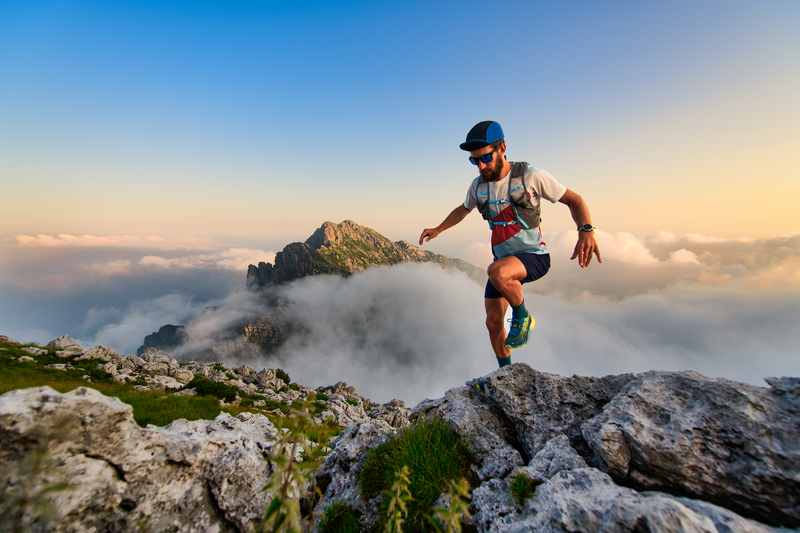
94% of researchers rate our articles as excellent or good
Learn more about the work of our research integrity team to safeguard the quality of each article we publish.
Find out more
ORIGINAL RESEARCH article
Front. Pharmacol. , 18 May 2021
Sec. Experimental Pharmacology and Drug Discovery
Volume 12 - 2021 | https://doi.org/10.3389/fphar.2021.655659
Human carboxylesterase 2 (CES2), one of the most abundant hydrolases distributed in the small intestine, has been validated as a key therapeutic target to ameliorate the intestinal toxicity caused by irinotecan. This study aims to discover efficacious CES2 inhibitors from natural products and to characterize the inhibition potentials and inhibitory mechanisms of the newly identified CES2 inhibitors. Following high-throughput screening and evaluation of the inhibition potency of more than 100 natural products against CES2, it was found that the biflavones isolated from Ginkgo biloba displayed extremely potent CES2 inhibition activities and high specificity over CES1 (>1000-fold). Further investigation showed that ginkgetin, bilobetin, sciadopitysin and isoginkgetin potently inhibited CES2-catalyzed hydrolysis of various substrates, including the CES2 substrate-drug irinotecan. Notably, the inhibition potentials of four biflavones against CES2 were more potent than that of loperamide, a marketed anti-diarrhea agent used for alleviating irinotecan-induced intestinal toxicity. Inhibition kinetic analyses demonstrated that ginkgetin, bilobetin, sciadopitysin and isoginkgetin potently inhibited CES2-catalyzed fluorescein diacetate hydrolysis via a reversible and mixed inhibition manner, with Ki values of less than 100 nM. Ensemble docking and molecular dynamics revealed that these biflavones could tightly and stably bind on the catalytic cavity of CES2 via hydrogen bonding and π-π stacking interactions, while the interactions with CES1 were awfully poor. Collectively, this study reports that the biflavones isolated from Ginkgo biloba are potent and highly specific CES2 inhibitors, which offers several promising lead compounds for developing novel anti-diarrhea agent to alleviate irinotecan-induced diarrhea.
Mammalian carboxylesterases (CES) are key members of serine hydrolases which play key roles in activation of ester prodrugs and hydrolytic metabolism of a variety of xenobiotics bearing ester bond(s) (Hosokawa, 2008; Hatfield et al., 2016). Human carboxylesterase 1 (CES1) and human carboxylesterase 2 (CES2) as two predominant CES isoforms that have been extensively investigated over the past few decades. The CES1 is primarily expressed in the liver, while CES2 is mainly expressed in the small intestine (Wang D. et al., 2018). The clinically drugs including temocapril, oseltamivir, and sacubitril have been reported as the CES1 substrate drugs, while CES2 has been revealed as a key enzyme responsible for the activation of several important anti-tumor prodrugs, such as irinotecan and capecitabine (Potter et al., 1998; Quinney et al., 2005; Shi et al., 2006; Ohura et al., 2011; Shi et al., 2016; Chen et al., 2018). It has been reported that CES2 participated in approximately 99% of the total conversion of irinotecan into SN-38 in the human small intestine (Humerickhouse et al., 2000; Charasson et al., 2004). Increasing evidence has indicated that co-administration with potent CES2 inhibitors may ameliorate irinotecan-induced severe diarrhea via blocking the overproduction of SN38 (the cytotoxic product of CPT-11) in human small intestine (Slatter et al., 1997; Hecht, 1998; Hicks et al., 2009). Currently, loperamide (LPA, a marketed anti-diarrhea agent) has been used for alleviating irinotecan-induced intestinal toxicity in clinical settings (Tobin et al., 2005; Baker, 2007). However, the moderate CES2 inhibition potency, as well as the potential adverse effects of LPA (such as headache, dizziness, constipation, nausea and flatulence), strongly limited the long-term administration of this anti-diarrhea agent (Hanauer, 2008; Eggleston et al., 2017; Miller et al., 2017; White, 2019). Therefore, it is urgent and necessary to find more efficacious CES2 inhibitors to reduce the excessive hydrolysis of CPT-11 into its toxic product SN-38 in the intestinal tract.
Over the past few decades, with the help of the highly specific substrates (including drug substrates and optical substrate) for CES2, a wide variety of natural products (such as tanshinones, triterpenoids and flavonoids) with strong to moderate CES2 inhibition activities were discovered (Zhu et al., 2015; Zou et al., 2016; Weng et al., 2018; Zhao et al., 2020). However, most reported naturally occurring CES2 inhibitors are moderate inhibitors (IC50 values are at the micromole levels), which motivates us to find more efficacious CES2 inhibitors. Recently, we have carried out high-throughput screening campaign to evaluate the inhibitory potentials of more than one hundred natural products on CES2. Among all tested natural products, four biflavones (ginkgetin, bilobetin, sciadopitysin and isoginkgetin) isolated from Ginkgo biloba leaves were found with the most potent CES2 inhibition activities (Figure 1 and Figure 2). It is well-known that the biflavones isolated from Ginkgo biloba leaves have been reported with many beneficial effects, such as anti-oxidative and anti-inflammatory, as well as anti-cancer and potential chemopreventive effects (Chang et al., 2018; Chen et al., 2019; Tao et al., 2019; Souza et al., 2020). Thus, the biflavones hold great promise for alleviating irinotecan-induced intestinal toxicity. Unfortunately, the inhibition potentials and inhibitory mechanisms of the biflavones against CES2 have not been well-characterized yet.
