- 1State Key Laboratory of Southwestern Chinese Medicine Resources, Chengdu University of Traditional Chinese Medicine, Chengdu, China
- 2School of Pharmacy and School of Public Health, Chengdu University of Traditional Chinese Medicine, Chengdu, China
- 3Department of Pharmacy, Affiliated Hospital of Southwest Medical University, Luzhou, China
- 4West China School of Pharmacy, State Key Laboratory of Biotherapy, West China Hospital, Sichuan University, Chengdu, China
Chinese materia medica (CMM) has been applied for the prevention and treatment of diseases for thousands of years. However, arrhythmia, myocardial ischemia, heart failure, and other cardiac adverse reactions during CMM application were gradually reported. CMM-induced cardiotoxicity has aroused widespread attention. Our review aimed to summarize the risk compounds, preclinical toxicity evaluation, and potential mechanisms of CMM-induced cardiotoxicity. All relevant articles published on the PubMed, Embase, and China National Knowledge Infrastructure (CNKI) databases for the latest twenty years were searched and manually extracted. The risk substances of CMM-induced cardiotoxicity are relatively complex. A single CMM usually contains various risk compounds, and the same risk substance may exist in various CMM. The active and risk substances in CMM may be transformed into each other under different conditions, such as drug dosage, medication methods, and body status. Generally, the risk compounds of CMM-induced cardiotoxicity can be classified into alkaloids, terpenoids, steroids, heavy metals, organic acids, toxic proteins, and peptides. Traditional evaluation methods of chemical drug-induced cardiotoxicity primarily include cardiac function monitoring, endomyocardial biopsy, myocardial zymogram, and biomarker determination. In the preclinical stage, CMM-induced cardiotoxicity should be systematically evaluated at the overall, tissue, cellular, and molecular levels, including cardiac function, histopathology, cytology, myocardial zymogram, and biomarkers. Thanks to the development of systematic biology, the higher specificity and sensitivity of biomarkers, such as genes, proteins, and metabolic small molecules, are gradually applied for evaluating CMM-induced cardiotoxicity. Previous studies on the mechanisms of CMM-induced cardiotoxicity focused on a single drug, monomer or components of CMM. The interaction among ion homeostasis (sodium, potassium, and calcium ions), oxidative damage, mitochondrial injury, apoptosis and autophagy, and metabolic disturbance is involved in CMM-induced cardiotoxicity. Clarification on the risk compounds, preclinical toxicity evaluation, and potential mechanisms of CMM-induced cardiotoxicity must be beneficial to guide new CMM development and post-marketed CMM reevaluation.
Introduction
Chinese materia medica (CMM) as an alternative therapy for disease treatment has become increasingly popular all over the world. A large number of clinical evidence has valued CMM, which is based on shifting compensatory homeostasis to the overall human body homeostasis, complementary to chemical medicine in the management of chronic disease (Fan et al., 2011). However, CMM formulations are often not subjected to premarket toxicity testing (Wang and Ren, 2002). Recently, more and more attention has been paid to the safety of CMM, including cardiotoxicity, hepatotoxicity, and nephrotoxicity of CMM. We previously reviewed some important information on CMM-induced liver injury (Pan et al., 2020). Drug-induced cardiotoxicity refers to the toxicity or negative effects of chemical drugs or CMM on the heart, including arrhythmia, myocardial ischemia, heart failure, and other cardiac adverse reactions (Li et al., 2015). A total of 81 drugs in the United States, Europe, and Asia from 1990 to 2013 were withdrawn from the market due to safety issues, including 16 drugs that caused arrhythmias (Li et al., 2016b). Chinese Adverse Drug Reaction Monitoring Report in 2018 showed that CMM accounted for 14.6% in adverse drug reactions/event reports and 8.7% in serious adverse reactions/event reports, and cardiovascular system damage caused by drugs accounted for 4.1% of all (National Medical Products Administration, 2019). General performances of drug-induced cardiotoxicity include electrocardiogram (ECG) abnormalities, myocardial infarction, impaired systolic and diastolic performance, functional remodeling and histopathological findings, and signs of apoptosis and degeneration. Acute cardiovascular injuries manifested as arterial hypertension, aortic dissection, arrhythmias, and myocardial ischemia. However, CMM-induced cardiotoxicity is easy to be ignored due to the lack of specific and detectable evaluation indicators.
At present, evaluation system on chemical drug-induced cardiotoxicity has been gradually established and improved, such as “The Clinical Evaluation of QT/QTc Interval Prolongation and Proarrhythmic Potential for Non-Antiarrhythmic Drugs” published by the International Conference on Harmonisation (ICH) in 2005, “Cardiovascular toxicity induced by chemotherapy, targeted agents and radiotherapy: ESMO Clinical Practice Guidelines” issued by the United States in 2012, and “Guidelines for Prevention and Treatment of Anthracycline-induced Cardiotoxicity” published by China in 2015 (Food and Drug Administration, 2005). However, the characteristics of multicomponent, multi-target, and multi-effect are involved in CMM-induced cardiotoxicity. There are not enough indicators of high specificity and accuracy to evaluate CMM-induced cardiotoxicity. Evaluation methods of cardiotoxicity caused by chemical drugs, such as anthracyclines, and chemotherapeutic and targeted drugs, are not fully applicable to those of CMM-induced cardiotoxicity. Therefore, evaluation strategies that meet the characteristics of CMM should be urgently established in the development and reevaluation of CMM. Previous studies mainly focused on chemical composition analysis, toxicity characteristics, mechanisms, and toxicokinetics on cardiotoxicity caused by single CMM or monomer compound of CMM. Our review aims to conclude these risk compounds, preclinical toxicity evaluation, and possible mechanisms of CMM-induced cardiotoxicity to guide clinical practice and promote the safety of drug application.
Literature Retrieval Methods
A target search of available literature was performed on the PubMed, Embase, and China National Knowledge Infrastructure (CNKI) from January 1, 2000 to February 29, 2020 with the following key search terms: (“Chinese materia medica,” OR “traditional Chinese medicine,” OR “Chinese herbal medicine,” OR “Chinese medicine,” OR “medicinal herb,” OR “Chinese medicinal herb,” OR “Chinese herbs,” OR “herbal medicines,” OR “herbs”) AND (“cardiotoxicity,” OR “cardiac toxicity,” OR “cardiac poisonous,” OR “cardiac adverse effects,” OR “cardiac side effects,” OR “cardiac adverse events,” OR “cardiovascular events,” OR “cardiac damage,” OR “cardiac risk”). Individual scientific principles were applied to ensure the quality and relevance of searched articles. Two authors independently screened titles and abstracts of articles, excluding articles not relevant to the topic. Those studies on Chinese herbal compound prescriptions were also excluded, and only the relevant studies on CMM, their extracts, and ingredients were retained. The required articles must include a description of CMM-induced cardiotoxicity with scientific data, including full-length experimental articles, reviews, English-only abstracts, case reports, and conference papers.
Risk Compounds of CMM-Induced Cardiotoxicity
The risk substances are the material basis for CMM-induced cardiotoxicity. Interestingly, the toxic material basis is closely related to the active material basis. Thus, misuse or overdose of CMM could trigger toxic effects instead of pharmacological action. According to different phytochemical structures, the risk compounds of CMM-induced cardiotoxicity are mainly classified into alkaloids, terpenoids, steroids, heavy metals, organic acids, and toxic proteins or peptides. Generally, more types of risk substances may be founded in a single CMM, while the same type of risk substance may exist in various CMM. Therefore, more attention should be paid to cardiotoxicity caused by CMM that contains these risk substances. The risk substances, toxicity evaluation indicators, and potential mechanisms of some common CMM-induced cardiac toxicity were concluded in Table 1.
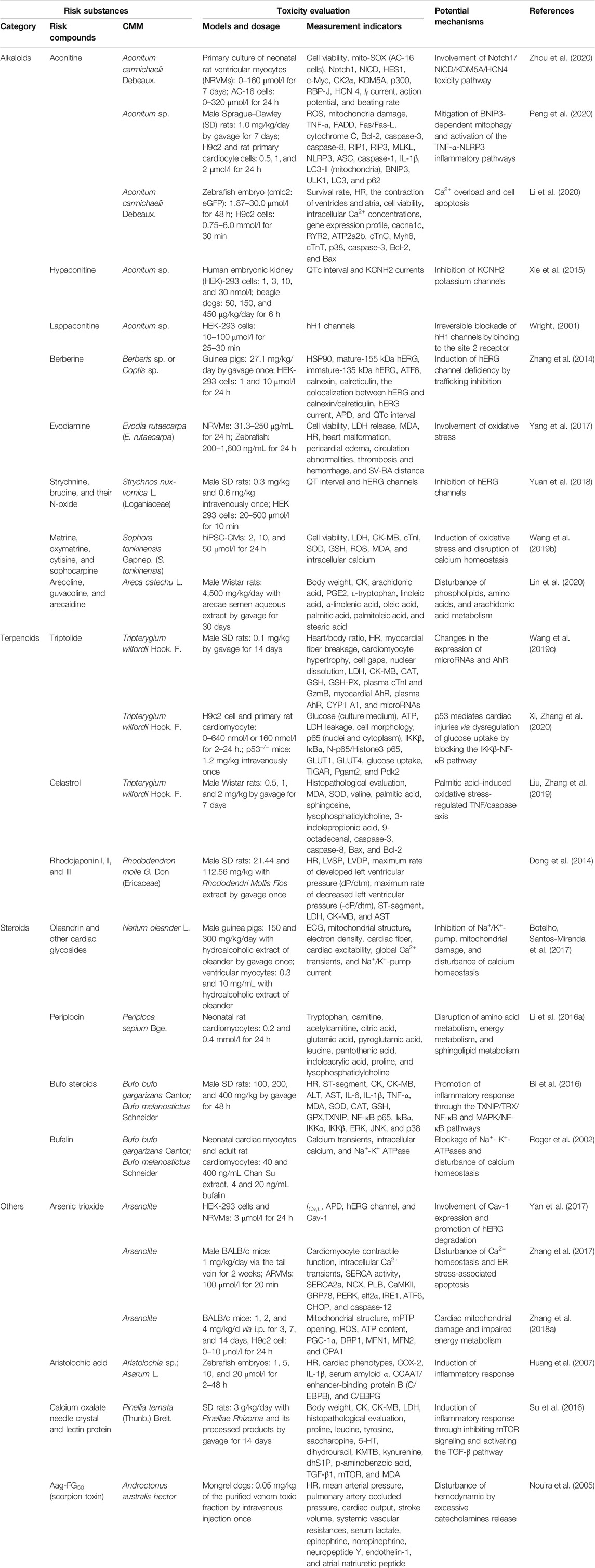
TABLE 1. Risk substances, toxicity evaluation, and potential mechanisms of some representative CMM-induced cardiotoxicity in vivo and in vitro.
Alkaloids
Diterpene Alkaloids
Alkaloids are the most common risk compounds in CMM-induced cardiotoxicity. Diterpenoid alkaloids are the largest category of alkaloids that lead to cardiac toxicity, which can be found in aconitum plants, such as Radix Aconiti lateralis Preparata, Radix Aconiti, Radix Aconiti Kusnezoffii, and Radix Aconiti Brachypodi, as well as their raw and processed products. According to different phytochemical structure, aconite alkaloids mainly include C-18, C-19, and C-20 types of diterpenoid alkaloids. C-19 diterpenoid alkaloids are the most common and most toxic ingredients, including aconitine, hypoaconitine, mesoaconitine, benzoyl aconitine, benzoyl hypoaconitine, and benzoyl mesoaconitine (Nyirimigabo et al., 2015). Aconitine and its analogs have been demonstrated to induce lethal malignant ventricular arrhythmias or acute myocardial infarction (Sun et al., 2014; Xie et al., 2015). Aconitine has even been developed into experimental tools to establish cardiac arrhythmic models in cardiovascular drug-related researches (Ma et al., 2018). In addition, other diterpenoid alkaloids, such as lappaconitine, ranaconitine, delvestidine, denudatine, secokaraconitine, and brachyaconitine, have also been shown to have obvious cardiotoxicity (Wang et al., 2014a; Nie et al., 2017).
Isoquinoline Alkaloids
Berberine, a class of isoquinoline alkaloids, is considered to be the major risk substance in various CMM, such as Coptis chinensis and Cortex Phellodendri Chinensis. It has been reported that berberine acutely inhibits human ether-a-go-go-related gene (hERG) currents and prolongs action potential duration in Xenopus oocytes (Li et al., 2001). Studies in vivo showed that high concentrations of alkaloids, including berberine, coptisine, palmatine, and jatrorrhizine, were found in heart tissues of mice. Results of in vitro experiments also suggested that berberine, coptisine, palmatine, and jatrorrhizine presented cytotoxicity in dose- and time-dependent manners in H9c2 cells (Ma et al., 2010). Some studies demonstrated that berberine, berbamine, palmatine, and oxyberberine could result in cardiac arrest and arrhythmia in a time- and dose-dependent manner (Zhang et al., 2018b). In addition to berberine and coptis alkaloids, Papaveraceae plant Chelidonium majus also contains other isoquinine alkaloids, such as sanguinarine, chelidonine, and chelerythrine. Direct effects of sanguinarine on the heart may be responsible for cardiac arrest and death (Hu et al., 2005). The concentration- and time-dependent apoptoses to necrosis were observed during chelerythrine administration in rat neonatal ventricular cardiomyocytes or human pluripotent stem cell–derived cardiomyocytes (Mioulane et al., 2012).
Indoles Alkaloids
Indoles alkaloids, such as strychnine and brucine isolated from Strychnos nux-vomica L., have been shown to be cardiotoxic. An automatic patch-clamp system was established to screen and evaluate strychnine for cardiotoxicity. Results showed that strychnine inhibited hERG, increased QT interval, and decreased heart rate (HR) in isolated rat hearts (Wang et al., 2018). Both strychnine and brucine were shown to exhibit the voltage-dependent inhibition of hERG channels (Yuan et al., 2018). Moreover, other indole alkaloids, such as evodiamine, vinblastine, and vincristine, are specified as the risk substances of Evodiae fructus and Catharanthus roseus, respectively. Results indicated that evodiamine led to cardiovascular side effects in primary cultured neonatal rat cardiomyocytes and zebrafish (Yang et al., 2017). Tubulin-binding drugs containing vinblastine and vincristine presented specific toxicity through pathologic, immunological, and transcriptomic analyses in cardiac endothelial cells (Mikaelian et al., 2010).
Organic Amine Alkaloids
Organic amine alkaloids, such as ephedrine and colchicines, have been gradually proved to be cardiotoxic. The study indicated that ephedrine isolated from Ephedra sinica Stapf could trigger a range of cardiovascular toxicities, including myocarditis, arrhythmias, myocardial infarction, cardiac arrest, heart failure, and sudden death (Samenuk et al., 2002; Adamson et al., 2004; Naik and Freudenberger, 2004; Nyska et al., 2005). Colchicine derived from Colchicum autumnale has been proved to impair impulse formation and conduction (Tochinai et al., 2014). Another study suggested that colchicine could directly damage cardiac endothelial cells to induce cardiotoxicity (Mikaelian et al., 2010).
Terpenoids
Terpenoids, such as triptolide, celastrol, and rhodojaponin, are other important compounds of CMM-induced cardiotoxicity. Triptolide, a highly promising diterpenoid triepoxide extracted from Tripterygium wilfordii, has been widely reported to elicit chest distress, cardiopalmus, bradyarrhythmia, and even cardiogenic shock (Huang et al., 2009). Some studies demonstrated that triptolide induced cardiomyocyte damage and cell apoptosis (Li et al., 2014; Zhou et al., 2015). Celastrol, a cell-permeable dienonephenolic triterpene compound, was also derived from Tripterygium wilfordii. Celastrol was revealed to have potent inhibitory activity on potassium channels, thereby inducing QT interval prolongation (Sun et al., 2006). Various toxic symptoms of zebrafish embryos were caused by celastrol, including appearance of heart linearization, heart membrane hemorrhage, and hemocyte accumulation in the cardiac region (Wang et al., 2009). Besides, grayanane diterpenoids, the main active component extracted from the fruits of Rhododendron molle, were related to cardiotoxicity (Zhou et al., 2014). A study on the toxicological effects of rhodojaponins demonstrated that myocardial injury was elicited by Rhododendron molle extract (Dong et al., 2014).
