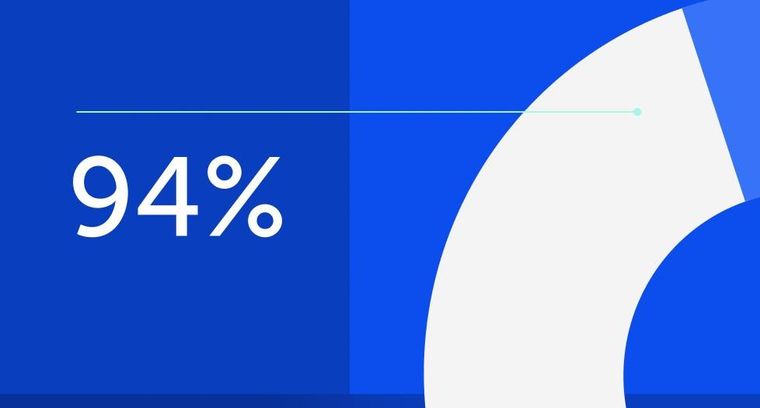
94% of researchers rate our articles as excellent or good
Learn more about the work of our research integrity team to safeguard the quality of each article we publish.
Find out more
REVIEW article
Front. Pharmacol., 29 September 2020
Sec. Neuropharmacology
Volume 11 - 2020 | https://doi.org/10.3389/fphar.2020.581098
α1-adrenergic receptors are G-Protein Coupled Receptors that are involved in neurotransmission and regulate the sympathetic nervous system through binding and activating the neurotransmitter, norepinephrine, and the neurohormone, epinephrine. There are three α1-adrenergic receptor subtypes (α1A, α1B, α1D) that are known to play various roles in neurotransmission and cognition. They are related to two other adrenergic receptor families that also bind norepinephrine and epinephrine, the β- and α2-, each with three subtypes (β1, β2, β3, α2A, α2B, α2C). Previous studies assessing the roles of α1-adrenergic receptors in neurotransmission and cognition have been inconsistent. This was due to the use of poorly-selective ligands and many of these studies were published before the characterization of the cloned receptor subtypes and the subsequent development of animal models. With the availability of more-selective ligands and the development of animal models, a clearer picture of their role in cognition and neurotransmission can be assessed. In this review, we highlight the significant role that the α1-adrenergic receptor plays in regulating synaptic efficacy, both short and long-term synaptic plasticity, and its regulation of different types of memory. We will also present evidence that the α1-adrenergic receptors, and particularly the α1A-adrenergic receptor subtype, are a potentially good target to treat a wide variety of neurological conditions with diminished cognition.
Raymond Ahlquist in 1948 (Ahlquist, 1948) first introduced the concept of different types of receptors called adrenergic receptors (ARs) which are activated by the same catecholamines, epinephrine (Epi) and norepinephrine (NE), but displayed opposite phenotypes in the body. He assigned them the subtypes of α and β. After the initial classification of α and β subtypes, decades of subsequent characterization in tissues during the 1980s further subdivided the α1-ARs into the α1A- and α1B-AR subtypes based upon pharmacological data. Using a series of several ligands in competition binding assays, the α1A-AR subtype was shown to display a 10–100-fold higher binding affinity compared to the α1B-AR subtype for a distinct series of ligands (Morrow and Creese, 1986). Subsequent cloning of the receptors confirmed this pharmacological distinction (Cotecchia et al., 1988; Laz et al., 1994; Perez et al., 1994). A few years later, another receptor was cloned that displayed novel pharmacology from the previous two subtypes and was named the α1D-AR (Perez et al., 1991). This classification of α1-ARs subtypes was approved by the IUPHAR Adrenergic Receptor Subcommittee in 1995 (Hieble et al., 1995). There exists a total of three AR families and nine subtypes (α1A, α1B, α1D, α2A, α2B, α2C, β1, β2, and β3) that display similar binding affinities but evoke different physiological effects for the same endogenous catecholamines. Signaling selectivity is achieved through the coupling to different G-proteins and effector systems in both temporal and spatial settings (Figure 1). There exists a modest number of selective agonists and antagonists to the different AR subtypes. The commercially available ligands that display at least a 10-fold in selectivity between the most related α1- versus α2-AR subtypes are shown in Table 1. Various ligands have often been employed as tools in research studies to dissect physiologies and signaling between the ARs but has often led to conflicting results, particularly the use of phentolamine which is not discriminating between the α1- versus α2-AR subtypes. Selectivity of at least 100-fold is required to significantly discriminate between the subtypes which none are commercially available except for the β2-AR agonists, salmeterol and formoterol. These two β2-AR agonists display 1,000-fold selectivity over the β1-AR by virtue of their ability to bind to the lipophilic transmembrane domains and increasing duration of action and are used clinically as bronchodilators to treat asthma (Lindén et al., 1996; Baker et al., 2015).
Figure 1 Adrenergic receptor family, subtypes, and signaling cascades. AC, adenylate cyclase; AR, adrenergic receptor; Ca, calcium; cAMP, cyclic adenosine-3',5'-monophosphate; DAG, diacylgycerol; IP, inositol phosphates; L-type Ca, L-type calcium channel; PLC, phospholipase C; PKA, protein kinase A; PKC, protein kinase C.
All nine AR receptors are expressed in the brain (Hertz et al., 2010). Localization of the specific α1-AR subtypes throughout the brain has been difficult to ascertain because of the lack of tools for their assessment. Commercially available antibodies to many G-protein coupled receptors (GPCRs), and particularly the α1-ARs, have too low of avidity for localization and in vivo studies (Jensen et al., 2009). This is because of the sparseness of selective epitopes on the extracellular surface of the receptors and their endogenous expression levels which are typically in the low femtomolar range, rendering only the most avid antibodies the ability to distinguishing their true signal from background (Webb et al., 2013).
Due to the lack of avid antibodies, initial localization studies used labeled oligonucleotides that showed distinct distribution of the α1B-and α1D-AR subtypes in the rat brain (McCune et al., 1993; Pieribone et al., 1994). However, these short DNA sequences are likely not specific enough and could not achieve a high degree of specific activity, resulting in low sensitivity and specificity. Early autoradiography studies used non-selective ligands that could not distinguish the α1-ARs subtypes but did indicate that α1-ARs are abundant throughout the rat brain (Unnerstall et al., 1985). In later studies, 3H-prazosin was used to first label all α1-AR subtypes, then competed off with the α1A-AR selective antagonist, WB4101 and then label compared with 3H-WB4101 alone (Blendy et al., 1990). Here, the α1B-AR was prominent in the thalamus, lateral amygdaloid nuclei, and cortical laminar areas. The α1A-AR was prominent in the entorhinal cortex, amygdala but with more widespread distribution than the α1B-AR. The α1A-AR was also present in the cortex but in a homogenous and not laminar distribution that the α1B-AR indicated. However, this study was limited in scope because only a select coronal section of the brain was analyzed and sensitivity was low due to the use of tritium (Blendy et al., 1990). Much later, in situ hybridization studies using the full-length cDNA sequence confirmed that the α1-AR subtypes are indeed abundant and differentially expressed in the rat brain (Domyancic and Morilak, 1997). However, while hybridization studies are sensitive, they are not quantitative because they do not detect the protein, only nucleic acids. Radioligand binding of dissected tissue also offers high sensitivity and these studies indicated discreet brain domains in humans with high α1-AR content in the hippocampus and prefrontal cortex with the lowest expression in the caudate and putamen (Shimohama et al., 1986).
Eventually the development of transgenic and knock-out (KO) mouse models of the α1-AR subtypes were made and, in addition, mouse models with the α1-ARs tagged with either an enhanced green fluorescent protein (EGFP), endogenous promoter-driven expression of EGFP, or placement of β-galactosidase gene to KO the receptor. Using this transgenic-tagged approach, the α1A- and α1B-ARs was shown to exhibit similar expression patterns in the CNS, though the relative abundance was different (Papay et al., 2004; Papay et al., 2006). Both α1-AR subtypes are expressed in the amygdala, hippocampus, cerebellum, cortex, hypothalamus, midbrain, and spinal areas. Both α1-AR subtypes were also found in the same cell types such as neurons, interneurons, progenitors, and stem cells (Papay et al., 2004; Papay et al., 2006; Gupta et al., 2009). Using the α1D-AR KO, 3H-prazosin, and comparing radioligand receptor binding to normal wild-type mice, there was only modest loss of radioligand binding in the cortex, olfactory bulb, and CA1/CA3 hippocampus indicating low amounts of the α1D-AR subtype in the brain (Sadalge et al., 2003). Using transgenic KO mice for the individual α1-AR subtypes in conjunction with ligand binding studies (i.e. based upon the loss of binding thereof), the relative distribution of α1-ARs in the brain has been estimated to be composed of ~55% α1A (Rokosh and Simpson, 2002), 35% α1B (Cavalli et al., 1997), and 10% α1D (Tanoue et al., 2002).
Interestingly, the α1A- or α1B-ARs were not found in brain vascular cells or adult astrocytes using these mouse models, despite previous studies using primary and cell culture lines indicating expression of α1-ARs. α1-ARs are highly abundant in vascular smooth muscle in the periphery. This disparity can be explained in two ways. First, while a large fragment of the endogenous promoter (~4kb) was used to drive systemic expression of the α1-ARs in the transgenic mouse models used for localization studies, a limiting factor is that there may have been missing distant promoter regions that are responsible for expression of the subtypes in astrocytes and the brain vasculature. There are also studies which indicate that the α1-AR abundance and receptor subtype switches when tissue is processed into cells in culture or when the cells are immortalized (Schwarz et al., 1985; Ishac et al., 1992; Kajiyama and Ui, 1994). In studies using in vivo electrophysiology, α1-AR assignment to astrocytic function was based upon blockage using 200 μM prazosin, a dose 200-fold over its specificity for α1-ARs and could be blocking the α2-AR, which has a lower affinity for prazosin (Ding et al., 2013).
