- 1Department of Pharmacy, The Second Xiangya Hospital, Central South University, Changsha, China
- 2Xiangya School of Pharmaceutical Sciences, Central South University, Changsha, China
- 3Department of Cancer Biology and Toxicology, Department of Pharmacology, College of Medicine, Markey Cancer Center, University of Kentucky, Lexington, KY, United States
DNA repair pathways are triggered to maintain genetic stability and integrity when mammalian cells are exposed to endogenous or exogenous DNA-damaging agents. The deregulation of DNA repair pathways is associated with the initiation and progression of cancer. As the primary anti-cancer therapies, ionizing radiation and chemotherapeutic agents induce cell death by directly or indirectly causing DNA damage, dysregulation of the DNA damage response may contribute to hypersensitivity or resistance of cancer cells to genotoxic agents and targeting DNA repair pathway can increase the tumor sensitivity to cancer therapies. Therefore, targeting DNA repair pathways may be a potential therapeutic approach for cancer treatment. A better understanding of the biology and the regulatory mechanisms of DNA repair pathways has the potential to facilitate the development of inhibitors of nuclear and mitochondria DNA repair pathways for enhancing anticancer effect of DNA damage-based therapy.
The DNA Repair Pathways
A variety of endogenous and exogenous DNA-damaging agents such as UV light, ionizing radiation (IR) and chemotherapeutic agents can lead to DNA lesions, including mismatches, single-strand breaks (SSBs), double-strand breaks (DSBs), chemical modifications of the bases or sugars, and interstrand or intrastrand cross-links. If the damage is not corrected, it will cause genomic instability and mutation, which is one of the cancer hallmarks (Hanahan and Weinberg, 2011). In order to prevent this situation, cells have evolved a series of mechanisms called DNA damage response (DDR) in order to deal with such lesions. DDR is a complex network that functions in different ways to target various DNA lesions, including signal transduction, transcriptional regulation, cell-cycle checkpoints, induction of apoptosis, damage tolerance processes, and multiple DNA repair pathways (Figure 1) (Giglia-Mari et al., 2011; Tian et al., 2015).
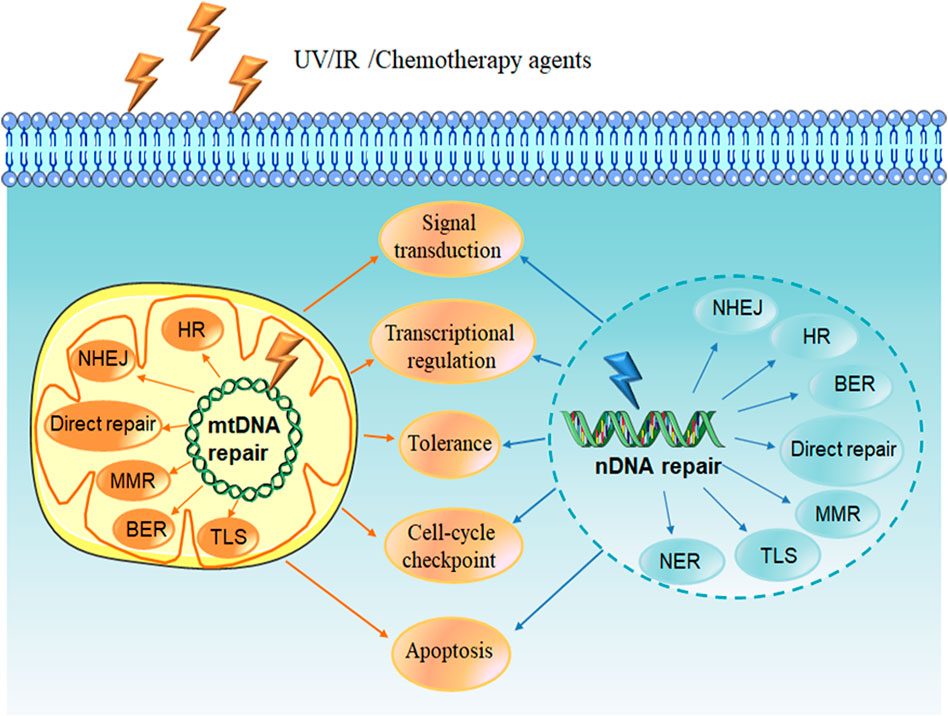
FIGURE 1. DNA damage response. DNA damage is caused by endogenous agent oxygen species (ROS) or exogenous agents such as UV light, ionizing radiation (IR) and chemotherapy agents. DNA damage response (DDR) is induced to deal with the lesions, including signal transduction, transcriptional regulation, cell-cycle checkpoints, induction of apoptosis, multiple DNA repair pathways as well as damage tolerance processes. DNA repair pathways include nuclear and mitochondrial DNA repair pathways. Direct repair, BER, MMR and recombinational repair (HR and NHEJ) are existence in both nuclear and mitochondrial repair systems. NER has been reported only appearance in nucleus, and the existence of TLS pathway in mitochondria is unknown. NDNA, nuclear DNA; MtDNA, mitochondrial DNA; BER, base excision repair; HR, homologous recombination repair; NHEJ, non-homologous end joining; MMR, mismatch repair; TLS, translesion synthesis; NER, nucleotide excision repair.
In mammalian cells, the two main organelles containing DNA are nucleus and mitochondria. Nuclear DNA (nDNA) repair systems are divided into the following major pathways: 1) direct reversal, which mainly repairs the lesion induced by alkylating agents, 2) base excision repair (BER), aiming at DNA breaks (SSBs) and non-bulky impaired DNA bases, 3) nucleotide excision repair (NER), correcting bulky, helix-distorting DNA lesions, 4) mismatch repair (MMR), repair of insertion/deletion loops (IDLs) and base-base mismatch, 5) recombinational repair, which is further divided into homologous recombination repair (HRR) and non-homologous end joining (NHEJ), primarily functioning at DNA double strand breaks, 6) alternative nonhomologous end joining (alt-NHEJ, MMEJ), involved in repair of DSBs, 7) translesion synthesis (TLS), which is more likely to be a DNA damage tolerance mechanism (Jackson and Bartek, 2009; Hosoya and Miyagawa, 2014). Mitochondrial DNA (mtDNA) repair pathways, including the direct reversal, BER, MMR, TLS and double-strand break repair (DSBR), can repair damaged DNA to maintain mitochondria genetic integrity, protect mtDNA against oxidative damage, and promote cell survival (Ohta, 2006; Saki and Prakash, 2017).
Role of DNA Repair Pathways in Cancer Biology
DNA repair pathways play an important role in the maintenance of genome stability and integrity through correcting the impaired DNA that may contribute to carcinogenesis (Clementi et al., 2020). Numerous studies have indicated that certain cancers are associated with the defect or mutation in the proteins of nuclear or mitochondrial DNA repair pathways (Pearl et al., 2015; Cerrato et al., 2016). For example, the defect in the ATM–Chk2–p53 pathway, which plays a crucial role in DNA double-strand breaks repair, promoted glioblastoma multiforme (GBM) formation and contributed to GBMs radiation resistance (Squatrito et al., 2010). The human syndrome hereditary nonpolyposis colorectal cancer (HNPCC), which connects with high degrees of microsatellite instability, is caused by germline mutations in MMR genes, and the tumorigenesis of this disease is connected with the defect in the MMR pathway (Hampel et al., 2005). People who carry an MMR gene mutation have the increased risk of a wide variety of cancers than their noncarrier relatives (Win et al., 2012). Two important homologous recombination (HR) DNA repair-related genes, BRCA1 and BRCA2 germline mutant confer the genetic predisposition to breast, ovarian cancer and pancreatic cancer (Riaz et al., 2017). In addition, the tumor microenvironment characteristic of hypoxia, low pH and nutrient deficiency, can give rise to genomic instability and tumor progress through downregulating DNA repair pathway. It has been reported that hypoxic circumstance can result in the reduction of MLH1 expression, a core protein in the MMR pathway (Mihaylova et al., 2003). The downregulation of RAD51, a key mediator of HRR, was observed in multiple cancer cell types induced by hypoxia, suggesting that the hypoxic tumor microenvironment can suppress the HRR pathway to cause genetic instability (Bindra et al., 2004; Lu et al., 2011). Tumor hypoxia also regulated the DDR by driving alternative splicing (Memon et al., 2016). Study in human pulmonary epithelial cells has found that the acidic conditions delayed DNA damaging compounds benzo[a]pyrene (B[a]P) metabolism and inhibited NER capacity, ultimately enhanced B[a]P-induced DNA damage (Shi et al., 2017). Recent studies have shown that extracellular nutrients have significant effects on genome integrity. Glutamine is the main source of carbon and nitrogen for tumor cells. Lack of glutamine led to DNA alkylation damage by inhibiting ALKBH activity and increased the sensitivity of cancer cells to alkylating agents (Tran et al., 2017). Glucose starvation also enhanced radiosensitivity of tumor cells by reducing DNA double-strand break (DSB) repair (Ampferl et al., 2018). Thus, the dysregulation of DNA repair pathways can contribute to the development of cancer by promoting genomic instability and mutation in mammal cells.
Targeting DNA Repair Pathways in Cancer Therapy
The most common cancer treatments, including chemo- or radiotherapy, are designed to induce cell death by direct or indirect DNA damage. However, tumor cells can initiate DNA repair pathways to resist these anticancer agents during chemo- or radiotherapy. Therefore, combination of the nuclear or mitochondrial DNA repair pathway inhibitors with anticancer agents may increase the tumor cell sensitivity to these agents.