Steroids
Steroids, such as cardiac glycosides, cardenolides, and steroid saponins, are also revealed to induce cardiotoxicity. Cardiac glycosides are the largest category of steroids, such as digitalis derivatives, oleandrine, and periplocin. Digitalis derivatives have been known as a representative compound of cardiac glycosides. Several studies showed that digitalis derivatives presented arrhythmogenic effects on the heart (Demiryurek et al., 2002; Demiryürek and Demiryürek, 2005; Ramirez-Ortega et al., 2007). Arrhythmias and electrical conduction disturbances promoted by digitalis derivatives are related to cardiomyocyte dysfunction (Botelho et al., 2017). Oleandrin, a cardiac glycoside derived from the leaves of Nerium indicum, has been shown to induce myocardial damage in vivo and in vitro (Poindexter et al., 2007; Zhou et al., 2019). Periplocin, the main substance in Periploca sepium Bunge, easily triggered cardiotoxicity because of improper application (Demiryürek and Demiryürek, 2005). Moreover, cardenolide is another representative steroid compound of CMM. Bufadienolides, including resibufogenin, arenobufagin, bufalin, and cinobufagin, are natural cardenolides, which are also the major pharmacological constituents of cinobufagin venom toad. Induced resibufogenin delayed after depolarization and triggered arrhythmias in cardiac fiber at high concentrations in vitro and in vivo (Xie et al., 2002). Arenobufagin was also reported to induce obvious myocardial damage (Deng et al., 2017; Hou et al., 2018). Steroid saponins in some CMM have also been shown to be cardiotoxic. For example, ophiopogonin D, a steroidal saponin derived from Ophiopogon japonicus, presented toxic effects on cardiomyocytes (Ren et al., 2017; Wang et al., 2019a). Ginsenosides Rb1 and Re were found to inhibit cardiac contraction in ventricular myocytes (Scott et al., 2001).
Other Risk Compounds
Heavy metals, organic acids, toxic proteins, and peptides may also induce some cardiac adverse reactions. As2O3, the main ingredient of the highly toxic substance arsenic, has been proved to have a variety of adverse cardiac reactions, such as cardiac arrhythmia, ventricular tachycardia, or even sudden cardiac death (Barbey et al., 2003; Balakumar and Kaur, 2009; Zhang et al., 2013b). As2O3 was also shown to lead to human cardiac developmental toxicity (Bao et al., 2019). Aristolochic acid derived from Aristolochia debilis, Aristolochia manshuriensis, or Asarum sieboldii has been found to specifically trigger heart defects in zebrafish embryos (Huang et al., 2007; Shi et al., 2017). Toxic proteins and peptides mainly derived from some plants or animals have been widely reported to exert cardiac toxicity effects. Scorpion toxins, such as BmSKTx1, BmBKTx1, BmTX1, BmTX2, and BmP09 identified from buthotoxin, are the main substances that are responsible for cardiotoxicity (Xu et al., 2004a; Xu et al., 2004b; Yao et al., 2005; Quintero-Hernandez et al., 2013). Centipede toxin, a type of polypeptide toxin purified from Scolopendra subspinipes, including SSD609 and SsTx, can induce cardiovascular system disorders (Sun et al., 2015; Luo et al., 2018).
Preclinical Evaluation of CMM-Induced Cardiotoxicity
The early identification of CMM-induced cardiotoxicity is quite difficult due to the lack of specific evaluation methods. Evaluation of chemical drug-induced cardiotoxicity can provide a reference for CMM-induced cardiotoxicity. CMM-induced cardiotoxicity presents a variety of manifestations, including abnormal ECG, myocardial infarction, impaired systolic and diastolic performance, functional remodeling and histopathological changes, and signs of myocardial apoptosis and degeneration. Thus, CMM-induced cardiotoxicity should be evaluated at the overall, tissue, cellular, and molecular levels, including cardiac function, morphological alteration in cardiac muscle tissue, cell or organelle structures and function, myocardial zymogram, and biomarkers.
Cardiac Function
At present, various clinical examination methods can be used to evaluate the effects of CMM on cardiac function. Generally, the evaluation of cardiac function involves HR, blood pressure, hemodynamic parameters, ECG, echocardiography, and magnetic resonance imaging (MRI) examination. ECG, the most common technique for monitoring drug-induced cardiotoxicity, can record bioelectrical changes during the occurrence, spread, and recovery of cardiac excitability. Various arrhythmias, ventricular atrial hypertrophy, myocardial infarction, and myocardial ischemia can be identified by ECG monitoring. Electrophysiological alterations of drug-induced cardiotoxicity mainly involve the prolongation of QT/QTc interval, action potential duration (APD), and ST-segment alteration. QT and QTc interval can be prolonged 12–24 h after myocardial infarction. QT interval prolongation is also significantly associated with arrhythmia, especially ventricular arrhythmia and torsades de pointes (TdP) (Halkin et al., 2001). Similarly, the prolongation of APD-induced arrhythmia, following ventricular hypertrophy, can also lead to impaired myocardial relaxation and contractile function (Odening et al., 2013). The risk substances of cardiotoxicity caused by CMM, such as arsenic trioxide, berberine, hypaconitine, aconitine, and strychnine, usually induce the prolongation of APD and QT/QTc interval, thus causing various arrhythmias, abnormal cardiac systolic, and other adverse reactions (Wang et al., 2008; Chen et al., 2010; Zhang et al., 2014; Xie et al., 2015; Wang et al., 2018). Aconitine not only triggered sinus rhythm with low-amplitude P waves and junctional rhythm conducting with narrow QRS complex but also presented diffuse ST-segment depression and the prolongation of QT interval (Karturi et al., 2016). Aconitine and Venenum Bufonis disrupted heart rhythm, influenced diastolic function, and significantly promoted ST-segment elevation (Sun et al., 2014; Bi et al., 2016). Rhododendron molle extract–treated rats also showed an ST-segment elevation, following acute ischemic tissue injury (Dong et al., 2014). Intravenous injection with toad extract provoked supraventricular arrhythmia (Kostakis and Byard, 2009). Therefore, ECG is one of the effective indicators for identifying the selective toxicity of CMM to the heart. ECG abnormality induced by CMM is able to reflect myocardial diseases, such as arrhythmia, conduction block, and myocardial ischemia. However, ECG is difficult to detect the early cardiotoxicity induced by CMM if left ventricular ejection fraction (LVEF) is normal. Thus, ECG should be combined with other indicators to systematically evaluate the cardiotoxicity of CMM.
Histopathology
Histopathological examination, the highest standard for determining chemical drug-induced cardiotoxicity, can also be applied for evaluating CMM-induced cardiotoxicity. Inflammatory cell infiltration, myocardial rupture, and hemorrhage were clearly observed after a high dose Aconitum carmichaelii Debx. treatment in the cardiac muscle tissue of mice (Cai et al., 2013). Myocardial fiber breakage, cell swelling, and interstitial congestion were found in a larger number of mice exposed to triptolide (Yang et al., 2016). The dilated intercellular spaces were observed in severely damaged myocardium of Venenum Bufonis–treated rats, which were accompanied by abundant eosinophilic cytoplasm, obvious inflammatory cell infiltrations, and moderate piecemeal necrosis (Dong et al., 2015). Zebrafish has been widely applied to study the cardiotoxicity of new drugs with the advantages of small size and transparent body. Morphological abnormalities in zebrafish were observed and quantitatively assessed, including heart malformation, pericardial edema, circulation abnormalities, thrombosis, and hemorrhage (Yang et al., 2017).
Cell Morphology
To elucidate the potential mechanism, evaluation on CMM-induced cardiotoxicity should be deepened to the cellular and molecular levels. As an alternative to traditional animal model assessment, cytological evaluation in vitro has significant advantages in identifying early, mild, and potential toxicity of CMM (Chen et al., 2017). Toxic effects of CMM on myocardial cells could be visually determined through cell morphology observation. At present, the inverted phase contrast microscopy, fluorescence microscopy, transmission electron microscopy, and laser scanning confocal microscopy provide convenience for observing the microstructure and submicroscopic structures in cells. A study utilized a fluorescent microscope to observe the effects of As2O3 on bone marrow mesenchymal stem cells (BMSCs) through Hoechst 33,342 staining (Cai et al., 2010). Results demonstrated that As2O3 induced abnormal morphological features, such as cellular shrinkage and broken nuclei in BMSCs. In addition, hyperchromatic and dense fluorescent particles within the massive apoptotic nuclear cytoplasm were observed under fluorescence microscopy after aconitine administration (Ma et al., 2018). Beating rhythm, sarcomere shortening, and Ca2+ transients of ventricular myocytes were assessed during aconitine-induced cardiotoxicity using video-based sarcomere contractility and calcium recording module (Sun et al., 2014). One study evaluated the effects of aconitine on electrophysiological changes of ventricular myocytes by the patch-clamp recording technique. Results showed that APD for both 50 and 90% repolarization was significantly prolonged by aconitine at a dose of 1 μmol/l (Zhou et al., 2013). Another study assessed cell shortening of ventricular myocytes by a video-based edge-detection system. Results suggested that cinobufagin decreased the maximum cell shortening along with the decrease in Ca2+ transients (Liu et al., 2014).
Myocardial Zymogram
Myocardial enzymes widely distributed in cardiomyocytes will be released into the blood circulation if myocardial cells are damaged. These myocardial enzymes, including creatine kinases (CK), creatine kinase isoenzymes (CK-MB), lactic dehydrogenase (LDH), aspartate aminotransferases (AST), and α-hydroxybutyrate acid dehydrogenases (α-HBDH), are able to reflect myocardial ischemic necrosis or cell membrane permeability, which together form myocardial zymogram to evaluate CMM-induced cardiotoxicity. For example, studies showed that As2O3 could increase serum AST, CK, CK-MB, LDH, and α-HBDH levels in chicken, which were related to myocardial injury (Zhang et al., 2013a; Li et al., 2017). Rhododendri Mollis Flos–induced cardiotoxicity was evaluated by measuring the plasma levels of LDH, CK-MB, and AST (Dong et al., 2014). After SD rats were gavaged with Tripterygium wilfordii water decoction for 14 consecutive days, LDH and CK-MB levels in serum were significantly reduced, indicating that these enzymes were expected to early predict and identify CMM-induced cardiotoxicity (Wang et al., 2016a). LDH, AST, CK, and α-HBDH have poor specificity for evaluating drug-induced cardiotoxicity, while CK-MB has high sensitivity and specificity for diagnosing acute myocardial infarction. Studies revealed that the cardiotoxicity of anthracyclines could be manifested as a significant increase in CK-MB, which could be used as an indicator for the auxiliary diagnosis of heart damage (Chen et al., 2016). CK-MB detection also has a high application value in neonatal myocardial injury after asphyxia, which can provide a strong reference for clinical diagnosis (Huang, 2019). CK-MB is also more widely used in clinical monitoring of cardiotoxicity caused by drugs than brain natriuretic peptide (BNP) and N-terminal brain natriuretic peptide precursor (NT-proBNP). Clinical observation of 112 cancer patients treated with paclitaxel found that the incidences of paclitaxel-induced arrhythmia and myocardial zymogram abnormality were, respectively, 26.8 and 44.6% in the normal group, while that in the experimental group were, respectively, as high as 37.3 and 68.4%, indicating that myocardial zymogram was more sensitive to CMM-induced cardiac toxicity than arrhythmia (Lu and Chen, 2010).
Biomarkers
Proteins or Polypeptides
Some novel cardiac dysfunction markers, such as natriuretic peptides and myocardial proteins, have also been gradually applied to assess the cardiotoxicity of CMM. These natriuretic peptides, including atrial natriuretic peptide (ANP), BNP, and NT-proBNP, regulate the homeostasis of circulating volume and blood pressure, which have been widely used as markers for heart failure (Namdari et al., 2016; Maisel et al., 2018). Aristolochic acid upregulated the expression of BNP and ANP during the induction of zebrafish heart failure model (Shi et al., 2017). Troponins, including troponin T (cTnT) and troponin I (cTnI), are highly specific and sensitive to myocardial injury. Serum levels of cTnI and cTnT were significantly elevated during myocardial injury or infarction and heart failure (Panteghini, 2004; Horacek et al., 2007). cTnI and plasma granulase B in SD rats were significantly increased by water decoction of Tripterygium wilfordii. Studies on the cardiotoxicity of triptolide and Radix Aconiti extract revealed that serum cTnI level increased after administration (Lin et al., 2011; Xi et al., 2018). Acute aconitine intoxication manifested as remarkable elevation in serum CK, CK-MB, and cTnI levels (Lin et al., 2011). Myoglobin can reflect the extent of myocardial injury and the degree of myocardial necrosis. Myoglobin significantly increased in the early stage of myocardial injury before myocardial enzyme production, indicating that myoglobin is more sensitive than serum CK-MB for CMM-induced cardiotoxicity. However, myoglobin should be combined with troponins as biomarkers to evaluate the cardiac toxicity of drugs because of the low expression of myoglobin in myocardium. Although natriuretic peptides and troponins are recommended for assessing myocardial injuries with the advantages of higher specificity and sensitivity than myocardial enzymes, they are still less used for studying CMM-induced cardiotoxicity in clinical application. Cardiac fatty acid-binding protein (FABP) in the blood is better than CK and cTnI in the diagnosis of early myocardial injury (especially ≤ 3 h), which has high specificity and sensitivity for predicting CMM-induced myocardial damage (Wang et al., 2014b).
Metabolic Small Molecules
Some studies showed that citric acid, glutathione (GSH), phosphatidylcholine, and uric acid in serum could be used as biological indicators for detecting CMM-induced cardiotoxicity. Once the cardiovascular system is damaged, citric acid in serum decreases, while sugar and amino acid levels increase. The removal of intracellular reactive oxygen species (ROS) is usually accompanied by a reduction in GSH. Phosphatidylcholine is converted to arachidonic acid under the catalysis of phospholipase A2. Arachidonic acid is further converted into thromboxane A2 and thromboxane B2 by cycloprostaglandin H2 and oxygenase prostaglandin G2, thus inducing a series of cardiovascular diseases, such as blood stasis and thrombus. Therefore, phosphatidylcholine content sharply decreases under certain pathological conditions. A study found that the contents of endogenous biomarkers GSH, phosphatidylcholine, and citric acid in rat serum decreased, while ascorbic acid, uric acid, d-galactose, tryptophan, and l-phenylalanine levels increased after combined administration with ginseng and aconite (He et al., 2015). Once myocardium is short of blood or energy, ATP will be rapidly degraded to hypoxanthine and xanthine, and the final metabolite uric acid will be produced in large quantities, in which a large amount of ROS is generated, suggesting that uric acid level can reflect cardiovascular system damage. More than 20 biomarkers in serum and myocardial tissue of rats were detected after continuous gavage with Asarum sieboldii for 28 days by the nuclear magnetic resonance hydrogen spectroscopy (1HNMR), which was represented by a significant increase in lipid, lactic acid, alanine, pyruvic acid, dimethylglycine, β-glucose, and α-glucose contents, and a significant decrease in glutamic acid and tyrosine contents (You, 2016). At present, carbohydrate metabolites (α-ketoglutarate, malic acid, glucose, fructose, and succinic acid), sphingolipids (sphingosine, dihydrosphingosine, and phytosphingosine), organic acids (citric acid, pantothenic acid, and taurine), and carnitines (L-palmitoylcarnitine, L-acetyl carnitine, and isobutyryl-l-carnitine) can also be used as biomarkers for identifying CMM-induced cardiotoxicity (Qi et al., 2014). Studies showed that 11 biomarkers significantly decreased following the increased doses of periplocin extracted from Periploca sepium Bunge, including carnitine, acetylcarnitine, lysophosphatidylcholine, proline, glutamic acid, leucine, pantothenic acid, tryptophan, indole acrylic acid, and citric acid (Li et al., 2016a). These metabolites may affect the normal physiological function of the heart in terms of cell membrane stability, oxidative stress, mitochondrial damage, energy metabolism, cell homeostasis, and cell-to-cell signaling and interaction.
