- 1Unit of Cardiac Sciences, Department of Medicine, Campus Bio-Medico University of Rome, Rome, Italy
- 2Unit of Endocrinology and Diabetes, Department of Medicine, Campus Bio-Medico University of Rome, Rome, Italy
- 3Department of Experimental Medicine, Section of Medical Pathophysiology, Food Science and Endocrinology, Sapienza University of Rome, Rome, Italy
- 4Department of Experimental Medicine, Sapienza University of Rome, Rome, Italy
In type 2 diabetes, anti-thrombotic management is challenging, and current anti-platelet agents have demonstrated reduced efficacy. Old and new anti-diabetic drugs exhibited—besides lowering blood glucose levels—direct and indirect effects on platelet function and on thrombotic milieu, eventually conditioning cardiovascular outcomes. The present review summarizes existing evidence on the effects of glucose-lowering agents on platelet properties, addressing pre-clinical and clinical research, as well as drug–drug interactions with anti-platelet agents. We aimed at expanding clinicians’ understanding by highlighting new opportunities for an optimal management of patients with diabetes and cardiovascular disease. We suggest how an improvement of the thrombotic risk in this large population of patients may be achieved by a careful and tailored combination of anti-diabetic and anti-platelet therapies.
Introduction
Type 2 Diabetes (T2D) represents a critical risk factor for cardiovascular disease (CVD). The incidence of acute thrombotic events such as acute myocardial infarction (MI) and stroke is significantly greater in diabetic patients compared to non-diabetics (Tancredi et al., 2015; Cavender et al., 2015; Nusca et al., 2018). Similarly, T2D is associated with worse prognosis following MI, increasing the risk of heart failure (HF) and mortality on long-term follow-up (Murcia et al., 2004).
Platelet dysfunction plays a central role in building up the cardiovascular risk of diabetic patients, since T2D is characterized by an enhanced thrombotic state related to endothelial dysfunction, activation of the coagulation cascade and increased platelet reactivity (Murcia et al., 2004; Gæde et al., 2003; Gresele et al., 2010; Nusca et al., 2019).
The pathophysiology underlying such abnormal platelet response has been largely investigated. Platelets from diabetic patients show high oxidative stress levels due to increased production of reactive oxygen species (ROS), partly mediated by activation of NADPH oxidase 2, in turn leading to amplified aggregation (Cangemi et al., 2012; Carnevale et al., 2014). T2D is associated with greater production of 8-iso-prostaglandin (PG) F2α, a stable compound of non-cyclooxygenase peroxidation of arachidonic acid inducing vasoconstriction and platelet activation (Davì et al., 1999). Furthermore, an enhanced thromboxane (TXB) platelet biosynthesis was demonstrated in diabetic patients (Davì et al., 1990), together with increased platelet susceptibility to aggregating agonists [adenosine diphosphate (ADP), thrombin] and diminished sensitivity to anti-thrombotic molecules such as prostacyclin (PGI2) and nitric oxide (NO) (Vinik et al., 2001). Finally, hyperglycemia has been reported to significantly affect the expression of several platelet and circulating microRNAs in diabetic patients; these small, non-coding RNA molecules have a central role in the modulation of platelet activity and represent potential biomarkers to investigate the response to specific anti-platelet treatments (Carino et al., 2016; Pordzik et al., 2019). Notably, increased shear stress in diseased vessels of diabetic patients may trigger sustained platelet aggregation independent of soluble agonists as well (Nesbitt et al., 2009), a particularly interesting aspect since major antiplatelet agents act exactly by preventing the release of these agonists.
According to this evidence, platelet hyper-reactivity certainly concurs to the development of life-threatening thrombotic complications in T2D patients, with current antiplatelet agents proven to be less effective in diabetic patients, with a high percentage of low or non-responders (Geisler et al., 2007; Angiolillo et al., 2014; Rivas Rios et al., 2018). Of note, an impaired response to antiplatelet therapy has been associated with worse long-term clinical outcomes in several investigations (Angiolillo et al., 2007; Patti et al., 2008; Brar et al., 2011). Altogether, this accounts for the continuous efforts to improve anti-platelet strategy in diabetic patients with CVD, especially when subjected to percutaneous coronary interventions (PCI) (Angiolillo and Ueno, 2011). More effective P2Y12 receptor antagonists such as ticagrelor and prasugrel have been associated with reduced thrombotic events in this high-risk subgroup, although this comes at the cost of higher hemorrhagic risk (Wiviott et al., 2008; James et al., 2010).
Nowadays, a new paradigm shift in diabetes treatment occurs, since clear evidence of cardiovascular benefits associated with novel anti-diabetes drugs has been reported in several randomized trials, moving toward a less “glucocentric” and more “cardioprotective” standpoint (Zinman et al., 2015; Neal et al., 2017; Wiviott et al., 2019; Marso, Daniels, et al., 2016; Kristensen et al., 2019; Marso, Bain, et al., 2016; Holman R. R. et al., 2017). Some glucose-lowering agents have also demonstrated antithrombotic effects in observational studies. The potential benefit on platelets may be related to the normalization of glycemic control, but other additional direct antithrombotic and anti-inflammatory mechanisms may be involved. These findings are clinically relevant since the modulation of platelet activation by anti-diabetic drugs may mitigate the risk of thrombotic events and contribute to cardiovascular protection in diabetic patients (Figure 1).
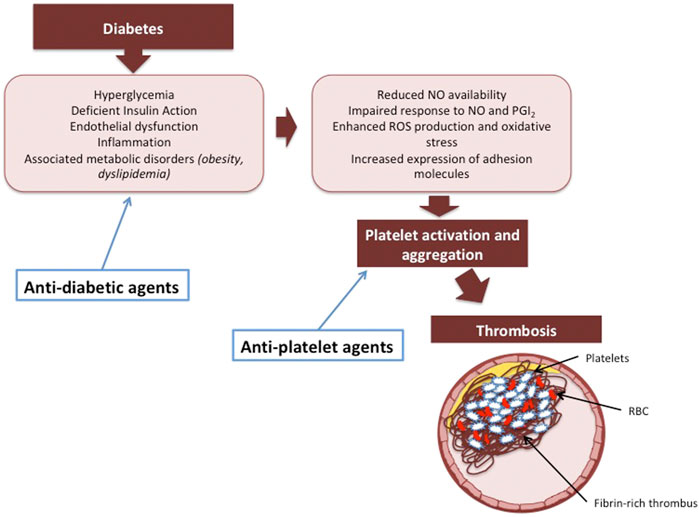
FIGURE 1. The potential synergistic effect of anti-diabetic and anti-platelet agents on preventing thrombosis in diabetic patients.
Nonetheless, the impact of “old and new” glucose-lowering agents on platelet function has not been completely clarified yet. Moreover, studies investigating insulin in this setting are limited and controversial. Herein, we review and discuss current knowledge about the impact of anti-diabetic agents on platelet function and their possible implications for T2D and CVD management.
Metformin
Metformin is a biguanide and exerts its antidiabetic action as an insulin sensitizer by inhibiting gluconeogenesis and stimulating muscle tissue and other insulin-dependent tissues to use glucose (Bailey, 1992). It also determines a lower absorption of glucose in the gastrointestinal tract. Of note, metformin does not induce hypoglycemia, and aside from gastrointestinal side effects when at a higher dosage, it represents a well-tolerated drug (Zilov et al., 2019). The American Diabetes Association recommends metformin as first-line therapy for T2D in newly diagnosed cases and it is the most widely used anti-diabetic drug.
Cardiovascular Outcomes
Metformin is associated with decreased cardiovascular risk in long-term clinical trials. In the UK Prospective Diabetes Study Group (UKPDS), metformin showed a significant reduction in cardiovascular events (MI and stroke) or all-cause mortality compared with diet or chlorpropamide or insulin (King et al., 1999). Similar findings were reported in the Hyperinsulinemia: the Outcome of its Metabolic Effects (HOME) randomized trial; in this study, treatment with metformin improved glycemic control and reduced macrovascular complications (MI, stroke, peripheral vascular disease) in diabetic patients already on insulin therapy on a 4.3 years clinical follow-up (Kooy et al., 2009). Of note, the HOME trial was largely conducted in patients with T2D without previous CVD. Interestingly, a longer duration of metformin treatment seems to improve survival, whereas, adding sulphonylureas to metformin has been demonstrated to attenuate its cardiovascular benefit (Lamanna et al., 2011). Table 1 summarizes the cardiovascular effects of metformin as well as those of all other anti-diabetic agents discussed in this review.
Effects on Platelets
Preclinical Studies
In an experimental study, metformin was able to normalize platelet response to different stimuli such as collagen and arachidonic acid in a hypercholesterolemic rabbit model (Tremoli et al., 1982). Moreover, metformin has been demonstrated to prevent venous and arterial thrombosis by inhibiting platelet activation and extracellular mitochondrial DNA release in carotid and vena cava animal models (Xin et al., 2016).
Clinical Studies
One of the first in vivo studies investigating the potential effect of metformin on platelets showed a significant decrease in maximum ADP-induced platelet aggregation with this agent (Gin et al., 1989). Metformin, administered with a dose of 1,700 mg daily, was added on top of insulin therapy in well-controlled type 1 diabetes (T1D) patients. After 21 days of treatment, no appreciable changes occurred on metabolic factors, such as fibrinogen, cholesterol and triglycerides levels, HbA1c and mean glycemia levels; nevertheless, the maximum platelet aggregation induced by ADP (1.25, 2.5, and 5 μmol doses) decreased significantly (Gin et al., 1989). Another small study in newly diagnosed T2D patients found that metformin improved oxidative stress, preserved anti-oxidant function and decreased platelet activation (Formoso et al., 2008). Specifically, metformin, but not gliclazide treatment, was associated with a significant decrease in 11-dehydro-TXB2 (11-dhTXB2) urinary excretion, a reliable parameter for the measurement of platelet activation in vivo (Formoso et al., 2008). Moreover, metformin has been demonstrated to significantly reduce 8-iso-PG F2α excretion after 12 weeks of treatment, leading to a concurrent increase in anti-oxidant vitamin A and E concentrations (Formoso et al., 2008). A reduced production of platelet superoxide anion (O2-) was also reported in patients treated with metformin compared with glibenclamide or diet (Gargiulo et al., 2002). Finally, long-term metformin seemed to influence dimensional platelet parameters: Dolasık et al. (2013) reported a decreased mean platelet volume in diabetic patients on metformin. Despite what has been described so far, Collier et al. suggested that the benefit of metformin on platelet reactivity was not a class effect but instead related to the glycemic control optimization. In fact, they reported no difference in terms of platelet parameters between metformin and gliclazide when an optimal glucose control was achieved (Collier et al., 1989). The main platelet effects of metformin are reported in Table 1, among the platelet effects of all other anti-diabetic molecules evaluated in this review.
Interaction with Anti-Platelet Drugs
Few studies also investigated the interaction between metformin and anti-platelet drugs, such as aspirin and clopidogrel. The use of metformin among diabetic patients on aspirin therapy was associated with a significantly reduced platelet activation: a higher percentage of patients on metformin in addition to aspirin presented a reduction of 11-dhTXB2 above 75% compared with those on aspirin only (52 vs. 20%, p = 0.027) (Gonçalves et al., 2014). Conversely, another recent study investigated the impact of metformin use on platelet reactivity assessed at 30–90 days by multiple-electrode aggregometry in T2D patients treated with dual antiplatelet therapy (DAPT) after PCI, and no apparent association between the use of metformin and platelet reactivity was found (Verdoia et al., 2021). Table 2 shows drug-drug interactions between anti-diabetic and anti-platelet agents with potential molecular mechanisms.
Sulphonylureas
Sulphonylureas can be divided into first (chlorpropamide) and second-generation (e.g., glibenclamide, gliclazide, glipizide) molecules. This class of agents stimulates insulin secretion by binding to receptors on pancreatic beta cells; furthermore, they have extra-pancreatic actions, inducing an increase in the number of peripheral insulin receptors, thus raising glucose uptake and inhibiting hepatic gluconeogenesis (Proks et al., 2002).
Cardiovascular Outcomes
Previous studies did not demonstrate any cardiovascular benefit of sulphonylureas but rather raised concerns regarding their safety (Table 1). In fact, experimental data suggested that these agents might impair myocardiocytes function by interfering with a myocardial ATP-sensitive potassium channel (Scognamiglio et al., 2002). Furthermore, hypoglycemia is more frequent with sulphonylureas than metformin (Maruthur et al., 2016).
Accordingly, in the previously reported UKPDS trial, adding sulphonylureas to metformin resulted in a reduced cardiovascular improvement (King et al., 1999). Likewise, other authors confirmed the increased risk associated with this combination therapy (Rao et al., 2008). A meta-analysis comparing these agents with other glucose-lowering agents in T2D, reported an increased risk of stroke and overall mortality with sulphonylureas; however, their use was not associated with any significant difference in the incidence of major adverse cardiac events (MACEs) (including MI). Of note, none of the trials included in this meta-analysis was designed or powered to detect cardiovascular events (Azoulay and Suissa, 2017). Conversely, another recent study, evaluating second- or third-generation sulphonylureas, showed no negative effect with regard of all-cause mortality, cardiac mortality, MI or stroke in patients receiving these molecules (Varvaki Rados et al., 2016).
Effects on Platelets
Preclinical Studies
Several in vitro studies have shown significant inhibition of ADP-induced platelet aggregation and a marked decrease in platelets adhesiveness with sulphonylureas (Klaff et al., 1981; Satoh et al., 1994). These anti-platelet effects might be mediated by the free radical scavenging ability of this class of agents. For the gliclazide molecule, this property relates to the unique presence of an amino-azabicyclo-octane ring, whereas, the platelet actions of glimepiride and glibenclamide seem to be linked to their influence on arachidonic acid metabolism (Violi et al., 1982; Satoh et al., 1994). Moreover, inhibitory effects of sulphonylureas on cyclooxygenase and lipoxygenase pathways have been reported (Satoh et al., 1994).
