- 1School of Pharmacy, Minzu University of China, Beijing, China
- 2Key Laboratory of Ethnomedicine (Minzu University of China), Minority of Education, Beijing, China
- 3Medical College of Qingdao Binhai University, Affiliated Hospital of Qingdao Binhai University, Qingdao, China
Marsdeniae tenacissimae Caulis is a traditional Chinese medicine, named Tongguanteng (TGT), that is often used for the adjuvant treatment of cancer. In our previous study, we reported that an ethyl acetate extract of TGT had inhibitory effects against adenocarcinoma A549 cells growth. To identify the components of TGT with anti-tumor activity and to elucidate their underlying mechanisms of action, we developed a technique for isolating compounds, which was then followed by cytotoxicity screening, network pharmacology analysis, and cellular and molecular experiments. We isolated a total of 19 compounds from a TGT ethyl acetate extract. Two novel steroidal saponins were assessed using an ultra-performance liquid chromatography-photodiode array coupled with quadrupole time-of-flight mass (UPLC-ESI-Q/TOF-MS). Then, we screened these constituents for anti-cancer activity against non-small cell lung cancer (NSCLC) in vitro and obtained six target compounds. Furthermore, a compound-target-pathway network of these six bioactive ingredients was constructed to elucidate the potential pathways that controlled anticancer effects. Approximately 205 putative targets that were associated with TGT, as well as 270 putative targets that were related to NSCLC, were obtained from online databases and target prediction software. Protein–protein interaction networks for drugs as well as disease putative targets were generated, and 18 candidate targets were detected based on topological features. In addition, pathway enrichment analysis was performed to identify related pathways, including PI3K/AKT, VEGF, and EGFR tyrosine kinase inhibitor resistance, which are all related to metabolic processes and intrinsic apoptotic pathways involving reactive oxygen species (ROS). Then, various cellular experiments were conducted to validate drug-target mechanisms that had been predicted using network pharmacology analysis. The experimental results showed the four C21 steroidal saponins could upregulate Bax and downregulate Bcl-2 expression, thereby changing the mitochondrial membrane potential, producing ROS, and releasing cytochrome C, which finally activated caspase-3, caspase-9, and caspase-8, all of which induced apoptosis in A549 cells. In addition, these components also downregulated the expression of MMP-2 and MMP-9 proteins, further weakening their degradation of extracellular matrix components and type IV collagen, and inhibiting the migration and invasion of A549 cells. Our study elucidated the chemical composition and underlying anti-tumor mechanism of TGT, which may be utilized in the treatment of lung cancer.
Introduction
Lung cancer severely affects human health and survival and has become the main cause of cancer-related deaths over the past several years (Gu et al., 2018). An estimated 1.6 million individuals have received a diagnosis of lung cancer, resulting in more than 1.3 million deaths worldwide in the past decade (Torre et al., 2015). In 2017, the US pointed out that approximately 85–90% of lung cancer cases were non-small cell lung cancer (NSCLC) (DeSantis et al., 2016; Paci et al., 2017). Over the past 10 years, various major advances in cancer research have uncovered the genetics and pathologies of NSCLC, facilitating the development of novel anticancer drugs (Hare et al., 2017). Interestingly, multiple bioactive ingredients obtained from Chinese herbal medicine have been considered potential candidates for the treatment of cancer (Tang et al., 2009; Gao et al., 2020).
Marsdeniae tenacissimae Caulis is the dried lianoid stem of Marsdenia tenacissima (Roxb.) Moon (Fam. Asclepiadaceae), known as “Tong-guang-teng” or “Tong-guang-san”, recorded in the 2009 edition of “Standards of Traditional Chinese Medicines in Hunan Province” and the 2020 edition of “Pharmacopeia of the People’s Republic of China”, and it can suppress cough, relieve wheezing, dispel phlegm, unblock lac feminium, clear heat, and remove toxins (Commission, C. P., 2020). The medicinal use of this plant can be traced back to the Ming Dynasty and was primarily recorded in “Dian Nan Ben Cao” by Mao Lan (1397–1470) (Wang et al., 2018). Extensive evidence indicates that C21 steroid glycosides, extracted by ethyl acetate from TGT, have a significant inhibitory effect against different cancer cell lines, such as A549, Caco-2, SACC83, PC-3, K562, and HepG2 (Ye et al., 2014; Wang et al., 2015). We found that the four C21 steroidal glycosides isolated from TGT had a higher rate of inhibition of A549 cells proliferation than other cell lines (Xu, 2018; Hu et al., 2020). To elucidate the relationship between the chemical structure and cytotoxic activities of steroidal glycosides and to investigate the anti-cancer mechanism of TGT, we isolated and characterized novel compounds from this medicinal plant.
Over the past decade, network-based pharmacological analyses have been employed to assess the mechanisms of herbs and formulae as well as their potential bioactive components at both the molecular and systemic levels (Fang et al., 2017). In particular, network pharmacology has been utilized by Chinese medicine researchers in order to predict the interactions between various components and targets (Mao et al., 2017; Zhang et al., 2019). Furthermore, network pharmacology is also a useful in silico prediction tool for identifying active components and elucidating the mechanisms of herbal medicines that, in turn, allows more investigations of these bioactive compounds.
This study developed an approach that integrated cytotoxicity screening, phytochemical analysis, cellular and molecular biology, and network pharmacology construction to identify effective antitumor substances and the underlying mechanisms of TGT. To our knowledge, this is the first integral study that employed several methods to identify efficacious antitumor substances and elucidated their mechanisms of action.
Materials and Methods
Chemicals and Materials
The dry cane of TGT was procured from Huayu Pharmaceutical Co., Ltd. (Guangzhou, Guangdong, China). Reference standards of TGT and marsdenoside H (purity >98%) (purity were obtained from the National Institutes for Food and Drug Control (Beijing, China). Four C21 steroidal glycosides, 11α-O-benzoyl-12β-O-tigloyltenacigenin B (TGT-15), marsdenoside C (TGT-7), 11α-O-tigloyl-12β-O-benzoyltenacigenin B (TGT-9), and 11α-O-2-methylbutyryl-l2β-O-benzoyltenacigenin B (TGT-13), were isolated from an ethyl acetate extract of TGT in our laboratory, and their purities were all >98% based on HPLC normalization and silica gel TLC analysis (Xu, 2018). Dulbecco’s modified Eagle’s medium (DMEM) was obtained from Gibco Invitrogen (Carlsbad, CA, United States). Fetal bovine serum (FBS) was obtained from Hyclone, Co. (Fremont, CA, United States). A549 cells were obtained from the National Infrastructure of Cell Line Resource (Beijing, China). Annexin-FITC cell apoptosis assay and cell cycle assay kits were obtained from Sanjian Biotechnology Co. (Tianjin, China). Reactive oxygen species and mitochondrial membrane potential assay kits were obtained from Boster Biological Technology (Wuhan, Hubei, China). Primary antibodies against MMP-2, cleaved caspase-3, Bcl-2, Bax, cytochrome C, and GAPDH were obtained from Abcam (Cambridge, United Kingdom). MMP-9, cleaved caspase-9, and cleaved caspase-8 were obtained from Shanghai Rebiosci Biotechnology Co. (Shanghai, China), and β-actin was obtained from Sigma–Aldrich (St. Louis, MO, United States). A CX-21 Ordinary Optical Microscope was obtained from Olympus (Shanghai, China). A DR-200Bs ELISA instrument was obtained from Wuxi Hiwell Diatek Instruments Co., Ltd (Wuxi, Jiangsu, China). A FACSCalibur flow cytometer was obtained from Becton, Dickinson and Company (BD, United States).
Marsdeniae tenacissimae Extract Preparation
The dry cane of Marsdeniae tenacissimae (5.0 kg) was soaked overnight in 85% ethanol-H2O until fully saturated and was extracted with 90 l 85% ethanol-H2O three times for two hours each time. The ethanol solvent was then concentrated under reduced pressure to yield a crude lysate, of which 940.3 g was obtained using an extractor at room temperature (25°C). The total ethanol extract was dissolved in water with a total volume of 5 l and then partitioned with petroleum ether (4 L × 4) for depigmentation, yielding 35.1 g of extract. The aqueous layer was sequentially partitioned with ethyl acetate (4 L × 8) and n-butanol (4 L × 8) to yield ethyl acetate-soluble (370.1 g) and n-butanol-soluble (340.0 g) fractions, respectively. The remaining part was the aqueous layer (154.7 g).
Marsdeniae tenacissimae Separation and Purification
The ethyl acetate layer extract was dissolved in organic solvent, and thin-layer chromatography was used to screen a solvent elution system. This ethyl acetate-soluble fraction was then subjected to silica gel column chromatography (using 10 times the amount of extract) and was eluted with petroleum ether–acetone (v/v, 50:1, 30:1, 10:1, 5:1, 3:1, 2:1, or 1:1) to obtain fractions Fr.1 to Fr.9 based on their TLC profiles. Fr.3 was mixed with a portion of Fr.4, and Fr.7 was mixed with a portion of Fr.8.
Fraction 1 was fractionated by silica gel column chromatography and was eluted with CHCl3–MeOH to obtain six fractions, namely, Fr.1.1–Fr.1.6 based on TLC analysis. Fr.1.3 and Fr.1.5 were fractionated on an ODS column with a 40–80% methanol–water gradient elution to obtain 4 (17.0 mg), 12 (9.6 mg), and 20 (10.5 mg), respectively.
Fraction 2 was fractionated using silica gel column chromatography and was eluted with CHCl3–MeOH to obtain six fractions, namely, Fr.2.1–Fr.2.6, based on TLC analysis. Fr.2.1 and Fr.2.4 on the ODS column with a methanol–water gradient were eluted to obtain 3 (17.5 mg), 9 (10.5 mg), and 10 (18.1 mg).
Fraction 3 was separated by silica gel column chromatography and preparative PHPLC (detection at a wavelength of 210 nm, 55% MeOH, 2.2 ml/min) to obtain 1 (10.6 mg), 2 (15.6 mg), 7 (10.8 mg), and 8 (15.6 mg). Fr.3.2 was resolved by ODS column chromatography and was eluted with CHCl3–MeOH to obtain 5 (13.3 mg). Fr.3.4 was resolved using ODS column chromatography, eluted with CHCl3–MeOH, and assessed using PHPLC (detection wavelength: 210 nm, 30% acetonitrile, and 2.2 ml/min) to obtain 11 (15.6 mg) and 19 (4.2 mg).
Fraction 5 was resolved by silica gel column chromatography and was eluted with CHCl3–MeOH to obtain three fractions, Fr.5.1–Fr.5.3, based on TLC analysis. Fr.5.2 was resolved by ODS column chromatography and was eluted with MeOH–water to obtain 6 (4.8 mg).
Fraction 6 was resolved by silica gel column chromatography and was eluted with CHCl3–MeOH to obtain four fractions, Fr.6.1–Fr.6.4, based on TLC analysis. Fr.6.1 was resolved by ODS column chromatography and was eluted with MeOH–water to obtain 13 (6.4 mg). Fr.6.4 was also resolved by ODS column chromatography and was eluted with MeOH–water. Subsequently, the distillates were further resolved by Sephadex LH-20 column chromatography and were eluted with MeOH–water to obtain 17 (4.0 mg).
Fraction 7 was resolved by silica gel column chromatography and was eluted with CHCl3–MeOH to obtain six fractions, Fr.7.1–Fr.7.6, using TLC analysis. Fr.7.2 was resolved by Sephadex LH-20 column chromatography and was eluted with MeOH–water to obtain 14 (6.4 mg). Fr.7.4 was separated by preparative PHPLC (detection at 210 nm, 30% acetonitrile, 2.2 ml/min) to obtain 15 (4.0 mg).
Fraction 9 was resolved using Sephadex LH-20 column chromatography and was eluted with MeOH–water and was further separated by silica gel column chromatography to obtain 18 (6.0 mg). Figure 1 shows the specific extraction and separation process.
Spectral Data
Compound 15, white powder,
Compound 18, white powder,
Constituent Identification
All LC-MS and MS/MS results were processed using MassLynx™ (V4.1). Molecular formula estimations of the compounds were performed using Elemental Composition software (Waters Technologies, Milford, MA, United States). Structure determination of the main compounds, such as the chemical structure, precise molecular mass, as well as potential molecular fragmentation pathways, was performed using Mass Fragment software. Previously published compounds were collected by comprehensively searching various databases such as PubMed1, Chemspider2, HMDB3, and Metlin4 (Guijas and Siuzdak, 2018). Validation of compounds with standard materials was performed using reference standards.
Cell Culture
A549 cells were propagated in Dulbecco’s modified Eagle medium (DMEM) supplemented with 10% fetal bovine serum (FBS) and 1% penicillin-streptomycin at 37°C in a humid atmosphere with 5% CO2.
