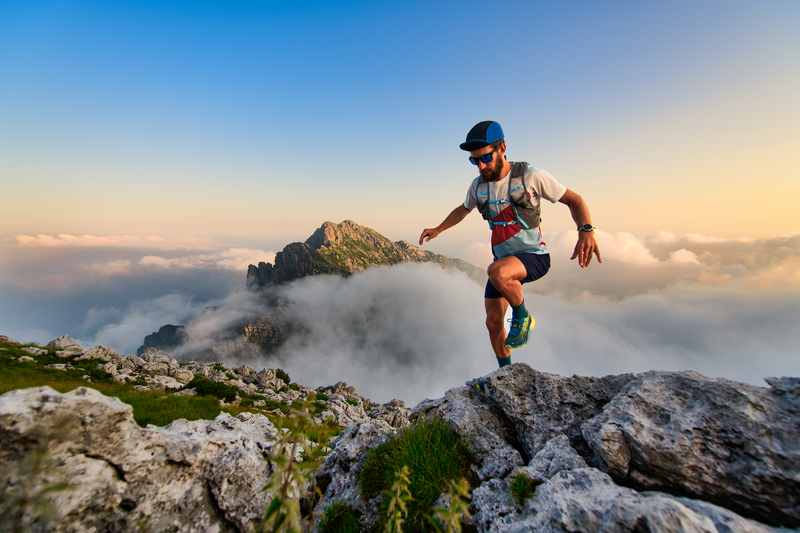
95% of researchers rate our articles as excellent or good
Learn more about the work of our research integrity team to safeguard the quality of each article we publish.
Find out more
REVIEW article
Front. Pharmacol. , 08 March 2021
Sec. Experimental Pharmacology and Drug Discovery
Volume 12 - 2021 | https://doi.org/10.3389/fphar.2021.632677
This article is part of the Research Topic Novel Therapeutic Interventions Against Infectious Diseases: COVID-19 View all 16 articles
COVID-19 pandemic has spread worldwide at an exponential rate affecting millions of people instantaneously. Currently, various drugs are under investigation to treat an enormously increasing number of COVID-19 patients. This dreadful situation clearly demands an efficient strategy to quickly identify drugs for the successful treatment of COVID-19. Hence, drug repurposing is an effective approach for the rapid discovery of frontline arsenals to fight against COVID-19. Successful application of this approach has resulted in the repurposing of some clinically approved drugs as potential anti-SARS-CoV-2 candidates. Several of these drugs are either antimalarials, antivirals, antibiotics or corticosteroids and they have been repurposed based on their potential to negate virus or reduce lung inflammation. Large numbers of clinical trials have been registered to evaluate the effectiveness and clinical safety of these drugs. Till date, a few clinical studies are complete and the results are primary. WHO also conducted an international, multi-country, open-label, randomized trials-a solidarity trial for four antiviral drugs. However, solidarity trials have few limitations like no placebos were used, additionally any drug may show effectiveness for a particular population in a region which may get neglected in solidarity trial analysis. The ongoing randomized clinical trials can provide reliable long-term follow-up results that will establish both clinical safety and clinical efficacy of these drugs with respect to different regions, populations and may aid up to worldwide COVID-19 treatment research. This review presents a comprehensive update on majorly repurposed drugs namely chloroquine, hydroxychloroquine, remdesivir, lopinavir-ritonavir, favipiravir, ribavirin, azithromycin, umifenovir, oseltamivir as well as convalescent plasma therapy used against SARS-CoV-2. The review also summarizes the data recorded on the mechanism of anti-SARS-CoV-2 activity of these repurposed drugs along with the preclinical and clinical findings, therapeutic regimens, pharmacokinetics, and drug-drug interactions.
The coronavirus disease of 2019 (COVID-19) pandemic has changed the scenario of the entire world, which has not been seen for a century. Influenza (H1N1 virus) outbreak of Spain that occurred in 1918 was the worst ever hit pandemic in recent history. Now, the current outbreak started in Wuhan, China, has globally spread to 219 countries and territories (WHO, 2020a). Severe acute respiratory syndrome coronavirus (CoV) 2 (SARS-CoV-2), a large ssRNA virus, is the causative agent of COVID-19, which primarily attacks the respiratory tract including associated organs. Additionally, the virus has shown to impact various other organs or body systems like the gastrointestinal system, nervous system etc (Jakhmola et al., 2020a; Jakhmola et al., 2020b; Sonkar et al., 2020). Currently new variants of SARS-CoV-2 are reported from different regions of the world. In December 2020, the United Kingdom variant of SARS-CoV-2 lineage B.1.1.7, now designated as Variant of Concern 202012/01 (VOC) and the South Africa variant named 501Y.V2 have been reported to spread widely within the country and displaced the other lineages of viruses (WHO, 2020c). By the end of first COVID-19 pandemic year the VOC-202012/01 variant was reported in 31 other countries/territories (WHO, 2020c). The receptor-binding domain of viral spike protein is essential in SARS-CoV-2 entry into the host cell via surface angiotensin-converting enzyme-2 (ACE-2) (Zhou et al., 2020) (Figure 1). Recently, another cell receptor Neuropilin-1 was found to be involved in SARS-CoV-2 entry (Cantuti-Castelvetri et al., 2020). The further life cycle of the virus inside the cell is similar to that of other coronaviruses. After binding to the receptor, the conformational change in the spike protein leads to virus fusion with the host cell membrane. The virus may transfer the RNA directly inside the cells or may proceed through the endosomal pathway (Simmons et al., 2005; Li, 2016; Hasan et al., 2020; Hoffmann et al., 2020). Upon translation of viral RNA, the viral replicase polyprotein PP1a and PP1ab are synthesized and cleaved into small products by viral endopeptidase (Van-Boheemen et al., 2012; Shereen et al., 2020). RNA dependent RNA polymerase (RdRp) produces subgenomic RNAs by discontinuous transcription (Hussain et al., 2005; Chen et al., 2020; Shereen et al., 2020). This further gets translated into respective viral proteins. After processing through the endoplasmic reticulum (ER), ER-Golgi intermediate compartment (ERGIC), and Golgi complex the viral RNA and proteins are assembled into virions (Lai and Cavanagh, 1997; Song et al., 2004). These virions are transported through vesicles and exocytosed for transmission. These steps of the viral life cycle are lucrative virus inhibition targets for different drugs (Figure 1).
FIGURE 1. Proposed mechanisms of repurposed drugs and therapies used against SARS-CoV-2 infection. SARS-CoV-2 interacts with cell surface receptors like ACE-2 and neuropilin to gain entry inside the cell. Umifenovir may interact with SARS-CoV-2 surface glycoproteins and lipids and obstruct the interaction with the entry receptor ACE-2. Anti-SARS-CoV-2 antibodies present in convalescent plasma may inhibit SARS-CoV-2 entry and subsequent infection transmission. Chloroquine, hydroxychloroquine and azithromycin may elevate endosomal pH and hinder viral entry and RNA release process. Chloroquine, hydroxychloroquine and azithromycin also shows immunomodulatory effects. Nucleoside inhibitors like remdesivir, favipiravir and ribavirin may inhibit RNA replication and suppress RNA-dependent RNA polymerase activity. Lopinavir may fraternize with viral protease altering the proteolysis. Oseltamivir may interplay with components involved in the exocytosis process, blocking the viral exit from the cell. Monoclonal antibodies against cytokine receptors and Corticosteroid shows anti-inflammatory actions against exaggerated immune response. (ACE-2-Angiotensin-converting enzyme 2, TMPRSS2 Transmembrane Serine protease 2, RdRp- RNA dependent RNA polymerase, ER- Endoplasmic reticulum, ERGIC- Endoplasmic reticulum-golgi intermediate complex. The displayed ACE-2-Spike interaction residues and RdRp structures are based on Protein databank structure ID: 6M0J and 6M71 respectively).
Currently, no specific drug or vaccine is available for the treatment of SARS-CoV-2 infected patients. Nonetheless, drug repurposing could prove to be advantageous tactics for finding COVID-19 treatment. Benefits of drug repurposing include cost-effectiveness, elimination of some clinical trial steps, sooner on-field availability, combining the candidate drugs with other possible drugs based on prior data, and generation of new information about the existing drugs mechanisms (Agrawal, 2015). The available knowledge of previous CoVs treatments, genomic sequences, and protein modeling studies has helped researchers put forward the potential COVID-19 drug candidates. The primarily investigated drugs are either antimalarials, antivirals, antibiotics, corticosteroids, and they have been repurposed based on their potential either to negate virus, reduce lung inflammation or other disease symptoms. In particular, chloroquine (CQ) hydroxychloroquine (HCQ) and azithromycin (AZM) are majorly used against COVID-19 as they initially showed reasonably good in vitro and in vivo antiviral activity against SARS-CoV, MERS-CoV and SARS-CoV-2. Lopinavir/ritonavir (LPV/RTV), which are anti-HIV drugs, were examined for COVID-19 as they were found to be effective in earlier CoV outbreaks. Moreover, remdesivir (RDV), an experimental anti-Ebola drug, was investigated for COVID-19 and received greater attention. Based on the appreciable preliminary data, FDA has issued EUA for CQ, HCQ, and RDV (FDA, 2020b; FDA, 2020c; FDA, 2020d). However, later the EUA for CQ and HCQ was revoked while EUA for RDV was re-issued by some amendments. Similarly, favipiravir (FPV), ribavirin (RBV), umifenovir (UFV), and oseltamivir (OTV) having a broad-spectrum antiviral activity were also clinically investigated against SARS-CoV-2. WHO put forward a solidarity clinical trial, a multi-country, open-label randomized trial, for the use of HCQ, RDV, LPV/RTV, or LPV/RTV in combination with Interferon (IFN) β-1a against COVID-19 (WHO, 2020b). The recent interim results of the solidarity trial declare that all these drugs had little or no effect on overall mortality, initiation of ventilation and duration of hospital stay in hospitalized patients (Pan et al., 2020). So far, to treat severe and critical COVID-19, only corticosteroids have proven effective (WHO, 2020b). New treatment options need to be added to the solidarity trial in the future. However, solidarity trials may have few limitations like it was focused on worldwide outcomes of considered treatments. Hence, if a particular drug shows comparatively better outcome in some regions or populations that could get neglected. Therefore, there is scope to conduct the clinical studies involving various suitable drugs with different treatment regimens and combinations.
In this review, we have provided updated comprehensive information about majorly repurposed drugs which are used as antivirals to combat SARS-CoV-2 infection. We attempted to collate and review the studies regarding all these drugs, which are dispersed in various distinct publications. Furthermore, we have also summarized the data recorded on the mechanism of anti-SARS-CoV-2 activity of these repurposed drugs along with the preclinical and clinical findings, therapeutic regimens, pharmacokinetics, and drug-drug interactions.
