- 1Tongji University Cancer Center, Shanghai Tenth People's Hospital, Tongji University School of Medicine, Shanghai, China
- 2Department of Laboratory Medicine, Changzheng Hospital, Naval Medical University, Shanghai, China
- 3Department of Orthopedic Surgery, Spine Center, Changzheng Hospital, Naval Medical University, Shanghai, China
- 4Basic Medical College, Naval Medical University, Shanghai, China
- 5Hebei Key Laboratory of Active Components and Functions in Natural Products, College of Chemical Engi-neering, Hebei Normal University of Science and Technology, Qinhuangdao, China
Lack of vascularization is directly associated with refractory wound healing in diabetes mellitus (DM). Enrichment of endothelial precursor cells (EPCs) is a promising but challenging approach for the treatment of diabetic wounds. Herein, we investigate the action of nicotinamide riboside (NR) on EPC function for improved healing of diabetic wounds. Db/db mice that were treated with NR-supplemented food (400 mg/kg/d) for 12 weeks exhibited higher wound healing rates and angiogenesis than untreated db/db mice. In agreement with this phenotype, NR supplementation significantly increased the number of blood EPCs and bone marrow (BM)-derived EPCs of db/db mice, as well as the tube formation and adhesion functions of BM-EPCs. Furthermore, NR-supplemented BM-EPCs showed higher expression of sirtuin 1 (Sirt1), phosphorylated adenosine monophosphate–activated protein kinase (p-AMPK), and lower expression of acetylated peroxisome proliferator–activated receptor γ coactivator (PGC-1α) than BM-EPCs isolated from untreated db/db mice. Knockdown of Sirt1 in BM-EPCs significantly abolished the tube formation and adhesion function of NR as well as the expression of p-AMPK and deacetylated PGC-1a. Inhibition of AMPK abolished the NR-regulated EPC function but had no effect on Sirt1 expression, demonstrating that NR enhances EPC function through the Sirt1-AMPK pathway. Overall, this study demonstrates that the oral uptake of NR enhances the EPC function to promote diabetic wound healing, indicating that NR supplementation might be a promising strategy to prevent the progression of diabetic complications.
Introduction
The prevalence of diabetes mellitus (DM) and DM-related complications has been convincingly described regarding clinical, social, and economic implications (American Diabetes Association, 2018; Sørensen et al., 2016). By 2030, there will be approximately 430 million people with DM worldwide (Ginter and Simko, 2012). The incidence rate of diabetic foot ulcers (DFUs) in this cohort is increasing to around 20%, with an average annual cost of $8,659 for one patient in the United States (American Diabetes Association, 2018; Ginter and Simko, 2012). Diabetic patients with foot ulcers are at a high risk of operative limb salvage programs, including even major and minor amputations (Wukich et al., 2017). As lower extremity amputations are most often attributed to intractable DFUs, earlier prevention and treatment for patients with DFUs definitely play a critical role.
Delayed wound closure in DM patients is involved in peripheral circulatory disorders, which are closely related to neovascularization and angiogenesis (Forsythe and Hinchliffe, 2016; Hugo et al., 2016). It is well known that endothelial precursor cells (EPCs) play an important role in modified angiogenesis of the wound healing process (Han et al., 2017a). Since EPCs are rich in the peripheral blood of adults, there have been evidence-based demonstrations of migration to the damage site and formation of new vessels, as well as maintenance of vascular homeostasis (Catrina and Zheng, 2016). In recent years, EPC transplantation therapy has become a promising measure for ischemic diseases; remarkably, it could speed up the tissue repair process and ameliorate pathological conditions, including nutrient deficiency, inflammation, ischemia, and lower EPC numbers (Li et al., 2018; Zhao et al., 2018). Unfortunately, clinical evidence suggests that both EPC number and EPC function are impaired in patients with DM compared to healthy individuals (Wils et al., 2017). Therefore, several studies have shown that pharmacologically or molecularly modified EPCs are key strategies for enhancing their functionality and therapeutic effects (Ishida et al., 2012; Yu et al., 2016; Han et al., 2017a). In diabetic mice models, metformin and acarbose have been reported to promote wound closure by recruiting bone marrow (BM)-EPCs and improving their activity (Yu et al., 2016; Han et al., 2017b). Nevertheless, the mechanisms that underlie EPC-mediated wound healing have not been fully elucidated. Thus, it is imperative to investigate the mechanisms and novel agents in order to manage diabetic wound healing.
Nicotinamide riboside (NR), a new form of vitamin B3, acts as a nicotinamide adenine dinucleotide (NAD) precursor (Lee and Yang, 2019). NAD not only plays an essential role in cellular metabolism and energy production but also is a cofactor for sirtuin deacetylases (Lee and Yang, 2019). An extensive literature has indicated that supplementation of NAD can ameliorate age-related neurodegenerative diseases, nonalcoholic fatty liver disease (NAFLD), and metabolic disorders (Cantó et al., 2012; Zhou et al., 2016; Xie et al., 2019). Neiva et al. (2005) have reported that supplementation of vitamin B complex resulted in accelerated periodontal wound healing. In addition, Wang et al. (2016) have shown that depletion of the NAD pool is associated with the impairment of EPC mobilization in diabetes. NR has promising characteristics for Alzheimer’s disease, insulin sensitivity, and diabetic neuropathy (Cantó et al., 2012; Trammell et al., 2016; Xie et al., 2019). In addition, an oral supplement of NR is available, with the brand name NIAGEN®. However, whether replenishing NAD by supplementation of NR can enhance EPC function and improve wound repair remains unknown.
In recent years, accumulated evidence has demonstrated that dysregulation of adenosine monophosphate–activated protein kinase (AMPK)-mediated EPC dysfunction is associated with diabetic complications (Yu et al., 2016; Han et al., 2017a). Activated AMPK by metformin can enhance EPC numbers in circulation and improve the tube formation of EPCs. Moreover, inactivating the sirtuin 1 (Sirt1)/AMPK pathway has been observed in obese mice, which aggravates metabolic disorders (Francini et al., 2019). In obese mice, resveratrol as a Sirt1 activator could upregulate the expression of Sirt1 and phosphorylated AMPK (p-AMPK), inducing glucose metabolism (Tiao et al., 2018). Silence of Sirt1 could abolish the promoting effects of NAD on EPC proliferation and migration (Wang et al., 2014).
Therefore, we hypothesized that NR treatment may improve the EPC function to promote wound healing in diabetic conditions. In this study, we show that NR supplementation promoted angiogenesis and wound healing in db/db mice, and that NR regulated the EPC function to promote angiogenesis through mediating the Sirt1/AMPK pathway.
Materials and Methods
Animals
Four-week-old male C57BLKS/J db/db mice and age-matched C57BL/6J mice were purchased from the Laboratory Animal Center of Hangzhou Medical College (Hangzhou, China). All mice were housed in cages at 23 ± 2°C in a humidity-controlled holding room with a 12-h light/dark schedule. Mice were provided with free access to water and food. All mice were handled in accordance with the National Institutes of Health’s Guide for the Care and Use of Laboratory Animals.
Experimental Protocols
The db/db mice were randomly separated into 2 experimental groups, either receiving common food or NR-supplemented food (approximately 400 mg/kg/day; Xie et al., 2019) for 12 weeks. NR was bought from Baikai Chemical Technology Co., Ltd. (Hangzhou, China, CAS: 1341-23-7) and mixed into the common food. The C57BL/6J mice received common pellets as a control group. Blood glucose was detected every week using a monitoring system (Maochang, Taipei, China). Whole blood samples of the mice were obtained from the tail veins. After 12 weeks of NR administration, body weight changes were determined in each group. Six mice of each group were used to produce a wound model and isolating of BM-EPCs (Figure 1A). At the end of the experiment, all mice were sacrificed by cervical dislocation.