The binding and affinity of ligands for the α1-AR subtypes is similar between humans, mice and rats, showing no species differences in their pharmacological behavior. However, their localization in the brain may be different (Palacios et al., 1987; Zilles et al., 1991; Szot et al., 2005). While there are similar expression of α1-ARs in the thalamus and cerebral cortex between rodents, pigs, and humans, the expression of α1-ARs may be higher in the human hippocampus with α1A-AR subtype mRNA expressed in dentate gyrus and α1D-AR subtype mRNA expressed in the CA1-3 regions (Palacios et al., 1987; Szot et al., 2005). However, these studies used non-selective ligands and could not discriminate the subtypes or used mRNA localization which does not detect protein levels. Using the single cell polymerase chain reaction technique, the α1A-AR subtype alone was localized in the rat CA1 interneurons (Hillman et al., 2005). In additional studies using phenylephrine and the α1A-AR selective antagonist WB4101, α1A-ARs also functionally regulated the interneuron by depolarizing and subsequently releasing GABA and somatostatin (Hillman et al., 2005; Hillman et al., 2007). Using the α1-AR transgenic mouse models tagged with the EGFP, the α1A-AR expressed its highest densities in the CA1, CA3, and dentate gyrus of the mouse hippocampus and the hypothalamus (Papay et al., 2006) while the α1B-AR appeared most highly expressed in the cerebral cortex (Papay et al., 2004). Using both the EGFP transgenic and KO mouse models of the α1A- and α1B-AR subtypes, the α1A-AR subtype alone was also found to regulate the CA1 hippocampal interneurons (Jurgens et al., 2009), suggesting that the hippocampus has high α1A-AR content.
Radioligand binding studies of discreet brain domains in humans also indicate high α1-AR content in the hippocampus and prefrontal cortex cognitive domains (Shimohama et al., 1986) which were confirmed in later studies (Palacios et al., 1987; Szot et al., 2005), and in agreement with the transgenic mouse localization studies. The high, presumably α1A-AR content in human hippocampus suggests that therapeutics designed to enhance cognition through stimulation of the α1A-AR subtype may be translatable to human disease.
The α1A-AR subtype alone also regulates neurogenesis in the mouse hippocampus. The α1A- but not α1B-AR transgenic mice that systemically overexpress constitutively active receptors which are continually signaling, increased BrdU incorporation into the subgranular and subventricular zones (Gupta et al., 2009; Jurgens et al., 2009; Collette et al., 2010). Wild-type normal mice also increased BrdU incorporation when given the α1A-AR selective agonist, cirazoline, and maintained elevated levels for at least 14 days of chase, indicating that BrdU was incorporated into stem cells which divide very slowly (Gupta et al., 2009). α1-AR stimulation of adult neurospheres or co-localization studies using transgenic mice with EGFP-tagged α1A-ARs indicated that α1B- and α1A-ARs are expressed on neural and glial progenitors (Gupta et al., 2009). The EGFP-tagged α1B -ARs in the transgenic mouse model was not expressed on stem cells but was expressed on progenitors in the rostral migratory pathway. However, the transgenic α1B-AR mice did not display evidence of increasing neurogenesis (Gupta et al., 2009) as assessed by BrdU incorporation. The regulation of neurogenesis by the α1A-AR may play a role in its synaptic plasticity, regulation of cognition, and therapeutics designed to activate this receptor subtype may provide some repair to the neurodegeneration that occurs in Alzheimer’s Disease.
The NE system originates primarily in the locus coeruleus in the mammalian central nervous system (CNS) where NE is synthesized and released. From this area, different and diffuse projections of NE-releasing neurons disperse throughout the CNS to innervate the hippocampus, spinal cord, prefrontal cortex, cerebellum, thalamus, cortex, and amygdala (Iversen et al., 2009). The distribution of afferents to many key structures suggests a critical regulatory role of NE in the CNS. The NE system can modulate a number of functions such as learning and memory (Nguyen and Connor, 2019), but also depression and anxiety, sleep and arousal, brain development, motor activity, sensory information processing such as pain or touch, and to increase neurogenesis (Ordway et al., 2007a; Gupta et al., 2009). NE can cause repetitive firing and spike adaptation in the cerebral cortex, an essential function in controlling the excitability of neurons in the CNS.
Disruption in the NE system is also involved in a number of neurological diseases including Alzheimer’s disease (AD), epilepsy, attention-deficit disorder, Parkinson’s disease, depression, schizophrenia, and posttraumatic stress disorder (Ordway et al., 2007b). α1-ARs play a key role in several neurological systems and several neurological diseases that are associated with the NE system. Specificity in the NE signaling system is achieved from the nine AR subtypes even though they bind NE with very similar affinities. This is because AR subtypes couple to different G-proteins and effector proteins (Figure 1) that are temporally and spatially expressed in varying tissues throughout the brain and periphery that result in discrete expression patterns, signal transduction pathways, and physiological regulations.
The assignment of general neurological functions mediated by specific α1-AR subtypes is limited due to the lack of commercially available subtype-selective ligands with at least 100-fold selectivity between the subtypes (Table 1). α1-ARs are expressed on motor (Shao and Sutin, 1992), pyramidal neurons (Lazzaro et al., 2010; Santana et al., 2013; Zhang et al., 2013), sensory (Xie et al., 2001; Nicholson et al., 2005), Purkinje (Crepel et al., 1987; Herold et al., 2005; Schambra et al., 2005), and multiple types of interneurons (Bergles et al., 1996). The α1-ARs invoke both excitatory and inhibitory functions through postsynaptic and presynaptic mechanisms usually involving phospholipase C, protein kinase C (PKC), and/or calcium (Figure 1). α1‐AR activation can increase the firing frequency of pyramidal and somatosensory neurons of the rat visual cortex through a PKC signaling pathway (Mouradian et al., 1991; Kobayashi et al., 2008) and at presynaptic terminals to increase the inhibition of rat Purkinje neurons through phospholipase C-mediated release of calcium (Herold et al., 2005). Presynaptic α1-ARs can enhance glutamate or acetylcholine release to increase their excitation via a PKC or calcium pathway in the prefrontal cortex (Mouradian et al., 1991; Marek and Aghajanian, 1996; Chen et al., 2006; Velásquez-Martinez et al., 2012; Luo et al., 2014) or to prime excitatory synapses (Gordon and Bains, 2003). PKC is known to be involved in the phosphorylation of synaptic proteins or enhancing calcium sensitivity involved in the process of exocytosis of the vesicles (Shimazaki et al., 1996; Stevens and Sullivan, 1998; Hilfiker and Augustine, 1999; Wu and Wu, 2001). There is abundance evidence that α1-AR activation can modulate GABA-mediated inhibition in various and diverse brain regions (Mouradian et al., 1991; Marek and Aghajanian, 1996; Alreja and Liu, 1996; Bergles et al., 1996; Marek and Aghajanian, 1996; Kawaguchi and Shindou, 1998; Croce et al., 2003; Braga et al., 2004; Dumont and Williams, 2004; Herold et al., 2005; Hirono and Obata, 2006; Lei et al., 2007; Hillman et al., 2009; Kobayashi et al., 2009; Yuan et al., 2009; Salgado et al., 2011). The mechanism of α1-ARs stimulation of GABA release has been ascribed to a decrease in cellular resting conductance (McCormick et al., 1991; Bergles et al., 1996), but may also involve an increase in calcium or PKC signaling.
α1-ARs may also affect non-neuronal functions and modulate synaptic transmission as they may be expressed in astrocytes (Shao and Sutin, 1992; Bekar et al., 2008) and Bergmann glial cells, a specialized astrocyte in the cerebellum (Kulik et al., 1999). a1A-AR mRNA was expressed in astrocytes and NG2+-oligodendrocyte progenitors, but the α1B-AR mRNA was not present in any freshly isolated glial cells from the mouse cerebral cortex (Hertz et al., 2010). Progenitors and differentiated rat oligodendrocytes in culture functionally expressed only the α1A-AR subtype as assessed by its ability to increase inositol phosphate generation which was blocked by α1A-AR selective antagonists, 5-methylurapidil and WB4101 (Khorchid et al., 2002). Using transgenic mice expressing EGFP-tagged α1A-ARs, α1A-AR expression was found in NG2+-oligodendrocyte progenitors but not in mature oligodendrocytes in vivo (Papay et al., 2006). As mentioned earlier, interpretation of α1-AR expression in tissue culture cells is limited because α1-AR protein abundance changes and receptor subtype switches when tissue is processed into cells for culture or when the cells are immortalized (Schwarz et al., 1985; Ishac et al., 1992; Kajiyama and Ui, 1994).
Astrocytes are involved in neuro-glial communication to regulate the homeostasis in the brain and synaptic efficacy by controlling ion concentrations and removing excess released glutamate via transporters to prevent toxicity (Verkhratsky et al., 2019). α1-ARs expressed in astrocytes and Bergmann glia invoke a calcium flux thought to be important for neurotransmitter release and synaptic plasticity (Gordon et al., 2009; Ben Achour et al., 2010; Ben Achour and Pascual, 2010). The LC releases NE throughout the cortex and cerebellum in a phasic manner when responding to novelty, startle, or sensory input (Aston-Jones et al., 1991) and caused widespread Ca+2 signaling in cortical astrocytes that appeared to be regulated solely by the α1-AR in awake and startled but not sedated mice (Ding et al., 2013). In a similar manner, α1-AR activation on astrocytes in the mouse ventral periaqueductal grey were sufficient to increase arousal by promoting glutamate transmission (Porter-Stransky et al., 2019). The Ca+2 transients in Bergmann glial cells in locomotion-induced mice were also blocked by an α1-AR but not a β-AR antagonist (Kulik et al., 1999; Paukert et al., 2014). Therefore, α1-AR mediated calcium release in astrocytes may be the regulator of the principle astrocytic function of neuro-glia communication and particularly during sensory stimuli.