O-6-Methylguanine-DNA Methyltransferase (MGMT)
The role of MGMT is to remove alkyl adducts from the O6 position of guanine. Thus, the protective effect of MGMT could diminish the cytotoxic effects of alkylating agents (Middleton and Margison, 2003), suggesting that MGMT activity is likely to be a useful marker of the sensitivity of cancer cells to alkylating agents. It has been reported that high MGMT expression in tumor cell is associated with the resistance to 1,3- bis- (2-chloroethyl) -1- nitrosourea (BCNU) and temozolomide (TMZ) (Happold et al., 2018; Hsu et al., 2018), which target the O6-position of guanine, resulting in cytotoxic and mutagenic DNA adducts (Rabik et al., 2006). Recently, researchers found that MGMT-mediated the resistance to DNA alkylating agents in cancer cell is profoundly dependent on the DNA repair enzyme PARP. Combination of temozolomide with PARP inhibitors (PARPi) in MGMT-positive cancer cells enhanced the anticancer effects (Erice et al., 2015; Jue et al., 2017).
The inactivation of MGMT in tumor cells has been appreciated as a therapeutic target for sensitizing cells to O6-alkylating agents (Maki et al., 2005). In vitro and in vivo studies demonstrated that O6-Benzylguanine (O6-BG), a typical pseudo-substrate that was developed to inactivate MGMT, in combination with O6-alkylating agents increased the therapeutic efficacy of chemotherapeutic alkylating agents (Maki, Murakami, 2005). Lomeguatrib (called O6-(4-bromothenyl) guanine, as well as PaTrin-2), another pseudo-substrate tested in clinical trials, has been shown to increase the therapeutic index of methylating agent temozolomide in nude mice bearing A375M human melanoma xenografts and patients with advanced solid tumors (Middleton et al., 2002; Ranson et al., 2006). Bobustuc GC et al. demonstrated that inhibition of MGMT suppressed the expression of survivin and enhanced the cytotoxicity of gemcitabine in pancreatic cancer (Bobustuc et al., 2015). Another approach to MGMT inactivation is to silence the MGMT gene expression through its promoter methylation. Several studies in animal models have suggested that the therapy of MGMT gene silence was able to overcome TMZ resistance and increase tumor cell death (Viel et al., 2013). Clinical study indicated that patients with glioblastoma containing a methylated MGMT promoter obtained more benefits from TMZ than those who did not have a methylated MGMT promoter (Hegi et al., 2005). Lately, it has been confirmed that MGMT gene methylation can be a biomarker for temozolomide (TMZ) treatment and a potent prognostic factor in patients with GBM (Kim et al., 2012; Iaccarino et al., 2015; Zhao et al., 2016; Binabaj et al., 2018). However, according to the data from National Cancer database (NCDB) indicated that only 4.9% of GBM patients have MGMT promoter methylation. Even though MGMT promoter methylation status has prognostic value, it is ignored in the United States (Lee et al., 2018). More researches need to conduct to identify the prognostic value of MGMT promoter methylation in tumor patients responding to alkylating agents.
Base Excision Repair
A number of investigations have shown that inhibition of BER pathway can enhance the sensitivity of cancer cells to alkylating agents and radiotherapy (Neijenhuis et al., 2005; Gao et al., 2019). The primary methods to prevent the activity of BER pathway focus on the development of AP endonuclease 1 (APE1) or Poly (ADP-ribose) polymerase (PARP) inhibitors.
Several studies indicated that methoxyamine (MX), a small alkoxyamine that can bind with the free aldehyde of AP site to prevent APE1 cleavage at AP sites, thereby inhibiting APE-1 endonuclease activity. Combined treatment with chemotherapeutic alkylating agent such as TMZ and BCNU could reinforce the cytotoxicity of alkylating agent by targeting BER pathway (Liu et al., 2003; Montaldi and Sakamoto-Hojo, 2013). Recently, based on preclinical studies, several clinical trials were conducted, for example combination therapy with MX and TMZ in patients with advanced solid tumors has completed (NCT00892385). Currently, phase Ⅰ clinical trials of MX in combination of TMZ is undergoing in patients with relapsed solid tumors and lymphomas (NCT01851369). MX combination with pemetrexed disodium, cisplatin, is now investigating in phase Ⅰ/II stage in patients with advanced malignant solid neoplasm (NCT02535312). Lucanthone, a topoisomerase II inhibitor as well as an APE1 endonuclease inhibitor, has been shown to reinforce the cell killing effect of alkylating agents in human breast cancer cell line MDA-MB-231 (Luo and Kelley, 2004). Lucanthone combination with radiation and TMZ in GBM patients was tested in phase Ⅱ clinical trial (NCT01587144). However, it was terminated in 2016. Another phase II clinical trial investigating lucanthone combination with radiation in patients with brain metastases from non-small cell lung cancer was withdrawn due to drug issues (NCT02014545).
PARP family is composed of 17 members, of which PARP1 and PARP2 are well-recognized DNA damage sensors, especially PARP1. PARP1 detect the region of damaged DNA and play a key role in several DNA repair pathway including BER, HHR and MMEJ (Konecny and Kristeleit, 2016). While PARP1 is best studied in BER and the mechanism of PARP inhibitor (PARPi) is based on trapping PARP1 on SSBs DNA site to inhibit BER repair. Finally, it converted SSBs into DSBs and impelled cell death in HR-deficiency tumor, for example BRCA1/2 mutations, RAD51 deficiency (Figure 2) (Konecny and Kristeleit, 2016; Brown et al., 2017; Lord and Ashworth, 2017; Oplustil O’Connor et al., 2016). In 2005, two pre-clinical researches published in nature indicated that BRCA1 or BRCA2 deficient cells highly sensitized to PARP inhibition (Farmer et al., 2005; Bryant et al., 2005). Based on the concept of “synthetic lethality”-targeting either gene alone in a synthetic lethal pair is tolerated, but simultaneous targeting both genes is lethal, researchers applied PARPi to BRCA mutation tumors (Dhillon et al., 2016). Several clinical trials using PARPi including Olaparib, Veliparib, Rucaparib (Table 1) as monotherapy for the treatment of patients with germline BRCA1/2 mutation tumors including advanced breast cancer, ovarian cancer, pancreatic cancer and prostate cancer presented significantly antitumor effect (Kaufman et al., 2015; Robson et al., 2017; Moore et al., 2018; Golan et al., 2019). Olaparib as maintenance therapy also significantly prolonged progression-free survival in advanced ovarian cancer patients with HRD-positive tumors who have achieved first-line standard therapy including bevacizumab. It has been approved by FDA for utilization of Olaparib in patients with advanced germline BRCA-mutated ovarian cancer following three or more prior lines of chemotherapy (Kim et al., 2015). On May 19, 2020, the FDA also approved Olaparib for patients with metastatic castration-resistant prostate cancer (mCRPC) carrying HRR gene-mutated based on NCT02987543. PAPR1 inhibitors in combination with IR or with other different anticancer agents are currently undergoing clinical trials for treatment of patients with BRCA1/2 mutation or HRR-deficiency advanced solid tumors, which shown promising clinical activity (Bang et al., 2017; Wilson et al., 2017; Loibl et al., 2018; Coleman et al., 2019; Farago et al., 2019; Konstantinopoulos et al., 2019; Liu et al., 2019).
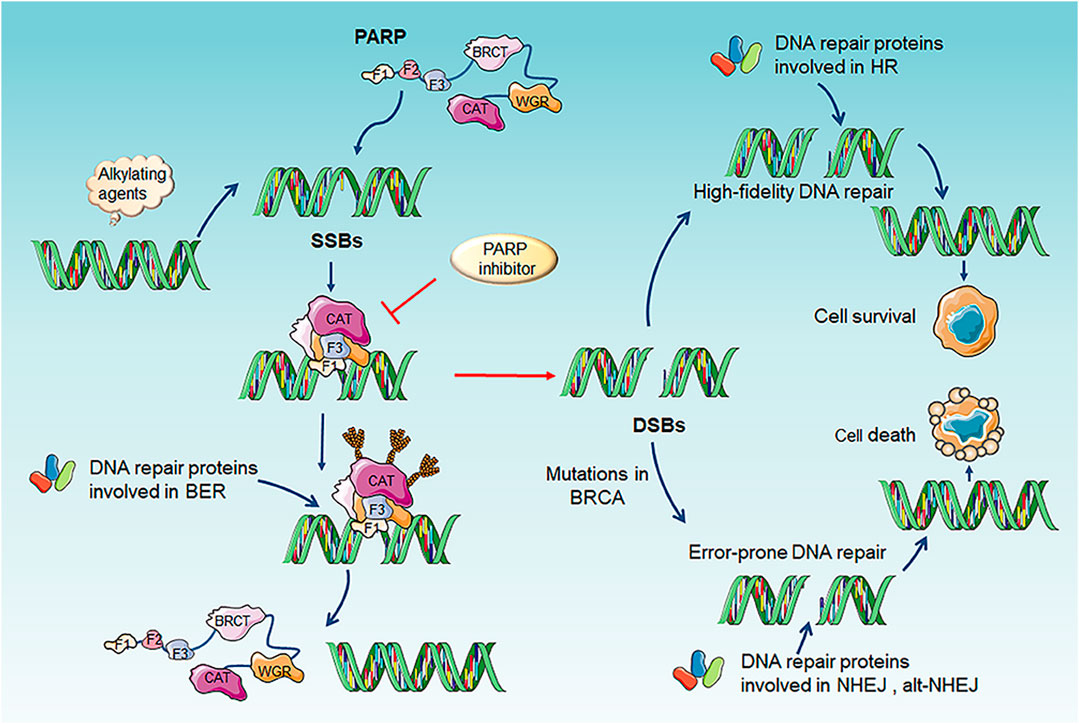
FIGURE 2. Mechanism and function of PARP and PARP inhibitors. The catalytic function of PARP1 is activated through binding to the SSBs site cuased by alkylating agents. Activated PARP1 undergo PARylation and recruitment of a serials of key DNA repair effectors involved in BER to repair DNA lesion. Finally, PARP1 release from DNA and regain inactive state. PARP inhibitors binds the catalytic site of PARP and impaired of the enzymatic activity of PARP which “trap” PARP1 on DNA, results in suppression of the catalytic cycle of PARP1 and BER. Trapping PARP1 on DNA lesion also collapses DNA replication fork, therefore transforming SSBs into genotoxic DSBs. This type of DNA lesion would normally induce HR for repairing damaged DNA. However, if HR-defective exist in tumor cells, including BRCA1/2 deficiency or mutation, another less effective and error-prone DSBs repair pathway NHEJ or alt-NHEJ could be utilized, which causing genomic instability, chromosomal fusions/translocations and subsequently inducing cell death. SSBs, single-strand breaks; DSB, double-strand break; BER, base excision repair; alt-NHEJ, alternative nonhomologous end joining; NHEJ, non-homologous end joining; HR, homologous recombination repair.