Klaff et al. investigated the effects of gliclazide and glyburide on platelets of diabetic patients, showing reduced aggregation in response to epinephrine and collagen compared with patients not receiving these agents (Klaff et al., 1981). Violi et al. reported an in vitro reduced activation in the thromboxane metabolic pathway after one-month treatment with gliclazide in a small cohort of T2D patients with abnormal platelet function (Violi et al., 1982). Other authors confirmed these findings with glyburide; this drug induced a significant suppression of phospholipase C's thrombin-induced activation with subsequent inhibition of platelet aggregation (Wada et al., 1999). Finally, glibenclamide has been demonstrated to inhibit platelet aggregation in murine platelets (Ting and Khasawneh, 2010).
Clinical Studies
The effect of glyburide on platelet aggregation was early investigated in a small clinical study enrolling 31 diabetic patients; a rapid (60 min) and significant decline in platelet aggregation after 5 mg glyburide ingestion compared to baseline was observed (de Bellis et al., 1984). Of note, gliclazide may be more effective in attenuating platelet aggregation than other sulphonylureas (Jennings et al., 1992; Konya et al., 2010). In particular Konya et al. demonstrated that T2D patients that switched from glibenclamide to gliclazide, titrating the dosage to reach the same glycemic control as the previous treatment (glibenclamide), exhibited a reduction in serotonin-induce platelet aggregates formation compared to that on glibenclamide treatment (Konya et al., 2010) (Table 1).
Interaction with Anti-Platelet Drugs
A recent study investigated the impact of concomitant use of sulphonylureas and clopidogrel on platelet function in a population of T2D patients undergoing PCI on DAPT (Harmsze et al., 2011). Sulphonylureas seemed to be associated with decreased platelet inhibition by clopidogrel quantified using ADP-induced light transmittance aggregometry. Of note, a 2.2-fold increased risk of high on-clopidogrel platelet reactivity was observed in diabetic patients treated with these anti-diabetic agents (Harmsze et al., 2011) (Table 2). These findings are probably due to the competition for isoenzyme CYP2C9 between sulfonylureas and clopidogrel. Interestingly, no drug–drug interactions due to competition for CYP2C9 between sulfonylureas and prasugrel or ticagrelor has been found. Conversely, the hypoglycemic effect of sulphonylureas seems to be potentiated if aspirin is co-administered; in fact, salicylates have been demonstrated to enhance sulphonylureas’ action by increasing insulin secretion. Moreover, aspirin may also displace sulphonylureas from its protein-binding site, enhancing their action (Krentz et al., 1994).
Thiazolidinediones
Thiazolidinediones (TZDs) (pioglitazone, rosiglitazone and troglitazone) act as agonists of the peroxisome proliferator-activated receptor gamma (PPAR-γ), a pleiotropic nuclear receptor and transcription factor most highly expressed in adipose tissue and in macrophages, bone, liver and pancreatic beta cells among other tissues (Yau et al., 2013). Thus, TZDs regulate adipogenesis and fatty acid oxidation and promote adipose tissue ability to store lipids, decreasing circulating fatty acids and improving insulin resistance, whereas they decrease lipotoxicity and increase insulin sensitivity and glucose uptake in muscle (Boden et al., 2005).
Cardiovascular Outcomes
Several randomized trials showed a potential for cardiovascular benefit with TDZs (Table 1). One of the largest trials, the PROspective Pioglitazone Clinical Trial In MacroVascular Events (PROactive) revealed a reduced incidence of the composite endpoint of death from any cause, nonfatal stroke or MI in T2D patients receiving pioglitazone compared with placebo in addition to other glucose-lowering drugs (Dormandy et al., 2005). However, these results have been counterbalanced to some extent by more frequent episodes of HF with pioglitazone (Dormandy et al., 2005). Similar data were reported from the Insulin Resistance Intervention after Stroke (IRIS) trial comparing pioglitazone vs. placebo in patients with insulin resistance and a recent stroke or transient ischemic attack (Viscoli et al., 2014). Patients assigned to TDZs reported a lower percentage of stroke or MI at 5 years compared with control subjects. Finally, a meta-analysis of major randomized controlled trials enrolling patients with stroke and abnormal glucose metabolism, showed that adding pioglitazone to standard therapy was associated with a 32% risk reduction of recurrent stroke and a 25% risk reduction of MACEs (DeFronzo et al., 2011).
Oppositely, another agent of this class, rosiglitazone, has been associated with an increased risk of cardiovascular events in several meta-analyses (Nissen SE et al., 2007; Selvin et al., 2008). The Rosiglitazone Evaluated for CV Outcomes in Oral Agent Combination Therapy for Type 2 Diabetes (RECORD) study was the only prospective trial to directly assess CV outcomes in patients treated with rosiglitazone in addition to metformin or sulphonylureas (Home et al., 2007; Home et al., 2009). The trial demonstrated no increase in the incidence of MI or cardiovascular death with this agent. However, the rate of HF hospitalizations was doubled in the rosiglitazone group compared with the control group (Home et al., 2009).
Effects on Platelets
Preclinical Studies
In animal experiments, Sprague-Dawley rats treated with pioglitazone for 7–10 days showed delayed arterial thrombus formation by reduced ADP- and arachidonic acid-induced platelet aggregation (Li et al., 2005). Similarly, pioglitazone treatment for 1 week reduced soluble P-selectin and P-selectin platelet expression in an insulin-resistant, obesity-prone KK mouse model (Bodary et al., 2005). Ishizuka et al. demonstrated that troglitazone and vitamin E, but not pioglitazone, have potent inhibitory effects on platelet aggregation via suppression of the thrombin-induced activation of phosphoinositide signaling in vitro (Ishizuka et al., 1998).
Clinical Studies
In clinical studies, pioglitazone administered for 3 weeks in obese women showed a significant reduction in platelet activation assessed by urinary TXB metabolite excretion (Basili et al., 2006). Rosiglitazone has also been proved to reduce E-selectin (Hetzel et al., 2005), sCD40L and vWillebrand factor levels (Sidhu et al., 2003), procoagulant mediators released by activated platelets. Moreover, both rosiglitazone and pioglitazone demonstrated to reduce plasminogen activator inhibitor-1 (PAI-1) levels when administered in combination with glimepiride (Derosa et al., 2005). Despite these positive results, a sub-analysis of The Fixed Combination of Pioglitazone and Metformin Improves Biomarkers of Platelet Function and Chronic Inflammation in Type 2 Diabetes Patients (PIOfix) study, a randomized, double-blind study comparing the effects of pioglitazone plus metformin with metformin plus glimepiride on diabetic patients, reported slight but not significant improvements in platelet function markers such as 11-dhTXB2 levels in pioglitazone group, whereas a clear benefit was observed in E-selectin and vWillebrand factor concentrations (Schöndorf et al., 2011). Furthermore, Chokesuwattanaskul et al. reported that administration of 15 mg of pioglitazone daily for 7 days did not reduce platelet aggregation with ADP, epinephrine, collagen or arachidonic acid pathways in healthy, non-diabetic volunteers (Chokesuwattanaskul et al., 2010) (Table 1).
Interaction With Anti-Platelet Drugs
Mongan et al. (2012) investigated the potential platelet inhibitory effect of pioglitazone alone and in combination with aspirin. Platelet function was evaluated 90 and 180 min after ingestion of a single 30 mg dose of pioglitazone in both diabetic and normal subjects on aspirin. Importantly, a significant potentiating effect on platelet inhibition of pioglitazone in addition to aspirin was observed, and the percentage of patients with aspirin resistance, defined as an arachidonic acid-induced aggregation ≥20% despite aspirin, decreased from 63 to 28% after the addition of pioglitazone. Moreover, pioglitazone resulted in a significant decrease in 11-dhTXB2 release. Nevertheless, another small, randomized study, aiming to assess the impact of pioglitazone on clopidogrel-mediated P2Y12 inhibitory effects in T2D patients observed no benefit coming from this association. A 14-days treatment with pioglitazone 30 mg daily was not associated with any enhancement in clopidogrel antiplatelet activity assessed by light transmittance aggregometry and VerifyNow system compared with placebo (Suryadevara et al., 2012). On the other hand, a recent pharmacodynamics study reported that clopidogrel may increase the exposure to pioglitazone effects by inhibiting its CYP2C8-mediated biotransformation; in fact, one of the clopidogrel metabolites, clopidogrel acyl-beta-d-glucuronide, has been found to be a strong time-dependent inhibitor of CYP2C8 in humans and it may possibly overexpose patients to the effects of this anti-diabetic agent (Itkonen et al., 2016) (Table 2).
Acarbose
Acarbose reduces postprandial hyperglycemia by inhibiting intestinal and salivary α-glucosidase; moreover, it acts with a non-competitive inhibition of pancreatic α-amylase (Hanefeld et al., 2008). Acarbose increases serum levels of Glucagon Like Peptide-1 (GLP-1) by regulating the reabsorption of bile acid or by stimulating L cells directly with the unabsorbed carbohydrates to achieve glycemic control (Zheng et al., 2013).
Cardiovascular Outcomes
The effects of acarbose on cardiovascular outcomes were investigated in the Stop Non-Insulin-Dependent Diabetes Mellitus (STOP-NIDDM) trial (Chiasson et al., 2003), recruiting patients with impaired glucose tolerance randomly allocated to acarbose or placebo over a mean follow-up of 3.3 years. In this study, acarbose treatment was associated with a 49% risk reduction in the development of MACEs. Moreover, a meta-analysis of longer-term clinical trials of acarbose in patients with T2D confirmed the lower risk for cardiovascular events with this agent, most notably for MI (Hanefeld et al., 2004; Hanefeld et al., 2004a) (Table 1).
On the other hand, other trials failed to demonstrate cardiovascular benefits for this agent, such as the acarbose Cardiovascular Evaluation (ACE) trial, enrolling Chinese patients with established CVD and impaired glucose tolerance. In this study, acarbose treatment did not reduce the incidence of cardiovascular events during a 5-years treatment period (Holman et al., 2017). Notably, the absence of cardiovascular benefit in this trial compared with the STOP-NIDDM study might reflect the lower dose of acarbose used, the difference in the ethnic group, or the more aggressive secondary cardiovascular prevention measures being recommended when the ACE trial took place.
Effects on Platelets
Preclinical Studies
In a rat animal model, Schafer et al. reported that chronic treatment with acarbose significantly reduced platelet activation due to acute hyperglycemia after oral administration of sucrose (Schäfer et al., 2004). Thus, the co-administration of acarbose, in addition to a sucrose load, reduced glucose absorption and attenuated platelet-bound fibrinogen and platelet surface-expression of P-selectin and glycoprotein 53 (Schäfer et al., 2004).
Clinical Studies
Santilli et al. evaluated the effects of acarbose on markers of lipid peroxidation and platelet activation (8-iso-PG F2α, 11-dhTXB2, plasma CD40 ligand and P-selectin) in T2D patients; 20-weeks acarbose treatment induced a time-dependent reduction in markers of oxidative stress and platelet activation that followed a progressive improvement of postprandial glucose fluctuations and long-term glycemic control (Santilli et al., 2010). Similar findings were reported also by Shimazu et al. (2009), demonstrating that acarbose significantly decreased platelet derived micro-particles and soluble P-selectin in patients with T2D. Furthermore, acarbose reduced in vivo platelet-monocyte aggregates formation in diabetic patients already at 60 min compared to untreated patients (Kaplar et al., 2001) (Table 1). No studies directly designed to analyze the interaction between acarbose and anti-platelets drugs have been published (Table 2).
Dipeptidyl Peptidase-4 Inhibitors
Dipeptidyl peptidase-4 inhibitors (DPP4i) (alogliptin, linagliptin, vildagliptin, saxagliptin, sitagliptin) suppress the breakdown of incretin hormones GLP-1 and glucose-dependent insulinotropic peptide, achieving glycemic control. DPP4i showed beneficial effects on blood pressure, postprandial lipemia, inflammatory markers, oxidative stress and endothelial function in patients with T2D (Scheen, 2013). Moreover, they exhibit enzymatic activity against dozens of peptide hormones and chemokines with roles in vascular pathophysiology, inflammation, stem cell homing and cell survival (Mulvihill and Drucker, 2014).
Cardiovascular Outcomes
Several randomized clinical trials have investigated the cardiovascular benefit of DPP4i with conflicting results (Table 1). However, most of those were non-inferiority studies, thus mainly aiming to demonstrate the safety rather than the effectiveness of these agents over standard glucose-lowering therapies.
In the Saxagliptin Assessment of Vascular Outcomes Recorded in Patients with Diabetes Mellitus (SAVOR-TIMI 53) and Examination of Cardiovascular Outcomes with Alogliptin vs. Standard of Care (EXAMINE) trials, DPP4i did not appear to increase the risk of MACEs (Scirica et al., 2013; White et al., 2013). Notwithstanding, both patients receiving saxagliptin and alogliptin experienced an increased incidence of HF admission compared with those randomized to placebo (HR 1.27, 95% CI 1.07–1.51, and HR 1.07, 95% CI 0.79–1.46, respectively). In light of these results, the Food and Drug Administration issued an updating warning over the cardiovascular safety of saxagliptin and alogliptin, to make patients and physicians aware of a potential increased risk of heart failure (FDA Drug Safety Communication: FDA adds warnings about heart failure risk to labels of type 2 diabetes medicines containing saxagliptin and alogliptin2016). Differently, the Trial Evaluating Cardiovascular Outcomes with Sitagliptin (TECOS) reported similar occurrence of cardiac events and HF hospitalizations in patients with T2D and CVD treated with sitagliptin and placebo (Green et al., 2015; Sharma et al., 2017).
No additional clinical benefit was observed with linagliptin compared with standard care in randomized clinical trials. Both the Cardiovascular Outcome Study of Linagliptin vs. Glimepiride in Patients With Type 2 Diabetes study (CAROLINA) (Marx et al., 2015), and, more recently, the CArdiovascular Safety and Renal Microvascular OutcomE with LINAgliptin in Patients With Type 2 Diabetes Mellitus at High Vascular Risk (CARMELINA) trial (Rosenstock et al., 2018; McGuire et al., 2019) did not report any difference in efficacy and safety outcomes between linagliptin and placebo.
Vildagliptin is the only DPP4i that lacks established or ongoing randomized controlled trials investigating cardiovascular outcomes; however, two large meta-analyses and a recent large european observational cohort study suggested that this agent was not associated with an increased risk of MACEs in a broad spectrum of populations (Mcinnes et al., 2015; Williams et al., 2017). Nevertheless, a small, randomized trial evaluating vildagliptin in patients with diabetes and HF with reduced ejection fraction, showed no increase in HF-related hospitalizations, but a harmful enlargement of left ventricular volumes of unknown cause with this drug (McMurray et al., 2018).