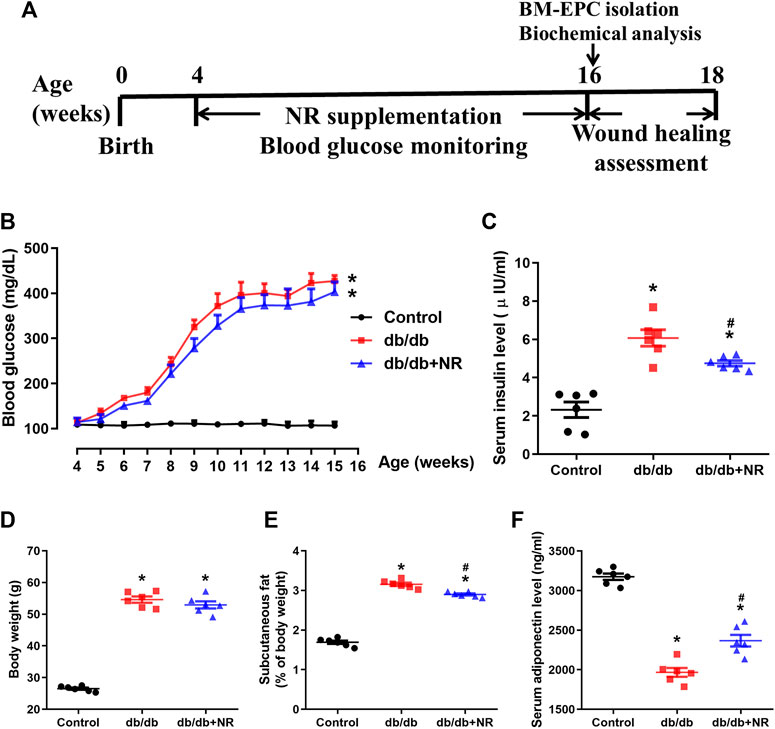
FIGURE 1. Effects of NR supplement on some diabetes mellitus–related symptoms. (A) Experimental schedule. Db/db mice (4 weeks old) were fed with common food or NR-supplied food (400 mg/kg/d) for consecutive 12 weeks, and the blood glucose was monitored every week. After that, each group mice were further divided into 2 cohorts: excisional wound experiment and BM-EPC isolation experiment. Blood glucose (B), serum insulin (C), body weight (D), subcutaneous fat (E), and serum adiponectin (F) of the mice treated with common food or NR-supplied food for 12 weeks. Values are mean ± SEM, (n = 6). *P < 0.05 vs. control; #P < 0.05 vs. db/db. BM-EPC, bone marrow–derived endothelial precursor cell.
Sample Collection
Blood was collected by excising the eyeballs. About 0.6 ml of blood was harvested. One half was used for EPC isolation, and the remaining half was placed at room temperature (RT) for 40 min and at 4°C for 2 h. The samples were then centrifuged at 3,000 ×g for 10 min, and the supernatant was collected and stored at −80°C for index measurement. After blood collection, the subcutaneous fat was carefully dissected and weighed.
Serum Insulin and Adiponectin Measurements
Serum insulin was determined using an ELISA kit from Shibayagi Co., Ltd. (Shibukawa, Japan). Serum concentration of adiponectin was also measured by commercial ELSA kits (adiponectin: AdipoGen, Incheon, Korea). All samples were measured according to the manufacturers’ protocols.
Wound Closure Test
Mice were anesthetized with phenobarbital sodium (60 mg/kg, i.p.). A 6-mm circular wound was made by punch biopsy (Wu et al., 2016; Lei et al., 2017). The wounds were clipped off the full thickness and subcutaneous tissue. The surgery mice were individually caged and placed on heat mats until fully recovered. Digital images of the wound on the dorsum were captured every 2 days until the end of the experiment for all experimental mice. The wound areas were analyzed by tracing the wound margins and calculated using Image-Pro Plus software version 6.0 (Media Cybernetics, Rockville, MD, United States). The closure was expressed as a percentage area of the original wound area.
Evaluation of Wound Angiogenesis
Mice were sacrificed by cervical dislocation after wound assay. The tissues around and under the wound were clipped off by scissors and incubated in 4% paraformaldehyde at RT overnight. Then, the tissues were transferred to 17% sucrose for 24 h at RT and embedded in paraffin for immunochemistry analyses to quantify the wound angiogenesis by CD31 staining (Han et al., 2017b). The fixed tissues were sliced into 5-μm-thick sections and blocked with 5% serum for 3 h. Samples were subsequently incubated with an anti-CD31 antibody (BD Biosciences, San Diego, CA, United States; 1:500, cat. no. 550274) for 1 h at RT and incubated with a secondary antibody (Vector Laboratories Ltd, Peterborough, UK; 1:800, cat. no. BA-9200) for 1 h each. The slides were counterstained with hematoxylin for another 2 min and were then examined under low-power fields (magnification ×100) and high-power fields (magnification ×200), respectively, by a light microscope (Leica, Wetzlar, Germany). A capillary was defined as CD31 positive staining, and the capillary numbers were calculated.
Blood EPCs and BM-EPC Assessment
Blood EPCs and BM-EPC frequencies were assayed by flow cytometry, as described in a previous study (Yu et al., 2016). The peripheral blood or BM was isolated by Ficoll density gradient centrifugation. After the cell layer was extracted and red blood cells were lysed, the cells were stained with FITC-Sca-1 (BD, San Diego, CA, United States; cat. no. 557405) and PE-Flk-1 (BD, San Diego, CA, United States; cat. no. 555308) antibodies to measure the frequency of EPCs (Sca-1+/Flk-1+).
BM-EPC Extraction
BM-EPC isolation was performed as previously described (Han et al., 2017a). Briefly, BM-EPCs were isolated from the femurs and tibias of mice from each group. Cells were maintained in endothelial growth medium-2 (EGM-2; Cambrex Corp, East Rutherford, NJ, United States) with 15% fetal bovine serum and incubated at 37°C with 5% carbon dioxide (CO2). The EGM-2 medium was replaced after 3 days, and cells were allowed to culture for another 4 days. BM-EPCs from different mice were pooled and then separated to a 12-well plate to evaluate the EPC function. In other cellular experiments, mature BM-EPCs were treated with the AMPK inhibitor compound C (10 μM; Sigma, St. Louis, MO, United States) or C75 (40 μg/ml; Sigma). After 24 h of compound C or 2 h of C75 stimulation, cell analysis was performed to evaluate the EPC functions. The doses and incubation time of compound C and C75 were referred to those in previous reports (Kim et al., 2004; Yu et al., 2016).
Measurement of NAD and Vascular Endothelial Growth Factor Concentration
Measurement of NAD concentration was performed according to the method previously described (Wang et al., 2011). The level of NAD+ in the cellular experiment was assayed using a NAD Quantification Kit (BioVision, Mountain View, CA, United States). The level of VEGF in EPCs was also measured with a commercial ELSA kit (R&D System, Minneapolis, MN, United States). All samples were measured according to the manufacturers’ protocols.
Determination of BM-EPC Function
A tube formation assay was performed to evaluate the BM-EPC function as described before (Yu et al., 2016). Briefly, a 96-well plate (Corning, Tewksbury, MA, United States) was coated with 50 μL/well of growth factor–reduced matrix gel (BD Biosciences, San Diego, CA, United States; cat. no. 356231) for 1 h. The cells were then placed on the Matrigel-coated plate with 5 × 105/ml concentration and maintained at 37°C with 5% CO2. After 8 h of incubation, images of the forming tubes were acquired under ×50 magnification using a light microscope. The number of tubes was calculated.
The adhesion ability assay was used to assess the EPC function as previously described (Han et al., 2017a). A total of 5 × 104 BM-EPCs were placed on the mouse vitronectin (1 μg/ml) coated 96-well plate per well. After 2 h incubation at 37°C with 5% CO2, nonadherent cells were softly removed by phosphate-buffered saline (PBS). Then, adherent cells were fixed with 2% paraformaldehyde for 15 min at RT and stained by Hoechst 33258 (10 μg/ml; Beyotime, Shanghai, China; cat. no. C1011). The stained cells (blue color) were observed using a fluorescence microscope (Leica, Wetzlar, Germany) at a magnification of ×400. Each well was counted in 3 random fields.
Small Interfering RNA Transfection
Transfection of siRNA using Lipofectamine 2000 (Invitrogen, Carlsbad, CA, United States) was done as previously described (Wang et al., 2014). The commercial siRNA targeting Sirt1 was purchased from Dharmacon (Lafayette, CO, United States). All steps were strictly followed in accordance with the manufacturer’s instructions. After transfection for 6 h, the medium containing siRNA was replaced. The medium was cultured for another 2 days, and the cells were used for EPC function assays or Western blot analysis.