FIGURE 1. The lg (IC50/nM) of more than 100 kinds of natural products and positive inhibitor (LPA) on CES2. All data were shown as mean ± SD of triplicate assays.
This study aimed to assess the inhibition potentials and specificity of four biflavones isolated from Ginkgo biloba against CES2, as well as to characterize the inhibitory mechanisms of these biflavones against CES2. For these purposes, a set of inhibition assays were used to assess the inhibition potentials and specificity of four biflavones against CES2, inhibition kinetic analyses, ensemble docking and molecular dynamics simulations were used to explore the action mechanisms of these four biflavones against CES2. All these studies will be advantageous for deciphering the interactions of four biflavones with CES2 inhibition, while the key findings present here will be promising to develop novel CES2 inhibitors for ameliorating CES2-associated drug toxicity.
Ginkgetin, bilobetin, sciadopitysin and isoginkgetin were ordered from Shanghai Standard Biotech Co., Ltd. (Shanghai, China). Loperamide (LPA) and fluorescein diacetate (FD) were purchased from TCI (Tokyo, Japan). Irinotecan (CPT-11) and corresponding active hydrolytic metabolite SN-38 were obtained from Alfa Aesar (Beijing, China) and HEOWNS (Tianjing, China), respectively. The purities of all tested biflavones were identified by LC-UV and the data indicated that the purities of tested biflavones were higher than 98% totally. The 4-methylumbelliferyl-acetate (4-MUA) was obtained from Sigma Chemical (Poole, Dorset, United Kingdom). Recombinant CES1 (Batch No.150006A), recombinant CES2 (Batch No.153015A). the pooled human liver microsomes from 50 individual donors (HLMs) and Pooled human intestinal microsomes (HIMs, lot no. X02801) were bought from Bioreclamation IVT (Baltimore, MD, United States) and stored at −80°C. The stock solution of each compound (100 mM) was dissolved with dimethyl sulfoxide (LC grade, Tedia, United States of America), which were stored at 4°C. LC grade acetonitrile (Tedia, United States), phosphate buffered saline (0.1 M, pH 6.8 and pH 7.4) and ultrapure water (produced by a Millipore water system) were used in following experiments.
The incubation system contains following components (total volume 200 μL): 2 μL DMSO/inhibitor, 2 μL HLMs (2 μg/ml, final concentration), 194 μL buffer PBS (pH = =7.4). Three concentrations of each inhibitor (1 μM, 10 μM and 100 μM) were established for preliminary screening. Two control groups were set up at the same time, one was the inhibitor-free system (adding equal volume of DMSO) represented the 100% enzyme activity, the other was the positive inhibitor (LPA). The incubation system pre-incubated at 37°C for 3 min minutes and 2 μL fluorescent substrate FD (5 μM, final concentration) was added for fluorescence analysis in fully automated fluorescent microplate reader (SpectraMax M4, Molecular Devices) (Wang et al., 2011).
CPT-11, a known CES2 substrate-drug, was also used to ascertain whether the identified four biflavones displayed strong inhibition on CES2-catalyzed CPT-11 hydrolysis. Experiments were performed in Eppendorf Tubes (EP, total volume 200 μL): 2 μL DMSO/inhibitor, 2 μL HLMs/HIMs (200 μg/ml, final concentration), and 194 μL PBS buffer (pH = 7.4). Two control groups were set up concurrently, an inhibitor-free (adding equal volume of DMSO) system regarded as 100% enzyme activity, and the other one with the positive inhibitor (LPA). The incubation system pre-incubated at 37°C for 3 min followed by adding 2 μL substrate CPT-11 (5 μM, final concentration), after incubated for another 50 min, reactions were terminated with an equal volume of 10% perchloric acid-ice acetonitrile (1:2). The mixture was centrifuged at 20,000 × g, supernatants were collected for follow-up analysis in LC system (Shao et al., 2012). Chromatographic separation was performed on a shim-pack GIST-HP C18 analytical column (50 mm × 2.1 mm, 3 μm particle size), using ammonium acetate (50 mmol/L, PH = 4), acetonitrile as mobile phase. The detection wavelength of CPT-11 was Ex/Em = 368 nm/432 nm, and the detection wavelength of SN38 was 368 nm/535 nm (Shao et al., 2012).
The incubation system contains following components (total volume 200 μL): 2 μL DMSO/inhibitor, 2 μL recombinant CES1 or recombinant CES2 (5 μg/ml, final concentration), 194 μL buffer PBS (pH = 7.4). Two control groups were set up simultaneously, one was no inhibitor system (adding equal volume of DMSO) regarded as 100% enzyme activity, and the other was the positive inhibitor (nevadensin for CES1 and LPA for CES2) (Wang Y. Q. et al., 2018; Abigerges et al., 1994). The incubation system pre-incubated at 37°C for 3 min and 2 μL substrate 4-MUA (100 μM, final concentration) was added for fluorescence analysis in fully automated fluorescent microplate reader (SpectraMax M4, Molecular Devices) (Gabriele et al., 2018).
The inhibition constant (Ki) and the inhibition modes of four potency inhibitors against CES2-catalyzed FD hydrolysis were explored by utilizing a cluster of kinetic assays for FD hydrolysis at the presence of incremental concentrations of each biflavone (Cheng and Prusoff, 1973). The Michaelis-Menten equations was adopted and transformed to render the Lineweaver-Burk plots, while the slopes of Lineweaver-Burk plots were applied to calculate the inhibition constant (Ki) values on the basis of kinetic data.
Due to the lack of crystal structure of CES2, a modeling structure of CES2 was generated using homologous modeling method by SWISS-MODEL (https://swissmodel.expasy.org/) based on the template structure of CES1 (PDB code: 5A7G) with the resolution of 1.48 Å (Bencharit et al., 2003; Andrew et al., 2018). QMEAN scoring function score of -2.30 validated the reliability of structure model (Benkert et al., 2008). In addition, CES1 and CES2 pertain to serine hydrolase family that catalyze the hydrolysis of numerous endogenous substances and xenobiotics (Zou et al., 2018). The catalytic residue serine is positioned in a deep gorge surrounded by multiple hydrophobic residues (Briand et al., 2019). Account for the flexibility of the catalytic pocket, structurally diverse compounds can be hydrolyzed (Zou et al., 2018). Consequently, comprehending the fluctuation was essential for docking calculation, and ensemble docking approach was applied to predict the binding energies and energetically preferred binding modes (Stoddard et al., 2010). Hence, we performed 1 μs molecular dynamics simulations of CES1 and CES2 to extensively sample the proteins’ conformations, and clustered the representative protein conformations with a tolerance of 2.5 Å root-mean-square deviations (RMSDs) using gromos method. As a result, 8 and 13 representative protein structures were obtained for CES1 and CES2, respectively. We presumed that four biflavones may have similar binding modes due to the similar skeletons, therefore, bilobetin with the most potent inhibitory potency for CES2 was selected to investigate the binding mode and selectivity. Taking the representative protein structures as receptors and bilobetin as ligand, molecular docking was conducted with AutoDock Vina (Version 1.1.2) based on Lamarckian genetic algorithm (Morris et al., 1998; Trott and Olson, 2010). Hydrogen atoms were added followed by assigning the Kollman charges. The center of grid box was set to 60 × 60 × 60 Å3 with the spacing of 0.375, enclosing the active site. Favorable binding conformations with the lowest binding energy were indicated for the follow-up calculation.
