or negative effect of TCMs in the cellular process, respectively.
Regulation of Uptake of FAs
FAs in the liver originate from the diet, de novo lipogenesis (DNL), and recycling of FAs released from adipose tissue during fasting (Mashek, 2013). FAs are taken in the intestinal lumen into enterocytes by two distinct mechanisms. In the first process, FAs diffuse passively through the apical membrane when luminal concentrations are higher than those inside the cell. The other mechanism of FA uptake is saturable and probably protein dependent as this transport occurs when the intracellular FA concentration is higher than that in the lumen.
Tanshinone ⅡA could decrease oxidized low-density lipoprotein (oxLDL)-induced expression of lectin-like oxidized LDL receptor-1 (LOX-1) and CD36 (Wen et al., 2020). And salvianolic acid B inhibited macrophage uptake of modified LDL in a scavenger receptor CD36–dependent manner (Bao et al., 2012). Curcuma longa L. suppressed the expression levels of CD36 and FATP, which were increased in HFD groups (Mun et al., 2019). Curcumin, a yellow-colored hydrophobic polyphenol, is the principal curcuminoid of the spice turmeric, the ground rhizome of Curcuma longa L.. Curcumin could also downregulate the mRNA level of Cd36 during adipocyte differentiation of 3T3-L1 cells (Zhao et al., 2011). CD36 mRNA and protein levels were decreased in high-fructose diet–induced rats when treated with green tea polyphenol (Qin et al., 2010). Epigallocatechin gallate (EGCG), a green tea bioactive polyphenol, also dose-dependently reversed HFD-induced effects on intestinal substrate transporters CD36, FATP4, and sodium-dependent glucose transporter 1 (Friedrich et al., 2012). In contrast, capsaicin or capsinoids could upregulate the expression of CD36 (Hong et al., 2015).
Regulation of Cholesterol Uptake
Classical cholesterol metabolism studies have confirmed that there are two main sources of cholesterol in the human body: exogenous cholesterol from the diet absorbed in the intestine and reabsorbed in the bile and endogenous cholesterol obtained through de novo synthesis from acetyl-CoA by the liver and peripheral tissue.
Exogenous cholesterol enters the enterocytes through NPC1L1 and the associated flotillins present on the apical surface of these cells. Curcumin could lower plasma cholesterol and prevent diet-induced hypercholesterolemia through modulating intestinal NPC1L1 expression via transcriptional regulation and the involvement of the sterol regulatory element–binding protein 2 (SREBP2) transcription factor (Kumar et al., 2011). In addition to NPC1L1-mediated cholesterol absorption from the intestinal lumen, another pathway is LDL receptor (LDLR)-mediated uptake of cholesterol containing LDL particles (LDL-c) from the blood. LDL in the blood is captured by LDLR on the cell surface and internalized, and as the endosomal pH decreases, LDLR dissociates from LDL and is recycled back to the surface for additional uptake. LDL is further delivered to lysosomes, and the carried cholesteryl esters (CEs) are hydrolyzed to cholesterol.
Tanshinone ⅡA and Crataegus pinnatifida Bunge can regulate the expressions of LDLR in the liver (Hu et al., 2016; Jia et al., 2016b). The Ldlr mRNA level was significantly higher in rats by treatment with an n-butanol extract (NE3) of Panax notoginseng (Burkill) F.H. Chen (Ji and Gong, 2007). The Scutellaria baicalensis Georgi extract also activated Ldlr genes in the liver. Co-administration of this extract with baicalin and metformin exerted a better effect on obesity-induced IR and lipid metabolism in a rat model system than treatment with metformin alone (Han et al., 2017). Also, proprotein convertase subtilisin/kexin type 9 (PCSK9), a negative regulator of LDLR, is also an SREBP2 target. Tanshinone ⅡA treatment inhibited the expression of PCSK9 and concomitantly increased LDLR activity (Chen et al., 2016).
Regulation of Gut Microbiota
The community of microorganisms living in the gastrointestinal tract in animals and humans has been shown to participate in various physiological and pathological processes in the gut and many other bodily processes. The link between the microbes in the human gut and the development of obesity and obesity-related diseases is becoming clearer. Studies have shown differences in the gut microbiota between obese individuals and lean individuals. Obesity and the associated metabolic syndromes are associated with microbiota alterations, including an increase in the ratio of Firmicutes to Bacteroidetes and in the relative abundance of Proteobacteria as well as alterations in specific bacteria such as Lactobacillus and Clostridium (Ley et al., 2005; Turnbaugh et al., 2006; Fei and Zhao, 2013; Cortés-Martín et al., 2020). There are also a reduced bacterial diversity and altered representations of bacterial genes and metabolic pathways (Turnbaugh et al., 2009; Wu et al., 2021).
Studies on both mice and humans show effects of gut microbiota on lipid metabolism by improving energy extraction from food, which is considered an environmental factor contributing to obesity and its comorbidities (Santacruz et al., 2009; Ridaura et al., 2013). Compared to lean mice, the gut microbiota of the obese mice have an increased capacity to harvest energy from the diet (Turnbaugh et al., 2006). Moreover, when compared with conventional mice, germ-free mice were able to resist obesity on a high-fat, high-carbohydrate Western diet, which could be explained by their intake of fewer calories, increased lipid excretion in the feces, and increased lipid oxidation in the intestine and peripheral tissues (Bäckhed et al., 2007; Rabot et al., 2010). In an observational study using fecal microbiota transplantation, the transplantation of feces from twins discordant for obesity into germ-free mice in a diet-dependent manner demonstrated transmissible, rapid, and modifiable effects of diet-by-microbiota interactions (Ridaura et al., 2013).
Many studies have emerged suggesting that the therapeutic potential of TCMs and their bioactive compounds may be due to the interaction with gut microbiota. Theabrownin, one of the most active and abundant pigments in pu-erh tea, altered the gut microbiota in both mice and humans and increased the levels of ileal conjugated bile acids by predominantly suppressing microbes associated with bile-salt hydrolase (BSH) activity. This in turn inhibited the intestinal farnesoid X receptor (FXR)–fibroblast growth factor 15 (FGF15) signaling pathway that increased hepatic production and fecal excretion of bile acids, thereby reducing hepatic cholesterol and decreasing lipogenesis (Huang et al., 2019). Green tea polyphenols decreased the relative abundance of Bacteroidetes and Fusobacteria and increased the relative abundance of Firmicutes as revealed by 16S rRNA gene sequencing analysis in canines with HFD-induced obesity (Li et al., 2020). The gut microbiota played an important role in the anti-obesity effects of celastrol, in which it promoted energy expenditure at a dose of 500 µg/kg body weight and improved the diversity of the gut microbiota with an increased ratio of Bacteroidetes to Firmicutes (Hu et al., 2020). Capsaicin has an anti-obesity effect through alterations in gut microbiota populations and short-chain FA concentrations, which were beneficial in prevention and treatment of obesity (Song et al., 2017; Rosca et al., 2020; Wang Y. et al., 2020). Resveratrol-induced gut microbiota modulated lipid metabolism, stimulated the development of beige adipocytes in white adipose tissue, reduced inflammation, and improved intestinal barrier function in HFD-fed mice. Therefore, the anti-obesity benefits of resveratrol might be through the “gut microbiota–adipose tissue” axis (Wang P, et al., 2020; Zhou et al., 2019).
Regulation of Lipid Transportation
Lipoproteins are the major carriers of lipids in circulation and participate in three major pathways that are responsible for the generation and transport of lipids within the body. The two major forms of circulating lipids in the body, TG and cholesterol, are packaged with apolipoproteins and PLs to form lipoproteins. The major forms of lipoproteins are chylomicrons, very low–density lipoprotein (VLDL), intermediate-density lipoprotein (IDL), low-density lipoprotein (LDL), and high-density lipoprotein (HDL), which differ in their size, density, composition, and functions.
In the exogenous pathway, dietary lipids, which mainly consist of TGs and some PLs, free FAs, and cholesterol, are packaged into chylomicrons by intestinal mucosal cells. These chylomicrons enter the lymphatic system and then the circulation, where TGs are released as free FAs by lipoprotein lipase (LPL) activity on the capillary endothelium. These free FAs are taken up by the muscle, adipose, and other peripheral tissues, whereas the remnants of chylomicrons are cleared by the liver. In the endogenous pathway, the liver produces VLDL, which interacts with LPL in the circulation to form IDL, with the release of TGs and free FAs. IDL is rapidly removed by the liver via the interaction of its apolipoprotein E component with LDLR. Furthermore, IDL forms LDL upon removal of TGs by hepatic lipase (HL). LDL, which is very high in cholesterol content, is in turn removed from the circulation by binding to LDLR in the liver and in extrahepatic tissues. HDL is an anti-atherogenic lipoprotein or “good cholesterol,” as it captures the cholesterol from peripheral tissues or other lipoproteins and transports it back to the liver by the third pathway, which is termed reverse cholesterol transport (Luo et al., 2020) (Figure 2).
Regulation of Lipoprotein Uptake
The liver has a role in the regulation of systemic lipid metabolism as it assembles and secretes TAG-rich VLDL particles into the systemic circulation for distribution of FAs to the peripheral tissues. Surface LDLR captures the circulating LDL via the extracellular ligand–binding domain. Many results suggest that green tea polyphenols inhibit the ubiquitin/proteasome-mediated upregulation of LDLR. This identified molecular mechanism might be related to the previously reported cholesterol-lowering and heart disease–preventative effects of green tea polyphenols (Kuhn et al., 2004). Trans-resveratrol exhibited the anti-atherogenic effect, at least, in part, by increased hepatic LDLR expression via proteolytic activation of SREBPs and subsequent LDL uptake (Yashiro et al., 2012).
By contrast, HDLs are generated by the intestine and the liver through the secretion of lipid-free apolipoprotein A-I (ApoA-I). ApoA-I then recruits cholesterol from these organs through the actions of ATP-binding cassette transporter A1 (ABCA1), forming nascent HDLs. In the peripheral tissues, nascent HDLs promote the efflux of cholesterol from tissues, including from macrophages, through the actions of ABCA1. Mature HDLs also promote this efflux, but through the actions of ATP-binding cassette transporter G (ABCA) 1. Tanshinone ⅡA reduced the lipid deposition in the liver. Moreover, it did not affect the serum lipid levels but reduced the levels of HDL middle subfractions and increased the levels of HDL large subfractions (Jia et al., 2016b). Treatment of THP-1 macrophages with baicalin significantly accelerated HDL-mediated but not ApoA-I–mediated cholesterol efflux. However, baicalin treatment increased the expression of scavenger receptor class B type I (SR-BI) in a dose- and time-dependent manner. Furthermore, baicalin increased the expression of peroxisome proliferator–activated receptor (PPAR) γ, a key regulator of reverse cholesterol transport, and liver X receptor (LXR) α (Yu et al., 2016). Administration of Curcuma longa L. significantly decreased the serum LDL and ApoB but increased the serum HDL and ApoA of healthy subjects (Ramirez-Bosca et al., 2000). Resveratrol treatment after 6 months decreased LDL-c, ApoB, oxLDL, and oxLDL/ApoB on statin-treated patients in primary cardiovascular disease prevention (Tome-Carneiro et al., 2012). The LPL was increased significantly in muscular tissues and decreased in adipose tissues by treatment with Crataegus pinnatifida Bunge flavonoids in mice (Fan et al., 2006). Finally, PNSs could also upregulate the mRNA expression of Lpl (Wang et al., 2016).
Regulation of Cholesterol Efflux
Cholesterol export from cells is mediated by ATP-binding cassette transporters. ABCA1 is expressed on the plasma membrane of most cells, including the basolateral surface of enterocytes. ABCG1 is most abundantly expressed on the surface of macrophages, whereas ABCG5 and ABCG8 are expressed at the apical surface of enterocytes and hepatocytes, forming a heterodimer. Excess cholesterol is esterified by acyl coenzyme A–cholesterol acyltransferases (ACATs). ABCA1 mediates cholesterol transport to ApoA-I in the blood, and this generates a nascent HDL that serves as an acceptor for ABCG1-mediated cholesterol efflux, leading to the production of HDL.