Western Blot and Immunoprecipitation
For the Western blot assay, the samples were separated by 8% or 10% SDS–PAGE and blotted onto the polyvinylidene difluoride membrane by electrophoretic transfer (Bio-Rad Laboratories, Inc, Hercules, CA, United States). The members were probed with specific antibodies overnight at 4°C and then incubated with secondary antibodies at RT for 2 h. A quantitative analysis of the visualized bands was performed with Scion Image analysis software (Bio-Rad Laboratories). The specific primary antibodies were Sirt1 (Cell Signaling Technology, Danvers, MA, United States; cat. no. 8469S), phosphorylated AMPKα (p-AMPKα; Cell Signaling Technology; cat. no. 50081S), AMPKα (Cell Signaling Technology; cat. no. 5832S), and GAPDH (Sigma; cat. no. G9295).
For the immunoprecipitation assay, cells were lysed with RAPI buffer by adding protease inhibitors (Beyotime, Shanghai, China). Forty microliters of rabbit serum were added to 1 ml of lysates to decrease the nonspecific binding. The protein samples were incubated with protein G agarose for 2 h and centrifuged at 15,000 g for 10 min to obtain supernatant. This supernatant was incubated with peroxisome proliferator–activated receptor γ coactivator (PGC-1α; Sigma; cat. no. ABE868) primary antibody overnight at 4°C. The protein G agarose was used to harvest the protein complex. The immunoprecipitated protein was then blotted on 10% SDS–PAGE for immunoblotting. Acetylated PGC-1a was measured using acetyl-lysine antibody (Abcam, Cambridge, MA, United States; cat. no. ab190479).
Statistical Analyses
Data were expressed as means ± SEM. Comparisons among the three groups were analyzed by one-way analysis of variance (ANOVA) followed by Tukey’s test using GraphPad Prism software (version 6). Comparisons between the two groups were performed using the unpaired Student’s t-test. Data in Figure 2A were analyzed by two-way ANOVA. Statistical significance was set at P less than 0.05.
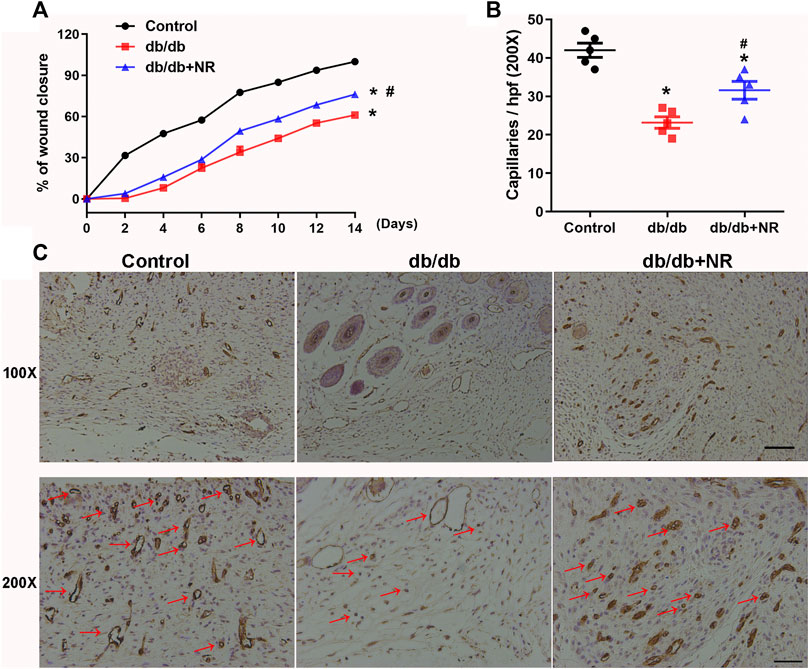
FIGURE 2. Effects of NR supplement on wound closure and wound angiogenesis in db/db mice. (A) Full-thickness skin wounds were made in common food and NR-supplied food fed db/db mice. Quantitative analysis of wound closure at indicated time intervals (B). Immunohistochemical analyses of CD31 in day-14 wounds of mice and quantitative study (C). Red arrows point out CD31-positive capillaries (100×, scale bar, 100 μm; 200×, scale bar, 50 μm). Values are mean ± SEM (n = 5). *P < 0.05 vs. control; #P < 0.05 vs. db/db.
Results
Effect of NR on Blood Glucose, Serum Insulin, Body Weight, and Serum Adiponectin Level in db/db Mice
To investigate the function of NR on wound healing in diabetic mice, the 4-week-old db/db mice were fed with NR-supplemented food for 12 weeks. As shown in Figure 1B, blood glucose was greatly increased in db/db mice compared with the age-matched normal C57BL/6J mice (P < 0.05). However, the NR supplement had little effect on blood glucose in db/db mice (P > 0.05; Figure 1B). Interestingly, the increased serum insulin in db/db mice was greatly reduced after NR treatment (4.75 ± 0.14 μIU/ml vs. 6.07 ± 0.43 μIU/ml, P < 0.05; Figure 1C). We also measured the body weight, subcutaneous fat, and adiponectin of the mice treated with or without NR. As shown in Figures 1D–F, the NR supplement had no significant effect on the body weight of db/db mice, but significantly decreased the subcutaneous fat ratio (2.90 ± 0.07% vs. 3.16 ± 0.10%, P < 0.05; Figure 1E) and increased adiponectin (2367 ± 73.31 ng/ml vs. 1967 ± 56.06 ng/ml, P < 0.05; Figure 1F) of db/db mice. These results suggest that NR supplementation has a minor effect on DM-related symptoms.
NR Accelerated Wound Closure and Angiogenesis in db/db Mice
To investigate the role of NR supplementation in the wound healing process in diabetic mice, circular and full-thickness cutaneous wounds were created on the dorsal skin of mice, and the wound areas were measured every 2 days. As shown in Figure 2A, the healing rate of db/db mice was dramatically slower than that of normal mice; interestingly, NR supplementation significantly accelerated the healing rate of db/db mice (P < 0.05; Figure 2A).
Since the wound healing rate is highly correlated with the extent of angiogenesis, we assessed the vasculature of the wound edges using CD31 staining. As shown in Figures 2B,C, the number of CD31-positive vascular structures in the wounds of db/db mice was markedly less than that of normal mice (23.20 ± 1.50 vs. 42.00 ± 1.84, P < 0.05). However, NR treatment significantly increased the CD31-positive vascular structures (31.60 ± 2.32 vs. 23.20 ± 1.50, P < 0.05). These results suggest that NR supplementation might increase angiogenesis to promote wound healing in db/db diabetic mice.
NR Increased EPC Number and Improved BM-EPC Function in db/db Mice
Previous studies have indicated that impaired angiogenesis in diabetic wounds is closely associated with the EPC number and function (Yu et al., 2016; Han et al., 2017a). Therefore, we isolated BM-EPCs and blood EPCs from db/db mice which were given common food and NR-supplemented food and then detected the NAD concentration, EPC number, tube formation, and cell adhesion function of BM-EPC in vitro. The concentration of NAD in cultured BM-EPCs (day 5 and day 7 in vitro) from db/db mice were remarkably lower than that of NAD in C57BL/6J mice (day 5: 73.50 ± 1.79% vs. 100.00 ± 3.00%, P < 0.05; day 7: 72.64 ± 1.77% vs. 100.00 ± 3.13%, P < 0.05; Figure 3A). However, NR successfully reversed the reduction of NAD concentration in BM-EPCs from db/db mice (day 5: 88.34 ± 1.42% vs. 73.50 ± 1.79%, P < 0.05; day 7: 87.06 ± 2.10% vs. 72.64 ± 1.77%, P < 0.05; Figure 3A). The EPC number in blood and in BM of db/db mice was reduced by 48% and 40%, respectively, compared with the control mice. NR supplementation greatly raised the frequencies of EPCs in blood (P < 0.05; Figure 3B) and BM EPCs (P < 0.05; Figure 3C) of db/db mice. Furthermore, tube formation (11.33 ± 1.28 vs. 29.50 ± 2.19, P < 0.05; Figures 3D,E) and cell adhesion function (41.97 ± 3.25% vs. 99.97 ± 3.28%, P < 0.05; Figures 3F,G) of BM-EPCs from db/db mice were significantly reduced compared with those of BM-EPCs from the control mice. NR supplementation also significantly elevated the tube formation (21.33 ± 1.38 vs. 11.33 ± 1.28, P < 0.05; Figures 3D,E) and adhesion ability (58.68 ± 2.73 vs. 41.97 ± 3.25, P < 0.05; Figures 3F,G) of BM-EPCs from db/db mice. These results suggest that NR supplementation might improve the BM-EPC function to promote angiogenesis, leading to accelerated wound healing.