Considering the overall safety results from DPP4i randomized trials, these molecules must be used with caution in patients with increased risk of heart failure.
Effects on Platelets
Preclinical Studies
Most of the DPP4i have been suggested to have anti-thrombotic effects. This is also related to their specific molecular structure: linagliptin, for example, contains a methylxanthine structure inhibiting phosphodiesterase and leading to increased intracellular cAMP levels. Thus, Steven et al. hypothesized that cAMP accumulation and subsequent phosphatase-A activation may represent one of the DPP4i anti-platelet mechanisms (Steven et al., 2017). In line with this, inhibition of DPP4 activity by diprotin A has been reported to induce platelet aggregation in human umbilical vein endothelial cells (Krijnen et al., 2012).
Additionally, DPP4 enzyme cleaves not only incretins, but also other substrates such as SDF-1α, peptide YY, and brain natriuretic peptide (Ussher and Drucker, 2012). Of these substrates, SDF-1α is the most potent chemokine known to induce cardiovascular protection by DPP4i, promoting stem cell repopulation and homing to ischemic tissues (Steven et al., 2017). Most of the DPP4i exhibited to suppress inflammatory and thrombogenic gene expression (Maeda et al., 2012; Matsubara et al., 2012; Birnbaum et al., 2016). Vildagliptin treatment has been shown to significantly reduce plasma fibrinogen and PAI-1 in an in vivo experiment (Dardik et al., 2003), whereas treatment of obese rats with saxagliptin decreased soluble levels of CD40 by over 10-fold (Mason et al., 2011). More recently, linagliptin was proven to significantly inhibit platelet aggregation by reducing platelet mitochondrial respiration and preserving cAMP-dependent phosphodiesterase. Moreover, this agent was also able to improve a carotid artery thrombosis model in vivo (Li et al., 2020).
Clinical Studies
Sitagliptin revealed to inhibit platelet aggregation in both healthy individuals and T2D patients. Fifty diabetic patients were treated with this agent for 3 months. After 1 and 3 months of DPP4i treatment, platelet aggregation, assessed in vivo by light transmission aggregometry, showed a significant reduction with sitagliptin (Table 1). The same findings were confirmed in vitro, using platelets from 10 healthy humans. Platelets pre-treated in vitro with 5 and 10 μg/ml of sitagliptin had a significant inhibition of thrombin-induced platelet aggregation (Gupta et al., 2012). Interestingly, the concentration-dependent antiplatelet activity of sitagliptin was attributed to the inhibitory effect on intracellular free calcium and tyrosine phosphorylation (Gupta et al., 2012).
Sodium–Glucose Cotransporter-2 Inhibitors
Sodium–glucose cotransporter-2 inhibitors (SGLT-2i) (empagliflozin, canagliflozin, dapagliflozin) act by inactivating sodium glucose cotransporters located in S2 and S3 segments of renal proximal tubules, thus leading to a net loss of sodium and glucose through urine excretion and improving glycemic control via insulin-independent mechanisms (Cavallari and Maddaloni, 2019; Yaribeygi et al., 2019).
Cardiovascular Outcomes
Large-scale randomized trials have demonstrated that SGLT-2i have a remarkable effect in reducing the incidence of MACEs in patients with T2D (Zelniker et al., 2019) (Table 1). Thus, the last European Society of Cardiology/European Association for the Study of Diabetes Guidelines recommend the use of this glucose-lowering class to lower the risk of HF hospitalization in patients with T2D and to reduce cardiovascular events in those at higher cardiovascular risk (Cosentino et al., 2020).
The Empagliflozin Cardiovascular Outcome Event Trial in Type 2 Diabetes Mellitus Patients–Removing Excess Glucose (EMPA-REG OUTCOME) was the first trial demonstrating an unexpected 34% risk reduction of cardiovascular death and HF admissions (35% risk reduction for the individual component of HF hospitalization) in patients treated with empagliflozin, corresponding to a number needed to treat to prevent one event of the composite outcome of 35 over 3 years (Zinman et al., 2015). Interestingly, even though only 10% of subjects in EMPA-REG trial had a pre-existing HF at baseline, the positive effects on HF hospitalizations and cardiovascular death were consistent in patients with or without previous HF (Fitchett et al., 2016). In accordance with the EMPA-REG OUTCOME, also the Canagliflozin Cardiovascular Assessment Study (CANVAS) trial observed a significant lower risk of CV death (22%) and HF hospitalization (33%) (Neal et al., 2017), whereas the Dapagliflozin Effect on Cardiovascular Events (DECLARE)-TIMI 58 trial reported a meaningful 17% reduction in the risk of the combined end point of cardiovascular death and HF hospitalizations with dapagliflozin (Kato et al., 2019).
A previous meta-analysis including more than 34,000 diabetic patients, of whom 60% with established cardiovascular disease, showed that SGLT-2i reduced the incidence of MACEs by 11%, as well as the incidence of cardiovascular death and HF hospitalization by 23% (Zelniker et al., 2019). Notably, SGLT-2i benefit emerged early after the initiation of the treatment and persisted on long-term follow-up (2–5 years). Moreover, if the reduction in MACEs occurrence was evident only in T2D patients with established CVD, the benefit in HF hospitalization was robust and consistent among the entire population, regardless of the previous presence of established atherosclerotic cardiovascular disease or a history of HF (Zelniker et al., 2019). These findings were confirmed by another recent meta-analysis containing results with ertuglifozin, another available SGLT2i (McGuire., et al., 2019). Indeed, SGLT2i are associated with a lower risk of MACEs, hospitalization for HF or cardiovascular death, and kidney outcomes, regardless of the presence or absence of pre-existing CVD.
Although the underlying mechanisms for the CV protection of SGLT-2i are not fully understood, a combination of several pathways are probably involved; the most studied mechanisms include the diuretic effect, reducing cardiac workload and myocardial oxygen consumption (Chilton et al., 2015; Gobel et al., 1978; Cavallari et al., 2020) and the beneficial effects of ketone bodies on the heart (Gobel et al., 1978; Chilton et al., 2015; Mudaliar et al., 2016; Sano et al., 2016; Pham and Chilton, 2017). Furthermore, recent investigations reported that empaglifozin is able to restore ion hemostasis in failing cardiac myocytes, reducing intracellular sodium overload by the inhibition of the sarcolemmal Na+/H+ exchanger (Bertero et al., 2018; Packer, 2019).
Effects on Platelets
Preclinical Studies
Both direct and indirect antithrombotic effects of SGLT-2i have been suggested. In a recent study by Steven et al., 6-weeks treatment with empaglifozin significantly reduced inflammatory state, endothelial dysfunction and platelet hyperactivity/aggregation observed in the aorta of Zucker diabetic fatty rats (Steven et al., 2017). The same authors found that the up-regulation of mRNA expression for inducible nitric oxide synthase (eNOS) and P-selectin was prevented by SGLT-2i treatment (Steven et al., 2017). Spigoni et al. showed a reduced expression of ADP-induced platelet activation markers (CD62p and PAC1), assessed by flow-citometry, in platelets isolated from peripheral blood of healthy subjects and incubated with empaglifozin and dapaglifozin (Spigoni et al., 2020). Finally, a recent study investigating the direct effects of SGLT-2i on platelet aggregation, demonstrated that SGLT-2 protein is not expressed on human platelets. Moreover, in contrast to previous findings, the same authors reported that glifozins (canagliflozin, dapagliflozin, empagliflozin) produced only mild platelet inhibition, but their anti-thrombotic effects are strongly potentiated by NO and PGI2, thus with co-administration of sodium nitroprusside and iloprost (Lescano et al., 2020).
Furthermore, it has been observed that SGLT-2i can indirectly inhibit platelet function by reducing ROS generation. In this regard, a recent in vitro study showed that empaglifozin and dapaglifozin restored NO bioavailability in TNFα-stimulated human coronary arterial endothelial cells probably by inhibiting the generation of ROS (Wang et al., 1998; Smyth et al., 2017). Similarly, in another ex vivo study by Aroon and colleagues, empagliflozin suppressed advanced glycation end products, restored eNOS activation and reduced interstitial and periarterial NO stress (Aroor et al., 2018).
Nevertheless, volume depletion and hemoconcentration induced by SGLT-2i raised the question about a possible pro-thrombotic effect; concern that was disproved by several investigations. Indeed, some authors observed that the hematocrit rise triggered by these agents may promote oxygen delivery to the myocardium and reduce workload of the proximal renal tubules, improving tubule-interstitial hypoxia (Sano et al., 2016). Along this line, Santos-Gallego et al. recently investigated the effects of chronic (2 months) empagliflozin treatment on platelet aggregation in a porcine model after ischemic injury induced by coronary occlusion, failing to show enhanced platelet aggregation or thrombus kinetics alterations (Santos-Gallego et al., 2018) (Table 1).
Clinical Studies
No “ad hoc” study investigated the anti-platelet effects of SGLT-2i in diabetic patients. Indirect information can be deduced from large clinical trials. In 2018 Zheng and colleagues compared the efficacy of SGLT-2i, GLP-1 agonists, and DPP4i on mortality and cardiovascular end points using a network meta-analysis of 236 trials with 176 310 participants, finding that SGLT-2 inhibitors were associated with reduction in all MIs [HR 0.86 (95% CI 0.77–0.97)] and nonfatal MIs [HR 0.84 (95% CI 0.72–0.98)] compared with the control groups (Zheng et al., 2018). On the other hand, the supposed procoagulant activity of SGLT2 is due to the reduction of volemia was also excluded by several studies reporting no increase in venous thromboembolism with the use of these agents (Ueda et al., 2018). No studies specifically designed to investigate the interaction between SGLT-2i with anti-platelet drugs are available to date.
GLP-1 Receptor Agonists
The incretin hormone GLP-1, mainly secreted from enteroendocrine cells after meal ingestion, exerts its insulin secretion and glucagon suppression effect in a glucose-dependent manner, upon binding to a specific GLP-1 Receptor (GLP-1R) (Sacks et al., 2018). This receptor represents the pharmacological target of GLP-1 receptor agonists (GLP-1RA). GLP-1 also inhibits beta-cell apoptosis, promotes beta-cell neogenesis, reduces circulating lipoproteins, delays gastric emptying and promotes satiety reducing food intake (Sacks et al., 2018).
Cardiovascular Outcomes
Several randomized trials focused on GLP-1RA cardiovascular effects. A recent meta-analysis by Kristensen et al. (2019), including all the large, placebo-controlled, published randomized trials, summarized the evidence regarding GLP-1RA. The authors examined the incidence of MACEs (a composite outcome of CV death, non-fatal stroke and non-fatal MI) in the overall population but also in several specific subgroups based on patients’ characteristics (previous CVD, body mass index, age, baseline HbA1c), trial duration, treatment dosing interval and GLP1-RA structural homology. Seven trials with a total of 56,004 patients in primary (stable cardiovascular disease) and secondary cardiovascular prevention (cardiovascular risk factors) were included: Evaluation of Lixisenatide in Acute Coronary Syndrome (ELIXA), Liraglutide Effect and Action in Diabetes: Evaluation of Cardiovascular Outcome Results (LEADER), Trial to Evaluate Cardiovascular and Other Long-term Outcomes With Semaglutide in Subjects With Type 2 Diabetes (SUSTAIN- 6), Exenatide Study of Cardiovascular Event Lowering Trial (EXSCEL), Albiglutide and cardiovascular outcomes in patients with type 2 diabetes and cardiovascular disease (Harmony Outcomes), Dulaglutide and cardiovascular outcomes in type 2 diabetes (REWIND) and Trial Investigating the Cardiovascular Safety of Oral Semaglutide in Subjects With Type 2 Diabetes (PIONEER 6). In the pooled analysis, treatment with a GLP-1RA led to a 12% relative risk reduction in MACEs (HR 0.88 95% CI 0.82–0.94; p < 0.0001); when considered separately, the relative risk reduction for death from cardiovascular causes and for fatal and non-fatal stroke (12 and 16%, respectively) were also statically significant (p < 0.0001). Similarly, GLP-1RA treatment was associated with a significant reduction in the incidence of fatal or non-fatal MI (p = 0.043). In subgroup analyses, no statistical heterogeneity was reported between patients in primary or secondary prevention or when considering the baseline HbA1c; however, the authors found a potentially smaller clinical effect with drugs based on exendin-4 (exenatide and lixisenatide) compared with those more structurally similar to native GLP-1 (albiglutide, dulaglutide, liraglutide, and semaglutide). Moreover, since GLP1-RA benefit was consistent across subgroups based on age and kidney function, and older age and lower eGFR were associated with a higher rate of MACEs, it may be speculated that the positive effects of this class of glucose-lowering agents may be greater in these high-risk subgroups.
Importantly, this meta-analysis showed for the first time a reduction in HF admissions, more evident in two trials (Harmony Outcomes and LEADER), with the greatest reduction in MI, a common precursor of HF. These clinical findings may suggest that GLP-1RA have mainly an anti-atherothrombotic effect, with a less pronounced direct effect on HF than seen with SGLT-2i (Table 1).
Effects on Platelets
Preclinical Studies
Several data suggest that the GLP1-RA may have a role in reducing platelet aggregation and thrombus formation (Table 1). Indeed, vascular smooth muscle cells appear to express the GLP-1 receptor which seems to exhibit a number of positive actions on the vascular endothelium (Almutairi et al., 2019). Moreover, recently, the presence of GLP-1R was also confirmed in platelets (Barale et al., 2017; Cameron-Vendrig et al., 2016; Marso, Bain, et al., 2016; Steven et al., 2017).