Synaptic plasticity is a change in the strength or efficacy of a synapse and is commonly believed to be part of the cognitive process. The changes that occur during short-term plasticity can last from milliseconds to seconds and are usually associated with short bursts or ripples in activity causing transient changes in calcium in the presynaptic cleft (Zucker and Regehr, 2002). Synaptic plasticity declines with age and is associated with neurodegenerative disorders, such as Alzheimer’s Disease (Bach et al., 1999). α1-AR activation can slow or stop the normal spontaneous discharges of the CA3 pyramidal neurons to the hippocampal CA1 subfield (Scanziani et al., 1993; Rutecki, 1995). Stimulation of α1-ARs can also abruptly suppress the generation of sharp wave-ripple complexes in hippocampal slices allowing for rapid interruption of activity, such as those needed when the synchronized hippocampal activity needs to be switched into attention-related activity for processing new information (Ul Haq et al., 2012). This correlated synchronized activity leads to synaptic modifications that increase synaptic strength or plasticity (Buzsaki, 1989).
Glutamatergic plasticity is increased through α1-AR stimulation in a cooperative mechanism with corticotropin-releasing factor by enhancing inositol tri-phosphate mediated calcium release (Tovar-Díaz et al., 2018). This form of NMDA-dependent synaptic plasticity was demonstrated on ventral tegmental area dopamine neurons and promoted α1-AR mediated drug-associated learning (Tovar-Díaz et al., 2018) and increased motor activity (Goertz et al., 2015). Transgenic mice overexpressing constitutive active α1A-ARs increased basal synaptic transmission and short-term plasticity as assessed by paired-pulse facilitation (PPF) at the mouse CA3 and CA1 synapse (Doze et al., 2011). PPF is a change in a paired stimulus of a synapse when observed under a short period of time (i.e milliseconds) and is a form of synaptic enhancement. The second evoked excitatory postsynaptic potential is enhanced during PPF when it is followed immediately after the first evoked excitatory postsynaptic potential (Foster and McNaughton, 1991) and is used as an indirect measure of the probability of neurotransmitter release. Changes in PPF that are associated with long-term potentiation (LTP) suggest a presynaptic mechanism (Schultz et al., 1994), as synapses that are facilitated or potentiated must increase neurotransmitter release. The above α1A-AR transgenic mouse model displayed both increased LTP and PPF, suggesting that the α1A-AR-mediated increased in short-term plasticity is mediated through a pre-synaptic mechanism (Doze et al., 2011), caused by an increase in calcium flux in the presynaptic zone which led to the release of neurotransmitter in the CA1 region. While there are many mechanisms to induce PPF, the primary mechanism appears to be through increased calcium flux in the synaptic cleft (Jackman and Regehr, 2017).
NE through activation of ARs increases the strength of synaptic transmission at glutamatergic synapses and modifies the synapses via cAMP signals and protein synthesis to increase long-term plasticity occurring over minutes to hours in duration (Hopkins and Johnston, 1984; Dahl and Sarvey, 1989; Harley, 1991; Bröcher et al., 1992; Harley and Sara, 1992; Huang and Kandel, 1996; Bramham et al., 1997; Erickson et al., 1997; Katsuki et al., 1997; Thomas and Palmiter, 1997a; Thomas and Palmiter, 1997b; Thomas and Palmiter, 1997c; Izumi and Zorumski, 1999; Watabe et al., 2000; Walling and Harley, 2004; Maity et al., 2020). As the signals involves cAMP, most previous studies have concluded that the sole AR in mediating NE effects on long-term plasticity have been the β-ARs (Maity et al., 2015; Hansen and Manahan-Vaughan, 2015; Nguyen and Gelinas, 2018). However, α1-ARs have been shown to mediate increased cAMP generation particularly in brain tissue independent of β-AR effects (Huang and Daly, 1972; Schultz and Daly, 1973; Stone et al., 1986; Stone et al., 1987).
A type of long-lasting plasticity is long-term potentiation (LTP) and is considered a major mechanism of learning and memory and has been mostly studied in the hippocampus. α1-ARs may be an important receptor in inducing long-lasting synaptic plasticity in the hippocampus (Sirviö and MacDonald, 1999). α1-AR agonists promoted while α1-AR antagonists blocked LTP formation in the rat CA1 hippocampus (Izumi and Zorumski, 1999) and may be dependent upon synergistic interaction with the β-AR through cAMP formation (Pedarzani and Storm, 1996). The activation of α1- and β-ARs facilitated tetanus-induced LTP at the mossy-fibers of the hippocampus (Hopkins and Johnston, 1984; Huang et al., 1996). α1-AR stimulation that could be blocked with prazosin also increased LTP in the dentate gyrus when acquisitioned during the learning of an active-avoidance behavior (Lv et al., 2016). The α1A-AR transgenic mice that systemically overexpresses the constitutively active α1A-AR subtype significantly improved LTP at the mouse CA3-CA1 synapse (Doze et al., 2011). These α1A-AR mice also showed increased cognition using the Barnes maze and a multi-T dry maze, while the similar mouse model that systemically expressing constitutive active α1B-AR subtype did not increase cognition (Doze et al., 2011). These transgenic studies imply that the α1A-AR subtype may be responsible for LTP and the cognitive-enhancing effects of α1-ARs.
α1-ARs also induce LTP in the neocortex. Using neocortical slices, α1-ARs stimulated on astrocytes caused the exocytosis of ATP which initiated a subsequent burst of ATP-mediated synaptic currents from ATP receptors on the pyramidal neurons inducing LTP (Pankratov and Lalo, 2015). α1-AR involvement in increasing LTP in the neocortex through releasing gliotransmitters was confirmed using terazosin to block the response. This work does suggest that α1-ARs may be involved in glia-neuron regulation of synaptic activity and plasticity. Calcium is released in the astrocytes through α1-AR activation which then can cause vesicular exocytosis of ATP to bind and activate purinergic receptors located on the adjacent pyramidal neurons (Pankratov and Lalo, 2015). Calcium is reported to be a significant signaling pathway in its communication with neurons for synaptic plasticity and LTP (Pascual et al., 2005; Guerra-Gomes et al., 2018).
α1-ARs also induced LTP in the prefrontal cortex (PFC) and is associated with increased cognition. Impaired α1-AR function following lesion in the ventral hippocampus decreased glutamatergic synaptic plasticity within the PFC and resulted in PFC-related cognitive dysfunction (Bhardwaj et al., 2014). This mechanism occurred through PKC‐dependent pathways in rat medial PFC (Luo et al., 2014) and required interaction with both glutamate release and N‐type Ca2+ channels (Luo et al., 2015b). α1-ARs also induce LTP in the ventral tegmental area on dopamine neurons through NMDA-mediated glutamatergic transmission utilizing a cooperative mechanism with corticotropin releasing factor to co-stimulate inositol triphosphate mediated calcium signaling (Tovar-Díaz et al., 2018).
Long-term depression (LTD) is also a form of long-term plasticity. Decreases in synaptic strength contribute to learning and memory functions by increasing flexibility in the synapse to store information (Heynen et al., 1996). LTD has been implicated in forms of learning and memory other than spatial memory such as the facilitation by exposure to novel objects (Manahan-Vaughan and Braunewell, 1999). Moreover, novelty exposure reversed LTP in the hippocampus (Xu et al., 1998). These findings suggest a correlation between LTD and novelty detection during learning and indicate that both LTD and LTP may impart different forms of synaptic information during spatial learning (Kemp and Manahan-Vaughan, 2004).
α1-ARs induced LTD at excitatory CA3–CA1 synapses in the rat hippocampus even when β-ARs were inhibited and 85% of the NE innervation was depleted by degeneration with the neurotoxin DSP-4 (Dyer-Reaves et al., 2019). α1-AR-mediated LTD proceeded through the ERK signaling pathway in hippocampal pyramidal neurons (Vanhoose et al., 2002; Scheiderer et al., 2008) and possessed characteristics of a novel form of synaptic plasticity (Hebb, 1949). This Hebbian-dependent LTD required coincident presynaptic and postsynaptic NMDAR activity (Scheiderer et al., 2004) and is independent of the “classical” LTD, induced by repetitive low frequency (1 Hz) synaptic stimulation (Mulkey and Malenka, 1992). The mechanism also involves postsynaptic activation of the α1-AR as the PPF ratio did not change (Scheiderer et al., 2004). In addition, there are reports that LTD required both co-activation of α1- and β-ARs (Katsuki et al., 1997) in addition to NMDA (Scheiderer et al., 2004) and the M1 muscarinic receptor (Scheiderer et al., 2008).
Serotonin neurons in the dorsal raphe nucleus regulate arousal and the modulate the response to stress (Joëls and Baram, 2009). Postsynaptic α1-AR activation using phenylephrine induced an inward current and LTD of the glutamate synapses on these serotonin neurons which was blocked using prazosin (Haj-Dahmane and Shen, 2014). Stress due to chronic restraint inhibited postsynaptic α1-AR mediated LTD on presynaptic glutamate synapses by downregulating the expression of the presynaptic CB1 cannabinoid receptor but had no effect on the α1-AR -mediated inward current. The lack of effect of stress on the α1-AR induced inward current suggests that chronic stress did not downregulate or desensitize the α1-ARs on these neurons. Synaptic plasticity may be induced via α1-AR-mediated LTD by increasing the expression of synaptic proteins (Abumaria et al., 2006). Activation of α1-ARs is also associated with inducing LTD on glutamate synapses in the visual cortex by postsynaptic mechanisms which alter the function of the α-Amino-3-hydroxy-5-methyl-4-isoxazolepropionic acid (AMPA) receptor (Kirkwood et al., 1999), NMDA (Treviño et al., 2012), and phospholipase C activation of inositol triphosphate release (Choi et al., 2005). Other brain areas that show postsynaptic α1-AR activation of LTD include the bed nucleus of the stria terminus that relays processing of the reward pathways (McElligott and Winder, 2008; McElligott et al, 2010), and in the prefrontal cortex through ERK and NMDA pathways (Marzo et al., 2010; Bhardwaj et al., 2014),
The α1-ARs have been long associated to play a role in cognition (Sirviö and McDonald, 1999); however, its extent was not well characterized because of the lack of subtype specific ligands and animal models. Some early studies suggested that α1-AR activation inhibit spatial memory and consolidation in primates but used very low numbers of animals or very high concentrations of ligands (Arnsten and Jentsch, 1997; Mao et al., 1999). There is another report that memory consolidation is inhibited by α1-AR activation in chicks (Gibbs and Summers, 2001) but this could be a species-related variable. NE is reported to have an inverted U-shaped dosage in the regulation of learning and memory (Baldi and Bucherelli, 2005) and is hypothesized to explain the mixed results of NE on memory. However, an alternate hypothesis is that high doses of NE cause desensitization and downregulation of the ARs, known to result in a negative feedback on its response and function (Rajagopal and Shenoy, 2018). As will be reviewed here, most other and later studies indicate that α1-AR activation facilitates memory, motor and motivational behavior, memory retention, and storage.