Genes
Omic technologies, especially genomics and transcriptomics, have gradually deepened in the field of drug toxicology, which provide new techniques for predicting new biomarkers to evaluate CMM-induced cardiotoxicity. Gene chip technology combined with real-time fluorescence quantitative analysis was applied to study acute toxicity of Venenum Bufonis on rat heart (Yang et al., 2011a). Results showed that Venenum Bufonis significantly altered the expression of Fxyd domain-containing ion transport regulator 3 (Fxyd3), Rho family GTPase 1 (Rnd1), ceruloplasmin, and Jun oncogene, which were involved in ion homeostasis, actin construction, and apoptosis. A study on the cardiotoxicity of aristolochic acid in zebrafish embryos revealed that a number of pro-inflammatory genes significantly increased, including COX-2, IL-1β, C/EBPB, C/EBPG, and SAA (Huang et al., 2007; Shi et al., 2017). In recent years, microRNAs have been linked to the cardiotoxicity of drugs, such as anthracyclines, bevacizumab, and cyclosporine A (Skala et al., 2019a). Some studies have found that the changes of some microRNAs are able to reflect the degree of myocardial injury in patients with acute myocardial infarction (AMI) (Yao, 2013). Studies suggested that As2O3 suppressed cardiac potassium channels via upregulating miR-21, miR-23a, miR-133, and miR-1 (Shan et al., 2013; Zhao et al., 2015). Although few studies about microRNAs are rarely involved in the cardiac toxicity of CMM, microRNAs have potential as early biomarkers and diagnostic tools for CMM-induced cardiotoxicity.
Mechanisms of CMM-Induced Cardiotoxicity
The risk compounds of CMM-induced cardiotoxicity directly or indirectly affect the structure and function of cardiomyocytes. Generally, multiple mechanisms interact with each other to induce cardiotoxicity, including ion homeostasis, oxidative stress and lipid peroxidation, mitochondrial damage, programmed cell death, inflammation, and metabolic disturbance (Figure 1).
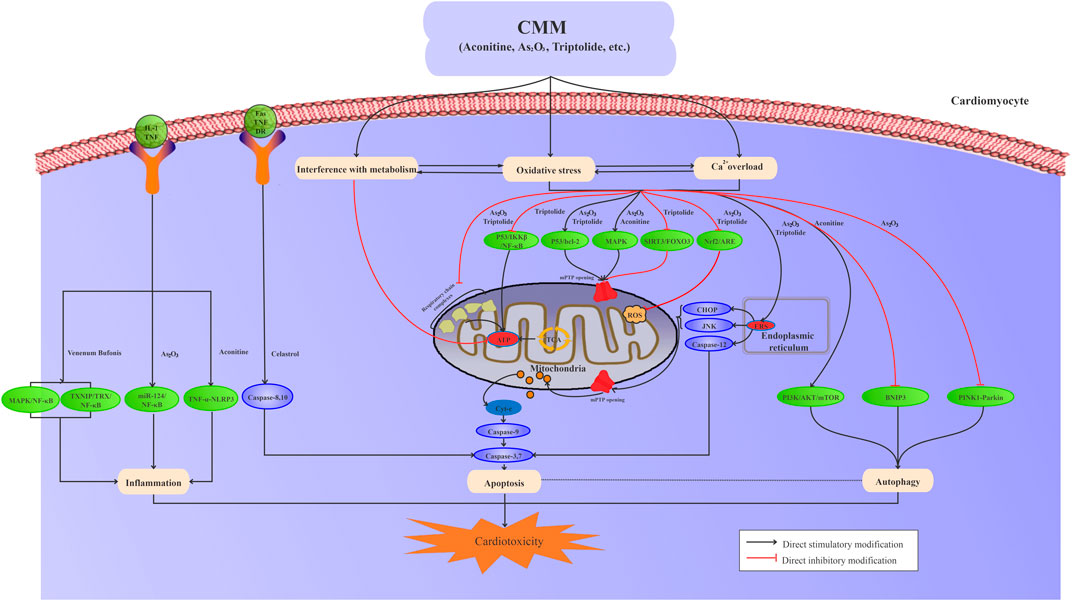
FIGURE 1. Interaction among oxidative stress, calcium overload, metabolic disturbance, mitochondrial damage, apoptosis, autophagy, and inflammation is involved in CMM-induced cardiotoxicity.
Interference With Ion Homeostasis
Various ion channels, including sodium, potassium, and calcium channels, are highly expressed on the myocardial cell membrane, which work together to maintain ion homeostasis in cardiomyocytes. Ion channel abnormalities on cardiomyocytes may lead to alterations in electrophysiological activity of the heart, ultimately inducing arrhythmias. The imbalance among sodium, potassium, and calcium ions in myocardial cells contributes to CMM-induced cardiotoxicity (Figure 2).
Sodium Channels
Sodium channel affects cardiomyocyte excitability, conductivity, auto-rhythm, and excitatory contraction coupling. The risk compounds of CMM-induced cardiotoxicity, especially alkaloids, can modulate the gating, permeability, and selectivity of voltage-gated sodium channels (VGSCs) by binding to site receptors. For example, aconitine binds to the site 2 receptor on VGSCs, thereby causing kinetic shift of sodium channels to trigger a persistent activation of sodium channels (Rao and Sikdar, 2000; Amran et al., 2004; Tak et al., 2016). In contrast to aconitine, a sodium channel agonist, lappaconitine irreversibly blocked human heart sodium channels by binding to the site 2 receptor (Wright, 2001). Moreover, batrachotoxin, one of the main active ingredients of Venenum Bufonis, is considered as a full activator on sodium channels, while aconitine and its analogs are served as partial activators (Wang et al., 2007). Grayanotoxins are toxic diterpenoids present in leaves of Rhododendron, Kalmia, Leucothoe, and Ericaceae (Yang et al., 2011b). Grayanotoxins exert selective effects on voltage-dependent sodium channels by eliminating fast sodium inactivation and inducing a hyperpolarizing shift in voltage dependence of channel activation (Maejima et al., 2003). Veratridine, a steroid-derived alkaloid derived from rhizomes of Veratrum album or seeds of Schoenocaulon officinale, preferentially binds to the activated sodium channels, thus impeding inactivation and increasing cell excitability (Chevalier et al., 2014). However, toxins secreted from scorpions are currently classified into two categories based on physiological effects on channel gating and binding properties: α-toxins that inhibit the fast inactivation process of sodium channels by binding to the site 3 receptor, and β-toxins that shift the threshold of channel activation to the negative membrane potentials by binding to the site 4 receptor (Ortiz and Possani, 2018; Wang et al., 2019b). SCN5A that is highly expressed in human cardiac muscle cells is the important gene encoding myocardial sodium channels, which controls the excitatory conduction of cardiac muscle cells. Studies showed that both aconitine and hypaconitine were shown to enhance mRNA expression of the SCN5A ion channel gene (Liu, 2007; Li et al., 2011). Abnormality in SCN5A gene expression can lead to an increase in sodium influx, which is associated with a variety of arrhythmias induced by some toxic CMM (Korkmaz et al., 2013).
Potassium Channels
The delayed rectifying potassium current (Ikr) channel protein on human cardiomyocytes is encoded by human hERG. Studies demonstrated that the loss of hERG function and the inhibition of Ikr caused by drugs prolonged QT interval and possibly induced TdP, thus leading to fatal arrhythmias (Liu et al., 2017). Some toxic CMM induce cardiotoxicity by directly inhibiting the expression of hERG channel protein or coding gene to suppress potassium channels. For instance, berberine blocked Ik1, Ik, and hERG channels expressed in Xenopus oocytes (Li et al., 2001). Diterpene alkaloids, such as aconitine and hypaconitine, induced QT interval prolongation through the inhibition of hERG potassium channels (Li et al., 2010; Xie et al., 2015; Kiss et al., 2017). Studies revealed that selectively targeting hERG channel contributes to the cardiotoxicity of strychnine (Wang et al., 2018). Celastrol inhibited the activities of hERG potassium channels, causal to QT interval prolongation (Sun et al., 2006). As2O3 induced expression deficiency of hERG potassium channel by decreasing specificity protein 1 (Sp1) level that upregulated hERG channel expression (Zhang et al., 2013b). MicroRNAs play a crucial role in regulating cardiac electrophysiological function by targeting potassium channel genes. Both miR-21 and miR-133 repressed the expression and transcription of hERG in As2O3-induced hERG inhibition. CMM also reduced Ikr through disrupting hERG trafficking without channel blockage. For example, berberine and arsenic induced hERG channel deficiency by inhibiting channel trafficking (Ficker et al., 2004; Eckhard Ficker and Peter Hawryluk, 2004). Unfolded protein response (UPR) was activated by channel trafficking inhibition, and endoplasmic reticulum (ER)-restricted hERG was ubiquitinated and degraded in lysosomes and proteasomes (Zhang et al., 2014). In addition, some CMM reduce Ikrvia promoting hERG channel protein degradation, which involves the regulation of related genes, proteins, and signaling pathways. The integral membrane protein caveolin-1 is co-localized with hERG on the cell surface, which has been reported to be involved in the drug-induced reduction of hERG plasma-membrane expression (Lin et al., 2008). Berberine was proved to reduce hERG membrane stability by disrupting caveolin-1 (Rodriguez-Menchaca et al., 2006; Yan et al., 2015). Lysosomal and proteasome degradation of hERG on the plasma membrane were accelerated during As2O3-induced cardiotoxicity. Further research showed that As2O3 decreased the caveolin-1 level, which accounted for As2O3-triggered hERG degradation (Yan et al., 2017). Certainly, some CMM can also affect potassium channels by suppressing Ito, Ik1, Ikur, and IkATP. Aconitine acted on both Ikur and Ik1 potassium channel inhibition (Wang et al., 2008; Kiss et al., 2017). Aconitine also could cause changes in Ito current channel of rat cardiomyocytes by reducing voltage-gated potassium channel 4.3 (Kv4.3) mRNA expression (Dong et al., 2009). Celastrol has a dual effect on Ik1 channel, including blocking potassium channel and inhibiting channel protein expression on cell surface (Sun et al., 2006). As2O3 activated IkATP and inhibited Ik1via upregulating miR-1 expression (Shan et al., 2013).
Calcium Homeostasis
Calcium is one of the most ubiquitous signal transduction molecules, which mediates various biological functions, such as cell excitability and conductivity, excitation–contraction coupling, and programmed cell death (Landstrom et al., 2017). Intracellular calcium homeostasis involves a dynamic balance between intracellular calcium intake and calcium emission. Abnormality of L-type Ca2+ currents (ICa-L), ryanodine receptor (RyR), sarcoplasmic reticulum Ca2+-ATPase (SERCA), and sodium–calcium exchanger (NCX) disrupts calcium homeostasis. Cardiac calcium homeostasis disorder contributes to adverse reactions induced by CMM. ICa-L is activated upon membrane depolarization, and then Ca2+ influx triggers the release of Ca2+via calcium release channels in sarcoplasmic reticulum (SR) (Bito et al., 2008; Eisner et al., 2009). Therefore, disturbance of ICa-L channel can lead to fatal cardiac arrhythmias (Eden et al., 2016). Bradycardia and hypotension caused by improper use of aconite may be attributed to the inhibition of ICa-L channels (Chou et al., 2018). Aconitine could block ICa-L channels and calcium-induced calcium release (CICR) in human-induced pluripotent stem cell–derived cardiomyocytes (hiPSC-CMs) (Wu et al., 2017). RyR is a calcium-release channel on ER/SR, which also plays a crucial role in maintaining intracellular calcium concentration. The sensitivity changes of RyR2 to Ca2+ release activation have been implicated in arrhythmias and heart failure (Dobrev and Wehrens, 2014; Landstrom et al., 2017). RyR2 activation may have a direct relationship with aconitine-induced arrhythmias (Fu et al., 2008). A study showed that aconitine significantly increased mRNA and protein expressions of RyR2 and NCX, thereby leading to uncoupling of Ca2+ signaling (Scriven et al., 2000; Bers, 2002). Oleander extract might markedly increase calcium concentration by inhibiting RyR in cardiomyocytes (Poindexter et al., 2007). RyR activity can be regulated by calmodulin (CaM) or calmodulin kinase (CaMK). For example, hypaconitine could downregulate CaM mRNA and protein expression, thus inhibiting CaMK II activation and RyR2 phosphorylation (Yi et al., 2012). Furthermore, CaM inhibits the opening of calcium release channel by binding to RyR2 (Sigalas et al., 2009a; Sigalas et al., 2009b). Intracellular Ca2+ diffusion is highly restricted due to the elaborate cytosolic Ca2+ chelating machinery. Intracellular calcium is mainly excreted through NCX and SERCA2a (Muller, 2018). NCX plays a pivotal role in regulating contractility and electrical activity in the heart. Studies found that arrhythmogenesis toxicity of aconitine was related to the increased expression of NCX (Fu et al., 2007; Zhou et al., 2013). NCX can operate in both Ca2+ efflux and influx modes depending on the internal and external concentrations of Na+ and Ca2+. Therefore, disturbance of NCX and SERCA function may destroy cytoplasmic calcium ion gradient, which not only induces calcium overload but also hinders the rapid entry of calcium ions into the cytosol after the ion channel is opened. Multiple studies have demonstrated that Ca2+ overload leads to oxidative damage and ER stress (Dong et al., 2010; Groenendyk et al., 2010; Chao et al., 2012; Guo et al., 2013; Xie et al., 2015). As2O3 inhibited SERCA2a activity in a time-dependent manner, thereby triggering ER stress (Zhang et al., 2016a). Aconitine-induced cardiotoxicity is related to intracellular Ca2+ elevation by accelerating ICa-L, increasing NCX expression, and decreasing the SERCA2a level (Zhou et al., 2013). Intracellular calcium homeostasis imbalance is one of the most important mechanisms underlying CMM-induced cardiotoxicity.
Ion Transport–Related ATPases
Ion transport–related ATPases also contribute to the cardiotoxicity of CMM. Sodium–potassium ATPase (Na+-K+ ATPase) maintains intracellular potassium and sodium ion gradients via consuming ATP. The steep sodium and potassium gradients in animal cells not only contribute to the rapid signal transmission through the opening of sodium or potassium selective channels in the plasma membrane in response to extracellular signals but also are used to facilitate the secondary transport of sugars, neurotransmitters, amino acids, metabolites, and some ions, such as H+, Ca2+, and Cl− (Clausen et al., 2017). Egyptian green toad Bufo viridis skin secretions and bufalin inhibited the Na+-K+ ATPase activity, thus inducing cardiac adverse reaction (Roger et al., 2002; Abdel-Rahman et al., 2010). Ca2+-ATPase and Ca2+-Mg2+ ATPase can promote the removal of Ca2+ from cell membrane after myocardial contraction. Once the activities of these Ca2+-ATPases decreased, intracellular Ca2+ continuously increased to Ca2+ overload. Aconitine could cause calcium overload, which involved the inhibition of Na+-K+ ATPase, Ca2+-ATPase, and Ca2+-Mg2+ ATPase activities (Zhang et al., 2016b).
Oxidative Stress
Oxidative stress is a result of overproduction of oxidative-free radicals and ROS (Newsholme et al., 2016). Excessive oxidative stress not only directly causes DNA and protein damage but also induces lipid peroxidation, calcium overload, mitochondrial structure and function damage, and cell death, thereby inducing myocardial damage (Manna et al., 2008; Zhang et al., 2013a; Xu et al., 2014; Yang et al., 2017). Previous studies found that triptolide increased intracellular ROS, which in turn depolarized mitochondrial membrane potential (MMP), reduced the Bax/Bcl-2 ratio, released cytochrome C, activated caspase-3, and ultimately triggered cell apoptosis (Zhou et al., 2014). Similarly, evodiamine also increased intracellular ROS level, induced lipid peroxidation, and caused changes in cell membrane stability, ultimately resulting in myocardial damage (Yang et al., 2017). Oxidative stress also can trigger the inflammatory reaction and inhibit ATPase activity. For example, ROS induced by As2O3 was able to activate pro-inflammatory genes and upregulate the expression of inflammatory cytokines, leading to the occurrence of inflammatory reaction in myocardium. As2O3 also inhibited the ROS-mediated myocardial membrane ATPase activity and disrupted the steady state of intracellular electrolytes (Binu et al., 2017; Li et al., 2017). Moreover, calcium mobilization can affect ROS generation, and on the contrary, the presence of ROS induces Ca2+ influx (Giambelluca and Gende, 2008; Holmstrom and Finkel, 2014). ROS can regulate calcium pump, enzyme, and receptor activities to maintain normal physiological function of cardiomyocytes (Feissner et al., 2009). Additionally, ROS impairs mitochondrial structure and function both alone and in combination with calcium overload (Webster, 2012). Mitochondria permeability transition pore (mPTP) is sensitive to both ROS and calcium overload, thereby triggering endogenous pathways of programmed necrosis and death.