Double Strand Breaks Repair
Among various DNA lesions, DSBs is the leading lethal damage that leads to cell death and genetic mutations. DNA-dependent protein kinase (DNA-PK), a member of the PI3K-related protein kinase (PIKK) family, is involved in DSBs repair pathway via non-homologous end joining (NHEJ) (Jette and Lees-Miller, 2015). It has been reported that DNA-PK activity plays a role in chemo-radiotherapy resistance (Wang Y. et al., 2018; Stefanski et al., 2019; Alikarami et al., 2017; Liu et al., 2015). Selective DNA-PK inhibitor have been developed, including NU7026 (Dolman et al., 2015), NU7441 (Yang et al., 2016), IC87361 and SU11752 (Shinohara et al., 2005). They could inhibit DSBs repair pathway and enhance the sensitivity of cancer cells to ionizing radiation or/and chemo-potentiation such as doxorubicin (Ciszewski et al., 2014). The combination of DNA-PK inhibitor M3814 with type II topoisomerase inhibitors, including doxorubicin, etoposide and pegylated liposomal doxorubicin, enhanced the efficacy of type II topoisomerase inhibitors in ovarian cancer xenografts (Wise et al., 2019). Several novel DNA-PK inhibitors including MSC2490484A, VX-984 (M9831), M3814 are under clinical trial as single-agent or combination with Chemo-radiotherapy (Table 2). Alexander K. Tsai et al. recently found that NU7441 combination with a multikinase inhibitor regorafenib altered immune microenvironment of melanomas and enhanced the efficacy of various immunotherapies (Tsai et al., 2017).
Ataxia-teleangectasia mutated (ATM) and ATM-RAD3-related (ATR) protein, like DNA-PK protein, are the members of PIKK family. They work as a transducer of the DSB signal, and are involved in the repair of DNA DSBs (Weber and Ryan, 2015). A large of ATM inhibitors, including KU-55933, KU-60019, KU-59403, CP-466722, AZ31, AZ32, AZD0156, and AZD1390, have been developed and their antitumor effects have been investigated (Jin and Oh, 2019). It has been reported that human tumor cells treated with KU-55933, a specific inhibitor of the ATM kinase, could sensitize tumor cells to the cytotoxic effects of IR and DNA DSBs-inducing chemotherapeutic agents such as etoposide, doxorubicin, and camptothecin (Hickson et al., 2004; Hoey et al., 2018). KU-60019, an improved ATM kinase inhibitor, acts as a highly effective radio-sensitizer in human glioma cells (Biddlestone-Thorpe et al., 2013). AZD0156, a newly discovered ATM inhibitor, has the potential to promote the survival of leukemia-bearing mice and now is under clinical trial (Morgado-Palacin et al., 2016). Preclinical study demonstrated that ATM inhibitor AZD1390 enhanced the radiosensitivity of tumor cells and extended animal survival in preclinical brain tumor models (Durant et al., 2018). AZD1390, as a radiosensitizer, is now undergoing two clinical trials in patients with brain cancer (NCT03423628) or non small cell lung cancer (NCT04550104). Many inhibitors aiming at both ATM and DNA-PK have been reported to have great potential as a chemo- and radiotherapy sensitizing agents in cancer therapy (Powell and Bindra, 2009).
The cell cycle checkpoint kinases CHK1 and CHK2 are downstream substrates of ATM /ATR, which act as the “central transducers” of the DDR (Pilie et al., 2019). Activation of these pathways is essential for the proper regulation of checkpoint and DNA repair (Smith et al., 2010). The ATM–Chk2 and ATR–Chk1 pathways respond to different DNA damages, ATM is activated at DSBs, whereas ATR is recruited to tracts of ssDNA (Di Benedetto et al., 2017). Subsequently, CHK1 and CHK2 activated by ATR and ATM respectively upon their recruitment to DNA damage sites. Protein kinase WEE1 functioned as furthest downstream in ATR/CHK1 pathway, which is indirectly regulated by DNA damage (Cleary et al., 2020). WEE1 actives the G2/M cell cycle checkpoint by impeding cyclin-dependent kinase 1 and 2 (CDK1/2) activity, thereby inducing cell cycle arrest and promoting DNA damage repair. Inhibition of WEE1 causes aberrant DNA replication and replication-dependent DNA damage in cells by suppressing CDK2 (Guertin et al., 2013). Recently, compounds targeting CHK1 are currently in clinical trials (Table 1). The first-in-class WEE1 kinase inhibitor AZD1775 is also undergoing a series of clinical trials as monotherapy or in combination with other therapies (Table 1).
mtDNA Repair Pathway
Recently, the exploration of novel anticancer strategies aiming at the differences in mitochondrial function and structure between normal cells and cancer cells has received intensive attention (Porporato et al., 2018). However, there are few studies that have discovered new anticancer approaches via targeting mtDNA repair pathway.
Like nDNA, efficient mtDNA repair pathway, especially BER pathway that mainly repairs ROS-induced lesion, may play an important role in cellular resistance to cancer therapeutic agents. MtDNA D-loop mutations were common in gastrointestinal cancer and correlated with carcinoma progression (Wang B. et al., 2018). It has been found that human breast cancer cells defective of mtDNA repair are more sensitive to oxidative damage than the control cells (Shokolenko et al., 2003). Grishko V I et al indicated that mtDNA repair pathways played an important role in protecting cells against ROS in normal HA1 Chinese hamster fibroblasts (Grishko et al., 2005). Another study clarified that mtDNA repair capacity was important for cellular resistance to oxidative damage by increasing their viability following exposure to oxidative stress (Shokolenko et al., 2003). Ueta E et al demonstrated that downregulation of the mtDNA repair-associated molecules, mitochondrial transcription factor A (mtTFA) and Polγ by using inhibitors of PI3K/Akt signaling in oral squamous cell carcinoma cells (OSC) increased the susceptibility of radio-sensitive OSC cells and radio-resistant OSC cells to gamma-rays (Ueta et al., 2008). This observation implied that PI3K/Akt signal inhibitors can suppress mtDNA repair capacity. Thus, these inhibitors combined with ionizing irradiation or chemotherapeutic drugs may be utilized as an effective strategy in cancer therapy.
DNA glycosylases are involved in the initiation step of BER that recognizes and removes the abnormal base (Anderson and Friedberg, 1980). 8-OxoG-recognizing DNA glycosylase 1 (OGG1) is an important DNA glycosylase for repair of 8-oxoguanine (8-oxoG), which is one of the major DNA lesions both of the nDNA and mtDNA, especially in mtDNA (Rachek et al., 2002). It has been found that tumor cells harboring overexpressed recombinant OGG1 were more proficient at repairing of oxidative damage to mtDNA, and had increased cellular survival under oxidative stress (Rachek et al., 2002; Yuzefovych et al., 2016). We previously found that Sirt3, a major mitochondrial NAD+-dependent deacetylase, physically associated with OGG1 and deacetylated this DNA glycosylase, and that deacetylation by Sirt3 prevented the degradation of the OGG1 protein and controlled its incision activity (Cheng et al., 2013). We further showed that regulation of the acetylation and turnover of OGG1 by Sirt3 played a critical role in repairing mitochondrial DNA (mtDNA) damage, protecting mitochondrial integrity, and preventing apoptotic cell death under oxidative stress. We observed that following ionizing radiation, human tumor cells with silencing of Sirt3 expression exhibited oxidative damage of mtDNA, as measured by the accumulation of 8-oxoG and 4,977 common deletion, showed more severe mitochondrial dysfunction, and underwent greater apoptosis, in comparison to the cells without silencing of Sirt3 expression. Our results not only reveal a new function and mechanism for Sirt3 in defending the mitochondrial genome against oxidative damage and in protecting from the genotoxic stress-induced apoptotic cell death, but also provide evidence supporting a new mtDNA repair pathway. Recently, researchers also proved that overexpression of mitochondrial OGG1 decreased breast cancer progression and metastasis (Yuzefovych et al., 2016).
In conclusion, combination of DNA repair pathway inhibitors with anticancer agents may enhance the tumor sensitivity to certain chemotherapeutic drugs and radiation. More effective and less toxic DNA-damaging agents have been developed and carried out in preclinical studies (Table 2). Based on the preclinical data, a number of clinical trials have been launched to test whether targeting DNA repair pathways can reinforce the efficacy of some anticancer drugs and benefit cancer patients (Table 1).