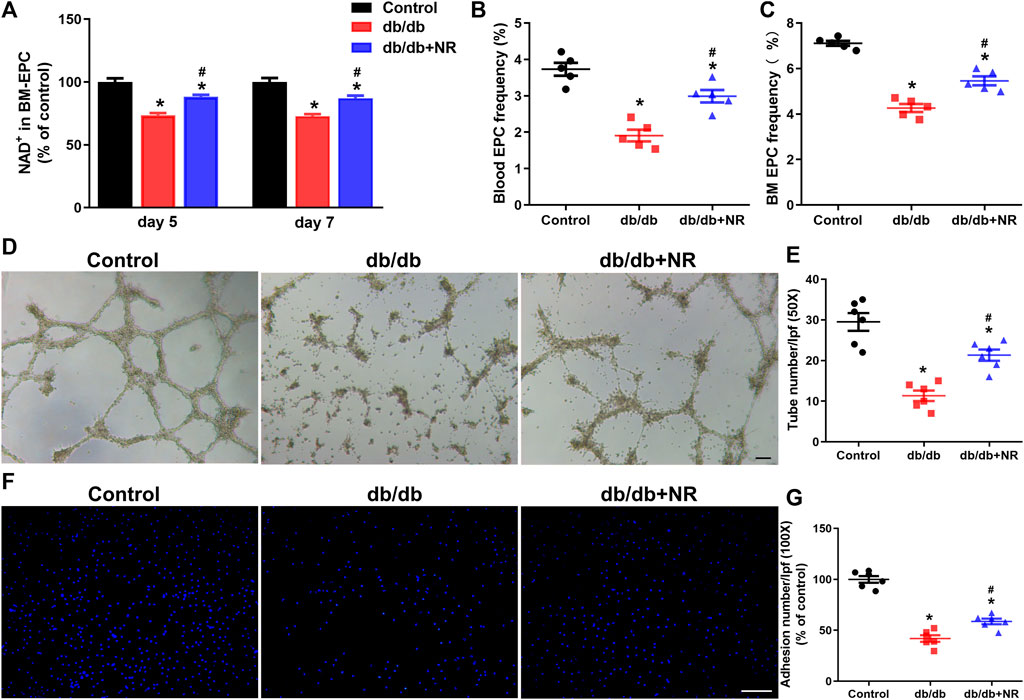
FIGURE 3. Effects of NR supplement on EPC number and BM-EPC function in db/db mice. (A) NAD concentrations in cultured BM-EPCs (day 5 and day 7 in vitro) isolated from mice. Blood EPC (B) and BM EPC (C) frequency in normal control and db/db mice. Tube formation (D,E) and cell adhesion (F,G) of BM EPC isolated from normal control and db/db mice. D: 50×; scale bar, 100μm; F: 100×; scale bar, 100 μm. Values are mean ± SEM (A, B, C: n = 5; D, E, F, G: n = 6). *P < 0.05 vs. Control; #P < 0.05 vs. db/db. BM, bone marrow; BM-EPC, bone marrow–derived endothelial precursor cell.
NR Enhanced SIRT1/AMPK Expression in BM-EPCs From db/db Mice
To confirm the potential mechanisms responsible for the therapeutic effect of NR supplementation on wound healing and angiogenesis, we detected the levels of Sirt1/AMPK, acetylated PGC-1α, and VEGF in BM-EPCs. As shown in the results of Western blot analysis, the downregulated Sirt1 and p-AMPK/AMPK expressions can be observed in BM-EPCs from db/db mice compared with those from the control mice (P < 0.05; Figures 4A,B). However, NR treatment significantly upregulated Sirt1 and p-AMPK/AMPK expressions in BM-EPCs compared with untreated cells from db/db mice (P < 0.05; Figures 4A,B). As PGC-1α deacetylation represents the activity of Sirt1, we performed immunoprecipitation WB experiment to detect acetylated PGC-1α. As shown in Figure 4C, the level of acetylated PGC-1α was obviously enhanced in BM-EPCs from db/db mice, which was inhibited after NR treatment, demonstrating NR enhances Sirt1 expression to deacetylate PGC-1α. Next, the level of VEGF in BM-EPCs was further evaluated. The results showed that VEGF concentration was significantly reduced in BM-EPCs from db/db mice compared with those from the control mice (415.9 ± 9.7 pg/ml vs. 638.3 ± 20.7 pg/ml, P < 0.05; Supplementary Figure S1A). Moreover, NR also increased the level of VEGF when compared to the cells from db/db mice (471.5 ± 15.4 pg/ml vs. 415.9 ± 9.7 pg/ml, P < 0.05; Supplementary Figure S1A). These results suggest that NR supplementation might promote BM-EPC function through increasing expression of Sirt1, deacetylated PGC-1α, p-AMPK/AMPK, and VEGF.
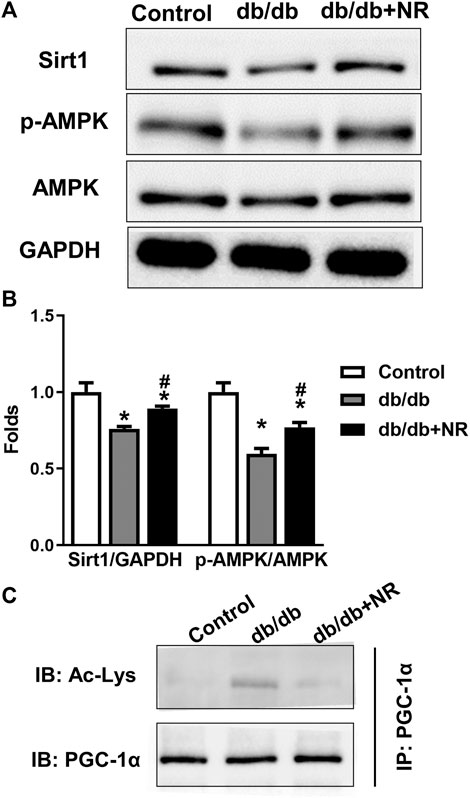
FIGURE 4. Effect of NR supplement on the expression of Sirt1/AMPK in BM-EPCs. (A) Western blot of Sirt1/AMPK in BM-EPCs isolated from normal control and diabetic mice. (B) Quantitative analysis of Sirt1 and p-AMPK expression taken as in (A). (C) The level of acetylated PGC-1α was measured. Values are mean ± SEM, (n = 3). *P < 0.05 vs. Control; #P < 0.05 vs. db/db.
Inhibition of Sirt1 Abolished the Protective Effects of NR Repletion on BM-EPC Function in db/db Mice
We next investigated how NR regulates Sirt1-mediated EPC function in diabetic mice. SiRNA was used to silence Sirt1 in BM-EPCs (Figure 5A). Sirt1 expression was significantly decreased using siRNA-mediated RNA interference in BM-EPCs (Figures 5B,C). Silencing of Sirt1 abolished the NR-enhanced tube formation (P < 0.05; Figures 5D,F) and adhesion abilities (P < 0.05; Figures 5E,G) in diabetic EPCs. Consistently, siRNA–Sirt1 treatment effectively inhibited NR-upregulated p-AMPK/AMPK and increased NR-inhibited acetylated PGC-1α (P < 0.05; Figures 5H–J). However, silencing of Sirt1 did not affect NR-increased VEGF in BM-EPCs of diabetic mice (P > 0.05; Supplementary Figure S1B). These results suggest that NR supplementation promotes Sirt1 expression to increase p-AMPK/AMPK and decrease acetylated PGC-1α expressions in BM-EPCs.