To further investigate the binding stability of the CES2-bilobetin complex, two binding modes with the best binding affinity (predicted by the ensemble docking as mentioned above) were selected as the initial conformations for molecular dynamics simulations by using Gromacs (Version 2019.3) software (Berendsen et al., 1995; Abraham et al., 2015). The force fields and simulation parameters were exploited in terms of previous research (Song et al., 2019a). Trajectory postprocessing and visualization were performed by Gromacs package and VMD (Version 1.9.3) (Humphrey et al., 1996). Finally, the induced-fit equilibrium state was selected as template to dock with the other three biflavones (Koshland, 1995). The detailed interactions against CES2 were further analyzed and compared.
All data were shown as mean ± SD of triplicate assays. The GraphPad Prism 8.0 was applied to determine the inhibition constants (including IC50 and Ki values).
In order to discover more efficacious CES2 inhibitors from natural products, more than 100 natural products were collected and their inhibition potentials against CES2 were assayed in HLMs under identical incubation conditions. For each tested compound, at least eight inhibitor concentrations were used to determine the IC50 values, by using FD as a fluorescent substrate for CES2. The IC50 values of 120 natural products against CES2-catalyzed FD hydrolysis ranged from 20 to 188,000 nM, which were listed in Supplementary Table S1. To compare the inhibition potentials of these natural products in a more intuitive way, the logarithmic transformation of the IC50 values in nanomolar were depicted in Figure 1. The dots below the horizontal dashed line represented that these inhibitors displayed relatively strong inhibitory effects on CES2 (less than 1000 nM). Delightfully, it was evident from Figure 1 that four biflavones isolated from Ginkgo biloba displayed the most potent inhibition against CES2-catalyzed FD in HLMs, with the calculated IC50 values to be 20.08, 24.20, 34.46 and 32.24 nM, for ginkgetin, bilobetin, sciadopitysin and isoginkgetin, respectively (Figures 1, 3). It should be noted that the inhibition potency of these four biflavones were much more potent than that of some reported naturally occurring CES2 inhibitors (such as magnolol, glabridin, Supplementary Table S1) and the positive inhibitor LPA (IC50 = 6240 nM, Supplementary Table S1) (Song et al., 2019a; Song et al., 2019b). These finding inspired us to further assess the inhibition potentials of these four biflavones against CES2-catalyzed CPT-11 hydrolysis.
FIGURE 3. Dose-inhibition curves of ginkgetin (A), bilobetin (B), sciadopitysin (C) and isoginkgetin (D) on CES2 using FD as the probe substrate in HLMs. All data were shown as mean ± SD of triplicate assays.
Furthermore, the inhibitory effects of four biflavones on CES2-catalyzed irinotecan hydrolysis were also investigated in HLMs (200 μg/ml, final concentration). As listed in Table 1, four biflavones could strongly inhibit CES2-catalyzed irinotecan hydrolysis in a dose-dependent manner (Figure 4). The apparent IC50 values of these four biflavones against CES2-catalyzed irinotecan hydrolysis in HLMs were also determined as 1.95, 0.64, 2.86 and 0.44 μM respectively (Figure 4; Table 1), which were much lower than that inhibition of LPA on CES2-catalyzed irinotecan hydrolysis (IC50 = 5.35 μM, Supplementary Figure S1). Notably, owing to the extremely low hydrolytic rate of irinotecan in HLM, the high concentration of microsomal protein was used for such inhibition assay which caused larger IC50 values of four tested biflavones against CES2-catalyzed irinotecan hydrolysis in HLMs. The higher IC50 value of four biflavones on CES2-catalyzed irinotecan hydrolysis in HLMs could be partially attributed to the high binding rates of these biflavones on the microsomal protein, which led to the low free concentrations of these tested biflavones in the incubation system (Austin et al., 2002). Furthermore, the inhibitory effect of four biflavones on CES2-mediated irinotecan hydrolysis was also investigated in HIMs. As listed in Figure 5, four biflavones could strongly inhibited CES2-mediated irinotecan hydrolysis in HIMs, with the apparent IC50 value of 0.3, 0.18, 0.29 and 0.94 μM, respectively. These results suggest that the biflavones from Ginkgo biloba are potent CES2 inhibitors against CES2-catalyzed hydrolysis of both optical substrate and substrate drug (irinotecan), which encourages us to further investigate the specificity and inhibitory mechanisms of these four biflavones against CES2.
TABLE 1. The inhibition parameters of four biflavones from Ginkgo biloba against CES2-catalyzed CPT-11 hydrolysis.
FIGURE 4. Dose-inhibition curves of ginkgetin (A), bilobetin (B), sciadopitysin (C) and isoginkgetin (D) on CES2 using CPT-11 as the probe substrate in HLMs. All data were shown as mean ± SD of triplicate assays.
FIGURE 5. Dose-inhibition curves of ginkgetin (A), bilobetin (B), sciadopitysin (C) and isoginkgetin (D) on CES2 using CPT-11 as the probe substrate in HIMs. All data were shown as mean ± SD of triplicate assays.
Next, the isoform specificity of these four biflavones against CES2 was assayed by using 4-MUA (a co-substrate for mammalian CES) as the substrate. As shown in Figure 6; Table 2, the inhibition of ginkgetin, bilobetin, sciadopitysin and isoginkgetin against CES2-catalyzed 4-MUA hydrolysis in recombinant CES2 were extremely potent, with the IC50 values as low as determined as 0.08, 0.14, 0.10 and 0.07 µM, respectively. Meanwhile, the inhibitory effects of four biflavones against CES1-catalyzed 4-MUA hydrolysis in recombinant CES1 have also been determined, and the results showed that the IC50 values were larger than 100 μM (Table 2). These findings clearly suggest that these four biflavones are isoform-specific inhibitors of CES2 over CES1.
FIGURE 6. Dose-inhibition curves of ginkgetin (A), bilobetin (B), sciadopitysin (C) and isoginkgetin (D) on CES2 using 4-MUA as the probe substrate in recombinant CES2. All data were shown as mean ± SD of triplicate assays.
TABLE 2. The inhibitory effects of four biflavones from Ginkgo biloba against CES-catalyzed 4-MUA hydrolysis.