In more recent studies using the transgenic and KO mouse models, the α1B-AR KO (Cavalli et al., 1997) resulted in impaired spatial learning to novelty and exploration (Spreng et al., 2001). The α1B-AR KO mice also showed a decrease in memory consolidation and fear-motivated exploration (Knauber and Müller, 2000a). α1D-AR KO mice did not display changes in spatial and emotional learning as well as contextual fear conditioning (Sadalge et al., 2003). Transgenic mice harboring constitutively active α1A-ARs that are constitutively stimulated have enhanced learning and memory using several cognitive behavioral tests, while α1A-AR KO mice (Rokosh and Simpson, 2002) showed deficits compared to wild-type (WT) controls (Doze et al., 2011; Collette et al., 2014). In the same study, WT mice given a 2-month treatment of cirazoline in the drinking water, which is 50-fold selective agonist for the α1A-AR versus the α1B-AR subtype, also displayed increased learning and memory (Doze et al., 2011). In addition, the α1A-AR transgenic displayed increased cognition in a battery of electrophysiological tests, such as basal synaptic transmission, PPF, and LTP compared with WT mice, consistent with the increased cognition displayed through behavioral studies (Doze et al., 2011). Together, these studies suggest that both the α1A- and α1B-AR but not the α1D-AR are involved in learning and memory processes.
Stressful events that result from fear or aversive experiences have been associated with NE activation. The BLA also regulates fear conditioning (LeDoux, 2000). There is high expression of the α1A-AR subtype in that region (Domyancic and Morilak, 1997; Papay et al., 2006). α1A-AR subtype activation stimulates GABA-mediated miniature inhibitory postsynaptic currents in the BLA (Braga et al., 2004), suggesting that α1A-ARs may regulate fear conditioning. Fear conditioning promoted the excitability of the BLA by decreasing GABAergic inhibition through α1-ARs (Skelly et al., 2017) suggesting that blockage of α1-ARs may promote fear conditioned memory in the BLA (Lazzaro et al., 2010; Bernardi and Lattal, 2012) and in the prefrontal cortex which impaired conditioned fear extinction after injection of the α1-AR antagonist prazosin (Do-Monte et al., 2010). A subsequent study indicated that there was no effect of prazosin on the accrual of fear memory or retrieval but fear that was already established during prazosin treatment was more readily extinguished due to effects on the initial learning phase of the trauma (Lucas et al., 2019). This suggests that prazosin treatment could be used as a prophylactic during newly acquired fear experiences to prevent extinction-resistance.
Prazosin has been used to treat conditions that involve the return of aversive memories, such as extinguishing trauma-induced nightmares and sleep problems commonly associated with post-traumatic stress disorder in veterans (Raskind et al., 2003; Boehnlein and Kinzie, 2007; Dierks et al., 2007; Raskind et al., 2007; Miller, 2008; Taylor et al., 2008) and appear at odds with the studies that indicate α1-AR blockage promotes fear conditioning. However, a randomized, double-blinded clinical trial indicated that prazosin had no effect on nightmares or sleep disorders in veterans (Petrakis et al., 2016), indicating that previous reports were anecdotal. There were, however, significant effects of prazosin on decreasing alcohol dependency (Petrakis et al., 2016), part of the reward memory system that α1-ARs activate (Wada et al., 2020) and this could account for some of the anecdotal occurrences. The study by Lucas et al. (2019) suggests that if prazosin was given before or during the trauma induced in the veterans, nightmares would be more readily extinguished, but not after the fear conditioning had already been established.
α1-AR blockage in enhancing fear conditioning memory is also in contrast to its general role of promoting memory enhancement. A recent study has shown differential effects of NE on fear conditioning depending if the release of NE is long lasting or transient and could explain some of the discrepancies in fear-induced memory versus memory storage or spatial memory. Transient or bursting NE activity from a simple startle response elevated calcium levels in cortical astrocytes through activation of α1-ARs but prolonged NE activity during a head-fixed conditioned fear response elevated cAMP which was driven through activation of β-ARs (Oe et al., 2020). This study would suggest that low vigilance or acute stress memories may be enhanced by α1-AR activation but high vigilance and chronic stress-induced memories such as fear conditioning is promoted mostly through β-AR activation. Indeed, during chronic stress situations, α1-AR mediated stimulation of GABAergic interneurons is inhibited (Braga et al., 2004).
Spatial and associative learning is commonly used in rodent studies of long-term memory and have been hypothesized to be dependent upon the dorsal hippocampus (Mahmoodi et al., 2010). Active allothetic place avoidance (AAPA) is a type of spatial navigational learning. In hippocampus-dependent learning using the AAPA task, the combination of the α1-AR antagonist prazosin and the β-AR antagonist propranolol impaired spatial avoidance learning (Petrasek et al., 2010). Similar effects were observed when prazosin was combined with α2-AR antagonist, idazoxan (Stuchlik and Vales, 2008a) or the D2 antagonist, sulpiride (Stuchlik et al., 2008b). α1-AR stimulation in the CA1 region of dorsal hippocampus improved spatial memory (Puumala et al., 1998) and histamine-induced spatial learning in the Morris water maze test (Torkaman-Boutorabi et al., 2014) and using a touchscreen trial unique non-matching to location task (Hvoslef-Eide et al., 2015). The transgenic mice that overexpress a constitutively active α1A-AR improved spatial memory in the Barnes, Morris and multi-T type mazes (Doze et al., 2011). KO of the α1A-AR gene (Doze et al., 2011) or the α1B-AR (Spreng et al., 2001) showed spatial memory impairments in the Morris water maze (Doze et al., 2011), while α1D-AR KO mice did not show deficits in spatial learning (Sadalge et al., 2003), but they did show deficits in working memory and attention (Mishima et al., 2004).
Corticosteroids, including glucocorticoids, along with norepinephrine are the two systems in the body that mediate the stress response and cause the body to adapt (de Kloet et al., 2005). Corticosteroids can have effects on learning and memory via interacting directly with their own receptors to mediate transcriptional or non-transcriptional effects due to stress (Wang et al., 2014) or interact and crosstalk with norepinephrine and its receptors in the hippocampus, prefrontal cortex, and the basolateral nucleus of the amygdala (Krugers et al., 2012) to increase astrocytic calcium waves, gliotransmitters, and glutamate release (Simard et al., 1999; Parpura et al., 2011) to effect cognition (Hassanpoor et al., 2014; Pearson-Leary et al., 2016; LaLumiere et al., 2017). Corticosteroid receptors also co-localize with α1-ARs and may even directly regulate each other (Williams et al., 1997). Glucocorticoid release during stress can cause spatial memory deficits in males and do so by increasing the frequency and amplitude of IPSCs. α1-AR induced increase in IPSCs are even further stimulated when co-stimulated with a stress-released glucocorticoid (Hartner and Schrader, 2018). This effect required the pretreatment with the synthetic glucocorticoid, dexamethasone, to prime the cells to respond to α1-AR activation, suggesting that corticosteroids modulate the signaling pathways of α1-ARs to mediate effects on memory (Hartner and Schrader, 2018). These results suggest a potential mechanism for spatial memory deficits caused by NE during increased stress which may be mediated through the α1-AR and its interactions with corticosteroid hormone receptors.
The PFC can regulate goal-directed or motivational-related behavior planning and attention processes (Robbins and Arnsten, 2009). Spatial working memory relies on the function of the PFC and is targeted by various therapeutics to treat cognitive dysfunction. The PFC is required for temporary information storage during the execution of complex tasks. NE innervates the PFC (Lewis and Morrison, 1989) from afferents from the locus coeruleus.
All three families of ARs (α1, α2, β) are expressed throughout the PFC (Nicholas et al., 1993; Pieribone et al., 1994). The α2A-AR subtype may play a role in PFC-dependent cognition (Wang et al., 2007) and along with β-ARs, α1-ARs are also required for spatial memory, as α1-AR agonists increase and antagonists inhibit the formation of working memory (Puumala et al., 1998) and promote both focused and flexible attention (Berridge and Spencer, 2016). The radial arm maze is a type of spatial working memory test which retention is improved upon α1-AR activation (Pussinen et al., 1997). Attentional set shifting is also a test of spatial working memory that is also dependent upon the PFC. When there are elevated levels of NE release presynaptic vesicles, rats improved performance in working memory. Under these conditions of elevated NE release, α1-AR but not β-AR blockers blocked the improvement in working memory using the attentional set shifting test (Lapiz and Morilak, 2006). The effects of PFC-infused with the α1-AR agonist phenylephrine improved spatial working memory in a location task in rats (Hvoslef-Eide et al., 2015).
Psychostimulants such as methylphenidate improve sustained memory through α1-ARs (Berridge et al., 2012). There is also an improvement in working memory with the cognitive-enhancing drug modafinil that is hypothesized to be mediated by α1-ARs since effects are blocked by prazosin (Duteil et al., 1990; Stone et al., 2002; Winder-Rhodes et al., 2010). Modafinil is a wake-promoting non-amphetamine neurochemical with complex properties that can directly stimulate cortical catecholamine levels and indirectly stimulate serotonin along with other neurotransmitters (Minzenberg and Carter, 2008; Chen et al., 2013; Scoriels et al., 2013). Together, these results suggest that α1-AR activation could be used to target enhancement of spatial working memory.