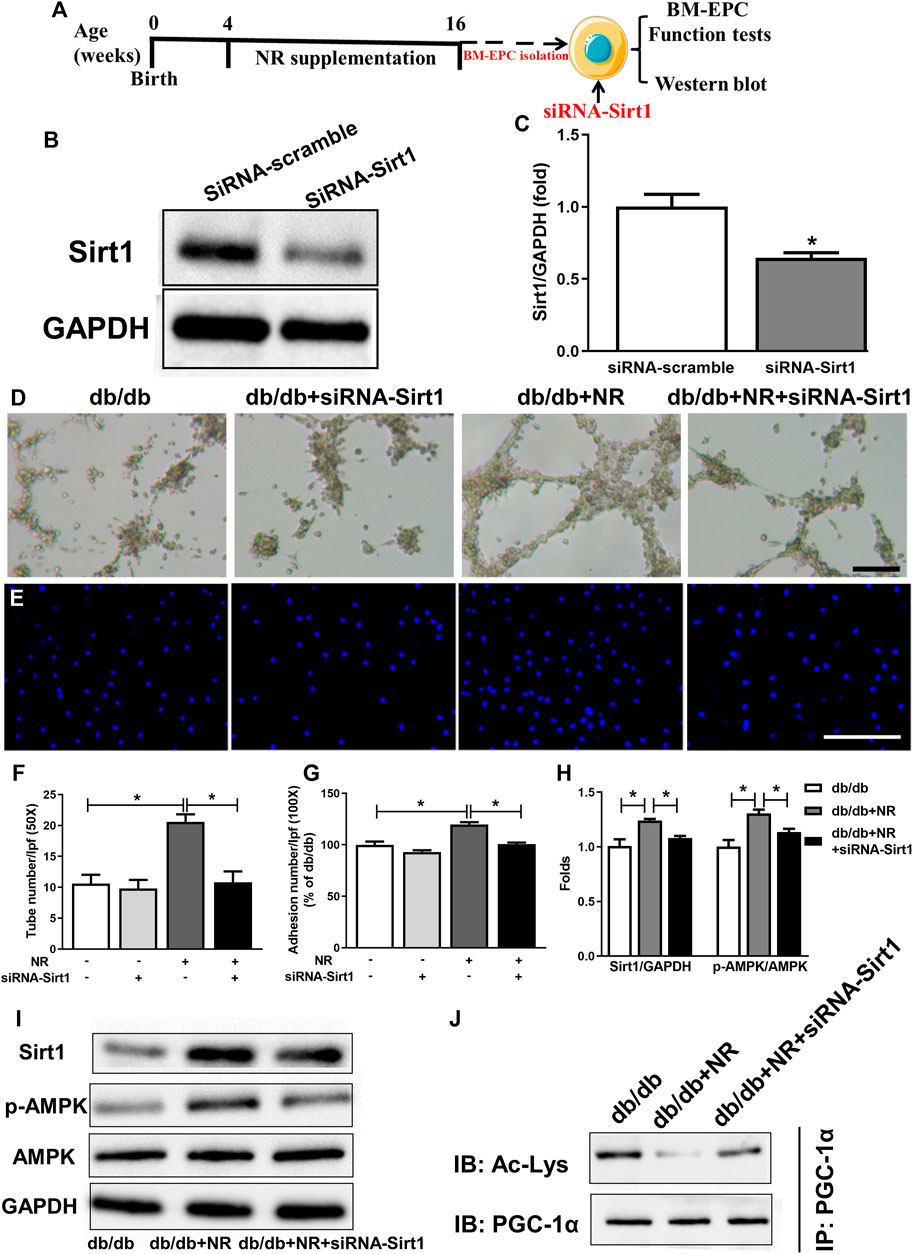
FIGURE 5. Inhibition of Sirt1 with siRNA-Sirt1 abolished the protective effects of NR repletion on BM-EPC function in db/db mice. (A) Experimental schedule. Db/db mice received the NR supplied food for 12 weeks, and then, BM-EPCs were isolated and transfected with siRNA targeting Sirt1. (B,C) Transfection of siRNA–Sirt1 obviously inhibited Sirt1 expression in BM-EPCs from db/db mice. *P < 0.05 vs. siRNA-Sirt1. Tube formation (D) and adhesion abilities of BM-EPCs (E) were measured. Quantitative analysis of tube number (F) and adhesion number (G). (H) Quantitative analysis of Sirt1, and p-AMPK expression was taken as in (I). (I) Western blot of Sirt1/AMPK in BM-EPCs treated with NR in the presence or absence of siRNA–Sirt1. (J) Representative immunoblot images of acetylated PGC-1α. D: 50×; scale bar, 100 μm; E: 100×; scale bar, 100 μm. Values are mean ± SEM, (B, C, H, I, J: n = 3; D–G: n = 5). *P < 0.05. BM-EPC, bone marrow–derived endothelial precursor cell; NS, no significance.
Inhibition of AMPK Prevented the Effects of NR on BM-EPC Function in db/db Mice
To further investigate whether AMPK signal is needed for NR-regulated BM-EPC function, two important antagonists of AMPK (compound C and C75, Jiang et al., 2018) were used to analyze the NR-mediated BM-EPC function. Inhibition of AMPK by compound C or C75 significantly inhibited the NR-increased tube formation (P < 0.05; Figures 6B,D; Supplementary Figures S2B,D) and adhesion (P < 0.05; Figures 6C,E; Supplementary Figures S2C,E). These results suggest that AMPK signal is needed for the NR-regulated BM-EPC function. Furthermore, inhibition of AMPK by compound C had no significant effects on NR-induced Sirt1 expression (P > 0.05; Figures 6B–D), demonstrating that AMPK is downstream of the Sirt1 signaling pathway. Taken together, all these data suggest that NR enhances EPC function through mediating the Sirt1/AMPK pathway.
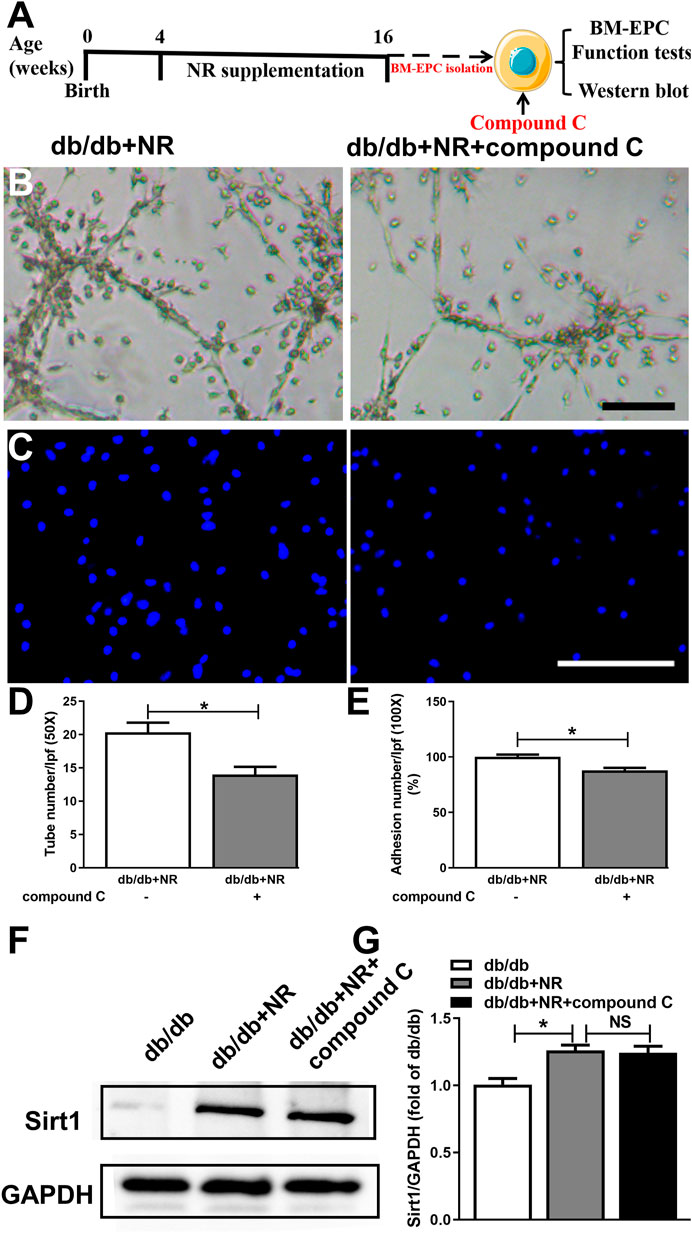
FIGURE 6. Inhibition of AMPK with compound C abolished the protective effects of NR supplement on BM-EPC function in db/db mice. (A) Illustration of experimental protocol. Db/db mice treated with NR for 12 weeks, and then, BM-EPCs were isolated and stimulated with compound C (10 μM). Tube formation (B) and adhesion (C) assay in BM-EPCs treated with NR in the presence or absence of compound C. Quantitated analysis of tube number (D) and adhesion number (E). (F–G) Compound C has no significant effect on Sirt1 expression in BM-EPCs of db/db mice treated with NR. B: 50×; scale bar, 100 μm; C: 100×; scale bar, 100 μm. Values are mean ± SEM (n = 3). *P < 0.05. BM-EPC, bone marrow–derived endothelial precursor cell; NS, no significance.