Cytotoxicity Experiment
The cytotoxic effects of M. tenacissimae extracts were assessed using the CCK-8 calorimetric procedure. Briefly, cells (density: 5 × 103 cells/well) were seeded into 96-well plates and cultured for 24 h. The supernatant was then discarded, and the cells were treated with extracts at various concentrations using a volume of 100 ul in 96-well plates to which DMEM was added with serum. Five wells were used for each concentration. Five blank controls with only the medium (no addition of cells) were used as the negative control group. After incubation for 24 h, the original medium in each well was replaced with 100 μl of medium containing 10% CCK-8. Thereafter, the 96-well plates were placed in an incubator for 3 h. The absorbance of each well at a wavelength of 450 nm was then determined with a microplate reader (TECAN, Switzerland). The experiment was performed three times in parallel.
Network Pharmacology Construction and Analysis
ChemSketch was used to draw the structure of the above six C21 steroidal saponins and to obtain their SMILES number. Then, we used SciFinder5 to confirm their molecular structure and obtained their CAS numbers. The Swiss Target Prediction6 (Gfeller et al., 2014) and STITCH7 (Kuhn et al., 2010) databases were used to screen potential targets of six C21 steroidal glycosides. The DigSee (Kim et al., 2017). DisGeNET8 (Pinero et al., 2015) and OMIM9 databases (Amberger et al., 2015) were employed to screen potential targets of NSCLC. Furthermore, the targets of disease were matched with the targets of the compound, and a compound-target-NSCLC network was constructed with Cytoscape 3.7.2 (Settle et al., 2018). The KEGG10 database (Amberger et al., 2015) was used to enrich the target signal pathway. Then, the compound-target and target-pathway networks were merged to obtain a compound-target-pathway network. String APP in Cytoscape 3.7.2 was employed, and the Network analysis and Generatestyle functions were used to generate the protein-target-interaction network. DAVID11 was used to conduct Gene Ontology (GO) and KEGG pathway analyses. Finally, we used the KEGG Mapper tool to obtain and integrate the pathway related to the anti-NSCLC effect of the six C21 steroidal saponins.
Migration Assay
The IC50 values of compounds TGT-7, TGT-9, TGT-13, and TGT-15 were determined to be 28.36, 44.01, 29.03, and 47.33 μm, respectively, in a previous study (Xu, 2018; Hu et al., 2020). Based on the IC50 values of compounds, we determined the concentration necessary for tests with A549 cells. A549 cells (density: 1 × 106 cells per well) were first seeded into six-well plates. Upon reaching a confluency of 90%, the A549 monolayer was scraped in the middle of each well using a 20 μl pipette tip, and then the plates were washed three times with PBS, and media with TGT-7, TGT-9, TGT-13, and TGT-15 were added separately at concentrations of 28 μM, 44 μM, 29 μM, and 47 μM, respectively, for 36 h. The control group was supplemented with DMEM. Then, three fields of every wound were selected, and the rate of wound closure was calculated using ImageJ (Wayne Rasband, National Institutes of Health, United States).
Invasion Assay
Matrigel® was left to stand at 4°C overnight and was then thawed. The Matrigel® gel was prepared with serum-free medium at a final concentration of 1 mg/ml. Approximately 100 μl of the prepared Matrigel® gel was vertically added to the bottom of the upper chamber, and 600 ul of each well consisting of 10% FBS was placed in the lower chamber. Then, 1 × 105 A549 cells were resuspended in 100 µl in the bottom of the well, supplemented with 0.1% BSA and TGT-7 (28 µM), TGT-9 (44 µM), TGT-13 (29 µM), or TGT-15 (47 µM) and cultured for 48 h to allow cell migration across the filter membrane. Cell fixation was performed with methanol for 30 min, followed by 1% crystal violet staining for 25 min and the washing away of excess crystal violet stain. A total of five images were randomly captured with an inverted microscope, which were then used to count the number of transmembrane cells.
Cell Cycle Analysis
Cell cycle analysis was conducted using flow cytometry. A549 cells were treated with TGT-7 (28 µM), TGT-9 (44 µM), TGT-13 (29 µM), and TGT-15 (47 µm) for 24 h, and then the cell pellet was washed three times in ice-cold PBS. The cells were shaken using 70% ethanol and resuspended in a −20°C refrigerator for at least 24 h. After the cells were fixed, they were centrifuged at 1,000 rpm for 5 min. The ethanol was discarded, and the cells were washed three times in ice-cold PBS. The cells were then centrifuged, the supernatant was decanted, and the cell pellet was resuspended in annexin-binding buffer. Then, 0.5 ml of PI/rnase was added to the cells and left to stand in the dark for approximately 15 min. Cell cycle analysis was immediately conducted using flow cytometry.
Cell Apoptosis Analysis
Cell apoptosis was analyzed using flow cytometry. A549 cells were treated with TGT-7 (28 μM, 56 µM), TGT-9 (44 μM, 88 µM), TGT-13 (29 μM, 58 µM), and TGT-15 (47 μM, 94 µM) for 24 h. Then, the supernatants were collected in a 15 ml centrifuge tube, and the adherent cells were trypsinized, detached, and collected in a corresponding centrifuge tube and centrifuged at 1,000 rpm for 5 min. Then, the supernatant was discarded, and the cells were washed twice using 1× binding buffer and were sequentially stained with Annexin V and PI following the manufacturer’s instructions. Finally, the A549 cells were observed by fluorescence microscopy and then analyzed by flow cytometry.
Mitochondrial Membrane Potential Assay
JC-1, a cationic fluorescent dye when added to living cells, is known to be localized exclusively in mitochondria, particularly in good physiological conditions characterized by sufficient mitochondrial membrane potential (∆Ψ). The current paper is dealing with the study of differences in the effects of four compounds (TGT-7, TGT-9, TGT-13, and TGT-15) on the JC-1 loading and fluorescence in A549 cells. A549 cells were treated with TGT-7 (28 µM), TGT-9 (44 µM), TGT-13 (29 µM), and TGT-15 (47 µM), respectively, for 24 h. Then, the A549 cells were stained using JC-1 at 37°C for 20 min, and images were captured using a fluorescence microscope. A decrease in the mitochondrial membrane potential was indicated by a change in the wavelength, i.e., from red to green. ImageJ was used to assess the intensity of red and green fluorescent emissions, which represented potential alterations in the mitochondrial membrane.
Intracellular ROS Detection
The amount of intracellular ROS produced was determined using an ROS assay. After the A549 cells were treated with TGT-7 (28 µM), TGT-9 (44 µM), TGT-13 (29 µM), and TGT-15 (47 µM) for 24 h, the A549 cells were incubated in the presence of 10 μM DCFH-DA at 37°C for 30 min, followed by washing twice using PBS. Finally, the A549 cells were assessed under a fluorescence microscope and were processed by flow cytometry to measure DCFH-DA fluorescence.
Western Blotting
A549 cells that were treated with various concentrations of TGT-7 (28 and 56 µM), TGT-9 (44 and 88 µM), TGT-13 (29 and 58 µM), and TGT-18 (47 and 94 µM), were lyzed using RIPA buffer containing protease inhibitors. Then, the BCA assay was performed to determine protein concentrations. Proteins were resolved by SDS-PAGE and then immunoblotted onto PVDF membranes. MMP-2, MMP-9, caspase-9, caspase-3, and caspase-8, Bax, Bcl-2, and cytochrome C primary antibodies were used to detect the corresponding proteins, followed by incubation with the corresponding secondary antibodies. Finally, ImageJ (developed by the National Institute of Health) was used to quantify the immunoblots, and the images presented are representative of three separate experiments.
Statistical Analysis
The experimental data were analyzed using SPSS 20.0. Unless otherwise stated, the data were presented as the arithmetic means of three independent experiments. The results were shown as the mean ± SD. We employed one-way ANOVA to assess variance when homogeneous variance was observed, with the least significant difference (LSD). In addition, the Dunnett T3 test was utilized when the variance was determined to be not uniform. Statistical significance was considered at *p < 0.05, **p < 0.01, and ***p < 0.001 in the analyses of TGT-7-, TGT-9-, TGT-13-, and TGT-15-treated vs. untreated control cells.
Results
Characterization of Marsdeniae tenacissimae Caulis Chemical Constituents
In this study, we reported the isolation of 19 compounds from the dry cane of Marsdeniae tenacissimae Caulis. We elucidated their structures, which included two novel compounds, namely, 11α-O-benzoyl-12β-O-tigloyltenac-igenin B (15, 4.0 mg) (Figure 2, Table 1) and sodium 5-hydroxy-4-(((2S,3R,4S,5R,6R)-5-hydroxy-3-(((2R,3R,4R,5R,6S)-5-hydroxy-6-((2-hydroxy-4,6-dimethoxy-6-oxohexan-3-yl)oxy)-4-methoxy-2-methyltetrahydro-2H-pyran-3-yl)oxy)-4-methoxy-6‐methyltetrahydro-2H-pyran-2-yl)oxy)-3-methoxyhexanoate (18, 6.0 mg) (Figure 3, Table 1), as well as 17 other known compounds (1–14, 17, 19, 20) (Figure 4, Supplementary Tables S1, S2).
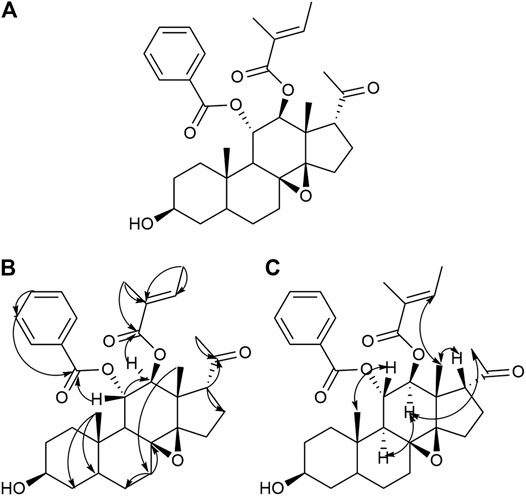
FIGURE 2. Structure (A), Key HMBC (H→C) correlation (B), and Key NOESY (H–H) correlation (C) of compound 15.
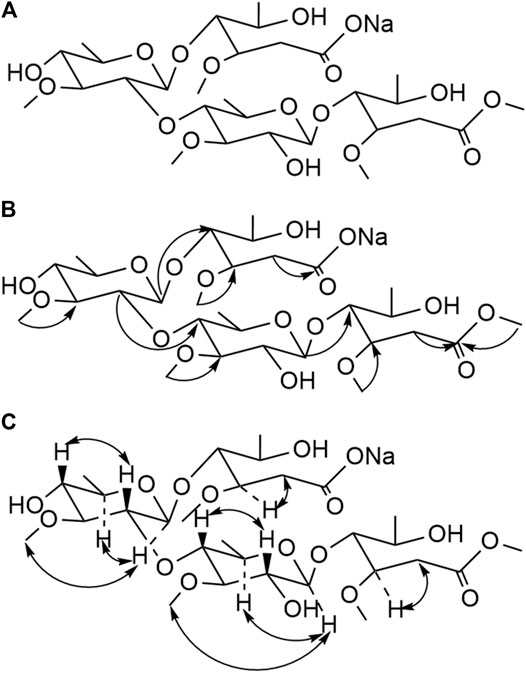
FIGURE 3. Structure (A), Key HMBC (H→C) correlation (B), and Key NOESY (H–H) correlation (C) of compound 18.
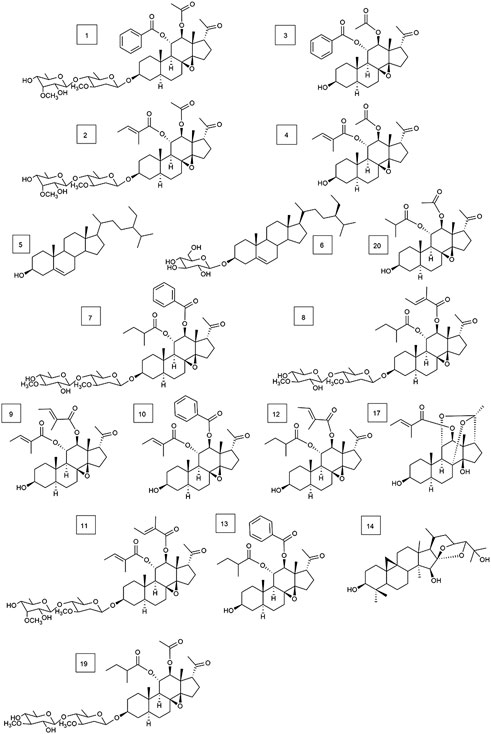
FIGURE 4. Structures of 17 types of known compounds isolated from Marsdenia tenacissima (compounds 1–14, 17, 19, and 20.).