The Relationship Between DNA Repair Pathways and Cancer Therapeutic Resistance
Resistance to cancer therapy remains the leading cause of treatment failure in cancer patients. DNA repair capacity (DRC) of tumor cells has been known to involve in drug resistance, including chemoradiotherapy, targeted therapy and immunotherapy. DNA damage inducing drug cisplatin is one of the most widely employed chemotherapeutic drugs. In a murine model of human lung cancer, tumor cells were initially effective with cisplatin treatment, but resistant emerged after prolonged treatment (Oliver et al., 2010). Cisplatin-resistant tumor cells exhibited higher level of DNA damage repair related genes and DRC, inhibition of NER pathway significantly enhanced the sensitivity of tumor cells to cisplatin (Oliver, Mercer, 2010; Wang et al., 2011). Low expression of 53BP1, a DDR protein involved in NHEJ, was associated with higher local recurrence in triple negative breast cancers (TNBC) patients treated with breast-conserving surgery and radiotherapy, indicating that 53BP1 may be a predictor of radio-resistance (Neboori et al., 2012). PTEN Y240 phosphorylation induced by ionizing radiation (IR), a standard treatment for glioblastoma (GBM) patients, promoted therapeutic resistance by enhancing DNA repair (Ma et al., 2019). Inhibiting DNA repair kinases could also prevent doxorubicin (DOX) resistance in breast cancer cells (Stefanski et al., 2019). Abnormal DNA repair activity was found in CDK4/6 inhibitors palbociclib-resistant breast cancer cells, whereas PARP inhibitors, olaparib and niraparib treatment could significantly inhibit palbociclib-resistant cancer cell viability (Kettner et al., 2019). In the recent years, immunotherapy is a major breakthrough in the field of cancer treatment. Therefore, the role of DDR in tumor immunotherapy has attracted much attention. Studies have shown deficiency of a specific DNA repair pathway was associated with immune checkpoint blockade (ICB) response. For example, MMR has been reported as a critical biomarker of response to immune checkpoint inhibitors in cancer (Le et al., 2017). Alterations in genes encoding MMR proteins often contribute to frameshift mutations, resulting in neoantigen generation (Germano et al., 2017). Phase II clinical trials proved that mismatch repair–deficient tumors exhibited higher responsive to PD-1 blockade compared with mismatch repair–proficient tumors(Asaoka et al., 2015). Based on lines of pre-clinical and clinical evidence, the US Food and drug Administration (FDA) has approved anti-PD-1 antibodies for the treatment of patients with MMR-deficient (Ruiz-Bañobre and Goel, 2019). On the contrary, researchers also found that colorectal cancer (CRC) patient with DNA mismatch repair deficiency (dMMR)/a high-level of microsatellite instability (MSI-H) exhibited intrinsic resistance to immune checkpoint immune checkpoint inhibitor (Gurjao et al., 2019). Metastatic urothelial carcinoma (mUC) shown relatively low response rates to PD-1/PD-L1 blockade (15–24%), whereas the presence of DDR gene mutations is a potential marker of clinical benefit from anti-PD-1/PD-L1 immune checkpoint inhibitors in mUC (Teo et al., 2018). Preclinical studies have also revealed that suppression of PARP induced PD-L1 expression and consequently caused immunosuppression (Jiao et al., 2017). Researches also elucidated that PARP inhibitor olaparib enhanced CD8+ T-cell recruitment and activation by activating the cGAS/STING pathway in BRCA1-deficient triple-negative breast cancer (Pantelidou et al., 2019). Therefore, multiple combination studies involving immune checkpoint inhibitors with DDR inhibitors are undergoing clinical trials, such as combination PARP inhibitor Niraparib and anti-PD-1 antibody pembrolizumab in patients with triple-negative breast cancer or ovarian cancer (NCT02657889). In the phase I, multi-center, dose-escalation study, patients with advanced solid tumors will receive WEE1 inhibitor AZD1775 (Adavosertib) in combination with MEDI4736 (durvalumab) (NCT02546661). These studies suggest that DRC plays a key role in cancer therapy resistance, therefore, evaluation of DNA repair phenotype before treatment could be of great value in clinical management of clinical therapeutic drugs or modalities.
A number of DDR inhibitors have currently come to market or under clinical development. PARP inhibitors are the first clinically approved DDR drugs based on the concept of “synthetic lethal” (Lord and Ashworth, 2017). PARP inhibitors have been widely used for cancer patients with BRCA1/2 mutation or HRR deficiency and showed promising clinical activity. However, resistance inevitably developed in the majority of patients and led to treatment failure. The mechanism of resistance to PARP inhibitors can be innate or acquired though clinical and preclinical studies. Preclinical studies demonstrated that overexpression of P-glycoprotein drug efflux transporter implicated in intrinsic resistance to Olaparib (Henneman et al., 2015). Resumption of PARformation due to poly (ADP-ribose) glycohydrolase (PARG) depletion conferred acquired resistance to PARP inhibition in BRCA2-deficient tumor cells (Gogola et al., 2018). PARP1 p. T910A mutation could override PARP1 inhibition promoted the secondary failure of Olaparib treatment (Gröschel et al., 2019). Another mechanism leading to resistance may restoration of HRR function or re-construction of replication fork stability by increasing RAD51 expression or re-expressing BRCA1/2 (Ter Brugge et al., 2016; Quigley et al., 2017; Clements et al., 2018; Lim et al., 2018; Marzio et al., 2019). Upregulation of certain oncogenic pathways such as Wnt/β-catenin signaling pathway or DDR related protein may also confer cancer cells insensitive to PARP inhibitors and providing some rationale for combination strategies with PARP inhibitors (Fukumoto et al., 2019; Watson et al., 2019; Liu et al., 2020).
Conclusion and Perspectives
Based on the relationship between DNA repair pathways and cancer development and progression, a new therapeutic strategy has emerged to increase the efficacy of DNA damaging agents through combination with inhibitors of DNA repair pathways. The inhibitors of several DNA repair pathways have been developed, and some of them are currently undergoing clinical trials. The therapeutic benefits of these agents should be further evaluated in cancer treatment, and the more specific inhibitors should be developed to reduce the adverse effect on normal tissues and cells. Many studies have demonstrated that the inhibition of DNA repair pathways may be an important way in anticancer therapies. However, we should realize that use of certain inhibitors of DNA repair pathways may have potential drawbacks. The combination of IR or chemotherapeutic agents with inhibitors of DNA repair pathway may increase the mutagenic lesions in surviving cells and lead to the development of secondary tumors. More attentions have been paid to the relationship between defective nuclear DNA repair pathway and therapeutic resistance but less about the association between the mitochondrial repair pathway and cancer cells. Due to the difference in mtDNA between cancer cells and normal cells, the development of mtDNA repair pathway inhibitors that can reduce the adverse effects to normal cells may be a more effective strategy to enhance the anticancer therapy than targeting nDNA. A better understanding on the mechanisms of mtDNA repair pathways shall facilitate the development of new effective chemo- and radiosensitizers by targeting mtDNA repair pathway in cancer therapy.
Author Contributions
LL drafted the manuscript. YG and XC designed the figure and table. JY and YC designed, reviewed, and finalized the manuscript. All authors contributed to the article and approved the submitted version.
Funding
This work was supported by grants from the National Natural Science Foundation of China 81422051, 81472593, and 31401208 (YC).
Conflict of Interest
The authors declare that the research was conducted in the absence of any commercial or financial relationships that could be construed as a potential conflict of interest.
References
Alao, J. P., and Sunnerhagen, P. (2009). The ATM and ATR inhibitors CGK733 and caffeine suppress cyclin D1 levels and inhibit cell proliferation. Radiat. Oncol. 4, 51. doi:10.1186/1748-717x-4-51
Alikarami, F., Safa, M., Faranoush, M., Hayat, P., and Kazemi, A. (2017). Inhibition of DNA-PK enhances chemosensitivity of B-cell precursor acute lymphoblastic leukemia cells to doxorubicin. Biomed. Pharmacother. 1077–1093. doi:10.1016/j.biopha.2017.08.022
Ampferl, R., Rodemann, H. P., Mayer, C., Hofling, T. T. A., and Dittmann, K. (2018). Glucose starvation impairs DNA repair in tumour cells selectively by blocking histone acetylation. Radiother. Oncol. 3, 465–470. doi:10.1016/j.radonc.2017.10.020
Anderson, C. T., and Friedberg, E. C. (1980). The presence of nuclear and mitochondrial uracil-DNA glycosylase in extracts of human KB cells. Nucleic Acids Res. 4, 875.