Discussion
In the present study, we have shown that 1) db/db mice administered with NR exhibited accelerated wound healing and alleviated angiogenesis accompanied by an increased concentration of NAD+, 2) these improvements are closely associated with enhanced an EPC number and function in db/db mice, and 3) the beneficial effects of NR were mediated in part through the activation of Sirt1/AMPK. Taken together, we show novel evidence for the therapeutic effect of NR on diabetic wound healing by improvement of EPC function via activating the Sirt1/AMPK signaling pathway in db/db mice.
Previous reports indicated that db/db mice with a C57BLKS/J background, a mutation of the leptin receptor gene, widely served as a mouse model for type 2 DM (T2DM) (Zhong et al., 2013). To study the effect of NR on diabetic wounds, 4-week-old db/db mice were used because they could rapidly develop hyperglycemia, dyslipidemia, and insulin resistance from 6 weeks of age, and DM-related complications commonly occur after 10 weeks of age (Zhong et al., 2013; Kang et al., 2014). In this study, we examined blood glucose and body mass in db/db mice. They showed slight increases in blood glucose and body weight after NR supplementation. Adiponectin is a novel adipokine, which was expressed exclusively in adipose tissue. It has been reported that hypoadiponectinemia was associated with insulin resistance, and obesity may reduce plasma adiponectin levels (Maebuchi et al., 2003; Matsubara, 2004). We found that 400 mg/kg NR noticeably prevented the accumulation of subcutaneous fat and serum insulin, and significantly upregulated the serum adiponectin levels; however, NR was unable to significantly decrease blood glucose and body weight. This evidence supports the fact that NR might be effective for improving some DM-related symptoms.
Refractory wound healing in diabetes as a severe and chronic DM-related complication imposes a huge burden on patients. A large number of the population are victims of untimely and inefficient wound closure, especially patients with diabetes, owing to impaired angiogenesis (Azizi et al., 2019). Currently, there is no satisfactory treatment for diabetic wounds. Niacinamide, known as a form of NR, has been used as an annexing agent of skin-care products for many years. It seems to improve the nutritional status of skin cells (Carraway, 2004). A previous report suggested that niacinamide has the potential to remodel fibroblast matrix deposition and advance wound care, partly through the enhancement of cellular migration and proliferation (Wessels et al., 2014). Moreover, a clinical research found that a vitamin B complex including vitamin B3 showed positive effects on wound recovery after periodontal surgery (Neiva et al., 2005). Relevant to these findings, administration of NR did accelerate wound healing in db/db mice. NR also increased capillary densities which were indicated by CD31 staining at the wound site of db/db mice. However, further studies are needed to define the underlying mechanisms of how NR bolsters wound recovery and angiogenesis.
It is well known that EPCs participate in new blood vessel formation and tissue repair as precursors of endothelial cells (Zigdon-Giladi et al., 2015). Emerging data have indicated that recruitment of EPCs into ischemic tissues is one of the critical events in neovascularization (Weng et al., 2018). Wang et al. (2016) have reported that depletion of the NAD pool links diabetic patients to impairment of EPC mobilization. Supplementation of the NAD pool with nicotinamide contributes to increased numbers of circulating blood EPCs in diabetic patients (Wang et al., 2016). In addition, dietary vitamin intake such as vitamin B1 and vitamin D is dramatically associated with more circulating EPCs and better flow-mediated dilation (FMD) in T2DM patients than in controls (Wong et al., 2008; Gurses et al., 2014). Overall, enhancing the NAD pool may be a powerful factor for enhancing EPC activation under diabetic conditions. Notably, replenishing of NR, a vitamin B3 analog, has beneficial effects on EPC numbers in blood and BM with ischemia stroke models (Wang et al., 2011). Our present study indicates that the NAD concentration was downregulated in BM-EPCs of db/db mice. An upregulated NAD pool by NR treatment not only elevated the EPC numbers in circulating blood and BM but also enhanced EPC bioactivities, including capacity for tubule-like formation and adhesion. Thus, further research is required to understand the molecular mechanism underlying EPC improvements by NR in diabetic conditions.
Sirt1 as a NAD-dependent deacetylase plays a crucial role in mediating intracellular NAD levels for maintaining energy balance (Wang et al., 2014). PGC-1α is a transcriptional coactivator and has the only target of Sirt1 (Liao et al., 2019). A previous study favored the protective effect of Sirt1 and acetylated PGC-1α on the biological activity of EPCs (Wang et al., 2014). Our results demonstrate that a NR-replenished NAD pool was able to modulate Sirt1 and acetylated PGC-1α expressions in BM-EPCs of diabetic mice. As is well known, AMPK is recognized as a sensor of energy regulation to achieve glucose homeostasis and is widely expressed in all tissues (Liao et al., 2019). In a streptozotocin-induced mice model, AMPK activation was significantly decreased in BM-EPCs (Yu et al., 2016). Furthermore, an impaired EPC function is closely related to the inactivation of the AMPK pathway (Yu et al., 2016). It has been reported that Sirt1 upregulates the expression of phosphorylated AMPK under exposure to diabetic conditions (Karbasforooshan and Karimi, 2018). The Sirt1/AMPK pathway can participate in hepatic steatosis in obese mice (Liou et al., 2020). Our results indicate that treatment of the NAD substrate with NR increased the expression of phosphorylated AMPK in diabetic EPCs. More importantly, inhibition of Sirt1 signaling abolished the improvement of EPC function accompanied by upregulated phosphorylated AMPK by NR in BM-EPCs. Interestingly, AMPK inhibitors (compound C and C75) could partly reverse the beneficial effects of NR on EPC functions but had no effect on Sirt1 expression. Therefore, we confirmed that NR could enhance the EPC function by regulation of the Sirt1/AMPK pathway to achieve acceleration of wound healing in db/db mice.
VEGF is involved in the progression of wound repair and is recognized as important solely for mediating angiogenesis. Generally, downregulated VEGF levels in diabetic wound tissue are associated with impaired healing states, and enhancing pharmacologic VEGF may be a useful target (Galiano et al., 2004). Moreover, VEGF promotes EPC homing and repair of injured endothelial cells (Odent Grigorescu et al., 2017). We found that supplementing the NAD substrate with NR improved the disrupted expressions of VEGF in diabetic mice. However, inhibition of Sirt1 failed to reverse the enhanced expression of VEGF by NR treatment. In this regard, our results suggest that there is no direct association between increased VEGF concentration and the Sirt1/AMPK pathway in EPCs.
Wound healing is a sophisticated process responding to tissue damage, which is associated with numbers of interaction of various cell types, cytokines, growth factors, and other molecules (Jeffcoate and Harding, 2003; Shen et al., 2021). Therefore, improvement of only one aspect is not enough to dramatically promote wound healing. In this study, we only show that NR supplementation could regulate the EPC function to promote angiogenesis during wound healing. Future studies will be conducted to evaluate the NR function with different doses of NR.
In summary, we have shown evidence that increasing the NAD pool by NR accelerates wound healing and promotes angiogenesis in a diabetic mice model, which is possibly associated with improvement of the impaired EPC function via regulating the Sirt1/AMPK pathway. This report implies new applications for NR supplementation in the daily diet of diabetic individuals to achieve early prevention of diabetic complications. Further research aimed at defining the mechanisms of NR on diabetic complications is required.
Data Availability Statement
The raw data supporting the conclusion of this article will be made available by the authors, without undue reservation.
Ethics Statement
The animal study was reviewed and approved by the Ethics Committee of Laboratory Animal Care and Welfare, Hangzhou Medical College.
Author Contributions
YW and GX contributed to this article with conception, literature review, and analysis; ZW and XB contributed to this article with drafting and editing the manuscript; JH and SS contributed to this study with reviewing the manuscript, supervision, and funding acquisition. All authors contributed to the article and approved the submitted version.