Compound 15 was obtained as a white powder. It was identified qualitatively by TLC and colored with anisaldehyde and concentrated sulfuric acid, displaying a yellow–green color, and dark spots were observed under a UV lamp at a wavelength of 254 nm. The ion peaks at m/z 573.4 [M+Na]+, 551.6 [M+H]+, 585.4 [M+Cl]–, and 549.1 [M−H]– for positive and negative ions were obtained using ESI-MS and were assumed to have a molecular weight of 550.3
Compound 18 was obtained as a white powder. The ion peaks, m/z 695.6 [M+H]+, 671.7 [M−Na]−, for the positive and negative ions, respectively, were obtained using ESI-MS and were assumed to have a molecular weight of 694.3
In addition, 17 known compounds (1–14, 17, 19, 20) were isolated from the ethyl acetate extract of Marsdeniae tenacissima Caulis (Figure 4) and were identified as tenacissoside I (1, 10.6 mg), tenacissoside G (2, 15.6 mg) (Zhang et al., 2010), 11α-O-benzoyl-12β-O-acetyltenacigenin B (3, 17.5 mg) (Yao et al., 2014), 11α-O-tigloyl-12β-O-acetyltenacigenin B (4, 17.0 mg), β-sitosterol (5, 13.3 mg), daucosterol (6, 4.8 mg) (Dong and Cui, 2013), marsdenoside C (7, 10.8 mg), marsdenoside A (8, 15.6 mg) (Deng et al., 2005), 11α-O-tigloyl-12β-O-benzoyltenacigenin B (9, 10.5 mg) (Liu and Kong, 2018), 11α-O-2-methylbutyryl-12β-O-tiglo-yltenacigenin B (10, 18.1 mg), marsdenoside B (11, 15.6 mg), 11α, 12β-O, O-ditigloyl-17β-tenacigenin B (12, 9.6 mg) 11α-O-2-methylbutyryl-l2β-O-benzoyltenacigenin B (13, 6.4 mg), cimigenol (14, 6.4 mg), 12β-O-tigloyltenacigenin A (17, 4.0 mg) (Li and Sun, 2008), tenacissoside H (19, 4.2 mg), and 11α-O-2-methylbutyryl-12β-O-acetyltenacigenin B (20, 10.5 mg). Cimigenol 14 was the first compound isolated from these extractions. The 1H NMR spectrum and 13C NMR spectrum spectrum data of these compounds are detailed in the supplementary file (Supplementary File S1, Supplementary Tables S1–S3).
Cell Cytotoxicity Assay of Marsdeniae tenacissimae Extracts
The inhibitory activity of all of the isolated compounds against A549 cells was assessed using an in vitro assay. The effect of Marsdeniae tenacissimae Caulis monomer compounds on the activity of A549 cells showed that six steroidal saponins effectively had inhibitory effects on A549 cells in vitro. The IC50 values of these six compounds and ginsenoside Rg3 were compared and were arranged in decreasing order as follows: compound 7 < compound 13 < compound 9 < compound 15 < ginsenoside Rg3 < compound 10 < compound 8 (Figure 5, Table 2).
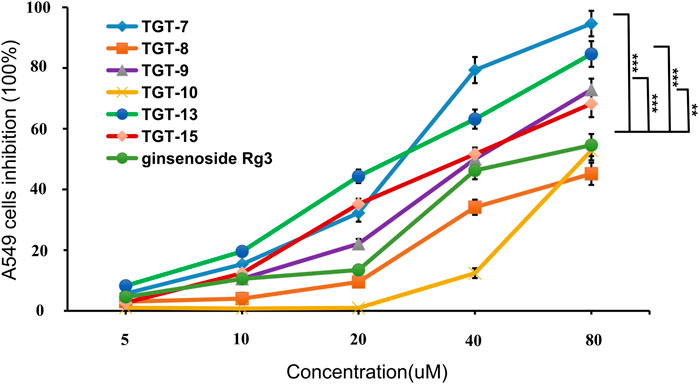
FIGURE 5. Cytotoxic activity of compounds 7, 8, 9, 10, 13, 15 and ginsenoside Rg3 against the growth of A549 cells. Values are expressed as the mean ± SD,***p < 0.001, **p < 0.01 vs. Ginsenoside Rg3 IC50 (n = 3).
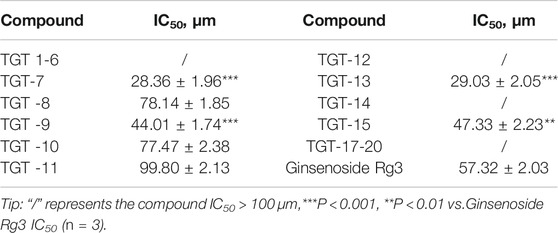
TABLE 2. The inhibitory effect of 19 compounds from Marsdeniae tenacissimae and Ginsenoside Rg3 on A549 cells.
Prediction of Putative Targets of Marsdeniae tenacissimae
Chemical Information and Construction of the Compound-Target-NSCLC Network
SMILES numbers of the compounds were downloaded from the Swiss Target Prediction (Daina et al., 2019) and STITCH databases (Kuhn et al., 2010) used in screening potential targets of the six effective C21 steroidal glycosides. A total of 247 potential targets of the six steroidal saponins were predicted (37 for TGT-7, 58 for TGT-8, 1 for TGT-9, 149 for TGT-10, 1 for TGT-13, and 1 for TGT-15) using the Swiss Target Prediction and STITCH databases, and 205 targets remained after deleting duplicates and false positives.
Searching for NSCLC targets was performed using DigSee (275, Evidence Sentence Score ≥0.6), DisGeNET (225, Score ≥0.1), Malacards (62), and OMIM (142). After the removal of overlapping genes, 270 NSCLC-related targets remained.
Finally, 18 distinct potentially therapeutic genes were identified as targets of the six C21 steroidal glycosides components. This network showed that in terms of anti-NSCLC activity, multiple components acting on multiple targets acted synergistically. Basic information on the six C21 steroidal saponins of Marsdeniae tenacissimae Caulis is shown in Table 3 and Figure 6.
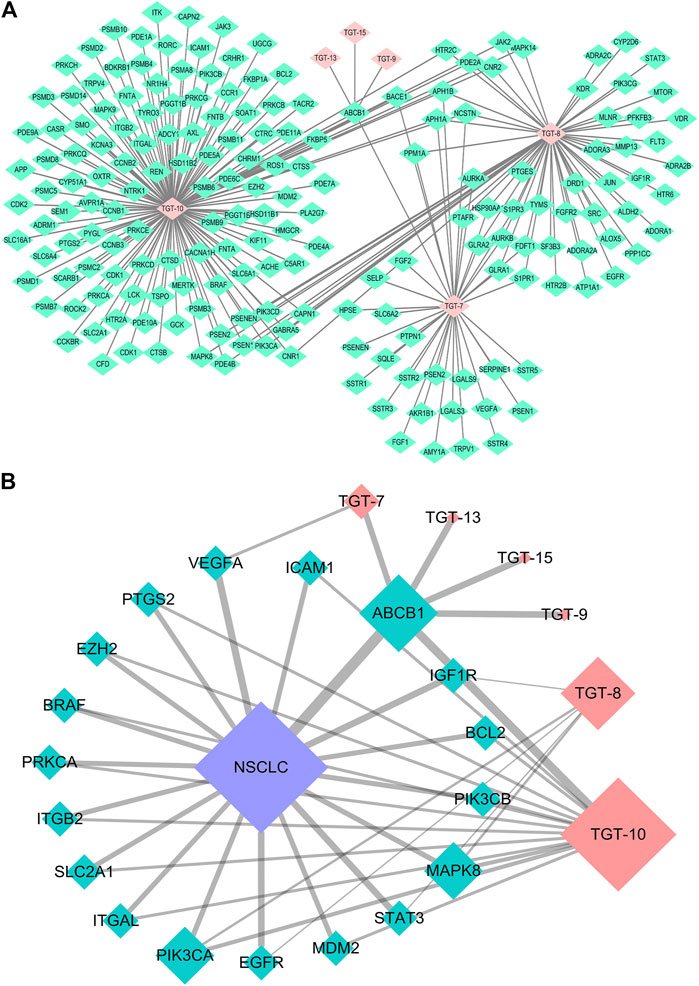
FIGURE 6. Network pharmacology approach of compounds 7, 8, 9, 10, 13, and 15 (A) Compound-target network. Pink represents C21 steroidal saponins (TGT-7, TGT-8, TGT-9, TGT-10, TGT-13, and TGT-15), and green represents their respective targets (B) Component-target-NSCLC network. Pink represents C21 steroidal saponins, blue represents NSCLC disease, and green represents their common targets.
Construction of the Compound-Target-Pathway Network
The KEGG and DAVID databases were then employed to enrich the target signal pathways. The network of the compound-target-pathway was constructed by Cyctoscape 3.7.2, consisting of 44 nodes and 175 edges. The edges indicated the interactions between active ingredients and targets and pathways.
This network showed that the six C21 steroidal saponins participated in the regulation of different pathways that were related to tumor pathogenesis via multi-target synergistic activity. These pathways included cancer-related pathways, PI3K/AKT, HIF-1 pathogenesis via multi-target synergistic activity-regulated changes in the tumor cell cycle, and angiogenesis, thus inhibiting cancer cell invasion and migration and inducing tumor apoptosis (Tables 4, 5Figures 7A, 8A).
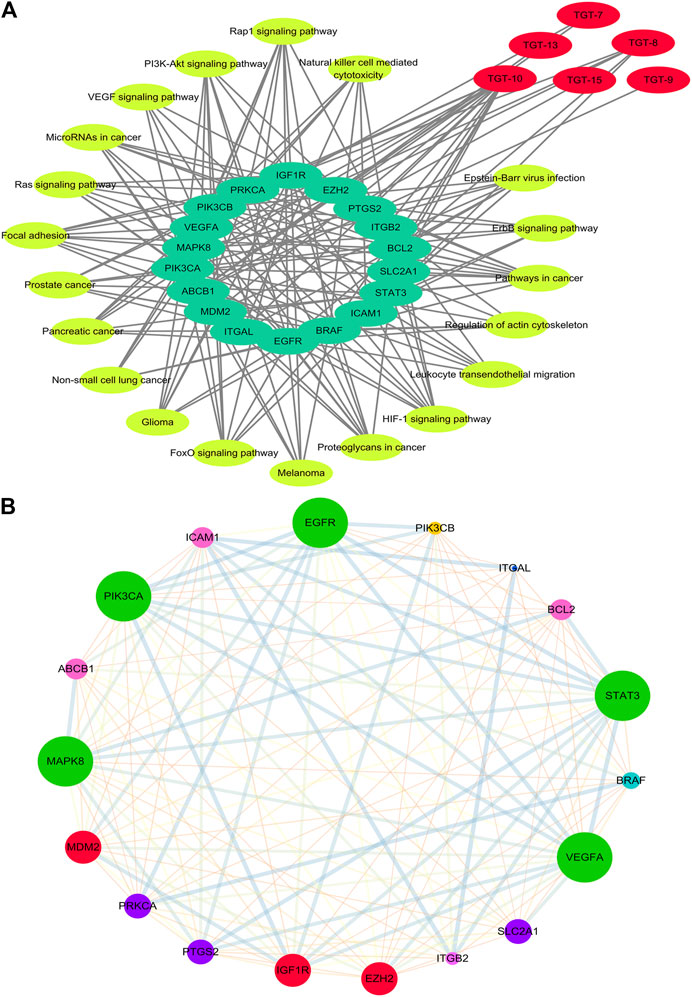
FIGURE 7. Network of Compound-target-pathway and Protein–protein interaction (A) Compound-target-pathway network. Red represents C21 steroidal saponins, bright yellow represents pathways, and green represents their targets (B) Protein–protein interaction network (PPI). The larger the node, the greater position occupied in the whole network. The lines between nodes represent the interactions between two proteins that are interconnected. Different-colored lines represent various types of interactions. The thicker the line, the closer the interaction.