Asaoka, Y., Ijichi, H., and Koike, K. (2015). PD-1 blockade in tumors with mismatch-repair deficiency. N. Engl. J. Med. 20, 1979. doi:10.1056/NEJMc1510353
Bang, Y. J., Xu, R. H., Chin, K., Lee, K. W., Park, S. H., Rha, S. Y., et al. (2017). Olaparib in combination with paclitaxel in patients with advanced gastric cancer who have progressed following first-line therapy (GOLD): a double-blind, randomised, placebo-controlled, phase 3 trial. Lancet Oncol. 12, 1637–1651. doi:10.1016/s1470-2045(17)30682-4
Biddlestone-Thorpe, L., Sajjad, M., Rosenberg, E., Beckta, J. M., Valerie, N. C., Tokarz, M., et al. (2013). ATM kinase inhibition preferentially sensitizes p53-mutant glioma to ionizing radiation. Clin. Cancer Res. 12, 3189–3200. doi:10.1158/1078-0432.ccr-12-3408
Binabaj, M. M., Bahrami, A., ShahidSales, S., Joodi, M., Joudi Mashhad, M., Hassanian, S. M., et al. (2018). The prognostic value of MGMT promoter methylation in glioblastoma: a meta-analysis of clinical trials. J. Cell. Physiol. 1, 378–386. doi:10.1002/jcp.25896
Bindra, R. S., Schaffer, P. J., Meng, A., Woo, J., Maseide, K., Roth, M. E., et al. (2004). Down-regulation of Rad51 and decreased homologous recombination in hypoxic cancer cells. Mol. Cell Biol. 19, 8504–8518. doi:10.1128/mcb.24.19.8504-8518.2004
Bobustuc, G. C., Patel, A., Thompson, M., Srivenugopal, K. S., Frick, J., Weese, J., et al. (2015). MGMT inhibition suppresses survivin expression in pancreatic cancer. Pancreas. 4, 626–635. doi:10.1097/mpa.0000000000000299
Brown, J. S., O’Carrigan, B., Jackson, S. P., and Yap, T. A. (2017). Targeting DNA repair in cancer: beyond PARP inhibitors. Canc. Discov. 1, 20–37. doi:10.1158/2159-8290.CD-16-0860
Bryant, H. E., Schultz, N., Thomas, H. D., Parker, K. M., Flower, D., Lopez, E., et al. (2005). Specific killing of BRCA2-deficient tumours with inhibitors of poly(ADP-ribose) polymerase. Nature. 7035, 913–917. doi:10.1038/nature03443
Carruthers, R., Ahmed, S. U., Strathdee, K., Gomez-Roman, N., Amoah-Buahin, E., Watts, C., et al. (2015). Abrogation of radioresistance in glioblastoma stem-like cells by inhibition of ATM kinase. Mol. Oncol. 1, 192–203. doi:10.1016/j.molonc.2014.08.003
Cerrato, A., Morra, F., and Celetti, A. (2016). Use of poly ADP-ribose polymerase [PARP] inhibitors in cancer cells bearing DDR defects: the rationale for their inclusion in the clinic. J. Exp. Clin. Cancer Res. 1, 179. doi:10.1186/s13046-016-0456-2
Cheng, Y., Ren, X., Gowda, A. S., Shan, Y., Zhang, L., Yuan, Y. S., et al. (2013). Interaction of Sirt3 with OGG1 contributes to repair of mitochondrial DNA and protects from apoptotic cell death under oxidative stress. Cell Death Dis. 4, e731. doi:10.1038/cddis.2013.254
Chowdhury, S. M., Surhland, C., Sanchez, Z., Chaudhary, P., Suresh Kumar, M. A., Lee, S., et al. (2015). Graphene nanoribbons as a drug delivery agent for lucanthone mediated therapy of glioblastoma multiforme. Nanomedicine 1, 109–118. doi:10.1016/j.nano.2014.08.001
Ciszewski, W. M., Tavecchio, M., Dastych, J., and Curtin, N. J. (2014). DNA-PK inhibition by NU7441 sensitizes breast cancer cells to ionizing radiation and doxorubicin. Breast Cancer Res. Treat 1, 47–55. doi:10.1007/s10549-013-2785-6
Cleary, J. M., Aguirre, A. J., Shapiro, G. I., and D’Andrea, A. D. (2020). Biomarker-guided development of DNA repair inhibitors. Mol. Cell. 6, 1070–1085. doi:10.1016/j.molcel.2020.04.035
Clementi, E., Inglin, L., Beebe, E., Gsell, C., Garajova, Z., and Markkanen, E. (2020). Persistent DNA damage triggers activation of the integrated stress response to promote cell survival under nutrient restriction. BMC Biol 1, 36. doi:10.1186/s12915-020-00771-x
Clements, K. E., Thakar, T., Nicolae, C. M., Liang, X., Wang, H. G., and Moldovan, G. L. (2018). Loss of E2F7 confers resistance to poly-ADP-ribose polymerase (PARP) inhibitors in BRCA2-deficient cells. Nucleic Acids Res. 17, 8898–8907. doi:10.1093/nar/gky657
Coleman, R. L., Fleming, G. F., Brady, M. F., Swisher, E. M., Steffensen, K. D., Friedlander, M., et al. (2019). Veliparib with first-line chemotherapy and as maintenance therapy in ovarian cancer. N. Engl. J. Med. 25, 2403–2415. doi:10.1056/NEJMoa1909707
Dhillon, K. K., Bajrami, I., Taniguchi, T., and Lord, C. J. (2016). Synthetic lethality: the road to novel therapies for breast cancer. Endocr. Relat. Cancer. 10, T39–T55. doi:10.1530/erc-16-0228
Di Benedetto, A., Ercolani, C., Mottolese, M., Sperati, F., Pizzuti, L., Vici, P., et al. (2017). Analysis of the ATR-Chk1 and ATM-Chk2 pathways in male breast cancer revealed the prognostic significance of ATR expression. Sci. Rep. 1, 8078. doi:10.1038/s41598-017-07366-7
Dolman, M. E., van der Ploeg, I., Koster, J., Bate-Eya, L. T., Versteeg, R., Caron, H. N., et al. (2015). DNA-dependent protein kinase as molecular target for radiosensitization of neuroblastoma cells. PLoS One. 12, e0145744. doi:10.1371/journal.pone.0145744
Durant, S. T., Zheng, L., Wang, Y., Chen, K., Zhang, L., Zhang, T., et al. (2018). The brain-penetrant clinical ATM inhibitor AZD1390 radiosensitizes and improves survival of preclinical brain tumor models. Sci. Adv. 6, eaat1719. doi:10.1126/sciadv.aat1719
Erice, O., Smith, M. P., White, R., Goicoechea, I., Barriuso, J., Jones, C., et al. (2015). MGMT expression predicts PARP-mediated resistance to temozolomide. Mol. Cancer Ther. 5, 1236–1246. doi:10.1158/1535-7163.mct-14-0810
Farago, A. F., Yeap, B. Y., Stanzione, M., Hung, Y. P., Heist, R. S., Marcoux, J. P., et al. (2019). Combination olaparib and temozolomide in relapsed small-cell lung cancer. Cancer Discov. 10, 1372–1387. doi:10.1158/2159-8290.cd-19-0582
Farmer, H., McCabe, N., Lord, C. J., Tutt, A. N., Johnson, D. A., Richardson, T. B., et al. (2005). Targeting the DNA repair defect in BRCA mutant cells as a therapeutic strategy. Nature. 7035, 917–921. doi:10.1038/nature03445
Fishel, M. L., Xia, H., McGeown, J., McIlwain, D. W., Elbanna, M., Craft, A. A., et al. (2019). Antitumor activity and mechanistic characterization of APE1/ref-1 inhibitors in bladder cancer. Mol. Cancer Ther. 11, 1947–1960. doi:10.1158/1535-7163.mct-18-1166
Foote, K. M., Blades, K., Cronin, A., Fillery, S., Guichard, S. S., Hassall, L., et al. (2013). Discovery of 4-{4-[(3R)-3-Methylmorpholin-4-yl]-6-[1-(methylsulfonyl)cyclopropyl]pyrimidin-2-y l}-1H-indole (AZ20): a potent and selective inhibitor of ATR protein kinase with monotherapy in vivo antitumor activity. J. Med. Chem. 5, 2125–2138. doi:10.1021/jm301859s
Fukumoto, T., Zhu, H., Nacarelli, T., Karakashev, S., Fatkhutdinov, N., Wu, S., et al. (2019). N(6)-Methylation of adenosine of FZD10 mRNA contributes to PARP inhibitor resistance. Cancer Res. 11, 2812–2820. doi:10.1158/0008-5472.can-18-3592
Gao, X., Wang, J., Li, M., Wang, J., Lv, J., Zhang, L., et al. (2019). Berberine attenuates XRCC1-mediated base excision repair and sensitizes breast cancer cells to the chemotherapeutic drugs. J. Cell Mol. Med. 10, 6797–6804. doi:10.1111/jcmm.14560
Germano, G., Lamba, S., Rospo, G., Barault, L., Magrì, A., Maione, F., et al. (2017). Inactivation of DNA repair triggers neoantigen generation and impairs tumour growth. Nature. 7683, 116–120. doi:10.1038/nature24673
Giglia-Mari, G., Zotter, A., and Vermeulen, W. (2011). DNA damage response. Cold Spring Harb. Perspect. Biol. 1, a000745. doi:10.1101/cshperspect.a000745
Gogola, E., Duarte, A. A., de Ruiter, J. R., Wiegant, W. W., Schmid, J. A., de Bruijn, R., et al. (2018). Selective loss of PARG restores PARylation and counteracts PARP inhibitor-mediated synthetic lethality. Cancer Cell. 6, 1078–1093.e12. doi:10.1016/j.ccell.2018.05.008
Golan, T., Hammel, P., Reni, M., Van Cutsem, E., Macarulla, T., Hall, M. J., et al. (2019). Maintenance olaparib for germline BRCA-mutated metastatic pancreatic cancer. N. Engl. J. Med. 4, 317–327. doi:10.1056/NEJMoa1903387
Grishko, V. I., Rachek, L. I., Spitz, D. R., Wilson, G. L., and LeDoux, S. P. (2005). Contribution of mitochondrial DNA repair to cell resistance from oxidative stress. J. Biol. Chem. 10, 8901–8905. doi:10.1074/jbc.M413022200
Gröschel, S., Hübschmann, D., Raimondi, F., Horak, P., Warsow, G., Fröhlich, M., et al. (2019). Defective homologous recombination DNA repair as therapeutic target in advanced chordoma. Nat. Commun. 1, 1635. doi:10.1038/s41467-019-09633-9
Guertin, A. D., Li, J., Liu, Y., Hurd, M. S., Schuller, A. G., Long, B., et al. (2013). Preclinical evaluation of the WEE1 inhibitor MK-1775 as single-agent anticancer therapy. Mol. Cancer Ther. 8, 1442–1452. doi:10.1158/1535-7163.MCT-13-0025