Next, the inhibitory mechanisms of four newly identified biflavone-type inhibitors were explored by performing a set of inhibition kinetic assays. Firstly, the IC50 shift assays were used to identify whether these biflavones were time-dependent inhibitors or reversible inhibitors against CES2 (Chen et al., 2016). As shown in Figure 7, the inhibition curves of these biflavones with different pre-incubation times (3 min or 33 min) were similar with each other, suggesting that biflavones were reversible inhibitors against CES2. Subsequently, a set of inhibition kinetic assays of these four biflavones against CES2-catalyzed FD hydrolysis were performed and the corresponding Lineweaver-Burk plots were depicted. As shown in Figure 8; Table 3, inhibition kinetic assays clearly demonstrated ginkgetin, bilobetin, sciadopitysin and isoginkgetin inhibited CES2-catalyzed FD hydrolysis in HLMs via a mixed inhibition manner, with Ki values of 32.85, 26.23, 68.77 and 32.10 nM, respectively. These findings suggest that these newly identified biflavone-type inhibitors exhibit relatively high affinity toward CES2.
FIGURE 7. The residual activity of CES2-catalyzed hydrolysis of FD in the presence of different concentrations of biflavones from Ginkgo biloba at different pre-incubation times of 3 and 33 min. All data were shown as mean ± SD of triplicate assays.
FIGURE 8. The inhibition kinetic and Lineweaver-Burk plots of ginkgetin (A,B), bilobetin (C,D), sciadopitysin (E,F) and isoginkgetin (G,H) against CES2-catalyzed FD hydrolysis in HLMs. All data were shown as mean ± SD of triplicate assays.
TABLE 3. The inhibition parameters and inhibition modes on four biflavones isolated from Ginkgo biloba against CES2-catalyzed FD hydrolysis in HLMs.
Comparing to the conventional docking simulations, ensemble docking approach can significantly improve the predictive performance (Amaro et al., 2018). To explore the mechanisms of the newly identified biflavones against CES2 over CES1 at the molecular level, ensemble docking simulations of bilobetin into CES2 and CES1 were performed firstly. As shown in Supplementary Figure S2; Supplementary Table S1, bilobetin could be well-docked into the catalytic cavity of CES2, with the binding energies of −9.7 kcal/mol and −10.8 kcal/mol of the top two preferred binding modes. By contrast, the lowest binding energy (−5.0 kcal/mol) of bilobetin toward CES1 was far above the CES2-bilobetin complex. These findings agreed well with the specificity of bilobetin on CES2 over CES1.
Next, molecular dynamic simulations were used to explore the stability of the top two preferred binding modes of the CES2-bilobetin complex. As depicted in Figure 9, mode 1 could form an equilibrium state during 100 ns simulation, while mode 2 underwent large fluctuations. This finding suggested that mode 1 represented a relatively stable CES2-bilobetin complex, in which a molecule of bilobetin stably bound on the catalytic cavity of CES2. Similarly, the other three biflavones could occupy the catalytic cavity of CES2 in a similar way, which could explain why these biflavones exhibited potent CES2 inhibition activity (Figure 10). The interactions between CES2 and four biflavones were also summarized, as shown in Figure 11; Table 4. For ginkgetin, this agent created strong interactions with Glu105, Glu103, Lys352, Glu227, Tyr159 and Asn342 via hydrogen bonding, also interacted with Phe106 and Trp348 via π-π stacking. Similariy, bilobetin created strong interactions with Met453, Asn344, Trp348, Glu103, Glu105, Val102, Ala150, Glu227 and Asn342 via hydrogen bonding, and interacted with Trp348 and Met453 via π-π stacking, and π-Sulfur interaction, respectively. For sciadopitysin, four key residues (Ser228, Gly347, Val102, and Glu103) surrounding to the catalytic cavity of CES2 were involved to form hydrogen bonds with this agent, while this agent could also interact with Phe469 and Trp348 via π-π stacking. Isoginkgetin created strong interactions with a panel of residues (Asn343, Asn342, Trp348, Glu103, Val102, Ala455) in CES2 via forming hydrogen bonds, while this agent also interacted with Trp348 and Phe153 via π-π stacking, and with Asp456 via amide-π stacking. Notably, Trp348 could interact with all tested biflavones via π-π stacking, indicating that this residue may play a crucial role in binding on CES2. In addition, we discussed the relationship of virtual screening scores and activities against 13 representative structures obtained from clustering of molecular dynamics simulations (Supplementary Figures S3, S4). As shown in Supplementary Table S3, the overall effect was not ideal, the highest Spearman R coefficient was 0.1474. Evaluating the best binding energies and average binding energies of total structures, the former approached the optimal coefficient. In summary, the 13 receptor conformations are inadequate to capture a more diverse panel of the 120 compounds. Hence, conformations in an ensemble should endow disparate weights and more precise scoring methods are required for virtual screening of hCES2 inhibitors.
FIGURE 9. RMSD vs. time plot of bilobetin originated from the two different binding modes toward CES2 by the ensemble docking method. Mode 1 reached an equilibrium state, while mode 2 underwent large fluctuations.
FIGURE 10. Aligned binding poses of four biflavones against CES2. The catalytic residue Ser228 was shown in cyan spheres in aerial view, ginkgetin, bilobetin, sciadopitysin, isoginkgetin were depicted with blue, yellow, gray and green, respectively. The active cavity of CES2 was displayed in red mesh.
FIGURE 11. Interaction analysis and spatial arrangement with ginkgetin (A,B), bilobetin (C,D), sciadopitysin (E,F), isoginkgetin (G,H) were proposed in 2D (left) and 3D (right) diagrams. Active site and pivotal residues were exhibited in red mesh and cyan sticks in stereo view.
As one of the most popular used herbal products, the extract from Ginkgo biloba leaves has been widely used for preparing dietary supplements and herbal medicines in both Eastern and Western countries. Over the past few decades, many studies around the world have demonstrated that Ginkgo biloba extract and its major constitutes may trigger clinically relevant herb-drug interactions via inhibition on drug-metabolizing enzymes (Wang et al., 2019). For example, Ginkgo biloba extract significantly inhibited a panel of human P450 enzymes, including CYP3A4 and CYP2C9. It has been reported that Ginkgo biloba extract may trigger clinically relevant herb-drug interactions (HDI) and bring bleeding or other side effects, when this herb is co-administrated with warfarin, an anticoagulant agent that is metabolized by CYP3A4 and CYP2C9 (Yang et al., 2006). These findings suggest that Ginkgo biloba extract and its major constitutes are able to modulate the pharmacokinetic properties of co-administrated drugs via modulating the function of drug-metabolizing enzymes. Considering that Ginkgo biloba extract are frequently used over the world and the recommended daily dose is very high (12 g/d), of which total biflavones were about 48.9 mg/d. The metabolic interactions of Ginkgo biloba ingredients with human drug-metabolizing enzymes and the potential risks should be carefully investigated (Qian and Markowitz, 2020).