In a recent in vitro study, Cameron-Vendrig et al. demonstrated that incubation with exenatide elicited a cAMP response in human megakaryocyte cell line, leading to a significant inhibition of thrombin-, ADP-, and collagen-induced platelet aggregation (Cameron-Vendrig et al., 2016). This GLP-1RA was also able to reduce thrombus formation in ex vivo perfusion chambers using human and mouse whole blood and in a mouse artery model. According to this study, the anti-thrombotic effects of exenatide were mediated by a cAMP-induced phosphatase A activation, with subsequent enhancement of eNOS activity (Cameron-Vendrig et al., 2016). Similar data were reported in another experimental study by Steven et al. (2017), where platelet GLP-1R activation by liraglutide significantly reduced endotoxemia-induced microvascular thrombosis. Specifically, in vitro experiments on human platelets revealed a dose-dependent inhibitory effect of liraglutide on platelet activity in response to ADP and thrombin (Steven, Jurk, et al., 2017). Furthermore, other studies reported that the anti-platelet effects of GLP-1 metabolites and analogues could be related to their ability to decrease oxidative stress by improving intracellular antioxidant defenses and decreasing ROS production through GLP-1 receptor-dependent and independent pathways (Oeseburg et al., 2010; Ceriello et al., 2013).
Clinical Studies
Barale et al. (2017) investigated the effects of a 15-min incubation with the native form GLP-1 (7–36), its degradation product GLP-1 (9–36) and the GLP1-RA liraglutide in platelets from 72 healthy volunteers. They observed that all these agonists significantly attenuated platelet aggregation by inducing an increase in the NO bioavailability, by stimulating cGMP production and enhancing the extent of phosphorylation of vasodilator-stimulated phosphoprotein (VASP)-ser239, and by reducing the activation of phosphatidylinositol 3-kinase (PI3-K)/Akt and mitogen-activated protein kinase/extracellular-signal-regulated kinase (MAPK/ERK)-2 pathways probably through a cGMP-dependent protein kinase (PKG)-dependent mechanism. Furthermore, a significant decrease in ROS production was observed with circulating GLP-1 metabolites and liraglutide (Barale et al., 2017). Of note, in this study, GLP-1 metabolites and its analogues were demonstrated to act on platelet function independently from their receptor. In fact, the authors showed that when platelets were preincubated with exendin, a specific GLP-1R antagonist, the platelet inhibitory effects were maintained using GLP-1 (9–39), GLP-1 (7–36) and liraglutide. Conversely, more recently, the same authors (Barale et al., 2019) did not find any anti-thrombotic properties of GLP-1RA in subjects with primary hypercholesterolemia. In fact, GLP-1 (7–36), GLP-1 (9–36) and liraglutide all failed to enhance the anti-aggregating effects of exogenous NO and to reduce ROS production in platelets from patients with primary hypercholesterolemia compared with normocholesterolemic controls. Moreover, a lipid-lowering treatment with simvastatin for three months did not restore platelet sensitivity to GLP-1 effects. A possible explanation for these results could imply the same target of GLP-1 and simvastatin. In fact, both drugs seem to share, at least in part, inhibition of platelet NADPH oxidase-derived ROS formation, so the authors supposed that platelet exposure to GLP-1 after a treatment with simvastatin was no longer able to further modify resistance to GLP-1 related peptides.
In addition, Kahal et al. (2015) investigated anti-platelet effects of liraglutide in obese women with or without polycystic ovarian syndrome. They found a significant inhibition in basal platelet P-selectin expression after 6-months treatment with liraglutide.
No studies specifically designed to find interactions between GLP-1RA with anti-platelet drugs are available to date.
Insulin Therapy
Indications for exogenous insulin therapy in patients with T2D include acute illness or surgery, pregnancy, glucose toxicity and contraindications to or failure to achieve goals with oral antidiabetic medications (Anon, 2020). Exogenous insulin is available as synthetic human insulin and insulin analogues, the latter being generally preferred. Insulins are typically classified based on their pharmacokinetic and pharmacodynamic (i.e., duration of action, absorption), as fast-acting (includes rapid-acting and short-acting insulins), intermediate-acting, and long-acting insulins.
Cardiovascular Outcomes
In experimental models, insulin has both pro- and anti-atherogenic actions (Potenza et al., 2009), suggesting a mixed effect on CVD. However, data available from randomized trials consistently indicate an absence of cardiovascular safety issues with insulin therapy. In the UKPDS trial, the incidence of cardiovascular events in the insulin group was not different from those observed with sulfonylureas or in the control group on conventional therapy (Turner, 1998). However, in the long-term post-trial follow-up, authors showed that patients randomized to insulin therapy had a significant lower cardiovascular morbidity and mortality, possibly due to the long-term glycemic control improvement obtained with intensified insulin therapy (Gerstein et al., 2008). Similar results emerge from the Diabetes Mellitus, Insulin Glucose Infusion in Acute Myocardial Infarction (DIGAMI) Study, which demonstrated a significant 25% reduction in mortality (at 3.4 years of follow-up) among patients with diabetes and MI who underwent an initial 24h glucose-insulin infusion followed by an intensified insulin therapy (Malmberg, 1997). However, other CVD outcome trials failed to show any effect (beneficial or detrimental) of insulin therapy on CVD morbidity or mortality (Gerstein et al., 2012), confirming the cardiovascular safety of insulin treatment in T2D patients. In this regards, it is worth noting that in the Intensive Diabetes Treatment and Cardiovascular Outcomes in T1D Study (DCCT/EDIC) cohort, intensive diabetes therapy was associated with a modest reduction in all-cause mortality rate when compared with conventional therapy (Orchard et al., 2015), showing long-term beneficial effects on CVD incidence persisting for up to 30 years (Nathan, 2014).
The UKPDS findings and the potential cardioprotective effects on insulin among people with new-onset T2D were also tested in a large and long-term trial, the Outcome Reduction with an Initial Glargine Intervention (ORIGIN) study. In this trial, subjects were randomized to receive insulin glargine or standard of care on the basis of local guidelines (The ORIGIN trial investigators, 2012). There were two co-primary outcomes: the first included a composite MACEs outcome (non-fatal MI, non-fatal stroke or death from cardiovascular causes) and the second one any of these events plus a revascularization procedure or hospitalization for HF. At the end of the study (median follow-up 6.2 years) the incidence of both co-primary outcomes did not differ significantly between treatment groups. The authors suggested that the insulin cardiovascular benefits reported in previous large trials might have been mediated by improvements in glycemic control rather than direct cardiovascular effects.
Effects on Platelets
The majority of studies on this topic combine preclinical and clinical research, which will be discussed in this section.
Physiologically, insulin has potent inhibitory effects on platelet hyperactivity, promoting NO production by activating eNOS through PI3K-dependent pathway and endothelin-1 secretion via MAPK-dependent pathway (Dimmeler et al., 1999; Mather et al., 2001). However this effect seems to be blunted in T2D. In fact, platelets of T2D patients have decreased sensitivity to insulin, which may lead to increased platelet reactivity and a higher risk of atherothrombotic events among insulin-treated subjects (Ferreira et al., 2006).
Spectre et al. investigated meal-induced platelet activation by comparing platelet P-selectin expression and fibrinogen binding, with or without stimulation with the thromboxane analog U46619 or ADP, following premeal injections of placebo or insulin aspart (Spectre et al., 2012). The standardized meal enhanced U46619-induced platelet P-selectin expression by 23% after placebo and it was more than doubled after premeal insulin. No changes in fibrinogen binding were found after meal intake with placebo, instead it was increased by 50–60% after premeal insulin (Spectre et al., 2012). These data suggest that postprandial hyperinsulinemia, rather than postprandial hyperglycemia, causes platelet hyperactivity mediated via pathways stimulated by TXB and ADP but not collagen in patients with T2D.
Few years later, the same authors, found no difference in the preprandial platelet activation between T1D and T2D patients; conversely, platelet-leukocyte aggregates were higher in T1D. After the standardized meal, platelet P-selectin expression, fibrinogen binding and platelet-leukocyte aggregates formation were approximately doubled in patients with T2D but unchanged in T1D. Thus, in the same study, 5 T1D volunteers were re-evaluated after their regular premeal insulin treatment, finding that, when premeal insulin was administered, platelet activation was increased. These findings confirmed the previous authors’ hypothesis that T1D patients, unable to secrete insulin, may have no platelet activation after the meal when no insulin is administered despite very high postprandial glucose levels, so postprandial platelet activation in diabetic patients may be more related to insulin rather than postprandial hyperglycemia (Spectre et al., 2016).
Interestingly, some data are available regarding the effect on platelets exerted by different type of insulin. Russo et al. showed that after 60 min of incubation of human platelets with insulin aspart or human regular insulin, the anti-aggregating effect was greater with insulin aspart than with human regular insulin (p = 0.027) (Russo et al., 2002).
However, the relationship between insulin therapy and platelet function remains controversial. The strongest evidence about the impact of insulin therapy on platelet activity appears to be more related to hyper or hypoglycemic events rather than to a direct effect on platelet function. On that note, insulin therapy in T2D is usually prescribed to patients with poor glucose control. Hyperglycemia enhances platelet activation and high-shear stress–induced activation, leading to arterial thrombosis, partly due to acute enhancement of the circulating levels of von Willebrand factor (Gresele et al., 2003). Furthermore, chronic hyperglycemia has been associated with a twofold rise in thrombin-antithrombin complexes and soluble tissue factor (Stegenga et al., 2006), nonenzymatic glycation of platelet glycoproteins, changes in their conformation and membrane lipid structure alterations (Angiolillo et al., 2006).
On the other hand, hypoglycemia, which is the main adverse effect of insulin therapy, also appears to be associated with platelet activation. A recent randomized trial showed that in healthy men acute hypoglycemia impairs fibrinolytic balance, increases platelet activation and coagulation biomarkers and reduces NO-mediated endothelial function (Joy et al., 2015). Additionally, other authors observed that in T1D subjects, hypoglycemic events result in activation of prothrombotic, proinflammatory and proatherogenic mechanisms (Gogitidze Joy et al., 2010).
In summary, chronic hyperglycemia as well as the higher risk of hypoglycemic events observed with insulin treatment appears to be more relevant that insulin physiological inhibition of platelet hyperactivity.
Conclusion
Enhanced thrombosis mediates most serious complications associated with T2D such as MI and stroke, with a significant impact on survival. Thus, patients with T2D warrant careful attention of cardiologists and usually undergo more aggressive and longer anti-platelet regimens compared with non-diabetics.
Importantly, in the last years, a significant revolution in the management of diabetic patients has begun with the introduction of new glucose-lowering agents that have been demonstrated to meaningfully reduce the incidence of MACEs. In this review, we summarized the main cardiovascular effects of these agents, focusing on their anti-platelet properties potentially preventing thrombosis. Underlying mechanisms involve improved glycemic control, but also increased nitric oxide bioavailability, reduced oxidative stress and, for certain molecules, a direct inhibition of platelet activation and aggregation. Further investigations are needed to evaluate how much these anti-thrombotic abilities may contribute to the overall clinical benefit observed in randomized trials. Moreover, potential synergism with antiplatelet agents should be verified in ad hoc studies.
Surely, this evidence on old and new glucose-lowering agents introduces a paradigm change in the clinical management of T2D since we could potentially prevent thrombosis in such patients through an optimal use of anti-platelet but also glucose-lowering agents. Hence, cardiologists should be able to carefully manage these drugs in order to provide an appropriate therapy tailored to patients’ glycometabolic and thrombotic risk.
Author Contributions
AN and DT—conceptualization, writing, review and editing of the manuscript; SP, SG, MC, LM—review of the literature, writing; MW, IC, EM—critical revision to scientific content; GU, SM, FG—conceptual guidance, critical revision and editing of the manuscript. All authors read, commented, and approved the final version of the manuscript.
Conflict of Interest
The authors declare that the research was conducted in the absence of any commercial or financial relationships that could be construed as a potential conflict of interest.
Abbreviations
T2D, type 2 diabetes; T1D, type 1 diabetes; CVD, cardiovascular disease; MI, myocardial infarction; HF, heart failure; ROS, reactive oxygen species; PG, prostaglandin; ADP, adenosine diphosphate; PGI2, prostacyclin; NO, nitric oxide; PCI, percutaneous coronary intervention; TXB, thromboxane; DAPT, dual antiplatelet therapy; MACE, major adverse cardiac events; TZDs, Thiazolidinediones; PAI-1, Plasminogen activator inhibitor-1; GLP-1, Glucagon like peptide-1; DPP4i, Dipeptidyl peptidase-4 inhibitors; SGLT-2i, Sodium–glucose cotransporter 2 inhibitors; NOS, nitric oxide synthase; GLP-1RA, GLP-1 receptor agonists.