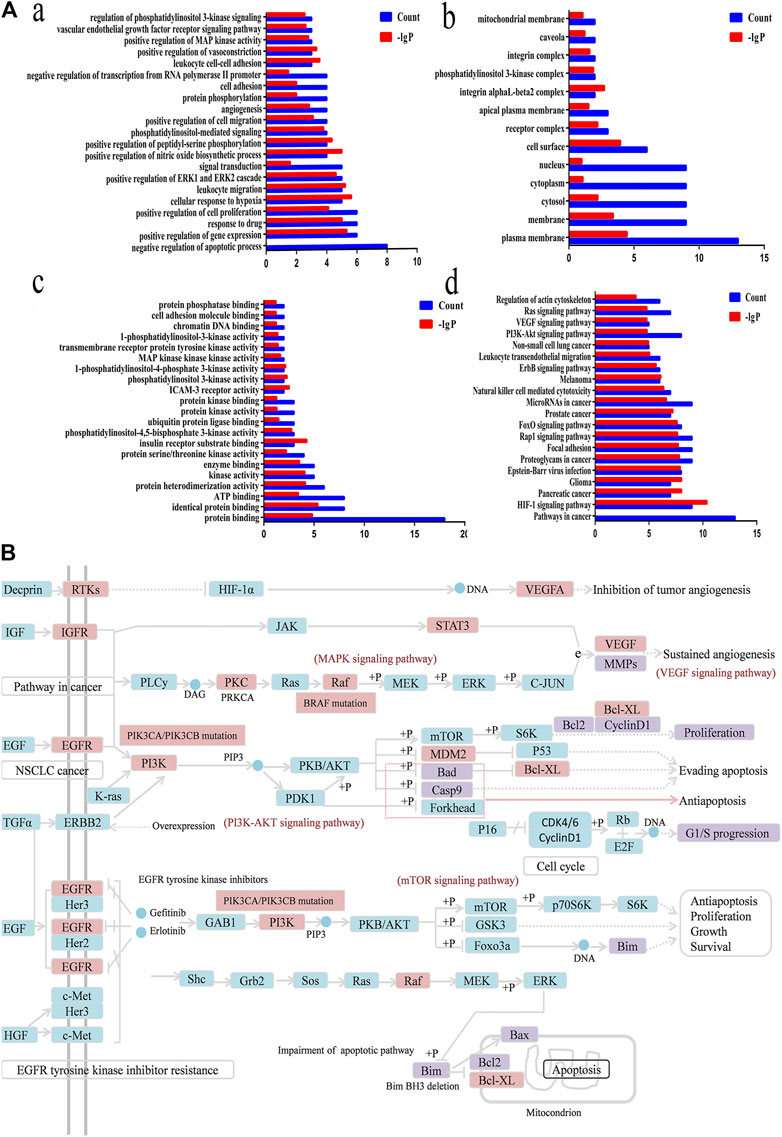
FIGURE 8. Functional annotation, pathway analysis and Anti-NSCLC pathway of C21 steroidal saponins. (A) Functional annotation and pathway analysis of key target genes of C21 steroidal saponins against NSCLC. (a) Enriched Gene Ontology terms for the BP of potential anti-NSCLC targets. (b) MF enrichment analysis of potential anti-NSCLC targets. (c) CC enrichment analysis of potential anti-NSCLC targets. (d) KEGG pathway of anti-NSCLC targets. (B) Anti-NSCLC pathway of C21 steroidal saponins. Blue squares represent targets in the pathway, pink squares represent anti-NSCLC targets, and purple squares represent targets that were verified in subsequent experiments in this study.
Construction and Analysis of the Protein-Interaction Network
STRING was used to assess target protein interactions. Figure 7B shows that the network graph consisted of 18 nodes and 127 edges. The definitions and equations for these parameters revealed the topological significance of the nodes in these networks, and the more important nodes showed higher quantitative values. DisGeNET was used to obtain the corresponding types of targets (Table 6). The results showed that signaling molecules, enzymes, and proteins were involved in the anti-lung cancer effect of Marsdeniae tenacissima.
Gene Function and Pathway Analysis
DAVID was used to conduct Gene Ontology (GO) and KEGG pathway analyses. A threshold of p < 0.05 was used in screening the biological process or pathway, and GraphPad Prism 7.0 was employed for drawing enriched terms in the CC, BP, and MF categories (Figure 8A).
BP analysis indicated that these targets were mainly related to biological processes, including negative regulation of apoptotic processes, positive control of cell proliferation, positive control of cell migration, angiogenesis, regulation of phosphatidylinositol 3-kinase signaling, and the vascular endothelial growth factor receptor signaling pathway (Figure 8A).
CC analysis revealed that markedly enriched terms were mainly concentrated in the formation of the phosphatidylinositol 3-kinase complex, plasma membrane, and receptor complex (Figure 8A).
MF analysis showed enriched terms including protein binding, ATP binding, protein serine/threonine kinase activity, phosphatidylinositol-4,5-bisphosphate3-kinase activity, ubiquitin protein ligase binding, phosphatidylinositol3-kinase activity, and 1-phosphatidylinositol-4-phosphate 3-kinase activity (Figure 8A).
Target Pathway Analysis
KEGG Mapper was used to obtain the pathway map of Marsdenia tenacissima resistance to NSCLC, and the major pathways were integrated to construct a pathway map (Figure 8B). The arrows in the figure indicated promoting effects, T-arrows represented inhibitory effects, the pathway targets were in blue, the network pharmacological prediction targets of resistance to NSCLC were in pink, and the experimentally verified targets were in purple. The figure showed that the anti-NSCLC effects of the six C21 steroidal saponins mainly involved pathways in cancer, including HIF-1 signaling, PI3K-Akt signaling, VEGF signaling, EGFR tyrosine kinase inhibitor resistance, and Ras signaling. The integrated pathway diagram was shown in (Figure 8B). To further explore the specific role and mechanism of its anti-NSCLC, we conducted preliminary experimental verification on the predicted potentially key targets in the pathway.
Effects of TGT-7, TGT-9, TGT-13, and TGT-15 on the Migration and Invasion of A549 Cells
To investigate whether TGT-7, TGT-9, TGT-13, and TGT-15 (Figure 9A) affected the migration and invasion of A549 cells, we first tested the four compounds (TGT-7, TGT-9, TGT-13, and TGT-15) in migration and invasion assays. A549 cells were exposed to TGT-7 (28 µm), TGT-9 (44 µm), TGT-13 (29 µm), and TGT-15 (47 µm) for 36 h. Migration experiments indicated that control cells significantly migrated after treatment with TGT-7 (28 µm), TGT-9 (44 µm), TGT-13 (29 µm), and TGT-15 (47 µm), with relative widths of the cell scratches of 0.852 ± 0.087, 0.549 ± 0.033, 0.909 ± 0.045, and 0.538 ± 0.056, respectively. Compared with the control group (0.443 ± 0.075), the results were statistically significant (Figure 9B). The invasion assays showed that many A549 cells in the control group were filtered from the upper region of transwell chambers and moved to the lower part after treatment with TGT-7 (28 µm), TGT-9 (44 µm), as well as TGT-13 (29 µm). The number of A549 cells that moved across the filtration membrane significantly decreased. However, after treatment with TGT-15 (47 µm), the number of A549 cells that moved across the filtration membrane significantly decreased. However, t-test results indicated the change was not significant. The number of A549-invading cells after treatment with TGT-7 (28 µm), TGT-9 (44 µm), TGT-13 (29 µm), and TGT-15 (47 µm) were 29.87 ± 0.70, 26.33 ± 0.50, 58.8 ± 0.92, and 66.00 ± 3.74, respectively. Compared with the control group (68.40 ± 2.09), the TGT-7 (28 µm), TGT-9 (44 µm), and TGT-13 (29 µm) groups were statistically significant (Figure 9C). These results showed that TGT-7, TGT-9, TGT-13, and TGT-15 could inhibit A549 cells migration and invasion.
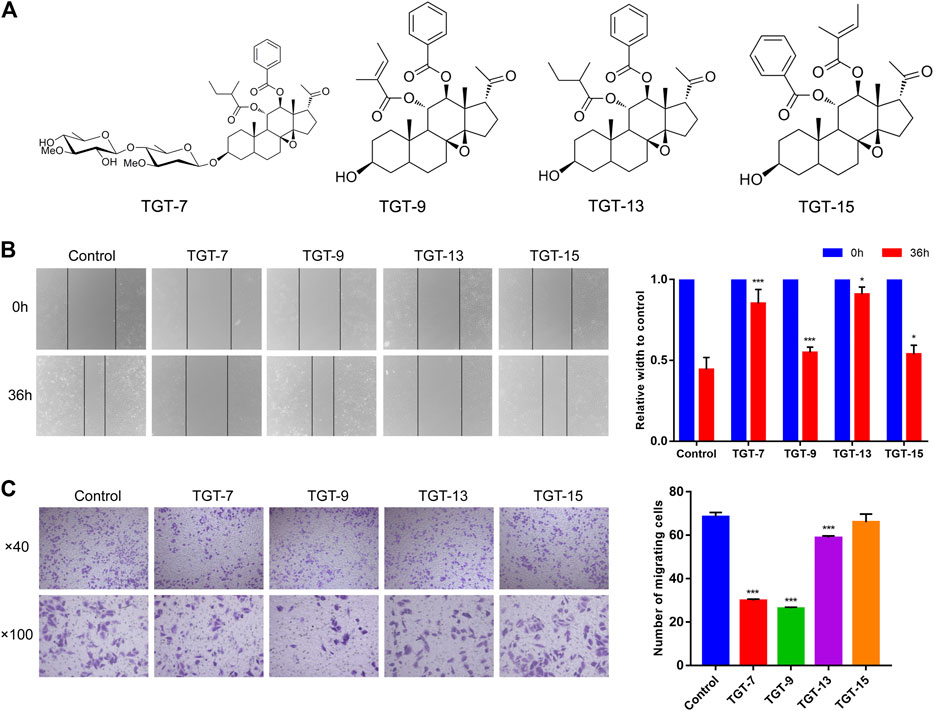
FIGURE 9. Effects of TGT-7 (28 µm), TGT-9 (44 µm), TGT-13 (29 µm), and TGT-15 (47 µm) on migration and invasion of A549 cells (A) Structure of marsdenoside C (TGT-7), 11α-O-tigloyl-12ofO-benzoyltenacigenin B (TGT-9), and 11-α-O-2-methylbutyryl-l2 BO-benzoyltenacigenin B (TGT-13), 11α-O-benzoyl-12acO-tigloyltenacigenin B (TGT-15) (B) Wound-healing assays were performed to measure the effect of the four C21 steroidal glycosides on the migration ability of A549 cells (original magnification ×40) (C) Transwell–Matrigel invasion assay was performed to evaluate the effect of the change in the four C21 steroidal glycosides on the migration ability of A549 cells (original magnification ×100). These results were obtained from three independent experiments, and all of the data are expressed as the mean ± SD, *p < 0.05, ***p < 0.001 vs. the control group (n = 3).
TGT-7, TGT-9, TGT-13, and TGT-15 Induced A549 Cells Cycle Arrest at the G0/G1 Phase
A549 cells were exposed to TGT-7 (28 µm), TGT-9 (44 µm), TGT-13 (29 µm), as well as TGT-15 (47 µm) for 24 h and were fixed and stained using propidium iodide, and alterations in the cell cycle were evaluated by flow cytometry. The results showed that the proportion of G0/G1 cells after treatment with TGT-7 (28 µm), TGT-9 (44 µm), TGT-13 (29 µm), as well as TGT-15 (47 µm) increased from 65.31 ± 3.79% to 75.58 ± 0.44%, 71.63 ± 2.02%, 80.27 ± 2.13%, and 69.17 ± 1.05%, respectively. Compared with the control group, the number of G0/G1 cells increased to different degrees (Figures 10A,B). These results demonstrated that TGT-7, TGT-9, TGT-13, and TGT-15 could arrest A549 cells at the G0/G1 phase.
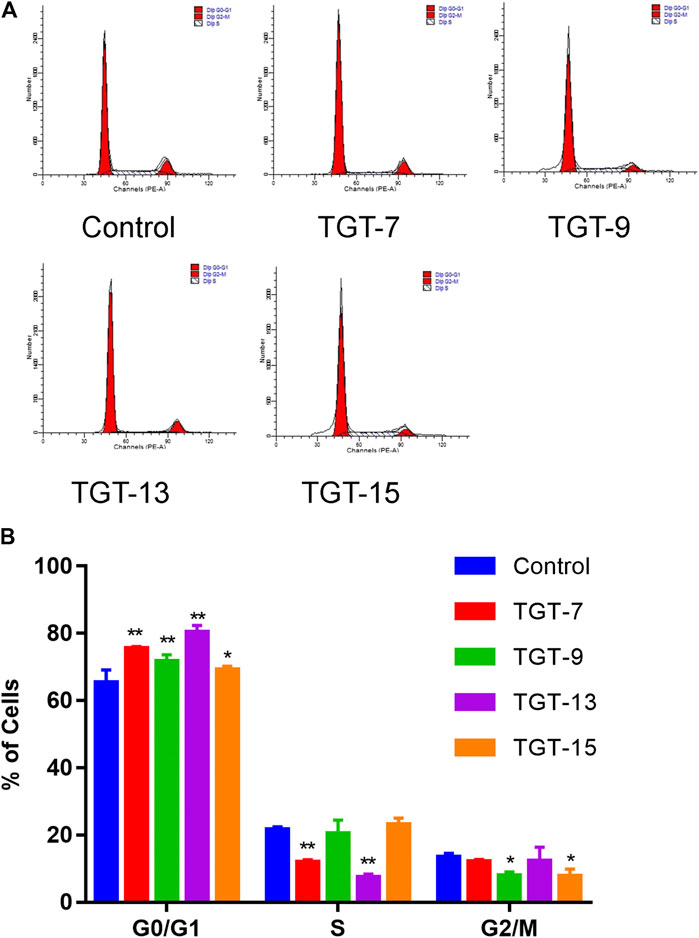
FIGURE 10. TGT-7 (28 µm), TGT-9 (44 µm), TGT-13 (29 µm) and TGT-15 (47 µm) induce A549 cells cycle arrest in the G0/G1 phase (A) Changes in cell cycle phases of A549 cells were detected by flow cytometry (B) The number of A549 cells in the G0/G1 phase were significantly increased. These results were obtained from three independent experiments, and all of the data are expressed as the mean ± SD, *p < 0.05, **p < 0.01 vs. the control group (n = 3).