Cardiomyocytes can activate an antioxidative stress cell defense system to resist myocardial damage caused by oxidative stress. However, long-term or excessive use of CMM that can induce cardiotoxicity will destroy the antioxidant defense system and further aggravate oxidative stress. For example, As2O3 reduced GSH-dependent antioxidant and anti-peroxidase enzymes, while increasing free radicals, intracellular Ca2+ concentration, and lipid peroxidation, thereby further exacerbating oxidative damage (Sfaxi et al., 2016; Varghese et al., 2017). Another study also showed that As2O3 induced ROS generation and downregulated the expression of Nrf2 and heme oxidase (HO-1) genes (Zhang et al., 2013a).
Mitochondrial Toxicity
Mitochondria are one of the most critical target organs for cardiotoxicity induced by CMM. Some CMM can directly damage mitochondrial structure and function. For example, As2O3 promoted mitochondrial peroxide production; significantly reduced the activity of respiratory chain complexes, such as mitochondrial complexes I, III, and IV; reduced MMP; and caused mitochondrial swelling in H9c2 cardiomyocytes (Vineetha et al., 2015). Mitochondrial dysfunction is characterized by an increase in mitochondrial outer membrane permeabilization (MOMP), which induces mitochondrial swelling following proapoptotic protein release, electron transport chain disruption, and ATP depletion (Zhang et al., 2018a). The opening of mPTP contributes to MOMP changes via the depletion of MMP. The persistent opening of mPTP is now recognized as a major inducement of cell death. Triptolide inhibited superoxide dismutase (SOD) and catalase (CAT) activities, induced ROS accumulation, reduced MMP, and eventually led to mitochondrial toxicity (Yang et al., 2016). Experiments in vivo and in vitro showed that triptolide permeabilized the outer mitochondrial membrane and promoted the opening of mPTP, thereby causing mitochondrial dysfunction and apoptosis (Wang et al., 2016b; Xi et al., 2018). In addition, CMM that has cardiotoxicity can also indirectly damage myocardial mitochondria by regulating mitochondrial outer membrane integrity, mitochondrial dynamics, mitochondria-dependent apoptosis genes, and proteins. For instance, triptolide triggered MOMP through the p53-regulated Bcl-2 family, which elicited mitochondria dysfunction and apoptosis (Xi et al., 2018). As2O3 reduced peroxisome proliferator-activated receptor gamma coactivator 1 (PGC-1α) level, and disrupted mitochondrial synthesis and fission and fusion function (Di et al., 2018).
Induction of Cell Death
Oxidative stress and calcium overload are the main inducements for triggering programmed cell death in cardiomyocytes. As mentioned above, long-term unreasonable use of cardiotoxic CMM can induce ROS generation and disturb calcium homeostasis. One study showed that As2O3 could initiate ER stress apoptosis pathway through ATF6, PERK, and IRE1 (Zhang et al., 2017). As an active programmed cell death pathway, apoptosis is regulated by multiple signaling pathways. Aconitine could upregulate the expression of p38 MAPKs, further damage MMP, induce changes in mitochondrial permeability, activate caspase-3, and ultimately result in mitochondria-dependent apoptosis (Sun et al., 2014). The p53 signaling pathway plays an important regulatory role in triptolide-induced apoptosis (Xi et al., 2018). Triptolide downregulated the expression of Nrf2 and induced the ROS-triggered mitochondrial apoptosis pathway (Vineetha et al., 2018). The serine/threonine kinase Akt signaling pathway was also found to be involved in the regulation of As2O3-induced mitochondrial apoptosis in cardiomyocytes (Wang et al., 2013). Autophagic degradation of cellular components is essential to maintain cellular homeostasis. Hence, autophagy, another type of programmed cell death, contributes to cardiotoxicity induced by CMM. Excessive release of ROS is one of the main endogenous factors to enhance autophagy (Scherz-Shouval et al., 2007). As2O3 induced autophagy and apoptosis by promoting oxidative stress (Fan et al., 2014). The signaling pathways currently known to participate in the regulation of autophagy comprise Parkin, ROCK, PI3K/Akt/mTOR, p70S6 kinase, and ERK1/2 (Huang et al., 2015; Zhou et al., 2015). Another study showed that As2O3 induced a perturbation in Parkin-mediated mitophagy (Watanabe et al., 2014). Besides, arsenic induced cardiac damage and autophagy via activating the PI3K/Akt/mTOR pathway in the hearts of chickens (Li et al., 2018b). Excessive autophagy was susceptible to various cardiovascular events, such as ischemia-reperfusion injury (Zheng and Bao, 2017). Autophagy can suppress apoptosis under normal physiological conditions. For example, aconitine induced autophagy and apoptosis, in which autophagy had an antagonistic effect on apoptosis (Hu et al., 2018). Whether autophagy is beneficial or detrimental depends on intracellular autophagic machinery and the burden of substrate receptor proteins targeted for autophagy (Baehrecke, 2005).
Involvement of Substance Metabolism
Amino Acid Metabolism
Amino acid metabolism affects protein synthesis, energy metabolism, redox balance, and intracellular material homeostasis. Metabolic disorders of various amino acids, such as tryptophan, leucine, alanine, proline, lysine, and tyrosine, were involved in CMM-induced cardiotoxicity. Tryptophan plays a vital role in maintaining cell growth and energy metabolism. In metabolomic studies of some CMM, such as Radix Aconiti Kusnezoffii (Caowu), Radix Aconiti Lateralis Preparata (Fuzi), Pinellia ternata, and Periploca sepium Bunge, tryptophan significantly reduced in myocardial cells, which affected energy supply to produce cardiotoxicity (Wang et al., 2012; Zhang et al., 2013c; Li et al., 2016a). In addition, experimental results showed that valine, leucine, and proline all decreased in cardiomyocytes exposed to periplocin, suggesting that amino acid metabolism disorders occurred during periplocin-induced cardiomyocyte toxicity (Li et al., 2016a). Lysine, alanine, and arginine in serum of Venenum Bufonis–treated rats showed a decreasing trend, indicating that oxidative stress injury and energy metabolism disturbance were induced by Venenum Bufonis (Dong et al., 2015). Different from the above amino acids, l-carnitine is a type of nonprotein amino acid. Carnitine and acetylcarnitine are also involved in fatty acid transport and oxidation by mitochondrial enzymes, which is essential for the formation of acetyl-CoA (Reuter and Evans, 2012; Li et al., 2015). Furthermore, l-carnitine also has antioxidant activity to improve mitochondrial function. Periplocin significantly reduced carnitine and acetylcarnitine in myocardial tissues, revealing that tricarboxylic acid (TCA) cycle related with energy metabolism was interfered (Li et al., 2016a). On the contrary, both l-carnitine and l-acetylcarnitine significantly increased after Pinelliae Rhizoma administration (Zhang et al., 2013c).
Lipid Metabolism
Fatty acids and their derivatives are involved in saturated fatty acid oxidation and unsaturated fatty acid peroxidation. β-oxidation of fatty acids, an important pathway for mitochondrial function in cardiomyocytes, is closely related to glucose metabolism and apoptosis in cardiomyocytes. In myocardial lipidomic study of Fuzi, arachidonic acid, linoleic acid, and eicosapentaenoic acid displayed declining tendencies, while saturated fatty acid levels, such as stearic acid and 5-tridecynoic acid, were upgraded. Peroxidation of unsaturated fatty acids produces active free radicals that induce oxidative stress. Accordingly, abnormal peroxidation of unsaturated fatty acids caused by Fuzi contributed to oxidation damage in myocardial mitochondria (Cai et al., 2013). Similarly, oleic acid and linoleic acid were downregulated, while arachidonic acid was upregulated in the metabolomic study of Venenum Bufonis. Venenum Bufonis could interfere with lipid metabolism through the inhibition of free fatty acid reacylation or the activation of protein kinase pathways, thereby inducing heart damage (Liang et al., 2011).
As a critical component of cellular membranes, phospholipids interact with all membrane proteins and various non-membrane proteins, thus mediating signal transduction. Significant changes of sphingosine, phosphatidylcholine, phosphatidylserine, phosphatidylethanolamine, lysophosphatidylethanolamine, and lysophosphatidylcholine were uncovered in CMM-induced cardiotoxicity. These substances are involved in metabolic pathways, such as phospholipid and sphingolipid metabolism. For example, Caowu- and Fuzi-induced cardiotoxicity were responsible for sphingolipid metabolism (Wang et al., 2012). Sphingolipids have emerged as key mediators of stress responses (An et al., 2011). Sphingolipid metabolism disorder resulted in increased plasma-membrane fluidity, which rendered them susceptible to ROS, thus enhancing oxidative damage to cellular proteins (Rao et al., 2007). Studies showed that perturbation of phospholipid or sphingolipid metabolism contributed to Pinellia ternate and periplocin-induced cardiotoxicity (Zhang et al., 2013c; Li et al., 2016a).
Glucose Metabolism
Abnormal blood glucose is an important trigger for cardiovascular diseases. The anaerobic glycolysis and aerobic oxidation of glucose in metabolism pathway are the main link for ATP production. Metabolomic studies showed that various cardiotoxic CMM induced glucose metabolism disturbance, which mainly involved some metabolites changes, such as pyruvic acid, malic acid, citric acid, and succinate (Li et al., 2008; Li et al., 2016a). These organic acids as an important intermediate of cell cycle affect TCA cycle of myocardial cells and energy metabolism. In addition, metabolomic analysis of aconitum alkaloids showed that lactate and lipids levels increased, while glucose reduced in myocardial cells, demonstrating that aconitum alkaloids induced abnormality in energy utilization with a higher glycolysis rate (Sun et al., 2009).
Other Mechanisms
DNA damage, inflammatory response, and trace element homeostasis imbalance may also be involved in CMM-induced cardiotoxicity. For instance, berberine and arsenic could induce DNA damage in heart cells (Manna et al., 2008; Xu et al., 2014). Triptolide inhibited RNA polymerase by covalently binding to a subunit of transcription initiation factor TFIIH, which led to the inhibition of nucleotide excision repair and DNA damage (Titov et al., 2011). Venenum Bufonis inhibited the expression of Fxyd domain-containing ion transport regulator 3 (Fxyd3) and ceruloplasmin (Cp) genes, which resulted in the accumulation of Fe2+ in cardiac cells to destroy contractile function of the heart (Yang et al., 2011a). Recently, inflammatory response plays a critical role in the development, course, severity, and outcomes of heart failure (Papadimitriou and Kalogeropoulos, 2015). As2O3 and aristolochic acid could trigger inflammatory response, which was related to the progressive development of cardiac defects (Li et al., 2017; Shi et al., 2017). A study on the cardiotoxicity of aristolochic acid in zebrafish embryos revealed that a number of pro-inflammatory genes increased, including COX-2, IL-1β, C/EBPB, C/EBPG, and SAA (Huang et al., 2007; Shi et al., 2017). Venenum Bufonis–induced cardiotoxicity might involve inflammatory response through TXNIP/TRX/NF-κB and MAPK/NF-κB pathways (Bi et al., 2016). In addition, As2O3 was reported to disturb trace elements’ homeostasis, which favored the progression of mitochondrial damage (Sfaxi et al., 2016; Li et al., 2018a).
Discussion
With the booming market of CMM worldwide, a new evaluation system must be formulated for assessing drug efficacy, effectiveness, and toxicity to optimize the therapeutic and preventive potential of CMM. In recent years, the safety of CMM has been paid more and more attention by clinicians, patients, and scientific researchers, such as the cardiotoxicity of aconitum species, the hepatotoxicity of Polygonum multiflorum, and the nephrotoxicity of aristolochic acids. Some CMM have been proven to present obvious cardiotoxicity in literature records, case reports, and experimental studies. In response to the problem of adverse cardiac reactions induced by CMM, research teams have carried out a large number of studies on the performances and characteristics, toxic substance basis, and mechanisms of cardiotoxicity induced by CMM, their extracts, or single compounds. Our review concluded the risk compounds, preclinical toxicity evaluation, and potential mechanisms of CMM-induced cardiotoxicity, which was beneficial to establish a preclinical evaluation system for CMM safety to promote the development of new drugs.
CMM has a complex source, numerous varieties, and a wide variety of phytochemical components. Most of CMM have substances with toxicity and efficacy dual characters, such as aconitine, bufotoxin, and cardiac glycosides (Peng, 2017; Peng et al., 2017). Furthermore, toxic compounds and active compounds containing the same type of CMM can be transformed into each other under different physiological and pathological conditions. For example, diester alkaloids derived from aconitum species are considered highly toxic substances, while they have also proved to be the main active ingredient for the treatment of pain and leukemia (Nie et al., 2017). In addition, chemical composition and content may change under different planting resources, processing methods, or drug compatibility. Thus, more and more researchers select risk compounds in CMM as the research objects to explore the mechanisms of CMM-induced cardiotoxicity. With the advancement of modern separation and analysis technologies, the types and quantities of monomer compounds in traditional toxic CMM are increasing. High-throughput analysis methods, such as omic technology, high content analysis, molecular docking, and network pharmacology, are used to determine the risk substances of CMM-induced cardiotoxicity (Shi et al., 2016; Su et al., 2016; He et al., 2019). Based on the phytochemical structures of toxic substances in CMM, these risk compounds mainly include alkaloids, terpenoids, steroids, heavy metals, organic acids, and toxic proteins or peptides. Alkaloids are the biggest category of risk compounds present in CMM that induce cardiotoxicity, including diterpene alkaloids, isoquinoline alkaloids, indole alkaloids, and others. Terpenoids are also an important substance of CMM-induced cardiotoxicity, such as triptolide, celastrol, and rhodojaponin. Various steroids, including cardiac glycosides, bufadienolides, and ophiopogonin, also contribute to CMM-induced cardiotoxicity. In addition, heavy metals, organic acids, toxic proteins, and peptides are involved in CMM-induced cardiotoxicity. Phytomedicine is easily affected by environmental pollution, thus inducing heavy metals to exceed the standard, and mineral drugs contain high levels of heavy metals, such as arsenic, mercury, and lead. Toxic plants and animals carry or secrete organic acids, toxic proteins, or peptides to defense natural enemies.
Multicomponent, multi-target, and multichannel characteristics of CMM make the toxicological mechanism very complicated. Most of the previous studies focus on single compound, single target, or single pathway to explore the mechanism of CMM-induced cardiotoxicity. With the development of various modern biological techniques, such as systematic toxicology, network toxicology, and computer toxicology, the network interaction mechanism in CMM-induced cardiotoxicity is gradually uncovered. We searched the related literature for the latest twenty years, mainly focusing on cell and animal experiments. Various mechanisms, including ion homeostasis, oxidative stress, mitochondrial damage, apoptosis and autophagy, and metabolic disturbance, are involved in CMM-induced cardiotoxicity. Excessive activation or inhibition of ion channels, including sodium, potassium, and calcium channels, contributes to arrhythmia. Ion channels can become the targets for many toxic CMM, such as aconitine, hypaconitine, berberine, and Venenum Bufonis. Therefore, imbalance among sodium, potassium, and calcium ions is the important mechanism of CMM-induced cardiotoxicity. Overproduction of ROS resulting in oxidative damage has been testified to be the vital factor for calcium overload, which suggested that oxidative damage affected intracellular calcium homeostasis. Mitochondria are highly susceptible to ROS generation. The risk substances in CMM can directly or indirectly influence mitochondrial structure and function, thus presenting mitochondrial toxicity. Both oxidative stress and abnormal ion homeostasis lead to excessive apoptosis and autophagy in cardiomyocytes. Apoptosis and autophagy-related genes or pathways may be activated or inhibited to regulate cell fate. Abnormal metabolism in cardiac cells also results in cardiotoxicity. Mitochondria toxicity induced by the risk compounds in CMM can affect cell energy metabolism. In addition, substance metabolism, including amino acid, lipids, and glucose, was also reported to be involved in the cardiotoxicity of CMM. Thus, multiple mechanisms may be orderly involved in the cardiotoxicity induced by CMM, and an interactive effect between these mechanisms is demonstrated. Although the material basis of CMM-induced toxicity and efficacy can be transformed into each other under certain conditions, researches on the dosage, opportunity, and body status of transformation mechanism between the toxicity and efficacy are still scarce. Therefore, the toxicity–efficacy transformation mechanism underlying the “dose–time–efficacy/toxicity” path is urgent to be clarified for evaluating the cardiotoxicity of CMM (Peng et al., 2008; Peng, 2017; Peng et al., 2017).