CQ and HCQ both belong to the 4-aminoquinoline chemical class (Devaux et al., 2020) with potential antimalarial and anti-inflammatory activities. These drugs are weak diprotic bases that increase the endosomal pH to hinder the host-virus fusion process (Devaux et al., 2020) (Figure 1; Table 1). In vitro studies have shown antiviral activity of CQ on MERS and SARS-CoV (Cong et al., 2018; Keyaerts et al., 2004). In addition, in vivo studies suggest potent activity of these drugs against human CoV-OC43, EV-A71, zika virus, and in vitro activity against influenza-A (Keyaerts et al., 2009; Tan et al., 2018; Li et al., 2017; Ooi et al., 2006). Recent in vitro studies report CQ and HCQ effectiveness against SARS-CoV-2 (Half maximal effective concentration (EC50) 2.71mM and 4.51mM, respectively) in Vero E6 cells (Liu J. et al., 2020). However, HCQ has in vitro activity with a lower EC50 for SARS-CoV-2 compared to CQ after 24h of growth (HCQ: 6.14μM and CQ: 23.90μM) (Yao X. et al., 2020). CQ treatment has demonstrated to reduce the recovery time and improved physiological conditions in COVID-19 patients. According to a randomized Chinese COVID-19 controlled trial, CQ (Dose 500mg bid, 15days) may work more efficiently than LPV/RTV (Huang M. et al., 2020). Another study compared the low dose (450mg bid for 1day followed by 450mg, 4days) and high dose (600mg bid, 10days) in combination with azithromycin (AZM) and OTV which determined that high dose CQ was associated with high mortality (Borba et al., 2020). A multicentre, randomized, open-label trial from China investigated the use of HCQ (1200mg daily for 3days, followed by a maintenance dose of 800mg daily) to standard care. The interpretation included that the HCQ treated group showed inadequate response compared to control (Tang et al., 2020). The combination of HCQ and AZM resulted in early viral clearance, as demonstrated by an open-label non-randomized clinical trial (Gautret et al., 2020). A meta-analysis report stated that compared to alone HCQ, the combination of HCQ and AZM significantly increased mortality in COVID patients (Fiolet et al., 2020). A United States based observational study interpreted that HCQ treated patients did not either benefit or suffer in terms of intubation or mortality (Geleris et al., 2020). A large-scale clinical trial was conducted in United Kingdom, a Randomized Evaluation of COVID-19 Therapy (RECOVERY Trial), to investigate various drug candidates or therapies including HCQ against severe COVID-19. The result demonstrated no efficacy of HCQ against COVID-19 (Horby et al., 2020b). Surprisingly FDA issued EUA for CQ and HCQ against COVID-19 on March 28, 2020 and was revoked on June 15, 2020 (FDA, 2020b; FDA, 2020c). Major side effects of these drugs include QT prolongations, and decreased insulin clearance and resistance (FDA, 2020b; FDA, 2020c). The overuse of CQ and HCQ could possibly lead to tissue injury in the liver, retina, skeletal, and cardiac muscle cells due to their lysosomal affinity (Satarker et al., 2020; Cohen, 2020). Therefore, studies recommend that physicians avoid high doses and exercise extreme caution in the compassionate use of CQ/HCQ, either alone or in combination with other antivirals (Acharya and Sayed, 2020). Currently 88 and 267 COVID-19 associated clinical trials have been registered for CQ and HCQ respectively (ClinicalTrials.gov, 2020b; ClinicalTrials.gov, 2020d).
LPV/RTV are approved anti-HIV drugs that specifically target HIV protease (Chandwani and Shuter, 2008). LPV is used in combination with RTV to elevate its half-life via cytochrome P450 suppression (Chandwani and Shuter, 2008). LPV is predicted to act on the viral 3-chymotrypsin-like protease (3CLpro) (Sisay, 2020) (Figure 1; Table 1). Previous studies have established in vitro LPV effectiveness against SARS-CoV at 4μg/ml (Chu et al., 2004). A cumulative in vivo study involving LPV and RTV against MERS revealed the EC50 of the two drugs as 11.6 and 24.9µM respectively with 50% cytotoxic concentration (CC50) values >50µM (Sheahan et al., 2020). However, no in vitro study is available of LPV/RTV against SARS-CoV-2. In China, a clinical trial for LPV/RTV against adult hospitalized COVID-19 patients was conducted (Cao et al., 2020). The study showed no benefit of LPV/RTV (Dose: 400mg/100mg bid, 14days) treatment compared to standard care control groups (Cao et al., 2020). Although LPV/RTV treated groups exhibited less serious complications than the controls. A Japanese case-study reports successful treatment of non-severe COVID-19 pneumonia patients with LPV/RTV (Wada et al., 2020). Another study of 47 patients reported that LPV/RTV treatment improved physiological condition without adverse events (Ye et al., 2020). In the study of 120 patients, if the LPV/RTV treatment is initiated within 10days of symptom onset, it significantly reduces viral shedding (Yan et al., 2020). LPV/RTV along with IFN-⍺ or RBV may improve the health of COVID-19 patients (Yuan et al., 2020). On the contrary, another report from Taiwan suggested that LPV/RTV treatment did not reduce the duration of viral shedding in infected patients (Cheng et al., 2020). A single-center, randomized, open-labeled, prospective clinical trial conducted by Huang et al. studied the effect of LPV/RTV plus IFN-α, LPV/RTV plus RBV plus IFN-α, and RBV plus IFN-α treatment on COVID-19 patients. All the three regimens showed no significant difference regarding their effectiveness against COVID-19. LPV/RTV when given in combination with RBV lead to more adverse events suggesting that these two drugs should not be administered together (Huang Y.-Q. et al., 2020). A meta-analysis study investigating randomized trials showed LPV/RTV may reduce mortality (Huang Y.-Q. et al., 2020; Verdugo-Paiva et al., 2020). Albeit some contradictory studies showed no statistically significant effect on reducing the death rate (Karolyi et al., 2020; Horby et al., 2020). A report stated the risk of bradycardia in elderly critically ill COVID-19 patients with RTV plasma overdose (Beyls et al., 2020). Adverse gastrointestinal effects such as diarrhea, nausea and vomiting have been observed in patients treated with LPV/RTV (Huang Y.-Q. et al., 2020; Liu W. et al., 2020; Vecchio et al., 2020). Therefore, it remains difficult to safely recommend LPV/RTV dose without compromising the benefit of the antiviral strategy. There is an urgency of a comprehensive pharmacokinetic/pharmacodynamic analysis for the upcoming clinical trials in similar critically ill COVID-19 patients (Lê et al., 2020). Currently, 90 clinical trials have been registered for LPV/RTV for COVID-19 (ClinicalTrials.gov, 2020e).
RDV is a prodrug of an adenosine triphosphate (ATP) analog and is converted into its active form GS-441524 on administration (Al-Tawfiq et al., 2020). It was initially proposed for the Ebola virus that acted by viral replication inhibition through premature termination of RNA transcription (Al-Tawfiq et al., 2020) and hence targeting RdRp. RDV metabolites have proved useful against yellow fever virus, Dengue virus type 2, influenza A, parainfluenza 3, and various delta CoVs (Cho et al., 2012). The parent nuclei of RDV is evaluated against alpha CoV (EC50 = 0.78μM), porcine delta CoV, SARS-like bat CoVs and MERS-like bat CoVs (Murphy et al., 2018; Brown et al., 2019; Amirian and Levy, 2020). Studies on SARS and MERS infected human airway epithelial cells (EC50 ≈ 0.07μM) and animal models demonstrated the viral polymerase inhibition ability of the drug (Agostini et al., 2018). The drug is efficient at EC50, 0.77μM, and CC50 > 100μM in Vero E6 cells against SARS-CoV-2 (Wang M. et al., 2020). Studies also revealed that RDV targeted structurally analogous regions of SARS-CoV-2 polymerase (Lo et al., 2020). Several in-vivo reports suggested that RDV decreased viral load, reduced pathological processes, alleviated mild symptoms, and improved pulmonary lesions in SARS-CoV-2 infected animals with adverse effect (Frediansyah et al., 2020; Badgujar et al., 2020; Pruijssers et al., 2020). The recommended dose of RDV is 200mg on Day 1 and 100mg daily for 5days (for non-severe cases) to 10days (severe cases). A similar dose was considered in numerous clinical trials. A randomized, open-label, phase 3 trial investigating RDV dose for 5days vs. 10days revealed that the treatment for 5days was comparatively beneficial (Spinner et al., 2020). A double-blinded, randomized, placebo-controlled trial, determined that severe COVID-19 patients treated with RDV showed fast recovery compared to control, though statistically insignificant (Wang Y. et al., 2020). Moreover, the RDV administration is not approved globally due to questionable safety. Although SOLIDARITY trial results denote that RDV is not beneficial against COVID-19, result of some recently completed clinical trials are contrary. A double-blinded, randomized, placebo-controlled trial from the United States showed that RDV treated hospitalized patients may recover faster with comparatively less adverse events and mortality than the placebo group (Beigel et al., 2020). Prominent adverse reactions were acute respiratory failure, decreased glomerular filtration rate, lymphocytopenia, pyrexia, hyperglycemia, increased anemia, increased creatine, and liver transaminases (Beigel et al., 2020; FDA, 2020d). Result of multicentre clinical trial published at the end of first year of the pandemic, showed that RDV given in combination with baricitinib (a Janus kinase inhibitor used to hinder intracellular signaling of cytokines) was effective compared to RDV alone in terms of reducing recovery time additionally speeding improvement (Kalil et al., 2020). Based on such positive results of RDV, it has been approved to use by various authorized platforms like FDA (Mahase and McCullough, 2020). An interesting investigation showed that RDV’s parent nucleotide GS-441524 is superior and less toxic than its pro-drug form and has shown efficacy in in vivo veterinary settings (Yan and Muller, 2020). Therefore, further investigation regarding the use of the parent nucleotide itself against COVID-19 should be driven with a faster pace. Currently, 78 COVID-19 associated clinical trials are registered with RDV (ClinicalTrials.gov, 2020g).
Favipiravir (FPV), an approved influenza treatment, is a pyrazinecarboxamide derivative (Furuta et al., 2013). It also showed efficacy against arenavirus, bunyavirus, flavivirus, filoviruses, and Ebola virus (Furuta et al., 2017). The prodrug after administration is transformed by host enzymes into the ribofuranosyl triphosphate derivative (T-705-RTP), a guanine analogue and suppresses the RdRp (Figure 1; Table 1). In vitro effectivity of FPV against SARS or MERS viruses have not been addressed. An in vitro study has shown inhibition of SARS-CoV-2 by FPV (EC50 = 61.88μM; CC50 = over 400μM) (Wang X. et al., 2020). In Japan, the approved dose of FPV against influenza is 1,600mg bid on day 1, followed by 600mg bid on days 2–5 with associated side effects (PMDA, 2020). A Chinese open-label, controlled study investigated the effects of FPV (Day 1; 1600mg twice and Day 2–14; 600mg bid) vs. LPV/RTV (Day 1–14; 400mg/100mg bid). The preliminary results indicated potent FPV action and fewer adverse effects than LPV/RTV (p < 0.001) (Cai et al., 2020). A report suggested treatment of COVID-19 patients with FPV during times of early symptoms, helped in reducing the SARS-CoV-2 presence in nasal secretions (McCullough, 2020). However, previous clinical trials have reported the variation in FPV plasma concentration between the United States and the Japanese population (Madelain et al., 2016). Therefore, more trials regarding global use of FPV should be considered. In a Japanese study FPV also showed to control inflammatory mediators and pneumonia progression in COVID-19 patients (Yamamura et al., 2020). Severe or critical COVID-19 patients showed improvements after treating with FPV (Takahashi et al., 2020) and FPV also led to improved lung histology (Kaptein et al., 2020). However, in a meta-analysis study, FVP proved to have significant clinical and radiological improvement without significant differences on viral clearance (Shrestha et al., 2020). For the use of FPV with respect to COVID-19, 45 clinical trials have been registered (ClinicalTrials.gov, 2020c).
Ribavirin (RBV), a broad-spectrum antiviral prodrug is metabolized in host into a guanosine analog (Gish, 2006). The drug showed antiviral efficacy against canine distemper virus, hepatitis C virus, Enterovirus 71, Chikungunya virus, and Semliki Forest virus, orthopoxvirus, influenza virus, flavi- and paramyxoviruses (Elia et al., 2008; Galli et al., 2018; Li et al., 2008; Briolant et al., 2004; Smee et al., 2001; Leyssen et al., 2005). A study observed reduced replication of the MERS-CoV in rhesus macaques upon treatment with IFN-α2b and RBV (Falzarano et al., 2013). RBV in combination with LPV/RTV was used in SARS-CoV and MERS-CoV trials (Yao T. et al., 2020). In the case of SARS-CoV-2 infection, an in vitro study determined the EC50 of RBV as 109.50uM (Wang X. et al., 2020). A study included RBV along with LPV/RTV and IFN-α in the treatment of hospitalized COVID-19 patients (Hung et al., 2020). The triple therapy was found to be beneficial to reduce disease symptoms and virus shedding compared to groups provided LPV-RTV alone. The dose of RBV considered was 400mg bid along with 400mg/100mg of LPV/RTV + IFN-α for 14days. A study assessed the impact of sofosbuvir/daclatasvir (antivirals) compared to RBV in treatment of COVID-19 patients. The mortality was higher (33%) in COVID-19 patients treated with RBV than that of sofosbuvir/daclatasvir (Eslami et al., 2020). A retrospective cohort study comparing RBV vs. supportive therapy stated that RBV did not help in reducing the mortality rate in COVID-19 patients (Tong et al., 2020). 15 clinical trials have been registered for the use of RBV alone or in combination with other COVID-19 drugs (ClinicalTrials.gov, 2020h).