References
Almutairi, M., Al Batran, R., and Ussher, J. R. (2019). Glucagon-like Peptide-1 Receptor Action in the Vasculature. Peptides 111, 26–32. doi:10.1016/j.peptides.2018.09.002
Angiolillo, D. J., Bernardo, E., Ramírez, C., Costa, M. A., Sabaté, M., Jimenez-Quevedo, P., et al. (2006). Insulin Therapy Is Associated with Platelet Dysfunction in Patients with Type 2 Diabetes Mellitus on Dual Oral Antiplatelet Treatment. J. Am. Coll. Cardiol. 48 (2), 298–304. doi:10.1016/j.jacc.2006.03.038
Angiolillo, D. J., Fernandez-Ortiz, A., Bernardo, E., Alfonso, F., Macaya, C., Bass, T. A., et al. (2007). Variability in Individual Responsiveness to Clopidogrel. J. Am. Coll. Cardiol. 49 (14), 1505–1516. doi:10.1016/j.jacc.2006.11.044
Angiolillo, D. J., Jakubowski, J. A., Ferreiro, J. L., Tello-Montoliu, A., Rollini, F., Franchi, F., et al. (2014). Impaired Responsiveness to the Platelet P2Y12 Receptor Antagonist Clopidogrel in Patients with Type 2 Diabetes and Coronary Artery Disease. J. Am. Coll. Cardiol. 64 (10), 1005–1014. doi:10.1016/j.jacc.2014.06.1170
Angiolillo, D. J., and Ueno, M. (2011). Optimizing Platelet Inhibition in Clopidogrel Poor Metabolizers. JACC: Cardiovasc. Intervent. 4 (4), 411–414. doi:10.1016/j.jcin.2011.03.001
Anon, J. (20202020). American Diabetes Association. 9. Pharmacologic Approaches to Glycemic Treatment: Standards of Medical Care in Diabetes-2020. Diabetes Care 43 (Suppl. 1), S98–S110. doi:10.2337/dc20-S009
Aroor, A. R., Das, N. A., Carpenter, A. J., Habibi, J., Jia, G., Ramirez-Perez, F. I., et al. (2018). Glycemic Control by the SGLT2 Inhibitor Empagliflozin Decreases Aortic Stiffness, Renal Resistivity Index and Kidney Injury. Cardiovasc. Diabetol. 17 (1), 108. doi:10.1186/s12933-018-0750-8
Azoulay, L., and Suissa, S. (2017). Sulfonylureas and the Risks of Cardiovascular Events and Death: A Methodological Meta-Regression Analysis of the Observational Studies. Dia Care 40 (5), 706–714. doi:10.2337/dc16-1943
Bailey, C. J. (1992). Biguanides and NIDDM. Diabetes Care 15 (6), 755–772. doi:10.2337/diacare.15.6.755
Barale, C., Buracco, S., Cavalot, F., Frascaroli, C., Guerrasio, A., and Russo, I. (2017). Glucagon-like Peptide 1-related Peptides Increase Nitric Oxide Effects to Reduce Platelet Activation. Thromb. Haemost. 117 (6), 1115–1128. doi:10.1160/TH16-07-0586
Barale, C., Frascaroli, C., Cavalot, F., and Russo, I. (2019). Hypercholesterolemia Impairs the Glucagon-like Peptide 1 Action on Platelets: Effects of a Lipid-Lowering Treatment with Simvastatin. Thromb. Res. 180, 74–85. doi:10.1016/j.thromres.2019.06.010
Basili, S., Pacini, G., Guagnano, M. T., Manigrasso, M. R., Santilli, F., Pettinella, C., et al. (2006). Insulin Resistance as a Determinant of Platelet Activation in Obese Women. J. Am. Coll. Cardiol. 48 (12), 2531–2538. doi:10.1016/j.jacc.2006.08.040
Bertero, E., Prates Roma, L., Ameri, P., and Maack, C. (2018). Cardiac Effects of SGLT2 Inhibitors: the Sodium Hypothesis. Cardiovasc. Res. 114 (1), 12–18. doi:10.1093/cvr/cvx149
Birnbaum, Y., Bajaj, M., Qian, J., and Ye, Y. (2016). Dipeptidyl Peptidase-4 Inhibition by Saxagliptin Prevents Inflammation and Renal Injury by Targeting the Nlrp3/ASC Inflammasome. BMJ Open Diab Res. Care 4 (1), e000227. doi:10.1136/bmjdrc-2016-000227
Bodary, P. F., Vargas, F. B., King, S. A. D., Jongeward, K. L., Wickenheiser, K. J., and Eitzman, D. T. (2005). Pioglitazone Protects against Thrombosis in a Mouse Model of Obesity and Insulin Resistance. J. Thromb. Haemost. 3 (10), 2149–2153. doi:10.1111/j.1538-7836.2005.01551.x
Boden, G., Homko, C., Mozzoli, M., Showe, L. C., Nichols, C., and Cheung, P. (2005). Thiazolidinediones Upregulate Fatty Acid Uptake and Oxidation in Adipose Tissue of Diabetic Patients. Diabetes 54 (3), 880–885. doi:10.2337/diabetes.54.3.880
Brar, S. S., ten Berg, J., Marcucci, R., Price, M. J., Valgimigli, M., Kim, H.-S., et al. (2011). Impact of Platelet Reactivity on Clinical Outcomes after Percutaneous Coronary Intervention. J. Am. Coll. Cardiol. 58 (19), 1945–1954. doi:10.1016/j.jacc.2011.06.059
Cameron-Vendrig, A., Reheman, A., Siraj, M. A., Xu, X. R., Wang, Y., Lei, X., et al. (2016). Glucagon-Like Peptide 1 Receptor Activation Attenuates Platelet Aggregation and Thrombosis. Diabetes 65 (6), 1714–1723. doi:10.2337/db15-1141
Cangemi, R., Pignatelli, P., Carnevale, R., Nigro, C., Proietti, M., Angelico, F., et al. (2012). Platelet Isoprostane Overproduction in Diabetic Patients Treated with Aspirin. Diabetes 61 (6), 1626–1632. doi:10.2337/db11-1243
Carino, A., De Rosa, S., Sorrentino, S., Polimeni, A., Sabatino, J., Caiazzo, G., et al. (2016). Modulation of Circulating MicroRNAs Levels during the Switch from Clopidogrel to Ticagrelor. Biomed. Res. Int. 2016, 1–5. doi:10.1155/2016/3968206
Carnevale, R., Loffredo, L., Sanguigni, V., Plebani, A., Rossi, P., Pignata, C., et al. (2014). Different Degrees of NADPH Oxidase 2 Regulation and In Vivo Platelet Activation: Lesson from Chronic Granulomatous Disease. Jaha 3 (3), e000920. doi:10.1161/JAHA.114.000920
Cavallari, I., and Maddaloni, E. (2019). Cardiovascular Effects of SGLT‐2 Inhibitors: What We Have Learned from Cardiovascular Outcome Trials and what We Still Need to Understand. Diabetes Metab. Res. Rev. 35 (4), e3124. doi:10.1002/dmrr.3124
Cavallari, I., Maddaloni, E., Pieralice, S., Mulé, M. T., Buzzetti, R., Ussia, G. P., et al. (2020). The vicious circle of left ventricular dysfunction and diabetes: From pathophysiology to emerging treatments. J. Clin. Endocrinol. Metab. 105, dgaa427. doi:10.1210/clinem/dgaa427
Cavender, M. A., Steg, P. G., Smith, S. C., Eagle, K., Ohman, E. M., Goto, S., et al. (2015). Impact of Diabetes Mellitus on Hospitalization for Heart Failure, Cardiovascular Events, and Death. Circulation 132 (10), 923–931. doi:10.1161/CIRCULATIONAHA.114.014796
Ceriello, A., Novials, A., Ortega, E., Canivell, S., La Sala, L., Pujadas, G., et al. (2013). Glucagon-like Peptide 1 Reduces Endothelial Dysfunction, Inflammation, and Oxidative Stress Induced by Both Hyperglycemia and Hypoglycemia in Type 1 Diabetes. Diabetes Care 36 (8), 2346–2350. doi:10.2337/dc12-2469
Chiasson, J.-L., Josse, R. G., Gomis, R., Hanefeld, M., Karasik, A., and Laakso, M.STOP-NIDDM Trial Research Group (2003). Acarbose Treatment and the Risk of Cardiovascular Disease and Hypertension in Patients with Impaired Glucose Tolerance. JAMA 290 (4), 486–494. doi:10.1001/jama.290.4.486
Chilton, R., Tikkanen, I., Cannon, C. P., Crowe, S., Woerle, H. J., Broedl, U. C., et al. (2015). Effects of Empagliflozin on Blood Pressure and Markers of Arterial Stiffness and Vascular Resistance in Patients with Type 2 Diabetes. Diabetes Obes. Metab. 17 (12), 1180–1193. doi:10.1111/dom.12572
Chokesuwattanaskul, W., Yaqub, Y., Suarez, J. A., Simoni, J., Simoni, G., Nugent, K. M., et al. (2010). Effect of Pioglitazone on Platelet Aggregation in a Healthy Cohort. Cardiology 116 (4), 253–256. doi:10.1159/000318024
Collier, A., Watson, H. H., Patrick, A. W., Ludlam, C. A., and Clarke, B. F. (1989). Effect of Glycaemic Control, Metformin and Gliclazide on Platelet Density and Aggregability in Recently Diagnosed Type 2 (Non-insulin-dependent) Diabetic Patients. Diabete Metab. 15 (6), 420–425.
Cosentino, F., Grant, P. J., Aboyans, V., Bailey, C. J., Ceriello, A., Delgado, V., et al. ESC Scientific Document Group. 2019 (2020). 2019 ESC Guidelines on Diabetes, Pre-diabetes, and Cardiovascular Diseases Developed in Collaboration with the EASD. Eur. Heart J. 41 (2), 255–323. doi:10.1093/eurheartj/ehz486
Dardik, B., Valentin, M., Schwarztkopf, C., Gutierrez, C., Stevens, D., Russell, M., et al. (2003). NVP-LAF237, a Dipeptidyl Peptidase IV Inhibitor, Improves Glucose Tolerance and Delays Gastric Emptying in Obese Insulin Resistant Cynomolgus Monkeys. Diabetes 52 (Suppl. 1), A322. doi:10.1007/s001250051445
Davì, G., Ciabattoni, G., Consoli, A., Mezzetti, A., Falco, A., Santarone, S., et al. (1999). In vivo formation of 8-Iso-Prostaglandin F2alpha and Platelet Activation in Diabetes Mellitus: Effects of Improved Metabolic Control and Vitamin E Supplementation. Circulation 99 (2), 224–229. doi:10.1161/01.cir.99.2.224
Davì, G., Catalano, I., Averna, M., Notarbartolo, A., Strano, A., Ciabattoni, G., et al. (1990). Thromboxane Biosynthesis and Platelet Function in Type II Diabetes Mellitus. N. Engl. J. Med. 322 (25), 1769–1774. doi:10.1056/NEJM199006213222503
De Bellis, R., Novoa, E., Dol, B., Cazerez, J., Arroyo, G., Balbela, B., et al. (1984). Changes in Platelet Aggregation Caused by Glyburide in Diabetic Patients. Clin. Ther. 6 (3), 335–343.
DeFronzo, R. A., Tripathy, D., Schwenke, D. C., Banerji, M., Bray, G. A., Buchanan, T. A., et al. ACT NOW Study (2011). Pioglitazone for Diabetes Prevention in Impaired GlucoseTolerance. N. Engl. J. Med. 364 (12), 1104–1115. doi:10.1056/NEJMoa1010949
Derosa, G., Cicero, A. F. G., Gaddi, A., Ragonesi, P. D., Piccinni, M. N., Fogari, E., et al. (2005). A Comparison of the Effects of Pioglitazone and Rosiglitazone Combined with Glimepiride on Prothrombotic State in Type 2 Diabetic Patients with the Metabolic Syndrome. Diabetes Res. Clin. Pract. 69 (1), 5–13. Epub 2004 Dec 29. doi:10.1016/j.diabres.2004.10.007
Dimmeler, S., Fleming, I., Fisslthaler, B., Hermann, C., Busse, R., and Zeiher, A. M. (1999). Activation of Nitric Oxide Synthase in Endothelial Cells by Akt-dependent Phosphorylation. Nature 399 (6736), 601–605. doi:10.1038/21224
Dolasık, I., Sener, S. Y., Celebı, K., Aydın, Z. M., Korkmaz, U., and Canturk, Z. (2013). The Effect of Metformin on Mean Platelet Volume in Dıabetıc Patients. Platelets 24 (2), 118–121. doi:10.3109/09537104.2012.674165
Dormandy, J. A., Charbonnel, B., Eckland, D. J., Erdmann, E., Massi-Benedetti, M., Moules, I. K., et al. (2005). Secondary Prevention of Macrovascular Events in Patients with Type 2 Diabetes in the PROactive Study (PROspective pioglitAzone Clinical Trial in macroVascular Events): a Randomised Controlled Trial. The Lancet 366 (9493), 1279–1289. doi:10.1016/S0140-6736(05)67528-9
FDA Drug Safety Communication (2016). FDA Drug Safety Communication : FDA Adds Warnings about Heart Failure Risk to Labels of Type 2 Diabetes Medicines Containing Saxagliptin and Alogliptin, 1–4.