TGT-7, TGT-9, TGT-13, and TGT-15 Induce A549 CellS Apoptosis
Annexin V-FITC/PI double-staining was employed to detect apoptotic cells. The A549 cells were treated with TGT-7 (28 µm, 56 µm), TGT-9 (44 µm, 88 µm), TGT-13 (29 µm, 58 µm), and TGT-15 (47 µm, 94 µm) for 24 h, and the results revealed higher green fluorescence staining relative to the control group (Figure 11A). In addition, the number of apoptotic cells was determined using flow cytometry, and compared with the control group, the apoptotic cells (the sum of early and late apoptotic cells) in the drug-treated group significantly increased, and an increase in concentration was correlated with a higher number of apoptotic cells (Figure 11B). These data suggested that TGT-7, TGT-9, TGT-13, and TGT-15 induced apoptosis in A549 cells in a concentration-dependent fashion.
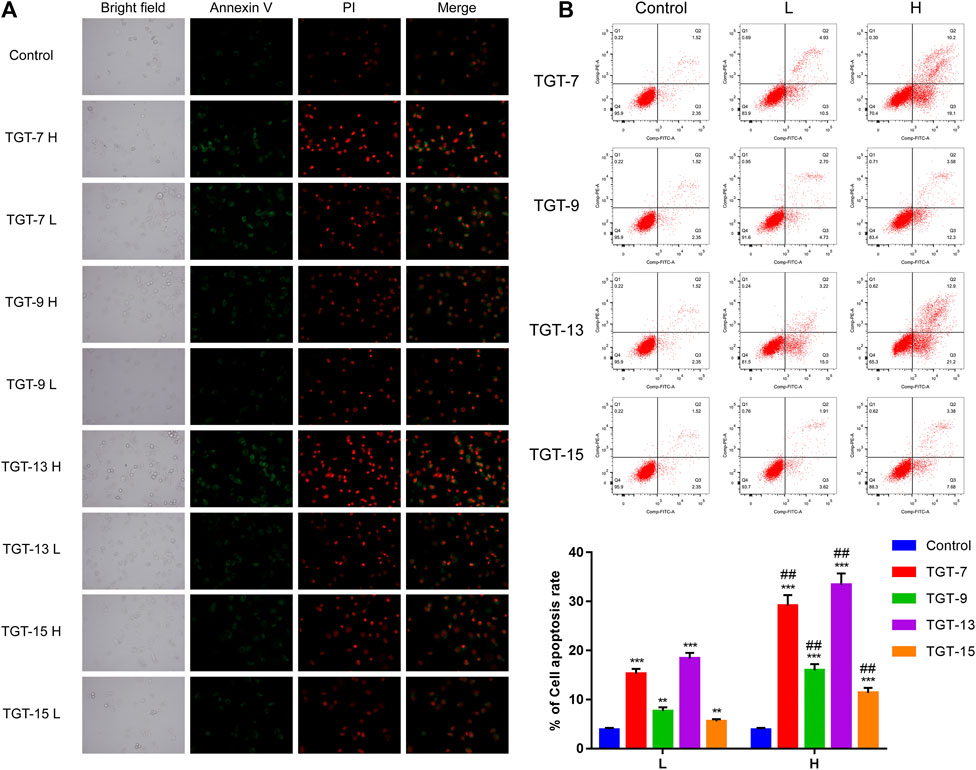
FIGURE 11. Effects of TGT-7 (28 μm, 56 μm), TGT-9 (44 μm, 88 μm), TGT-13 (29 μM, 58 μm), and TGT-15 (47 μm, 94 μm) on the apoptosis of A549 cells (A) Fluoroscope micrographs of apoptotic cells after Annexin V-FITC/PI double staining. Apoptotic cells are indicated in green, whereas necrotic cells are indicated in red (original magnification: ×100) (B) Cell apoptosis profiles were assessed using Annexin V-FITC/PI staining and flow cytometry. The biparametric histogram reveals cells in the early (bottom right quadrant) and late apoptotic states (upper right quadrant); viable cells are shown as double-negatives (bottom left quadrant). The numbers show the percentages of each fraction. These results were obtained from three independent experiments, and all data are expressed as the mean ± SD, **p < 0.01, ***p < 0.001 vs. the control group, ##p < 0.01, vs. the low-dose group (n = 3).
TGT-7, TGT-9, TGT-13, and TGT-15 Reduce the Mitochondrial Membrane Potential of A549 Cells
Next, we assessed the effects of TGT-7, TGT-9, TGT-13, and TGT-15 on A549 cells mitochondrial membrane potential by evaluating changes in the red–green fluorescence ratio after JC-1 staining. The results showed that in A549 cells treated with TGT-7, TGT-9, TGT-13, and TGT-15, green fluorescence increased, red fluorescence decreased, and the ratio of red–green fluorescence decreased from 14.24 ± 1.14 to 3.10 ± 0.43, 2.74 ± 0.55, 9.54 ± 0.58, and 9.64 ± 1.10, respectively. Compared to the control group, we observed statistically significant differences (Figure 12A). Then, we verified the findings through flow cytometry experiments, and the results were consistent with the above results (Figure 12B). These results demonstrated that TGT-7, TGT-9, TGT-13, and TGT-15 treatment resulted in depolarization of the mitochondrial membrane potential.
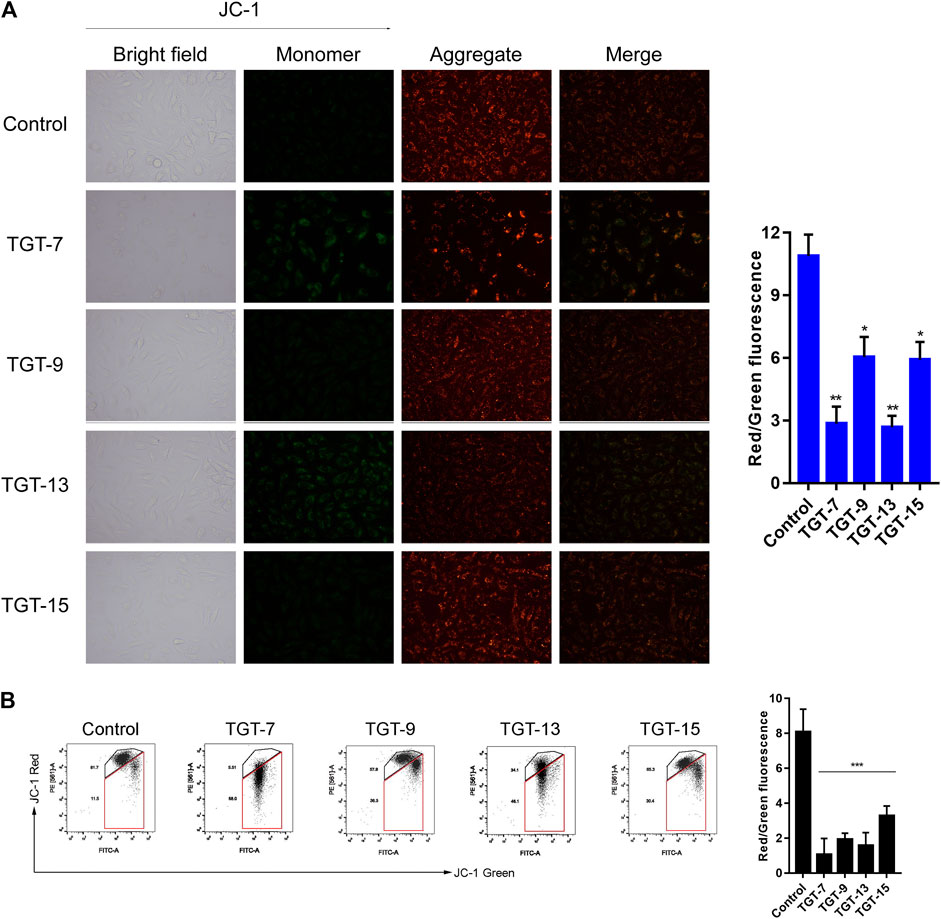
FIGURE 12. TGT-7, TGT-9, TGT-13, and TGT-15 cause mitochondrial membrane potential dysfunction of A549 cells (A) Mitochondrial membrane potential observed using fluorescence miscopy (original magnification ×200) (B) Mitochondrial membrane potential observed using flow cytometry. These results were obtained from three independent experiments, and all of the data are expressed as the mean ± SD, *p < 0.05, **p < 0.01, ***p < 0.001 vs. the control group (n = 3).
TGT-7, TGT-9, TGT-13, and TGT-15 Increase Intracellular ROS Levels in A549 Cells
We also examined the effects of TGT-7, TGT-9, TGT-13, and TGT-15 on ROS production in A549 cells by measuring changes in ROS in A549 cells after DCFH-DA staining. The experimental results showed that after treatment with TGT-7, TGT-9, TGT-13, and TGT-15, compared to the control group, green fluorescence in the A549 cells significantly increased. This indicated that these four compounds all caused an increase in intracellular ROS levels (Figures 13A–C). These results demonstrated that TGT-7, TGT-9, TGT-13, and TGT-15 all induced ROS production in A549 cells, which led to an increase in the concentration of ROS.
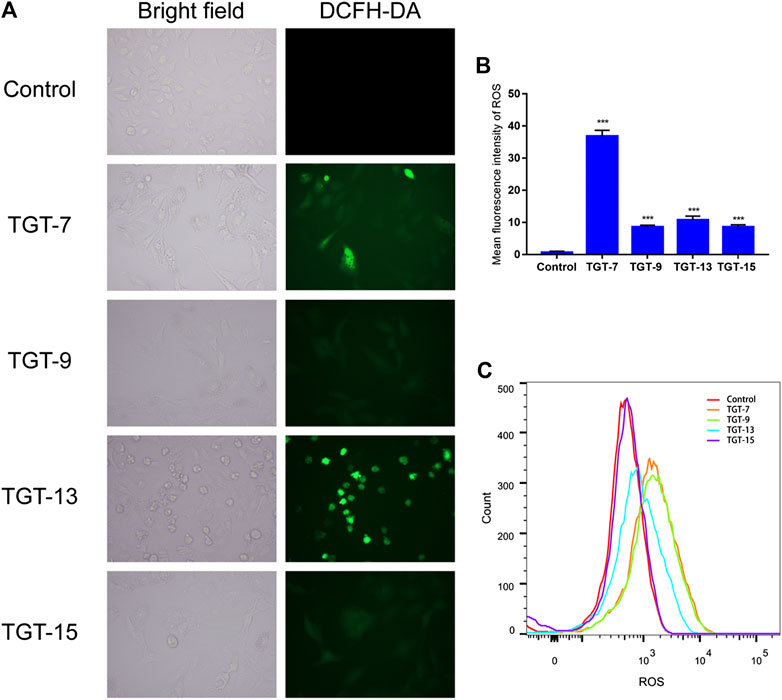
FIGURE 13. TGT-7, TGT-9, TGT-13, and TGT-15 increase intracellular ROS levels in A549 cells (A) and (B) Intracellular ROS levels of A549 cells observed using fluorescence microscopy (original magnification ×200) (C) Intracellular ROS levels of A549 cells determined by flow cytometry. Results were obtained from three separate experiments, and data are presented as the mean ± SD, ***p < 0.001 vs. the control group (n = 3).