Azithromycin (AZM) is a semisynthetic macrolide antibiotic belonging to the azalide class (Ballow and Amsden, 1992). It has bactericidal effects and targets the protein synthesis process of bacteria. AZM has also been shown to inhibit influenza, zika, dengue, and Ebola viruses (Damle et al., 2020; Wang M. et al., 2020). Specifically, a study showed AZM induced reduction in rhinovirus replication 7-fold in primary bronchial epithelial cells without inducing cell death (Schögler et al., 2015). The in vitro EC50 for AZM against SARS‐CoV‐2 was 2.12µM (EC90: 8.65µM) following a 72‐hour incubation post-infection (MOI of 0.002) (Hughes et al., 2020). The addition of AZM with HCQ was efficient in virus elimination in COVID-19 patients (Gautret et al., 2020). The dose of 500mg on day 1 followed by 250mg/day, the next 4days was used in compliment to HCQ dose of 200mg, three times/day, for 10days. Some investigations suggested HCQ and AZM combination to be beneficial in reducing mortality in COVID-19 patients (Bonny et al., 2020; Arshad et al., 2020). A case report showed AZM provided with HCQ proved to be an effective treatment approach in pregnant women against the SARS-CoV-2 infection and associated with reduced mortality (Sisti et al., 2020). In contrast, a report from the United States stated that neither HCQ nor AZM separately or together could reduce the mortality of COVID-19 patients compared to the control group (Rosenberg et al., 2020). Moreover, treatment of AZM and HCQ was associated with greater changes in QTc in COVID-19 patients (Mercuro et al., 2020). Few other studies also reported that AZM included in treating COVID-19 patients did not provide any beneficial effect (Rodríguez-Molinero et al., 2020; Furtado et al., 2020; Cavalcanti et al., 2020). 122 clinical trials have been registered for the use of AZM alone or in combination with other drugs against COVID-19 (ClinicalTrials.gov, 2020a).
Umifenovir (UFV) is an indolyl carboxylic acid widely recognized as Arbidol (Blaising et al., 2014). It is used as a treatment and prevention measure against influenza virus (Blaising et al., 2014). It has direct antiviral and host-targeting action. UFV can interact with virus protein or lipid components and may hinder different stages of the viral life cycle (Blaising et al., 2014). In vitro analysis of the antiviral activity of arbidol against several human respiratory viruses, namely influenza-A virus, respiratory syncytial virus, rhinovirus type-14, coxsackievirus-B3 and adenovirus type-7 is demonstrated (Shi et al., 2007). Inhibition of SARS-CoV replication on UFV treatment was demonstrated in vitro. UFV is also known to inhibit various isolates of zika virus in multiple cell lines (Fink et al., 2018). The inhibitory action of the drug against SARS-CoV-2 in Vero E6 cells (MOI of 0.05) has been demonstrated. The EC50 and CC50 were 4.11 and 31.79μM, respectively (Wang X. et al., 2020). Briefly, the study showed enhanced inhibitory activity at early stages compared to the post-entry stage (Figure 1). A small-scale study suggested post-exposure prophylaxis (PEP) use of UFV in people exposed to COVID-19 patients (Zhang et al., 2020). Another study determined that arbidol monotherapy was superior to LPV/RTV against COVID-19 (Zhu et al., 2020). COVID-19 patients provided with UFV along with LPV/RTV showed better outcomes compared to patients who received LPV/RTV only (Deng et al., 2020). A contrary study reported that UFV was not beneficial to improve the condition of the patient or viral clearance (Lian et al., 2020). Moreover, another study suggested arbidol + LPV/RTV were associated with many adverse events (Wen et al., 2020). In most of the studies, a dose of 200mg thrice a day was considered. According to a meta-analysis, UFV was not effective in terms of reducing the SARS-CoV-2 elimination from the infected patient in terms of detection in diagnostic tests and even hospital length of stay of hospitalized patients (Huang D. et al., 2020). There is no evidence to support the use of UFV for improving patient‐important outcomes in patients with COVID‐19. 11 registered clinical trials include UFV use in COVID-19 treatment (ClinicalTrials.gov, 2020i).
Oseltamivir (OTV) is a synthetic derivative prodrug of ethyl ester with antiviral activity (Schade et al., 2014). It acts as a neuraminidase inhibitor against the influenza virus and is also effective for various avian influenza virus strains (Ward et al., 2005). An in vitro OTV study on H5N1 influenza showed that the IC50 was 0.1–4.9nM (Govorkova et al., 2009). However, an in vivo study involving H5N1 infection required a longer course and higher dosage of OTV (Borio et al., 2018). No in vitro study against SARS-CoV-2 is conducted for OTV. The COVID-19 originated in China during flu season, and hence earlier, many patients received OTV treatment until the causative agent SARS-CoV-2 was discovered. Some current clinical trials have used OTV in combination with other major therapeutic candidates. A study showed that the drug exhibited no positive result on COVID-19 (Wang D. et al., 2020). 20 clinical trials have been registered which include OTV in the treatment panel of COVID-19 (ClinicalTrials.gov, 2020f).
Apart from antiviral drugs another probable efficient antiviral strategy includes the use of convalescent plasma collected from the recovered patients containing the anti-SARS-CoV-2 antibodies. Convalescent plasma obtained from patients recovered from COVID-19 carry receptor binding domain specific antibodies with potent antiviral activity (Robbiani et al., 2020). These antibodies can directly interact with SARS-CoV-2 proteins and could block the viral entry into the cell (Figure 1). In August 2020, FDA issued EUA for the use of convalescent plasma in hospitalized patients (FDA, 2021a). The samples were considered of high titer if it followed one of the following criteria-a neutralizing antibody titer of ≥250 as per Broad Institute’s neutralizing antibody assay, a signal-to-cut off (S/C) of ≥12 as per Ortho VITROS IgG assay, or a level of ≥1:2,880 in the Mount Sinai COVID-19 ELISA IgG Antibody Test (FDA, 2021a; FDA, 2021b; FDA, 2021c). Units with low titer should be specified and considered to use if high titer samples were not available. The initial dose of 200ml is recommended and further the dose is advised as per condition and requirement of the patient. However, clinical trials have used different values of titer or doses and generally convalescent plasma was examined using immunoassays instead of viral neutralization assays. For example, a study reported use of no minimum neutralizing-antibody titer and single dose of 200–500ml plasma as per the patient’s condition (Joyner et al., 2020a). While in an open label phase II multicentre randomized controlled trial (PLACID Trial) from India, two doses of 200ml with titers ranging from 1:20 to ≥1:1,280 (from immunoassay) was used. In a Chinese trial, single dose of median volume of 200–250ml with titer ≥1: 1:640 was used (Li et al., 2020). Although various studies have shown efficacy of this therapy (Ahn et al., 2020; Duan et al., 2020; Abolghasemi et al., 2020; Hegerova et al., 2020; Xia et al., 2020), some clinical trials have demonstrated that use of convalescent plasma did not reduced the hospitalization duration, severity, or mortality compared to the control groups (Simonovich et al., 2020; Li et al., 2020; Agarwal et al., 2020). Recently completed randomized, double-blind, placebo-controlled trial from Argentina showed reduced disease progression in patients treated with high titer (>1:1,000) convalescent plasma (Libster et al., 2021). Also, another multicentre study from Poland stated that convalescent plasma can be given as supportive therapy to COVID-19 patients due to availability and low frequency adverse events (Moniuszko-Malinowska et al., 2020). Another large-scale observational analysis of patients from the United States who received the convalescent plasma put forward the opinion that this therapy could be beneficial if provided in early days of symptoms onset (Joyner et al., 2020b, Effect of Convalescent Plasma on Mortality among Hospitalized Patients with COVID-19: Initial Three-Month Experience, 2020). The titers of neutralizing antibodies from donor and viral titers in recipient should be considered for providing the convalescent plasma and further clinical outcomes should be studied for optimizing the therapy. There is a lack of studies exclusively investigating the effect of convalescent plasma treatment on SARS-CoV-2 infected children or pregnant women. Additionally, the effectivity of convalescent plasma in patients infected with new SARS-CoV-2 variants also needs to be tested. The ongoing trials may shed more light on efficacy of this therapy against COVID-19 patients. However, many trials were terminated due to reduced cases in the study region. Currently, overall 172 clinical trials have been registered to investigate the use of convalescent plasma in COVID-19 patients (ClinicalTrials.gov, 2021a).
Other than the repurposed drugs the development of anti-SARS-CoV-2 drugs has been accelerated. Recently, a hydroxymethylketone derivative PF-00835,231 showed potency to block protease of SARS-CoV-2 in pre-clinical experiments (Hoffman et al., 2020). This drug has also shown to have suitable pharmaceutical properties and has gathered as an intravenous therapy to cure the disease. Another drug AT-527, a purine nucleotide prodrug, which has shown pan-genotypic efficacy against hepatitis C infection (Good et al., 2020) has also been considered against COVID-19 in a multinational clinical trial (Clinical trial no. NCT04396106). Apart from antiviral drugs, the strategies to tackle increased inflammatory responses during COVID-19 have also been investigated in various studies. Corticosteroids, due to their potent anti-inflammatory effects have gained importance in this regard. Numerous studies investigated a glucocorticoid-dexamethasone but its importance is recently highlighted in large scale RECOVERY trial (Horby et al., 2020a) and further gained recommendation of its use from various platforms. The daily dose of 6mg dexamethasone for 10days was used for hospitalized patients and showed reduced mortality on 28th day compared to the control groups (Horby et al., 2020a). Currently there are 45 registered clinical trials for corticosteroid use against COVID-19 (ClinicalTrials.gov, 2021b).
Understanding the relationship between the pharmacokinetic properties and the therapeutic effect or side-effect of a drug is clinically important (Takahashi, 2000). The bioavailability, volume of distribution, protein binding, half-life, and elimination are the key determinants of successful drug therapy. Especially in severe COVID-19 cases, complex clinical situations may arise due to multiple organ failure and the consequences of drug action cannot be predicted without sufficient pharmacokinetic data (Zaim et al., 2020; Wang T. et al., 2020). The relevant information can be obtained from preclinical and large randomized clinical trials. However, clinicians will continue to confront the challenge of deciding the dosage of repurposed drugs until the pharmacokinetics parameters are better assessed in COVID-19. Furthermore, multi-drug therapy is unavoidable in the treatment of COVID‐19, especially for those patients with pre-existing diseases (Jafari et al., 2020). Therefore, drug-drug interactions (DDIs) are the major concern in clinical practice. It is too early to precisely estimate the effect of DDIs between the experimental drugs used to treat COVID-19 and other prescription drugs. Similarly, the impact of DDIs on pre-existing clinical conditions may not be clearly ruled out. Because, the currently available COVID-19 clinical results are mostly obtained from a relatively short-term study and was not performed in patients taking specific drugs for pre-existing illness (Sciaccaluga et al., 2020). Moreover, clinically significant DDIs can be rationalized in relevant studies performed on appropriate patient populations with high accuracy. Herein, we recapitulate the pharmacokinetics and DDIs of some COVID-19 repurposed drugs under consideration. Additionally, we report the in silico pharmacokinetics prediction of all repurposed drugs discussed in this review (Table 2).
Generally, the drugs are evaluated for potential risk of DDIs during drug development stage to determine the effect of cytochrome P450 (CYP) and P-glycoprotein mediated interactions (Elmeliegy et al., 2020). However, a lack of published clinical data in this area is a major setback. Some efforts are made to document the potential DDIs and they can be accessed from the COVID-19 Drug Interactions site (Liverpool COVID-19 interactions, 2021) published by the Liverpool Drug Interaction Group and the IBM Micromedex Drug Interaction Checking site (IBM Micromedex, 2021) maintained by IBM Watson Health, Greenwood Village, Colorado, United States.