In clinical studies, there are few specific evaluation indicators for CMM-induced cardiotoxicity. At present, evaluation on CMM-induced cardiotoxicity mainly takes the indicators of cardiac function and structural damage caused by chemical drugs as a reference, including ECG, myocardial zymogram, echocardiography, endomyocardial biopsy, and biomarkers. In preclinical evaluation or new drug development stage, the cardiotoxicity of CMM should be systematically evaluated at the overall, tissue, cellular, and molecular levels. Overall evaluation is mainly based on cardiac function evaluation, including conventional ECG, echocardiography, and MRI, which is especially manifested with QT/QTc interval prolongation or TdP. Comprehensive study of QT/QTc is the most critical link of drug cardiac safety evaluation (Food and Drug Administration, 2005). Observation on the histopathological changes of the heart caused by CMM is one of the specific diagnostic criteria. For example, although endomyocardial biopsy technique is recognized as the most sensitive and specific method for evaluating cardiac toxicity induced by drugs, it is invasive examination with high technical requirements (Jones and Miles, 2005). At the cellular level, it is possible to observe changes in cell morphology, myocardial cell pulsation, cell survival rate, and cell membrane integrity, which are of great significance for revealing the mechanism of drug-induced cardiotoxicity. Serum myocardial zymogram, including CK, CK-MB, LDH, and AST, may be slightly different because of different test kits. Due to the poor specificity of LDH, AST, and CK for assessing cardiotoxicity, CK-MB is the commonly used clinical myocardial enzyme. Drug-induced heart injury can lead to many biomarkers changes in the early stage, including proteins or polypeptides, metabolic small molecules, and genes. Common proteins or peptides include cardiac troponins, BNP, NT-proBNP, ANP, and H-FABP. cTnT/TnI can detect early cardiotoxicity caused by anthracyclines. BNP and NT-proBNP can objectively determine the severity of heart failure. H-FABP is the most ideal myocardial marker for identifying myocardial injury. Small metabolic molecules include citric acid, GSH, phosphatidylcholine, and uric acid in serum. Metabolomic method is expected to find more specific small metabolic molecules for evaluating CMM-induced cardiotoxicity (Su et al., 2016; Liu et al., 2019). In addition, genes related to cardiotoxicity are also used to early identify the cardiotoxicity of CMM, such as transcription factors, inflammatory factors, pathway regulatory genes, and microRNAs. MicroRNAs have potential as early biomarkers and diagnostic tools for cardiotoxicity (Skala et al., 2019a).
Conclusion
Numerous reports about drug safety have brought more cautions that CMM is associated with several cardiovascular events, suggesting that evaluation on the cardiac safety of CMM is quite urgent. CMM-induced cardiotoxicity is characterized by diverse components, multiple manifestations, and complex mechanisms. Thus, we concluded the risk compounds, preclinical toxicity evaluation, and potential mechanisms of CMM-induced cardiotoxicity through screening the related studies for the last twenty years. It is worth noting that a complex network regulation relationship among these risk compounds, toxic effects, and toxic mechanisms is being gradually revealed. Frontier technologies, such as omics, systematic toxicology, and network pharmacology, provide important support for revealing this network interaction relationship. Our review is greatly beneficial to understand the relationship between the toxicity and efficacy induced by CMM and provide a reference for establishing the cardiac safety evaluation system of CMM.
Author Contributions
All authors listed have made a substantial, direct, and intellectual contribution to the work and approved it for publication.
Funding
This work was supported by the National Natural Science Foundation of China (82004056, 82003879, 81891010, 81891012, and 81630101), Sichuan Science and Technology Agency Foundation (2018JZ0081), and Xinlin Scholar Research Promotion Project of Chengdu University of Traditional Chinese Medicine (XGZX2011).
Conflict of Interest
The authors declare that the research was conducted in the absence of any commercial or financial relationships that could be construed as a potential conflict of interest.
Abbreviations
CMM Chinese materia medica
ECG Electrocardiogram
ICH International Conference on Harmonisation
CNKI China National Knowledge Infrastructure
hERG Human ether-a-go-go-related gene
HR Heart rate
MRI Magnetic resonance imaging
APD Action potential duration
TdP Torsades de pointes
LVEF Left ventricular ejection fraction
BMSCs Bone marrow mesenchymal stem cells
CK Creatine kinases
CK-MB Creatine kinase isoenzymes
LDH Lactic dehydrogenase
AST Aspartate aminotransferases
>α-HBDH α-Hydroxybutyrate acid dehydrogenases
BNP Brain natriuretic peptide
NT-proBNP N-terminal brain natriuretic peptide precursor
ANP Atrial natriuretic peptide
cTnT Troponin T
cTnI Troponin I
FABP Fatty acid–binding protein
GSH Glutathione
ROS Reactive oxygen species
1HNMR Nuclear magnetic resonance hydrogen spectroscopy
Fxyd3 Fxyd domain-containing ion transport regulator 3 Fxyd domain-containing ion transport regulator 3
Rnd1 Rho family GTPase 1
AMI Acute myocardial infarction
VGSCs Voltage-gated sodium channels
Sp1 Specificity protein 1
UPR Unfolded protein response
ER Endoplasmic reticulum
Kv4.3 Voltage-gated potassium channel 4.3
ICa-L L-type Ca2+ currents
RyR Ryanodine receptor
SERCA Sarcoplasmic reticulum Ca2+-ATPase
NCX Sodium–calcium exchanger
SR Sarcoplasmic reticulum
CICR Calcium-induced calcium release
hiPSC-CMs Human-induced pluripotent stem cell–derived cardiomyocytes
CaM Calmodulin
CaMK Calmodulin kinase
MMP Mitochondrial membrane potential
mPTP Mitochondria permeability transition pore
HO-1 Heme oxidase
MOMP Mitochondrial outer membrane permeabilization
PGC-1α Peroxisome proliferator-activated receptor gamma coactivator 1
TCA Tricarboxylic acid
Fxyd3 Fxyd domain-containing ion transport regulator 3 Fxyd domain-containing ion transport regulator 3
Cp Ceruloplasmin
References
Abdel-Rahman, M. A., Ahmed, S. H., and Nabil, Z. I. (2010). In vitro cardiotoxicity and mechanism of action of the Egyptian green toad Bufo viridis skin secretions. Toxicol. Vitro 24 (2), 480–485. doi:10.1016/j.tiv.2009.09.021
Adamson, P. B., Suarez, J., Ellis, E., Kanaly, T., and Vanoli, E. (2004). Ephedrine increases ventricular arrhythmias in conscious dogs after myocardial infarction. J. Am. Coll. Cardiol. 44 (8), 1675–1678. doi:10.1016/j.jacc.2004.07.041
Amran, M. S., Hashimoto, K., and Homma, N. (2004). Effects of sodium-calcium exchange inhibitors, KB-R7943 and SEA0400, on aconitine-induced arrhythmias in guinea pigs in vivo, in vitro, and in computer simulation studies. J. Pharmacol. Exp. Ther. 310 (1), 83–89. doi:10.1124/jpet.104.066951
An, D., Na, C., Bielawski, J., Hannun, Y. A., and Kasper, D. L. (2011). Membrane sphingolipids as essential molecular signals for Bacteroides survival in the intestine. Proc. Natl. Acad. Sci. USA 108 (Suppl. 1), 4666–4671. doi:10.1073/pnas.1001501107
Baehrecke, E. H. (2005). Autophagy: dual roles in life and death? Nat. Rev. Mol. Cell Biol. 6 (6), 505–510. doi:10.1038/nrm1666
Balakumar, P., and Kaur, J. (2009). Arsenic exposure and cardiovascular disorders: an overview. Cardiovasc. Toxicol. 9 (4), 169–176. doi:10.1007/s12012-009-9050-6
Bao, Z., Han, Z., Zhang, B., Yu, Y., Xu, Z., Ma, W., et al. (2019). Arsenic trioxide blocked proliferation and cardiomyocyte differentiation of human induced pluripotent stem cells: implication in cardiac developmental toxicity. Toxicol. Lett. 309, 51–58. doi:10.1016/j.toxlet.2019.03.008
Barbey, J. T., Pezzullo, J. C., and Soignet, S. L. (2003). Effect of arsenic trioxide on QT interval in patients with advanced malignancies. J. Clin. Oncol. 21 (19), 3609–3615. doi:10.1200/JCO.2003.10.009
Bers, D. M. (2002). Cardiac excitation-contraction coupling. Nature 415 (6868), 198–205. doi:10.1038/415198a
Bi, Q. R., Hou, J. J., Qi, P., Ma, C. H., Feng, R. H., Yan, B. P., et al. (2016). TXNIP/TRX/NF-κB and MAPK/NF-κB pathways involved in the cardiotoxicity induced by Venenum Bufonis in rats. Sci. Rep. 6, 22759. doi:10.1038/srep22759
Binu, P., Priya, N., Abhilash, S., Vineetha, R. C., and Nair, R. H. (2017). Studies on curative efficacy of monoterpene eugenol on anti- leukemic drug arsenic trioxide induced cardiotoxicity. Biomed. Pharmacother. 91, 559–566. doi:10.1016/j.biopha.2017.04.087
Bito, V., Heinzel, F. R., Biesmans, L., Antoons, G., and Sipido, K. R. (2008). Crosstalk between L-type Ca2+ channels and the sarcoplasmic reticulum: alterations during cardiac remodelling. Cardiovasc. Res. 77 (2), 315–324. doi:10.1093/cvr/cvm063
Botelho, A. F. M., Santos-Miranda, A., Joca, H. C., Mattoso, C. R. S., de Oliveira, M. S., Pierezan, F., et al. (2017). Hydroalcoholic extract from Nerium oleander L. (Apocynaceae) elicits arrhythmogenic activity. J. Ethnopharmacol. 206, 170–177. doi:10.1016/j.jep.2017.05.031
Cai, B. Z., Meng, F. Y., Zhu, S. L., Zhao, J., Liu, J. Q., Liu, C. J., et al. (2010). Arsenic trioxide induces the apoptosis in bone marrow mesenchymal stem cells by intracellular calcium signal and caspase-3 pathways. Toxicol. Lett. 193 (2), 173–178. doi:10.1016/j.toxlet.2010.01.001
Cai, Y., Gao, Y., Tan, G., Wu, S., Dong, X., Lou, Z., et al. (2013). Myocardial lipidomics profiling delineate the toxicity of traditional Chinese medicine Aconiti Lateralis radix praeparata. J. Ethnopharmacol. 147 (2), 349–356. doi:10.1016/j.jep.2013.03.017
Chao, C. C., Huang, C. C., Lu, D. Y., Wong, K. L., Chen, Y. R., Cheng, T. H., et al. (2012). Ca2+ store depletion and endoplasmic reticulum stress are involved in P2X7 receptor-mediated neurotoxicity in differentiated NG108-15 cells. J. Cell Biochem. 113 (4), 1377–1385. doi:10.1002/jcb.24010
Chen, E. H., Liang, J. Q., and Song, X. N. (2016). Analysis on cardiotoxicity related indexes of anthracycline antitumor drugs. Shenzhen J. Integr. Tradit Chin. West Med. 26 (12), 24–25. doi:10.3892/mco.2019.1854
Chen, H. M., Xie, X. F., and Peng, C. (2017). In vitro cytotoxicity evaluation indicator and test methods applied in toxicology of traditional Chinese medicine. Chin. J. Exper Tradit Med. Formulae 23 (22), 202–210. doi:10.13422/j.cnki.syfjx.2017220202
Chen, X., Shan, H., Zhao, J., Hong, Y., Bai, Y., Sun, I., et al. (2010). L-type calcium current (ICa,L) and inward rectifier potassium current (Ik1) are involved in QT prolongation induced by arsenic trioxide in rat. Cell Physiol Biochem. 26 (6), 967–974. doi:10.1159/000324005
Chevalier, M., Amuzescu, B., Gawali, V., Todt, H., Knott, T., Scheel, O., et al. (2014). Late cardiac sodium current can be assessed using automated patch-clamp. F1000Res. 3, 245. doi:10.12688/f1000research.5544.1
Chou, P. Y., Wang, C. C., Tai, C. J., Yang, T. L., and Tang, Y. J. (2018). Bradycardia and hypotension from improper use of aconite root: a case report and brief review. Complement. Med. Res. 25 (5), 338–343. doi:10.1159/000489179
Clausen, M. V., Hilbers, F., and Poulsen, H. (2017). The structure and function of the Na,K-ATPase isoforms in health and disease. Front. Physiol. 8, 371. doi:10.3389/fphys.2017.00371
Demiryürek, A. T., Yildiz, G., Eşiyok, S., and Altuğ, S. (2002). Protective effects of poly (ADP-ribose) synthase inhibitors on digoxin-induced cardiotoxicity in guinea-pig isolated hearts. Pharmacol. Res. 45 (3), 189–194. doi:10.1006/phrs.2002.0945
Demiryürek, A. T., and Demiryürek, S. (2005). Cardiotoxicity of digitalis glycosides: roles of autonomic pathways, autacoids and ion channels. Autonom Auta Pharm. 25 (2), 35–52. doi:10.1111/j.1474-8673.2004.00334.x
Deng, L. J., Wang, L. H., Peng, C. K., Li, Y. B., Huang, M. H., Chen, M. F., et al. (2017). Fibroblast activation protein α activated tripeptide bufadienolide antitumor prodrug with reduced cardiotoxicity. J. Med. Chem. 60 (13), 5320–5333. doi:10.1021/acs.jmedchem.6b01755
Di, W., Lv, J., Jiang, S., Lu, C., Yang, Z., Ma, Z., et al. (2018). PGC-1: the energetic regulator in cardiac metabolism. Curr. Issues Mol. Biol. 28, 29–46. doi:10.21775/cimb.028.029
Dobrev, D., and Wehrens, X. H. (2014). Role of RyR2 phosphorylation in heart failure and arrhythmias: controversies around ryanodine receptor phosphorylation in cardiac disease. Circ. Res. 114 (8), 1311–1319. doi:10.1161/CIRCRESAHA.114.300568
Dong, G., Wei, D., Wang, J., Guo, P., Li, M., Yang, M., et al. (2015). Study of the cardiotoxicity of Venenum Bufonis in rats using an 1H NMR-based metabolomics approach. PLoS One 10 (3), e0119515. doi:10.1371/journal.pone.0119515
Dong, L. C., Zhang, X. H., Ma, J., Luo, N., Song, W., Li, P., et al. (2014). The integrated pharmacokinetics of major rhodojaponins correlates with the cardiotoxicity after oral administration of Rhododendri Mollis Flos extract in rats. J. Ethnopharmacol. 157, 69–78. doi:10.1016/j.jep.2014.09.021
Dong, S., Teng, Z., Lu, F. H., Zhao, Y. J., Li, H., Ren, H., et al. (2010). Post-conditioning protects cardiomyocytes from apoptosis via PKC(epsilon)-interacting with calcium-sensing receptors to inhibit endo(sarco)plasmic reticulum-mitochondria crosstalk. Mol. Cell Biochem. 341 (1-2), 195–206. doi:10.1007/s11010-010-0450-5
Dong, X., Zhao, S. P., Liu, Y., et al. (2009). Protective effect of Liquiritin on cardiocyte injury of neonate rat induced by aconitine. China J. Tradi Chin. Med. Pharm. 24 (2), 163–166.