TGT-7, TGT-9, TGT-13, and TGT-15 Modulate Migration and Apoptosis-Related Key Proteins
To further clarify the potential molecular mechanisms of TGT-7, TGT-9, TGT-13, and TGT-15 inhibiting the growth of A549 cells, we next examined key proteins that were closely related to migration and apoptosis. The results showed that TGT-7, TGT-9, TGT-13, and TGT-15 decreased MMP-2 and MMP-9 expression in A549 cells. However, this gradually decreased with increasing concentrations and showed a dose-dependent manner. In addition, we examined the expression patterns of cytochrome C, Bax, Bcl-2, cleaved caspase-3, and cleaved caspase-9 (Figures 14A–C). The results showed that the high and low concentrations of TG-T-7, TGT-9, TGT-13, and TGT-15 increased the expression of cytochrome C, and the high and low concentrations of TGT-7, TGT-9, and TGT-13 and the high concentrations of TGT-15 increased the expression of caspase-9 and caspase-3, indicating that all four compounds could promote the release of mitochondrial cytochrome C and TGT-7, TGT-9, and TGT-13 could activate caspase-9 and then activate caspase-3 to induce apoptosis. TGT-7, TGT-9, TGT-13, and TGT-15 could increase the expression of cleaved caspase-8 at high concentrations, which suggested that TGT-7, TGT-9, TGT-13, and TGT-15 could induce apoptosis in the mitochondrial pathway and possibly through the death receptor pathway.
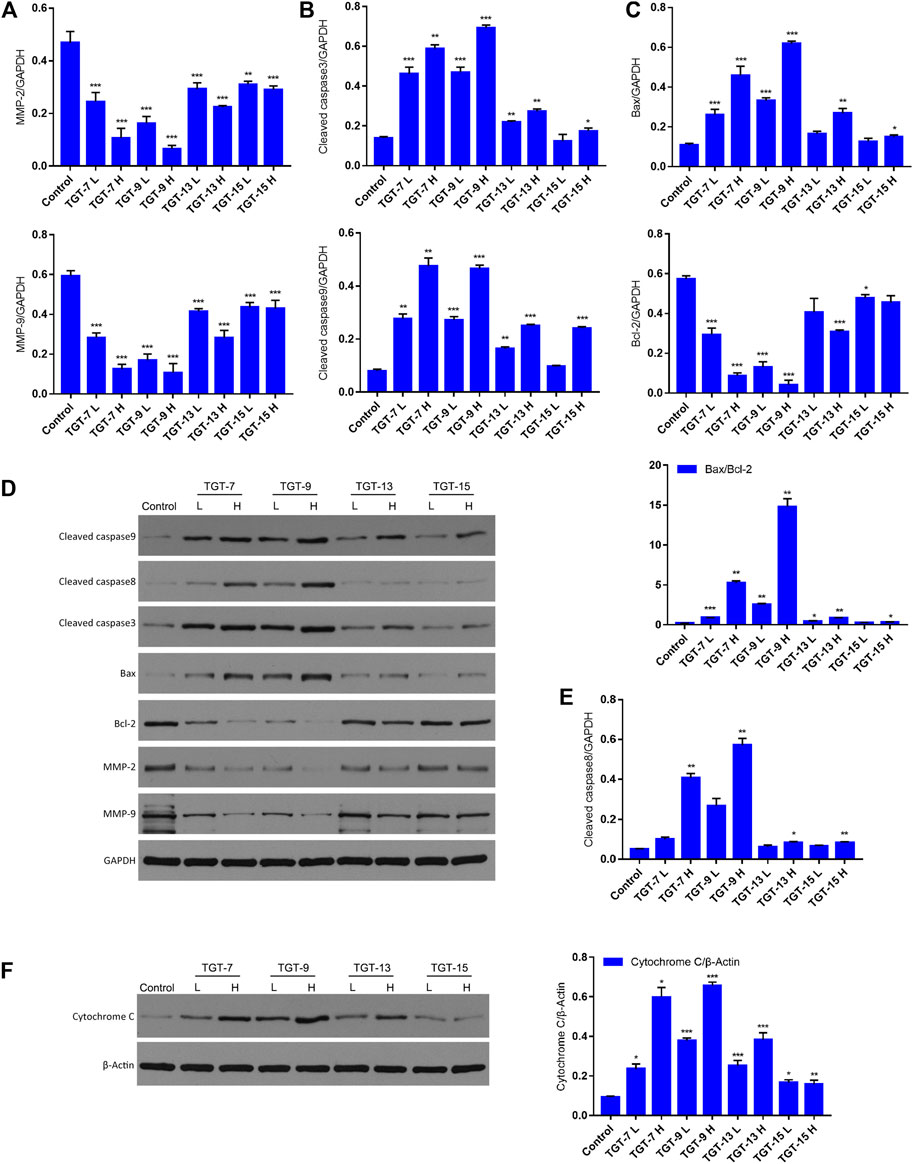
FIGURE 14. TGT-7, TGT-9, TGT-13, and TGT-15 modulate migration and expression of apoptosis-related key proteins (A) The histograms show MMP2 and MMP9 expression (B) Histograms showing cleaved caspase-3 and cleaved caspase-9 expression (C) Histograms showing Bax and Bcl-2 expression (D) Migration and apoptosis-related key proteins were analyzed by Western blotting using GAPDH for data normalization (E) Histograms showing cleaved caspase-8 expression (F) Expression of cytochrome C analyzed by Western blotting and using β-Actin for data normalization. These results were obtained from three independent experiments, and all of the data are expressed as the mean ± SD, *p < 0.05, **p < 0.01, ***p < 0.001 vs. the control group (n = 3).
Discussion
Marsdeniae tenacissimae Caulis extract (Xiao-Ai-Ping) has been clinically used in the treatment of malignant tumors, such as liver cancer, leukemia, lung cancer, etc. Some scholars have specifically used the MTT method to detect the effect of extracts of TGT on the proliferation of normal human lymphocyte cells induced by concanavalin A (ConA) and lipopolysaccharide (LPS). Results have shown that TGT extracts have no obvious cytotoxic effects on normal immune cells and hematopoietic stem cells in vitro, but can promote the proliferation of T and B cells, which were closely related to tumor patients’ immunity capacity against the aforementioned diseases (Chen et al., 2010; Wang et al., 2017; Zheng et al., 2017; Zhan et al., 2019). Studies have found that C21 steroidal glycosides in Marsdeniae tenacissimae Caulis were the main active components. Intriguingly, extensive research demonstrated that C21 steroidal glycosides harbor anti-tussive, anti-asthma, and antitumor activities (Pang et al., 2018; Wang et al., 2018).
Our preliminary research showed the ethyl acetate extracted from a TGT extract had the strongest growth inhibition against A549 cells in vitro. We also demonstrated the anti-tumor activity of this extract in vivo. Results from others showed that LLC tumor-bearing male C57BL/6 mice in a cisplatin group lost more weight than others, indicating M. tenacissima extracts did not cause severe side effects while reducing tumor size (Xu, 2018; Hu et al., 2020). Therefore, this study was aimed at separating the components of an ethyl acetate fraction in order to identify compounds with strong anti-NSCLC activity.
First, we isolated and purified the ethyl acetate part of Marsdeniae tenacissimae Caulis. A total of 19 compounds were isolated from an ethyl acetate fraction, 15 of which were C21 steroidal glycosides (Figure 1). At the same time, we elucidated their structures, which included 2 novel compounds, namely, 11α-O-benzoyl-12β-O-tigloyltenacigenin B 15) (Figure 2, Table 1) and sodium 5-hydroxy-4-( ( (2S,3R,4S,5R,6R)-5-hydroxy-3-(((2R,3R,4R,5R,6S)-5-hydroxy-6-((2-hydroxy-4,6-dimethoxy-6-oxohexan-3-yl)oxy)-4-methoxy-2-methyltetrahydro-2H-pyran-3-yl)oxy)-4-methoxy-6-methyltetrahydro-2H-pyran-2-yl)oxy)-3-methoxyhexanoate 18) (Figure 3, Table 1), and 17 known compounds (1–14, 17, 19, 20) (Figure 4, Supplementary Tables S1,S2). Among all the isolated monomeric compounds, most were C21 steroids. These findings indicated that C21 steroidal saponins might be the effective anti-tumor components of Marsdeniae tenacissimae Caulis.
Second, we evaluated all of the isolated compounds against A549 cells using an in vitro assay (Table 2). The results indicated that the six types of steroidal saponins had inhibitory effects against A549 cells in vitro, as their IC50 values were less than 100 µm. At the same time, we observed that A549 cells were sensitive to these four C21 steroidal glycosides in a dose-dependent manner after 24 h of drug stimulation (Xu, 2018). Moreover, we assessed the cytotoxic effect of the four C21 steroidal glycosides against BEAS-2B normal human pulmonary epithelial cells using trypan blue dye exclusion assay, and the results showed that they had no obvious cytotoxicity against BEAS-2B cells.
Next, we analyzed the structure–activity relationships of these isolated compounds. Their structures were very similar, but their activities varied widely in A549 cells in vitro (Table 2). The main differences in their structure were due to 11-position and 12-position substituents. When the 12-position was acetyl-substituted, these compounds were essentially inactive in A549 cells in vitro, such as compounds 1, 2, 3, 4, and 19. When the 12-position was substituted by benzoyl, they had the best activity, such as compounds 7, 9, and 13, followed by methylcrotonyl substitution (compounds 8, 10, and 15). Therefore, we postulated that the steroidal compounds that acted on the activity of A549 cells in vitro were mainly C-12 substituents, and when benzoyl was substituted, the inhibitory effect against A549 cells was the strongest, whereas the effect of the sugar chain was minimal. Differences in activity were attributable to variations in substituents at the C-12 position. This finding might be further utilized as a reference for structural modifications to identify active compounds.
Traditional Chinese medicine (TCM) network pharmacology is a novel research approach that predicts target profiles as well as the pharmacological actions of various herbal compounds and identifies drug–gene–disease comodule correlations to determine the integrated rules and network regulatory effects of different herbal formulae (Wu et al., 2016). This provides a new paradigm for elucidating the pharmacodynamic substance basis and unraveling the mechanisms of action of TCM (Lee et al., 2019; Zhang et al., 2019).
In order to better determine the best anti-NSCLC substances and potential mechanisms of M. tenacissimae, we preliminarily used network pharmacology to screen six active compounds. Network pharmacological analysis showed that steroidal saponins imparted anti-cancer effects mainly via 18 targets that were closely related to the PI3K/AKT, RAS/RAF/MEK/ERK, VEGF, and MAPK signaling pathways. These pathways were associated with angiogenesis, cell cycle change, migration, invasion, and cancer cell apoptosis (Figures 6B, 7A). According to the size of the node and degree in the network of the NSCLC-compound-target and PPI (Figure 7B), we determined the anti-NSCLC effect of a compound and the importance of a protein in the development of NSCLC so as to provide references for follow-up research focused on deepening our understanding of this mechanism.
One of the major goals of cancer treatment is to disrupt tumor cell proliferation via cell cycle progression blockage (Evan and Vousden, 2001; Wang et al., 2020). Currently, a number of chemotherapeutic drugs can block tumor cells in the G0/G1, S, or G2/M phases and thus can achieve the aim of inhibiting tumor cell proliferation (Schwartz and Shah, 2005; Chen et al., 2016). Based on the results of literature research and our above network pharmacological analysis, our experiment demonstrated that the four C21 steroidal glycosides (TGT-7, TGT-9, TGT-13, and TGT-15) also blocked A549 cells at the G0/G1 phase and prevented cells from progressing toward the S (DNA replication) and M (cell division) phases, as well as decreased the rates of cell growth and proliferation (Figure 10). Cell proliferation was closely linked to the cell cycle, which normally operates in an orderly manner under the supervision of cell cycle-related genes, and thus cancer occurs when cell cycle errors cause cells to proliferate (Yang et al., 2008).
MMP-2 and MMP-9 have been strongly linked to angiogenesis, invasion, and metastasis in tumor cells (Webb et al., 2017). Our study illustrated that four C21 steroidal glycosides could decrease the expression of MMP-2 and MMP-9 in A549 cells. Moreover, with increasing concentration, their expression slowly decreased in a dose-dependent manner (Figures 14A,D).
Apoptosis induction is considered an essential mechanism of antitumor therapeutics (Stepczynska et al., 2001). Previous studies have revealed that anticancer agents impart anti-proliferative effects using two distinct apoptosis pathways that involve mitochondria or death receptors (Kuo et al., 2018; Han et al., 2019). Specifically, the mitochondria-associated pathway is a classic intrinsic pathway that is caused by ROS overproduction, which in turn results in the depletion of △φin (Shi et al., 2014; Wang et al., 2019). Generally, various protein molecules participate in regulating the mitochondrial apoptotic pathway such as pro-apoptotic members (Bax and Bad) and anti-apoptotic members (Bcl-2 and Bcl-xl) (Dong et al., 2019). Moreover, the pathway activated specific pivotal proteinases such as initiator caspase-9 and effector caspase-3 and subsequently resulted in DNA fragmentation as well as nuclear PARP degradation during apoptosis (Duangprompo et al., 2016; Chao et al., 2018). Therefore, Annexin V-FITC/PI staining was conducted to detect apoptotic alterations that may be related to A549 cells cytotoxicity. We detected changes in fluorescence intensities after A549 cells were stained by Annexin V-FITC/PI and observed that the four C21 steroidal glycosides influenced A549 cells apoptosis. In this experiment, we discovered that the rate of cell apoptosis increased in the drug treatment group as measured by flow cytometry and was positively correlated with the drug concentration. Therefore, the results indicated that the four C21 steroidal glycosides could effectively promote A549 cells apoptosis (Figure 11).