Although the metabolic interactions of Ginkgo biloba ingredients with CYPs or UGTs have been investigated, the inhibitory effects of Ginkgo biloba ingredients on CES, an important class of non-CYP enzymes participating in hydrolysis of ester drugs, have not been studied yet. In this study, the inhibitory effects of abundant biflavones from Ginkgo biloba on CES2 were carefully investigated for the first time. Our finding clearly demonstrated that the biflavones isolated from Ginkgo biloba are potent and highly specific inhibitors of CES2 over CES1. Considering that CES2 plays crucial roles in hydrolytic metabolism of a wide range of therapeutic agents bearing ester or amide bond(s), much attention should be paid when Ginkgo biloba extract or Ginkgo biloba-containing dietary supplements are co-administrated with CES2 substrate-drugs. For example, CES2 can hydrolyze the antitumor drug LY2334737 (Pratt et al., 2013), the prodrug of Gemcitabine, potent inhibition of CES2 may impair the antitumor potency of LY2334737. Besides, cocaine can be hydrolyzed to its inactive metabolite by CES2 (Wu et al., 2003), strong inhibition of CES2 may slow down the metabolic clearance of these substrate-drugs in vivo, and then modulate its treatment outcomes.
Taking into account that intestinal CES2 is one of the key targets involving in CPT-11 induced life-threatening diarrhea, Ginkgo biloba leaves extract or Ginkgo biloba-related dietary supplements can be used for ameliorating such intestinal toxicity via strong inhibition on intestinal CES2. In this study, our results clearly demonstrated that the biflavones isolated from Ginkgo biloba displayed potent inhibitory effects against CES2, which might partially block the over-accumulation of SN-38 in the small intestine and then ameliorate CPT-11 associated life-threatening diarrhea. In view of the tissue distribution of two CES isoforms (CES2 is abundantly expressed in the small intestine but CES1 is abundantly expressed in the liver), as well as the poor oral bioavailability of the biflavones in Ginkgo biloba, it is easily conceivable that the local exposure of these biflavones will be much higher than that in the liver, following oral administration of Ginkgo biloba leaves extract. It should be noted that CES2 inhibitors may affect CPT-11 hydrolysis in the liver, which in turn, reducing the plasma exposure and the in vivo anti-tumor efficacy of SN38. Thus, an ideal CES2 inhibitor is expected to reduce SN-38 formation in the intestinal tract, but does not affect the hydrolysis of CPT-11 in the human liver. In this study, our findings demonstrated that these biflavones displayed very high specificity toward CES2 over CES1. In these cases, the intestinal CES2 is more likely inhibited by these biflavones but CES1 (a known serine hydrolase responsible for lipid metabolism) in the liver is hardly inhibited by these natural products.
From the perspective of drug development, biflavones are promising lead compounds for the development of novel anti-diarrhea agents for ameliorating irinotecan-induced intestinal toxicity via targeting CES2, owing to these compounds display potent CES2 inhibition activities, excellent isoform-specificity, good safety profiles and high local exposure in the gastrointestinal tract. Furthermore, it has been reported that the biflavones isolated from Ginkgo biloba leaves possess a range of bioactivities (such as anti-oxidative, anti-inflammatory), which are also beneficial for the patients with irinotecan-induced intestinal toxicity. In near future, to develop more efficacious biflavone-type CES2 inhibitors, the naturally occurring biflavones could be further modified by the medicinal chemists, to improve the CES2 inhibition potency, safety, and drug-likeness properties (as oral drugs). It is well-known that the biflavones are abundantly distributed in a range of medicinal plants (such as Ginkgo biloba, St. John’s wort) (van Beek and Montoro, 2009; Hou et al., 2019), while the scheme for total synthesis of biflavones has also been reported (Li et al., 1997; Ndoile and van Heerden, 2013). Thus, the structurally diverse biflavones could be easily obtained for the structure-activity relationship studies and the pharmacological assays. Structurally, the biflavones isolated from Ginkgo biloba leaves bear several phenolic groups, in which the C-7 phenolic group plays a crucial role CES2 inhibition (via forming a hydrogen bond with the residues in the catalytic cavity of CES2). Thus, the key interactions between the C-7 phenolic group in these biflavones and the catalytic cavity of CES2 should be conserved in the development of novel biflavone-type CES2 inhibitors.
In summary, this study reported that four biflavones isolated from Ginkgo biloba displayed potent CES2 inhibition potency and high specificity over CES1 (>1000-fold). These biflavones showed potent inhibition potentials CES2-catalyzed hydrolysis of various substrates, including the CES2 substrate-drug irinotecan, while their inhibition potentials were more potent than that of loperamide (a marketed anti-diarrhea agent used for alleviating irinotecan-induced intestinal toxicity). Inhibition kinetic analyses showed that ginkgetin, bilobetin, sciadopitysin and isoginkgetin potently inhibited CES2-meidated FD hydrolysis via a reversible and mixed inhibition manner, with Ki values of less than 100 nM. Furthermore, ensemble docking and molecular dynamics give deeper insights into the molecular mechanisms of these newly identified CES2 inhibitors. All these findings clearly demonstrated that the biflavones isolated from Ginkgo biloba were potent and reversible CES2 inhibitors, which offered several promising lead compounds for the medicinal chemists to develop novel CES2 inhibitors for some specific purposes, such as modulating the pharmacokinetic profiles of CES2-substrate drugs and alleviating irinotecan-induced diarrhea.
The raw data supporting the conclusion of this article will be made available by the authors, without undue reservation.
GG, SF, WQ and YS conceived the study and designed the experimental process. RH and XG contributed to the ensemble docking and molecular dynamics analysis. DP, JS and YL performed the bio-assays experiments; YS, JH and SJ carried out data analysis and supervised this study; GG and YS wrote the manuscript and interpretation of data.
This work was financially supported by the NSF of China (81922070, 81973286, 81973168, 82003847), Cultivation Project of Clinical Research of Shanghai ShenkangHospital Development Center (SHDC12018X30), Natural Science Foundation of Shanghai Science and Technology Commission (19ZR1452100, 20ZR 1459300), Key Program of Yueyang Hospital of Shanghai University of Traditional Chinese Medicine (2019YYZ01), the Three-year Action Plan of Shanghai TCM Development (ZY-(2018-2020)-CCCX-5001), Program of Shanghai Academic/Technology Research Leader (18XD1403600), Shanghai Talent Development Fund (2019093) and Innovative Leading Talents of Qinghai Province (2018 & 2019).
The authors declare that the research was conducted in the absence of any commercial or financial relationships that could be construed as a potential conflict of interest.
The Supplementary Material for this article can be found online at: https://www.frontiersin.org/articles/10.3389/fphar.2021.655659/full#supplementary-material.
CES, carboxylesterases; CES2, human carboxylesterases 2; CES1, human carboxylesterases 1; FD, fluorescein diacetate; CPT-11, Irinotecan; LPA, loperamide; DMSO, dimethyl sulfoxide; HLMs, human liver microsomes; HIMs, human intestinal microsomes; IC50, Half the maximum inhibitory concentration; Ki, Inhibition constant; PBS, phosphate buffered saline; SN-38, 7-Ethyl-10-hydroxy-camptothecin; 4-MUA, 4-methylumbelliferyl acetate; EP, eppendorf tubes; RMSDs, root-mean-square deviations; MD, molecular dynamics simulation; CYPs, cytochrome P450 enzymes; UGTs, uridine diphosphate-glucuronosyltransferases.