Eden, M., Meder, B., Völkers, M., Poomvanicha, M., Domes, K., Branchereau, M., et al. (2016). Myoscape controls cardiac calcium cycling and contractility via regulation of L-type calcium channel surface expression. Nat. Commun. 7, 11317. doi:10.13155/ncomms11317
Eisner, D. A., Kashimura, T., Venetucci, L. A., and Trafford, A. W. (2009). From the ryanodine receptor to cardiac arrhythmias. Circ. J. 73 (9), 1561–1567. doi:10.1253/circj.cj-09-0478
Fan, X. J., Yu, H., and Ren, J. (2011). Homeostasis and compensatory homeostasis: bridging western medicine and traditional Chinese medicine. Curr. Cardiol. Rev. 7, 43–46. doi:10.2174/157340311795677671
Fan, Y., Chen, M., Meng, J., Yu, L., Tu, Y., Wan, L., et al. (2014). Arsenic trioxide and resveratrol show synergistic anti-leukemia activity and neutralized cardiotoxicity. PLoS One 9 (8), e105890. doi:10.1371/journal.pone.0105890
Feissner, R. F., Skalska, J., Gaum, W. E., and Sheu, S. S. (2009). Crosstalk signaling between mitochondrial Ca2+ and ROS. Front. Biosci. (Landmark Ed) 14, 1197–1218. doi:10.2741/3303
Ficker, E., Kuryshev, Y. A., Dennis, A. T., Obejero-Paz, C., Wang, L., Hawryluk, P., et al. (2004). Mechanisms of arsenic-induced prolongation of cardiac repolarization. Mol. Pharmacol. 66 (1), 33–44. doi:10.1124/mol.66.1.33
Food and Drug Administration (2005). ICH E14 the Clinical evaluation of QT/QTcinterval prolongation and proarrhythmic potential for non-antiarrhythmic drugs. Silver Spring, MD: Food and Drug Administration.
Fu, M., Li, R. X., Fan, L., He, G. W., Thornburg, K. L., and Wang, Z. (2008). Sarcoplasmic reticulum Ca2+ release channel ryanodine receptor (RyR2) plays a crucial role in aconitine-induced arrhythmias. Biochem. Pharmacol. 75 (11), 2147–2156. doi:10.1016/j.bcp.2008.02.027
Fu, M., Wu, M., Wang, J. F., Qiao, Y. J., and Wang, Z. (2007). Disruption of the intracellular Ca2+ homeostasis in the cardiac excitation-contraction coupling is a crucial mechanism of arrhythmic toxicity in aconitine-induced cardiomyocytes. Biochem. Biophys. Res. Commun. 354 (4), 929–936. doi:10.1016/j.bbrc.2007.01.082
Giambelluca, M. S., and Gende, O. A. (2008). Hydrogen peroxide activates calcium influx in human neutrophils. Mol. Cell Biochem. 309 (1-2), 151–156. doi:10.1007/s11010-007-9653-9
Groenendyk, J., Sreenivasaiah, P. K., Kim, D. H., Agellon, L. B., and Michalak, M. (2010). Biology of endoplasmic reticulum stress in the heart. Circ. Res. 107 (10), 1185–1197. doi:10.1161/CIRCRESAHA.110.227033
Guo, J., Bian, Y., Bai, R., Li, H., Fu, M., and Xiao, C. (2013). Globular adiponectin attenuates myocardial ischemia/reperfusion injury by upregulating endoplasmic reticulum Ca2+-ATPase activity and inhibiting endoplasmic reticulum stress. J. Cardiovasc. Pharmacol. 62 (2), 143–153. doi:10.1097/FJC.0b013e31829521af
Halkin, A., Roth, A., Lurie, I., Fish, R., Belhassen, B., and Viskin, S. (2001). Pause-dependent torsade de pointes following acute myocardial infarction: a variant of the acquired long QT syndrome. J. Am. Coll. Cardiol. 38 (4), 1168–1174. doi:10.1016/s0735-1097(01)01468-1
He, J., Wu, P., Dong, Y., and Gao, R. (2019). Adverse reactions analysis of Aconiti Lateralis Radix Praeparata and mechanism prediction of cardiac toxicity by network pharmacology. China J. Chin. Mater. Med. 44 (5), 1010–1018. doi:10.19540/j.cnki.cjcmm.20181205.003
He, J. L., Zhao, J. W., Ma, Z. C., Liang, Q. D., Wang, Y. G., Tan, H. L., et al. (2015). Cardiotoxicity study of Shenfu compatibility in rats based on metabonomics. Zhongguo Zhong Yao Za Zhi 40 (14), 2743–2747. doi:10.4268/cjcmm20151412
Holmström, K. M., and Finkel, T. (2014). Cellular mechanisms and physiological consequences of redox-dependent signalling. Nat. Rev. Mol. Cell Biol. 15 (6), 411–421. doi:10.1038/nrm3801
Horacek, J. M., Pudil, R., Jebavy, L., Tichy, M., Zak, P., and Maly, J. (2007). Assessment of anthracycline-induced cardiotoxicity with biochemical markers. Exp. Oncol. 29 (4), 309–313.
Hou, W., Huang, Z. X., Xu, H. G., Lin, J., Zhang, D. M., Peng, Q. L., et al. (2018). Hybrids of arenobufagin and benzoisoselenazol reducing the cardiotoxicity of arenobufagin. Bioorg. Med. Chem. Lett. 28 (20), 3391–3394. doi:10.1016/j.bmcl.2018.08.038
Hu, C. M., Cheng, Y. W., Liao, J. W., Cheng, H. W., and Kang, J. J. (2005). Induction of contracture and extracellular Ca2+ influx in cardiac muscle by sanguinarine: a study on cardiotoxicity of sanguinarine. J. Biomed. Sci. 12 (2), 399–407. doi:10.1007/s11373-005-3007-y
Hu, T. T., Tang, X., Wen, H., Xie, X., Peng, C., and Rao, C. (2018). Study the effects and mechanism of autophagy in H9C2 cardiomyocytes apoptosis induced by aconitine. J. Chin. Med. Mater. 41 (5), 1191–1196. doi:10.13863/j.issn1001-4454.2018.05.039
Huang, C. C., Chen, P. C., Huang, C. W., and Yu, J. (2007). Aristolochic Acid induces heart failure in zebrafish embryos that is mediated by inflammation. Toxicol. Sci. 100 (2), 486–494. doi:10.1093/toxsci/kfm235
Huang, G. Z., Li, L., and Liu, L. (2009). Pathological study on autopsy died of Tripterygium intoxication—report of 4 cases. Zhongguo Zhong Xi Yi Jie He Za Zhi 29 (2), 165–168.
Huang, J. W. (2019). The clinical value of Mb, cTnI and CK-MB in myocardial injury after neonatal asphyxia. J. Clin. Med. Liter 6 (98), 178. doi:10.16281/j.cnki.jocml.2019.98.110
Huang, Y. C., Yu, H. S., and Chai, C. Y. (2015). Roles of oxidative stress and the ERK1/2, PTEN and p70S6K signaling pathways in arsenite-induced autophagy. Toxicol. Lett. 239 (3), 172–181. doi:10.1016/j.toxlet.2015.09.022
Jones, R. L., and Miles, D. W. (2005). Use of endomyocardial biopsy to assess anthracycline-induced cardiotoxicity. Lancet Oncol. 6 (2), 67. doi:10.1016/S1470-2045(05)01719-5
Karturi, S. P., Gudmundsson, H., Akhtar, M., Jahangir, A., and Choudhuri, I. (2016). Spectrum of cardiac manifestations from aconitine poisoning. Heartrhythm Case Rep. 2 (5), 415–420. doi:10.1016/j.hrcr.2016.05.007
Kiss, T., Borcsa, B., Orvos, P., Tálosi, L., Hohmann, J., and Csupor, D. (2017). Diterpene lipo-alkaloids with selective activities on cardiac K+ channels. Planta Med. 83 (17), 1321–1328. doi:10.1055/s-0043-109556
Korkmaz, S., Zitron, E., Bangert, A., Seyler, C., Li, S., Hegedüs, P., et al. (2013). Provocation of an autoimmune response to cardiac voltage-gated sodium channel NaV1.5 induces cardiac conduction defects in rats. J. Am. Coll. Cardiol. 62 (4), 340–349. doi:10.1016/j.jacc.2013.04.041
Kostakis, C., and Byard, R. W. (2009). Sudden death associated with intravenous injection of toad extract. Forensic Sci. Int. 188 (1–3), e1–e5. doi:10.1016/j.forsciint.2009.02.006
Landstrom, A. P., Dobrev, D., and Wehrens, X. H. T. (2017). Calcium signaling and cardiac arrhythmias. Circ. Res. 120 (12), 1969–1993. doi:10.1161/CIRCRESAHA.117.310083
Li, A., Guo, X., Xie, J., Liu, X., Zhang, Z., Li, Y., et al. (2016a). Validation of biomarkers in cardiotoxicity induced by Periplocin on neonatal rat cardiomyocytes using UPLC-Q-TOF/MS combined with a support vector machine. J. Pharm. Biomed. Anal. 123, 179–185. doi:10.1016/j.jpba.2016.02.014
Li, B. X., Yang, B. F., Zhou, J., Xu, C. Q., and Li, Y. R. (2001). Inhibitory effects of berberine on Ik1, Ik, and HERG channels of cardiac myocytes. Acta Pharmacol. Sin 22 (2), 125–131.
Li, L., Sun, B., Zhang, Q., Fang, J., Ma, K., Li, Y., et al. (2008). Metabonomic study on the toxicity of Hei-Shun-Pian, the processed lateral root of Aconitum carmichaelii Debx. (Ranunculaceae). J. Ethnopharmacol. 116 (3), 561–568. doi:10.1016/j.jep.2008.01.014
Li, M., Xie, X., Chen, H., Xiong, Q., Tong, R., Peng, C., et al. (2020). Aconitine induces cardiotoxicity through regulation of calcium signaling pathway in zebrafish embryos and in H9c2 cells. J. Appl. Toxicol. 40 (6), 780–793. doi:10.1002/jat.3943
Li, S., Zhao, H., Wang, Y., Shao, Y., Wang, B., Wang, Y., et al. (2018b). Regulation of autophagy factors by oxidative stress and cardiac enzymes imbalance during arsenic or/and copper induced cardiotoxicity in Gallus gallus. Ecotoxicol Environ. Saf. 148, 125–134. doi:10.1016/j.ecoenv.2017.10.018
Li, S., Zhao, H. J., Wang, Y., Shao, Y., Liu, Y., and Xing, Y. (2018a). Arsenic-induced cardiotoxicity correlates with mitochondrial damage and trace elements imbalance in broiler chickens. Poult. Sci. 98 (2), 734–744. doi:10.3382/ps/pey469
Li, S. W., Sun, X., He, Y., Guo, Y., Zhao, H. J., Hou, Z. J., et al. (2017). Assessment of arsenic trioxide in the heart of Gallus gallus: alterations of oxidative damage parameters, inflammatory cytokines, and cardiac enzymes. Environ. Sci. Pollut. Res. Int. 24 (6), 5781–5790. doi:10.1007/s11356-016-8223-7
Li, X., Zhang, R., Zhao, B., Lossin, C., and Cao, Z. (2016b). Cardiotoxicity screening: a review of rapid-throughput in vitro approaches. Arch. Toxicol. 90 (8), 1803–1816. doi:10.1007/s00204-015-1651-1
Li, X. J., Jiang, Z. Z., and Zhang, L. Y. (2014). Triptolide: progress on research in pharmacodynamics and toxicology. J. Ethnopharmacol. 155 (1), 67–79. doi:10.1016/j.jep.2014.06.006
Li, Y., Ju, L., Hou, Z., Deng, H., Zhang, Z., Wang, L., et al. (2015). Screening, verification, and optimization of biomarkers for early prediction of cardiotoxicity based on metabolomics. J. Proteome Res. 14 (6), 2437–2445. doi:10.1021/pr501116c
Li, Y., Tu, D., Xiao, H., Du, Y., Zou, A., Liao, Y., et al. (2010). Aconitine blocks HERG and Kv1.5 potassium channels. J. Ethnopharmacol. 131 (1), 187–195. doi:10.1016/j.jep.2010.06.025
Li, Z. Y., Tan, P., Zhu, K. J., Li, F., and Sun, J. (2011). Effects of hypoaconitine on mRNA expression of sodium channel SCN5A and sodium-calcium exchange protein in myocardial cells. Guangdong Med. J. 32 (20), 2637–2639. doi:10.13820/j.cnki.gdyx.2011.20.006
Liang, X. P., Zhang, Z., Hu, P., and Zhong, H.-F. (2011). Metabonomics study on the acute toxicity of Toad Venom. Chem. J. Chin. Univ. 32 (01), 38–43.
Lin, C. C., Phua, D. H., Deng, J. F., and Yang, C. C. (2011). Aconitine intoxication mimicking acute myocardial infarction. Hum. Exp. Toxicol. 30 (7), 782–785. doi:10.1177/0960327110385960
Lin, J., Lin, S., Choy, P. C., Shen, X., Deng, C., Kuang, S., et al. (2008). The regulation of the cardiac potassium channel (HERG) by caveolin-1. Biochem. Cell Biol. 86 (5), 405–415. doi:10.1139/o08-118
Lin, Q., Wang, C., Jia, Z., Xiong, H., Xue, X., Liu, M., et al. (2020). UPLC-HDMS-based on serum metabolomics reveals the toxicity of arecae semen. J. Ethnopharmacol. 247, 112223. doi:10.1016/j.jep.2019.112223
Liu, C., Yang, J., Fu, W., Qi, S., Wang, C., Quan, C., et al. (2014). Coactivation of the PI3K/Akt and ERK signaling pathways in PCB153-induced NF-κB activation and caspase inhibition. Toxicol. Appl. Pharmacol. 277 (3), 270–278. doi:10.1016/j.taap.2014.03.027
Liu, C., Zhang, C., Wang, W., Yuan, F., He, T., Chen, Y., et al. (2019). Integrated metabolomics and network toxicology to reveal molecular mechanism of celastrol induced cardiotoxicity. Toxicol. Appl. Pharmacol. 383, 114785. doi:10.1016/j.taap.2019.114785
Liu, Y., Li, D., Nie, D., Liu, S. K., Qiu, F., Liu, M. T., et al. (2017). Arsenic trioxide and angiotensin II have inhibitory effects on HERG protein expression: evidence for the role of PML SUMOylation. Oncotarget 8 (28), 45447–45458. doi:10.18632/oncotarget.17563
Liu, Y. (2007). Study on the myocardial toxicity of Aconitum alkaloidsMaster. Shenyang, China: Shenyang Pharmaceutical University.
Lu, Y. C., and Chen, D. C. (2010). Clinical observation of paclitaxel-induced cardiotoxicity. Natl. Med. Front. China 5 (18), 49–50. doi:10.3969/j.issn.1673-5552.2010.18.0033
Luo, L., Li, B., Wang, S., Wu, F., Wang, X., Liang, P., et al. (2018). Centipedes subdue giant prey by blocking KCNQ channels. Proc. Natl. Acad. Sci. USA 115 (7), 1646–1651. doi:10.1073/pnas.1714760115
Ma, B. L., Ma, Y. M., Shi, R., Wang, T. M., Zhang, N., Wang, C. H., et al. (2010). Identification of the toxic constituents in rhizoma coptidis. J. Ethnopharmacol. 128 (2), 357–364. doi:10.1016/j.jep.2010.01.047
Ma, L. Q., Yu, Y., Chen, H., Li, M., Ihsan, A., Tong, H. Y., et al. (2018). Sweroside alleviated aconitine-induced cardiac toxicity in H9c2 cardiomyoblast cell line. Front. Pharmacol. 9, 1138. doi:10.3389/fphar.2018.01138
Maejima, H., Kinoshita, E., Seyama, I., and Yamaoka, K. (2003). Distinct sites regulating grayanotoxin binding and unbinding to D4S6 of Na(v)1.4 sodium channel as revealed by improved estimation of toxin sensitivity. J. Biol. Chem. 278 (11), 9464–9471. doi:10.1074/jbc.M212133200
Maisel, A. S., Duran, J. M., and Wettersten, N. (2018). Natriuretic peptides in heart failure: atrial and B-type natriuretic peptides. Heart Fail. Clin. 14 (1), 13–25. doi:10.1016/j.hfc.2017.08.002
Manna, P., Sinha, M., and Sil, P. C. (2008). Arsenic-induced oxidative myocardial injury: protective role of arjunolic acid. Arch. Toxicol. 82 (3), 137–149. doi:10.1007/s00204-007-0272-8
Mikaelian, I., Buness, A., de Vera-Mudry, M. C., Kanwal, C., Coluccio, D., Rasmussen, E., et al. (2010). Primary endothelial damage is the mechanism of cardiotoxicity of tubulin-binding drugs. Toxicol. Sci. 117 (1), 144–151. doi:10.1093/toxsci/kfq189
Mioulane, M., Foldes, G., Ali, N. N., Schneider, M. D., and Harding, S. E. (2012). Development of high content imaging methods for cell death detection in human pluripotent stem cell-derived cardiomyocytes. J. Cardiovasc. Transl Res. 5 (5), 593–604. doi:10.1007/s12265-012-9396-1
Müller, M. (2018). [Disturbances of calcium homeostasis]. Anasthesiol Intensivmed Notfallmed Schmerzther 53 (7-08), 516–528. doi:10.1055/s-0043-121655
Naik, S. D., and Freudenberger, R. S. (2004). Ephedra-associated cardiomyopathy. Ann. Pharmacother. 38 (3), 400–403. doi:10.1345/aph.1D408
Namdari, M., Eatemadi, A., and Negahdari, B. (2016). Natriuretic peptides and their therapeutic potential in heart failure treatment: an updated review. Cell Mol. Biol. (Noisy-le-grand) 62 (11), 1–7. doi:10.14715/cmb/2016.62.11.1
National Medical Products Administration (2019). National adverse drug reaction monitoring annual report. Chin. J. Drug Eval. 36, p476–480.