Ferreira, I. A., Mocking, A. I. M., Feijge, M. A. H., Gorter, G., van Haeften, T. W., Heemskerk, J. W. M., et al. (2006). Platelet Inhibition by Insulin Is Absent in Type 2 Diabetes Mellitus. Atvb 26 (2), 417–422. doi:10.1161/01.ATV.0000199519.37089.a0
Fitchett, D., Zinman, B., Wanner, C., Lachin, J. M., Hantel, S., Salsali, A., et al. (2016). Heart Failure Outcomes with Empagliflozin in Patients with Type 2 Diabetes at High Cardiovascular Risk: Results of the EMPA-REG OUTCOMEtrial. Eur. Heart J. 37 (19), 1526–1534. doi:10.1093/eurheartj/ehv728
Formoso, G., De Filippis, E. A., Michetti, N., Di Fulvio, P., Pandolfi, A., Bucciarelli, T., et al. (2008). Decreasedin Vivo Oxidative Stress and Decreased Platelet Activation Following Metformin Treatment in Newly Diagnosed Type 2 Diabetic Subjects. Diabetes Metab. Res. Rev. 24 (3), 231–237. doi:10.1002/dmrr.794
Gaede, P., Vedel, P., Larsen, N., Jensen, G. V., Parving, H. H., and Pedersen, O. (2003). Multifactorial Intervention and Cardiovascular Disease in Patients with Type 2 Diabetes. N. Engl. J. Med. 348 (5), 383–393. doi:10.1056/NEJMoa021778
Gargiulo, P., Caccese, D., Pignatelli, P., Brufani, C., De Vito, F., Marino, R., et al. (2002). Metformin Decreases Platelet Superoxide Anion Production in Diabetic Patients. Diabetes Metab. Res. Rev. 18 (2), 156–159. doi:10.1002/dmrr.282
Geisler, T., Anders, N., Paterok, M., Langer, H., Stellos, K., Lindemann, S., et al. (2007). Platelet Response to Clopidogrel Is Attenuated in Diabetic Patients Undergoing Coronary Stent Implantation. Diabetes Care 30 (2), 372–374. doi:10.2337/dc06-1625
Gerstein, H. C., Gerstein, H. C., Bosch, J., Dagenais, G. R., Díaz, R., Jung, H., et al. (2012). Basal Insulin and Cardiovascular and Other Outcomes in Dysglycemia. N. Engl. J. Med. 367 (4), 319–328. doi:10.1056/NEJMoa1203858
Gerstein, H. C., Gerstein, H. C., Miller, M. E., Byington, R. P., Goff, D. C., Bigger, J. T., et al. (2008). Effects of Intensive Glucose Lowering in Type 2 Diabetes. N. Engl. J. Med. 358 (24), 2545–2559. doi:10.1056/NEJMoa0802743
Gin, H., Freyburger, G., Boisseau, M., and Aubertin, J. (1989). Study of the Effect of Metformin on Platelet Aggregation in Insulin-dependent Diabetics. Diabetes Res. Clin. Pract. 6 (1), 61–67. doi:10.1016/0168-8227(89)90058-2
Gobel, F. L., Norstrom, L. A., Nelson, R. R., Jorgensen, C. R., and Wang, Y. (1978). The Rate-Pressure Product as an Index of Myocardial Oxygen Consumption during Exercise in Patients with Angina Pectoris. Circulation 57 (3), 549–556. doi:10.1161/01.cir.57.3.549
Gogitidze Joy, N., Hedrington, M. S., Briscoe, V. J., Tate, D. B., Ertl, A. C., and Davis, S. N. (2010). Effects of Acute Hypoglycemia on Inflammatory and Pro-atherothrombotic Biomarkers in Individuals with Type 1 Diabetes and Healthy Individuals. Diabetes Care 33 (7), 1529–1535. doi:10.2337/dc09-0354
Gonçalves, L. H., Silva, M. V. F., Duarte, R. C. F., Dusse, L. M. S., Fernandes, A. P., Bosco, A. A., et al. (2014). Acetylsalicylic Acid Therapy: Influence of Metformin Use and Other Variables on Urinary 11-dehydrothromboxane B2 Levels. Clinica Chim. Acta 429, 76–78. doi:10.1016/j.cca.2013.11.028
Green, J. B., Bethel, M. A., Armstrong, P. W., Buse, J. B., Engel, S. S., Garg, J., et al. TECOS Study Group (2015). Effect of Sitagliptin on Cardiovascular Outcomes in Type 2 Diabetes. N. Engl. J. Med. 373 (3), 232–242. doi:10.1056/NEJMoa1501352
Gresele, P., Guglielmini, G., De Angelis, M., Ciferri, S., Ciofetta, M., Falcinelli, E., et al. (2003). Acute, Short-Term Hyperglycemia Enhances Shear Stress-Induced Platelet Activation in Patients with Type II Diabetes Mellitus. J. Am. Coll. Cardiol. 41 (6), 1013–1020. doi:10.1016/s0735-1097(02)02972-8
Gresele, P., Marzotti, S., Guglielmini, G., Momi, S., Giannini, S., Minuz, P., et al. (2010). Hyperglycemia-induced Platelet Activation in Type 2 Diabetes Is Resistant to Aspirin but Not to a Nitric Oxide-Donating Agent. Diabetes Care 33 (6), 1262–1268. doi:10.2337/dc09-2013
Gupta, A. K., Verma, A. K., Kailashiya, J., Singh, S. K., and Kumar, N. (2012). Sitagliptin: Anti-platelet Effect in Diabetes and Healthy Volunteers. Platelets 23 (8), 565–570. doi:10.3109/09537104.2012.721907
Hanefeld, M., Cagatay, M., Petrowitsch, T., Neuser, D., Petzinna, D., and Rupp, M. (2004). Acarbose Reduces the Risk for Myocardial Infarction in Type 2 Diabetic Patients: Meta-Analysis of Seven Long-Term Studies. Eur. Heart J. 25 (1), 10–16. doi:10.1016/s0195-668x(03)00468-8
Hanefeld, M., Chiasson, J. L., Koehler, C., Henkel, E., Schaper, F., and Temelkova-Kurktschiev, T. (2004a). Acarbose Slows Progression of Intima-Media Thickness of the Carotid Arteries in Subjects with Impaired Glucose Tolerance. Stroke 35 (5), 1073–1078. doi:10.1161/01.STR.0000125864.01546.f2
Hanefeld, M., Schaper, F., and Koehler, C. (2008). Effect of Acarbose on Vascular Disease in Patients with Abnormal Glucose Tolerance. Cardiovasc. Drugs Ther. 22 (3), 225–231. doi:10.1007/s10557-008-6091-1
Harmsze, A. M., Van Werkum, J. W., Moral, F., Ten Berg, J. M., Hackeng, C. M., Klungel, O. H., et al. (2011). Sulfonylureas and On-Clopidogrel Platelet Reactivity in Type 2 Diabetes Mellitus Patients. Platelets 22 (2), 98–102. doi:10.3109/09537104.2010.530359
Hetzel, J., Balletshofer, B., Rittig, K., Walcher, D., Kratzer, W., Hombach, V., et al. (2005). Rapid Effects of Rosiglitazone Treatment on Endothelial Function and Inflammatory Biomarkers. Atvb 25 (9), 1804–1809. doi:10.1161/01.ATV.0000176192.16951.9a
Holman, R. R., Coleman, R. L., Chan, J. C. N., Chiasson, J. L., Feng, H., Ge, J., et al. ACE Study Group (2017). Effects of Acarbose on Cardiovascular and Diabetes Outcomes in Patients with Coronary Heart Disease and Impaired Glucose Tolerance (ACE): a Randomised, Double-Blind, Placebo-Controlled Trial. Lancet Diabetes Endocrinol. 5 (11), 877–886. doi:10.1016/S2213-8587(17)30309-1
Holman, R. R., Bethel, M. A., Mentz, R. J., Thompson, V. P., Lokhnygina, Y., Buse, J. B., et al. EXSCEL Study Group (2017a). Effects of Once-Weekly Exenatide on Cardiovascular Outcomes in Type 2 Diabetes. N. Engl. J. Med. 377 (13), 1228–1239. doi:10.1056/NEJMoa1612917
Home, P. D., Pocock, S. J., Beck-Nielsen, H., Curtis, P. S., Gomis, R., Hanefeld, M., et al. RECORD Study Team (2009). Rosiglitazone Evaluated for Cardiovascular Outcomes in Oral Agent Combination Therapy for Type 2 Diabetes (RECORD): a Multicentre, Randomised, Open-Label Trial. The Lancet 373 (9681), 2125–2135. doi:10.1016/S0140-6736(09)60953-3
Home, P. D., Pocock, S. J., Beck-Nielsen, H., Gomis, R., Hanefeld, M., Jones, N. P., et al. RECORD Study Group (2007). Rosiglitazone Evaluated for Cardiovascular Outcomes - an Interim Analysis. N. Engl. J. Med. 357 (1), 28–38. doi:10.1056/NEJMoa073394
Ishizuka, T., Itaya, S., Wada, H., Ishizawa, M., Kimura, M., Kajita, K., et al. (1998). Differential Effect of the Antidiabetic Thiazolidinediones Troglitazone and Pioglitazone on Human Platelet Aggregation Mechanism. Diabetes 47 (9), 1494–1500. doi:10.2337/diabetes.47.9.1494
Itkonen, M. K., Tornio, A., Neuvonen, M., Neuvonen, P. J., Niemi, M., and Backman, J. T. (2016). Clopidogrel Markedly Increases Plasma Concentrations of CYP2C8 Substrate Pioglitazone. Drug Metab. Disposition 44 (8), 1364–1371. doi:10.1124/dmd.116.070375
James, S., Angiolillo, D. J., Cornel, J. H., Erlinge, D., Husted, S., Kontny, F., et al. PLATO Study Group (2010). Ticagrelor vs. Clopidogrel in Patients with Acute Coronary Syndromes and Diabetes: a Substudy from the PLATelet Inhibition and Patient Outcomes (PLATO) Trial. Eur. Heart J. 31 (24), 3006–3016. doi:10.1093/eurheartj/ehq325
Jennings, P. E., Scott, N. A., Saniabadi, A. R., and Belch, J. J. F. (1992). Effects of Gliclazide on Platelet Reactivity and Free Radicals in Type II Diabetic Patients: Clinical Assessment. Metabolism 41 (5 Suppl. 1), 36–39. doi:10.1016/0026-0495(92)90093-p
Joy, N. G., Tate, D. B., Younk, L. M., and Davis, S. N. (2015). Effects of Acute and Antecedent Hypoglycemia on Endothelial Function and Markers of Atherothrombotic Balance in Healthy Humans. Diabetes 64 (7), 2571–2580. doi:10.2337/db14-1729
Kahal, H., Aburima, A., Ungvari, T., Rigby, A. S., Coady, A. M., Vince, R. V., et al. (2015). The Effects of Treatment with Liraglutide on Atherothrombotic Risk in Obese Young Women with Polycystic Ovary Syndrome and Controls. BMC Endocr. Disord. 15, 14. doi:10.1186/s12902-015-0005-6
Kaplar, M., Kappelmayer, J., Veszpremi, A., Szabo, K., and Udvardy, M. (2001). The Possible Association of In Vivo Leukocyte-Platelet Heterophilic Aggregate Formation and the Development of Diabetic Angiopathy. Platelets 12 (7), 419–422. doi:10.1080/09537100120078368
Kato, E. T., Silverman, M. G., Mosenzon, O., Zelniker, T. A., Cahn, A., Furtado, R. H. M., et al. (2019). Effect of Dapagliflozin on Heart Failure and Mortality in Type 2 Diabetes Mellitus. Circulation 139 (22), 2528–2536. doi:10.1161/CIRCULATIONAHA.119.040130
King, P., Peacock, I., and Donnelly, R. (1999). The UK Prospective Diabetes Study (UKPDS): Clinical and Therapeutic Implications for Type 2 Diabetes. Br. J. Clin. Pharmacol. 48 (5), 643–648. doi:10.1046/j.1365-2125.1999.00092.x
Klaff, L. J., Kernoff, L., Vinik, A. I., Jackson, W. P. U., and Jacobs, P. (1981). Sulfonylureas and Platelet Function. Am. J. Med. 70 (3), 627–630. doi:10.1016/0002-9343(81)90585-4
Konya, H., Hasegawa, Y., Hamaguchi, T., Satani, K., Umehara, A., Katsuno, T., et al. (2010). Effects of Gliclazide on Platelet Aggregation and the Plasminogen Activator Inhibitor Type 1 Level in Patients with Type 2 Diabetes Mellitus. Metabolism 59 (9), 1294–1299. doi:10.1016/j.metabol.2009.12.004
Kooy, A., de Jager, J., Lehert, P., Bets, D., Wulffelé, M. G., Donker, A. J. M., et al. (2009). Long-term Effects of Metformin on Metabolism and Microvascular and Macrovascular Disease in Patients with Type 2 Diabetes Mellitus. Arch. Intern. Med. 169 (6), 616–625. doi:10.1001/archinternmed.2009.20
Krentz, A. J., Ferner, R. E., and Bailey, C. J. (1994). Comparative Tolerability Profiles of Oral Antidiabetic Agents. Drug Saf. 11 (4), 223–241. doi:10.2165/00002018-199411040-00002
Krijnen, P. A. J., Hahn, N. E., Kholová, I., Baylan, U., Sipkens, J. A., Alphen, F. P., et al. (2012). Loss of DPP4 Activity Is Related to a Prothrombogenic Status of Endothelial Cells: Implications for the Coronary Microvasculature of Myocardial Infarction Patients. Basic Res. Cardiol. 107 (1), 233. doi:10.1007/s00395-011-0233-5
Kristensen, S. L., Rørth, R., Jhund, P. S., Docherty, K. F., Sattar, N., Preiss, D., et al. (2019). Cardiovascular, Mortality, and Kidney Outcomes with GLP-1 Receptor Agonists in Patients with Type 2 Diabetes: a Systematic Review and Meta-Analysis of Cardiovascular Outcome Trials. Lancet Diabetes Endocrinol. 7 (10), 776–785. doi:10.1016/S2213-8587(19)30249-9
Lamanna, C., Monami, M., Marchionni, N., and Mannucci, E. (2011). Effect of Metformin on Cardiovascular Events and Mortality: a Meta-Analysis of Randomized Clinical Trials. Diabetes Obes. Metab. 13 (3), 221–228. doi:10.1111/j.1463-1326.2010.01349.x
Lescano, C. H., Leonardi, G., Torres, P. H. P., Amaral, T. N., de Freitas Filho, L. H., Antunes, E., et al. (2020). The Sodium-Glucose Cotransporter-2 (SGLT2) Inhibitors Synergize with Nitric Oxide and Prostacyclin to Reduce Human Platelet Activation. Biochem. Pharmacol. 182, 114276. doi:10.1016/j.bcp.2020.114276
Li, D., Chen, K., Sinha, N., Zhang, X., Wang, Y., Sinha, A., et al. (2005). The Effects of PPAR-? Ligand Pioglitazone on Platelet Aggregation and Arterial Thrombus Formation. Cardiovasc. Res. 65 (4), 907–912. doi:10.1016/j.cardiores.2004.11.027
Li, Y., Li, R., Feng, Z., Wan, Q., and Wu, J. (2020). Linagliptin Regulates the Mitochondrial Respiratory Reserve to Alter Platelet Activation and Arterial Thrombosis. Front. Pharmacol. 11, 585612. doi:10.3389/fphar.2020.585612