Two antimalarial drugs CQ and HCQ, with or without a macrolide antibiotic AZM, have been studied in multiple clinical trials for the treatment of COVID-19. QTc prolongation, Torsade de Pointes, ventricular arrhythmia, and cardiac deaths are major risks of CQ and HCQ. QT prolongation and potentially life-threatening arrhythmias with HCQ therapy originate from its pharmacodynamics action (O’Laughlin et al., 2016). CQ and HCQ are moderate inhibitors of cytochrome P450 (CYP) 2D6, and potential inhibitors of P-glycoprotein (P-gp) (Rendic and Guengerich, 2020). Therefore, these drugs cause a wide range of potential DDIs by altering the plasma concentration of several drugs. HCQ increases the plasma concentrations of amiodaron, dabigatran, edoxaban, cyclosporine, tacrolimus and sirolimus and decreases the bioavailability of carbamazepine and rifampicin with concomitant use (Liverpool COVID-19 interactions, 2021). The co-administration of HCQ with anti-tubercular drugs such as isoniazid or ethambutol increases the risk of peripheral neuropathy in diabetic patients. CQ and HCQ may decrease the activity of RDV and therefore co-administration of these drugs is not recommended. AZM is not metabolized by cytochromes P450 and it is not a substrate/inhibitor of CYP450. AZM is a known P-glycoprotein (P-gp) inhibitor and, if co-administered with P-gp substrates, it may result in increased serum levels requiring special therapeutic dose monitoring (Scherrmann et al., 2020).
RDV is a prodrug that inhibits viral RNA polymerases. The metabolic stability of RDV studied in various animal models showed that it was relatively stable in the intestine (t1/2 = 40.3–114.1min) but unstable in the liver (t1/2 < 3.9min) (FDA, 2020a). The hepatic instability and the complete first-pass effect prevented oral delivery of RDV. Therefore, the drug is administered through the intravenous route (IV). The IV administration of RDV (200mg) to healthy humans produced AUC0-24 values of 4.8μM/h with moderate protein binding. The in vitro metabolism studies of RDV suggest that it was predominantly metabolized by CYP2C8, CYP2D6, and CYP3A4. It is extensively metabolized in hepatic tissues, and the rate of metabolism by CYP3A4 alone was estimated as 42.1%. The elimination studies carried out in rats and monkeys showed that kidney and bile excretion were the major routes of elimination of RDV. It has a low potential for significant drug-drug interactions because of its rapid clearance. However, the antiviral activity effect of RDV is reduced when co-administered with CQ or HCQ (COVID-19 treatment update, FDA). It is because of the interference of CQ on the intracellular metabolic activation of RDV. Therefore, the co-administration of inhibitors of such CYPs can lead to a potentially high risk of toxic effect (Cattaneo et al., 2020). In a case study it was reported that RDV induced acute hepatotoxic effect in a male COVID-19 patient and realized the toxic effect was due to probable interaction of P-glycoprotein (P-gp) inhibitors (Leegwater et al., 2020). The clinical history of the patient described that the patient was treated with the P-gp inhibitors like chloroquine and amiodarone along with RDV. An adverse effect of an increase in hepatic transaminase activity was also observed in the clinical trial of RDV. RDV was not genotoxic, and it does not impair male fertility (Singh et al., 2020). Based on these preliminary findings, the FDA had granted a EUA for RDV for the treatment of COVID-19 patients (Ison et al., 2020; FDA, 2020d) and was last reissued on October 22, 2020 with some amendments.
The combination of LPV and RTV was approved for the treatment of HIV infection and has recently been investigated in COVID-19 patients (Jean et al., 2020). RTV-boosted LPV (400/100mg) was orally administered to COVID-19 patients. LPV is predominantly metabolized by CYP3A4 isoenzyme, and RTV is a strong inhibitor of CYP3A4 (Chen, 2005; Gregoire et al., 2020). Therefore, RTV prevented the metabolism of LPV. The concentrations of LPV in COVID-19 patients were extremely high compared with HIV-infected patients. No severe adverse events were reported in the clinical trials of LPV and RTV. However, these two drugs can inhibit metabolism and increase plasma levels of several drugs that may induce toxic effects. The potentially severe DDIs were recorded for the concurrent administration of HCQ and LPV/RTV in hospitalized COVID-19 patients (Cattaneo et al., 2020). Cattaneo, et al., reported that more than fifty percent of category D based DDIs and they are attributed to LPV/RTV. The risk of QT interval prolongation by LPV/RTV therapy may be due to inhibition of human ether-a-go-go related gene (hERG) (Sciaccaluga et al., 2020). The cardiotoxicity risk ratio of LPV/RTV is double that of HCQ and AZM (Cattaneo et al., 2020). Furthermore, RTV is shown to increase in the bioavailability and half-life of immunosuppressant drugs such as tacrolimus and cyclosporine by inhibition of CYP3A (Zijp et al., 2020).
The clinical trial results of FPV showed that the peak plasma concentration was achieved at 2h after oral administration (Du and Chen, 2020). The plasma protein binding of FPV was observed 54% in humans. FPV is metabolized in the hepatic tissues majorly by aldehyde oxidase (AO), and partly by xanthine oxidase (Gowen et al., 2015). The metabolites of FPV are rapidly excreted by the kidneys. Particularly, FPV is a mechanism-based AO inhibitor and affects the action of AO in a concentration‐dependent manner. Furthermore, potential DDIs between FPV, cimetidine, and zaleplon have been already reported (Renwick et al., 2002). The chances of occurring DDIs between FPV and citalopram, famciclovir, zaleplon and sulindac are higher as these drugs are also metabolized by AO (Du and Chen, 2020). In vivo study showed inhibitory effect of FPV on CYP2C8 isoenzyme. Therefore, more caution was necessary with anticancer agents such as tamoxifen (AO inhibitor) and paclitaxel (CYP2C8 substrate) (Jafari et al., 2020). Furthermore, a clinical study showed that FPV increases the concentrations of antidiabetic drugs such as pioglitazone or repaglinide with concomitant use that leads to the risk of hypoglycemia. Therefore, a great deal of attention must be paid by clinicians in designing the therapeutic dosage regimen.
For containing the devastating scenario of COVID-19 pandemic, the identification of potent and less toxic therapeutics for COVID-19 is a key research priority. Current research efforts are intensified on the evaluation of existing drugs against SARS-CoV-2 infection. Despite several challenges in SARS-CoV-2 infection, the drug repurposing strategy has proved its important role in the rapid discovery of an effective treatment for COVID-19. Only judicious evaluation of these repurposed drugs may show real insights on its clinical effectiveness and clinical safety in COVID-19 patients. Furthermore, it is essential to address the issue of drug-drug interaction of the repurposed drugs in COVID-19 patients with comorbidities (Jakhmola et al., 2020c). In our knowledge, the present review adequately provides all relevant information currently needed to assist clinicians and researchers working in this area.
HJ and OI devised the idea of study. OI, SJ, and EM contributed to literature search. OI, SJ, and EM prepared the initial draft of manuscript. OI developed tables and figure. HJ did the proof reading, correction, modifications and final drafting.
The authors declare that the research was conducted in the absence of any commercial or financial relationships that could be construed as a potential conflict of interest.
We thank the Department of Biotechnology and Ministry of Human Resource and Development, Govt. of India for the fellowship to OI and SJ, respectively, in the form of a research stipend. The funding organization has not played any role in the study design or the preparation of the manuscript. We appreciate Ms. Annu Rani and our other lab colleagues for their insights, discussions and advice. We gratefully acknowledge the Indian Institute of Technology Indore and Devi Ahilya Vishwavidyalaya, Indore for providing facilities and support.
Abolghasemi, H., Eshghi, P., Cheraghali, A. M., Imani Fooladi, A. A., Bolouki Moghaddam, F., Imanizadeh, S., et al. (2020). Clinical efficacy of convalescent plasma for treatment of COVID-19 infections: results of a multicenter clinical study. Transfus. Apher. Sci. 59, 102875. doi:10.1016/j.transci.2020.102875
Acharya, Y., and Sayed, A. (2020). Chloroquine and hydroxychloroquine as a repurposed agent against COVID-19: a narrative review. Ther. Adv. Infect. Dis. 7, 2049936120947517. doi:10.1177/2049936120947517
Agarwal, A., Mukherjee, A., Kumar, G., Chatterjee, P., Bhatnagar, T., and Malhotra, P. (2020). Convalescent plasma in the management of moderate covid-19 in adults in India: open label phase II multicentre randomised controlled trial (PLACID Trial). BMJ 371, m3939. doi:10.1136/bmj.m3939
Agostini, M. L., Andres, E. L., Sims, A. C., Graham, R. L., Sheahan, T. P., Lu, X., et al. (2018). Coronavirus susceptibility to the antiviral remdesivir (GS-5734) is mediated by the viral polymerase and the proofreading exoribonuclease. MBio 9, e00221-18. doi:10.1128/mBio.00221-18
Agrawal, P. (2015). Advantages and challenges in drug Re-profiling. J. Pharmacovigilance s2, e002. doi:10.4172/2329-6887.s2-e002
Ahn, J. Y., Sohn, Y., Lee, S. H., Cho, Y., Hyun, J. H., Baek, Y. J., et al. (2020). Use of convalescent plasma therapy in two COVID-19 patients with acute respiratory distress syndrome in korea. J. Korean. Med. Sci. 35, e149. doi:10.3346/jkms.2020.35.e149
Al-Tawfiq, J. A., Al-Homoud, A. H., and Memish, Z. A. (2020). remdesivir as a possible therapeutic option for the COVID-19. Trav. Med. Infect. Dis. 34, 101615. doi:10.1016/j.tmaid.2020.101615
Amirian, E. S., and Levy, J. K. (2020). Current knowledge about the antivirals remdesivir (GS-5734) and GS-441524 as therapeutic options for coronaviruses. One Health 9, 100128. doi:10.1016/j.onehlt.2020.100128
Arshad, S., Kilgore, P., Chaudhry, Z. S., Jacobsen, G., Wang, D. D., Huitsing, K., et al. 2020). Treatment with hydroxychloroquine, azithromycin, and combination in patients hospitalized with COVID-19. Int. J. Infect. Dis. 97, 396–403. doi:10.1016/j.ijid.2020.06.099
Badgujar, K. C., Ram, A. H., Zanznay, R., Kadam, H., and Badgujar, V. C. (2020). Remdesivir for COVID-19: a review of pharmacology, mechanism of action, in-vitro activity and clinical use based on available case studies. J. Drug Deliv. Ther. 10, 264–270. doi:10.22270/jddt.v10i4-s.4313
Ballow, C. H., and Amsden, G. W. (1992). Azithromycin: the first azalide antibiotic. Ann. Pharmacother. 26, 1253–1261. doi:10.1177/106002809202601014
Beigel, J. H., Tomashek, K. M., Dodd, L. E., Mehta, A. K., Zingman, B. S., Kalil, A. C., et al. (2020). Remdesivir for the treatment of covid-19 - final report. N. Engl. J. Med. 383 (19), 1813–1826. doi:10.1056/NEJMoa2007764
Beyls, C., Martin, N., Hermida, A., Abou-Arab, O., and Mahjoub, Y. (2020). Lopinavir-ritonavir treatment for COVID-19 infection in intensive care unit: risk of bradycardia. Circ. Arrhythm. Electrophysiol. 13, e008798. doi:10.1161/CIRCEP.120.008798
Blaising, J., Polyak, S. J., and Pécheur, E. I. (2014). Arbidol as a broad-spectrum antiviral: an update. Antivir. Res. 107, 84–94. doi:10.1016/j.antiviral.2014.04.006
Bonny, A., Talle, M. A., Ngantcha, M., and Tayebjee, M. H. (2020). Early treatment of COVID-19 patients with hydroxychloroquine and azithromycin: a retrospective analysis of 1061 cases in Marseille, France (2020). Trav. Med. Infect. Dis. 35, 101738. doi:10.1016/j.tmaid.2020.101861
Borba, M. G. S., Val, F. F. A., Sampaio, V. S., Alexandre, M. A. A., Melo, G. C., Brito, M., et al. (2020). Effect of high vs low doses of chloroquine diphosphate as adjunctive therapy for patients hospitalized with severe acute respiratory syndrome coronavirus 2 (SARS-CoV-2) infection: a randomized clinical trial. JAMA Netw. Open 3, e208857. doi:10.1001/jamanetworkopen.2020.8857
Borio, L. L., Toner, E., and Bartlett, J. (2018). 08-11-2005 - overview of oseltamivir and key points | CBN article. Clinicians’ biosecurity news | johns hopkins center for health security. Available at: https://www.centerforhealthsecurity.org/cbn/2005/cbnTOPICS_081105.html (Accessed June 25, 2020).