Mitochondria serve as the regulatory center of endogenous pathways of apoptosis. Mitochondrial status can be interrogated by measuring the mitochondrial membrane potential (Green and Reed, 1998). Based on this point, our experimental results showed that the four C21 steroidal glycosides could reduce the mitochondrial membrane potential, indicating that this might induce A549 apoptosis via endogenous pathways of the mitochondria (Figure 12). ROS are mainly produced in the mitochondria, and ROS overproduction could lead to lipid overoxidation of the mitochondrial membrane, further influencing the mitochondrial membrane potential and triggering the release of cytochrome C, which, in turn, induces endogenous apoptosis (Zhao and Xu, 2001). Some studies have shown that ROS can also cause exogenous apoptosis mainly by increasing the sensitivity of tumor cells to Fasl, then activating caspase-8 to mediate exogenous apoptosis through the death receptor Fas/Fasl pathway (Shang et al., 2016). In our experiment, the effect of the four C21 steroidal glycosides on the ROS level was assessed in A549 cells. The experimental results indicated that the green fluorescence of every drug group was significantly more intense relative to the control group after TGT-7, TGT-9, TGT-13, and TGT-15 treatment. Thus, this experiment clearly showed that TGT-7, 9, 13, and 15 could raise the level of ROS in A549 cells and then induce cell apoptosis (Figure 13).
The cytochrome C-mediated mitochondrial apoptosis pathway is controlled by the Bcl-2 protein family, and in terms of apoptosis, the Bcl-2 family consists of two members, anti-apoptotic protein (Bcl-2) and pro-apoptotic protein (Bax). The ratio of these two is usually an indicator of apoptosis (Adams and Cory, 1998; Li et al., 2017). In our studies, the expression level of Bax increased with increasing concentrations of TGT-7, TGT-9, TGT-13, and TGT-15, whereas the Bcl-2 expression level showed the opposite pattern, i.e., it was negatively correlated with dose: an increase in the Bax/Bcl-2 ratio induced the release of cytochrome C that in turn induced cell apoptosis (Figures 14B–D,F). Caspase-8 is a major apoptosis factor in the death receptor pathway (Munoz-Pinedo and Lopez-Rivas, 2018). The four C21 steroidal glycosides at high concentrations could increase cleaved caspase-8 protein expression, suggesting that the four C21 steroidal glycosides could induce apoptosis via the death receptor pathway in addition to the mitochondrial pathway (Figures 14D,E).
Conclusion
In summary, we first isolated and characterized 19 major constituents of Marsdeniae tenacissimae Caulis by 1H-NMR, 13C-NMR and DEPT, 2D NMR (1H-1HCOSY NOESY, HSQC and HMBC) spectra. Then, we demonstrated that the six main active components of ethyl acetate dramatically suppressed A549 cancer cell proliferation. Furthermore, network pharmacology analysis of the six compounds of Marsdeniae tenacissimae Caulis revealed that possible targets were mainly related to the positive regulation of ROS-associated metabolic processes, as well as intrinsic apoptotic pathways. Next, a series of cellular tests verified the results of the network pharmacology prediction. The four C21 steroidal glycosides (TGT-7, TGT-9, TGT-13, and TGT-15) disrupted A549 cells migration and invasion via downregulation of MMP-2 and MMP-9 expression. We also found that C21 steroidal glycosides of Marsdeniae tenacissimae Caulis triggered apoptosis of A549 cells through a mitochondrial-mediated pathway via upregulation of Bax and downregulation of Bcl-2 expression, thus releasing cytochrome C and finally activating caspase-3 and caspase-9. At the same time, the four C21 steroidal glycosides also activated caspase-8, which activated the death receptor pathway to promote apoptosis. The four C21 steroidal glycosides disrupted A549 growth and triggered apoptosis via mitochondrial and death receptor pathways (Figure 15).
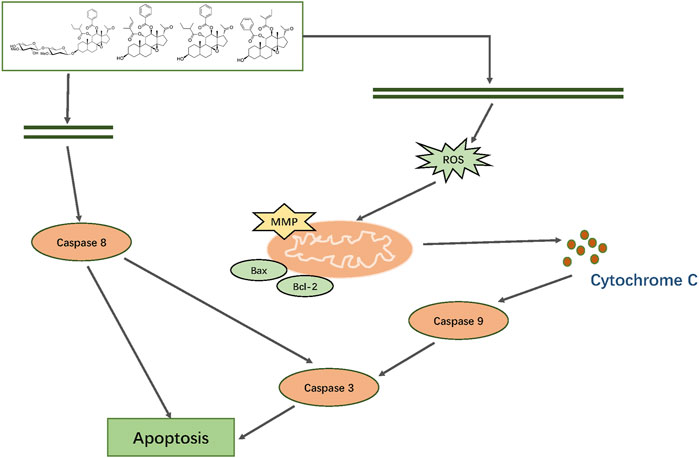
FIGURE 15. Mechanism by which TGT-7, TGT-9, TGT-13, and TGT-15 isolated from Marsdeniae tenacissimae disrupt A549 cells growth and induce apoptosis via mitochondrial and death receptor pathways.
At present, there are many studies on the treatment of cancer by Marsdeniae tenacissimae Caulis and its clinical preparation Xiao-Ai-Ping injection, but these studies have not excavated its pharmacodynamic material basis. In our research, we have defined the antitumor compounds as well as their mechanisms, which can be potentially employed as a therapeutic option for the treatment alone of NSCLC or in combination with anticancer chemical drugs to reduce their toxicity and side effects. In addition, we also analyzed the structure-activity relationship of these isolated compounds, which provides experimental basis for the development of clinical anticancer drugs or to improve the clinical efficacy of existing anticancer chemical drugs by structural modification in the future. Due to the approaching completion time, the relevant targets and pathways predicted by the network pharmacology in this study on the anti-NSCLC caused by M. tenacissima have not been fully verified, which will be studied in our later research.
Data Availability Statement
All datasets generated for this study are included in the article/Supplementary Material.
Ethics Statement
The animal experiment research related to this subject has obtained ethical certification, but this research does not involve animal experiments.
Author Contributions
PL performed the experiment and wrote the essay. D-WX and R-TL processed the data. S-HW and Y-LH modified the paper. J-YL, L-WK, S-YS, and Y-HH assisted the completion of the experiment. T-XL designed the experiment and provided financial support.
Funding
The National Natural Science Foundation of China (No. 81973977) and the Creative Item of Innovative Team of Ministry of Education (No. IRT-13R63) supported this study.
Conflict of Interest
The authors declare that the research was conducted in the absence of any commercial or financial relationships that could be construed as a potential conflict of interest.
Acknowledgments
We thank LetPub (www.letpub.com) for its linguistic assistance during the preparation of this manuscript.
Supplementary Material
The Supplementary Material for this article can be found online at: https://www.frontiersin.org/articles/10.3389/fphar.2021.518406/full#supplementary-material.
Footnotes
1https://www.ncbi.nlm.nih.gov/pubmed
4https://metlin.scripps.edu/landing_page.php?pgcontent=mainPage
5https://sso.cas.org/as/kNab3/resume/as/authorization.ping
6http://www.swisstargetprediction.ch/
10https://www.genome.jp/kegg/ligand.html
11https://david.ncifcrf.gov/relatedGenes.jsp
References
Adams, J. M., and Cory, S. (1998). The Bcl-2 protein family: arbiters of cell survival. Science 281 (5381), 1322–1326. doi:10.1126/science.281.5381.1322
Amberger, J. S., Bocchini, C. A., Schiettecatte, F., Scott, A. F., and Hamosh, A. (2015). OMIM.org: online Mendelian Inheritance in Man (OMIM), an online catalog of human genes and genetic disorders. Nucleic Acids Res. 43 (Database issue), D789–D798. doi:10.1093/nar/gku1205
Chao, T.-L., Wang, T.-Y., Lee, C.-H., Yiin, S.-J., Ho, C.-T., Wu, S.-H., et al. (2018). Anti-cancerous effect of inonotus taiwanensis polysaccharide extract on human acute monocytic leukemia cells through ROS-independent intrinsic mitochondrial pathway. Int. J. Mol. Sci. 19 (2), 393. doi:10.3390/ijms19020393
Chen, B., Chen, L., J, O., and X, S. (2010). Effect of extractive of marsdenia tenacissima on human normal immunocytes and hemopoietic stem cells in vitro. Chin. Clin. Oncol. 15 (10), 887–890.
Chen, F., Zheng, S. L., Hu, J. N., Sun, Y., He, Y. M., Peng, H., et al. (2016). Octyl ester of ginsenoside Rh2 induces apoptosis and G1 cell cycle arrest in human HepG2 cells by activating the extrinsic apoptotic pathway and modulating the akt/p38 MAPK signaling pathway. J. Agric. Food Chem. 64 (40), 7520–7529. doi:10.1021/acs.jafc.6b03519
Commission, C. P. (2020). “Pharmacopoeia of the People's Republic of China (Part I)”, in: Marsdeniae Tenacissimae Caulis. (Beijing: The Medicine Science and Technology Press of China).
Daina, A., Michielin, O., and Zoete, V. (2019). SwissTargetPrediction: updated data and new features for efficient prediction of protein targets of small molecules. Nucleic Acids Res. 47 (W1), W357–w364. doi:10.1093/nar/gkz382
Deng, J., Liao, Z., and Chen, D. (2005). Marsdenosides A-H, polyoxypregnane glycosides from Marsdenia tenacissima. Phytochemistry 66 (9), 1040–1051. doi:10.1016/j.phytochem.2005.03.018
DeSantis, C. E., Siegel, R. L., Sauer, A. G., Miller, K. D., Fedewa, S. A., Alcaraz, K. I., et al. (2016). Cancer statistics for African Americans, 2016: progress and opportunities in reducing racial disparities. CA: A Cancer J. Clinicians 66 (4), 290–308. doi:10.3322/caac.21340
Dong, Y., and Cui, M. (2013). Current situation and development trend of Chinese medicine information research. Zhongguo Zhong Xi Yi Jie He Za Zhi 33 (4), 559–564.