Maeda, S., Matsui, T., and Yamagishi, S.-i. (2012). Vildagliptin Inhibits Oxidative Stress and Vascular Damage in Streptozotocin-Induced Diabetic Rats. Int. J. Cardiol. 158 (1), 171–173. doi:10.1016/j.ijcard.2012.04.087
Malmberg, K. (1997). Prospective Randomised Study of Intensive Insulin Treatment on Long Term Survival after Acute Myocardial Infarction in Patients with Diabetes Mellitus. BMJ 314 (7093), 1512. doi:10.1136/bmj.314.7093.1512
Marso, S. P., Daniels, G. H., Brown-Frandsen, K., Kristensen, P., Mann, J. F. E., Nauck, M. A., et al. LEADER Steering Committee (2016). Liraglutide and Cardiovascular Outcomes in Type 2 Diabetes. N. Engl. J. Med. 375 (4), 311–322. doi:10.1056/NEJMoa1603827
Maruthur, N. M., Tseng, E., Hutfless, S., Wilson, L. M., Suarez-Cuervo, C., Berger, Z., et al. (2016). Diabetes Medications as Monotherapy or Metformin-Based Combination Therapy for Type 2 Diabetes. Ann. Intern. Med. 164 (11), 740–751. doi:10.7326/M15-2650
Marx, N., Rosenstock, J., Kahn, S. E., Zinman, B., Kastelein, J. J., Lachin, J. M., et al. (2015). Design and Baseline Characteristics of the CARdiovascular Outcome Trial of LINAgliptin versus Glimepiride in Type 2 Diabetes (CAROLINA). Diabetes Vasc. Dis. Res. 12 (3), 164–174. doi:10.1177/1479164115570301
Mason, R. P., Jacob, R. F., Kubant, R., Walter, M. F., Bellamine, A., Jacoby, A., et al. (2011). Effect of Enhanced Glycemic Control with Saxagliptin on Endothelial Nitric Oxide Release and CD40 Levels in Obese Rats. Jat 18 (9), 774–783. doi:10.5551/jat.7666
Mather, K., Anderson, T. J., and Verma, S. (2001). Insulin Action in the Vasculature: Physiology and Pathophysiology. J. Vasc. Res. 38 (5), 415–422. doi:10.1159/000051074
Matsubara, J., Sugiyama, S., Sugamura, K., Nakamura, T., Fujiwara, Y., Akiyama, E., et al. (2012). A Dipeptidyl Peptidase-4 Inhibitor, Des-Fluoro-Sitagliptin, Improves Endothelial Function and Reduces Atherosclerotic Lesion Formation in Apolipoprotein E-Deficient Mice. J. Am. Coll. Cardiol. 59 (3), 265–276. doi:10.1016/j.jacc.2011.07.053
McGuire, D. K., Alexander, J. H., Johansen, O. E., Perkovic, V., Rosenstock, J., Cooper, M. E., et al. (2019). Linagliptin Effects on Heart Failure and Related Outcomes in Individuals with Type 2 Diabetes Mellitus at High Cardiovascular and Renal Risk in CARMELINA. Circulation 139 (3), 351–361. doi:10.1161/CIRCULATIONAHA.118.038352
McInnes, G., Evans, M., Del Prato, S., Stumvoll, M., Schweizer, A., Lukashevich, V., et al. (2015). Cardiovascular and Heart Failure Safety Profile of Vildagliptin: a Meta-Analysis of 17 000 Patients. Diabetes Obes. Metab. 17 (11), 1085–1092. doi:10.1111/dom.12548
McMurray, J. J. V., Ponikowski, P., Bolli, G. B., Lukashevich, V., Kozlovski, P., Kothny, W., et al. (2018). Effects of Vildagliptin on Ventricular Function in Patients with Type 2 Diabetes Mellitus and Heart Failure. JACC: Heart Fail. 6 (1), 8–17. doi:10.1016/j.jchf.2017.08.004
Monami, M., Genovese, S., and Mannucci, E. (2013). Cardiovascular Safety of Sulfonylureas: a Meta-Analysis of Randomized Clinical Trials. Diabetes Obes. Metab. 15 (10), 938–953. doi:10.1111/dom.12116
Mongan, J., Mieszczanska, H. Z., Smith, B. H., Messing, S. P., Phipps, R. P., and Francis, C. W. (2012). Pioglitazone Inhibits Platelet Function and Potentiates the Effects of Aspirin: a Prospective Observation Study. Thromb. Res. 129 (6), 760–764. doi:10.1016/j.thromres.2011.12.019
Mudaliar, S., Alloju, S., and Henry, R. R. (2016). Can a Shift in Fuel Energetics Explain the Beneficial Cardiorenal Outcomes in the EMPA-REG OUTCOME Study? A Unifying Hypothesis. Dia Care 39 (7), 1115–1122. doi:10.2337/dc16-0542
Mulvihill, E. E., and Drucker, D. J. (2014). Pharmacology, Physiology, and Mechanisms of Action of Dipeptidyl Peptidase-4 Inhibitors. Endocr. Rev. 35 (6), 992–1019. doi:10.1210/er.2014-1035
Murcia, A. M., Hennekens, C. H., Lamas, G. A., Jiménez-Navarro, M., Rouleau, J. L., Flaker, G. C., et al. (2004). Impact of Diabetes on Mortality in Patients with Myocardial Infarction and Left Ventricular Dysfunction. Arch. Intern. Med. 164 (20), 2273–2279. doi:10.1001/archinte.164.20.2273
Nathan, D. M.DCCT/EDIC Research Group (2014). The Diabetes Control and Complications Trial/epidemiology of Diabetes Interventions and Complications Study at 30 years: Overview. Dia Care 37 (1), 9–16. doi:10.2337/dc13-2112
Neal, B., Perkovic, V., Mahaffey, K. W., de Zeeuw, D., Fulcher, G., Erondu, N., et al. CANVAS Program Collaborative Group (2017). Canagliflozin and Cardiovascular and Renal Events in Type 2 Diabetes. N. Engl. J. Med. 377 (7), 644–657. doi:10.1056/NEJMoa1611925
Nesbitt, W. S., Westein, E., Tovar-Lopez, F. J., Tolouei, E., Mitchell, A., Fu, J., et al. (2009). A Shear Gradient-dependent Platelet Aggregation Mechanism Drives Thrombus Formation. Nat. Med. 15 (6), 665–673. doi:10.1038/nm.1955
Nissen, S. E., and Wolski, K. (2007). Effect of Rosiglitazone on the Risk of Myocardial Infarction and Death from Cardiovascular Causes. N. Engl. J. Med. 356 (24), 2457–2471. doi:10.1056/NEJMoa072761
Nusca, A., Tuccinardi, D., Albano, M., Cavallaro, C., Ricottini, E., Manfrini, S., et al. (2018). Glycemic variability in the development of cardiovascular complications in diabetes. Diabetes Metab. Res. Rev. 34, e3047. doi:10.1002/dmrr.3047
Nusca, A., Tuccinardi, D., Proscia, C., Melfi, R., Manfrini, S., Nicolucci, A., et al. (2019). Incremental role of glycaemic variability over HbA1c in identifying type 2 diabetic patients with high platelet reactivity undergoing percutaneous coronary intervention. Cardiovasc. Diabetol. 18, 147. doi:10.1186/s12933-019-0952-8
Oeseburg, H., de Boer, R. A., Buikema, H., van der Harst, P., van Gilst, W. H., and Silljé, H. H. W. (2010). Glucagon-like Peptide 1 Prevents Reactive Oxygen Species-Induced Endothelial Cell Senescence through the Activation of Protein Kinase A. Atvb 30 (7), 1407–1414. doi:10.1161/ATVBAHA.110.206425
Orchard, T. J., Nathan, D. M., Zinman, B., Cleary, P., Brillon, D., Backlund, J.-Y. C., et al. (2015). Association between 7 Years of Intensive Treatment of Type 1 Diabetes and Long-Term Mortality. JAMA 313 (1), 45–53. doi:10.1001/jama.2014.16107
Packer, M. (2019). Reconceptualization of the Molecular Mechanism by Which Sodium-Glucose Cotransporter 2 Inhibitors Reduce the Risk of Heart Failure Events. Circulation 140 (6), 443–445. doi:10.1161/CIRCULATIONAHA.119.040909
Patti, G., Nusca, A., Mangiacapra, F., Gatto, L., D'Ambrosio, A., and Di Sciascio, G. (2008). Point-of-Care Measurement of Clopidogrel Responsiveness Predicts Clinical Outcome in Patients Undergoing Percutaneous Coronary Intervention. J. Am. Coll. Cardiol. 52 (14), 1128–1133. doi:10.1016/j.jacc.2008.06.038
Pham, S. V., and Chilton, R. J. (2017). EMPA-REG OUTCOME: The Cardiologist's Point of View. Am. J. Cardiol. 120 (1S), S53–S58. doi:10.1016/j.amjcard.2017.05.011
Pordzik, J., Jakubik, D., Jarosz-Popek, J., Wicik, Z., Eyileten, C., De Rosa, S., et al. (2019). Significance of Circulating microRNAs in Diabetes Mellitus Type 2 and Platelet Reactivity: Bioinformatic Analysis and Review. Cardiovasc. Diabetol. 18 (1), 113. doi:10.1186/s12933-019-0918-x
Potenza, M. A., Addabbo, F., and Montagnani, M. (2009). Vascular Actions of Insulin with Implications for Endothelial Dysfunction. Am. J. Physiology-Endocrinology Metab. 297 (3), E568–E577. doi:10.1152/ajpendo.00297.2009
Proks, P., Reimann, F., Green, N., Gribble, F., and Ashcroft, F. (2002). Sulfonylurea Stimulation of Insulin Secretion. Diabetes 51 (Suppl. 3), S368–S376. doi:10.2337/diabetes.51.2007.s368
Rados, D. V., Pinto, L. C., Remonti, L. R., Leitão, C. B., and Gross, J. L. (2016). The Association between Sulfonylurea Use and All-Cause and Cardiovascular Mortality: A Meta-Analysis with Trial Sequential Analysis of Randomized Clinical Trials. Plos Med. 13 (4), e1001992. doi:10.1371/journal.pmed.1001992
Rao, A. D., Kuhadiya, N., Reynolds, K., and Fonseca, V. A. (2008). Is the Combination of Sulfonylureas and Metformin Associated with an Increased Risk of Cardiovascular Disease or All-Cause Mortality?: a Meta-Analysis of Observational Studies. Diabetes Care 31 (8), 1672–1678. doi:10.2337/dc08-0167
Rivas Rios, J. R., Franchi, F., Rollini, F., and Angiolillo, D. J. (2018). Diabetes and antiplatelet therapy: from bench to bedside. Cardiovasc. Diagn. Ther. 8, 594–609. doi:10.21037/cdt.2018.05.09
Rosenstock, J., Perkovic, V., Perkovic, V., Alexander, J. H., Cooper, M. E., Marx, N., et al. (2018). Rationale, Design, and Baseline Characteristics of the CArdiovascular Safety and Renal Microvascular outcomE Study with LINAgliptin (CARMELINA): a Randomized, Double-Blind, Placebo-Controlled Clinical Trial in Patients with Type 2 Diabetes and High Cardio-Renal Risk. Cardiovasc. Diabetol. 17 (1), 39. doi:10.1186/s12933-018-0682-3
Russo, I., Massucco, P., Mattiello, L., Cavalot, F., Anfossi, G., and Trovati, M. (2002). Comparison between the Effects of the Rapid Recombinant Insulin Analog Aspart and Those of Human Regular Insulin on Platelet Cyclic Nucleotides and Aggregation. Thromb. Res. 107 (1–2), 31–37. doi:10.1016/s0049-3848(02)00182-2
Sacks, D., Baxter, B., Campbell, B. C. V., Carpenter, J. S., Cognard, C., Dippel, D., et al. (2018). Multisociety Consensus Quality Improvement Revised Consensus Statement for Endovascular Therapy of Acute Ischemic Stroke: From the American Association of Neurological Surgeons (AANS), American Society of Neuroradiology (ASNR), Cardiovascular and Interventional Radiology Society of Europe (CIRSE), Canadian Interventional Radiology Association (CIRA), Congress of Neurological Surgeons (CNS), European Society of Minimally Invasive Neurological Therapy (ESMINT), European Society of Neuroradiology (ESNR), European Stroke Organization (ESO), Society for Cardiovascular Angiography and Interventions (SCAI), Society of Interventional Radiology (SIR), Society of NeuroInterventional Surgery (SNIS), and World Stroke Organization (WSO). J. Vasc. Interv. Radiol. 29 (6), 441–453. doi:10.1177/174749301877871310.1016/j.jvir.2017.11.026
Sano, M., Takei, M., Shiraishi, Y., and Suzuki, Y. (2016). Increased Hematocrit during Sodium-Glucose Cotransporter 2 Inhibitor Therapy Indicates Recovery of Tubulointerstitial Function in Diabetic Kidneys. J. Clin. Med. Res. 8 (12), 844–847. doi:10.14740/jocmr2760w
Santilli, F., Formoso, G., Sbraccia, P., Averna, M., Miccoli, R., Di Fulvio, P., et al. (2010). Postprandial Hyperglycemia Is a Determinant of Platelet Activation in Early Type 2 Diabetes Mellitus. J. Thromb. Haemost. 8 (4), 828–837. doi:10.1111/j.1538-7836.2010.03742.x
Santos-Gallego, C. G., Zafar, M., Antonio, R. S., Ibanez, J. A. R., Botija, M. B. P., Ishikawa, K., et al. (2018). The Sglt2 Inhibitor Empagliflozin Does Not Exhibit Pro Thrombotic Effects. J. Am. Coll. Cardiol. 71 (11 Suppl.), A1852. doi:10.1016/S0735-1097(18)32393-3
Satoh, K., Ozaki, Y., Yatomi, Y., and Kume, S. (1994). Effects of Sulfonylurea Agents on Platelet Arachidonic Acid Metabolism; Study on Platelet Homogenates. Biochem. Pharmacol. 48 (5), 1053–1055. doi:10.1016/0006-2952(94)90379-4
Schäfer, A., Widder, J., Eigenthaler, M., Bischoff, H., Ertl, G., and Bauersachs, J. (2004). Increased Platelet Activation in Young Zucker Rats with Impaired Glucose Tolerance Is Improved by Acarbose. Thromb. Haemost. 92 (1), 97–103. doi:10.1160/TH04-02-0118
Scheen, A. J. (2013). Cardiovascular Effects of Gliptins. Nat. Rev. Cardiol. 10 (2), 73–84. doi:10.1038/nrcardio.2012.183
Schöndorf, T., Musholt, P. B., Hohberg, C., Forst, T., Lehmann, U., Fuchs, W., et al. (2011). The Fixed Combination of Pioglitazone and Metformin Improves Biomarkers of Platelet Function and Chronic Inflammation in Type 2 Diabetes Patients: Results from the PIOfix Study. J. Diabetes Sci. Technol. 5 (2), 426–432. doi:10.1177/193229681100500233