Tanshinone IIA treatment suppressed the expression of miR-33a, an ABCA1 negative regulator, whereas it upregulated the expression levels of ABCA1, SREBP2, PCSK9, cholesterol 7α-hydroxylase (CYP7A1), CD36, and LDLR in hyperlipidemic rats (Jia et al., 2016a; Jia et al., 2016b). The expression of PPARα and ApoA-Ⅰ was significantly downregulated in the hyperlipidemia group with tanshinone IIA treatment (Yi-Xin et al., 2017). A high dose of Crataegus pinnatifida Bunge increased the expressions of ApoA-I gene and HDL-c in HFD-fed mice (Shih et al., 2013). In addition, curcumin increased cholesterol efflux by activating and upregulating the expression of LXR and ABCA1 in subcutaneous adipocytes isolated from rabbits (Dong et al., 2011). The Curcuma longa L. oil treatment significantly increased the hepatic expression of PPARα, LXRα, CYP7A1, ABCA1, ABCG5, ABCG8, and LPL accompanied by a reduced SREBP2 and 3-hydroxy-3-methylglutaryl coenzyme A reductase (HMGCR) expression. Curcuma longa L. oil treatment also suppressed NPC1L1 expression in the jejunum compared with high-cholesterol diets (Singh et al., 2013). The expression of the reverse cholesterol transporters ABCA1 and ABCG1 was highly expressed in the livers of mice on pu-erh tea intervention (Huang et al., 2019). Furthermore, apolipoprotein B100 (ApoB100) is a constitutive protein of LDL-c, and it was significantly downregulated by pu-erh tea extract (PTE) treatment (Hu et al., 2017).
Cholesterol and sitosterol can be exported by the ABCG5–ABCG8 heterodimers to the intestinal lumen and bile, where cholesterol is extracted by bile salts. Re-synthesis of cholesterol induces pathways for cholesterol export and storage and acts to suppress further cholesterol biosynthesis. When treated with NE3, the concentrations of serum TC, TG, and LDL-c in rats showed a significant dose-dependent decrease. Expression level analysis indicated that both LXR targeting genes including ABCA1, ABCG5, and ABCG8 and FXR targeting genes including ApoC2 and a short heterodimer partner were significantly induced by NE3 (Ji and Gong, 2007). In addition, CE combines with ApoB to form lipoproteins, which are transported outside the cell by exocytosis. Curcuma longa L., resveratrol, and PTE could significantly decrease the level of ApoB (Ramirez-Bosca et al., 2000; Tome-Carneiro et al., 2012; Hu et al., 2017). Celastrol was able to effectively suppress weight and attenuate high-fat–mediated oxidative injury by improving ABCA1 expression, reducing the levels of TC, TG, LDL-c, and ApoB in the plasma, and increasing antioxidant enzyme activities and inhibiting nicotinamide adenine dinucleotide phosphate (NADPH) oxidase activity (Wang C. et al., 2014).
Furthermore, CYP7A1 regulates the balance between cholesterol supply and metabolism by catalyzing the rate-limiting step of bile acid biosynthesis. Scutellaria baicalensis Georgi activated the Cyp7a1 gene in the liver (Han et al., 2017). Crataegus pinnatifida Bunge could counteract the downregulation of CYP7A1 and LDLR with the stimulation effect of HFD (Hu et al., 2016). And NE3 significantly decreased the expression level of the Cyp7a1 gene (Ji and Gong, 2007). In contrast, the expression of Cyp7a1 was markedly increased in the triptolide-treated group (Yang et al., 2017).
Regulation of Lipid Synthesis
Excessive lipid synthesis is another cause of obesity and hyperlipidemia. Lipids synthesized in the liver are packaged in very low–density lipoproteins (VLDLs) and delivered to adipose tissue for storage. Clearly, TCMs play important roles in lipid synthesis, including FA and TAG synthesis as well as cholesterol biosynthesis (Figure 3).
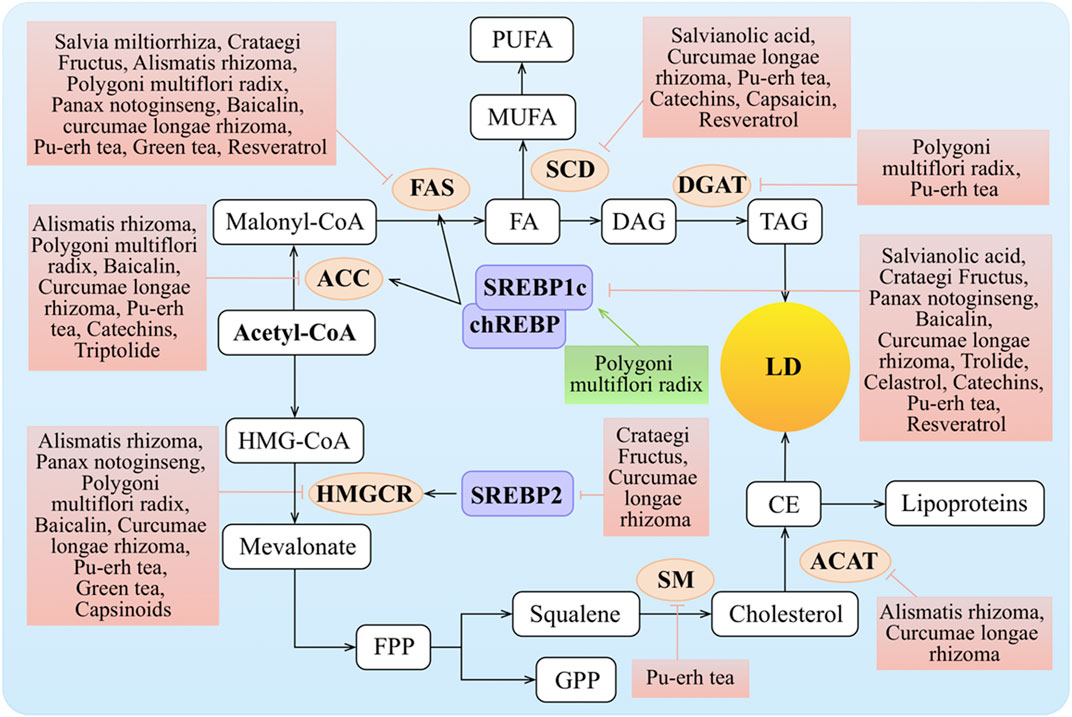
FIGURE 3. Molecular mechanisms of TCMs in lipid synthesis. FA entering the hepatocyte is rapidly “activated” by acyl-CoA, and this is also termed the de novo lipogenesis (DNL) of FA. DNL is mainly regulated via the rate-limiting enzymes such as ACC and FAS, while the expression and activation of ACC and FAS are regulated by SREBP1c and chREBP. Transcription of the genes encoding SREBP1c and chREBP is stimulated by insulin via LXR and inhibited by FAs. The synthesis of MUFA regulates by SCD. FAs are typically esterified to TG and subsequently packaged into VLDL for export or stored as intracellular LDs. HMGCR and SM as the rate-limiting enzymes are key regulators of the synthesis process of cholesterol. Cholesterol can be converted to CE by ACAT for storage in LDs or for secretion as lipoproteins. Meanwhile, SREBP2 can increase the level of HMGCR. ↑/⊥ depicts the positive or negative effect of TCMs in the cellular process, respectively.
Regulation of Fatty Acid Synthesis
The DNL of FAs from acetyl-CoA to fatty acyl-CoA is mainly regulated by acetyl-CoA carboxylase (ACC) and fatty acid synthase (FAS) as the rate-limiting enzymes. Stearoyl-CoA desaturase (SCD) is a central enzymatic node in the conversion of saturated fatty acids (SFAs) into mono-unsaturated fatty acids (MUFAs) (AM et al., 2017). MUFAs represent the precursors of several lipids essential for plasma membranes, such as TGs, CEs, diacylglycerols, and wax esters. Transcriptional regulation of Acc and Fas is primarily through SREBP1c and carbohydrate-responsive element–binding protein (chREBP). SCD expression is regulated by diverse hormonal and nutritional factors and is positively regulated by Srebp1c, chrebp, and Lxr (Wang Y., et al., 2015). Both transcription factors are activated by Lxr.
Salvianolic acids, the major water-soluble ingredients of Salvia miltiorrhiza Bunge, reduced ovariectomy-induced body weight gain, attenuated the expressions of hepatic lipogenic genes, such as Srebp1, Fas, and Scd, and decreased the TG and TC via blocking signal transducer and activator of transcription (STAT)-3/SREBP1 signaling (Chen et al., 2018). The hepatic Fas and Srebp1c mRNA levels were reduced in mice fed on the Crataegus pinnatifida Bunge diet compared to the standard diet (Zhang et al., 2014). The levels of FAS and ACC in the plasma were generally reduced after administration of Polygonum multiflorum Thunb. (Xian et al., 2017). Alisma plantago-aquatica L. inhibited adipocyte differentiation by downregulating the expression of PPARγ, CCAAT/enhancer-binding protein β (C/EBPβ), and FAS (Park et al., 2014) and blocked hepatic lipid production by regulating hepatic lipogenic genes including Fas, Acc, and glycerol-3-phosphate acyltransferase (GPAT) (Choi et al., 2019). Alisol B 23-acetate, a natural triterpenoid isolated from Alisma plantago-aquatica L., decreased hepatic lipogenesis through decreasing hepatic levels of SREBP1c, FAS, ACC, and SCD (Meng et al., 2017). Panax notoginseng (Burkill) F.H. Chen could change the fat and inflammation of liver tissue through decreasing expression levels of SREBP1c, ACC, and FAS (Ji and Gong, 2007; Yan-Xia et al., 2011; Zhang et al., 2020). The expression levels of SREBP1c, ACC, and FAS were downregulated in the Curcuma longa L. groups compared to the HFD groups (Ejaz et al., 2009; Ahn et al., 2010; Zhao et al., 2011; Mun et al., 2019). Baicalin treatment significantly attenuated methionine and choline–deficient diet (MCD)–induced hepatic lipid accumulation partly through regulating the expression of SREBP1c, FAS, and ACC (Xi et al., 2015; Zhang J. et al., 2018). Capsaicin inhibited the early adipogenic differentiation, lipogenesis, and maturation of adipocytes with concomitant repression of PPARγ and SCD (Ibrahim et al., 2015). The PTE intake tended to decrease Srebp1c, Acc, and Fas mRNA expressions in the liver of the mice (Shimamura et al., 2013), and PTE also downregulated Scd and Srebp in Caenorhabditis elegans to suppress fat accumulation (Ding et al., 2015; Hu et al., 2017). On treatment with green tea, the expression of lipogenesis-related genes Acc, Fas, and Scd was downregulated in the liver (Kim et al., 2009; Friedrich et al., 2012). The transcriptional activities of Srebp1c and forkhead box protein O1 (FOXO1) were significantly decreased by EGCG (Kim et al., 2010). Resveratrol exerted anti-obesity effects via mechanisms involving downregulation of ACC, FAS (Alberdi et al., 2011), and SCD (Zhang et al., 2012) and upregulation of the key adipogenic gene Srebp1c (Kim et al., 2011; Khaleel et al., 2018) in an HFD model. Celastrol decreased hepatic SREBP1 expression (Zhang et al., 2017). However, triptolide treatment increased the expression of LXR and its target gene, Srebp1, in both male and female rats and increased the expression of ACC only in the female rats (Jiang et al., 2016).
Regulation of TAG Synthesis
TAG production can come from exogenous FAs in the circulation or intracellular FAs generated by glycolysis and lipogenesis from glucose supplied by excess dietary intake. TAG synthesis is catalyzed by diacylglycerol acyltransferase (DGAT) in the last biosynthesis step. Polygonum multiflorum Thunb. supplementation significantly downregulated the expression of Pparγ and Dgat2 genes in obese mice (Choi et al., 2018).
Regulation of Cholesterol Biosynthesis
An increased level of LDL-c and/or TC is a pronounced phenotype of dyslipidemia. Ultimately, it is due to elevated cholesterol. Cholesterol plays an important role in human physiological functions. Almost all cells can synthesize cholesterol, and in this process, three crucial players of the cholesterol biosynthetic pathway are required, SREBP2, which functions as a master transcriptional regulator of cholesterol biosynthesis, and two rate-limiting enzymes of the biosynthetic pathway: HMGCR and squalene monooxygenase (SM).
Regulation of HMGCR and ACAT
As the rate-limiting enzyme for cholesterol biosynthesis, HMGCR is highly regulated at the transcriptional, translational, and post-translational levels (Goldstein and Brown, 1990). The formation of CE is another important means of preventing the accumulation of free cholesterol in cells, as this ACAT-mediated pathway directs the storage or secretion of cholesterol.
Crataegus pinnatifida Bunge could suppress the stimulation effect of HFD on the transcription of Hmgcr, and the transcriptional activity of the Hmgcr promoter was inhibited by Crataegus pinnatifida Bunge in a dose-dependent manner (Hu et al., 2016). HMGCR was generally reduced after administration of Polygonum multiflorum Thunb. (Xian et al., 2017). Alisma plantago-aquatica L. showed comparatively high inhibition against ACAT and HMGCR activities in rat livers (Choi et al., 2019). Panax notoginseng (Burkill) F.H. Chen also reduced the levels of hepatic HMGCR in HFD rats (Xia et al., 2011).