Dong, Y., Qiu, P., Zhu, R., Zhao, L., Zhang, P., Wang, Y., et al. (2019). A combined phytochemistry and network pharmacology approach to reveal the potential antitumor effective substances and mechanism of phellinus igniarius. Front. Pharmacol. 10, 266. doi:10.3389/fphar.2019.00266
Duangprompo, W., Aree, K., Itharat, A., and Hansakul, P. (2016). Effects of 5,6-dihydroxy-2,4-dimethoxy-9,10-dihydrophenanthrene on G2/M cell cycle arrest and apoptosis in human lung carcinoma cells. Am. J. Chin. Med. 44 (7), 1473–1490. doi:10.1142/S0192415X16500828
Evan, G. I., and Vousden, K. H. (2001). Proliferation, cell cycle and apoptosis in cancer. Nature 411 (6835), 342–348. doi:10.1038/35077213
Fang, J., Wang, L., Wu, T., Yang, C., Gao, L., Cai, H., et al. (2017). Network pharmacology-based study on the mechanism of action for herbal medicines in Alzheimer treatment. J. Ethnopharmacol. 196, 281–292. doi:10.1016/j.jep.2016.11.034
Gao, Y., Chen, S., Sun, J., Su, S., Yang, D., Xiang, L., et al. (2020). Traditional Chinese medicine may be further explored as candidate drugs for pancreatic cancer: a review. Phytotherapy Res. 35 (2), 603–628. doi:10.1002/ptr.6847
Gfeller, D., Grosdidier, A., Wirth, M., Daina, A., Michielin, O., and Zoete, V. (2014). SwissTargetPrediction: a web server for target prediction of bioactive small molecules. Nucleic Acids Res. 42 (W1), W32–W38. doi:10.1093/nar/gku293
Green, D. R., and Reed, J. C. (1998). Mitochondria and apoptosis. Science 281 (5381), 1309–1312. doi:10.1126/science.281.5381.1309
Gu, X., Zheng, R., Xia, C., Zeng, H., Zhang, S., Zou, X., et al. (2018). Interactions between life expectancy and the incidence and mortality rates of cancer in China: a population-based cluster analysis. Cancer Commun. 38 (1), 44. doi:10.1186/s40880-018-0308-x
Guijas, C., and Siuzdak, G. (2018). Reply to comment on metlin: a technology platform for identifying knowns and unknowns. Anal. Chem. 90 (21), 13128–13129. doi:10.1021/acs.analchem.8b04081
Han, K. W. W., Po, W. W., Sohn, U. D., and Kim, H.-J. (2019). Benzyl isothiocyanate induces apoptosis via reactive oxygen species-initiated mitochondrial dysfunction and DR4 and DR5 death receptor activation in gastric adenocarcinoma cells. Biomolecules 9 (12), 839. doi:10.3390/biom9120839
Hare, J. I., Lammers, T., Ashford, M. B., Puri, S., Storm, G., and Barry, S. T. (2017). Challenges and strategies in anti-cancer nanomedicine development: an industry perspective. Adv. Drug Deliv. Rev. 108, 25–38. doi:10.1016/j.addr.2016.04.025
Hu, Y., Liu, P., Kang, L., Li, J., Li, R., and Liu, T. (2020). Mechanism of Marsdenia tenacissima extract promoting apoptosis of lung cancer by regulating Ca2+/CaM/CaMK signaling. J. Ethnopharmacol. 251, 112535. doi:10.1016/j.jep.2019.112535
Kim, J., Kim, J.-j., and Lee, H. (2017). An analysis of disease-gene relationship from Medline abstracts by DigSee. Sci. Rep. 7 (1), 40154. doi:10.1038/srep40154
Kuhn, M., Szklarczyk, D., Franceschini, A., Campillos, M., von Mering, C., Jensen, L. J., et al. (2010). Stitch 2: an interaction network database for small molecules and proteins. Nucleic Acids Res. 38 (suppl_1), D552–D556. doi:10.1093/nar/gkp937
Kuo, H.-M., Tseng, C.-C., Chen, N.-F., Tai, M.-H., Hung, H.-C., Feng, C.-W., et al. (2018). MSP-4, an antimicrobial peptide, induces apoptosis via activation of extrinsic fas/FasL- and intrinsic mitochondria-mediated pathways in one osteosarcoma cell line. Mar. Drugs 16 (1), 8. doi:10.3390/md16010008
Lee, W.-Y., Lee, C.-Y., Kim, Y.-S., and Kim, C.-E. (2019). The methodological trends of traditional herbal medicine employing network pharmacology. Biomolecules 9 (8), 362. doi:10.3390/biom9080362
Li, H., Lv, B., Kong, L., Xia, J., Zhu, M., Hu, L., et al. (2017). Nova1 mediates resistance of rat pheochromocytoma cells to hypoxia-induced apoptosis via the Bax/Bcl-2/caspase-3 pathway. Int. J. Mol. Med. 40 (4), 1125–1133. doi:10.3892/ijmm.2017.3089
Li, R., and Sun, H. (2008). Studies on the chemical constituents and bioactivities of five Schisandra medicina lspecies and Elsholtzia bodinier. J. Graduate Sch. Chin. Acad. Sci. (04), 569–575.
Liu, M., and Kong, J.-Q. (2018). The enzymatic biosynthesis of acylated steroidal glycosides and their cytotoxic activity. Acta Pharmaceutica Sinica B 8 (6), 981–994. doi:10.1016/j.apsb.2018.04.006
Mao, Y., Hao, J., Jin, Z.-Q., Niu, Y.-Y., Yang, X., Liu, D., et al. (2017). Network pharmacology-based and clinically relevant prediction of the active ingredients and potential targets of Chinese herbs in metastatic breast cancer patients. Oncotarget 8 (16), 27007–27021. doi:10.18632/oncotarget.15351
Muñoz-Pinedo, C., and López-Rivas, A. (2018). A role for caspase-8 and TRAIL-R2/DR5 in ER-stress-induced apoptosis. Cell Death Differ 25 (1), 226. doi:10.1038/cdd.2017.155
Paci, E., Puliti, D., Lopes Pegna, A., Carrozzi, L., Picozzi, G., Falaschi, F., et al. (2017). Mortality, survival and incidence rates in the ITALUNG randomised lung cancer screening trial. Thorax 72 (9), 825–831. doi:10.1136/thoraxjnl-2016-209825
Pang, X., Kang, L.-P., Fang, X.-M., Yu, H.-S., Han, L.-F., Zhao, Y., et al. (2018). C21 steroid derivatives from the Dai herbal medicine Dai-Bai-Jie, the dried roots of Marsdenia tenacissima, and their screening for anti-HIV activity. J. Nat. Med. 72 (1), 166–180. doi:10.1007/s11418-017-1126-1
Pinero, J., Queralt-Rosinach, N., Bravo, A., Deu-Pons, J., Bauer-Mehren, A., Baron, M., et al. (2015). DisGeNET: a discovery platform for the dynamical exploration of human diseases and their genes. Database (Oxford) 2015 (0), bav028. doi:10.1093/database/bav028
Schwartz, G. K., and Shah, M. A. (2005). Targeting the cell cycle: a new approach to cancer therapy. J. Clin. Oncol. 23 (36), 9408–9421. doi:10.1200/jco.2005.01.5594
Settle, B., Otasek, D., Morris, J. H., and Demchak, B. (2018). Copycat layout: network layout alignment via Cytoscape automation. F1000Res 7, 822. doi:10.12688/f1000research.15144.2
Shang, H.-S., Shih, Y.-L., Lu, T.-J., Lee, C.-H., Hsueh, S.-C., Chou, Y.-C., et al. (2016). Benzyl isothiocyanate (BITC) induces apoptosis of GBM 8401 human brain glioblastoma multiforms cells via activation of caspase-8/Bid and the reactive oxygen species-dependent mitochondrial pathway. Environ. Toxicol. 31 (12), 1751–1760. doi:10.1002/tox.22177
Shi, H., Cui, J., Guan, A., and Zhao, Y. (2008). Chemical constituents of Marsdenia tenacissima. Chin. Traditional Herbal Drugs 39 (07), 970–972.
Shi, Y.-L., Feng, S., Chen, W., Hua, Z.-C., Bian, J.-J., and Yin, W. (2014). Mitochondrial inhibitor sensitizes non-small-cell lung carcinoma cells to TRAIL-induced apoptosis by reactive oxygen species and Bcl-XL/p53-mediated amplification mechanisms. Cell Death Dis 5 (12), e1579. doi:10.1038/cddis.2014.547
Stepczynska, A., Lauber, K., Engels, I. H., Janssen, O., Kabelitz, D., Wesselborg, S., et al. (2001). Staurosporine and conventional anticancer drugs induce overlapping, yet distinct pathways of apoptosis and caspase activation. Oncogene 20 (10), 1193–1202. doi:10.1038/sj.onc.1204221
Tang, J., Feng, Y., Tsao, S., Wang, N., Curtain, R., and Wang, Y. (2009). Berberine and Coptidis rhizoma as novel antineoplastic agents: a review of traditional use and biomedical investigations. J. Ethnopharmacol. 126 (1), 5–17. doi:10.1016/j.jep.2009.08.009
Torre, L. A., Bray, F., Siegel, R. L., Ferlay, J., Lortet-Tieulent, J., and Jemal, A. (2015). Global cancer statistics, 2012. CA: A Cancer J. Clinicians 65 (2), 87–108. doi:10.3322/caac.21262
Wang, F., Fan, Q. X., Wang, H. H., Han, D. M., Song, N. S., and Lu, H. (2017). Efficacy and safety of Xiaoaiping combined with chemotherapy in the treatment of advanced esophageal cancer. Zhonghua Zhong Liu Za Zhi 39 (6), 453–457. doi:10.3760/cma.j.issn.0253-3766.2017.06.010
Wang, K., Gong, Q., Zhan, Y., Chen, B., Yin, T., Lu, Y., et al. (2019). Blockage of autophagic flux and induction of mitochondria fragmentation by paroxetine hydrochloride in lung cancer cells promotes apoptosis via the ROS-MAPK pathway. Front. Cel Dev. Biol. 7, 397. doi:10.3389/fcell.2019.00397
Wang, Q., Cao, J., Wang, P., and Ge, M. (2015). Antitumor effect of C21 steroidal glycosides on adenoid cystic carcinoma cell line SACC83. Clin. Lab. 61 (10), 1553–1560. doi:10.7754/clin.lab.2015.141218
Wang, X., Chang, H., Gao, G., Su, B., Deng, Q., Zhou, H., et al. (2020). Silencing of PRDM5 increases cell proliferation and inhibits cell apoptosis in glioma. Int. J. Neurosci. 131 (2), 144–153. doi:10.1080/00207454.2020.1733563
Wang, X., Yan, Y., Chen, X., Zeng, S., Qian, L., Ren, X., et al. (2018). The antitumor activities of Marsdenia tenacissima. Front. Oncol. 8, 473. doi:10.3389/fonc.2018.00473
Webb, A. H., Gao, B. T., Goldsmith, Z. K., Irvine, A. S., Saleh, N., Lee, R. P., et al. (2017). Inhibition of MMP-2 and MMP-9 decreases cellular migration, and angiogenesis in in vitro models of retinoblastoma. BMC Cancer 17 (1), 434. doi:10.1186/s12885-017-3418-y
Wu, C. W., Lu, L., Liang, S. W., Chen, C., and Wang, S. M. (2016). Application of drug-target prediction technology in network pharmacology of traditional Chinese medicine. Zhongguo Zhong Yao Za Zhi 41 (3), 377–382. doi:10.4268/cjcmm20160303
Xu, D. W. (2018). Study on the substance basis and mechanism of Marsdenia tenacissima regulating apoptosis and inhibiting the growth and metastasis of A549 cells. PhD Thesis. Beijing: Minzu University of China
Yang, C., Przyborski, S., Cooke, M. J., Zhang, X., Stewart, R., Anyfantis, G., et al. (2008). A key role for telomerase reverse transcriptase unit in modulating human embryonic stem cell proliferation, cell cycle dynamics, and in vitro differentiation. Stem Cells 26 (4), 850–863. doi:10.1634/stemcells.2007-0677
Yao, S., To, K. K.-W., Wang, Y.-Z., Yin, C., Tang, C., Chai, S., et al. (2014). Polyoxypregnane steroids from the stems of Marsdenia tenacissima. J. Nat. Prod. 77 (9), 2044–2053. doi:10.1021/np500385b
Ye, B., Yang, J., Li, J., Niu, T., and Wang, S. (2014). In vitro and in vivo antitumor activities of tenacissoside C from Marsdenia tenacissima. Planta Med. 80 (1), 29–38. doi:10.1055/s-0033-1360128
Zhan, J., Shi, L.-l., Wang, Y., Wei, B., and Yang, S.-l. (2019). In Vivo study on the effects of xiaoaiping on the stemness of hepatocellular carcinoma cells. Evidence-Based Complement. Altern. Med. 2019, 1–10. doi:10.1155/2019/4738243
Zhang, H., Tan, A.-M., Zhang, A.-Y., Chen, R., Yang, S.-B., and Huang, X. (2010). Five new C21 steroidal glycosides from the stems of Marsdenia tenacissima. Steroids 75 (2), 176–183. doi:10.1016/j.steroids.2009.11.003
Zhang, R., Zhu, X., Bai, H., and Ning, K. (2019). Network pharmacology databases for traditional Chinese medicine: review and assessment. Front. Pharmacol. 10, 123. doi:10.3389/fphar.2019.00123
Zhao, Y. G., and Xu, J. X. (2001). Mitochondria, reactive oxygen species and apoptosis. Prog. Biochem. Biophys. 28 (2).
Keywords: Marsdeniae tenacissimae, C21 steroidal saponins, network pharmacology, migration, invasion, apoptosis, A549 cells, NSCLC
Citation: Liu P, Xu D-W, Li R-T, Wang S-H, Hu Y-L, Shi S-Y, Li J-Y, Huang Y-H, Kang L-W and Liu T-X (2021) A Combined Phytochemistry and Network Pharmacology Approach to Reveal Potential Anti-NSCLC Effective Substances and Mechanisms in Marsdenia tenacissima (Roxb.) Moon (Stem). Front. Pharmacol. 12:518406. doi: 10.3389/fphar.2021.518406
Received: 27 March 2020; Accepted: 12 February 2021;
Published: 29 April 2021.
Edited by:
Francisco Estévez, University of Las Palmas de Gran Canaria, SpainReviewed by:
Chee Wun How, Monash University Malaysia, MalaysiaJuan Francisco Leon, University of South Carolina, United States
Copyright © 2021 Liu, Xu, Li, Wang, Hu, Shi, Li, Huang, Kang and Liu. This is an open-access article distributed under the terms of the Creative Commons Attribution License (CC BY). The use, distribution or reproduction in other forums is permitted, provided the original author(s) and the copyright owner(s) are credited and that the original publication in this journal is cited, in accordance with accepted academic practice. No use, distribution or reproduction is permitted which does not comply with these terms.
*Correspondence: Tong-Xiang Liu, dG9uZ3hsaXUxMjNAaG90bWFpbC5jb20=