Gurjao, C., Liu, D., Hofree, M., AlDubayan, S. H., Wakiro, I., Su, M. J., et al. (2019). Intrinsic resistance to immune checkpoint blockade in a mismatch repair-deficient colorectal cancer. Cancer Immunol. Res. 8, 1230–1236. doi:10.1158/2326-6066.CIR-18-0683
Hampel, H., Frankel, W. L., Martin, E., Arnold, M., Khanduja, K., Kuebler, P., et al. (2005). Screening for the Lynch syndrome (hereditary nonpolyposis colorectal cancer). N. Engl. J. Med. 18, 1851–1860. doi:10.1056/NEJMoa043146
Hanahan, D., and Weinberg, R. A. (2011). Hallmarks of cancer: the next generation. Cell. 5, 646–674. doi:10.1016/j.cell.2011.02.013
Happold, C., Stojcheva, N., Silginer, M., Weiss, T., Roth, P., Reifenberger, G., et al. (2018). Transcriptional control of O(6) -methylguanine DNA methyltransferase expression and temozolomide resistance in glioblastoma. J. Neurochem. 6, 780–790. doi:10.1111/jnc.14326
Hegi, M. E., Diserens, A. C., Gorlia, T., Hamou, M. F., de Tribolet, N., Weller, M., et al. (2005). MGMT gene silencing and benefit from temozolomide in glioblastoma. N. Engl. J. Med. 10, 997–1003. doi:10.1056/NEJMoa043331
Henneman, L., van Miltenburg, M. H., Michalak, E. M., Braumuller, T. M., Jaspers, J. E., Drenth, A. P., et al. (2015). Selective resistance to the PARP inhibitor olaparib in a mouse model for BRCA1-deficient metaplastic breast cancer. Proc. Natl. Acad. Sci. U.S.A. 27, 8409–8414. doi:10.1073/pnas.1500223112
Hickson, I., Zhao, Y., Richardson, C. J., Green, S. J., Martin, N. M., Orr, A. I., et al. (2004). Identification and characterization of a novel and specific inhibitor of the ataxia-telangiectasia mutated kinase ATM. Cancer Res. 24, 9152–9159. doi:10.1158/0008-5472.can-04-2727
Hoey, C., Ray, J., Jeon, J., Huang, X., Taeb, S., Ylanko, J., et al. (2018). miRNA-106a and prostate cancer radioresistance: a novel role for LITAF in ATM regulation. Mol. Oncol. 8, 1324–1341. doi:10.1002/1878-0261.12328
Hosoya, N., and Miyagawa, K. (2014). Targeting DNA damage response in cancer therapy. Cancer Sci. 4, 370–388. doi:10.1111/cas.12366
Hsu, S. H., Chen, S. H., Kuo, C. C., and Chang, J. Y. (2018). Ubiquitin-conjugating enzyme E2 B regulates the ubiquitination of O(6)-methylguanine-DNA methyltransferase and BCNU sensitivity in human nasopharyngeal carcinoma cells. Biochem. Pharmacol. 158, 327–338. doi:10.1016/j.bcp.2018.10.029
Huang, F., and Mazin, A. V. (2014). A small molecule inhibitor of human RAD51 potentiates breast cancer cell killing by therapeutic agents in mouse xenografts. PLoS One. 6, e100993. doi:10.1371/journal.pone.0100993
Iaccarino, C., Orlandi, E., Ruggeri, F., Nicoli, D., Torricelli, F., Maggi, M., et al. (2015). Prognostic value of MGMT promoter status in non-resectable glioblastoma after adjuvant therapy. Clin. Neurol. Neurosurg. 132, 1–8. doi:10.1016/j.clineuro.2015.01.029
Jackson, S. P., and Bartek, J. (2009). The DNA-damage response in human biology and disease. Nature. 7267, 1071–1078. doi:10.1038/nature08467
Jette, N., and Lees-Miller, S. P. (2015). The DNA-dependent protein kinase: a multifunctional protein kinase with roles in DNA double strand break repair and mitosis. Prog. Biophys. Mol. Biol. 2-3, 194–205. doi:10.1016/j.pbiomolbio.2014.12.003
Jiao, S., Xia, W., Yamaguchi, H., Wei, Y., Chen, M. K., Hsu, J. M., et al. (2017). PARP inhibitor upregulates PD-L1 expression and enhances cancer-associated immunosuppression. Clin. Cancer Res. 14, 3711–3720. doi:10.1158/1078-0432.ccr-16-3215
Jin, M. H., and Oh, D. Y. (2019). ATM in DNA repair in cancer. Pharmacol. Ther. [Epub ahead of print]. doi:10.1016/j.pharmthera.2019.07.002
Jue, T. R., Nozue, K., Lester, A. J., Joshi, S., Schroder, L. B., Whittaker, S. P., et al. (2017). Veliparib in combination with radiotherapy for the treatment of MGMT unmethylated glioblastoma. J. Transl. Med. 1, 61. doi:10.1186/s12967-017-1164-1
Kaufman, B., Shapira-Frommer, R., Schmutzler, R. K., Audeh, M. W., Friedlander, M., Balmana, J., et al. (2015). Olaparib monotherapy in patients with advanced cancer and a germline BRCA1/2 mutation. J. Clin. Oncol. 3, 244–250. doi:10.1200/jco.2014.56.2728
Kettner, N. M., Vijayaraghavan, S., Durak, M. G., Bui, T., Kohansal, M., Ha, M. J., et al. (2019). Combined inhibition of STAT3 and DNA repair in palbociclib-resistant ER-positive breast cancer. Clin. Cancer Res. 13, 3996–4013. doi:10.1158/1078-0432.ccr-18-3274
Kim, G., Ison, G., McKee, A. E., Zhang, H., Tang, S., Gwise, T., et al. (2015). FDA approval summary: olaparib monotherapy in patients with deleterious germline BRCA-mutated advanced ovarian cancer treated with three or more lines of chemotherapy. Clin. Cancer Res. 19, 4257–4261. doi:10.1158/1078-0432.ccr-15-0887
Kim, Y. S., Kim, S. H., Cho, J., Kim, J. W., Chang, J. H., Kim, D. S., et al. (2012). MGMT gene promoter methylation as a potent prognostic factor in glioblastoma treated with temozolomide-based chemoradiotherapy: a single-institution study. Int. J. Radiat. Oncol. Biol. Phys. 3, 661–667. doi:10.1016/j.ijrobp.2011.12.086
King, H. O., Brend, T., Payne, H. L., Wright, A., Ward, T. A., Patel, K., et al. (2017). RAD51 is a selective DNA repair target to radiosensitize glioma stem cells. Stem Cell Rep. 1, 125–139. doi:10.1016/j.stemcr.2016.12.005
Konecny, G. E., and Kristeleit, R. S. (2016). PARP inhibitors for BRCA1/2-mutated and sporadic ovarian cancer: current practice and future directions. Br. J. Cancer. 10, 1157–1173. doi:10.1038/bjc.2016.311
Konstantinopoulos, P. A., Barry, W. T., Birrer, M., Westin, S. N., Cadoo, K. A., Shapiro, G. I., et al. (2019). Olaparib and α-specific PI3K inhibitor alpelisib for patients with epithelial ovarian cancer: a dose-escalation and dose-expansion phase 1b trial. Lancet Oncol. 4, 570–580. doi:10.1016/s1470-2045(18)30905-7
Koosha, F., Neshasteh-Riz, A., Takavar, A., Eyvazzadeh, N., Mazaheri, Z., Eynali, S., et al. (2017). The combination of A-966492 and Topotecan for effective radiosensitization on glioblastoma spheroids. Biochem. Biophys. Res. Commun. 4, 1092–1097. doi:10.1016/j.bbrc.2017.08.018
Le, D. T., Durham, J. N., Smith, K. N., Wang, H., Bartlett, B. R., Aulakh, L. K., et al. (2017). Mismatch repair deficiency predicts response of solid tumors to PD-1 blockade. Science. 6349, 409–413. doi:10.1126/science.aan6733
Lee, A., Youssef, I., Osborn, V. W., Safdieh, J., Becker, D. J., and Schreiber, D. (2018). The utilization of MGMT promoter methylation testing in United States hospitals for glioblastoma and its impact on prognosis. J. Clin. Neurosci. 51, 85–90. doi:10.1016/j.jocn.2018.02.009
Lim, K. S., Li, H., Roberts, E. A., Gaudiano, E. F., Clairmont, C., Sambel, L. A., et al. (2018). USP1 is required for replication fork protection in BRCA1-deficient tumors. Mol. Cell. 6, 925–941.e4. doi:10.1016/j.molcel.2018.10.045
Liu, J. F., Barry, W. T., Birrer, M., Lee, J. M., Buckanovich, R. J., Fleming, G. F., et al. (2019). Overall survival and updated progression-free survival outcomes in a randomized phase II study of combination cediranib and olaparib versus olaparib in relapsed platinum-sensitive ovarian cancer. Ann. Oncol. 4, 551–557. doi:10.1093/annonc/mdz018
Liu, L., Cai, S., Han, C., Banerjee, A., Wu, D., Cui, T., et al. (2020). ALDH1A1 contributes to PARP inhibitor resistance via enhancing DNA repair in BRCA2(-/-) ovarian cancer cells. Mol. Cancer Ther. 1, 199–210. doi:10.1158/1535-7163.mct-19-0242
Liu, L., Yan, L., Donze, J. R., and Gerson, S. L. (2003). Blockage of abasic site repair enhances antitumor efficacy of 1,3-bis-(2-chloroethyl)-1-nitrosourea in colon tumor xenografts. Mol. Cancer Ther. 10, 1061
Liu, Y., Zhang, L., Liu, Y., Sun, C., Zhang, H., Miao, G., et al. (2015). DNA-PKcs deficiency inhibits glioblastoma cell-derived angiogenesis after ionizing radiation. J. Cell. Physiol. 5, 1094–1103. doi:10.1002/jcp.24841
Loibl, S., O'Shaughnessy, J., Untch, M., Sikov, W. M., Rugo, H. S., McKee, M. D., et al. (2018). Addition of the PARP inhibitor veliparib plus carboplatin or carboplatin alone to standard neoadjuvant chemotherapy in triple-negative breast cancer (BrighTNess): a randomised, phase 3 trial. Lancet Oncol. 4, 497–509. doi:10.1016/s1470-2045(18)30111-6
Lord, C. J., and Ashworth, A. (2017). PARP inhibitors: synthetic lethality in the clinic. Science. 6330, 1152–1158. doi:10.1126/science.aam7344