Briolant, S., Garin, D., Scaramozzino, N., Jouan, A., and Crance, J. M. (2004). In vitro inhibition of Chikungunya and Semliki Forest viruses replication by antiviral compounds: synergistic effect of interferon-alpha and ribavirin combination. Antivir. Res 61, 111–117. doi:10.1016/j.antiviral.2003.09.005
Brown, A. J., Won, J. J., Graham, R. L., Dinnon, K. H., Sims, A. C., Feng, J. Y., et al. (2019). Broad spectrum antiviral remdesivir inhibits human endemic and zoonotic deltacoronaviruses with a highly divergent RNA dependent RNA polymerase. Antivir. Res 169, 104541. doi:10.1016/j.antiviral.2019.104541
Cai, Q., Yang, M., Liu, D., Chen, J., Shu, D., Xia, J., et al. (2020). Experimental treatment with favipiravir for COVID-19: an open-label control study. Proc. Est. Acad. Sci. Eng. 6 (10), 1192–1198. doi:10.1016/j.eng.2020.03.007
Cantuti-Castelvetri, L., Ojha, R., Pedro, L. D., Djannatian, M., Franz, J., Kuivanen, S., et al. (2020). Neuropilin-1 facilitates SARS-CoV-2 cell entry and infectivity. Science 370 (6518), 856–860. doi:10.1126/science.abd2985
Cao, B., Wang, Y., Wen, D., Liu, W., Wang, J., Fan, G., et al. (2020). A trial of lopinavir-ritonavir in adults hospitalized with severe covid-19. N. Engl. J. Med. 382, 1787–1799. doi:10.1056/NEJMoa2001282
Cattaneo, D., Pasina, L., Maggioni, A. P., Giacomelli, A., Oreni, L., Covizzi, A., et al. (2020). Drug-drug interactions and prescription appropriateness in patients with COVID-19: a retrospective analysis from a reference hospital in northern Italy. Drugs Aging 37, 925–933. doi:10.1007/s40266-020-00812-8
Cavalcanti, A. B., Zampieri, F. G., Rosa, R. G., Azevedo, L. C. P., Veiga, V. C., and Avezum, A. (2020). Hydroxychloroquine with or without azithromycin in mild-to-moderate covid-19. N. Engl. J. Med. 383 (21), 2041–2052. doi:10.1056/NEJMoa2019014
Chandwani, A., and Shuter, J. (2008). Lopinavir/ritonavir in the treatment of HIV-1 infection: a review. Ther. Clin. Risk Manag. 4, 1023–1033. doi:10.2147/tcrm.s3285
Chen, J., Wang, S., Jia, X., Bajimaya, S., Lin, H., Tam, V. H., et al. (2005). Disposition of flavonoids via recycling: comparison of intestinal versus hepatic disposition. Drug Metab. Dispos. 33, 1777. doi:10.1124/dmd.105.003673
Chen, Y., Liu, Q., and Guo, D. (2020). Emerging coronaviruses: genome structure, replication, and pathogenesis. J. Med. Virol. 92 (4), 418–423. doi:10.1002/jmv.25681
Cheng, C. Y., Lee, Y. L., Chen, C. P., Lin, Y. C., Liu, C. E., Liao, C. H., et al. (2020). Lopinavir/ritonavir did not shorten the duration of SARS CoV-2 shedding in patients with mild pneumonia in Taiwan. J. Microbiol. Immunol. Infect. 53, 488–492. doi:10.1016/j.jmii.2020.03.032
Cho, A., Saunders, O. L., Butler, T., Zhang, L., Xu, J., Vela, J. E., et al. (2012). Synthesis and antiviral activity of a series of 1'-substituted 4-aza-7,9-dideazaadenosine C-nucleosides. Bioorg. Med. Chem. Lett. 22, 2705–2707. doi:10.1016/j.bmcl.2012.02.105
Chu, C. M., Cheng, V. C., Hung, I. F., Wong, M. M., Chan, K. H., Chan, K. S., et al. (2004). Role of lopinavir/ritonavir in the treatment of SARS: initial virological and clinical findings. Thorax 59, 252–256. doi:10.1136/thorax.2003.012658
ClinicalTrials.gov (2020a). Azithromycin in hospitalized COVID-19 patients (AIC). Available at: https://clinicaltrials.gov/ct2/results?cond=Covid-19&term=azithromycin&cntry=&state=&city=&dist= (Accessed January 10, 2020).
ClinicalTrials.gov (2020b). Chloroquine covid-19–list results. Available at: https://clinicaltrials.gov/ct2/results?cond=Covid-19&term=chloroquine&cntry=&state=&city=&dist= (Accessed January 10, 2020).
ClinicalTrials.gov (2020c). Favipiravir covid-19. Available at: https://clinicaltrials.gov/ct2/results?cond=Covid-19&term=Favipiravir&cntry=&state=&city=&dist= (Accessed January 10, 2020).
ClinicalTrials.gov (2020d). Hydroxychloroquine covid-19. Available at: https://clinicaltrials.gov/ct2/results?cond=Covid-19&term=hydroxychloroquine&cntry=&state=&city=&dist= (Accessed January 10, 2020).
ClinicalTrials.gov (2020e). Lopinavir covid-19. Available at: https://clinicaltrials.gov/ct2/results?cond=Covid-19&term=lopinavir&cntry=&state=&city=&dist= (Accessed January 10, 2020).
ClinicalTrials.gov (2020f). Oseltamivir covid-19. Available at: https://clinicaltrials.gov/ct2/results?cond=Covid-19&term=Oseltamivir&cntry=&state=&city=&dist= (Accessed January 10, 2020).
ClinicalTrials.gov (2020g). Remdesivir covid-19. Available at: https://clinicaltrials.gov/ct2/results?cond=Covid-19&term=remdesivir&cntry=&state=&city=&dist= (Accessed January 10, 2020).
ClinicalTrials.gov (2020h). Ribavirin covid-19. Available at: https://clinicaltrials.gov/ct2/results?cond=Covid-19&term=Ribavirin&cntry=&state=&city=&dist= (Accessed January 10, 2020).
ClinicalTrials.gov (2020i). Umifenovir covid-19. Available at: https://clinicaltrials.gov/ct2/results?cond=Covid-19&term=Umifenovir&cntry=&state=&city=&dist= (Accessed January 10, 2020).
ClinicalTrials.gov (2021a). Convalescent plasma covid19. Available at: https://www.clinicaltrials.gov/ct2/results?term=convalescent+plasma&cond=Covid19 (Accessed January 13, 2021).
ClinicalTrials.gov (2021b). Dexamethasone covid19. Available at: https://www.clinicaltrials.gov/ct2/results?term=dexamethasone&cond=Covid19&Search=Apply&age_v=&gndr=&type=&rslt= (Accessed January 13, 2021).
Cohen, M. S. (2020). Hydroxychloroquine for the prevention of covid-19 - searching for evidence. N. Engl. J. Med. 383, 585–586. doi:10.1056/NEJMe2020388
Cong, Y., Hart, B. J., Gross, R., Zhou, H., Frieman, M., Bollinger, L., et al. (2018). MERS-CoV pathogenesis and antiviral efficacy of licensed drugs in human monocyte-derived antigen-presenting cells. PLoS One 13, e0194868. doi:10.1371/journal.pone.0194868
Damle, B., Vourvahis, M., Wang, E., Leaney, J., and Corrigan, B. (2020). Clinical pharmacology perspectives on the antiviral activity of azithromycin and use in COVID-19. Clin. Pharmacol. Ther. 108 (2), 201–211. doi:10.1002/cpt.1857
Deng, L., Li, C., Zeng, Q., Liu, X., Li, X., Zhang, H., et al. (2020). Arbidol combined with LPV/r versus LPV/r alone against Corona Virus Disease 2019: a retrospective cohort study. J. Infect. 9,100128. doi:10.1016/j.jinf.2020.03.002
Devaux, C. A., Rolain, J. M., Colson, P., and Raoult, D. (2020). New insights on the antiviral effects of chloroquine against coronavirus: what to expect for COVID-19?. Int. J. Antimicrob. Agents 55, 105938. doi:10.1016/j.ijantimicag.2020.105938
Du, Y., and Chen, X. (2020). Favipiravir: pharmacokinetics and concerns about clinical trials for 2019‐nCoV infection. Clin. Pharmacol. Ther. 108 (2), 242–247. doi:10.1002/cpt.1844
Duan, K., Liu, B., Li, C., Zhang, H., Yu, T., Qu, J., et al. (2020). Effectiveness of convalescent plasma therapy in severe COVID-19 patients. Proc. Natl. Acad. Sci. U.S.A. 117, 9490–9496. doi:10.1073/pnas.2004168117
Elia, G., Belloli, C., Cirone, F., Lucente, M. S., Caruso, M., Martella, V., et al. (2008). In vitro efficacy of ribavirin against canine distemper virus. Antivir. Res. 77, 108–113. doi:10.1016/j.antiviral.2007.09.004
Elmeliegy, M., Vourvahis, M., Guo, C., and Wang, D. D. (2020). Effect of P-glycoprotein (P-gp) inducers on exposure of P-gp substrates: review of clinical drug-drug interaction studies. Clin. Pharmacokinet. 59, 699–714. doi:10.1007/s40262-020-00867-1
Eslami, G., Mousaviasl, S., Radmanesh, E., Jelvay, S., Bitaraf, S., Simmons, B., et al. (2020). The impact of sofosbuvir/daclatasvir or ribavirin in patients with severe COVID-19. J. Antimicrob. Chemother. 75, 3366–3372. doi:10.1093/jac/dkaa331
Falzarano, D., de Wit, E., Rasmussen, A. L., Feldmann, F., Okumura, A., Scott, D. P., et al. (2013). Treatment with interferon-α2b and ribavirin improves outcome in MERS-CoV-infected rhesus macaques. Nat. Med. 19, 1313–1317. doi:10.1038/nm.3362
FDA (2020a). FDA EAU letter -Remdesivir -COVID-19. Available at: https://www.fda.gov/media/137564/download (Accessed November 3, 2020).
FDA (2020b). FDA fact sheet -Chloroquine phosphate -COVID-19 Available at: https://www.fda.gov/media/136536/download (Accessed November 4, 2020).
FDA (2020c). FDA fact sheet -Hydroxychloroquine sulfate -COVID-19. Available at: https://www.fda.gov/media/136537/download (Accessed November 4, 2020).
FDA (2020d). FDA FACT sheet -Remdesivir -COVID-19. Available at: https://www.fda.gov/media/143189/download (Accessed November 4, 2020).
FDA (2021a). FDA EAU letter –Convalescent plasma. Available at: https://www.fda.gov/media/141477/download (Accessed January 13, 2021).
FDA (2021b). FDA EAU clinical memorandom Convalescent plasma Available at: https://www.fda.gov/media/141480/download (Accessed January 13, 2021).
FDA (2021c). FDA Fact sheet –Convalescent plasma -COVID-19. Available at: https://www.fda.gov/media/141478/download (Accessed January 13, 2021).