The mechanism of α1-AR regulation of PFC-mediated spatial working memory is likely due to increasing the release of glutamate from glutamatergic terminals within the PFC and promoting a persistent excitatory effect of pyramidal neurons (Marek and Aghajanian, 1999; Zhang et al., 2013; Bhardwaj et al., 2014). It is enhanced when the presynaptic α1-ARs are facilitated by postsynaptic α2-ARs inhibition of hyperpolarization-activated cyclic nucleotide-gated cation channels (Zhang et al., 2013) or post-synaptic via PKC-mediated enhancement of AMPA and NMDA excitatory currents (Luo et al., 2014).
In another study, phenylephrine also increased GABAergic transmission onto the pyramidal neurons in the medial PFC through inhibiting the interneuron inwardly rectifying potassium channels (Kirs), which caused the depolarization of the interneuron leading to an increased calcium influx through calcium channels (Luo et al., 2015a). The disruption of GABAergic transmission in the PFC can also produce impairments in working memory (Enomoto et al., 2011; Bañuelos et al., 2014). Therefore, α1-ARs may work to improve spatial working memory through both glutamatergic and GABAergic mechanisms.
The medial PFC is also involved in reward-related memories by receiving major dopamine (DA) neurotransmission from the ventral tegmental area (VTA), which then projects back onto the VTA and the nucleus accumbens in the forebrain. These networks play a prominent role in the reward circuitry (Tzschentke, 2000; Schultz, 2015).
The VTA receives major inhibitory GABAergic innervation from the nucleus accumbens as well as other areas in the brain (Jhou et al., 2009a; Jhou et al., 2009b) that controls their patterns in firing (Paladini and Tepper, 1999; Lobb et al., 2010) and contributes to burst activation and prolonged activity of DA neurons in the nucleus accumbens and prefrontal cortex (Paladini et al., 2003; Jhou et al., 2009a; Lobb et al., 2010; Morikawa and Paladini, 2011; Lohani et al., 2018). Since burst-firing can result in increased efficacy and enhanced neurotransmitter release in the presynaptic terminal (Fisher et al., 1997; Floresco et al., 2003), GABAergic regulation of DA bursting activity is one way to modulate reward-related memories. DA can cause the co-activation of α1-ARs (Leedham and Pennefather, 1986; Rey et al., 2001; Cornil et al., 2002; Zhang et al., 2004; Lazou et al., 2006; Lin et al., 2008) and α1-ARs are expressed on presynaptic terminals in the nucleus accumbens to regulate DA neurotransmission (Paladini et al., 2001; Cui et al., 2004; Mitrano et al., 2012).
DA neurons increased short-term burst firing in reaction to rewards while drugs that are addicting produce repetitive bursting (Covey et al., 2014; Keiflin and Janak, 2015). α1-ARs have also been shown to increase VTA-DA neurotransmission and induce burst firing (Grenhoff et al., 1993; Grenhoff and Svensson, 1993; Grenhoff et al., 1995; Paladini and Williams, 2004). Presynaptic α1-AR also facilitated glutamatergic inputs that affect VTA-DA neurotransmission (Velásquez-Martinez et al., 2012; Bocklisch et al., 2013) and participates in addiction-related effects (Cecchi et al., 2002; Jimenez-Rivera et al., 2006; Greenwell et al., 2009). α1-AR stimulation revealed a cooperative mechanism with corticotropin-releasing factor (CRF) on VTA neurons that increased NMDA receptor-mediated glutamatergic plasticity to induce the learning of cocaine-associated behavior (Tovar-Díaz et al., 2018). The CRF co-stimulated inositol triphosphate-mediated calcium release along with the α1-AR activation of these signals and blockage of the conditioning was suppressed by co-administration of both CRF and α1-AR blockers (Tovar-Díaz et al., 2018). α1-AR stimulation in the medial PFC increased cocaine craving, effects that were blocked by the α1-AR antagonist terazosin (Wada et al., 2020). α1-AR blockage by prazosin also decreased the motivational memory of nicotine (Forget et al., 2009). These results suggest that changes in the α1-AR signaling induced through drugs of abuse could be part of the neuromodulation occurring in the reward circuitry during the development of addicting behavior.
The entorhinal cortex (EC) facilitates the neuronal connections and communication between the hippocampus and cortical areas that are required for consolidation and recall of memories (Haist et al., 2001; Squire et al., 2004; Dolcos et al., 2005; Steffenach et al., 2005). Alteration in communication between the principal cells and the interneurons in the EC is a mechanism for the ability to process spatial information which is disrupted when there are spatial memory deficits (Couey et al., 2013; Pastoll et al., 2013).
The EC expresses α1-ARs (Stanton et al., 1987) and is prominent in α1A-AR density (Papay et al., 2006). α1-ARs increased spontaneous inhibitory postsynaptic currents (IPSCs) in both frequency and amplitude when recorded from the principal neurons in the EC (Lei et al., 2007). DA facilitated the α1-ARs-mediated GABA release in the EC by inhibiting Kirs, a potassium channel which further depolarizes interneurons resulting in Ca+ influx via T-type Ca+ channels (Cilz et al., 2014). Inhibitory inputs are important for the activity in the EC. Changes in the signal-to-noise ratio of the inhibitory signals could alter the theta-nested gamma oscillations that are needed for spatial memory processing (Chrobak and Buzsaki, 1998; Quilichini et al., 2010; Colgin, 2015; Colgin, 2016).
α1-AR activation can enhance memory recall and consolidation. The α1-AR antagonist, prazosin, blocked the NE-facilitated effects of reconsolidation during fear conditioning (Gazarini et al., 2013). Using a discriminative avoidance task, α1-ARs were necessary for the consolidation both short-term and intermediate-term memory in the chick (Gibbs and Bowser, 2010). This mechanism was suggested to be mediated through an increased calcium release though astrocytic α1-ARs as effects were blocked with metabolic inhibitors for astrocytes (Gibbs and Bowser, 2010). Astrocytes, unlike neurons, mediate learning and memory upon glycogenolysis needed for the synthesis of glutamate (Gibbs et al., 2008; Newman et al., 2011).
NE regulation of the basolateral nucleus of the amygdala (BLA) is involved in the consolidation and storage of memory (Ferry and McGaugh, 2000). While the β-ARs are usually considered the main mechanism of NE’s effects on memory consolidation through the cAMP pathway (Ikegaya et al., 1997; Ferry and McGaugh, 1999), β-ARs and α1-AR may be needed together to enhance memory storage in the BLA. α1-AR blockage in the BLA decreased the stimulation of cAMP through a β-AR agonist or the increased effect of a synthetic cAMP analog on memory storage (Ferry et al., 1999a; Ferry et al., 1999b), while activation of α1-ARs can potentiate β-AR-mediated increases in cAMP formation in the BLA to enhance memory storage (Ferry et al., 1999a; Ferry et al., 1999b). Phenylephrine alone impaired memory retention in the BLA but when infused with the α2-AR antagonist yohimbine increased memory retention (Ferry and Quirarte, 2012) suggesting that the cross talk of activating presynaptic α2-ARs led to the memory impairing effects. KO mice that has the gene deletion for the α1B-AR indicated a decrease in latency in the passive avoidance test suggesting deficits in memory consolidation (Knauber and Müller, 2000b).
In the rat dorsal hippocampal CA1 regions, α1-AR activation reversed cannabinoid-induced amnesia (Moshfegh et al., 2011). Pre-test dorsal hippocampal intra-CA1 administration of the α1-AR agonist phenylephrine reversed the loss of memory during retrieval that was induced with the synthetic cannabinoid agonist, WIN55,212-2. Pre-test use of an α1-AR antagonist prazosin inhibited the WIN55,212-2 response (Moshfegh et al., 2011). There was a similar effect of α1-AR activation on reversing scopolamine-induced amnesia (Azami et al., 2010) and when agonists were administered before electroconvulsive shocks that decreased the induced amnesia and enhanced recall using a passive avoidance test (Anand et al., 2001).
The mammalian main olfactory bulb (MOB) receives major modulatory input from the locus coeruleus (McLean et al., 1989). MOB exhibits one of the highest densities of α1-ARs using autoradiography of the non-selective radiolabeled antagonist I125-HEAT (Jones et al., 1985) and specifically for the α1A-AR subtype (Domyancic and Morilak, 1997; Papay et al., 2016). Olfactory stimuli evokes NE release in the MOB and NE is an important signal in specific olfactory learning, memory, reward-motivated discrimination, and pheromonal regulation of reproductive/maternal behaviors through the excitation of mitral cells (Pissonnier et al., 1985; Sullivan et al., 1989; Kendrick et al., 1991; Sullivan et al., 1992; Rangel and Leon, 1995; Jiang et al., 1996; Brennan et al., 1998; Guérin et al., 2008; Mandairon et al., 2008; Eckmeier and Shea, 2014; Harvey and Heinbockel, 2018).
Both NE and the α1-AR agonist phenylephrine increased evoked activation of mitral cells that were also inhibited by the α1A-AR selective antagonist WB-4101 (Ciombor et al., 1999). The inward current caused by α1-ARs are mediated by decreased K+ conductance (Hayar et al., 2001). α1-AR are known to be a major effector of the NE released in the MOB and the resulting GABAergic inhibition on the mitral cells, increasing their excitation (Perez et al., 1987; Mouly et al., 1995; Ciombor et al., 1999; Nai et al., 2010) and particularly though the α1A-AR subtype (Ciombor et al., 1999; Zimnik et al., 2013). Transgenic mice that systemically overexpress constitutively active α1A-ARs or in normal mice given the α1A-AR selective agonist, cirazoline, increased adult neurogenesis as assessed though increased BrdU incorporation in the subventricular and subgranular zones and the number of neuronal progenitors migrating to the MOB (Gupta et al., 2009). The MOB receives and continually integrates newly generated neurons through neurogenesis all throughout adult life (Ming and Song, 2005). These neurons develop and integrate as GABAergic interneurons (Panzanelli et al., 2009; Valley et al., 2013). Sensory synaptic plasticity commonly occurs during dynamic increases in inhibition (Carcea and Froemke, 2013). Together, these results suggest that the α1A-AR subtype could be responsible for the various forms of learning and memory in the MOB through its ability to increase GABAergic interneuron inhibition of mitral cells.