In addition, relative to the HFD control group, hamsters fed a curcumin-supplemented HFD had lower hepatic cholesterol and TG levels and HMGCR and ACAT activities, along with an increased FA β-oxidation activity (Jang et al., 2008). Scutellaria baicalensis Georgi, pu-erh tea, or green tea intervention repressed the expression of HMGCR in the liver (Yamashita et al., 2016; Han et al., 2017). However, capsinoids significantly increased HMGCR in the liver (Hong et al., 2015). Treatment with theabrownin, one of the most active and abundant pigments in pu-erh tea, increased two bile acid synthetic genes, Cyp7a1 and Cyp7b1, in HFD-treated mice (Zeng et al., 2015; Huang et al., 2019).
Regulation of Lipid Decomposition
Cytoplasmic lipid droplets (LDs) are multiprotein-coated structures that serve as dynamic TAG storage pools and are involved in several aspects of lipid metabolism. The LDs are mainly rich in TAGs and CEs. There are two pathways that are known to break down TAGs in LDs: cytoplasmic lipolysis and lysosomal-mediated autophagy (lipophagy). Adipose triglyceride hydrolase (ATGL) plays a key role in the lipolysis pathway, breaking down TAGs into diglycerides (DAGs) and FAs. FAs further undergo oxidative decomposition in the mitochondria via carnitine palmitoyl transferase 1 (CPT1) and provide energy (Figure 4).
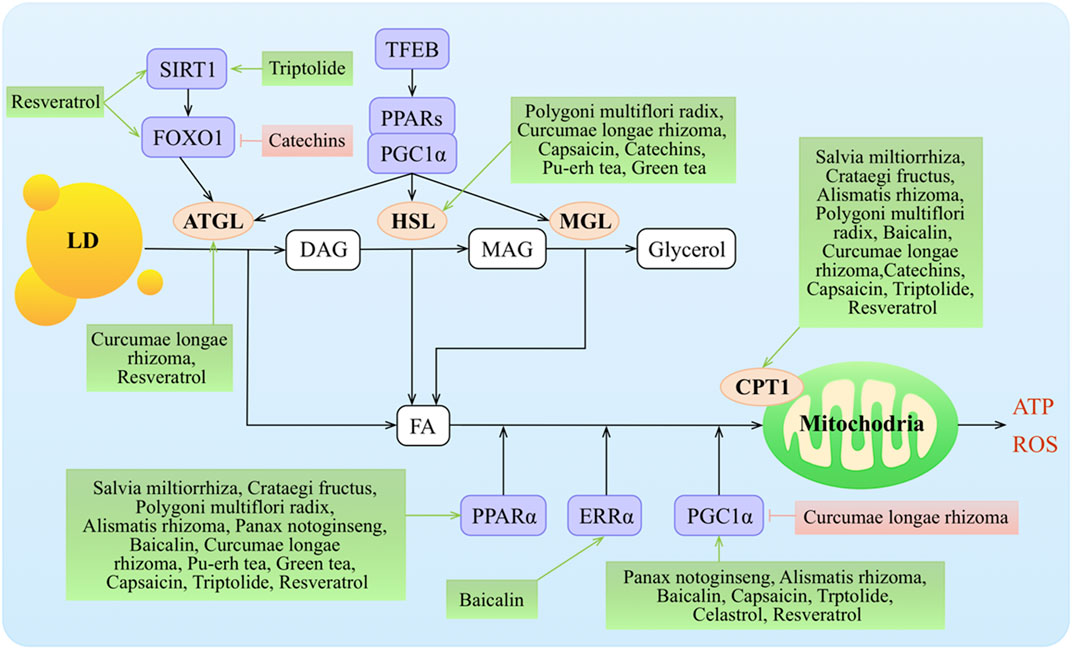
FIGURE 4. Molecular mechanisms of TCMs in lipid decomposition. TCMs stimulate lipolysis from fat stores in the liver, white adipose tissue, and dietary fat sources (high-fat diets) to generate FAs that enter the hepatic cells via protein transporters. TG stored as lipid droplets can be hydrolyzed back to FAs via classic lipases (ATGL, HSL, and MGL) and lipophagy (by regulating TFEB, SIRT1, and FOXO1), undergo mitochondrial β-oxidation by the activity of various co-activators or nuclear receptors (such as PPARα, ERRα, and PGC1α), and target the transcription of gene Cpt1. Malonyl-CoA, an intermediate in DNL, inhibits CPT1 action and downregulates FA oxidation. ↑/⊥ depicts the positive or negative effect of TCMs in the cellular process, respectively.
Regulation of LD Decomposition
ATGL initiates TAG hydrolysis to form diacylglycerol and FAs. Hormone-sensitive lipase (HSL) (Lafontan and Langin, 2009; Rodriguez et al., 2010) and monoacylglycerol lipase (MGL) complete the process by consecutively hydrolyzing diacylglycerols into MAGs and FAs and then hydrolyzing MAGs into glycerol and FAs (Zechner et al., 2017). Transcription of Atgl is controlled by sirtuin 1 (SIRT1)–mediated deacetylation of FOXO1 and by PPARγ co-activator 1α (PGC1α) (Chakrabarti et al., 2011; Chen et al., 2021). The PPAR–PGC1α axis also regulates the transcriptional expression of Hsl (Albert et al., 2014; Farhan et al., 2014) and Mgl (Rakhshandehroo et al., 2007).
Curcumin treatment upregulated the expression of ATGL and resulted in acceleration of lipolysis (Valentine et al., 2019). Pu-erh tea administration significantly lowered plasma TC and TG concentrations and the LDL-c level but did not affect HDL-c levels. Moreover, pu-erh tea significantly increased LPL, HL, and HSL activities in epididymal fat tissue in rats with HFD-induced obesity (Cao et al., 2011). Resveratrol acted mainly on ATGL to regulate lipolytic activity in humans and murine adipocytes (Lasa et al., 2012) and increased Sirt1, Foxo1, and adiponectin mRNA expressions (Costa Cdos et al., 2011; Timmers et al., 2011; Lasa et al., 2012).
Regulation of β-Oxidation of FAs
β-Oxidation in the mitochondria is the predominant oxidative pathway for energy production in the liver. β-Oxidation consists of a cycling process involving dehydrogenation, hydration, dehydrogenation, and acylation that produces acetyl-CoA. In this process, the most important enzyme is CPT1 (Houten et al., 2016). The primary regulators of β-oxidation are the transcription factors Pparα and Pgc1α, whose action is upregulated by FAs and glucagon and suppressed via insulin (Pawlak et al., 2015).
The expression of PPARα was significantly downregulated in a hyperlipidemia group with tanshinone IIA treatment in rats (Yi-Xin et al., 2017). CPT1, PPARα, and its downstream targets were activated with hawthorn leaf flavonoids in an HFD model (Kuo et al., 2009; Li et al., 2015; Dong et al., 2017). Alisol B 23-acetate administration increased lipid metabolism via inducing PPARα, CPT1α, and LPL (Meng et al., 2017). Alisma plantago-aquatica L. suppressed the mRNA levels of hepatic Pgc1α, estrogen-related receptor (ERR)γ, and PGC1α-dependent enzyme (G6Pase) that are involved in gluconeogenesis in liver tissue (Jeong Hyang Sook %J Korea Journal of Herbology, 2013). Polygonum multiflorum Thunb. supplementation significantly upregulated the Pparα, Cpt1, Cpt2, Ucp1, and Hsl mRNA levels compared with the HFD group (Choi et al., 2018). PNSs regulated lipid metabolism by upregulating the expression of transcriptional factors, such as Pparα, Pparγ, and Pgc1α (Wang et al., 2016). PPARα and CPT1 expressions were upregulated in the Curcuma longa L.–treated groups (Mun et al., 2019). Finally, Curcuma longa L. treatment in high-fructose diet (HFrD)-fed rats repressed hepatic expression of PGC as compared to the rats fed an HFrD alone, suggesting a protective effect of Curcuma longa L. by modulating the expression of lipogenic genes in the liver (Singh et al., 2015). Treatment with baicalin ameliorated diet-induced obesity through directly activating hepatic CPT1 as well as increasing the expression of PPARα (Zhang J. et al., 2018), ERRα, and PGC1α (Takizawa et al., 2015; Dai et al., 2018).
Treatment with capsinoids significantly increased the expression of CPT1, adiponectin, and Pparα and Pgc1α mRNA in the liver (Kang et al., 2010; Lee et al., 2013; Hong et al., 2015; Panchal et al., 2018). The expression of PPARα was also increased in the HFD/pu-erh tea groups (Sun et al., 2019). Green tea increased the expression of CPT1 and PPARα and decreased the expression of LXR (Chen et al., 2009; Kim et al., 2009; Axling et al., 2012) while increasing HSL in mesenteric adipose tissue concomitantly with the HFD (Cunha et al., 2013). Resveratrol increased CPT1 activities (Gomez-Zorita et al., 2012), had a higher agonistic activity of PPARα (Takizawa et al., 2015), and significantly increased SIRT1 and PGC1α levels and citrate synthase activity and improved muscle mitochondrial respiration (Timmers et al., 2011). Finally, celastrol augmented PGC1α expression in adipocytes and skeletal muscles (Fang et al., 2019).
Conclusions and Perspectives
Human obesity is quickly becoming widespread, and treatment of it and its comorbidities is an important clinical challenge. Targeting lipid metabolism as a potential treatment has attracted a great deal of attention as a primary therapeutic target. TCMs have been widely used as anti-obesity treatments for a very long time. In this review, how TCMs modulate major features of lipid metabolism was systematically summarized. Collation and integration of these data has produced a comprehensive register for the mechanisms of TCMs’ action in anti-obesity (Table 2).
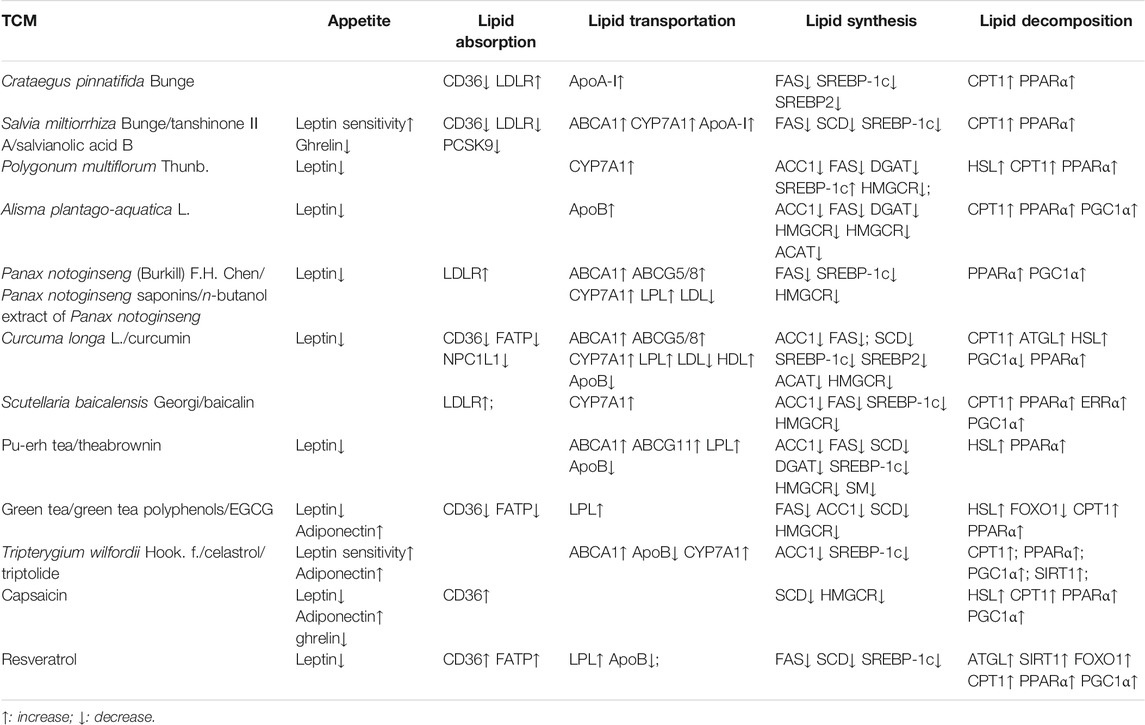
TABLE 2. A summary of studies demonstrating the effects of TCM on lipid metabolism in animal models and humans.