Fink, S. L., Vojtech, L., Wagoner, J., Slivinski, N. S. J., Jackson, K. J., Wang, R., et al. (2018). The antiviral drug arbidol inhibits zika virus. Sci. Rep. 8, 8989. doi:10.1038/s41598-018-27224-4
Fiolet, T., Guihur, A., Rebeaud, M., Mulot, M., Peiffer-Smadja, N., and Mahamat-Saleh, Y. (2020). Effect of hydroxychloroquine with or without azithromycin on the mortality of coronavirus disease 2019 (COVID-19) patients: a systematic review and meta-analysis. Clin. Microbiol. Infect. 27 (1), 19–27. doi:10.1016/j.cmi.2020.08.022
Frediansyah, A., Nainu, F., Dhama, K., Mudatsir, M., and Harapan, H. (2020). Remdesivir and its antiviral activity against COVID-19: a systematic review. Clin. Epidemiol. Glob. Health 9, 123–127. doi:10.1016/j.cegh.2020.07.011
Furtado, R. H. M., Berwanger, O., Fonseca, H. A., Corrêa, T. D., Ferraz, L. R., Lapa, M. G., et al. (2020). Azithromycin in addition to standard of care versus standard of care alone in the treatment of patients admitted to the hospital with severe COVID-19 in Brazil (COALITION II): a randomised clinical trial. Lancet 396, 959–967. doi:10.1016/s0140-6736(20)31862-6
Furuta, Y., Gowen, B. B., Takahashi, K., Shiraki, K., Smee, D. F., and Barnard, D. L. (2013). Favipiravir (T-705), a novel viral RNA polymerase inhibitor (2013). Antivir. Res. 100, 446–454. doi:10.1016/j.antiviral.2013.09.015
Furuta, Y., Komeno, T., and Nakamura, T. (2017). Favipiravir (T-705), a broad spectrum inhibitor of viral RNA polymerase. Proc. Jpn. Acad. Ser. B Phys. Biol. Sci. 93, 449–463. doi:10.2183/pjab.93.027
Galli, A., Mens, H., Gottwein, J. M., Gerstoft, J., and Bukh, J. (2018). Antiviral effect of ribavirin against HCV associated with increased frequency of G-to-A and C-to-U transitions in infectious cell culture model. Sci. Rep. 8, 1–13. doi:10.1038/s41598-018-22620-2
Gautret, P., Lagier, J. C., Parola, P., Meddeb, L., Mailhe, M., and Doudier, B. (2020). Hydroxychloroquine and azithromycin as a treatment of COVID-19: results of an open-label non-randomized clinical trial. Int. J. Antimicrob. Agents 57 (1), 106239. doi:10.1016/j.ijantimicag.2020.106239
Geleris, J., Sun, Y., Platt, J., Zucker, J., Baldwin, M., Hripcsak, G., et al. (2020). Observational study of hydroxychloroquine in hospitalized patients with covid-19. N. Engl. J. Med. 382, 2411–2418. doi:10.3410/f.737908482.793576143
Gish, R. G. (2006). Treating HCV with ribavirin analogues and ribavirin-like molecules. J. Antimicrob. Chemother. 57, 8–13. doi:10.1093/jac/dki405
Good, S. S., Moussa, A., Zhou, X.-J., Pietropaolo, K., and Sommadossi, J.-P. (2020). Preclinical evaluation of AT-527, a novel guanosine nucleotide prodrug with potent, pan-genotypic activity against hepatitis C virus. PLoS One 15, e0227104. doi:10.1371/journal.pone.0227104
Govorkova, E. A., Ilyushina, N. A., McClaren, J. L., Naipospos, T. S. P., Douangngeun, B., and Webster, R. G. (2009). Susceptibility of highly pathogenic H5N1 influenza viruses to the neuraminidase inhibitor oseltamivir differs in vitro and in a mouse model. Antimicrob. Agents Chemother. 53, 3088–3096. doi:10.1128/aac.01667-08
Gowen, B. B., Sefing, E. J., Westover, J. B., Smee, D. F., Hagloch, J., Furuta, Y., et al. (2015). Alterations in favipiravir (T-705) pharmacokinetics and biodistribution in a hamster model of viral hemorrhagic fever. Antivir. Res. 121, 132–137. doi:10.1016/j.antiviral.2015.07.003
Gregoire, M., Le Turnier, P., Gaborit, B. J., Veyrac, G., Lecomte, R., Boutoille, D., et al. (2020). Lopinavir pharmacokinetics in COVID-19 patients. J. Antimicrob. Chemother. 75 (9), 2702–2704. doi:10.1093/jac/dkaa195
Hasan, A., Paray, B. A., Hussain, A., Qadir, F. A., Attar, F., Aziz, F. M., et al. (2020). A review on the cleavage priming of the spike protein on coronavirus by angiotensin-converting enzyme-2 and furin. J. Biomol. Struct. Dyn. 2020, 1–9. doi:10.1080/07391102.2020.1754293
Hegerova, L., Gooley, T. A., Sweerus, K. A., Maree, C., Bailey, N., Bailey, M., et al. (2020). Use of convalescent plasma in hospitalized patients with COVID-19: case series. Blood 136, 759–762. doi:10.1182/blood.2020006964
Hoffman, R. L., Kania, R. S., Brothers, M. A., Davies, J. F., Ferre, R. A., Gajiwala, K. S., et al. (2020). Discovery of ketone-based covalent inhibitors of coronavirus 3CL proteases for the potential therapeutic treatment of COVID-19. J. Med. Chem. 63, 12725–12747. doi:10.1021/acs.jmedchem.0c01063
Hoffmann, M., Kleine-Weber, H., Schroeder, S., Krüger, N., Herrler, T., Erichsen, S., et al. (2020). SARS-CoV-2 cell entry depends on ACE2 and TMPRSS2 and is blocked by a clinically proven protease inhibitor. Cell 181, 271–280.e8. doi:10.1016/j.cell.2020.02.052
Horby, P., Lim, W. S., Emberson, J. R., Mafham, M., Bell, J. L., Linsell, L., et al. RECOVERY Collaborative Group (2020a). Dexamethasone in hospitalized patients with covid-19 - preliminary report. N. Engl. J. Med. [Epub ahead of print]. doi:10.1056/NEJMoa2021436
Horby, P., Mafham, M., Linsell, L., Bell, J. L., Staplin, N., Emberson, J. R., et al. RECOVERY Collaborative Group (2020b). Effect of hydroxychloroquine in hospitalized patients with covid-19. N. Engl. J. Med. 383, 2030–2040. doi:10.1093/eurheartj/ehaa935
Horby, P. W., Mafham, M., Bell, J. L., Linsell, L., Staplin, N., Emberson, J., et al. (2020). Lopinavir–ritonavir in patients admitted to hospital with COVID-19 (RECOVERY): a randomised, controlled, open-label, platform trial. Lancet 396, 1345–1352. doi:10.1016/s0140-6736(20)32013-4
Huang, D., Yu, H., Wang, T., Yang, H., Yao, R., and Liang, Z. (2020). Efficacy and safety of umifenovir for coronavirus disease 2019 (COVID-19): a systematic review and meta-analysis. J. Med. Virol. 93 (1), 481–490. doi:10.1002/jmv.26256
Huang, M., Tang, T., Pang, P., Li, M., Ma, R., Lu, J., et al. (2020). Treating COVID-19 with chloroquine. J. Mol. Cel Biol. 12, 322–325. doi:10.1093/jmcb/mjaa014
Huang, Y.-Q., Tang, S.-Q., Xu, X.-L., Zeng, Y.-M., He, X.-Q., Li, Y., et al. (2020). No statistically apparent difference in antiviral effectiveness observed among ribavirin plus interferon-alpha, lopinavir/ritonavir plus interferon-alpha, and ribavirin plus lopinavir/ritonavir plus interferon-alpha in patients with mild to moderate coronavirus disease 2019: results of a randomized, open-labeled prospective study. Front. Pharmacol. 11, 1071. doi:10.3389/fphar.2020.01071
Hughes, J. H., Sweeney, K., Ahadieh, S., and Ouellet, D. (2020). Predictions of systemic, intracellular, and lung concentrations of azithromycin with different dosing regimens used in COVID-19 clinical trials. CPT Pharmacometrics Syst. Pharmacol. 9 (8), 435–443. doi:10.1002/psp4.12537
Hung, I. F. N., Lung, K. C., Tso, E. Y. K., Liu, R., Chung, T. W. H., Chu, M. Y., et al. (2020). Triple combination of interferon beta-1b, lopinavir–ritonavir, and ribavirin in the treatment of patients admitted to hospital with COVID-19: an open-label, randomised, phase 2 trial. Lancet 395, 1695–1704. doi:10.1016/S0140-6736(20)31042-4
Hussain, S., Chen, Y., Yang, Y., Xu, J., Peng, Y., Wu, Y., et al. (2005). Identification of novel subgenomic RNAs and noncanonical transcription initiation signals of severe acute respiratory syndrome coronavirus. J. Virol. 79 (9), 5288–5295. doi:10.1128/jvi.79.9.5288-5295.2005
IBM Micromedex (2021). IBM Watson health products: system status. Available at: https://www.micromedexsolutions.com/ (Accessed January 13, 2021).
Ison, M. G., Wolfe, C., and Boucher, H. W. (2020). Emergency use authorization of remdesivir: the need for a transparent distribution process. JAMA 323 (23), 2365–2366. doi:10.1001/jama.2020.8863
Jafari, A., Dadkhahfar, S., and Perseh, S. (2020). Considerations for interactions of drugs used for the treatment of COVID-19 with anti-cancer treatments. Crit. Rev. Oncol. Hematol. 151, 102982. doi:10.1016/j.critrevonc.2020.102982
Jakhmola, S., Indari, O., Baral, B., Kashyap, D., Varshney, N., Das, A., et al. (2020c). Comorbidity assessment is essential during COVID-19 treatment. Front. Physiol. 11, 984. doi:10.3389/fphys.2020.00984
Jakhmola, S., Indari, O., Chatterjee, S., and Jha, H. C. (2020a). SARS-CoV-2, an underestimated pathogen of the nervous system. SN Compr. Clin. Med. 2 (11), 2137–2146. doi:10.1007/s42399-020-00522-7
Jakhmola, S., Indari, O., Kashyap, D., Varshney, N., Rani, A., Sonkar, C., et al. (2020b). Recent updates on COVID-19: a holistic review. Heliyon 6, e05706. doi:10.1016/j.heliyon.2020.e05706
Jean, S. S., Lee, P. I., and Hsueh, P. R. (2020). Treatment options for COVID-19: the reality and challenges (2020). J. Microbiol. Immunol. Infect. 53, 436–443. doi:10.1016/j.jmii.2020.03.034
Joyner, M. J., Bruno, K. A., Klassen, S. A., Kunze, K. L., Johnson, P. W., Lesser, E. R., et al. (2020a). Safety update: COVID-19 convalescent plasma in 20,000 hospitalized patients. Mayo Clin. Proc. 95, 1888–1897. doi:10.1016/j.mayocp.2020.06.028
Joyner, M. J., Senefeld, J. W., Klassen, S. A., Mills, J. R., Johnson, P. W., Theel, E. S., et al. (2020b). Effect of convalescent plasma on mortality among hospitalized patients with COVID-19: initial three-month experience. Available at: https://ncrc.jhsph.edu/research/effect-of-convalescent-plasma-on-mortality-among-hospitalized-patients-with-covid-19-initial-three-month-experience/ (Accessed January 13, 2021).
Kalil, A. C., Patterson, T. F., Mehta, A. K., Tomashek, K. M., Wolfe, C. R., Ghazaryan, V., et al. (2020). Baricitinib plus remdesivir for hospitalized adults with covid-19. N. Engl. J. Med. [Epub ahead of print]. doi:10.1056/NEJMoa2031994
Kaptein, S. J. F., Jacobs, S., Langendries, L., Seldeslachts, L., ter Horst, S., Liesenborghs, L., et al. (2020). Favipiravir at high doses has potent antiviral activity in SARS-CoV-2−infected hamsters, whereas hydroxychloroquine lacks activity. Proc. Natl. Acad. Sci. 117, 26955–26965. doi:10.1073/pnas.2014441117
Karolyi, M., Pawelka, E., Mader, T., Omid, S., Kelani, H., Ely, S., et al. (2020). Hydroxychloroquine versus lopinavir/ritonavir in severe COVID-19 patients : results from a real-life patient cohort. Wien. Klin. Wochenschr. [Epub ahead of print]. doi:10.1007/s00508-020-01720-y
Keyaerts, E., Li, S., Vijgen, L., Rysman, E., Verbeeck, J., Van Ranst, M., et al. (2009). Antiviral activity of chloroquine against human coronavirus OC43 infection in newborn mice. Antimicrob. Agents Chemother. 53, 3416–3421. doi:10.1128/aac.01509-08
Keyaerts, E., Vijgen, L., Maes, P., Neyts, J., and Van Ranst, M. (2004). In vitro inhibition of severe acute respiratory syndrome coronavirus by chloroquine. Biochem. Biophys. Res. Commun. 323, 264–268. doi:10.1016/j.bbrc.2004.08.085
Lê, M. P., Jaquet, P., Patrier, J., Wicky, P.-H., Le Hingrat, Q., Veyrier, M., et al. (2020). Pharmacokinetics of lopinavir/ritonavir oral solution to treat COVID-19 in mechanically ventilated ICU patients. J. Antimicrob. Chemother. 75, 2657–2660. doi:10.1093/jac/dkaa261
Lai, M. M., and Cavanagh, D. (1997). The molecular biology of coronaviruses. Adv. Virus Res. 48, 1–100.