Funding
This study was supported by the National Natural Science Foundation of China (Grant No. 81772363 and 51802221), the Shanghai Sailing Program (Grant No. 21YF1458200), the Youth Initial funding of Naval Medical University (Grant No. 2018QN13), and the Special Fund of Changzheng Hospital (Grant No. 2019CZJS104).
Conflict of Interest
The authors declare that the research was conducted in the absence of any commercial or financial relationships that could be construed as a potential conflict of interest.
Supplementary Material
The Supplementary Material for this article can be found online at: https://www.frontiersin.org/articles/10.3389/fphar.2021.671563/full#supplementary-material
References
American Diabetes Association (2018). National Diabetes Statistics Report. Diabetes Care 2018, dci180007. doi:10.2337/dci18-0007
Azizi, S., Kheirandish, R., and Salarpoor, M. (2019). Topical Effect of Allogenous Serum Rich in Growth Factors (SRGF) on Diabetic Skin Wound in Rat. Transfus. Apher. Sci. 58 (4), 498–504. doi:10.1016/j.transci.2019.05.014
Cantó, C., Houtkooper, R. H., Pirinen, E., Youn, D. Y., Oosterveer, M. H., Cen, Y., et al. (2012). The NAD+ Precursor Nicotinamide Riboside Enhances Oxidative Metabolism and Protects against High-Fat Diet-Induced Obesity. Cel Metab. 15 (6), 838–847. doi:10.1016/j.cmet.2012.04.022
Carraway, J. (2004). Using Aldara, Copper Peptide, and Niacinamide for Skin Care. Aesthet. Surg. J. 24 (1), 83–84. doi:10.1016/j.asj.2003.10.009
Catrina, S.-B., and Zheng, X. (2016). Disturbed Hypoxic Responses as a Pathogenic Mechanism of Diabetic Foot Ulcers. Diabetes Metab. Res. Rev. 32, 179–185. doi:10.1002/dmrr.2742
Forsythe, R. O., and Hinchliffe, R. J. (2016). Assessment of Foot Perfusion in Patients with a Diabetic Foot Ulcer. Diabetes Metab. Res. Rev. 32, 232–238. doi:10.1002/dmrr.2756
Francini, F., Schinella, G. R., and Ríos, J.-L. (2019). Activation of AMPK by Medicinal Plants and Natural Products: its Role in Type 2 Diabetes Mellitus. Mini Rev Med Chem. 19 (11), 880–901. doi:10.2174/1389557519666181128120726
Galiano, R. D., Tepper, O. M., Pelo, C. R., Bhatt, K. A., Callaghan, M., Bastidas, N., et al. (2004). Topical Vascular Endothelial Growth Factor Accelerates Diabetic Wound Healing through Increased Angiogenesis and by Mobilizing and Recruiting Bone Marrow-Derived Cells. Am. J. Pathol. 164 (6), 1935–1947. doi:10.1016/S0002-9440(10)63754-6
Ginter, E., and Simko, V. (2012). Type 2 Diabetes Mellitus, Pandemic in 21st Century. Adv. Exp. Med. Biol. 771, 42–50. doi:10.1007/978-1-4614-5441-0_6
Gurses, K. M., Tokgozoglu, L., Yalcin, M. U., Kocyigit, D., Dural, M., Canpinar, H., et al. (2014). Markers of Subclinical Atherosclerosis in Premenopausal Women with Vitamin D Deficiency and Effect of Vitamin D Replacement. Atherosclerosis 237 (2), 784–789. doi:10.1016/j.atherosclerosis.2014.10.096
Han, X., Deng, Y., Yu, J., Sun, Y., Ren, G., Cai, J., et al. (2017a). Acarbose Accelerates Wound Healing via Akt/eNOS Signaling indb/dbMice. Oxidative Med. Cell Longevity 2017, 1–11. doi:10.1155/2017/7809581
Han, X., Tao, Y., Deng, Y., Yu, J., Sun, Y., and Jiang, G. (2017b). Metformin Accelerates Wound Healing in Type 2 Diabetic Db/db Mice. Mol. Med. Rep. 16 (6), 8691–8698. doi:10.3892/mmr.2017.7707
Hugo, W., Zaretsky, J. M., Sun, L., Song, C., Moreno, B. H., Hu-Lieskovan, S., et al. (2016). Genomic and Transcriptomic Features of Response to Anti-PD-1 Therapy in Metastatic Melanoma. Cell 165 (1), 35–44. doi:10.1016/j.cell.2016.02.065
Ishida, Y., Kimura, A., Kuninaka, Y., Inui, M., Matsushima, K., Mukaida, N., et al. (2012). Pivotal Role of the CCL5/CCR5 Interaction for Recruitment of Endothelial Progenitor Cells in Mouse Wound Healing. J. Clin. Invest. 122 (2), 711–721. doi:10.1172/JCI43027
Jeffcoate, W. J., and Harding, K. G. (2003). Diabetic Foot Ulcers. The Lancet 361 (9368), 1545–1551. doi:10.1016/S0140-6736(03)13169-8
Jiang, S., Li, T., Ji, T., Yi, W., Yang, Z., Wang, S., et al. (2018). AMPK: Potential Therapeutic Target for Ischemic Stroke. Theranostics 8 (16), 4535–4551. doi:10.7150/thno.25674
Kang, S., Lee, J., Lee, E., Jung, D., Song, C., Park, S., et al. (2014). Fermentation with Aquilariae Lignum Enhances the Anti-diabetic Activity of Green Tea in Type II Diabetic Db/db Mouse. Nutrients 6 (9), 3536–3571. doi:10.3390/nu6093536
Karbasforooshan, H., and Karimi, G. (2018). The Role of SIRT1 in Diabetic Retinopathy. Biomed. Pharmacother. 97, 190–194. doi:10.1016/j.biopha.2017.10.075
Kim, E.-K., Miller, I., Aja, S., Landree, L. E., Pinn, M., McFadden, J., et al. (2004). C75, a Fatty Acid Synthase Inhibitor, Reduces Food Intake via Hypothalamic AMP-Activated Protein Kinase. J. Biol. Chem. 279 (19), 19970–19976. doi:10.1074/jbc.M402165200
Lee, H. J., and Yang, S. J. (2019). Nicotinamide Riboside Regulates Inflammation and Mitochondrial Markers in AML12 Hepatocytes. Nutr. Res. Pract. 13 (1), 3–10. doi:10.4162/nrp.2019.13.1.3
Lei, H., Wang, Y., Zhang, T., Chang, L., Wu, Y., and Lai, Y. (2017). TLR3 Activation Induces S100A7 to Regulate Keratinocyte Differentiation after Skin Injury. Sci. China Life Sci. 60 (2), 158–167. doi:10.1007/s11427-016-0027-2
Li, H., Liu, Q., Wang, N., Xu, Y., Kang, L., Ren, Y., et al. (2018). Transplantation of Endothelial Progenitor Cells Overexpressing miR-126-3p Improves Heart Function in Ischemic Cardiomyopathy. Circ. J. 82 (9), 2332–2341. doi:10.1253/circj.CJ-17-1251
Liao, Z., Zhang, J., Wang, J., Yan, T., Xu, F., Wu, B., et al. (2019). The Anti-nephritic Activity of a Polysaccharide from Okra (Abelmoschus Esculentus (L.) Moench) via Modulation of AMPK-Sirt1-PGC-1α Signaling axis Mediated Anti-oxidative in Type 2 Diabetes Model Mice. Int. J. Biol. Macromolecules 140, 568–576. doi:10.1016/j.ijbiomac.2019.08.149
Liou, C. J., Wu, S. J., Shen, S. C., Chen, L. C., Chen, Y. L., and Huang, W. C. (2020). Phloretin Ameliorates Hepatic Steatosis through Regulation of Lipogenesis and Sirt1/AMPK Signaling in Obese Mice. Cell Biosci 10, 114. doi:10.1186/s13578-202-00477-1
Maebuchi, M., Machidori, M., Urade, R., Ogawa, T., and Moriyama, T. (2003). Low Resistin Levels in Adipose Tissues and Serum in High-Fat Fed Mice and Genetically Obese Mice: Development of an ELISA System for Quantification of Resistin. Arch. Biochem. Biophys. 416 (2), 164–170. doi:10.1016/s0003-9861(03)00279-0