While TCMs clearly play roles in anti-obesity, the side effects of TCMs should not be overlooked. Hepatotoxicity is the main side effect of TCMs (Tarantino et al., 2009; Frenzel and Teschke, 2016). In many cases, the side effects are caused by incorrect processing, usage, dosage, or compatibility. For example, the use of the processed Polygonum multiflorum Thunb. caused less damage to the liver than the unprocessed Polygonum multiflorum Thunb. (Tu et al., 2015), while EGCG caused dose-dependent hepatotoxicity in mice under dietary restriction, but not in mice fed a normal diet (Shi et al., 2020). In addition, Tripterygium wilfordii Hook. f. is normally hepatotoxic, but the classical compatibility of Tripterygium wilfordii Hook. f. and Lysimachia christinae Hance can detoxify the poison of Tripterygium wilfordii Hook. f. (Wang et al., 2018; Wang J., et al., 2015). Triptolide and celastrol are two major components of Tripterygium wilfordii Hook. f.. Interestingly, triptolide is hepatotoxic, while celastrol showed protection from liver injury (Hasnat et al., 2019; Xu et al., 2021). These cases suggest that correct processing, usage, dosage, and compatibility under the application guidance based on long experience can greatly reduce side effects. Therefore, for the use of TCMs in anti-obesity, following the doctor’s advice and guidelines of the TCMs is essential to ensure the efficacy of the TCMs and also avoid side effects as much as possible.
Moreover, the capacity of TCMs to inhibit obesity is attracting increasing attention. TCMs are being advocated as another major breakthrough for therapeutic intervention for obesity-related diseases. However, only a fraction of the medically active substances available in TCMs have been identified, and the unidentified natural products have great potential. Modern technologies enable the detailed analysis of TCM extracts to identify active substances. These phytochemicals, in the form of the TCMs themselves, extracts, or purified components, can be combined with existing treatments to reduce the prevalence of obesity and its complications. Taking advantage of TCM effects on therapeutic interventions for the treatment of obesity-related diseases may be another breakthrough for integrated medicine.
Author Contributions
QF, FX, and XZ designed the study. QF and XZ wrote the manuscript. BL supervised the work and revised the manuscript. All authors have read and approved the manuscript.
Funding
This work was supported by the Ministry of Science and Technology of the People’s Republic of China grant (2018YFA0800700), the National Natural Science Foundation of China grants (81700520, U1702288, U1702287, 31671230, 91857113, 31860323, and 32071281), the Yunnan Applied Basic Research Projects 2018FB117 and 2019FY003021, and the Key Project for Yunnan Provincial Traditional Chinese Medicine Joint 2018FF001(-004).
Conflict of Interest
The authors declare that the research was conducted in the absence of any commercial or financial relationships that could be construed as a potential conflict of interest.
References
Aboonabi, A., Meyer, R. R., and Singh, I. (2019). The Association between Metabolic Syndrome Components and the Development of Atherosclerosis. J. Hum. Hypertens. 33 (12), 844–855. doi:10.1038/s41371-019-0273-0
Ahima, R. S., and Antwi, D. A. (2008). Brain Regulation of Appetite and Satiety. Endocrinol. Metab. Clin. North America 37 (4), 811–823. doi:10.1016/j.ecl.2008.08.005
Ahn, J., Lee, H., Kim, S., and Ha, T. (2010). Curcumin-induced Suppression of Adipogenic Differentiation Is Accompanied by Activation of Wnt/β-Catenin Signaling. Am. J. Physiology-Cell Physiol. 298 (6), C1510–C1516. doi:10.1152/ajpcell.00369.2009
Alberdi, G., Rodríguez, V. M., Miranda, J., Macarulla, M. T., Arias, N., Andrés-Lacueva, C., et al. (2011). Changes in white Adipose Tissue Metabolism Induced by Resveratrol in Rats. Nutr. Metab. 8 (1), 29. doi:10.1186/1743-7075-8-29
Albert, J. S., Yerges-Armstrong, L. M., Horenstein, R. B., Pollin, T. I., Sreenivasan, U. T., Chai, S., et al. (2014). Null Mutation in Hormone-Sensitive Lipase Gene and Risk of Type 2 Diabetes. N. Engl. J. Med. 370 (24), 2307–2315. doi:10.1056/NEJMoa1315496
Am, A., LJohani, D. N., Syed, D. N., and Ntambi, J. M. (2017). Insights into Stearoyl-CoA Desaturase-1 Regulation of Systemic Metabolism. Trends Endocrinol. Metab. 28 (12), 831–842. doi:10.1016/j.tem.2017.10.003
Arem, H., and Irwin, M. L. (2013). Obesity and Endometrial Cancer Survival: a Systematic Review. Int. J. Obes. 37 (5), 634–639. doi:10.1038/ijo.2012.94
Aronne, L. J., Wadden, T. A., Peterson, C., Winslow, D., Odeh, S., and Gadde, K. M. (2013). Evaluation of Phentermine and Topiramate versus Phentermine/topiramate Extended-Release in Obese Adults. Obesity 21 (11), 2163–2171. doi:10.1002/oby.20584
Axling, U., Olsson, C., Xu, J., Fernandez, C., Larsson, S., Ström, K., et al. (2012). Green tea Powder and Lactobacillus Plantarum Affect Gut Microbiota, Lipid Metabolism and Inflammation in High-Fat Fed C57BL/6J Mice. Nutr. Metab. 9 (1), 105. doi:10.1186/1743-7075-9-105
Bäckhed, F., Manchester, J. K., Semenkovich, C. F., and Gordon, J. I. (2007). Mechanisms Underlying the Resistance to Diet-Induced Obesity in Germ-free Mice. Pnas 104 (3), 979–984. doi:10.1073/pnas.0605374104
Bao, Y., Wang, L., Xu, Y., Yang, Y., Wang, L., Si, S., et al. (2012). Salvianolic Acid B Inhibits Macrophage Uptake of Modified Low Density Lipoprotein (mLDL) in a Scavenger Receptor CD36-dependent Manner. Atherosclerosis 223 (1), 152–159. doi:10.1016/j.atherosclerosis.2012.05.006
Bennett, A. (1873). An Experimental Inquiry into the Physiological Actions of Theine, Caffeine, Guaranine, Cocaine, and Theobromine. Edinb. Med. J. 19 (4), 323–341.
Bolin, A. P., Sousa-Filho, C. P. B., Marinovic, M. P., Rodrigues, A. C., and Otton, R. (2020). Polyphenol-rich green tea Extract Induces Thermogenesis in Mice by a Mechanism Dependent on Adiponectin Signaling. J. Nutr. Biochem. 78, 108322. doi:10.1016/j.jnutbio.2019.108322
Campfield, L., Smith, F., Guisez, Y., Devos, R., and Burn, P. (1995). Recombinant Mouse OB Protein: Evidence for a Peripheral Signal Linking Adiposity and central Neural Networks. Science 269 (5223), 546–549. doi:10.1126/science.7624778
Cao, Z.-H., Gu, D.-H., Lin, Q.-Y., Xu, Z.-Q., Huang, Q.-C., Rao, H., et al. (2011). Effect of Pu-Erh tea on Body Fat and Lipid Profiles in Rats with Diet-Induced Obesity. Phytother. Res. 25 (2), 234–238. doi:10.1002/ptr.3247
Chakrabarti, P., English, T., Karki, S., Qiang, L., Tao, R., Kim, J., et al. (2011). SIRT1 Controls Lipolysis in Adipocytes via FOXO1-Mediated Expression of ATGL. J. Lipid Res. 52 (9), 1693–1701. doi:10.1194/jlr.M014647
Chen, C.-C., Kuo, C.-H., Leu, Y.-L., and Wang, S.-H. (2021). Corylin Reduces Obesity and Insulin Resistance and Promotes Adipose Tissue browning through SIRT-1 and β3-AR Activation. Pharmacol. Res. 164, 105291. doi:10.1016/j.phrs.2020.105291
Chen, H.-C., Chen, P.-Y., Wu, M.-J., Tai, M.-H., and Yen, J.-H. (2016). Tanshinone IIA Modulates Low Density Lipoprotein Uptake via Down-Regulation of PCSK9 Gene Expression in HepG2 Cells. PLoS One 11 (9), e0162414. doi:10.1371/journal.pone.0162414
Chen, J., Yue, J., Liu, J., Liu, Y., Jiao, K.-L., Teng, M.-Y., et al. (2018). Salvianolic Acids Improve Liver Lipid Metabolism in Ovariectomized Rats via Blocking STAT-3/SREBP1 Signaling. Chin. J. Nat. Medicines 16 (11), 838–845. doi:10.1016/s1875-5364(18)30125-0
Chen, N., Bezzina, R., Hinch, E., Lewandowski, P. A., Cameron-Smith, D., Mathai, M. L., et al. (2009). Green tea, Black tea, and Epigallocatechin Modify Body Composition, Improve Glucose Tolerance, and Differentially Alter Metabolic Gene Expression in Rats Fed a High-Fat Diet. Nutr. Res. 29 (11), 784–793. doi:10.1016/j.nutres.2009.10.003
Choi, E., Jang, E., and Lee, J.-H. (2019). Pharmacological Activities of Alisma Orientale against Nonalcoholic Fatty Liver Disease and Metabolic Syndrome: Literature Review. Evidence-Based Complement. Altern. Med. 2019, 1–15. doi:10.1155/2019/2943162
Choi, R.-Y., Lee, H.-I., Ham, J. R., Yee, S.-T., Kang, K.-Y., and Lee, M.-K. (2018). Heshouwu (Polygonum Multiflorum Thunb.) Ethanol Extract Suppresses Pre-adipocytes Differentiation in 3T3-L1 Cells and Adiposity in Obese Mice. Biomed. Pharmacother. 106, 355–362. doi:10.1016/j.biopha.2018.06.140
Cortés-Martín, A., Iglesias-Aguirre, C. E., Meoro, A., Selma, M. V., and Espín, J. C. (2020). There Is No Distinctive Gut Microbiota Signature in the Metabolic Syndrome: Contribution of Cardiovascular Disease Risk Factors and Associated Medication. Microorganisms 8 (3), 416. doi:10.3390/microorganisms8030416
Costa, C. d. S., Rohden, F., Hammes, T. O., Margis, R., Bortolotto, J. W., Padoin, A. V., et al. (2011). Resveratrol Upregulated SIRT1, FOXO1, and Adiponectin and Downregulated PPARγ1-3 mRNA Expression in Human Visceral Adipocytes. Obes. Surg. 21 (3), 356–361. doi:10.1007/s11695-010-0251-7
Courdavault, V., O’Connor, S. E., Oudin, A., Besseau, S., and Papon, N. (2020). Towards the Microbial Production of Plant-Derived Anticancer Drugs. Trends Cancer 6 (6), 444–448. doi:10.1016/j.trecan.2020.02.004
Cunha, C. A., Lira, F. S., Rosa Neto, J. C., Pimentel, G. D., Souza, G. I. H., da Silva, C. M. G., et al. (2013). Green tea Extract Supplementation Induces the Lipolytic Pathway, Attenuates Obesity, and Reduces Low-Grade Inflammation in Mice Fed a High-Fat Diet. Mediators Inflamm. 2013, 1–8. doi:10.1155/2013/635470
Dai, J., Liang, K., Zhao, S., Jia, W., Liu, Y., Wu, H., et al. (2018). Chemoproteomics Reveals Baicalin Activates Hepatic CPT1 to Ameliorate Diet-Induced Obesity and Hepatic Steatosis. Proc. Natl. Acad. Sci. USA 115 (26), E5896–E5905. doi:10.1073/pnas.1801745115
de Beer, M., Hofsteenge, G. H., Koot, H., Hirasing, R., Delemarre-van de Waal, H., and Gemke, R. (2007). Health-related-quality-of-life in Obese Adolescents Is Decreased and Inversely Related to BMI. Acta Paediatr. 96 (5), 710–714. doi:10.1111/j.1651-2227.2007.00243.x
Dickson, D. J. H. (1823). On the Febrifuge Power of the Sulphate of Quinine. Edinb. Med. Surg. J. 19 (77), 571–573.
Ding, Y., Zou, X., Jiang, X., Wu, J., Zhang, Y., Chen, D., et al. (2015). Pu-erh tea Down-Regulates Sterol Regulatory Element-Binding Protein and Stearyol-CoA Desaturase to Reduce Fat Storage in Caenorhaditis Elegans. PLoS One 10 (2), e0113815. doi:10.1371/journal.pone.0113815
Dobbs, R., James, M., Woetzel, J., Sawers, C., Thompson, F., Peter, C., et al. 2014. How the World Could Better Fight Obesity.