Leegwater, E., Strik, A., Wilms, E. B., Bosma, L. B. E., Burger, D. M., Ottens, T. H., et al. (2020). Drug-induced liver injury in a COVID-19 patient: potential interaction of remdesivir with P-glycoprotein inhibitors. Clin. Infect. Dis. [Epub ahead of print]. doi:10.1093/cid/ciaa883
Leyssen, P., Balzarini, J., De Clercq, E., and Neyts, J. (2005). The predominant mechanism by which ribavirin exerts its antiviral activity in vitro against flaviviruses and paramyxoviruses is mediated by inhibition of IMP dehydrogenase. J. Virol. 79, 1943–1947. doi:10.1128/jvi.79.3.1943-1947.2005
Li, C., Zhu, X., Ji, X., Quanquin, N., Deng, Y.-Q., Tian, M., et al. (2017). Chloroquine, a FDA-approved drug, prevents zika virus infection and its associated congenital microcephaly in mice. EBioMedicine 24, 189–194. doi:10.1016/j.ebiom.2017.09.034
Li, F. (2016). Structure, function, and evolution of coronavirus spike proteins. Annu. Rev. Virol. 3, 237–261. doi:10.1146/annurev-virology-110615-042301
Li, L., Zhang, W., Hu, Y., Tong, X., Zheng, S., Yang, J., et al. (2020). Effect of convalescent plasma therapy on time to clinical improvement in patients with severe and life-threatening COVID-19: a randomized clinical trial. JAMA 324, 460–470. doi:10.3410/f.738066145.793578637
Li, Z.-H., Li, C.-M., Ling, P., Shen, F.-H., Chen, S.-H., Liu, C.-C., et al. (2008). Ribavirin reduces mortality in enterovirus 71-infected mice by decreasing viral replication. J. Infect. Dis. 197, 854–857. doi:10.1086/527326
Lian, N., Xie, H., Lin, S., Huang, J., Zhao, J., and Lin, Q. (2020). Umifenovir treatment is not associated with improved outcomes in patients with coronavirus disease 2019: a retrospective study. Clin. Microbiol. Infect. 26, 917–921. doi:10.1016/j.cmi.2020.04.026
Libster, R., Pérez Marc, G., Wappner, D., Coviello, S., Bianchi, A., Braem, V., et al. (2021). Early high-titer plasma therapy to prevent severe covid-19 in older adults. N. Engl. J. Med. [Epub ahead of print]. doi:10.1056/NEJMoa2033700
Liu, J., Cao, R., Xu, M., Wang, X., Zhang, H., Hu, H., et al. (2020). Hydroxychloroquine, a less toxic derivative of chloroquine, is effective in inhibiting SARS-CoV-2 infection in vitro. Cell Discov. 6, 16. doi:10.1038/s41421-020-0156-0
Liu, W., Zhou, P., Chen, K., Ye, Z., Liu, F., Li, X., et al. (2020). Efficacy and safety of antiviral treatment for COVID-19 from evidence in studies of SARS-CoV-2 and other acute viral infections: a systematic review and meta-analysis. CMAJ 192, E734–E744. doi:10.1503/cmaj.200647
Liverpool COVID-19 interactions (2021). Interactions for colchicine and aspirin (as an antiplatelet COVID-19 adjunct therapy) are now on the checker. Available at: https://www.covid19-druginteractions.org/ (Accessed January 13, 2021).
Lo, M. K., Albariño, C. G., Perry, J. K., Chang, S., Tchesnokov, E. P., Guerrero, L., et al. (2020). Remdesivir targets a structurally analogous region of the Ebola virus and SARS-CoV-2 polymerases. Proc. Natl. Acad. Sci. U.S.A. 117, 26946–26954. doi:10.1073/pnas.2012294117
Madelain, V., Nguyen, T. H. T., Olivo, A., de Lamballerie, X., Guedj, J., Taburet, A.-M., et al. (2016). Ebola virus infection: review of the pharmacokinetic and pharmacodynamic properties of drugs considered for testing in human efficacy trials. Clin. Pharmacokinet. 55, 907–923. doi:10.1007/s40262-015-0364-1
Mahase, E. (2020). Covid-19: US approves remdesivir despite WHO trial showing lack of efficacy. BMJ 371, m4120. doi:10.1136/bmj.m4120
McCullough, P. A. (2020). Favipiravir and the need for early ambulatory treatment of SARS-CoV2 infection (COVID-19). Antimicrob. Agents Chemother. [Epub ahead of print]. doi:10.1128/AAC.02017-20
Mercuro, N. J., Yen, C. F., Shim, D. J., Maher, T. R., McCoy, C. M., Zimetbaum, P. J., et al. (2020). Risk of QT interval prolongation associated with use of hydroxychloroquine with or without concomitant azithromycin among hospitalized patients testing positive for coronavirus disease 2019 (COVID-19). JAMA Cardiol. [Epub ahead of print]. doi:10.1001/jamacardio.2020.1834
Moniuszko-Malinowska, A., Czupryna, P., Zarębska-Michaluk, D., Tomasiewicz, K., Pancewicz, S., Rorat, M., et al. (2020). Convalescent plasma transfusion for the treatment of COVID-19—experience from Poland: a multicenter study. J. Clin. Med. Res. 10, 28. doi:10.3390/jcm10010028
Murphy, B. G., Perron, M., Murakami, E., Bauer, K., Park, Y., Eckstrand, C., et al. (2018). The nucleoside analog GS-441524 strongly inhibits feline infectious peritonitis (FIP) virus in tissue culture and experimental cat infection studies. Vet. Microbiol. 219, 226–233. doi:10.1016/j.vetmic.2018.04.026
O’Laughlin, J. P., Mehta, P. H., and Wong, B. C. (2016). Life threatening severe QTc prolongation in patient with systemic lupus erythematosus due to hydroxychloroquine. Case Rep. Cardiol. 2016, 4626279. doi:10.1155/2016/4626279
Ooi, E. E., Chew, J. S. W., Loh, J. P., and Chua, R. C. S. (2006). In vitro inhibition of human influenza A virus replication by chloroquine. Virol. J. 3, 1–3. doi:10.1186/1743-422X-3-39
Pan, H., Peto, R., Henao-Restrepo, A.-M., Preziosi, M.-P., Sathiyamoorthy, V., Karim, Q. A., et al. WHO Solidarity Trial Consortium (2020). Repurposed antiviral drugs for covid-19 - interim WHO solidarity trial results. N. Engl. J. Med. [Epub ahead of print]. doi:10.1056/NEJMoa2023184
PMDA (2020). An evaluation report of Avigan (favipiravir), Toyama Chemical Co., Ltd. Available at: https://www.pmda.go.jp/files/000210319.pdf (Accessed November 3, 2020).
Pruijssers, A. J., George, A. S., Schäfer, A., Leist, S. R., Gralinksi, L. E., Dinnon, K. H., et al. (2020). Remdesivir inhibits SARS-CoV-2 in human lung cells and chimeric SARS-CoV expressing the SARS-CoV-2 RNA polymerase in mice. Cell Rep. 32, 107940. doi:10.1016/j.celrep.2020.107940
Rendic, S., and Guengerich, F. P. (2020). Metabolism and interactions of chloroquine and hydroxychloroquine with human cytochrome P450 enzymes and drug transporters. Curr. Drug Metab. 21, 1127–1135. doi:10.2174/1389200221999201208211537
Renwick, A. B., Ball, S. E., Tredger, J. M., Price, R. J., Walters, D. G., Kao, J., et al. (2002). Inhibition of zaleplon metabolism by cimetidine in the human liver: in vitro studies with subcellular fractions and precision-cut liver slices. Xenobiotica 32, 849–862. doi:10.1080/00498250210158221
Robbiani, D. F., Gaebler, C., Muecksch, F., Lorenzi, J. C. C., Wang, Z., Cho, A., et al. (2020). Convergent antibody responses to SARS-CoV-2 in convalescent individuals. Nature 584, 437–442. doi:10.1038/s41586-020-2456-9
Rodríguez-Molinero, A., Pérez-López, C., Gálvez-Barrón, C., Miñarro, A., Macho, O., López, G. F., et al. (2020). Observational study of azithromycin in hospitalized patients with COVID-19. PLoS One 15, e0238681. doi:10.1371/journal.pone.0238681
Rosenberg, E. S., Dufort, E. M., Udo, T., Wilberschied, L. A., Kumar, J., Tesoriero, J., et al. (2020). Association of treatment with hydroxychloroquine or azithromycin with in-hospital mortality in patients with COVID-19 in New York state. JAMA 323 (24), 2493–2502. doi:10.1001/jama.2020.8630
Satarker, S., Ahuja, T., Banerjee, M., E, V. B., Dogra, S., Agarwal, T., et al. (2020). Hydroxychloroquine in COVID-19: potential mechanism of action against SARS-CoV-2. Curr. Pharmacol. Rep. [Epub ahead of print]. doi:10.1007/s40495-020-00231-8
Schögler, A., Kopf, B. S., Edwards, M. R., Johnston, S. L., Casaulta, C., Kieninger, E., et al. (2015). Novel antiviral properties of azithromycin in cystic fibrosis airway epithelial cells. Eur. Respir. J. 45, 428–439. doi:10.1183/09031936.00102014
Schade, D., Kotthaus, J., Riebling, L., Kotthaus, J., Müller-Fielitz, H., Raasch, W., et al. (2014). Development of novel potent orally bioavailable oseltamivir derivatives active against resistant influenza A. J. Med. Chem. 57, 759–769. doi:10.1021/jm401492x
Scherrmann, J. M. (2020). Intracellular ABCB1 as a possible mechanism to explain the synergistic effect of hydroxychloroquine-azithromycin combination in COVID-19 therapy. AAPS J. 22, 86. doi:10.1208/s12248-020-00465-w
Sciaccaluga, C., Cameli, M., Menci, D., Mandoli, G. E., Sisti, N., Cameli, P., et al. (2020). COVID-19 and the burning issue of drug interaction: never forget the ECG. Postgrad. Med. J. [Epub ahead of print]. doi:10.1136/postgradmedj-2020-138093
Sheahan, T. P., Sims, A. C., Leist, S. R., Schäfer, A., Won, J., Brown, A. J., et al. (2020). Comparative therapeutic efficacy of remdesivir and combination lopinavir, ritonavir, and interferon beta against MERS-CoV. Nat. Commun. 11, 222. doi:10.1038/s41467-019-13940-6
Shereen, M. A., Khan, S., Kazmi, A., Bashir, N., and Siddique, R. (2020). COVID-19 infection: origin, transmission, and characteristics of human coronaviruses. J. Adv. Res. [Epub ahead of print]. doi:10.1016/j.jare.2020.03.005
Shi, L., Xiong, H., He, J., Deng, H., Li, Q., Zhong, Q., et al. (2007). Antiviral activity of arbidol against influenza A virus, respiratory syncytial virus, rhinovirus, coxsackie virus and adenovirus in vitro and in vivo. Arch. Virol. 152, 1447–1455. doi:10.1007/s00705-007-0974-5
Shrestha, D. B., Budhathoki, P., Khadka, S., Shah, P. B., Pokharel, N., and Rashmi, P. (2020). Favipiravir versus other antiviral or standard of care for COVID-19 treatment: a rapid systematic review and meta-analysis. Virol. J. 17, 141. doi:10.1186/s12985-020-01412-z
Simmons, G., Gosalia, D. N., Rennekamp, A. J., Reeves, J. D., Diamond, S. L., and Bates, P. (2005). Inhibitors of cathepsin L prevent severe acute respiratory syndrome coronavirus entry. PNAS 102, 11876–11881. doi:10.1073/pnas.0505577102
Simonovich, V. A., Burgos Pratx, L. D., Scibona, P., Beruto, M. V., Vallone, M. G., Vázquez, C., et al. (2020). A randomized trial of convalescent plasma in covid-19 severe pneumonia. N. Engl. J. Med. [Epub ahead of print]. doi:10.1056/NEJMoa2031304
Singh, A. K., Singh, A., Singh, R., and Misra, A. (2020). Remdesivir in COVID-19: a critical review of pharmacology, pre-clinical and clinical studies. Diabetes Metab. Syndr. 14, 641–648. doi:10.1016/j.dsx.2020.05.018
Sisay, M. (2020). 3CLpro inhibitors as a potential therapeutic option for COVID-19: available evidence and ongoing clinical trials. Pharmacol. Res. 156, 104779. doi:10.1016/j.phrs.2020.104779
Sisti, G., Schiattarella, A., and Sisti, A. (2020). Treatment of COVID-19 pregnancy with hydroxychloroquine and azithromycin: a case report. Acta Biomedica 91, e2020123. doi:10.23750/abm.v91i4.10216
Smee, D. F., Bray, M., and Huggins, J. W. (2001). Antiviral activity and mode of action studies of ribavirin and mycophenolic acid against orthopoxviruses in vitro. Antivir. Chem. Chemother. 12 (6), 327–335. doi:10.1177/095632020101200602
Song, H. C., Seo, M. Y., Stadler, K., Yoo, B. J., Choo, Q. L., and Coates, S. R. (2004). Synthesis and characterization of a native, oligomeric form of recombinant severe acute respiratory syndrome coronavirus spike glycoprotein. J. Virol. 78, 10328–10335. doi:10.1128/JVI.78.19.10328-10335.2004
Sonkar, C., Kashyap, D., Varshney, N., Baral, B., and Jha, H. C. (2020). Impact of gastrointestinal symptoms in COVID-19: a molecular approach. SN Compr. Clin. Med. [Epub ahead of print]. doi:10.1007/s42399-020-00619-z
Spinner, C. D., Gottlieb, R. L., Criner, G. J., Arribas López, J. R., Cattelan, A. M., Soriano Viladomiu, A., et al. (2020). Effect of remdesivir vs standard care on clinical status at 11 Days in patients with moderate COVID-19: a randomized clinical trial. JAMA 324, 1048–1057. doi:10.1001/jama.2020.16349
Takahashi, H. (2000). Developmental changes in pharmacokinetics and pharmacodynamics of warfarin enantiomers in Japanese children. Clin. Pharmacol. Ther. 68, 541–555. doi:10.1067/mcp.2000.110977
Takahashi, H., Iwasaki, Y., Watanabe, T., Ichinose, N., Okada, Y., Oiwa, A., et al. (2020). Case studies of SARS-CoV-2 treated with favipiravir among patients in critical or severe condition. Int. J. Infect. Dis. 100, 283–285. doi:10.1016/j.ijid.2020.08.047
Tan, Y. W., Yam, W. K., Sun, J., and Chu, J. J. H. (2018). An evaluation of chloroquine as a broad-acting antiviral against hand, foot and mouth disease. Antivir. Res. 149, 143–149. doi:10.1016/j.antiviral.2017.11.017
Tang, W., Cao, Z., Han, M., Wang, Z., Chen, J., Sun, W., et al. (2020). Hydroxychloroquine in patients with mainly mild to moderate coronavirus disease 2019: open label, randomised controlled trial. BMJ 2020, 369. doi:10.1136/bmj.m1849
Tong, S., Su, Y., Yu, Y., Wu, C., Chen, J., Wang, S., et al. (2020). Ribavirin therapy for severe COVID-19: a retrospective cohort study. Int. J. Antimicrob. Agents 56, 106114. doi:10.1016/j.ijantimicag.2020.106114
van Boheemen, S., de Graaf, M., Lauber, C., Bestebroer, T. M., Raj, V. S., Zaki, A. M., et al. (2012). Genomic characterization of a newly discovered coronavirus associated with acute respiratory distress syndrome in humans. MBio 3, e00473-12. doi:10.1128/mBio.00473-12
Vecchio, G., Zapico, V., Catanzariti, A., Carboni Bisso, I., and Las Heras, M. (2020). Adverse effects of lopinavir/ritonavir in critically ill patients with COVID-19. Medicina 80, 439–441.