Abigerges, D., Armand, J. P., Chabot, G. G., Da Costa, L., Fadel, E., Cote, C., et al. (1994). Irinotecan (CPT-11) High-Dose Escalation Using Intensive High-Dose Loperamide to Control Diarrhea. J. Natl. Cancer 86 (6), 446–449. doi:10.1093/jnci/86.6.446
Abraham, M., Murtola, T., Schulz, R., Páll, S., Smith, J. C., Hess, B., et al. (2015). GROMACS: High Performance Molecular Simulations through Multi-Level Parallelism from Laptops to Supercomputers. SoftwareX 1, 19–25. doi:10.1016/J.SOFTX.2015.06.001
Amaro, R. E., Baudry, J., Chodera, J., Demir, O., McCammon, J. A., Miao, Y., et al. (2018). Ensemble Docking in Drug Discovery. Biophys. J. 114 (10), 2271–2278. doi:10.1016/j.bpj.2018.02.038
Andrew, W., Martino, B., Stefan, B., Gabriel, S., Gerardo, T., Rafal, G., et al. (2018). SWISS-MODEL: Homology Modelling of Protein Structures and Complexes. Nucleic Acids Res. 46, W296–W303.
Austin, R. P., Barton, P., Cockroft, S. L., Wenlock, M. C., and Riley, R. J. (2002). The Influence of Nonspecific Microsomal Binding on Apparent Intrinsic Clearance, and its Prediction from Physicochemical Properties. Drug Metab. Dispos 30 (12), 1497–1503. doi:10.1124/dmd.30.12.1497
Baker, D. E. (2007). Loperamide: a Pharmacological Review. Rev. Gastroenterol. Disord. 7 (Suppl. 3), S11–S18.
Bencharit, S., Morton, C. L., Xue, Y., Potter, P. M., and Redinbo, M. R. (2003). Structural Basis of Heroin and Cocaine Metabolism by a Promiscuous Human Drug-Processing Enzyme. Nat. Struct. Biol. 10 (5), 349–356. doi:10.1038/nsb919
Benkert, P., Tosatto, S. C., and Schomburg, D. (2008). QMEAN: A Comprehensive Scoring Function for Model Quality Assessment. Proteins 71 (1), 261–277. doi:10.1002/prot.21715
Berendsen, H. J. C., Vanderspoel, D., and Vandrunen, R. (1995). Gromacs - a Message-Passing Parallel Molecular-Dynamics Implementation. Comput. Phys. Commun. 91 (1-3), 43–56. doi:10.1016/0010-4655(95)00042-E
Briand, E., Thomsen, R., Linnet, K., Rasmussen, H. B., Brunak, S., and Taboureau, O. (2019). Combined Ensemble Docking and Machine Learning in Identification of Therapeutic Agents with Potential Inhibitory Effect on Human CES1. Molecules 24 (15), 2747. doi:10.3390/molecules24152747
Chang, L., Liu, T., Chai, Z., Jie, S., Li, Z., Liu, M., et al. (2018). lincRNA-p21 Mediates the Anti-Cancer Effect of Ginkgo Biloba Extract EGb 761 by Stabilizing E-Cadherin Protein in Colon Cancer. Med. Sci. Monit. 24, 9488–9496. doi:10.12659/MSM.911924
Charasson, V., Bellott, R., Meynard, D., Longy, M., Gorry, P., and Robert, J. (2004). Pharmacogenetics of Human Carboxylesterase 2, an Enzyme Involved in the Activation of Irinotecan into SN-38. Clin. Pharmacol. Ther. 76 (6), 528–535. doi:10.1016/j.clpt.2004.08.007
Chen, F., Zhang, B., Parker, R. B., and Laizure, S. C. (2018). Clinical Implications of Genetic Variation in Carboxylesterase Drug Metabolism. Expert Opin. Drug Metab. Toxicol. 14 (2), 131–142. doi:10.1080/17425255.2018.1420164
Chen, T. R., Wei, L. H., Guan, X. Q., Huang, C., Liu, Z. Y., Wang, F. J., et al. (2019). Biflavones from Ginkgo Biloba as Inhibitors of Human Thrombin. Bioorg. Chem. 92, 103199. doi:10.1016/j.bioorg.2019.103199
Chen, Z. H., Zhang, S. X., Long, N., Lin, L. S., Chen, T., Zhang, F. P., et al. (2016). An Improved Substrate Cocktail for Assessing Direct Inhibition and Time-dependent Inhibition of Multiple Cytochrome P450s. Acta Pharmacol. Sin 37 (5), 708–718. doi:10.1038/aps.2016.10
Cheng, Y., and Prusoff, W. H (1973). Relationship between the Inhibition Constant (K1) and the Concentration of Inhibitor Which Causes 50 Per Cent Inhibition (I50) of an Enzymatic Reaction. Biochem. Pharmacol. 22 (23), 3099–3108. doi:10.1016/0006-2952(73)90196-2
Eggleston, W., Clark, K. H., and Marraffa, J. M. (2017). Loperamide Abuse Associated with Cardiac Dysrhythmia and Death. Ann. Emerg. Med. 69 (1), 83–86. doi:10.1016/j.annemergmed.2016.03.047
Gabriele, M., Puccini, P., Lucchi, M., Vizziello, A., Gervasi, P., and Longo, V. (2018). Presence and Inter-Individual Variability of Carboxylesterases (CES1 and CES2) in Human Lung. Biochem. Pharmacol. 150, 64–71. doi:10.1016/j.bcp.2018.01.028
Hanauer, S. B. (2008). The Role of Loperamide in Gastrointestinal Disorders. Rev. Gastroenterol. Disord. 8 (1), 15–20.
Hatfield, M. J., Umans, R. A., Hyatt, J. L., Edwards, C. C., Wierdl, M., Tsurkan, L., et al. (2016). Carboxylesterases: General Detoxifying Enzymes. Chem. Biol. Interact. 259 (Pt B), 327–331. doi:10.1016/j.cbi.2016.02.011
Hecht, J. R. (1998). Gastrointestinal Toxicity or Irinotecan. Oncology (Williston Park) 12 (8 Suppl. 6), 72–78.