Dong, P., Pan, L., Zhang, X., Zhang, W., Wang, X., Jiang, M., et al. (2017). Hawthorn ( Crataegus Pinnatifida Bunge) Leave Flavonoids Attenuate Atherosclerosis Development in apoE Knock-Out Mice. J. Ethnopharmacology 198, 479–488. doi:10.1016/j.jep.2017.01.040
Dong, S.-z., Zhao, S.-p., Wu, Z.-h., Yang, J., Xie, X.-z., Yu, B.-l., et al. (2011). Curcumin Promotes Cholesterol Efflux from Adipocytes Related to PPARgamma-LXRalpha-ABCA1 Passway. Mol. Cel. Biochem. 358 (1-2), 281–285. doi:10.1007/s11010-011-0978-z
Ejaz, A., Wu, D., Kwan, P., and Meydani, M. (2009). Curcumin Inhibits Adipogenesis in 3T3-L1 Adipocytes and Angiogenesis and Obesity in C57/BL Mice. J. Nutr. 139 (5), 919–925. doi:10.3945/jn.108.100966
Elias, C. F., Aschkenasi, C., Lee, C., Kelly, J., Ahima, R. S., Bjorbæk, C., et al. (1999). Leptin Differentially Regulates NPY and POMC Neurons Projecting to the Lateral Hypothalamic Area. Neuron 23 (4), 775–786. doi:10.1016/s0896-6273(01)80035-0
Fan, C., Yan, J., Qian, Y., Wo, X., and Gao, L. (2006). Regulation of Lipoprotein Lipase Expression by Effect of Hawthorn Flavonoids on Peroxisome Proliferator Response Element Pathway. J. Pharmacol. Sci. 100 (1), 51–58. doi:10.1254/jphs.fp0050748
Fang, P., He, B., Yu, M., Shi, M., Zhu, Y., Zhang, Z., et al. (2019). Treatment with Celastrol Protects against Obesity through Suppression of Galanin-Induced Fat Intake and Activation of PGC-1α/GLUT4 axis-mediated Glucose Consumption. Biochim. Biophys. Acta (Bba) - Mol. Basis Dis. 1865 (6), 1341–1350. doi:10.1016/j.bbadis.2019.02.002
Farhan, S. M. K., Robinson, J. F., McIntyre, A. D., Marrosu, M. G., Ticca, A. F., Loddo, S., et al. (2014). A Novel LIPE Nonsense Mutation Found Using Exome Sequencing in Siblings with Late-Onset Familial Partial Lipodystrophy. Can. J. Cardiol. 30 (12), 1649–1654. doi:10.1016/j.cjca.2014.09.007
Farooqi, I. S., Bullmore, E., Keogh, J., Gillard, J., O'Rahilly, S., and Fletcher, P. C. (2007). Leptin Regulates Striatal Regions and Human Eating Behavior. Science 317 (5843), 1355. doi:10.1126/science.1144599
Fei, N., and Zhao, L. (2013). An Opportunistic Pathogen Isolated from the Gut of an Obese Human Causes Obesity in Germfree Mice. Isme j 7 (4), 880–884. doi:10.1038/ismej.2012.153
Frenzel, C., and Teschke, R. (2016). Herbal Hepatotoxicity: Clinical Characteristics and Listing Compilation. Ijms 17 (5), 588. doi:10.3390/ijms17050588
Friedman, J. M. (1997). The Alphabet of Weight Control. Nature 385 (6612), 119–120. doi:10.1038/385119a0
Friedrich, M., Petzke, K. J., Raederstorff, D., Wolfram, S., and Klaus, S. (2012). Acute Effects of Epigallocatechin Gallate from green tea on Oxidation and Tissue Incorporation of Dietary Lipids in Mice Fed a High-Fat Diet. Int. J. Obes. 36 (5), 735–743. doi:10.1038/ijo.2011.136
Gadde, K. M., Apolzan, J. W., and Berthoud, H.-R. (2018). Pharmacotherapy for Patients with Obesity. Clin. Chem. 64 (1), 118–129. doi:10.1373/clinchem.2017.272815
Goldstein, J. L., and Brown, M. S. (1990). Regulation of the Mevalonate Pathway. Nature 343 (6257), 425–430. doi:10.1038/343425a0
Gómez-Zorita, S., Fernández-Quintela, A., Macarulla, M. T., Aguirre, L., Hijona, E., Bujanda, L., et al. (2012). Resveratrol Attenuates Steatosis in Obese Zucker Rats by Decreasing Fatty Acid Availability and Reducing Oxidative Stress. Br. J. Nutr. 107 (2), 202–210. doi:10.1017/s0007114511002753
González-Muniesa, P., Mártinez-González, M.-A., Hu, F. B., Després, J.-P., Matsuzawa, Y., Loos, R. J. F., et al. (2017). Obesity. Nat. Rev. Dis. Primers 3, 17034. doi:10.1038/nrdp.2017.34
Halaas, J., Gajiwala, K., Maffei, M., Cohen, S., Chait, B., Rabinowitz, D., et al. (1995). Weight-reducing Effects of the Plasma Protein Encoded by the Obese Gene. Science 269 (5223), 543–546. doi:10.1126/science.7624777
Han, K., Bose, S., Wang, J.-H., Lim, S.-k., Chin, Y.-W., Kim, Y.-M., et al. (2017). In Vivo therapeutic Effect of Combination Treatment with Metformin and Scutellaria Baicalensis on Maintaining Bile Acid Homeostasis. PLoS One 12 (9), e0182467. doi:10.1371/journal.pone.0182467
Hasnat, M., Yuan, Z., Naveed, M., Khan, A., Raza, F., Xu, D., et al. (2019). Drp1-associated Mitochondrial Dysfunction and Mitochondrial Autophagy: a Novel Mechanism in Triptolide-Induced Hepatotoxicity. Cell. Biol. Toxicol. 35 (3), 267–280. doi:10.1007/s10565-018-9447-8
Hong, Q., Xia, C., Xiangying, H., and Quan, Y. (2015). Capsinoids Suppress Fat Accumulation via Lipid Metabolism. Mol. Med. Rep. 11 (3), 1669–1674. doi:10.3892/mmr.2014.2996
Hossain, P., Kawar, B., and El Nahas, M. (2007). Obesity and Diabetes in the Developing World - A Growing Challenge. N. Engl. J. Med. 356 (3), 213–215. doi:10.1056/NEJMp068177
Houten, S. M., Violante, S., Ventura, F. V., and Wanders, R. J. A. (2016). The Biochemistry and Physiology of Mitochondrial Fatty Acid β-Oxidation and its Genetic Disorders. Annu. Rev. Physiol. 78, 23–44. doi:10.1146/annurev-physiol-021115-105045
Hu, H.-J., Luo, X.-G., Dong, Q.-Q., Mu, A., Shi, G.-L., Wang, Q.-T., et al. (2016). Ethanol Extract of Zhongtian Hawthorn Lowers Serum Cholesterol in Mice by Inhibiting Transcription of 3-Hydroxy-3-Methylglutaryl-CoA Reductase via Nuclear Factor-Kappa B Signal Pathway. Exp. Biol. Med. (Maywood) 241 (6), 667–674. doi:10.1177/1535370215627032
Hu, W.-Y., Ma, X.-H., Zhou, W.-Y., Li, X.-X., Sun, T.-T., and Sun, H. (2017). Preventive Effect of Silibinin in Combination with Pu-Erh tea Extract on Non-alcoholic Fatty Liver Disease in Ob/ob Mice. Food Funct. 8 (3), 1105–1115. doi:10.1039/c6fo01591c
Hu, W., Wang, L., Du, G., Guan, Q., Dong, T., Song, L., et al. (2020). Effects of Microbiota on the Treatment of Obesity with the Natural Product Celastrol in Rats. Diabetes Metab. J. 44 (5), 747–763. doi:10.4093/dmj.2019.0124
Huang, F., Zheng, X., Ma, X., Jiang, R., Zhou, W., Zhou, S., et al. (2019). Theabrownin from Pu-Erh tea Attenuates Hypercholesterolemia via Modulation of Gut Microbiota and Bile Acid Metabolism. Nat. Commun. 10 (1), 4971. doi:10.1038/s41467-019-12896-x
Huang, H.-C., and Lin, J.-K. (2012). Pu-erh tea, green tea, and Black tea Suppresses Hyperlipidemia, Hyperleptinemia and Fatty Acid Synthase through Activating AMPK in Rats Fed a High-Fructose Diet. Food Funct. 3 (2), 170–177. doi:10.1039/c1fo10157a
Ibrahim, M., Jang, M., Park, M., Gobianand, K., You, S., Yeon, S.-H., et al. (2015). Capsaicin Inhibits the Adipogenic Differentiation of Bone Marrow Mesenchymal Stem Cells by Regulating Cell Proliferation, Apoptosis, Oxidative and Nitrosative Stress. Food Funct. 6 (7), 2165–2178. doi:10.1039/c4fo01069h
Incio, J., Ligibel, J. A., McManus, D. T., Suboj, P., Jung, K., Kawaguchi, K., et al. (2018). Obesity Promotes Resistance to Anti-VEGF Therapy in Breast Cancer by Up-Regulating IL-6 and Potentially FGF-2. Sci. Transl. Med. 10 (432), eaag0945. doi:10.1126/scitranslmed.aag0945
Jang, E.-M., Choi, M.-S., Jung, U. J., Kim, M.-J., Kim, H.-J., Jeon, S.-M., et al. (2008). Beneficial Effects of Curcumin on Hyperlipidemia and Insulin Resistance in High-Fat-Fed Hamsters. Metabolism 57 (11), 1576–1583. doi:10.1016/j.metabol.2008.06.014
Jeong, Hyang Sook %J Korea Journal of Herbology. 2013. "Efficacy of Alismatis Orientale Rhizoma on Obesity Induced by High Fat Diet." 28 (3):95–106. doi:10.6116/kjh.2013.28.3.95
Ji, W., and Gong, B. Q. (2007). Hypolipidemic Effects and Mechanisms of Panax Notoginseng on Lipid Profile in Hyperlipidemic Rats. J. Ethnopharmacology 113 (2), 318–324. doi:10.1016/j.jep.2007.06.022
Ji-Ping, L., Ren-Chao, T., Xiao-Meng, S., Hao-Yue, Z., Shuai, S., Ai-Zhen, X., et al. (2021). Comparison of Main Chemical Composition of Plantago Asiatica L. And P. depressa Willd. Seed Extracts and Their Anti-obesity Effects in High-Fat Diet-Induced Obese Mice. Phytomedicine 81, 153362. doi:10.1016/j.phymed.2020.153362
Jia, L., Song, N., Yang, G., Ma, Y., Li, X., Lu, R., et al. (2016a). Effects of Tanshinone IIA on the Modulation of miR-33a and the SREBP-2/Pcsk9 Signaling Pathway in Hyperlipidemic Rats. Mol. Med. Rep. 13 (6), 4627–4635. doi:10.3892/mmr.2016.5133
Jia, L., Zhang, N., Xu, Y., Chen, W.-n., Zhu, M.-l., Song, N., et al. (2016b). Tanshinone IIA Affects the HDL Subfractions Distribution Not Serum Lipid Levels: Involving in Intake and Efflux of Cholesterol. Arch. Biochem. Biophys. 592, 50–59. doi:10.1016/j.abb.2016.01.001
Jiang, Z., Huang, X., Huang, S., Guo, H., Wang, L., Li, X., et al. (2016). Sex-Related Differences of Lipid Metabolism Induced by Triptolide: The Possible Role of the LXRα/SREBP-1 Signaling Pathway. Front. Pharmacol. 7, 87. doi:10.3389/fphar.2016.00087
Jimoh, A., Tanko, Y., Ayo, J. O., Ahmed, A., and Mohammed, A. (2018). Resveratrol Increases Serum Adiponectin Level and Decreases Leptin and Insulin Level in an Experimental Model of Hypercholesterolemia. Pathophysiology 25 (4), 411–417. doi:10.1016/j.pathophys.2018.08.005
Kadowaki, T., Yamauchi, T., and Kubota, N. (2008). The Physiological and Pathophysiological Role of Adiponectin and Adiponectin Receptors in the Peripheral Tissues and CNS. FEBS Lett. 582 (1), 74–80. doi:10.1016/j.febslet.2007.11.070
Kang, J.-H., Tsuyoshi, G., Han, I.-S., Kawada, T., Kim, Y. M., and Yu, R. (2010). Dietary Capsaicin Reduces Obesity-Induced Insulin Resistance and Hepatic Steatosis in Obese Mice Fed a High-Fat Diet. Obesity (Silver Spring) 18 (4), 780–787. doi:10.1038/oby.2009.301
Kaukua, J., Pekkarinen, T., Sane, T., and Mustajoki, P. (2003). Health-related Quality of Life in Obese Outpatients Losing Weight with Very-Low-Energy Diet and Behaviour Modification: a 2-y Follow-Up Study. Int. J. Obes. 27 (9), 1072–1080. doi:10.1038/sj.ijo.0802366
Khaleel, E. F., Abdel-Aleem, G. A., and Mostafa, D. G. (2018). Resveratrol Improves High-Fat Diet Induced Fatty Liver and Insulin Resistance by Concomitantly Inhibiting Proteolytic Cleavage of Sterol Regulatory Element-Binding Proteins, Free Fatty Acid Oxidation, and Intestinal Triglyceride Absorption. Can. J. Physiol. Pharmacol. 96 (2), 145–157. doi:10.1139/cjpp-2017-0001
Kim, H.-J., Jeon, S.-M., Lee, M.-K., Jung, U. J., Shin, S.-K., and Choi, M.-S. (2009). Antilipogenic Effect of green tea Extract in C57BL/6J-Lepob/obmice. Phytother. Res. 23 (4), 467–471. doi:10.1002/ptr.2647
Kim, H., Hiraishi, A., Tsuchiya, K., and Sakamoto, K. (2010). (−) Epigallocatechin Gallate Suppresses the Differentiation of 3T3-L1 Preadipocytes through Transcription Factors FoxO1 and SREBP1cEpigallocatechin Gallate Suppresses the Differentiation of 3T3-L1 Preadipocytes through Transcription Factors FoxO1 and SREBP1c. Cytotechnology 62 (3), 245–255. doi:10.1007/s10616-010-9285-x