Verdugo-Paiva, F., Izcovich, A., Ragusa, M., and Rada, G. (2020). Lopinavir-ritonavir for COVID-19: a living systematic review. Medwave 20, e7967. doi:10.5867/medwave.2020.06.7966
Wada, T., Shimode, K., Hoshiyama, T., Takayama, Y., and Yamaoka, K. (2020). Three novel COVID-19 pneumonia cases successfully treated with lopinavir/ritonavir. Front. Med. 7 [Epub ahead of print]. doi:10.3389/fmed.2020.00241
Wang, D., Hu, B., Hu, C., Zhu, F., Liu, X., Zhang, J., et al. (2020). Clinical characteristics of 138 hospitalized patients with 2019 novel coronavirus-infected pneumonia in wuhan, China. JAMA [Epub ahead of print]. doi:10.1001/jama.2020.1585
Wang, M., Cao, R., Zhang, L., Yang, X., Liu, J., Xu, M., et al. (2020). Remdesivir and chloroquine effectively inhibit the recently emerged novel coronavirus (2019-nCoV) in vitro. Cell Res. 30, 269–271. doi:10.1038/s41422-020-0282-0
Wang, T., Du, Z., Zhu, F., Cao, Z., An, Y., Gao, Y., et al. (2020). Comorbidities and multi-organ injuries in the treatment of COVID-19. Lancet 395, e52. doi:10.1016/S0140-6736(20)30558-4
Wang, X., Cao, R., Zhang, H., Liu, J., Xu, M., Hu, H., et al. (2020). The anti-influenza virus drug, arbidol is an efficient inhibitor of SARS-CoV-2 in vitro. Cel Discov. 6, 28. doi:10.1038/s41421-020-0169-8
Wang, Y., Zhang, D., Du, G., Du, R., Zhao, J., Jin, Y., et al. (2020). Remdesivir in adults with severe COVID-19: a randomised, double-blind, placebo-controlled, multicentre trial (2020). Lancet 395, 1569–1578. doi:10.1016/S0140-6736(20)31022-9
Ward, P., Small, I., Smith, J., Suter, P., and Dutkowski, R. (2005). Oseltamivir (Tamiflu) and its potential for use in the event of an influenza pandemic. J. Antimicrob. Chemother. 55, i5–i21. doi:10.1093/jac/dki018
Wen, C. Y., Xie, Z. W., Li, Y. P., Deng, X. L., Chen, X. T., Cao, Y., et al. (2020). Real-world efficacy and safety of lopinavir/ritonavir and arbidol in treating with COVID-19 : an observational cohort study. Zhonghua Nei Ke Za Zhi 59, E012. doi:10.3760/cma.j.cn112138-20200227-00147
WHO (2020a). Coronavirus Disease (COVID-19) situation reports. Available at: https://www.who.int/emergencies/diseases/novel-coronavirus-2019/situation-reports (Accessed July 3, 2020).
WHO (2020b). Solidarity clinical trial for COVID-19 treatments. Available at: https://www.who.int/emergencies/diseases/novel-coronavirus-2019/global-research-on-novel-coronavirus-2019-ncov/solidarity-clinical-trial-for-covid-19-treatments (Accessed November 4, 2020).
WHO (2020c). SARS-CoV-2 variants. Available at: http://www.who.int/csr/don/31-december-2020-sars-cov2-variants/en/ (Accessed January 13, 2021).
Xia, X., Li, K., Wu, L., Wang, Z., Zhu, M., Huang, B., et al. (2020). Improved clinical symptoms and mortality among patients with severe or critical COVID-19 after convalescent plasma transfusion. Blood 136, 755–759. doi:10.1182/blood.2020007079
Yamamura, H., Matsuura, H., Nakagawa, J., Fukuoka, H., Domi, H., and Chujoh, S. (2020). Effect of favipiravir and an anti-inflammatory strategy for COVID-19. Crit. Care 24, 413. doi:10.1186/s13054-020-03137-5
Yan, D., Liu, X.-Y., Zhu, Y.-N., Huang, L., Dan, B.-T., Zhang, G.-J., et al. (2020). Factors associated with prolonged viral shedding and impact of Lopinavir/Ritonavir treatment in hospitalised non-critically ill patients with SARS-CoV-2 infection. Eur. Respir. J. [Epub ahead of print]. doi:10.1183/13993003.00799-2020
Yan, V. C., and Muller, F. L. (2020). Advantages of the parent nucleoside GS-441524 over remdesivir for covid-19 treatment. ACS Med. Chem. Lett. 11, 1361–1366. doi:10.1021/acsmedchemlett.0c00316
Yao, T., Qian, J., Zhu, W., Wang, Y., and Wang, G. (2020). A systematic review of lopinavir therapy for SARS coronavirus and MERS coronavirus—a possible reference for coronavirus disease‐19 treatment option. J. Med. Virol. 92, 556–563. doi:10.1002/jmv.25729
Yao, X., Ye, F., Zhang, M., Cui, C., Huang, B., Niu, P., et al. (2020). In Vitro antiviral activity and projection of optimized dosing design of hydroxychloroquine for the treatment of severe acute respiratory syndrome coronavirus 2 (SARS-CoV-2). Clin. Infect. Dis. [Epub ahead of print]. doi:10.1093/cid/ciaa237
Ye, X.-T., Luo, Y.-L., Xia, S.-C., Sun, Q.-F., Ding, J.-G., Zhou, Y., et al. (2020). Clinical efficacy of lopinavir/ritonavir in the treatment of Coronavirus disease 2019. Eur. Rev. Med. Pharmacol. Sci. 24, 3390–3396. doi:10.26355/eurrev_202003_20706
Yuan, J., Zou, R., Zeng, L., Kou, S., Lan, J., Li, X., et al. (2020). The correlation between viral clearance and biochemical outcomes of 94 COVID-19 infected discharged patients. Inflamm. Res. 69, 599–606. doi:10.1007/s00011-020-01342-0
Zaim, S., Chong, J. H., Sankaranarayanan, V., and Harky, A. (2020). COVID-19 and multiorgan response. Curr. Probl. Cardiol. 45, 100618. doi:10.1016/j.cpcardiol.2020.100618
Zhang, J.-N., Wang, W.-J., Peng, B., Peng, W., Zhang, Y.-S., Wang, Y.-L., et al. (2020). Potential of arbidol for post-exposure prophylaxis of COVID-19 transmission—a preliminary report of a retrospective cohort study. Curr. Med. Sci. [Epub ahead of print]. doi:10.1007/s11596-020-2203-3
Zhou, P., Yang, X.-L., Wang, X.-G., Hu, B., Zhang, L., Zhang, W., et al. (2020). A pneumonia outbreak associated with a new coronavirus of probable bat origin. Nature 579, 270–273. doi:10.1038/s41586-020-2012-7
Zhu, Z., Lu, Z., Xu, T., Chen, C., Yang, G., Zha, T., et al. (2020). Arbidol monotherapy is superior to lopinavir/ritonavir in treating COVID-19. J. Infect. 81, e21–e23. doi:10.1016/j.jinf.2020.03.060
Zijp, T. R., Toren-Wielema, M. L., Nannan Panday, P. V., Kosterink, J. G. W., Berger, S. P., and Touw, D. J. (2020). Important interactions of immunosuppressants with experimental therapies for novel coronavirus disease (COVID-19): how to act. Ther. Drug Monit. 42, 652–653. doi:10.1097/FTD.0000000000000766
Keywords: COVID-19, SARS-CoV-2, drug repurposing, antivirals, mechanism of action, pharmacokinetics
Citation: Indari O, Jakhmola S, Manivannan E and Jha HC (2021) An Update on Antiviral Therapy Against SARS-CoV-2: How Far Have We Come?. Front. Pharmacol. 12:632677. doi: 10.3389/fphar.2021.632677
Received: 23 November 2020; Accepted: 15 January 2021;
Published: 08 March 2021.
Edited by:
Sugunadevi Sakkiah, National Center for Toxicological Research (FDA), United StatesReviewed by:
Arnab K. Chatterjee, Calibr at Scripps Research, United StatesCopyright © 2021 Indari, Jakhmola, Manivannan and Jha. This is an open-access article distributed under the terms of the Creative Commons Attribution License (CC BY). The use, distribution or reproduction in other forums is permitted, provided the original author(s) and the copyright owner(s) are credited and that the original publication in this journal is cited, in accordance with accepted academic practice. No use, distribution or reproduction is permitted which does not comply with these terms.
*Correspondence: Hem Chandra Jha, aGVtY2poYUBpaXRpLmFjLmlu
Disclaimer: All claims expressed in this article are solely those of the authors and do not necessarily represent those of their affiliated organizations, or those of the publisher, the editors and the reviewers. Any product that may be evaluated in this article or claim that may be made by its manufacturer is not guaranteed or endorsed by the publisher.
Research integrity at Frontiers
Learn more about the work of our research integrity team to safeguard the quality of each article we publish.