These studies are opposite to what was shown in the accessory olfactory bulb located at the posterior region of the olfactory bulb. Presynaptic activation of α1-ARs increased GABA-induced miniature IPSCs frequency to increase the release of GABA from granule cells in the accessory olfactory bulb and decrease the excitability of mitral cells (Araneda and Firestein, 2006). α2-ARs are also present in the MOB but their activation suppressed IPSCs (Nai et al., 2009; Nai et al., 2010). NE effects on the MOB have been shown to be biphasic and can cause both excitation and inhibition of mitral cells (Okutani et al., 1998). Together, these results could suggest that way that α1-ARs regulate olfactory memories could be different in the accessory olfactory bulb versus the MOB.
Pathology and degeneration of the neurons in the EC may also be contributors to AD progression (Hyman et al., 1984; Kotzbauer et al., 2001) and NE innervation in the medial EC is decreased in rodent models of AD (Chalermpalanupap et al., 2017; Rorabaugh et al., 2017). There is also sprouting of NE fibers in the hippocampus and PFC of subjects with AD, consistent with regeneration that is observed after neuronal loss (Szot et al., 2006). AD patients have elevated NE levels even during cell loss which is thought to be due to compensatory changes (Szot et al., 2006).
The LC is the provider of NE innervation (Compton et al., 1995; Aston-Jones, 2004), and modulates the synaptic efficiency needed for cognition (Harley, 1991; Kemp and Manahan-Vaughan, 2004; Scheiderer et al., 2004; Harley, 2007). Degeneration of the LC along with the first signs of tau pathology in AD has been well documented (Forno, 1966; Yamada and Mehraein, 1977; Zarow et al., 2003; Grudzien et al., 2007; Braak et al., 2011; Jucker and Walker, 2011; Arendt et al., 2015; Chalermpalanupap et al., 2017; Kelly et al., 2017; Theofilas et al., 2017). The hippocampus showed a decline in LTP with advancing age indicating an impairment in synaptic plasticity (Landfield and Lynch, 1977). Visual-induced memory loss in the perirhinal cortex during AD is also impaired through decreases in LTD in a mouse model of AD (Tamagnini et al., 2012). The study of Dyer-Reaves et al. (2019) indicated that α1-ARs induced LTD even when 85% of the NE innervation was lost through degeneration, suggesting that α1-AR agonists could be used as a treatment for the cognitive decline associated with AD that is due to neurodegeneration.
α1-ARs have been previously associated with AD, but there are no previous studies exploring the effect of AR agonists and antagonists in clinical studies, only assessed through changes in receptor density and mRNA. Changes in α1-AR function may contribute to aging process in the loss of memory function. There is a report that α1-AR density is upregulated in the aged mouse brain and improved passive avoidance learning, supporting a role for these receptors in age-related cognitive decline (Knauber and Müller, 2000b). Changes in PFC function can have effects on learning and memory (Brozoski et al., 1979) and is also an area of degeneration in AD (Poirel et al., 2018). The mRNA expression of the α1A-AR specifically was significantly decreased in the layers of the PFC in patients with AD with no changes in the α2-AR (Szot et al., 2007) and there is an α1A-AR polymorphism associated with AD (Hong et al., 2001). Overall α1-AR receptor density as assessed through radioligand binding in AD is also reduced by 25% (Shimohama et al., 1986). Age-related impairments in spatial memory using the Morris water maze in rats indicated that stimulation of the α1-AR improved cognition (Riekkinen et al., 1997). These results suggest that therapies to increase α1-AR signaling may be able to improve cognitive decline in AD.
After AD, vascular dementia is the second-most frequent form of dementia. Agonistic autoantibodies against the α1-ARs were found to be significantly present in 50% of people diagnosed with dementia, and in particular with those who also had heart disease (Karczewski et al., 2012a; Hempel et al., 2016; Thyrian et al., 2018). These autoantibodies bound to the first extracellular loop of the α1A-AR subtype, suggesting that this was the epitope used by the body to generate the autoantibodies (Karczewski et al., 2012a). The first extracellular loop of the α1A-AR would be able to confer specificity of the antibody to this subtype because the extracellular loops of the ARs are the least conserved between family members in their amino acid sequence. Cognitive function can be stabilized over a long period with the complete removal of the antibodies (Karczewski et al., 2018). Using animal models, it was demonstrated that α1-AR autoantibodies caused vascular impairment in the brain and induced a type of vascular dementia (Zhou et al., 2008; Karczewski et al., 2012a; Karczewski et al., 2012b; Pohlmann et al., 2014). While these results suggest that activation of α1-ARs may lead to disease progression, GPCR autoantibodies may be generated only after the disease is present, suggesting that the body is trying to compensate for the loss of the receptor function during the course of the disease. For example, agonistic autoantibodies against the angiotensin receptor induced vasoconstriction as seen for the agonist, angiotensin II, only in ischemic but not normal arteries (Lukitsch et al., 2012). Similar results were found when antagonistic autoantibodies of the β2-AR only inactivated the receptor during ischemic but not normal cell culture conditions in myocytes (Wallukat and Wollenberger, 1987). Therefore, agonistic autoantibodies against the α1A-AR may develop during AD to compensate for their loss in receptor density as documented by Szot et al. (2007) and Shimohama et al. (1986).
Interestingly, autoantibodies against the α1-AR were often found together with β2-AR autoantibodies in dementia patients (Karczewski et al., 2012a; Perez et al., 2019). The β2-AR autoantibodies were directed against the amino acid sequences in the second extracellular loop but could display both agonist and antagonistic effects (Mijares et al., 2000). It was postulated that receptor autoantibodies can act as an agonist when the α1 and β2-AR receptors dimerize and as an antagonist on the receptor monomer. While both α1- and β2-AR can form receptor homodimers, only the α1B-AR subtype was shown to not form heterodimers with the β2-AR (Stanasila et al., 2003). It could be speculated that the α1A-AR can form heterodimers with the β2-AR and autoantibodies against them can trans-inhibit their function, produce negative allosteric interactions, altered or new trafficking of signaling pathways, which are common effects with GPCR heterodimers (Haack and McCarty, 2011).
A major function and limitation of the α1-AR subtypes are the ability to contract vascular smooth muscle which results in the regulation of blood pressure. α1-ARs efficiently couple to the Gq G-protein that activates the effector phospholipase C causing the release of inositol triphosphate (IP3) from membrane phospholipids. It is IP release that increases intracellular calcium that contracts smooth muscle causing increased blood pressure for agonists that activate this receptor (Heagerty et al., 1986; Huzoor-Akbar et al., 1989). This side effect of blood pressure regulation is a major reason why drug development essentially halted for this receptor. All of the commercially available ligands for the α1-AR have the potential to interact with the other subtypes and/or crossover to β-AR or α2-ARs. There are no existing ligands that demonstrate more than 100-fold selectivity between the α1-AR subtypes. Ligands with 50 to 100-fold selectivity are good research tools to dissect functions of the different subtypes, but not selective enough for therapeutics.
Allosteric modulators are currently being pursued in the pharmaceutical industry as the next wave of therapeutics to treat disease. There are several advantages to using allosteric modulators when compared to current orthosteric agonists (Christopoulos, 2002). The effects of allosteric ligands are saturating; once the allosteric sites are fully occupied by a drug, there is no further observed allosteric effect. In contrast, drugs that bind to the orthosteric site can have continuous effects and compete for occupancy determined by the relative concentrations of the two species (i.e. endogenous neurotransmitter vs. orthosteric drug). Therefore, there is a ceiling to the potential effects of an allosteric drug and can be given in high doses without the concern of causing additional side effects from overstimulating the system. Another advantage of allosteric drugs is their ability to selectively activate the receptor only in places in which the endogenous agonist is binding and signaling. This is achieved because allosteric modulators are often ligand-specific and signaling-biased in the conformational changes they induce (i.e. NE and cAMP specific). In addition, normal neurohumoral signaling involves the activation of nerves that release neurotransmitters in temporal and spatial settings. An allosteric modulator can cause its effects only when the endogenous agonist it specifically modulates is present. If neurotransmission is reduced, the allosteric drug would have little effect, even if the neurotransmitter is still present in the body. Furthermore, positive allosteric modulators may enhance signaling when receptors are degenerating since they potentiate or enhance existing signals. Allosteric drugs also could have greater receptor selectivity. The amino acids that contribute to allosteric binding sites are usually different from those that comprise the orthosteric binding site, which are usually the regions with highest conservation between receptor subtypes. For example, the amino acids that comprise the agonist binding pocket for ARs share a number of common residues. Therefore, while all the ARs may be stimulated by NE, only an α1A-AR positive allosteric modulator will enhance the α1A-AR NE-mediated effects, achieving subtype selective responses.
Conformational changes and signaling-bias, crucial benefits of allosteric modulators were recognized early for the α1-ARs. In the early 1980s it was recognized that the way phenethylamine agonists bound and signaled through the α1-AR was different from the way imidazoline agonists interacted (Ruffolo and Waddell, 1982). While most imidazolines have better selectivity for α2-ARs (Ruffolo and Waddell, 1983), key differences in the substitutions can render imidazolines α1-AR selective (Ruffolo et al, 1980; Hieble et al., 1986; Knepper et al., 1995). The α1A-ARs are preferentially activated by imidazolines (Minneman et al., 1994) and imidazolines have biased-signaling towards the cAMP response when compared to the primary pathway and blood pressure-inducing signaling of IP/Ca+2 even though they are not allosteric modulators (Evans et al., 2011; da Silva et al., 2017). α1A-AR imidazoline partial agonists have been shown to mediate functions that are uncoupled from blood pressure at low doses (Blue et al, 2004; Musselman et al., 2004). Based upon the hypothesis that α1A-AR stimulation would increase cognition, my laboratory developed a series of positive allosteric modulators that are not agonists and do not evoke an IP response on their own, but conformationally potentiate the cAMP response of NE (Perez et al., 2019). It is postulated that cAMP is the cognitive signal that induces memory formation through α-ARs (Ferry et al., 1999a; Ferry et al., 1999b) as well as for NE-mediated effects on memory (Liang et al., 1986; Liang et al., 1990; Introini-Collison et al., 1991; Katsuki et al., 1997; 82-84; 65). These α1A-AR positive allosteric modulators are currently in pre-clinical studies.