Scirica, B. M., Bhatt, D. L., Braunwald, E., Steg, P. G., Davidson, J., Hirshberg, B., et al. (2013). Saxagliptin and Cardiovascular Outcomes in Patients with Type 2 Diabetes Mellitus. N. Engl. J. Med. 369 (14), 1317–1326. doi:10.1056/NEJMoa1307684
Scognamiglio, R., Avogaro, A., Vigili de Kreutzenberg, S., Negut, C., Palisi, M., Bagolin, E., et al. (2002). Effects of Treatment with Sulfonylurea Drugs or Insulin on Ischemia-Induced Myocardial Dysfunction in Type 2 Diabetes. Diabetes 51 (3), 808–812. doi:10.2337/diabetes.51.3.808
Selvin, E., Bolen, S., Yeh, H.-C., Wiley, C., Wilson, L. M., Marinopoulos, S. S., et al. (2008). Cardiovascular Outcomes in Trials of Oral Diabetes Medications. Arch. Intern. Med. 168 (19), 2070–2080. doi:10.1001/archinte.168.19.2070
Sharma, A., Green, J. B., Dunning, A., Lokhnygina, Y., Al-Khatib, S. M., Lopes, R. D., et al. TECOS Study Group (2017). Causes of Death in a Contemporary Cohort of Patients with Type 2 Diabetes and Atherosclerotic Cardiovascular Disease: Insights from the TECOS Trial. Dia Care 40 (12), 1763–1770. doi:10.2337/dc17-1091
Shimazu, T., Inami, N., Satoh, D., Kajiura, T., Yamada, K., Iwasaka, T., et al. (2009). Effect of Acarbose on Platelet-Derived Microparticles, Soluble Selectins, and Adiponectin in Diabetic Patients. J. Thromb. Thrombolysis 28 (4), 429–435. doi:10.1007/s11239-008-0301-3
Sidhu, J. S., Cowan, D., and Kaski, J.-C. (2003). The Effects of Rosiglitazone, a Peroxisome Proliferator-Activated Receptor-Gamma Agonist, on Markers of Endothelial Cell Activation, C-Reactive Protein, and Fibrinogen Levels in Non-diabetic Coronary Artery Disease Patients. J. Am. Coll. Cardiol. 42 (10), 1757–1763. doi:10.1016/j.jacc.2003.04.001
Smyth, E., Solomon, A., Birrell, M. A., Smallwood, M. J., Winyard, P. G., Tetley, T. D., et al. (2017). Influence of Inflammation and Nitric Oxide upon Platelet Aggregation Following Deposition of Diesel Exhaust Particles in the Airways. Br. J. Pharmacol. 174 (13), 2130–2139. doi:10.1111/bph.13831
Spectre, G., Östenson, C.-G., Li, N., and Hjemdahl, P. (2012). Postprandial Platelet Activation Is Related to Postprandial Plasma Insulin rather Than Glucose in Patients with Type 2 Diabetes. Diabetes 61 (9), 2380–2384. doi:10.2337/db11-1806
Spectre, G., Stålesen, R., Östenson, C.-G., and Hjemdahl, P. (2016). Meal-induced Platelet Activation in Diabetes Mellitus Type 1 or Type 2 Is Related to Postprandial Insulin rather Than Glucose Levels. Thromb. Res. 141, 93–97. doi:10.1016/j.thromres.2016.03.009
Spigoni, V., Fantuzzi, F., Carubbi, C., Pozzi, G., Masselli, E., Gobbi, G., et al. (2020). Sodium-glucose Cotransporter 2 Inhibitors Antagonize Lipotoxicity in Human Myeloid Angiogenic Cells and ADP-dependent Activation in Human Platelets: Potential Relevance to Prevention of Cardiovascular Events. Cardiovasc. Diabetol. 19 (1), 46. doi:10.1186/s12933-020-01016-5
Stegenga, M. E., van der Crabben, S. N., Levi, M., de Vos, A. F., Tanck, M. W., Sauerwein, H. P., et al. (2006). Hyperglycemia Stimulates Coagulation, whereas Hyperinsulinemia Impairs Fibrinolysis in Healthy Humans. Diabetes 55 (6), 1807–1812. doi:10.2337/db05-1543
Steven, S., Jurk, K., Kopp, M., Kröller-Schön, S., Mikhed, Y., Schwierczek, K., et al. (2017). Glucagon-like Peptide-1 Receptor Signalling Reduces Microvascular Thrombosis, Nitro-Oxidative Stress and Platelet Activation in Endotoxaemic Mice. Br. J. Pharmacol. 174 (12), 1620–1632. doi:10.1111/bph.13549
Steven, S., Oelze, M., Hanf, A., Kröller-Schön, S., Kashani, F., Roohani, S., et al. (2017a). The SGLT2 Inhibitor Empagliflozin Improves the Primary Diabetic Complications in ZDF Rats. Redox Biol. 13, 370–385. doi:10.1016/j.redox.2017.06.009
Suryadevara, S., Ueno, M., Tello-Montoliu, A., Ferreiro, J., Desai, B., Rollini, F., et al. (2012). Effects of Pioglitazone on Platelet P2Y12-Mediated Signalling in Clopidogrel-Treated Patients with Type 2 Diabetes Mellitus. Thromb. Haemost. 108 (5), 930–936. doi:10.1160/TH12-06-0397
Tancredi, M., Rosengren, A., Svensson, A.-M., Kosiborod, M., Pivodic, A., Gudbjörnsdottir, S., et al. (2015). Excess Mortality Among Persons with Type 2 Diabetes. N. Engl. J. Med. 373 (18), 1720–1732. doi:10.1056/NEJMoa1504347
Ting, H. J., and Khasawneh, F. T. (2010). Glybenclamide: an Antidiabetic with In Vivo Antithrombotic Activity. Eur. J. Pharmacol. 649 (1–3), 249–254. doi:10.1016/j.ejphar.2010.09.009
Tremoli, E., Ghiselli, G., Maderna, P., Colli, S., and Sirtori, C. R. (1982). Metformin Reduces Platelet Hypersensitivity in Hypercholesterolemic Rabbits. Atherosclerosis 41 (1), 53–60. doi:10.1016/0021-9150(82)90069-7
Udell, J. A., Bhatt, D. L., Braunwald, E., Cavender, M. A., Mosenzon, O., Steg, P. G., et al. (2015). Saxagliptin and Cardiovascular Outcomes in Patients with Type 2 Diabetes Mellitus and Moderate or Severe Renal Impairment: Observations from the SAVOR-TIMI 53 Trial. Dia Care 38 (4), dc141850–705. doi:10.2337/dc14-1850
Ueda, P., Svanström, H., Melbye, M., Eliasson, B., Svensson, A.-M., Franzén, S., et al. (2018). Sodium Glucose Cotransporter 2 Inhibitors and Risk of Serious Adverse Events: Nationwide Register Based Cohort Study. BMJ 363, k4365. doi:10.1136/bmj.k4365
Ussher, J. R., and Drucker, D. J. (2012). Cardiovascular Biology of the Incretin System. Endocr. Rev. Endocr Rev 33 (2), 187–215. doi:10.1210/er.2011-1052
Verdoia, M., Pergolini, P., Rolla, R., Ceccon, C., Caputo, M., Aimaretti, G., et al. (2021). Use of Metformin and Platelet Reactivity in Diabetic Patients Treated with Dual Antiplatelet Therapy. Exp. Clin. Endocrinol. Diabetes 129 (1), 43–49. doi:10.1055/a-0787-1382
Vinik, A. I., Erbas, T., Park, T. S., Nolan, R., and Pittenger, G. L. (2001). Platelet Dysfunction in Type 2 Diabetes. Diabetes Care 24 (8), 1476–1485. doi:10.2337/diacare.24.8.1476
Violi, F., De Mattia, G. C., Alessandri, C., Perrone, A., and Vezza, E. (1982). The Effects of Gliclazide on Platelet Function in Patients with Diabetes Mellitus. Curr. Med. Res. Opin. 8 (3), 200–203. doi:10.1185/03007998209112384
Viscoli, C. M., Brass, L. M., Carolei, A., Conwit, R., Ford, G. A., Furie, K. L., et al. IRIS Trial investigators (2014). Pioglitazone for Secondary Prevention after Ischemic Stroke and Transient Ischemic Attack: Rationale and Design of the Insulin Resistance Intervention after Stroke Trial. Am. Heart J. 168 (6), 823–829. doi:10.1016/j.ahj.2014.07.016
Wada, H., Ishizuka, T., Itaya, S., Yamada, K., Kajita, K., Kimura, M., et al. (1999). Inhibitory Effect of Glyburide on Thrombin-Induced Platelet Aggregation and Phosphoinositide Metabolism in Normal Human Platelets. Platelets 10 (1), 45–51. doi:10.1080/09537109976347
Wang, G.-R., Zhu, Y., Halushka, P. V., Lincoln, T. M., and Mendelsohn, M. E. (1998). Mechanism of Platelet Inhibition by Nitric Oxide: In Vivo Phosphorylation of Thromboxane Receptor by Cyclic GMP-dependent Protein Kinase. Proc. Natl. Acad. Sci. 95 (9), 4888–4893. doi:10.1073/pnas.95.9.4888
White, W. B., Cannon, C. P., Heller, S. R., Nissen, S. E., Bergenstal, R. M., Bakris, G. L., et al. (2013). Alogliptin after Acute Coronary Syndrome in Patients with Type 2 Diabetes. N. Engl. J. Med. 369 (14), 1327–1335. doi:10.1056/NEJMoa1305889
Williams, R., de Vries, F., Kothny, W., Serban, C., Lopez-Leon, S., Chu, C., et al. (2017). Cardiovascular Safety of Vildagliptin in Patients with Type 2 Diabetes: A European Multi-Database, Non-interventional Post-authorization Safety Study. Diabetes Obes. Metab. 19 (10), 1473–1478. doi:10.1111/dom.12951
Wiviott, S. D., Braunwald, E., Angiolillo, D. J., Meisel, S., Dalby, A. J., Verheugt, F. W. A., et al. (2008). Greater Clinical Benefit of More Intensive Oral Antiplatelet Therapy with Prasugrel in Patients with Diabetes Mellitus in the Trial to Assess Improvement in Therapeutic Outcomes by Optimizing Platelet Inhibition with Prasugrel-Thrombolysis in Myocardial Infarction 38. Circulation 118 (16), 1626–1636. doi:10.1161/CIRCULATIONAHA.108.791061
Wiviott, S. D., Raz, I., Bonaca, M. P., Mosenzon, O., Kato, E. T., Cahn, A., et al. (2018). The Design and Rationale for the Dapagliflozin Effect on Cardiovascular Events (DECLARE)-TIMI 58 Trial. Am. Heart J. 200, 83–89. doi:10.1016/j.ahj.2018.01.012
Wiviott, S. D., Raz, I., Bonaca, M. P., Mosenzon, O., Kato, E. T., Cahn, A., et al. (2019). Dapagliflozin and Cardiovascular Outcomes in Type 2 Diabetes. N. Engl. J. Med. 380 (4), 347–357. doi:10.1056/NEJMoa1812389
Xin, G., Wei, Z., Ji, C., Zheng, H., Gu, J., Ma, L., et al. (2016). Metformin Uniquely Prevents Thrombosis by Inhibiting Platelet Activation and mtDNA Release. Sci. Rep. 6, 36222. doi:10.1038/srep36222
Yaribeygi, H., Atkin, S. L., Butler, A. E., and Sahebkar, A. (2019). Sodium-glucose Cotransporter Inhibitors and Oxidative Stress: An Update. J. Cel Physiol. 234 (4), 3231–3237. doi:10.1002/jcp.26760
Yau, H., Rivera, K., Lomonaco, R., and Cusi, K. (2013). The Future of Thiazolidinedione Therapy in the Management of Type 2 Diabetes Mellitus. Curr. Diab Rep. 13 (3), 329–341. doi:10.1007/s11892-013-0378-8
Zelniker, T. A., Wiviott, S. D., Raz, I., Im, K., Goodrich, E. L., Bonaca, M. P., et al. (2019). SGLT2 Inhibitors for Primary and Secondary Prevention of Cardiovascular and Renal Outcomes in Type 2 Diabetes: a Systematic Review and Meta-Analysis of Cardiovascular Outcome Trials. The Lancet 393 (10166), 31–39. doi:10.1016/S0140-6736(18)32590-X
Zheng, M.-y., Yang, J.-h., Shan, C.-y., Zhou, H.-t., Xu, Y.-g., Wang, Y., et al. (2013). Effects of 24-week Treatment with Acarbose on Glucagon-like Peptide 1 in Newly Diagnosed Type 2 Diabetic Patients: a Preliminary Report. Cardiovasc. Diabetol. 12, 73. doi:10.1186/1475-2840-12-73
Zheng, S. L., Roddick, A. J., Aghar-Jaffar, R., Shun-Shin, M. J., Francis, D., Oliver, N., et al. (2018). Association between Use of Sodium-Glucose Cotransporter 2 Inhibitors, Glucagon-like Peptide 1 Agonists, and Dipeptidyl Peptidase 4 Inhibitors with All-Cause Mortality in Patients with Type 2 Diabetes. JAMA 319 (15), 1580–1591. doi:10.1001/jama.2018.3024
Zilov, A. V., Abdelaziz, S. I., AlShammary, A., Al Zahrani, A., Amir, A., Assaad Khalil, S. H., et al. (2019). Mechanisms of Action of Metformin with Special Reference to Cardiovascular Protection. Diabetes Metab. Res. Rev. 35 (7), e3173. doi:10.1002/dmrr.3173
Keywords: anti-diabetic therapies, glucose-lowering drugs, platelet, anti-platelet agents, cardiovascular disease, thrombotic risk, diabetes
Citation: Nusca A, Tuccinardi D, Pieralice S, Giannone S, Carpenito M, Monte L, Watanabe M, Cavallari I, Maddaloni E, Ussia GP, Manfrini S and Grigioni F (2021) Platelet Effects of Anti-diabetic Therapies: New Perspectives in the Management of Patients with Diabetes and Cardiovascular Disease. Front. Pharmacol. 12:670155. doi: 10.3389/fphar.2021.670155
Received: 20 February 2021; Accepted: 16 April 2021;
Published: 12 May 2021.
Edited by:
Daniele Torella, University of Catanzaro, ItalyReviewed by:
Antonella De Angelis, University of Campania Luigi Vanvitelli, ItalyEleonora Cianflone, University of Catanzaro, Italy
Copyright © 2021 Nusca, Tuccinardi, Pieralice, Giannone, Carpenito, Monte, Watanabe, Cavallari, Maddaloni, Ussia, Manfrini and Grigioni. This is an open-access article distributed under the terms of the Creative Commons Attribution License (CC BY). The use, distribution or reproduction in other forums is permitted, provided the original author(s) and the copyright owner(s) are credited and that the original publication in this journal is cited, in accordance with accepted academic practice. No use, distribution or reproduction is permitted which does not comply with these terms.
*Correspondence: Annunziata Nusca, YS5udXNjYUB1bmljYW1wdXMuaXQ=
†These authors have contributed equally to this work and share first authorship