Newsholme, P., Cruzat, V. F., Keane, K. N., Carlessi, R., and de Bittencourt, P. I. (2016). Molecular mechanisms of ROS production and oxidative stress in diabetes. Biochem. J. 473 (24), 4527–4550. doi:10.1042/BCJ20160503C
Nie, J., Wang, F., Ji, T., Zhao, J., and Zhao, F. (2017). Assessment of in vitro cardiotoxicity of extract fractions and diterpene alkaloids from Aconitum leucostomum Worosch: a short communication. J. Pharm. Biomed. Anal. 137, 84–89. doi:10.1016/j.jpba.2017.01.003
Nouira, S., Elatrous, S., Besbes, L., Boukef, R., Devaux, C., Aubrey, N., et al. (2005). Neurohormonal activation in severe scorpion envenomation: correlation with hemodynamics and circulating toxin. Toxicol. Appl. Pharmacol. 208 (2), 111–116. doi:10.1016/j.taap.2005.01.017
Nyirimigabo, E., Xu, Y., Li, Y., Wang, Y., Agyemang, K., and Zhang, Y. (2015). A review on phytochemistry, pharmacology and toxicology studies of Aconitum. J. Pharm. Pharmacol. 67 (1), 1–19. doi:10.1111/jphp.12310
Nyska, A., Murphy, E., Foley, J. F., Collins, B. J., Petranka, J., Howden, R., et al. (2005). Acute hemorrhagic myocardial necrosis and sudden death of rats exposed to a combination of ephedrine and caffeine. Toxicol. Sci. 83 (2), 388–396. doi:10.1093/toxsci/kfi034
Odening, K. E., Jung, B. A., Lang, C. N., Cabrera Lozoya, R., Ziupa, D., Menza, M., et al. (2013). Spatial correlation of action potential duration and diastolic dysfunction in transgenic and drug-induced LQT2 rabbits. Heart Rhythm 10 (10), 1533–1541. doi:10.1016/j.hrthm.2013.07.038
Ortiz, E., and Possani, L. D. (2018). Scorpion toxins to unravel the conundrum of ion channel structure and functioning. Toxicon 150, 17–27. doi:10.1016/j.toxicon.2018.04.032
Pan, X., Zhou, J., Chen, Y., Xie, X., Rao, C., Liang, J., et al. (2020). Classification, hepatotoxic mechanisms, and targets of the risk ingredients in traditional Chinese medicine-induced liver injury. Toxicol. Lett. 323, 48–56. doi:10.1016/j.toxlet.2020.01.026
Panteghini, M. (2004). Role and importance of biochemical markers in clinical cardiology. Eur. Heart J. 25 (14), 1187–1196. doi:10.1016/j.ehj.2004.04.026
Papadimitriou, L., and Kalogeropoulos, A. P. (2015). Inflammatory biomarkers and therapeutic targets in heart failure. Curr. Med. Chem. 22 (23), 2716–2726. doi:10.2174/0929867322666150415152532
Peng, C. (2017). Thought and practice of multidimensional evaluation and integrated analysis on toxicity and efficacy of radix Aconiti lateralis praeparata. World Chin. Med. 12 (11), 2543–2550. doi:10.1016/j.jpba.2017.06.049
Peng, C., Wang, C. E., and Lin, N. (2008). The significance and practice of fundamental research on the correlation between toxicity and efficacy of toxic Chinese materia medica. Pharmacol. Clin. Chin. Mater. Med. 24 (1), 71–73. doi:10.13412/j.cnki.zyyl.2008.01.004
Peng, C., Xiao, X. H., Li, S., et al. (2017). Research progress and Frontier of integrated analysis of “toxicity” and “effect” of traditional Chinese medicine. Bull. Natl. Nat. Sci. Found China 31 (2), 176–183. doi:10.3389/fphar.2020.556885
Peng, F., Zhang, N., Wang, C., Wang, X., Huang, W., Peng, C., et al. (2020). Aconitine induces cardiomyocyte damage by mitigating BNIP3-dependent mitophagy and the TNFα-NLRP3 signalling axis. Cell Proliferat. 53 (1). doi:10.1111/cpr.12701
Poindexter, B. J., Feng, W., Dasgupta, A., and Bick, R. J. (2007). Oleandrin produces changes in intracellular calcium levels in isolated cardiomyocytes: a real-time fluorescence imaging study comparing adult to neonatal cardiomyocytes. J. Toxicol. Environ. Health Part A. 70 (6), 568–574. doi:10.1080/15287390600882408
Qi, Z. Z., Zhang, Y. L., Wang, S. L., Wang, C., Wang, X., Huang, Z., et al. (2014). Research on biomarkers in rats with cardiac inury induced by isoprenaline. J. Biomed. Eng. Res. 33 (4), 240–244. doi:10.19529/j.cnki.1672-6278.2014.04.008
Quintero-Hernandez, V., Jiménez-Vargas, J. M., Gurrola, G. B., Valdivia, H. H., and Possani, L. D. (2013). Scorpion venom components that affect ion-channels function. Toxicon 76, 328–342. doi:10.1016/j.toxicon.2013.07.012
Ramirez-Ortega, M., Zarco, G., Maldonado, V., Carrillo, J. F., Ramos, P., Ceballos, G., et al. (2007). Is digitalis compound-induced cardiotoxicity, mediated through guinea-pig cardiomyocytes apoptosis?. Eur. J. Pharmacol. 566 (1–3), 34–42. doi:10.1016/j.ejphar.2007.03.033
Rao, R. P., Yuan, C., Allegood, J. C., Rawat, S. S., Edwards, M. B., Wang, X., et al. (2007). Ceramide transfer protein function is essential for normal oxidative stress response and lifespan. Proc. Natl. Acad. Sci. USA 104 (27), 11364–11369. doi:10.1073/pnas.0705049104
Rao, S., and Sikdar, S. K. (2000). Modification of alpha subunit of RIIA sodium channels by aconitine. Pflugers Arch. 439 (3), 349–355. doi:10.1007/s004249900121
Ren, S. J., Xu, H. H., Li, M., and Hao, F.-R. (2017). Cytotoxicity of ophiopogonin D′ for rat H9c2 cardiomyocytes. Chin. J. Pharmacol. Toxicol. 31 (4), 325–331. doi:10.1002/jbt.21917
Reuter, S. E., and Evans, A. M. (2012). Carnitine and acylcarnitines: pharmacokinetic, pharmacological and clinical aspects. Clin. Pharmacokinet. 51 (9), 553–572. doi:10.2165/11633940-000000000-00000
Rodriguez-Menchaca, A., Ferrer-Villada, T., Lara, J., Fernandez, D., Navarro-Polanco, R. A., and Sanchez-Chapula, J. A. (2006). Block of HERG channels by berberine: mechanisms of voltage- and state-dependence probed with site-directed mutant channels. J. Cardiovasc. Pharmacol. 47 (1), 21–29. doi:10.1097/01.fjc.0000191564.52242.00
Roger, R. J., Bick, B. J., Sweney, R. R., and Dasgupta, A. 2002). Effects of Chan Su, a traditional Chinese medicine, on the calcium transients of isolated cardiomyocytes: cardiotoxicity due to more than Na, K-ATPase blocking. Life Sci. 72 (6), 699–709. doi:10.1016/s0024-3205(02)02302-0
Samenuk, D., Link, M. S., Homoud, M. K., Contreras, R., Theoharides, T. C., Wang, P. J., et al. (2002). Adverse cardiovascular events temporally associated with ma huang, an herbal source of ephedrine. Mayo Clin. Proc. 77 (1), 12–16. doi:10.4065/77.1.12
Scherz-Shouval, R., Shvets, E., Fass, E., Shorer, H., Gil, L., and Elazar, Z. (2007). Reactive oxygen species are essential for autophagy and specifically regulate the activity of Atg4. EMBO J. 26 (7), 1749–1760. doi:10.1038/sj.emboj.7601623
Scott, G. I., Colligan, P. B., Ren, B. H., and Ren, J. (2001). Ginsenosides Rb1 and Re decrease cardiac contraction in adult rat ventricular myocytes: role of nitric oxide. Br. J. Pharmacol. 134, 1159–1165. doi:10.1038/sj.bjp.0704377
Scriven, D. R., Dan, P., and Moore, E. D. (2000). Distribution of proteins implicated in excitation-contraction coupling in rat ventricular myocytes. Biophys. J. 79 (5), 2682–2691. doi:10.1016/S0006-3495(00)76506-4
Sfaxi, I., Charradi, K., Limam, F., El May, M. V., and Aouani, E. (2016). Grape seed and skin extract protects against arsenic trioxide induced oxidative stress in rat heart. Can. J. Physiol. Pharmacol. 94 (2), 168–176. doi:10.1139/cjpp-2015-0088
Shan, H., Zhang, Y., Cai, B., Chen, X., Fan, Y., Yang, L., et al. (2013). Upregulation of microRNA-1 and microRNA-133 contributes to arsenic-induced cardiac electrical remodeling. Int. J. Cardiol. 167 (6), 2798–2805. doi:10.1016/j.ijcard.2012.07.009
Shi, J., Cao, B., Wang, X. W., Aa, J. Y., Duan, J. A., Zhu, X. X., et al. (2016). Metabolomics and its application to the evaluation of the efficacy and toxicity of traditional Chinese herb medicines. J. Chromatogr. B Analyt Technol. Biomed. Life Sci. 1026, 204–216. doi:10.1016/j.jchromb.2015.10.014
Shi, X., Verma, S., Yun, J., Brand-Arzamendi, K., Singh, K. K., Liu, X., et al. (2017). Effect of empagliflozin on cardiac biomarkers in a zebrafish model of heart failure: clues to the EMPA-REG OUTCOME trial? Mol. Cell Biochem. 433 (1-2), 97–102. doi:10.1007/s11010-017-3018-9
Sigalas, C., Bent, S., Kitmitto, A., O'Neill, S., and Sitsapesan, R. (2009a). Ca2+-calmodulin can activate and inactivate cardiac ryanodine receptors. Br. J. Pharmacol. 156 (5), 794–806. doi:10.1111/j.1476-5381.2008.00092.x
Sigalas, C., Mayo-Martin, M. B., Jane, D. E., and Sitsapesan, R. (2009b). Ca2+-calmodulin increases RyR2 open probability yet reduces ryanoid association with RyR2. Biophys. J. 97 (7), 1907–1916. doi:10.1016/j.bpj.2009.07.027
Skála, M., Hanousková, B., Skálová, L., and Matoušková, P. (2019a). MicroRNAs in the diagnosis and prevention of drug-induced cardiotoxicity. Arch. Toxicol. 93 (1), 1–9. doi:10.1007/s00204-018-2356-z
Su, T., Tan, Y., Tsui, M. S., Yi, H., Fu, X. Q., Li, T., et al. (2016). Metabolomics reveals the mechanisms for the cardiotoxicity of Pinelliae Rhizoma and the toxicity-reducing effect of processing. Sci. Rep. 6, 34692. doi:10.1038/srep34692
Sun, B., Li, L., Wu, S., Zhang, Q., Li, H., Chen, H., et al. (2009). Metabolomic analysis of biofluids from rats treated with Aconitum alkaloids using nuclear magnetic resonance and gas chromatography/time-of-flight mass spectrometry. Anal. Biochem. 395 (2), 125–133. doi:10.1016/j.ab.2009.08.014
Sun, G. B., Sun, H., Meng, X. B., Hu, J., Zhang, Q., Liu, B., et al. (2014). Aconitine-induced Ca2+ overload causes arrhythmia and triggers apoptosis through p38 MAPK signaling pathway in rats. Toxicol. Appl. Pharmacol. 279 (1), 8–22. doi:10.1016/j.taap.2014.05.005
Sun, H., Liu, X., Xiong, Q., Shikano, S., and Li, M. (2006). Chronic inhibition of cardiac Kir2.1 and HERG potassium channels by celastrol with dual effects on both ion conductivity and protein trafficking. J. Biol. Chem. 281 (9), 5877–5884. doi:10.1074/jbc.M600072200
Sun, P., Wu, F., Wen, M., Yang, X., Wang, C., Li, Y., et al. (2015). A distinct three-helix centipede toxin SSD609 inhibits I(ks) channels by interacting with the KCNE1 auxiliary subunit. Sci. Rep. 5, 13399. doi:10.1038/srep13399
Tak, S., Lakhotia, M., Gupta, A., Sagar, A., Bohra, G., and Bajari, R. (2016). Aconite poisoning with arrhythmia and shock. Indian Heart J. 68 (Suppl. 2), S207–S209. doi:10.1016/j.ihj.2015.08.010
Titov, D. V., Gilman, B., He, Q. L., Bhat, S., Low, W. K., Dang, Y., et al. (2011). XPB, a subunit of TFIIH, is a target of the natural product triptolide. Nat. Chem. Biol. 7 (3), 182–188. doi:10.1038/nchembio.522
Tochinai, R., Suzuki, K., Nagata, Y., Ando, M., Hata, C., Komatsu, K., et al. (2014). Cardiotoxic changes of colchicine intoxication in rats: electrocardiographic, histopathological and blood chemical analysis. J. Toxicol. Pathol. 27 (3-4), 223–230. doi:10.1293/tox.2014-0013
Varghese, M. V., Abhilash, M., Alex, M., Paul, M. V. S., Prathapan, A., Raghu, K. G., et al. (2017). Attenuation of arsenic trioxide induced cardiotoxicity through flaxseed oil in experimental rats. Redox Rep. 22 (6), 346–352. doi:10.1080/13510002.2017.1289313
Vineetha, R. C., Binu, P., Arathi, P., and Nair, R. H. (2018). L-ascorbic acid and α-tocopherol attenuate arsenic trioxide-induced toxicity in H9c2 cardiomyocytes by the activation of Nrf2 and Bcl2 transcription factors. Toxicol. Mech. Methods 28 (5), 353–360. doi:10.1080/15376516.2017.1422578
Vineetha, V. P., Soumya, R. S., and Raghu, K. G. (2015). Phloretin ameliorates arsenic trioxide induced mitochondrial dysfunction in H9c2 cardiomyoblasts mediated via alterations in membrane permeability and ETC complexes. Eur. J. Pharmacol. 754, 162–172. doi:10.1016/j.ejphar.2015.02.036
Wang, H. Y., Zuo, A. X., Sun, Y., and Rao, G. X. (2014a). Chemical constituents from aerial part of Aconitum brachypodum. Zhong Yao Cai 37 (8), 1391–1395. doi:10.13863/j.issn1001-4454.2014.08.021
Wang, J., Wang, N. N., Ge, Y. X., Tan, H.-L., Ma, Z.-C., Wang, Y.-G., et al. (2019a). Ophiopogonin D protects cardiomyocytes against ophiopogonin D′-induced injury through suppressing endoplasmic reticulum stress. China J. Chin. Mater. Med. 44 (9), 1876–1881. doi:10.19540/j.cnki.cjcmm.20190102.003
Wang, M., Sun, G. B., Sun, X., Wang, H. W., Meng, X. B., Qin, M., et al. (2013). Cardioprotective effect of salvianolic acid B against arsenic trioxide-induced injury in cardiac H9c2 cells via the PI3K/Akt signal pathway. Toxicol. Lett. 216 (2-3), 100–107. doi:10.1016/j.toxlet.2012.11.023
Wang, R., Wang, M., Wang, S., Yang, K., Zhou, P., Xie, X., et al. (2019b). An integrated characterization of contractile, electrophysiological, and structural cardiotoxicity of Sophora tonkinensis Gapnep. in human pluripotent stem cell-derived cardiomyocytes. Stem Cell Res. Ther. 10 (1), 20. doi:10.1186/s13287-018-1126-4
Wang, S. F., Liu, K. C., Wang, X. M., He, Q. X., Han, L. W., and Hou, H. R. (2009). Preliminary study on cardiotoxicity of celastrol to zebrafish embryo. Chin. Pharmacol. Bull. 25 (5), 634–636.