Lu, Y., Chu, A., Turker, M. S., and Glazer, P. M. (2011). Hypoxia-induced epigenetic regulation and silencing of the BRCA1 promoter. Mol. Cell Biol. 16, 3339–3350. doi:10.1128/mcb.01121-10
Luo, M., and Kelley, M. R. (2004). Inhibition of the human apurinic/apyrimidinic endonuclease (APE1) repair activity and sensitization of breast cancer cells to DNA alkylating agents with lucanthone. Anticancer Res. 4, 2127
Ma, H., Takahashi, A., Yoshida, Y., Adachi, A., Kanai, T., Ohno, T., et al. (2015). Combining carbon ion irradiation and non-homologous end-joining repair inhibitor NU7026 efficiently kills cancer cells. Radiat. Oncol. 10, 225. doi:10.1186/s13014-015-0536-z
Ma, J., Benitez, J. A., Li, J., Miki, S., Ponte de Albuquerque, C., Galatro, T., et al. (2019). Inhibition of nuclear PTEN tyrosine phosphorylation enhances glioma radiation sensitivity through attenuated DNA repair. Cancer Cell. 3, 504–518.e7. doi:10.1016/j.ccell.2019.01.020
Maki, Y., Murakami, J., Asaumi, J., Tsujigiwa, H., Nagatsuka, H., Kokeguchi, S., et al. (2005). Role of O6-methylguanine-DNA methyltransferase and effect of O6-benzylguanine on the anti-tumor activity of cis-diaminedichloroplatinum(II) in oral cancer cell lines. Oral Oncol. 10, 984–993. doi:10.1016/j.oraloncology.2005.05.011
Marzio, A., Puccini, J., Kwon, Y., Maverakis, N. K., Arbini, A., Sung, P., et al. (2019). The F-box domain-dependent activity of EMI1 regulates PARPi sensitivity in triple-negative breast cancers. Mol. Cell. 2, 224–237.e6. doi:10.1016/j.molcel.2018.11.003
Memon, D., Dawson, K., Smowton, C. S., Xing, W., Dive, C., and Miller, C. J. (2016). Hypoxia-driven splicing into noncoding isoforms regulates the DNA damage response. NPJ Genom. Med. 1, 16020. doi:10.1038/npjgenmed.2016.20
Middleton, M. R., and Margison, G. P. (2003). Improvement of chemotherapy efficacy by inactivation of a DNA-repair pathway. Lancet Oncol. 1, 37–44. doi:10.1016/s1470-2045(03)00959-8
Middleton, M. R., Thatcher, N., McMurry, T. B., McElhinney, R. S., Donnelly, D. J., and Margison, G. P. (2002). Effect of O6-(4-bromothenyl)guanine on different temozolomide schedules in a human melanoma xenograft model. Int. J. Cancer. 5, 615–617. doi:10.1002/ijc.10532
Mihaylova, V. T., Bindra, R. S., Yuan, J., Campisi, D., Narayanan, L., Jensen, R., et al. (2003). Decreased expression of the DNA mismatch repair gene Mlh1 under hypoxic stress in mammalian cells. Mol. Cell Biol. 9, 3265–3273. doi:10.1128/mcb.23.9.3265-3273.2003
Montaldi, A. P., and Sakamoto-Hojo, E. T. (2013). Methoxyamine sensitizes the resistant glioblastoma T98G cell line to the alkylating agent temozolomide. Clin. Exp. Med. 4, 279–288. doi:10.1007/s10238-012-0201-x
Moore, K., Colombo, N., Scambia, G., Kim, B. G., Oaknin, A., Friedlander, M., et al. (2018). Maintenance olaparib in patients with newly diagnosed advanced ovarian cancer. N. Engl. J. Med. 26, 2495–2505. doi:10.1056/NEJMoa1810858
Morgado-Palacin, I., Day, A., Murga, M., Lafarga, V., Anton, M. E., Tubbs, A., et al. (2016). Targeting the kinase activities of ATR and ATM exhibits antitumoral activity in mouse models of MLL-rearranged AML. Sci. Signal. 445, ra91. doi:10.1126/scisignal.aad8243
Muralidharan, S. V., Bhadury, J., Nilsson, L. M., Green, L. C., McLure, K. G., and Nilsson, J. A. (2016). BET bromodomain inhibitors synergize with ATR inhibitors to induce DNA damage, apoptosis, senescence-associated secretory pathway and ER stress in Myc-induced lymphoma cells. Oncogene. 36, 4689–4697. doi:10.1038/onc.2015.521
Neboori, H. J., Haffty, B. G., Wu, H., Yang, Q., Aly, A., Goyal, S., et al. (2012). Low p53 binding protein 1 (53BP1) expression is associated with increased local recurrence in breast cancer patients treated with breast-conserving surgery and radiotherapy. Int. J. Radiat. Oncol. Biol. Phys. 5, e677–83. doi:10.1016/j.ijrobp.2012.01.089
Neijenhuis, S., Begg, A. C., and Vens, C. (2005). Radiosensitization by a dominant negative to DNA polymerase beta is DNA polymerase beta-independent and XRCC1-dependent. Radiother. Oncol. 2, 123–128. doi:10.1016/j.radonc.2005.06.020
Ohta, S. (2006). Contribution of somatic mutations in the mitochondrial genome to the development of cancer and tolerance against anticancer drugs. Oncogene. 34, 4768–4776. doi:10.1038/sj.onc.1209602
Oleinick, N. L., Biswas, T., Patel, R., Tao, M., Patel, R., Weeks, L., et al. (2016). Radiosensitization of non-small-cell lung cancer cells and xenografts by the interactive effects of pemetrexed and methoxyamine. Radiother. Oncol. 2, 335–341. doi:10.1016/j.radonc.2016.10.007
Oliver, T. G., Mercer, K. L., Sayles, L. C., Burke, J. R., Mendus, D., Lovejoy, K. S., et al. (2010). Chronic cisplatin treatment promotes enhanced damage repair and tumor progression in a mouse model of lung cancer. Genes Dev. 8, 837–852. doi:10.1101/gad.1897010
Oplustil O’Connor, L., Rulten, S. L., Cranston, A. N., Odedra, R., Brown, H., Jaspers, J. E., et al. (2016). The PARP inhibitor AZD2461 provides insights into the role of PARP3 inhibition for both synthetic lethality and tolerability with chemotherapy in preclinical models. Cancer Res. 20, 6084–6094. doi:10.1158/0008-5472.CAN-15-3240
Pantelidou, C., Sonzogni, O., De Oliveria Taveira, M., Mehta, A. K., Kothari, A., Wang, D., et al. (2019). PARP inhibitor efficacy depends on CD8(+) T-cell recruitment via intratumoral STING pathway activation in BRCA-deficient models of triple-negative breast cancer. Cancer Discov. 6, 722–737. doi:10.1158/2159-8290.cd-18-1218
Pearl, L. H., Schierz, A. C., Ward, S. E., Al-Lazikani, B., and Pearl, F. M. (2015). Therapeutic opportunities within the DNA damage response. Nat. Rev. Cancer. 3, 166–180. doi:10.1038/nrc3891
Pilie, P. G., Tang, C., Mills, G. B., and Yap, T. A. (2019). State-of-the-art strategies for targeting the DNA damage response in cancer. Nat. Rev. Clin. Oncol. 2, 81–104. doi:10.1038/s41571-018-0114-z
Porporato, P. E., Filigheddu, N., Pedro, J. M. B., Kroemer, G., and Galluzzi, L. (2018). Mitochondrial metabolism and cancer. Cell Res. 3, 265–280. doi:10.1038/cr.2017.155
Powell, S. N., and Bindra, R. S. (2009). Targeting the DNA damage response for cancer therapy. DNA Repair. 9, 1153–1165. doi:10.1016/j.dnarep.2009.04.011
Quigley, D., Alumkal, J. J., Wyatt, A. W., Kothari, V., Foye, A., Lloyd, P., et al. (2017). Analysis of circulating cell-free DNA identifies multiclonal heterogeneity of BRCA2 reversion mutations associated with resistance to PARP inhibitors. Cancer Discov. 9, 999–1005. doi:10.1158/2159-8290.cd-17-0146
Rabik, C. A., Njoku, M. C., and Dolan, M. E. (2006). Inactivation of O6-alkylguanine DNA alkyltransferase as a means to enhance chemotherapy. Cancer Treat Rev. 4, 261–276. doi:10.1016/j.ctrv.2006.03.004
Rachek, L. I., Grishko, V. I., Musiyenko, S. I., Kelley, M. R., LeDoux, S. P., and Wilson, G. L. (2002). Conditional targeting of the DNA repair enzyme hOGG1 into mitochondria. J. Biol. Chem. 47, 44932–44937. doi:10.1074/jbc.M208770200
Ranson, M., Middleton, M. R., Bridgewater, J., Lee, S. M., Dawson, M., Jowle, D., et al. (2006). Lomeguatrib, a potent inhibitor of O6-alkylguanine-DNA-alkyltransferase: phase I safety, pharmacodynamic, and pharmacokinetic trial and evaluation in combination with temozolomide in patients with advanced solid tumors. Clin. Cancer Res. 5, 1577–1584. doi:10.1158/1078-0432.ccr-05-2198
Riaz, N., Blecua, P., Lim, R. S., Shen, R., Higginson, D. S., Weinhold, N., et al. (2017). Pan-cancer analysis of bi-allelic alterations in homologous recombination DNA repair genes. Nat. Commun. 1, 857. doi:10.1038/s41467-017-00921-w
Robson, M., Im, S. A., Senkus, E., Xu, B., Domchek, S. M., Masuda, N., et al. (2017). Olaparib for metastatic breast cancer in patients with a germline BRCA mutation. N. Engl. J. Med. 6, 523–533. doi:10.1056/NEJMoa1706450
Ruiz-Bañobre, J., and Goel, A. (2019). DNA mismatch repair deficiency and immune checkpoint inhibitors in gastrointestinal cancers. Gastroenterology. 4, 890–903. doi:10.1053/j.gastro.2018.11.071
Saki, M., and Prakash, A. (2017). DNA damage related crosstalk between the nucleus and mitochondria. Free Radic. Biol. Med. 216–227. doi:10.1016/j.freeradbiomed.2016.11.050
Seo, Y., and Kinsella, T. J. (2009). Essential role of DNA base excision repair on survival in an acidic tumor microenvironment. Cancer Res. 18, 7285–7293. doi:10.1158/0008-5472.can-09-0624