Matsubara, M. (2004). Plasma Adiponectin Decrease in Women with Nonalcoholic Fatty Liver. Endocr. J. 51 (6), 587–593. doi:10.1507/endocrj.51.587
Neiva, R. F., Al-Shammari, K., Nociti, F. H., Soehren, S., and Wang, H.-L. (2005). Effects of Vitamin-B Complex Supplementation on Periodontal Wound Healing. J. Periodontol. 76 (7), 1084–1091. doi:10.1902/jop.2005.76.7.1084
Odent Grigorescu, G., Rosca, A.-M., Preda, M. B., Tutuianu, R., Simionescu, M., and Burlacu, A. (2017). Synergic Effects of VEGF-A and SDF-1 on the Angiogenic Properties of Endothelial Progenitor Cells. J. Tissue Eng. Regen. Med. 11 (11), 3241–3252. doi:10.1002/term.2233
Shen, X., Shen, X., Li, B., Zhu, W., Fu, Y., Xu, R., et al. (2021). Abnormal Macrophage Polarization Impedes the Healing of Diabetes-Associated Tooth Sockets. Bone 143, 115618. doi:10.1016/j.bone.2020.115618
Sørensen, M., Arneberg, F., Line, T. M., and Berg, T. J. (2016). Cost of Diabetes in Norway 2011. Diabetes Res. Clin. Pract. 122, 124–132. doi:10.1016/j.diabres.2016.10.012
Tiao, M.-M., Lin, Y.-J., Yu, H.-R., Sheen, J.-M., Lin, I.-C., Lai, Y.-J., et al. (2018). Resveratrol Ameliorates Maternal and Post-weaning High-Fat Diet-Induced Nonalcoholic Fatty Liver Disease via Renin-Angiotensin System. Lipids Health Dis. 17 (1), 178. doi:10.1186/s12944-018-0824-3
Trammell, S. A. J., Weidemann, B. J., Chadda, A., Yorek, M. S., Holmes, A., Coppey, L. J., et al. (2016). Nicotinamide Riboside Opposes Type 2 Diabetes and Neuropathy in Mice. Sci. Rep. 6, 26933. doi:10.1038/srep26933
Wang, P., Du, H., Zhou, C.-C., Song, J., Liu, X., Cao, X., et al. (2014). Intracellular NAMPT-NAD+-SIRT1 Cascade Improves Post-ischaemic Vascular Repair by Modulating Notch Signalling in Endothelial Progenitors. Cardiovasc. Res. 104 (3), 477–488. doi:10.1093/cvr/cvu220
Wang, P., Xu, T.-Y., Guan, Y.-F., Tian, W.-W., Viollet, B., Rui, Y.-C., et al. (2011). Nicotinamide Phosphoribosyltransferase Protects against Ischemic Stroke through SIRT1-dependent Adenosine Monophosphate-Activated Kinase Pathway. Ann. Neurol. 69 (2), 360–374. doi:10.1002/ana.22236
Wang, P., Yang, X., Zhang, Z., Song, J., Guan, Y.-F., Zou, D.-J., et al. (2016). Depletion of NAD Pool Contributes to Impairment of Endothelial Progenitor Cell Mobilization in Diabetes. Metabolism 65 (6), 852–862. doi:10.1016/j.metabol10.1016/j.metabol.2016.03.006
Weng, Z., Wang, C., Zhang, C., Xu, J., Chai, Y., Jia, Y., et al. (2018). All-trans Retinoic Acid Improves the Viability of Ischemic Skin Flaps in Diabetic Rat Models. Diabetes Res. Clin. Pract. 142, 385–392. doi:10.1016/j.diabres.2018.06.019
Wessels, Q., Pretorius, E., Smith, C. M., and Nel, H. (2014). The Potential of a Niacinamide Dominated Cosmeceutical Formulation on Fibroblast Activity and Wound Healing In Vitro. Int. Wound J. 11 (2), 152–158. doi:10.1111/j.1742-481x.2012.01052.x
Wils, J., Favre, J., and Bellien, J. (2017). Modulating Putative Endothelial Progenitor Cells for the Treatment of Endothelial Dysfunction and Cardiovascular Complications in Diabetes. Pharmacol. Ther. 170, 98–115. doi:10.1016/j.pharmthera.2016.10.014
Wong, C.-Y., Qiuwaxi, J., Chen, H., Li, S.-W., Chan, H.-T., Tam, S., et al. (2008). Daily Intake of Thiamine Correlates with the Circulating Level of Endothelial Progenitor Cells and the Endothelial Function in Patients with Type II Diabetes. Mol. Nutr. Food Res. 52 (12), 1421–1427. doi:10.1002/mnfr.200800056
Wu, Y., Quan, Y., Liu, Y., Liu, K., Li, H., Jiang, Z., et al. (2016). Hyperglycaemia Inhibits REG3A Expression to Exacerbate TLR3-Mediated Skin Inflammation in Diabetes. Nat. Commun. 7, 13393. doi:10.1038/ncomms13393
Wukich, D. K., Sadoskas, D., Vaudreuil, N. J., and Fourman, M. (2017). Comparison of Diabetic Charcot Patients with and without Foot Wounds. Foot Ankle Int. 38 (2), 140–148. doi:10.1177/1071100716673985
Xie, X., Gao, Y., Zeng, M., Wang, Y., Wei, T.-F., Lu, Y.-B., et al. (2019). Nicotinamide Ribose Ameliorates Cognitive Impairment of Aged and Alzheimer's Disease Model Mice. Metab. Brain Dis. 34 (1), 353–366. doi:10.1007/s11011-018-0346-8
Yu, J.-W., Deng, Y.-P., Han, X., Ren, G.-F., Cai, J., and Jiang, G.-J. (2016). Metformin Improves the Angiogenic Functions of Endothelial Progenitor Cells via Activating AMPK/eNOS Pathway in Diabetic Mice. Cardiovasc. Diabetol. 15, 88. doi:10.1186/s12933-016-0408-3
Zhao, Y., Song, J., Bi, X., Gao, J., Shen, Z., Zhu, J., et al. (2018). Thymosin β4 Promotes Endothelial Progenitor Cell Angiogenesis via a Vascular Endothelial Growth Factor-dependent M-echanism. Mol. Med. Rep. 18 (2), 2314–2320. doi:10.3892/mmr.2018.9199
Zhong, X., Chung, A. C. K., Chen, H. Y., Dong, Y., Meng, X. M., Li, R., et al. (2013). miR-21 Is a Key Therapeutic Target for Renal Injury in a Mouse Model of Type 2 Diabetes. Diabetologia 56 (3), 663–674. doi:10.1007/s00125-012-2804-x
Zhou, C.-C., Yang, X., Hua, X., Liu, J., Fan, M.-B., Li, G.-Q., et al. (2016). Hepatic NAD+deficiency as a Therapeutic Target for Non-alcoholic Fatty Liver Disease in Ageing. Br. J. Pharmacol. 173 (15), 2352–2368. doi:10.1111/bph.13513
Keywords: diabetes mellitus, endothelial precursor cells, nicotinamide riboside, wound healing, sirtuin 1, adenosine monophosphate–activated protein kinase
Citation: Wang Z-h, Bao X-g, Hu J-j, Shen S-b, Xu G-h and Wu Y-l (2021) Nicotinamide Riboside Enhances Endothelial Precursor Cell Function to Promote Refractory Wound Healing Through Mediating the Sirt1/AMPK Pathway. Front. Pharmacol. 12:671563. doi: 10.3389/fphar.2021.671563
Received: 24 February 2021; Accepted: 22 April 2021;
Published: 12 May 2021.
Edited by:
Beatriz Suarez-Alvarez, Central University Hospital of Asturias, SpainReviewed by:
Maria Monsalve, Autonomous University of Madrid, SpainKarima Ait-Aissa, The University of Iowa, United States
Copyright © 2021 Wang, Bao, Hu, Shen, Xu and Wu. This is an open-access article distributed under the terms of the Creative Commons Attribution License (CC BY). The use, distribution or reproduction in other forums is permitted, provided the original author(s) and the copyright owner(s) are credited and that the original publication in this journal is cited, in accordance with accepted academic practice. No use, distribution or reproduction is permitted which does not comply with these terms.
*Correspondence: Ye‐lin Wu, c2tfd3V5ZWxpbkB0b25namkuZWR1LmNu; Guo‐hua Xu, eHVndW9odWFtYWlsQHNtbXUuZWR1LmNu
†These authors have contributed equally to this work