There are three published reports of allosteric modulation at α1-ARs, and all are described as negative allosteric modulators with no known clinically-useful application as of yet (Leppik et al., 2000; Sharpe et al., 2003; Campbell et al., 2017). Positive allosteric modulators have also been developed for the β2-AR (Ahn et al., 2018; Liu et al., 2019). While these modulators are selective for the β2-AR and display cooperative signals with agonists, they are not ligand-selective nor signaling-biased. However, all these studies give hope for the eventual development of allosteric modulators for the ARs that may be used to treat disease and specifically for AD.
There is substantial evidence that α1-ARs play an important role in synaptic efficacy and plasticity with effects on increasing learning and memory functions. There is no longer any controversy on their roles in cognition that was prevalent in the 1990s and early 2000s due to the use of nonselective ligands or using too high concentrations of ligands that would cross talk with other ARs. α1-ARs are expressed on a wide variety of neurons but also on glia and both contribute to its effects on cognition. α1-AR activation can improve conditioned fear, spatial, reward, olfactory, storage, recall and consolidation of memories. Consolidating and understanding the mechanisms involved in neurotransmission, learning, and memory may lead to new treatments in neurodegenerative diseases and age or disease-mediated cognitive decline. With the advent of allosteric drugs for GPCRs, α1-ARs that may become an effective treatment option in AD and other dementias without the unwanted side effects on blood pressure.
The author confirms being the sole contributor of this work and has approved it for publication.
This work was supported by a grant from NIH RO1AG066627 and The Edward N. & Della L. Thome Memorial Foundation Awards Program in Alzheimer’s Disease Drug Discovery Research.
The author declares that the research was conducted in the absence of any commercial or financial relationships that could be construed as a potential conflict of interest.
Abumaria, N., Rygula, R., Havemann-Reinecke, U., Rüther, E., Bodemer, W., Roos, C., et al. (2006). Identification of genes regulated by chronic social stress in the rat dorsal raphe nucleus. Cell Mol. Neurobiol. 26, 145–162. doi: 10.1007/s10571-006-9024-1
Ahlquist, R. P. (1948). A study of the adrenotropic receptors. Am. J. Physiol. 153, 586–600. doi: 10.1152/ajplegacy.1948.153.3.586
Ahn, S., Pani, B., Kahsai, A. W., Olsen, E. K., Husemoen, G., Vestergaard, M., et al. (2018). Small-Molecule Positive Allosteric Modulators of the β2-Adrenoceptor Isolated from DNA-Encoded Libraries. Mol. Pharmacol. 94, 850–861. doi: 10.1124/mol.118.111948
Alreja, M., Liu, W. (1996). Noradrenaline induces IPSCs in rat medial septal/diagonal band neurons: involvement of septohippocampal GABAergic neurons. J. Physiol. 494 (Pt 1)(Pt 1), 201–215.
Anand, A., Andrade, C., Sudha, S., Guido, S., Venkataraman, B. V. (2001). Phenylephrine and ECS-induced retrogradee amnesia. J. ECT. 17, 166–169. doi: 10.1097/00124509-200109000-00003
Araneda, R. C., Firestein, S. (2006). Adrenergic Enhancement of Inhibitory Transmission in the Accessory Olfactory Bulb. J. Neurosci. 26 (12), 3292–3298. doi: 10.1523/JNEUROSCI.4768-05.2006
Arendt, T., Brückner, M. K., Morawski, M., Jäger, C., Gertz, H.-J. (2015). Early neuron loss in Alzheimer’s disease: cortical or subcortical? J. Neurosci. Methods 3, 10. doi: 10.1186/s40478-015-0187-1
Arnsten, A. F., Jentsch, J. D. (1997). The α1-adrenergic agonist, cirazoline, impairs spatial working memory performance in aged monkeys. Pharmacol. Biochem. Behav. 58, 55–59. doi: 10.1016/S0091-3057(96)00477-7
Aston-Jones, G., Chiang, C., Alexinsky, T. (1991). Discharge of noradrenergic locus coeruleus neurons in behaving rats and monkeys suggests a role in vigilance. Prog. Brain Res. 88, 501–520. doi: 10.1016/S0079-6123(08)63830-3
Aston-Jones, G. (2004). “Locus coeruleus, A5 and A7 noradrenergic cell groups,” in The Rat Nervous System. Ed. Paxinos, G. (San Diego, CA: Elsevier), 259–294.
Azami, N. S., Piri, M., Oryan, S., Jahanshahi, M., Babapour, V., Zarrindast, M. R. (2010). Involvement of dorsal hippocampal alpha-adrenergic receptors in the effect of scopolamine on memory retrieval in inhibitory avoidance task. Neurobiol. Learn. Mem. 93, 455–462. doi: 10.1016/j.nlm.2010.01.003
Bach, M. E., Barad, M., Son, H., Zhuo, M., Lu, Y. F., Shih, R., et al. (1999). Age-related defects in spatial memory are correlated with defects in the late phase of hippocampal long-term potentiation in vitro and are attenuated by drugs that enhance the cAMP signaling pathway. Proc. Natl. Acad. Sc.i U.S.A. 96, 5280–5285. doi: 10.1073/pnas.96.9.5280
Baker, J. G., Proudman, R. G., Hill, S. J. (2015). Salmeterol’s extreme β2 selectivity is due to residues in both extracellular loops and transmembrane domains. Mol. Pharmacol. 87 (1), 103–120. doi: 10.1124/mol.114.095364
Baldi, E., Bucherelli, C. (2005). The Inverted “U-Shaped” Dose-Effect Relationships in Learning and Memory: Modulation of Arousal and Consolidation. Nonlinear. Biol. Toxicol. Med. 3 (1), 9–21. doi: 10.2201/nonlin.003.01.002
Bañuelos, C., Beas, B. S., McQuail, J. A., Gilbert, R. J., Frazier, C. J., Setlow, B., et al. (2014). Prefrontal cortical GABAergic dysfunction contributes to age-related working memory impairment. J. Neurosci. 34, 3457–3466. doi: 10.1523/JNEUROSCI.5192-13.2014
Bekar, L. K., He, W., Nedergaard, M. (2008). Locus coeruleus alpha-adrenergic-mediated activation of cortical astrocytes in vivo. Cereb. Cortex. 18 (12), 2789–2795. doi: 10.1093/cercor/bhn040
Ben Achour, S., Pascual, O. (2010). Glia: the many ways to modulate synaptic plasticity. Neurochem. Int. 57, 440–445. doi: 10.1016/j.neuint.2010.02.013
Ben Achour, S., Pont-Lezica, L., Béchade, C., Pascual, O. (2010). Is astrocyte calcium signaling relevant for synaptic plasticity? Neuron Glia Biol. 6 (3), 147–155. doi: 10.1017/S1740925X10000207
Bergles, D. E., Doze, V. A., Madison, D. V., Smith, S. J. (1996). Excitatory actions of norepinephrine on multiple classes of hippocampal CA1 interneurons. J. Neurosci. 16, 572–585. doi: 10.1523/JNEUROSCI.16-02-00572.1996
Bernardi, R. E., Lattal, K. M. (2012). Prazosin differentially affects extinction of cocaine conditioned place preference on the basis of dose and initial preference. Neuroreport. 23, 1048–1051. doi: 10.1097/WNR.0b013e32835ad246
Berridge, C. W., Spencer, R. C. (2016). Differential cognitive actions of norepinephrine a2 and a1 receptor signaling in the prefrontal cortex. Brain Res. 1641 (Pt B), 189–196. 15. doi: 10.1016/j.brainres.2015.11.024
Berridge, C., Shumsky, J. S., Andrzejewski, M. E., McGaughy, J., Spencer, R. C., Devilbiss, D., et al. (2012). Differential sensitivity to psychostimulants across prefrontal cognitive tasks: differential involvement of Noradrenergic α1- vs. α2-Receptors. Biol. Psychiatry 71, 467–473. doi: 10.1016/j.biopsych.2011.07.022
Bhardwaj, S. K., Tse, Y. C., Ryan, R., Wong, T. P., Srivastava, L. K. (2014). Impaired adrenergic-mediated plasticity of prefrontal cortical glutamate synapses in rats with developmental disruption of the ventral hippocampus. Neuropsychopharmacology. 39 (13), 2963–2973. doi: 10.1038/npp.2014.142
Blendy, J. A., Grimm, L. J., Perry, D. C., West-Johnsrud, L., Kellar, K. J. (1990). Electroconvulsive shock differentially increases binding to alpha-1 adrenergic receptor subtypes in discrete regions of rat brain. J. Neurosci. 10 (8), 2580–2586. doi: 10.1523/JNEUROSCI.10-08-02580.1990
Blue, D. R., Daniels, D. V., Gever, J. R., Jett, M. F., O'Yang, C., Tang, H. M., et al. (2004). Pharmacological characteristics of Ro 115-1240, a selective α1A/L-adrenoceptor partial agonist: a potential therapy for stress urinary incontinence. BJU Int. 93 (1), 162–170. doi: 10.1111/j.1464-410X.2004.04577.x
Bocklisch, C., Pascoli, V., Wong, J. C., House, D. R., Yvon, C., de Roo, M., et al. (2013). Cocaine disinhibits dopamine neurons by potentiation of GABA transmission in the ventral tegmental area. Science. 341, 1521–1525. doi: 10.1126/science.1237059
Boehnlein, J. K., Kinzie, J. D. (2007). Pharmacologic reduction of CNS noradrenergic activity in PTSD: the case for clonidine and prazosin. J. Psychiatr. Pract. 13, 72–8. doi: 10.1097/01.pra.0000265763.79753.c1