Shi, Q., Maas, L., Veith, C., Van Schooten, F. J., and Godschalk, R. W. (2017). Acidic cellular microenvironment modifies carcinogen-induced DNA damage and repair. Arch. Toxicol. 6, 2425–2441. doi:10.1007/s00204-016-1907-4
Shi, Y., Wang, Y., Qian, J., Yan, X., Han, Y., Yao, N., et al. (2020). MGMT expression affects the gemcitabine resistance of pancreatic cancer cells. Life Sci. 259, 118148. doi:10.1016/j.lfs.2020.118148
Shinohara, E. T., Geng, L., Tan, J., Chen, H., Shir, Y., Edwards, E., et al. (2005). DNA-dependent protein kinase is a molecular target for the development of noncytotoxic radiation-sensitizing drugs. Cancer Res. 12, 4987–4992. doi:10.1158/0008-5472.can-04-4250
Shokolenko, I. N., Alexeyev, M. F., Robertson, F. M., LeDoux, S. P., and Wilson, G. L. (2003). The expression of Exonuclease III from E. coli in mitochondria of breast cancer cells diminishes mitochondrial DNA repair capacity and cell survival after oxidative stress. DNA Repair. 2, 471–482. doi:10.1016/s1568-7864(03)00019-3
Smith, J., Tho, L. M., Xu, N., and Gillespie, D. A. (2010). The ATM-Chk2 and ATR-Chk1 pathways in DNA damage signaling and cancer. Adv. Cancer Res. 73–112. doi:10.1016/b978-0-12-380888-2.00003-0
Squatrito, M., Brennan, C. W., Helmy, K., Huse, J. T., Petrini, J. H., and Holland, E. C. (2010). Loss of ATM/Chk2/p53 pathway components accelerates tumor development and contributes to radiation resistance in gliomas. Cancer Cell. 6, 619–629. doi:10.1016/j.ccr.2010.10.034
Stefanski, C. D., Keffler, K., McClintock, S., Milac, L., and Prosperi, J. R. (2019). APC loss affects DNA damage repair causing doxorubicin resistance in breast cancer cells. Neoplasia. 12, 1143–1150. doi:10.1016/j.neo.2019.09.002
Sunada, S., Kanai, H., Lee, Y., Yasuda, T., Hirakawa, H., Liu, C., et al. (2016). Nontoxic concentration of DNA-PK inhibitor NU7441 radio-sensitizes lung tumor cells with little effect on double strand break repair. Cancer Sci. 9, 1250–1255. doi:10.1111/cas.12998
Teng, P. N., Bateman, N. W., Darcy, K. M., Hamilton, C. A., Maxwell, G. L., Bakkenist, C. J., et al. (2015). Pharmacologic inhibition of ATR and ATM offers clinically important distinctions to enhancing platinum or radiation response in ovarian, endometrial, and cervical cancer cells. Gynecol. Oncol. 3, 554–561. doi:10.1016/j.ygyno.2014.12.035
Teo, M. Y., Seier, K., Ostrovnaya, I., Regazzi, A. M., Kania, B. E., Moran, M. M., et al. (2018). Alterations in DNA damage response and repair genes as potential marker of clinical benefit from PD-1/PD-L1 blockade in advanced urothelial cancers. J. Clin. Oncol. 17, 1685–1694. doi:10.1200/jco.2017.75.7740
Ter Brugge, P., Kristel, P., van der Burg, E., Boon, U., de Maaker, M., Lips, E., et al. (2016). Mechanisms of therapy resistance in patient-derived xenograft models of BRCA1-deficient breast cancer. J. Natl. Cancer Inst. 112, 1075. doi:10.1093/jnci/djw148
Tian, H., Gao, Z., Li, H., Zhang, B., Wang, G., Zhang, Q., et al. (2015). DNA damage response--a double-edged sword in cancer prevention and cancer therapy. Cancer Lett. 1, 8–16. doi:10.1016/j.canlet.2014.12.038
Tran, T. Q., Ishak Gabra, M. B., Lowman, X. H., Yang, Y., Reid, M. A., Pan, M., et al. (2017). Glutamine deficiency induces DNA alkylation damage and sensitizes cancer cells to alkylating agents through inhibition of ALKBH enzymes. PLoS Biol. 11, e2002810. doi:10.1371/journal.pbio.2002810
Tsai, A. K., Khan, A. Y., Worgo, C. E., Wang, L. L., Liang, Y., and Davila, E. (2017). A multikinase and DNA-PK inhibitor combination immunomodulates melanomas, suppresses tumor progression, and enhances immunotherapies. Cancer Immunol. Res. 9, 790–803. doi:10.1158/2326-6066.CIR-17-0009
Ueta, E., Sasabe, E., Yang, Z., Osaki, T., and Yamamoto, T. (2008). Enhancement of apoptotic damage of squamous cell carcinoma cells by inhibition of the mitochondrial DNA repairing system. Cancer Sci. 11, 2230–2237. doi:10.1111/j.1349-7006.2008.00918.x
Vazquez-Ortiz, G., Chisholm, C., Xu, X., Lahusen, T. J., Li, C., Sakamuru, S., et al. (2014). Drug repurposing screen identifies lestaurtinib amplifies the ability of the poly (ADP-ribose) polymerase 1 inhibitor AG14361 to kill breast cancer associated gene-1 mutant and wild type breast cancer cells. Breast Cancer Res. 3, R67. doi:10.1186/bcr3682
Viel, T., Monfared, P., Schelhaas, S., Fricke, I. B., Kuhlmann, M. T., Fraefel, C., et al. (2013). Optimizing glioblastoma temozolomide chemotherapy employing lentiviral-based anti-MGMT shRNA technology. Mol. Ther. 3, 570–579. doi:10.1038/mt.2012.278
Wang, B., Qiao, L., Wang, Y., Zeng, J., Chen, D., Guo, H., et al. (2018). Mitochondrial DNA D-loop lesions with the enhancement of DNA repair contribute to gastrointestinal cancer progression. Oncol. Rep. 6, 3694–3704. doi:10.3892/or.2018.6724
Wang, Y., Xu, H., Liu, T., Huang, M., Butter, P. P., Li, C., et al. (2018). Temporal DNA-PK activation drives genomic instability and therapy resistance in glioma stem cells. JCI Insight. 3, e98096. doi:10.1172/jci.insight.98096
Wang, L. E., Yin, M., Dong, Q., Stewart, D. J., Merriman, K. W., Amos, C. I., et al. (2011). DNA repair capacity in peripheral lymphocytes predicts survival of patients with non-small-cell lung cancer treated with first-line platinum-based chemotherapy. J. Clin. Oncol. 31, 4121–4128. doi:10.1200/JCO.2010.34.3616
Watson, Z. L., Yamamoto, T. M., McMellen, A., Kim, H., Hughes, C. J., Wheeler, L. J., et al. (2019). Histone methyltransferases EHMT1 and EHMT2 (GLP/G9A) maintain PARP inhibitor resistance in high-grade serous ovarian carcinoma. Clin. Epigenet. 1, 165. doi:10.1186/s13148-019-0758-2
Weber, A. M., and Ryan, A. J. (2015). ATM and ATR as therapeutic targets in cancer. Pharmacol. Ther. 149, 124–138. doi:10.1016/j.pharmthera.2014.12.001
Wilson, R. H., Evans, T. J., Middleton, M. R., Molife, L. R., Spicer, J., Dieras, V., et al. (2017). A phase I study of intravenous and oral rucaparib in combination with chemotherapy in patients with advanced solid tumours. Br. J. Cancer. 7, 884–892. doi:10.1038/bjc.2017.36
Win, A. K., Young, J. P., Lindor, N. M., Tucker, K. M., Ahnen, D. J., Young, G. P., et al. (2012). Colorectal and other cancer risks for carriers and noncarriers from families with a DNA mismatch repair gene mutation: a prospective cohort study. J. Clin. Oncol. 9, 958–964. doi:10.1200/jco.2011.39.5590
Wise, H. C., Iyer, G. V., Moore, K., Temkin, S. M., Gordon, S., Aghajanian, C., et al. (2019). Activity of M3814, an oral DNA-PK inhibitor, in combination with topoisomerase II inhibitors in ovarian cancer models. Sci. Rep. 1, 18882. doi:10.1038/s41598-019-54796-6
Wu, X., Luo, Q., Zhao, P., Chang, W., Wang, Y., Shu, T., et al. (2019). MGMT-activated DUB3 stabilizes MCL1 and drives chemoresistance in ovarian cancer. Proc. Natl. Acad. Sci. U.S.A. 8, 2961–2966. doi:10.1073/pnas.1814742116
Yang, C., Wang, Q., Liu, X., Cheng, X., Jiang, X., Zhang, Y., et al. (2016). NU7441 enhances the radiosensitivity of liver cancer cells. Cell. Physiol. Biochem. 5, 1897–1905. doi:10.1159/000445551
Yuzefovych, L. V., Kahn, A. G., Schuler, M. A., Eide, L., Arora, R., Wilson, G. L., et al. (2016). Mitochondrial DNA repair through OGG1 activity attenuates breast cancer progression and metastasis. Cancer Res. 1, 30–34. doi:10.1158/0008-5472.can-15-0692
Zhao, H., Wang, S., Song, C., Zha, Y., and Li, L. (2016). The prognostic value of MGMT promoter status by pyrosequencing assay for glioblastoma patients' survival: a meta-analysis. World J. Surg. Oncol. 1, 261. doi:10.1186/s12957-016-1012-4
Keywords: DNA damage, DNA repair pathways, mitochondrial DNA, drug resistance, cancer therapy
Citation: Li L-y, Guan Y-d, Chen X-s, Yang J-m and Cheng Y (2021) DNA Repair Pathways in Cancer Therapy and Resistance. Front. Pharmacol. 11:629266. doi: 10.3389/fphar.2020.629266
Received: 14 November 2020; Accepted: 31 December 2020;
Published: 08 February 2021.
Edited by:
Zhe-Sheng Chen, St. John’s University, United StatesCopyright © 2021 Li, Guan, Chen, Yang and Cheng. This is an open-access article distributed under the terms of the Creative Commons Attribution License (CC BY). The use, distribution or reproduction in other forums is permitted, provided the original author(s) and the copyright owner(s) are credited and that the original publication in this journal is cited, in accordance with accepted academic practice. No use, distribution or reproduction is permitted which does not comply with these terms.
*Correspondence: Yan Cheng, eWFuY2hlbmdAY3N1LmVkdS5jbg==