Hicks, L. D., Hyatt, J. L., Stoddard, S., Tsurkan, L., Edwards, C. C., Wadkins, R. M., et al. (2009). Improved, Selective, Human Intestinal Carboxylesterase Inhibitors Designed to Modulate 7-Ethyl-10-[4-(1-Piperidino)-1-Piperidino]carbonyloxycamptothecin (Irinotecan; CPT-11) Toxicity. J. Med. Chem. 52 (12), 3742–3752. doi:10.1021/jm9001296
Hosokawa, M. (2008). Structure and Catalytic Properties of Carboxylesterase Isozymes Involved in Metabolic Activation of Prodrugs. Molecules 13 (2), 412–431. doi:10.3390/molecules13020412
Hou, X. D., Guan, X., Cao, Y. F., Weng, Z. M., Hu, Q., Liu, H., et al. (2019). Inhibition of Pancreatic Lipase by the Constituents in St. John's Wort: In vitro and In Silico Investigations. Int. J. Biol. Macromol. 145, 620–633. doi:10.1016/j.ijbiomac.2019.12.231
Humerickhouse, R., Lohrbach, K., Li, L., Bosron, W. F., and Dolan, M. E. (2000). Characterization of CPT-11 Hydrolysis by Human Liver Carboxylesterase Isoforms hCE-1 and hCE-2. Cancer Res. 60 (5), 1189–1192.
Humphrey, W., Dalke, A., and Schulten, K. (1996). VMD: Visual Molecular Dynamics. J. Mol. Graph 14 (1), 33–38. doi:10.1016/0263-7855(96)00018-5
Koshland, D. (1995). The Key–Lock Theory and the Induced Fit Theory. Angew. Chem. 33, 2375–2378. doi:10.1002/ANIE.199423751
Li, H., Nehira, T., Hagiwara, M., and Harada, N. (1997). Total Synthesis and Absolute Stereochemistry of the Natural Atropisomer of the Biflavone 4',4"',7,7"-Tetra-O-Methylcupressuflavone. J. Org. Chem. 62 (21), 7222–7227. doi:10.1021/jo970670w
Miller, H., Panahi, L., Tapia, D., Tran, A., and Bowman, J. D. (2017). Loperamide Misuse and Abuse. J. Am. Pharm. Assoc. 57 (2S), S45–S50. doi:10.1016/j.japh.2016.12.079
Morris, G. M., Goodsell, D. S., Halliday, R. S., Huey, R., Hart, W. E., Belew, R. K., et al. (1998). Automated Docking Using a Lamarckian Genetic Algorithm and an Empirical Binding Free Energy Function. J. Comput. Chem. 19 (14), 1639–1662. doi:10.1002/(Sici)1096-987x(19981115)19:14<1639::Aid-Jcc10>3.0.Co;2-B
Ndoile, M. M., and van Heerden, F. R. (2013). Total Synthesis of Ochnaflavone. Beilstein J. Org. Chem. 9, 1346–1351. doi:10.3762/bjoc.9.152
Ohura, K., Nozawa, T., Murakami, K., and Imai, T. (2011). Evaluation of Transport Mechanism of Prodrugs and Parent Drugs Formed by Intracellular Metabolism in Caco-2 Cells with Modified Carboxylesterase Activity: Temocapril as a Model Case. J. Pharm. Sci. 100 (9), 3985–3994. doi:10.1002/jps.22628
Potter, P. M., Wolverton, J. S., Morton, C. L., Wierdl, M., and Danks, M. K. (1998). Cellular Localization Domains of a Rabbit and a Human Carboxylesterase: Influence on Irinotecan (CPT-11) Metabolism by the Rabbit Enzyme. Cancer Res. 58 (16), 3627–3632.
Pratt, S. E., Durland-Busbice, S., Shepard, R. L., Heinz-Taheny, K., Iversen, P. W., and Dantzig, A. H. (2013). Human Carboxylesterase-2 Hydrolyzes the Prodrug of Gemcitabine (LY2334737) and Confers Prodrug Sensitivity to Cancer Cells. Clin. Cancer Res. 19 (5), 1159–1168. doi:10.1158/1078-0432.CCR-12-1184
Qian, Y. L., and Markowitz, J. S. (2020). Natural Products as Modulators of CES1 Activity. Drug Metab. Dispos 48 (10), 993–1007. doi:10.1124/dmd.120.000065
Quinney, S., Sanghani, S., Davis, W., Hurley, T., Sun, Z., Murry, D., et al. (2005). Hydrolysis of Capecitabine to U2-Deoxy-5-Fluorocytidine by Human Carboxylesterases and Inhibition by Loperamide. J. Pharmacol. Exp. Ther. 313, 1011–1016. doi:10.1124/JPET.104.081265
Shao, T. F., Zheng, Y. T., Xu, J. L., and Cai, W. M. (2012). An Analytical Method Built for Irinotecan and its Active Metabolite SN-38 in Human Plasma Using HPLC-FLD. Chin. J. Hosp. Pharm. 32, 17–19.
Shi, D., Yang, J., Yang, D., LeCluyse, E. L., Black, C., You, L., et al. (2006). Anti-influenza Prodrug Oseltamivir Is Activated by Carboxylesterase Human Carboxylesterase 1, and the Activation Is Inhibited by Antiplatelet Agent Clopidogrel. J. Pharmacol. Exp. Ther. 319 (3), 1477–1484. doi:10.1124/jpet.106.111807
Shi, J., Wang, X., Nguyen, J., Wu, A., Bleske, B., and Zhu, H. (2016). Sacubitril Is Selectively Activated by Carboxylesterase 1 (CES1) in the Liver and the Activation Is Affected by CES1 Genetic Variation. Drug Metab. Dispos. 44, 554–559. doi:10.1124/dmd.115.068536
Slatter, J. G., Su, P., Sams, J. P., Schaaf, L. J., and Wienkers, L. C. (1997). Bioactivation of the Anticancer Agent CPT-11 to SN-38 by Human Hepatic Microsomal Carboxylesterases and the In Vitro Assessment of Potential Drug Interactions. Drug Metab. Dispos. 25 (10), 1157–1164.