Kim, J. E., Lee, M. H., Nam, D. H., Song, H. K., Kang, Y. S., Lee, J. E., et al. (2013). Celastrol, an NF-Κb Inhibitor, Improves Insulin Resistance and Attenuates Renal Injury in Db/db Mice. PLoS One 8 (4), e62068. doi:10.1371/journal.pone.0062068
Kim, S., Jin, Y., Choi, Y., and Park, T. (2011). Resveratrol Exerts Anti-obesity Effects via Mechanisms Involving Down-Regulation of Adipogenic and Inflammatory Processes in Mice. Biochem. Pharmacol. 81 (11), 1343–1351. doi:10.1016/j.bcp.2011.03.012
Ko, C.-W., Qu, J., Black, D. D., and Tso, P. (2020). Regulation of Intestinal Lipid Metabolism: Current Concepts and Relevance to Disease. Nat. Rev. Gastroenterol. Hepatol. 17 (3), 169–183. doi:10.1038/s41575-019-0250-7
Kubota, N., Yano, W., Kubota, T., Yamauchi, T., Itoh, S., Kumagai, H., et al. (2007). Adiponectin Stimulates AMP-Activated Protein Kinase in the Hypothalamus and Increases Food Intake. Cel Metab. 6 (1), 55–68. doi:10.1016/j.cmet.2007.06.003
Kuhn, D. J., Burns, A. C., Kazi, A., and Ping Dou, Q. (2004). Direct Inhibition of the Ubiquitin-Proteasome Pathway by Ester Bond-Containing green tea Polyphenols Is Associated with Increased Expression of Sterol Regulatory Element-Binding Protein 2 and LDL Receptor. Biochim. Biophys. Acta (Bba) - Mol. Cel Biol. Lipids 1682 (1-3), 1–10. doi:10.1016/j.bbalip.2003.12.006
Kumar, P., Malhotra, P., Ma, K., Singla, A., Hedroug, O., Saksena, S., et al. (2011). SREBP2 Mediates the Modulation of Intestinal NPC1L1 Expression by Curcumin. Am. J. Physiology-Gastrointestinal Liver Physiol. 301 (1), G148–G155. doi:10.1152/ajpgi.00119.2011
Kuo, D.-H., Yeh, C.-H., Shieh, P.-C., Cheng, K.-C., Chen, F.-A., and Cheng, J.-T. (2009). Effect of Shanzha, a Chinese Herbal Product, on Obesity and Dyslipidemia in Hamsters Receiving High-Fat Diet. J. Ethnopharmacology 124 (3), 544–550. doi:10.1016/j.jep.2009.05.005
Lafontan, M., and Langin, D. (2009). Lipolysis and Lipid Mobilization in Human Adipose Tissue. Prog. Lipid Res. 48 (5), 275–297. doi:10.1016/j.plipres.2009.05.001
Langley, J. N. (1918). On the Stimulation and Paralysis of Nerve Cells and Nerve Endings. J. Physiol. 52 (4), 247–266. doi:10.1113/jphysiol.1918.sp001828
Lasa, A., Schweiger, M., Kotzbeck, P., Churruca, I., Simón, E., Zechner, R., et al. (2012). Resveratrol Regulates Lipolysis via Adipose Triglyceride Lipase. J. Nutr. Biochem. 23 (4), 379–384. doi:10.1016/j.jnutbio.2010.12.014
Lazo, M., and Clark, J. (2008). The Epidemiology of Nonalcoholic Fatty Liver Disease: a Global Perspective. Semin. Liver Dis. 28 (4), 339–350. doi:10.1055/s-0028-1091978
Lee, G.-R., Shin, M. K., Yoon, D.-J., Kim, A.-R., Yu, R., Park, N.-H., et al. (2013). Topical Application of Capsaicin Reduces Visceral Adipose Fat by Affecting Adipokine Levels in High-Fat Diet-Induced Obese Mice. Obesity 21 (1), 115–122. doi:10.1002/oby.20246
Ley, R. E., Bäckhed, F., Turnbaugh, P., Lozupone, C. A., Knight, R. D., and Gordon, J. I. (2005). Obesity Alters Gut Microbial Ecology. Proc. Natl. Acad. Sci. 102 (31), 11070–11075. doi:10.1073/pnas.0504978102
Li, Y., Wang, X., and Shen, Z. (2017). Traditional Chinese Medicine for Lipid Metabolism Disorders. Am. J. Transl Res. 9 (5), 2038–2049.
Li, Y., Rahman, S. U., Huang, Y., Zhang, Y., Ming, P., Zhu, L., et al. (2020). Green tea Polyphenols Decrease Weight Gain, Ameliorate Alteration of Gut Microbiota, and Mitigate Intestinal Inflammation in Canines with High-Fat-Diet-Induced Obesity. J. Nutr. Biochem. 78, 108324. doi:10.1016/j.jnutbio.2019.108324
Li, Z., Xu, J., Zheng, P., Xing, L., Shen, H., Yang, L., et al. (2015). Hawthorn Leaf Flavonoids Alleviate Nonalcoholic Fatty Liver Disease by Enhancing the Adiponectin/AMPK Pathway. Int. J. Clin. Exp. Med. 8 (10), 17295–17307.
Licinio, J., Caglayan, S., Ozata, M., Yildiz, B. O., de Miranda, P. B., O'Kirwan, F., et al. (2004). Phenotypic Effects of Leptin Replacement on Morbid Obesity, Diabetes Mellitus, Hypogonadism, and Behavior in Leptin-Deficient Adults. Proc. Natl. Acad. Sci. 101 (13), 4531–4536. doi:10.1073/pnas.0308767101
Liu, J., Lee, J., Salazar Hernandez, M. A., Mazitschek, R., and Ozcan, U. (2015). Treatment of Obesity with Celastrol. Cell 161 (5), 999–1011. doi:10.1016/j.cell.2015.05.011
Luo, J., Yang, H., and Song, B.-L. (2020). Mechanisms and Regulation of Cholesterol Homeostasis. Nat. Rev. Mol. Cel. Biol. 21 (4), 225–245. doi:10.1038/s41580-019-0190-7
Ma, N., Zhang, Z., Liao, F., Jiang, T., and Tu, Y. (2020). The Birth of Artemisinin. Pharmacol. Ther. 216, 107658. doi:10.1016/j.pharmthera.2020.107658
Martel, J., Ojcius, D. M., Chang, C.-J., Lin, C.-S., Lu, C.-C., Ko, Y.-F., et al. (2017). Anti-obesogenic and Antidiabetic Effects of Plants and Mushrooms. Nat. Rev. Endocrinol. 13 (3), 149–160. doi:10.1038/nrendo.2016.142
Mashek, D. G. (2013). Hepatic Fatty Acid Trafficking: Multiple forks in the Road. Adv. Nutr. 4 (6), 697–710. doi:10.3945/an.113.004648
Meikle, P. J., and Summers, S. A. (2017). Sphingolipids and Phospholipids in Insulin Resistance and Related Metabolic Disorders. Nat. Rev. Endocrinol. 13 (2), 79–91. doi:10.1038/nrendo.2016.169
Meng, Q., Duan, X.-p., Wang, C.-y., Liu, Z.-h., Sun, P.-y., Huo, X.-k., et al. (2017). Alisol B 23-acetate Protects against Non-alcoholic Steatohepatitis in Mice via Farnesoid X Receptor Activation. Acta Pharmacol. Sin 38 (1), 69–79. doi:10.1038/aps.2016.119
Montague, C. T., Farooqi, I. S., Whitehead, J. P., Soos, M. A., Rau, H., Wareham, N. J., et al. (1997). Congenital Leptin Deficiency Is Associated with Severe Early-Onset Obesity in Humans. Nature 387 (6636), 903–908. doi:10.1038/43185
Mun, J., Kim, S., Yoon, H.-G., You, Y., Kim, O.-K., Choi, K.-C., et al. (2019). Water Extract of Curcuma Longa L. Ameliorates Non-alcoholic Fatty Liver Disease. Nutrients 11 (10), 2536. doi:10.3390/nu11102536
Navekar, R., Rafraf, M., Ghaffari, A., Asghari-Jafarabadi, M., and Khoshbaten, M. (2017). Turmeric Supplementation Improves Serum Glucose Indices and Leptin Levels in Patients with Nonalcoholic Fatty Liver Diseases. J. Am. Coll. Nutr. 36 (4), 261–267. doi:10.1080/07315724.2016.1267597
Nissen, S. E., Wolski, K. E., Prcela, L., Wadden, T., Buse, J. B., Bakris, G., et al. (2016). Effect of Naltrexone-Bupropion on Major Adverse Cardiovascular Events in Overweight and Obese Patients with Cardiovascular Risk Factors. Jama 315 (10), 990–1004. doi:10.1001/jama.2016.1558
Panchal, S., Bliss, E., and Brown, L. (2018). Capsaicin in Metabolic Syndrome. Nutrients 10 (5), 630. doi:10.3390/nu10050630
Park, Y.-J., Kim, M.-S., Kim, H.-R., Kim, J.-M., Hwang, J.-K., Yang, S.-H., et al. (2014). Ethanol Extract ofAlismatis rhizomeInhibits Adipocyte Differentiation of OP9 Cells. Evidence-Based Complement. Altern. Med. 2014, 1–9. doi:10.1155/2014/4150972014
Pawlak, M., Lefebvre, P., and Staels, B. (2015). Molecular Mechanism of PPARα Action and its Impact on Lipid Metabolism, Inflammation and Fibrosis in Non-alcoholic Fatty Liver Disease. J. Hepatol. 62 (3), 720–733. doi:10.1016/j.jhep.2014.10.039
Pearl, R. L., Wadden, T. A., Tronieri, J. S., Berkowitz, R. I., Chao, A. M., Alamuddin, N., et al. (2018). Short- and Long-Term Changes in Health-Related Quality of Life with Weight Loss: Results from a Randomized Controlled Trial. Obesity 26 (6), 985–991. doi:10.1002/oby.22187
Pelleymounter, M., Cullen, M., Baker, M., Hecht, R., Winters, D., Boone, T., et al. (1995). Effects of the Obese Gene Product on Body Weight Regulation in Ob/ob Mice. Science 269 (5223), 540–543. doi:10.1126/science.7624776
Pi-Sunyer, X., Astrup, A., Fujioka, K., Greenway, F., Halpern, A., Krempf, M., et al. (2015). A Randomized, Controlled Trial of 3.0 Mg of Liraglutide in Weight Management. N. Engl. J. Med. 373 (1), 11–22. doi:10.1056/NEJMoa1411892
Pinhas-Hamiel, O., Singer, S., Pilpel, N., Fradkin, A., Modan, D., and Reichman, B. (2006). Health-related Quality of Life Among Children and Adolescents: Associations with Obesity. Int. J. Obes. 30 (2), 267–272. doi:10.1038/sj.ijo.0803107
Qin, B., Polansky, M. M., Harry, D., and Anderson, R. A. (2010). Green tea Polyphenols Improve Cardiac Muscle mRNA and Protein Levels of Signal Pathways Related to Insulin and Lipid Metabolism and Inflammation in Insulin-Resistant Rats. Mol. Nutr. Food Res. 54 (Suppl. 1), S14–S23. doi:10.1002/mnfr.200900306
Rabot, S., Membrez, M., Bruneau, A., Gérard, P., Harach, T., Moser, M., et al. (2010). Germ-free C57BL/6J Mice Are Resistant to High-Fat-Diet-Induced Insulin Resistance and Have Altered Cholesterol Metabolism. FASEB J. 24 (12), 4948–4959. doi:10.1096/fj.10-164921
Rakhshandehroo, M., Sanderson, L. M., Matilainen, M., Stienstra, R., Carlberg, C., de Groot, P. J., et al. (2007). Comprehensive Analysis of PPARα-dependent Regulation of Hepatic Lipid Metabolism by Expression Profiling. PPAR Res. 2007, 1–13. doi:10.1155/2007/26839
Ramírez-Boscá, A., Soler, A., Carrión, M. A., Díaz-Alperi, J., Bernd, A., Quintanilla, C., et al. (2000). An Hydroalcoholic Extract of Curcuma Longa Lowers the Apo B/apo A Ratio. Implications for Atherogenesis Prevention. Mech. Ageing Dev. 119 (1-2), 41–47. doi:10.1016/s0047-6374(00)00169-x
Ridaura, V. K., Faith, J. J., Rey, F. E., Cheng, J., Duncan, A. E., Kau, A. L., et al. (2013). Gut Microbiota from Twins Discordant for Obesity Modulate Metabolism in Mice. Science 341 (6150), 1241214. doi:10.1126/science.1241214
Rocha, V. Z., and Libby, P. (2009). Obesity, Inflammation, and Atherosclerosis. Nat. Rev. Cardiol. 6 (6), 399–409. doi:10.1038/nrcardio.2009.55
Rodriguez, J. A., Ben Ali, Y., Abdelkafi, S., Mendoza, L. D., Leclaire, J., Fotiadu, F., et al. (2010). In Vitro stereoselective Hydrolysis of Diacylglycerols by Hormone-Sensitive Lipase. Biochim. Biophys. Acta (Bba) - Mol. Cel Biol. Lipids 1801 (1), 77–83. doi:10.1016/j.bbalip.2009.09.020
Rosca, A. E., Iesanu, M. I., Zahiu, C. D. M., Voiculescu, S. E., Paslaru, A. C., and Zagrean, A.-M. (2020). Capsaicin and Gut Microbiota in Health and Disease. Molecules 25 (23), 5681. doi:10.3390/molecules25235681
Santacruz, A., Marcos, A., Wärnberg, J., Martí, A., Martin-Matillas, M., Campoy, C., et al. (2009). Interplay between Weight Loss and Gut Microbiota Composition in Overweight Adolescents. Obesity (Silver Spring) 17 (10), 1906–1915. doi:10.1038/oby.2009.112