Wang, S. R., Chen, X., Ling, S., Ni, R. Z., Guo, H., and Xu, J. W. (2019c). MicroRNA expression, targeting, release dynamics and early-warning biomarkers in acute cardiotoxicity induced by triptolide in rats. Biomed. Pharmacother. 111, 1467–1477. doi:10.1016/j.biopha.2018.12.109
Wang, S., Chen, X., Ling, S., Guo, H., Ni, R., Dang, Y., et al. (2016a). The preliminary study of biomarkers in plasma caused by Tripterygium and aconite's acute toxicity. Lishizhen Med. Mater. Med. Res. 27 (3), 759–761. doi:10.3969/j.issn.1008-0805.2016.03.093
Wang, S. Y., Tikhonov, D. B., Mitchell, J., Zhorov, B. S., and Wang, G. K. (2007). Irreversible block of cardiac mutant Na+ channels by batrachotoxin. Channels (Austin) 1 (3), 179–188. doi:10.4161/chan.4437
Wang, T., Chen, X., Yu, J., Du, Q., Zhu, J., Yang, M., et al. (2018). High-throughput electrophysiology screen revealed cardiotoxicity of strychnine by selectively targeting hERG channel. Am. J. Chin. Med. 46 (8), 1825–1840. doi:10.1142/s0192415x1850091x
Wang, W., Yang, Y., Xiong, Z., Kong, J., Fu, X., Shen, F., et al. (2016b). Inhibition of glycogen synthase kinase 3beta ameliorates triptolide-induced acute cardiac injury by desensitizing mitochondrial permeability transition. Toxicol. Appl. Pharmacol. 313, 195–203. doi:10.1016/j.taap.2016.10.007
Wang, X., Wang, H., Zhang, A., Lu, X., Sun, H., Dong, H., et al. (2012). Metabolomics study on the toxicity of aconite root and its processed products using ultraperformance liquid-chromatography/electrospray-ionization synapt high-definition mass spectrometry coupled with pattern recognition approach and ingenuity pathways analysis. J. Proteome Res. 11 (2), 1284–1301. doi:10.1021/pr200963e
Wang, Y., Guo, Z., and Huang, L. (2014b). Value of different biochemical markers in early diagnosis of acute myocardial infarction. Nan Fang Yi Ke Da Xue Xue Bao 34 (9), 1347–1350.
Wang, Y. J., Chen, B. S., Lin, M. W., Lin, A. A., Peng, H., Sung, R. J., et al. (2008). Time-dependent block of ultrarapid-delayed rectifier K+ currents by aconitine, a potent cardiotoxin, in heart-derived H9c2 myoblasts and in neonatal rat ventricular myocytes. Toxicol. Sci. 106 (2), 454–463. doi:10.1093/toxsci/kfn189
Wang, Z. G., and Ren, J. (2002). Current status and future direction of Chinese herbal medicine. Trends Pharmacol. Sci. 23, 347–348. doi:10.1016/s0165-6147(02)02051-5
Watanabe, M., Funakoshi, T., Unuma, K., Aki, T., and Uemura, K. (2014). Activation of the ubiquitin-proteasome system against arsenic trioxide cardiotoxicity involves ubiquitin ligase Parkin for mitochondrial homeostasis. Toxicology 322, 43–50. doi:10.1016/j.tox.2014.04.008
Webster, K. A. (2012). Mitochondrial membrane permeabilization and cell death during myocardial infarction: roles of calcium and reactive oxygen species. Future Cardiol. 8 (6), 863–884. doi:10.2217/fca.12.58
Wright, S. N. (2001). Irreversible block of human heart (hH1) sodium channels by the plant alkaloid lappaconitine. Mol. Pharmacol. 59 (2), 183–192. doi:10.1124/mol.59.2.183
Wu, J., Wang, X., Chung, Y. Y., Koh, C. H., Liu, Z., Guo, H., et al. (2017). L-type calcium channel inhibition contributes to the proarrhythmic effects of aconitine in human cardiomyocytes. PLoS One 12 (1), e0168435. doi:10.1371/journal.pone.0168435
Xi, Y., Wang, W., Wang, L., Pan, J., Cheng, Y., Shen, F., et al. (2018). Triptolide induces p53-dependent cardiotoxicity through mitochondrial membrane permeabilization in cardiomyocytes. Toxicol. Appl. Pharmacol. 355, 269–285. doi:10.1016/j.taap.2018.07.011
Xi, Y., Zhang, Y., Pan, J., Chen, S., Lu, S., Shen, F., et al. (2020). Triptolide dysregulates glucose uptake via inhibition of IKKβ-NF-κB pathway by p53 activation in cardiomyocytes. Toxicol. Lett. 318, 1–11. doi:10.1016/j.toxlet.2019.10.001
Xie, J. T., Maleckar, S. A., and Yuan, C. S. (2002). Resibufogenin, a single compound isolated from toad venom, can induce cardiac toxicity. Herbals Complement. Med. 3 (1), 24–28.
Xie, S., Jia, Y., Liu, A., Dai, R., and Huang, L. (2015). Hypaconitine-induced QT prolongation mediated through inhibition of KCNH2 (hERG) potassium channels in conscious dogs. J. Ethnopharmacol. 166, 375–379. doi:10.1016/j.jep.2015.03.023
Xu, C. B., Wang, L., Hui, X. J., Ma, X. P., Bi, X., and Zhao, Q. (2014). Genetic toxicity of berberine on mouse heart. Amr 884-885, 634–637. doi:10.4028/www.scientific.net/amr.884-885.634
Xu, C. Q., Brône, B., Wicher, D., Bozkurt, O., Lu, W. Y., Huys, I., et al. (2004a). BmBKTx1, a novel Ca2+-activated K+ channel blocker purified from the Asian scorpion Buthus martensi Karsch. J. Biol. Chem. 279 (33), 34562–34569. doi:10.1074/jbc.M312798200
Xu, C. Q., He, L. L., Brône, B., Martin-Eauclaire, M. F., Van Kerkhove, E., Zhou, Z., et al. (2004b). A novel scorpion toxin blocking small conductance Ca2+ activated K+ channel. Toxicon 43 (8), 961–971. doi:10.1016/j.toxicon.2004.01.018
Yan, M., Feng, L., Shi, Y., Wang, J., Liu, Y., Li, F., et al. (2017). Mechanism of As2O3-induced action potential prolongation and using hiPS-CMs to evaluate the rescue efficacy of drugs with different rescue mechanism. Toxicol. Sci. 158 (2), 379–390. doi:10.1093/toxsci/kfx098
Yan, M., Zhang, K., Shi, Y., Feng, L., Lv, L., and Li, B. (2015). Mechanism and pharmacological rescue of berberine-induced hERG channel deficiency. Drug Des. Devel Ther. 9, 5737–5747. doi:10.2147/DDDT.S91561
Yang, A. W., Fan, X. M., Li, X., Liang, Q. L., Wang, Y. M., and Luo, G. A. (2011a). Acute toxicity of Venenum Bufonis and compatibility of heart musk protecting pills by microarray expression analysis. Chem. J. Chin. Univ. 32 (5), 1058–1064.
Yang, W., Ma, L., Li, S., Cui, K., Lei, L., and Ye, Z. (2017). Evaluation of the cardiotoxicity of evodiamine in vitro and in vivo. Molecules 22 (6), 943. doi:10.3390/molecules22060943
Yang, Y., Wang, W., Xiong, Z., Kong, J., Wang, L., Qiu, Y., et al. (2016). Resveratrol protects against triptolide-induced cardiotoxicity through SIRT3 signaling pathway in vivo and in vitro. Pharmazie 71 (9), 514–523. doi:10.1691/ph.2016.6597
Yang, Y. X., Tao, M., Ma, J. H., Dong, X. P., and Tan, Y. Z. (2011b). Advances in studies on diterpenoids toxic compounds from rhododendrons. Asia Pac. Tradi Med. 7 (7), 168–170.
Yao, J., Chen, X., Li, H., Zhou, Y., Yao, L., Wu, G., et al. (2005). BmP09, a “long chain” scorpion peptide blocker of BK channels. J. Biol. Chem. 280 (15), 14819–14828. doi:10.1074/jbc.M412735200
Yao, Y. X. (2013). “Cirulating miRs provide dynamic evaluation of myocardial injury and early warning for perioperative myocardial infarction,” Doctor, Chinese academy of medical sciences. Beijing, China: Peking Union Medical College.
Yi, M., Peng, W., Chen, X., Wang, J., and Chen, Y. (2012). Effect of hypaconitine combined with liquiritin on the expression of calmodulin and connexin43 in rat cardiac muscle in vivo. J. Pharm. Pharmacol. 64 (11), 1654–1658. doi:10.1111/j.2042-7158.2012.01532.x
You, J. E. (2016). Carditotoxicity study of Asarum in SD rats on pharmacology and NMR metabolomics. Master thesis. Wuhan, China: Hubei University of Chinese Medicine.
Yuan, C., Luo, Z., Zhou, Y., Lei, S., Xu, C., Peng, C., et al. (2018). Removal of hERG potassium channel affinity through introduction of an oxygen atom: molecular insights from structure-activity relationships of strychnine and its analogs. Toxicol. Appl. Pharmacol. 360, 109–119. doi:10.1016/j.taap.2018.09.042
Zhang, J. Y., Sun, G. B., Wang, M., Liao, P., Du, Y. Y., Yang, K., et al. (2016a). Arsenic trioxide triggered calcium homeostasis imbalance and induced endoplasmic reticulum stress-mediated apoptosis in adult rat ventricular myocytes. Toxicol. Res. (Camb) 5 (2), 682–688. doi:10.1039/c5tx00463b
Zhang, J. Y., Wang, M., Wang, R. Y., Sun, X., Du, Y. Y., Ye, J. X., et al. (2018a). Salvianolic acid A ameliorates arsenic trioxide-induced cardiotoxicity through decreasing cardiac mitochondrial injury and promotes its anticancer activity. Front. Pharmacol. 9, 487. doi:10.3389/fphar.2018.00487
Zhang, J. Y., Zhang, B., Wang, M., Wang, W., Liao, P., Sun, G. B., et al. (2017). Calcium homeostasis and endoplasmic reticulum stress are involved in Salvianolic acid B-offered protection against cardiac toxicity of arsenic trioxide. Oncotarget 8 (57), 97384–97393. doi:10.18632/oncotarget.22127
Zhang, K., Zhi, D., Huang, T., Gong, Y., Yan, M., Liu, C., et al. (2014). Berberine induces hERG channel deficiency through trafficking inhibition. Cell Physiol. Biochem. 34 (3), 691–702. doi:10.1159/000363034
Zhang, M. Y., Yu, Y. Y., Wang, S. F., Zhang, Q., Wu, H. W., Wei, J. Y., et al. (2018b). Cardiotoxicity evaluation of nine alkaloids from Rhizoma Coptis. Hum. Exp. Toxicol. 37 (2), 185–195. doi:10.1177/0960327117695633
Zhang, W., Guo, C., Gao, R., Ge, M., Zhu, Y., and Zhang, Z. (2013a). The protective role of resveratrol against arsenic trioxide-induced cardiotoxicity. Evid. Based Complement. Alternat Med. 2013, 407839. doi:10.1155/2013/407839
Zhang, X., Zhao, B., Dong, Y., Dai, L., Hu, T., Xie, X., et al. (2016b). Effects of compatibility of ginsenosides Rb1 and aconitine on ATP enzyme and related ion of myocardial cells in newborn rats. Chin. J. Exper Tradit Med. Formulae 22 (7), 112–115. doi:10.13422/j.cnki.syfjx.2016070112
Zhang, Y., Dong, Z., Jin, L., Zhang, K., Zhao, X., Fu, J., et al. (2013b). Arsenic trioxide-induced hERG K+ channel deficiency can be rescued by matrine and oxymatrine through up-regulating transcription factor Sp1 expression. Biochem. Pharmacol. 85 (1), 59–68. doi:10.1016/j.bcp.2012.09.002
Zhang, Z. H., Zhao, Y. Y., Cheng, X. L., Dai, Z., Zhou, C., Bai, X., et al. (2013c). General toxicity of Pinellia ternata (Thunb.) Berit. in rat: a metabonomic method for profiling of serum metabolic changes. J. Ethnopharmacol 149 (1), 303–310. doi:10.1016/j.jep.2013.06.039
Zhao, X., Shi, Y. Q., Yan, C. C., Feng, P. F., Wang, X., Zhang, R., et al. (2015). Up-regulation of miR-21 and miR-23a Contributes to As2 O3 -induced hERG Channel Deficiency. Basic Clin. Pharmacol. Toxicol. 116 (6), 516–523. doi:10.1111/bcpt.12348
Zheng, Q., and Bao, Y. M. (2017). Regulatory effects of traditional Chinese medicine on autophagy in myocardial ischemia reperfusion injury. Zhongguo Zhong Yao Za Zhi 42 (15), 2925–2929. doi:10.19540/j.cnki.cjcmm.20170714.010
Zhou, C., Yu, F., Zeng, P., Zhang, T., Huang, H., Chen, W., et al. (2019). Circadian sensitivity to the cardiac glycoside oleandrin is associated with diurnal intestinal P-glycoprotein expression. Biochem. Pharmacol. 169, 113622. doi:10.1016/j.bcp.2019.08.024
Zhou, J., Xi, C., Wang, W., Fu, X., Jinqiang, L., Qiu, Y., et al. (2014). Triptolide-induced oxidative stress involved with Nrf2 contribute to cardiomyocyte apoptosis through mitochondrial dependent pathways. Toxicol. Lett. 230 (3), 454–466. doi:10.1016/j.toxlet.2014.08.017
Zhou, J., Xi, C., Wang, W., Yang, Y., Qiu, Y., and Huang, Z. (2015). Autophagy plays an important role in triptolide-induced apoptosis in cardiomyocytes. Toxicol. Lett. 236 (3), 168–183. doi:10.1016/j.toxlet.2015.05.013
Zhou, W., Qiu, L.-z., Liu, H., Deng, H.-F., Yue, L.-X., and Gao, Y. (2020). Notch1-mediated histone demethylation of HCN4 contributes to aconitine-induced ventricular myocardial dysrhythmia. Toxicol. Lett. 327, 19–31. doi:10.1016/j.toxlet.2020.03.017
Keywords: Chinese materia medica, cardiotoxicity, risk compounds, preclinical toxicity evaluation, potential mechanisms
Citation: Zhou J, Peng F, Cao X, Xie X, Chen D, Yang L, Rao C, Peng C and Pan X (2021) Risk Compounds, Preclinical Toxicity Evaluation, and Potential Mechanisms of Chinese Materia Medica–Induced Cardiotoxicity. Front. Pharmacol. 12:578796. doi: 10.3389/fphar.2021.578796
Received: 01 July 2020; Accepted: 29 January 2021;
Published: 30 March 2021.
Edited by:
Emilio Benfenati, Istituto di Ricerche Farmacologiche Mario Negri (IRCCS), ItalyReviewed by:
Jun Ren, University of Washington, United StatesFang Ye, Huazhong University of Science and Technology, China
Copyright © 2021 Zhou, Peng, Cao, Xie, Chen, Yang, Rao, Peng and Pan. This is an open-access article distributed under the terms of the Creative Commons Attribution License (CC BY). The use, distribution or reproduction in other forums is permitted, provided the original author(s) and the copyright owner(s) are credited and that the original publication in this journal is cited, in accordance with accepted academic practice. No use, distribution or reproduction is permitted which does not comply with these terms.
*Correspondence: Cheng Peng, cGVuZ2NoZW5nY2hlbmdkdUAxMjYuY29t; Xiaoqi Pan, cGFueGlhb3FpMTEwNEBjZHV0Y20uZWR1LmNu
†These authors have contributed equally to this work