Song, Y. Q., Guan, X. Q., Weng, Z. M., Wang, Y. Q., Chen, J., Jin, Q., et al. (2019a). Discovery of a Highly Specific and Efficacious Inhibitor of Human Carboxylesterase 2 by Large-Scale Screening. Int. J. Biol. Macromol. 137, 261–269. doi:10.1016/j.ijbiomac.2019.06.235
Song, Y. Q., Weng, Z. M., Dou, T. Y., Finel, M., Wang, Y. Q., Ding, L. L., et al. (2019b). Inhibition of Human Carboxylesterases by Magnolol: Kinetic Analyses and Mechanism. Chem. Biol. Interact. 308, 339–349. doi:10.1016/j.cbi.2019.06.003
Souza, G. A. d., Marqui, S. V. d., Matias, J. N., Guiguer, É. L., and Barbalho, S. M. (2020). Effects of Ginkgo Biloba on Diseases Related to Oxidative Stress. Planta Med. 86, 376–386. doi:10.1055/a-1109-3405
Stoddard, S. V., Yu, X., Potter, P. M., and Wadkins, R. M. (2010). In Silico Design and Evaluation of Carboxylesterase Inhibitors. J. Pest Sci. 35 (3), 240–249. doi:10.1584/jpestics.R10-06
Tao, Z., Jin, W., Ao, M., Zhai, S., Xu, H., and Yu, L. (2019). Evaluation of the Anti-inflammatory Properties of the Active Constituents in Ginkgo Biloba for the Treatment of Pulmonary Diseases. Food Funct. 10 (4), 2209–2220. doi:10.1039/c8fo02506a
Tobin, P. J., Hong, Y., Seale, J. P., Rivory, L. P., and McLachlan, A. J. (2005). Loperamide Inhibits the Biliary Excretion of Irinotecan (CPT-11) in the Rat Isolated Perfused Liver. J. Pharm. Pharmacol. 57 (1), 39–45. doi:10.1211/0022357055100
Trott, O., and Olson, A. J. (2010). AutoDock Vina: Improving the Speed and Accuracy of Docking with a New Scoring Function, Efficient Optimization, and Multithreading. J. Comput. Chem. 31 (2), 455–461. doi:10.1002/jcc.21334
van Beek, T. V., and Montoro, P. (2009). Chemical Analysis and Quality Control of Ginkgo Biloba Leaves, Extracts, and Phytopharmaceuticals. J. Chromatogr. A 1216 (11), 2002–2032. doi:10.1016/j.chroma.2009.01.013
Wang, D., Song, Y., Wang, Y., Li, H., Ge, G., and Yang, L. (2019). Carboxylesterases Mediated Herb-Drug Interactions: A Systematic Review. TMR Modern Herbal Med. 2 (1). 25–35. doi:10.12032/TMRmhm2017B40
Wang, D., Zou, L., Jin, Q., Hou, J., Ge, G., and Yang, L. (2018). Human Carboxylesterases: a Comprehensive Review. Acta Pharm. Sin B 8 (5), 699–712. doi:10.1016/j.apsb.2018.05.005
Wang, J., Williams, E. T., Bourgea, J., Wong, Y. N., and Patten, C. J. (2011). Characterization of Recombinant Human Carboxylesterases: Fluorescein Diacetate as a Probe Substrate for Human Carboxylesterase 2. Drug Metab. Dispos. 39 (8), 1329–1333. doi:10.1124/dmd.111.039628
Wang, Y. Q., Weng, Z. M., Dou, T. Y., Hou, J., Wang, D. D., Ding, L. L., et al. (2018). Nevadensin Is a Naturally Occurring Selective Inhibitor of Human Carboxylesterase 1. Int. J. Biol. Macromol. 120 (Pt B), 1944–1954. doi:10.1016/j.ijbiomac.2018.09.178
Weng, Z. M., Ge, G. B., Dou, T. Y., Wang, P., Liu, P. K., Tian, X. H., et al. (2018). Characterization and Structure-Activity Relationship Studies of Flavonoids as Inhibitors against Human Carboxylesterase 2. Bioorg. Chem. 77, 320–329. doi:10.1016/j.bioorg.2018.01.011
White, C. M. (2019). Loperamide: A Readily Available but Dangerous Opioid Substitute. J. Clin. Pharmacol. 59 (9), 1165–1169. doi:10.1002/jcph.1449
Wu, M. H., Chen, P., Remo, B. F., Cook, E. H., Das, S., and Dolan, M. E. (2003). Characterization of Multiple Promoters in the Human Carboxylesterase 2 Gene. Pharmacogenetics 13 (7), 425–435. doi:10.1097/00008571-200307000-00008
Yang, X. X., Hu, Z. P., Duan, W., Zhu, Y. Z., and Zhou, S. F. (2006). Drug-herb Interactions: Eliminating Toxicity with Hard Drug Design. Curr. Pharm. Des. 12 (35), 4649–4664. doi:10.2174/138161206779010440
Zhao, Y.-S., Qian, X., Guan, X., Song, P.-F., Song, Y., He, R., et al. (2020). Discovery of Natural Alkaloids as Potent and Selective Inhibitors against Human Carboxylesterase 2. Bioorg. Chem. 105, 104367.
Zhu, Q., Bang, T. H., Ohnuki, K., Sawai, T., Sawai, K., and Shimizu, K. (2015). Inhibition of Neuraminidase by Ganoderma Triterpenoids and Implications for Neuraminidase Inhibitor Design. Sci. Rep. 5, 13194. doi:10.1038/srep13194
Zou, L. W., Jin, Q., Wang, D. D., Qian, Q. K., Hao, D. C., Ge, G. B., et al. (2018). Carboxylesterase Inhibitors: An Update. Curr. Med. Chem. 25 (14), 1627–1649. doi:10.2174/0929867325666171204155558
Zou, L. W., Li, Y. G., Wang, P., Zhou, K., Hou, J., Jin, Q., et al. (2016). Design, Synthesis, and Structure-Activity Relationship Study of Glycyrrhetinic Acid Derivatives as Potent and Selective Inhibitors against Human Carboxylesterase 2. Eur. J. Med. Chem. 112, 280–288. doi:10.1016/j.ejmech.2016.02.020
Keywords: biflavones, inhibition, specificity, irinotecan-induced diarrhea, human carboxylesterase 2 (CES2)
Citation: Song Y-Q, He R-J, Pu D, Guan X-Q, Shi J-H, Li Y-G, Hou J, Jia S-N, Qin W-W, Fang S-Q and Ge G-B (2021) Discovery and Characterization of the Biflavones From Ginkgo biloba as Highly Specific and Potent Inhibitors Against Human Carboxylesterase 2. Front. Pharmacol. 12:655659. doi: 10.3389/fphar.2021.655659
Received: 19 January 2021; Accepted: 08 April 2021;
Published: 18 May 2021.
Edited by:
Tingjun Hou, Zhejiang University, ChinaReviewed by:
Robert Parker, University of Tennessee Health Science Center (UTHSC), United StatesCopyright © 2021 Song, He, Pu, Guan, Shi, Li, Hou, Jia, Qin, Fang and Ge. This is an open-access article distributed under the terms of the Creative Commons Attribution License (CC BY). The use, distribution or reproduction in other forums is permitted, provided the original author(s) and the copyright owner(s) are credited and that the original publication in this journal is cited, in accordance with accepted academic practice. No use, distribution or reproduction is permitted which does not comply with these terms.
*Correspondence: Wei-Wei Qin, d3dxaW5AZnVkYW4uZWR1LmNu; Sheng-Quan Fang, ZnNxMjAwMzIwMDNAMTYzLmNvbQ==; Guang-Bo Ge, Z2VndWFuZ2JvQGRpY3AuYWMuY24=
†These authors have contributed equally to this work.
Disclaimer: All claims expressed in this article are solely those of the authors and do not necessarily represent those of their affiliated organizations, or those of the publisher, the editors and the reviewers. Any product that may be evaluated in this article or claim that may be made by its manufacturer is not guaranteed or endorsed by the publisher.
Research integrity at Frontiers
Learn more about the work of our research integrity team to safeguard the quality of each article we publish.