Seo, B. R., Bhardwaj, P., Choi, S., Gonzalez, J., Andresen Eguiluz, R. C., Wang, K., et al. (2015). Obesity-dependent Changes in Interstitial ECM Mechanics Promote Breast Tumorigenesis. Sci. Transl. Med. 7 (301), 301ra130. doi:10.1126/scitranslmed.3010467
Sham, T.-T., Chan, C.-O., Wang, Y.-H., Yang, J.-M., Mok, D. K.-W., and Chan, S.-W. (2014). A Review on the Traditional Chinese Medicinal Herbs and Formulae with Hypolipidemic Effect. Biomed. Res. Int. 2014, 1–21. doi:10.1155/2014/925302
Shi, Z., Zhu, J.-x., Guo, Y.-m., Niu, M., Zhang, L., Tu, C., et al. (2020). Epigallocatechin Gallate during Dietary Restriction - Potential Mechanisms of Enhanced Liver Injury. Front. Pharmacol. 11, 609378. doi:10.3389/fphar.2020.609378
Shih, C.-C., Lin, C.-H., Lin, Y.-J., and Wu, J.-B. (2013). Validation of the Antidiabetic and Hypolipidemic Effects of Hawthorn by Assessment of Gluconeogenesis and Lipogenesis Related Genes and AMP-Activated Protein Kinase Phosphorylation. Evidence-Based Complement. Altern. Med. 2013, 1–12. doi:10.1155/2013/597067
Shimamura, Y., Yoda, M., Sakakibara, H., Matsunaga, K., and Masuda, S. (2013). Pu-erh tea Suppresses Diet-Induced Body Fat Accumulation in C57BL/6J Mice by Down-Regulating SREBP-1c and Related Molecules. Biosci. Biotechnol. Biochem. 77 (7), 1455–1460. doi:10.1271/bbb.130097
Singh, V., Jain, M., Misra, A., Khanna, V., Prakash, P., Malasoni, R., et al. (2015). Curcuma Oil Ameliorates Insulin Resistance & Associated Thrombotic Complications in Hamster & Rat. Indian J. Med. Res. 141 (6), 823–832. doi:10.4103/0971-5916.160719
Singh, V., Jain, M., Misra, A., Khanna, V., Rana, M., Prakash, P., et al. (2013). Curcuma Oil Ameliorates Hyperlipidaemia and Associated Deleterious Effects in golden Syrian Hamsters. Br. J. Nutr. 110 (3), 437–446. doi:10.1017/S0007114512005363
Smeets, A. J., and Westerterp-Plantenga, M. S. (2009). The Acute Effects of a Lunch Containing Capsaicin on Energy and Substrate Utilisation, Hormones, and Satiety. Eur. J. Nutr. 48 (4), 229–234. doi:10.1007/s00394-009-0006-1
Smith, S. R., Weissman, N. J., Anderson, C. M., Sanchez, M., Chuang, E., Stubbe, S., et al. (2010). Multicenter, Placebo-Controlled Trial of Lorcaserin for Weight Management. N. Engl. J. Med. 363 (3), 245–256. doi:10.1056/NEJMoa0909809
Solas, M., Milagro, F. I., Martínez-Urbistondo, D., Ramirez, M. J., and Martínez, J. A. (2016). Precision Obesity Treatments Including Pharmacogenetic and Nutrigenetic Approaches. Trends Pharmacol. Sci. 37 (7), 575–593. doi:10.1016/j.tips.2016.04.008
Song, J.-X., Ren, H., Gao, Y.-F., Lee, C.-Y., Li, S.-F., Zhang, F., et al. (2017). Dietary Capsaicin Improves Glucose Homeostasis and Alters the Gut Microbiota in Obese Diabetic Ob/ob Mice. Front. Physiol. 8, 602. doi:10.3389/fphys.2017.00602
Song, W.-Y., and Choi, J.-H. (2016). KoreanCurcuma Longa L. Induces Lipolysis and Regulates Leptin in Adipocyte Cells and Rats. Nutr. Res. Pract. 10 (5), 487–493. doi:10.4162/nrp.2016.10.5.487
Stoll, B. (1998). Teenage Obesity in Relation to Breast Cancer Risk. Int. J. Obes. 22 (11), 1035–1040. doi:10.1038/sj.ijo.0800769
Sun, Y., Wang, Y., Song, P., Wang, H., Xu, N., Wang, Y., et al. (2019). Anti-obesity Effects of Instant Fermented Teas In Vitro and in Mice with High-Fat-Diet-Induced Obesity. Food Funct. 10 (6), 3502–3513. doi:10.1039/c9fo00162j
Takizawa, Y., Nakata, R., Fukuhara, K., Yamashita, H., Kubodera, H., and Inoue, H. (2015). The 4′-Hydroxyl Group of Resveratrol Is Functionally Important for Direct Activation of PPARα. PLoS One 10 (3), e0120865. doi:10.1371/journal.pone.0120865
Tarantino, G., Pezzullo, M. G., Minno, M. N. D. d., Milone, F., Pezzullo, L. S., Milone, M., et al. (2009). Drug-induced Liver Injury Due to "natural Products" Used for Weight Loss: a Case Report. Wjg 15 (19), 2414–2417. doi:10.3748/wjg.15.2414
Tian, C., Ye, X., Zhang, R., Long, J., Ren, W., Ding, S., et al. (2013). Green Tea Polyphenols Reduced Fat Deposits in High Fat-Fed Rats via Erk1/2-Pparγ-Adiponectin Pathway. PLoS One 8 (1), e53796. doi:10.1371/journal.pone.0053796
Timmers, S., Konings, E., Bilet, L., Houtkooper, R. H., van de Weijer, T., Goossens, G. H., et al. (2011). Calorie Restriction-like Effects of 30 Days of Resveratrol Supplementation on Energy Metabolism and Metabolic Profile in Obese Humans. Cel Metab. 14 (5), 612–622. doi:10.1016/j.cmet.2011.10.002
Tomé-Carneiro, J., Gonzálvez, M., Larrosa, M., García-Almagro, F. J., Avilés-Plaza, F., Parra, S., et al. (2012). Consumption of a Grape Extract Supplement Containing Resveratrol Decreases Oxidized LDL and ApoB in Patients Undergoing Primary Prevention of Cardiovascular Disease: a Triple-Blind, 6-month Follow-Up, Placebo-Controlled, Randomized Trial. Mol. Nutr. Food Res. 56 (5), 810–821. doi:10.1002/mnfr.201100673
Tu, C., Jiang, B. Q., Zhao, Y. L., Li, C. Y., Li, N., Li, X. F., et al. (2015). [Comparison of Processed and Crude Polygoni Multiflori Radix Induced Rat Liver Injury and Screening for Sensitive Indicators]. Zhongguo Zhong Yao Za Zhi. 40 (4), 654–660. doi:10.4268/cjcmm20150416
Tung, N. H., Nakajima, K., Uto, T., Hai, N. T., Long, D. D., Ohta, T., et al. (2017). Bioactive Triterpenes from the Root of Salvia Miltiorrhiza Bunge. Phytother. Res. 31 (9), 1457–1460. doi:10.1002/ptr.5877
Turnbaugh, P. J., Hamady, M., Yatsunenko, T., Cantarel, B. L., Duncan, A., Ley, R. E., et al. (2009). A Core Gut Microbiome in Obese and Lean Twins. Nature 457 (7228), 480–484. doi:10.1038/nature07540
Turnbaugh, P. J., Ley, R. E., Mahowald, M. A., Magrini, V., Mardis, E. R., and Gordon, J. I. (2006). An Obesity-Associated Gut Microbiome with Increased Capacity for Energy Harvest. Nature 444 (7122), 1027–1031. doi:10.1038/nature05414
Valentine, C., Ohnishi, K., Irie, K., and Murakami, A. (2019). Curcumin May Induce Lipolysis via Proteo-Stress in Huh7 Human Hepatoma Cells. J. Clin. Biochem. Nutr. 65 (2), 91–98. doi:10.3164/jcbn.19-7
Waisundara, V. Y., Hsu, A., Tan, B. K.-H., and Huang, D. (2009). Baicalin Reduces Mitochondrial Damage in Streptozotocin-Induced Diabetic Wistar Rats. Diabetes Metab. Res. Rev. 25 (7), 671–677. doi:10.1002/dmrr.1005
Wang, C., Shi, C., Yang, X., Yang, M., Sun, H., and Wang, C. (2014). Celastrol Suppresses Obesity Process via Increasing Antioxidant Capacity and Improving Lipid Metabolism. Eur. J. Pharmacol. 744, 52–58. doi:10.1016/j.ejphar.2014.09.043
Wang, J.-M., Cai, H., Li, J.-H., Chen, R.-X., Zhang, Y.-Y., Li, J.-Y., et al. (2018). Detoxication Mechanisms of Radix Tripterygium Wilfordii via Compatibility with Herba Lysimachia Christinae in S180-Bearing Mice by Involving Nrf2. Biosci. Rep. 38 (4). doi:10.1042/bsr20180429
Wang, J., Miao, M., Zhang, Y., Liu, R., Li, X., Cui, Y., et al. (2015). Quercetin Ameliorates Liver Injury Induced with Tripterygium Glycosides by Reducing Oxidative Stress and Inflammation. Can. J. Physiol. Pharmacol. 93 (6), 427–433. doi:10.1139/cjpp-2015-0038
Wang, P., Li, D., Ke, W., Liang, D., Hu, X., and Chen, F. (2020). Resveratrol-induced Gut Microbiota Reduces Obesity in High-Fat Diet-Fed Mice. Int. J. Obes. 44 (1), 213–225. doi:10.1038/s41366-019-0332-1
Wang, Q., Li, C., Zhang, Q., Wang, Y., Shi, T., Lu, L., et al. (2016). The Effect of Chinese Herbs and its Effective Components on Coronary Heart Disease through PPARs-Pgc1α Pathway. BMC Complement. Altern. Med. 16 (1), 514. doi:10.1186/s12906-016-1496-z
Wang, S., Moustaid-Moussa, N., Chen, L., Mo, H., Shastri, A., Su, R., et al. (2014). Novel Insights of Dietary Polyphenols and Obesity. J. Nutr. Biochem. 25 (1), 1–18. doi:10.1016/j.jnutbio.2013.09.001
Wang, Y., Tang, C., Tang, Y., Yin, H., and Liu, X. (2020). Capsaicin Has an Anti-obesity Effect through Alterations in Gut Microbiota Populations and Short-Chain Fatty Acid Concentrations. Food Nutr. Res. 64. doi:10.29219/fnr.v64.3525
Wang, Y., Viscarra, J., Kim, S.-J., and Sul, H. S. (2015). Transcriptional Regulation of Hepatic Lipogenesis. Nat. Rev. Mol. Cel. Biol. 16 (11), 678–689. doi:10.1038/nrm4074
Wen, J., Chang, Y., Huo, S., Li, W., Huang, H., Gao, Y., et al. (2020). Tanshinone IIA Attenuates Atherosclerosis via Inhibiting NLRP3 Inflammasome Activation. Aging 13 (1), 910–932. doi:10.18632/aging.202202
Wille, N., Erhart, M., Petersen, C., and Ravens-Sieberer, U. (2008). The Impact of Overweight and Obesity on Health-Related Quality of Life in Childhood - Results from an Intervention Study. BMC Public Health 8, 421. doi:10.1186/1471-2458-8-421
Wu, L., Yan, Q., Chen, F., Cao, C., and Wang, S. (2021). Bupleuri Radix Extract Ameliorates Impaired Lipid Metabolism in High-Fat Diet-Induced Obese Mice via Gut Microbia-Mediated Regulation of FGF21 Signaling Pathway. Biomed. Pharmacother. 135, 111187. doi:10.1016/j.biopha.2020.111187
Xi, Y., Wu, M., Li, H., Dong, S., Luo, E., Gu, M., et al. (2015). Baicalin Attenuates High Fat Diet-Induced Obesity and Liver Dysfunction: Dose-Response and Potential Role of CaMKKβ/AMPK/ACC Pathway. Cell. Physiol. Biochem. 35 (6), 2349–2359. doi:10.1159/000374037
Xia, W., Sun, C., Zhao, Y., and Wu, L. (2011). Hypolipidemic and Antioxidant Activities of Sanchi (Radix Notoginseng) in Rats Fed with a High Fat Diet. Phytomedicine 18 (6), 516–520. doi:10.1016/j.phymed.2010.09.007
Xian, Z., Liu, Y., Xu, W., Duan, F., Guo, Z., and Xiao, H. (2017). The Anti-hyperlipidemia Effects of Raw Polygonum Multiflorum Extract In Vivo. Biol. Pharm. Bull. 40 (11), 1839–1845. doi:10.1248/bpb.b17-00218
Xin-Min, M. O., Liu, R., Jian-Ping, L. I., Fan, J. J., and Gan, G. X. (2010). Effects of Danshen on Non-alcoholic Fatty Liver in Serum Leptin Ghrelin. Chinese Archives of Traditional Chinese Medicine 28 (11), 2252–2254. doi:10.13193/j.archtcm.2010.11.14.moxm.064
Xu, S., Feng, Y., He, W., Xu, W., Xu, W., Yang, H., et al. (2021). Celastrol in Metabolic Diseases: Progress and Application Prospects. Pharmacol. Res. 167, 105572. doi:10.1016/j.phrs.2021.105572
Yamashita, M., Kumazoe, M., Nakamura, Y., Won, Y. S., Bae, J., Yamashita, S., et al. (2016). The Combination of Green Tea Extract and Eriodictyol Inhibited High-Fat/High-Sucrose Diet-Induced Cholesterol Upregulation Is Accompanied by Suppression of Cholesterol Synthesis Enzymes. J. Nutr. Sci. Vitaminol 62 (4), 249–256. doi:10.3177/jnsv.62.249
Yan-Xia, S. U., Xun, Y. H., and Chen, Z. Y. (2011). Effect of Sanchi on Hepatic Expression of SREBP1c in Rats with Alcoholic Liver Disease. Chinese Journal of Clinical Pharmacology and Therapeutics 16 (2), 148–154.
Yang, C.-y., Wang, J., Zhao, Y., Shen, L., Jiang, X., Xie, Z.-g., et al. (2010). Anti-diabetic Effects of Panax Notoginseng Saponins and its Major Anti-hyperglycemic Components. J. Ethnopharmacology 130 (2), 231–236. doi:10.1016/j.jep.2010.04.039
Yang, J., Sun, L., Wang, L., Hassan, H. M., Wang, X., Hylemon, P. B., et al. (2017). Activation of Sirt1/FXR Signaling Pathway Attenuates Triptolide-Induced Hepatotoxicity in Rats. Front. Pharmacol. 8, 260. doi:10.3389/fphar.2017.00260
Yashiro, T., Nanmoku, M., Shimizu, M., Inoue, J., and Sato, R. (2012). Resveratrol Increases the Expression and Activity of the Low Density Lipoprotein Receptor in Hepatocytes by the Proteolytic Activation of the Sterol Regulatory Element-Binding Proteins. Atherosclerosis 220 (2), 369–374. doi:10.1016/j.atherosclerosis.2011.11.006
Yi-Xin, M. A., Jia, L. Q., Song, N., Wang, J. Y., Xue, L., Zhang, N., et al. (2017). Effects of Tanshinone ⅡA on Liver Proteome Expression of Hyperlipidemia Rats by iTRAQ Technology. Chinese Journal of Integrated Traditional and Western Medicine. doi:10.7661/j.cjim.20170315.041
Yu, H. R., Sheen, J. M., Tiao, M. M., Tain, Y. L., Chen, C. C., Lin, I. C., et al. (2019). Resveratrol Treatment Ameliorates Leptin Resistance and Adiposity Programed by the Combined Effect of Maternal and Post-Weaning High-Fat Diet. Mol. Nutr. Food Res. 63 (13), 1802385. doi:10.1002/mnfr.201801385
Yu, R., Lv, Y., Wang, J., Pan, N., Zhang, R., Wang, X., et al. (2016). Baicalin Promotes Cholesterol Efflux by Regulating the Expression of SR-BI in Macrophages. Exp. Ther. Med. 12 (6), 4113–4120. doi:10.3892/etm.2016.3884
Zechner, R., Madeo, F., and Kratky, D. (2017). Cytosolic Lipolysis and Lipophagy: Two Sides of the Same coin. Nat. Rev. Mol. Cel. Biol. 18 (11), 671–684. doi:10.1038/nrm.2017.76
Zeng, L., Yan, J., Luo, L., and Zhang, D. (2015). Effects of Pu-Erh tea Aqueous Extract (PTAE) on Blood Lipid Metabolism Enzymes. Food Funct. 6 (6), 2008–2016. doi:10.1039/c5fo00362h
Zhang, J., Zhang, H., Deng, X., Zhang, N., Liu, B., Xin, S., et al. (2018). Baicalin Attenuates Non-alcoholic Steatohepatitis by Suppressing Key Regulators of Lipid Metabolism, Inflammation and Fibrosis in Mice. Life Sci. 192, 46–54. doi:10.1016/j.lfs.2017.11.027
Zhang, X.-H., Huang, B., Choi, S.-K., and Seo, J.-S. (2012). Anti-obesity Effect of Resveratrol-Amplified Grape Skin Extracts on 3T3-L1 Adipocytes Differentiation. Nutr. Res. Pract. 6 (4), 286–293. doi:10.4162/nrp.2012.6.4.286
Zhang, X., Zhang, B., Zhang, C., Sun, G., and Sun, X. (2020). Effect of Panax Notoginseng Saponins and Major Anti-obesity Components on Weight Loss. Front. Pharmacol. 11, 601751. doi:10.3389/fphar.2020.601751
Zhang, Y., Geng, C., Liu, X., Li, M., Gao, M., Liu, X., et al. (2017). Celastrol Ameliorates Liver Metabolic Damage Caused by a High-Fat Diet through Sirt1. Mol. Metab. 6 (1), 138–147. doi:10.1016/j.molmet.2016.11.002
Zhang, Y., Kishi, H., and Kobayashi, S. (2018). Add-on Therapy with Traditional Chinese Medicine: An Efficacious Approach for Lipid Metabolism Disorders. Pharmacol. Res. 134, 200–211. doi:10.1016/j.phrs.2018.06.004
Zhang, Y., Zhang, L., Geng, Y., and Geng, Y. (2014). Hawthorn Fruit Attenuates Atherosclerosis by Improving the Hypolipidemic and Antioxidant Activities in Apolipoprotein E-Deficient Mice. Jat 21 (2), 119–128. doi:10.5551/jat.19174
Zhao, D., Liu, J., Wang, M., Zhang, X., and Zhou, M. (2019). Epidemiology of Cardiovascular Disease in China: Current Features and Implications. Nat. Rev. Cardiol. 16 (4), 203–212. doi:10.1038/s41569-018-0119-4
Zhao, J., Sun, X.-B., Ye, F., and Tian, W.-X. (2011). Suppression of Fatty Acid Synthase, Differentiation and Lipid Accumulation in Adipocytes by Curcumin. Mol. Cel. Biochem. 351 (1-2), 19–28. doi:10.1007/s11010-010-0707-z
Zhou, L., Xiao, X., Zhang, Q., Zheng, J., and Deng, M. (2019). Deciphering the Anti-obesity Benefits of Resveratrol: The "Gut Microbiota-Adipose Tissue" Axis. Front. Endocrinol. 10, 413. doi:10.3389/fendo.2019.00413
Glossary
ABCA ATP-binding cassette transporter A
ABCG ATP-binding cassette transporter G
ACAT acyl coenzyme A–cholesterol acyltransferase
ACC acetyl-CoA carboxylase
ApoA apolipoprotein A
ARH arcuate hypothalamus
AS atherosclerosis
ATGL adipose triglyceride hydrolase
BMI body mass index
BSH bile-salt hydrolase
C/EBPβ CCAAT/enhancer-binding protein β
CD36 cluster of differentiation 36
chREBP carbohydrate-responsive element–binding protein
ChP Pharmacopoeia of the People's Republic of China
CNS central nervous system
CPT1 carnitine palmitoyl transferase 1
CEs cholesterol esters
CVD cardiovascular disease
CYP7A1 cholesterol 7α-hydroxylase
DGAT diacylglycerol acyltransferase
DNL de novo lipogenesis
EGCG epigallocatechin gallate
ERR estrogen-related receptor
FA fatty acid
FAS fatty acid synthase
FATP fatty acid transport protein
FGF15 fibroblast growth factor 15
FOXO1 forkhead box protein O1
FXR farnesoid X receptor
GLP1 glucagon-like peptide 1
GPAT glycerol-3-phosphate acyltransferase
G6Pase PGC1α-dependent enzyme
HDL high-density lipoprotein
HFD high-fat diet
HFrD high-fructose diet
HL hepatic lipase
HMGCR 3-hydroxy-3-methylglutaryl coenzyme A reductase
HNF4α hepatocyte nuclear receptor 4α
HSL hormone-sensitive lipase
IDL intermediate-density lipoprotein
IR insulin resistance
LDL low-density lipoprotein
LDL-c LDL particles
LDLR LDL receptor
LD lipid droplet
LOX-1 lectin-like oxidized LDL receptor-1
LPL lipoprotein lipase
LXR liver X receptor
MAG monoacylglycerol
MCD MCD methionine and choline–deficient diet
MGL monoacylglycerol lipase
MUFA mono-unsaturated fatty acid
NADPH nicotinamide adenine dinucleotide phosphate
NAFLD non-alcoholic fatty liver disease
NE3 n-butanol extract of Panax notoginseng (Burkill) F.H. Chen
NPC1L1 Niemann–Pick C1-like 1
oxLDL oxidized low-density lipoprotein
PCSK9 proprotein convertase subtilisin/kexin type 9
PGC1α PPARγ co-activator 1α
PL phospholipid
PNSs Panax notoginseng (Burkill) F.H. Chen saponins
PPAR peroxisome proliferator–activated receptor
PTE pu-erh tea extract
SCD stearoyl-CoA desaturase
SM squalene monooxygenase
SR-BI scavenger receptor class B type I
SREBP sterol regulatory element–binding protein
T2D type 2 diabetes
TAG triacylglycerol
TC total cholesterol
TCM traditional Chinese medicine
TG triglyceride
TrpV1 transient receptor potential cation channel subfamily V member 1
VLDL very low–density lipoprotein
WHO World Health Organization
WT wild type
Keywords: traditional Chinese medicines, obesity-related metabolic diseases, anti-obesity effect, lipid metabolism, mechanisms
Citation: Fan Q, Xu F, Liang B and Zou X (2021) The Anti-Obesity Effect of Traditional Chinese Medicine on Lipid Metabolism. Front. Pharmacol. 12:696603. doi: 10.3389/fphar.2021.696603
Received: 17 April 2021; Accepted: 01 June 2021;
Published: 21 June 2021.
Edited by:
Marcello Locatelli, University of Studies G. d’Annunzio Chieti and Pescara, ItalyReviewed by:
Carmine Finelli, Ospedale Cav. R. Apicella–ASL Napoli 3 Sud, ItalyGiovanni Tarantino, University of Naples Federico II, Italy
Copyright © 2021 Fan, Xu, Liang and Zou. This is an open-access article distributed under the terms of the Creative Commons Attribution License (CC BY). The use, distribution or reproduction in other forums is permitted, provided the original author(s) and the copyright owner(s) are credited and that the original publication in this journal is cited, in accordance with accepted academic practice. No use, distribution or reproduction is permitted which does not comply with these terms.
*Correspondence: Furong Xu, Znhyb25nOTlAMTYzLmNvbQ==; Bin Liang, bGlhbmdiNzNAeXVuLmVkdS5jbg==; Xiaoju Zou, eGlhb2p1em91QDE2My5jb20=