Braak, H., Thal, D. R., Ghebremedhin, E., Del Tredici, K. (2011). Stages of the pathologic process in Alzheimer disease: age categories from 1 to 100 years. J. Neuropathol. Exp. Neurol. 70, 960–969. doi: 10.1097/NEN.0b013e318232a379
Braga, M. F., Aroniadou-Anderjaska, V., Manion, S. T., Hough, C. J., Li, H. (2004). Stress impairs alpha(1A) adrenoceptor-mediated noradrenergic facilitation of GABAergic transmission in the basolateral amygdala. Neuropsychopharmacology 29, 45–58. doi: 10.1038/sj.npp.1300297
Bramham, C. R., Bacher-Svendsen, K., Sarvey, J. M. (1997). LTP in the lateral perforant path is β-adrenergic receptor-dependent. Neuroreport. 8, 719–724. doi: 10.1097/00001756-199702100-00028
Brennan, P. A., Schellinck, H. M., de la Riva, C., Kendrick, K. M., Keverne, E. B. (1998). Changes in neurotransmitter release in the main olfactory bulb following an olfactory conditioning procedure in mice. Neuroscience. 87, 583–590. doi: 10.1016/S0306-4522(98)00182-1
Bröcher, S., Artola, A., Singer, W. (1992). Agonists of cholinergic and noradrenergic receptors facilitate synergistically the induction of long-term potentiation in slices of rat visual cortex. Brain. Res. 573, 27–36. doi: 10.1016/0006-8993(92)90110-U
Brozoski, T. J., Brown, R. M., Rosvold, H. E., Goldman, P. S. (1979). Cognitive deficit caused by regional depletion of dopamine in prefrontal cortex of rhesus monkey. Science 205, 929–932. doi: 10.1126/science.112679
Buzsaki, G. (1989). Two stage model of memory trace formation: a role for “noisy” brain states. Neuroscience. 31, 551–570. doi: 10.1016/0306-4522(89)90423-5
Campbell, A. P., Wakelin, L. P., Denny, W. A., Finch, A. M. (2017). Homobivalent Conjugation Increases the Allosteric Effect of 9-aminoacridine at the α1-Adrenergic Receptors. Mol. Pharmacol. 91 (2), 135–144. doi: 10.1124/mol.116.105874
Carcea, I., Froemke, R. C. (2013). Cortical plasticity, excitatory-inhibitory balance, and sensory perception. Prog. Brain. Res. 207, 65–90. doi: 10.1016/B978-0-444-63327-9.00003-5
Cavalli, A., Lattion, A. L., Hummler, E., Nenniger, M., Pedrazzini, T., Aubert, J. F., et al. (1997). Decreased blood pressure response in mice deficient of the alpha1b-adrenergic receptor. Proc. Natl. Acad. Sci. USA. 94, 11589–11594. doi: 10.1073/pnas.94.21.11589
Cecchi, M., Khoshbouei, H., Javors, M., Morilak, D. A. (2002). Modulatory effects of norepinephrine in the lateral bed nucleus of the stria terminalis on behavioral and neuroendocrine responses to acute stress. Neuroscience 112, 13–21. doi: 10.1016/S0306-4522(02)00062-3
Chalermpalanupap, T., Weinshenker, D., Rorabaugh, J. M. (2017). Down but not out: the consequences of pretangle tau in the locus coeruleus. Neural. Plast. 2017, 7829507. doi: 10.1155/2017/7829507
Chen, Q., Li, D. P., Pan, H. L. (2006). Presynaptic alpha1-adrenergic receptors differentially regulate synaptic glutamate and GABA release to hypothalamic presympathetic neurons. J. Pharmacol. Exp. Ther. 316, 733–742. doi: 10.1124/jpet.105.094797
Chen, C. R., Yang, S. R., Liu, Y. Y., Qu, W. M., Urade, Y., Huang, Z. L. (2013). Roles of adrenergic α1 and dopamine D1 and D2 receptors in the mediation of the desynchronization effects of modafinil in a mouse EEG synchronization model. PloS One 8 (10), e76102. doi: 10.1371/journal.pone.0076102
Choi, S. Y., Chang, J., Jiang, B., Seol, G.-H., Min, S.-S., Han, J.-S., et al. (2005). Multiple receptors coupled to phospholipase C gate long-term depression in visual cortex. J. Neurosci. 25 (49), 11433–11443. doi: 10.1523/JNEUROSCI.4084-05.2005
Christopoulos, A. (2002). Allosteric binding sites on cell-surface receptors: novel targets for drug discovery. Nat. Rev. Drug Discov. 1, 198–210.
Chrobak, J. J., Buzsaki, G. (1998). Gamma oscillations in the entorhinal cortex of the freely behaving rat. J. Neurosci. 18, 388–398. doi: 10.1523/JNEUROSCI.18-01-00388.1998
Cilz, N. I., Kurada, L., Hu, B., Lei, S. (2014). Dopaminergic modulation of GABAergic transmission in the entorhinal cortex: concerted roles of α1 adrenoreceptors, inward rectifier K+, and T-type Ca2+ channels. Cereb. Cortex. 24 (12), 3195–3208. doi: 10.1093/cercor/bht177
Ciombor, K. J., Ennis, M., Shipley, M. T. (1999). Norepinephrine increases rat mitral cell excitatory responses to weak olfactory nerve input via alpha1-receptors in vitro. Neuroscience. 90 (2), 595–606. doi: 10.1016/s0306-4522(98)00437-0
Colgin, L. L. (2015). Theta-gamma coupling in the entorhinal-hippocampal system. Curr. Opin. Neurobiol. 31, 45–50. doi: 10.1016/j.conb.2014.08.001
Colgin, L. L. (2016). Rhythms of the hippocampal network. Nat. Rev. Neurosci. 17, 239–249. doi: 10.1038/nrn.2016.21
Collette, K., Fagerlie, R., Haselton, J., Perez, D. M., Doze, V. (2010). Norepinephrine, through activation of α1A-ARs, stimulates production of new neurons, leading to an alleviation of depression and anxiety. FASEB. J. 24, 1058–1057. doi: 10.1096/fasebj.24.1_supplement.1058.7
Collette, K. M., Zhou, X. D., Amoth, H. M., Lyons, M. J., Papay, R. S., Sens, D. A., et al. (2014). Long-term α1B-adrenergic receptor activation shortens lifespan, while α1A-adrenergic receptor stimulation prolongs lifespan in association with decreased cancer incidence. Age. 36, 9675. doi: 10.1007/s11357-014-9675-7
Compton, D. M., Dietrich, K. L., Smith, J. S., Davis, B. K. (1995). Spatial and non-spatial learning in the rat following lesions to the nucleus locus coeruleus. NeuroReport. 7, 177–182. doi: 10.1097/00001756-199512000-00043
Cornil, C. A., Balthazart, J., Motte, P., Massotte, L., Seutin, V. (2002). Dopamine activates noradrenergic receptors in the preoptic area. J. Neurosci. 22, 9320–9330. doi: 10.1523/JNEUROSCI.22-21-09320.2002
Cotecchia, S., Schwinn, D. A., Randall, R. R., Lefkowitz, R. J., Caron, M. G., Kobilka, B. K. (1988). Molecular cloning and expression of the cDNA for the hamster alpha1-adrenergic receptor. Proc. Natl. Acad. Sci. U. S. A. 85, 7159–7163. doi: 10.1073/pnas.85.19.7159
Couey, J. J., Witoelar, A., Zhang, S. J., Zheng, K., Ye, J., Dunn, B., et al. (2013). Recurrent inhibitory circuitry as a mechanism for grid formation. Nat. Neurosci. 16, 318–324. doi: 10.1038/nn.3310
Covey, D. P., Roitman, M. F., Garris, P. A. (2014). Illicit dopamine transients: reconciling actions of abused drugs. Trends. Neurosci. 37, 200–210. doi: 10.1016/j.tins.2014.02.002
Crepel, F., Debono, M., Flores, R. (1987). a-Adrenergic inhibition of rat Cerebellar purkinje cells in vitro: A voltage-clamp study. J. Physiol. 383, 487–498. doi: 10.1113/jphysiol.1987.sp016423
Croce, A., Astier, H., Recasens, M., Vignes, M. (2003). Opposite effects of alpha1- and beta-adrenoceptor stimulation on both glutamate- and gamma-aminobutyric acid-mediated spontaneous transmission in cultured rat hippocampal neurons. J. Neurosci. Res. 71, 516–525. doi: 10.1002/jnr.10516
Cui, G., Okamoto, T., Morikawa, H. (2004). Spontaneous opening of T-type Ca2+ channels contributes to the irregular firing of dopamine neurons in neonatal rats. J. Neurosci. 24, 11079–11087. doi: 10.1523/JNEUROSCI.2713-04.2004
da Silva, E. D., Sato, M., Merlin, J., Broxton, N., Hutchinson, D. S., Ventura, S., et al. (2017). Factors influencing biased agonism in recombinant cells expressing the human α1A-adrenoceptor. Br. J. Pharmacol. 174 (14), 2318–2333. doi: 10.1111/bph.13837
Dahl, D., Sarvey, J. M. (1989). Norepinephrine induces pathway-specific long-lasting potentiation and depression in the hippocampal dentate gyrus. Proc. Natl. Acad. Sci. USA. 86, 4776–4780. doi: 10.1073/pnas.86.12.4776
de Kloet, E. R., Joëls, M., Holsboer, F. (2005). Stress and the brain: from adaptation to disease. Nat. Rev. Neurosci. 6, 463–475. doi: 10.1038/nrn1683
Dierks, M. R., Jordan, J. K., Sheehan, A. H. (2007). Prazosin treatment of nightmares related to posttraumatic stress disorder. Ann. Pharmacother. 41, 1013–1017. doi: 10.1345/aph.1H588
Ding, F., O’Donnell, J., Thrane, A. S., Zeppenfeld, D., Kang, H., Xie, L., et al. (2013). a1-Adrenergic receptors mediate coordinated Ca2+ signaling of cortical astrocytes in awake, behaving mice. Cell Calcium. 54 (6), 387–394. doi: 10.1016/j.ceca.2013.09.001
Do-Monte, F. H., Allensworth, M., Carobrez, A. P. (2010). Impairment of contextual conditioned fear extinction after microinjection of α-1-adrenergic blocker prazosin into the medial prefrontal cortex. Behav. Brain Res. 211, 89–95.
Dolcos, F., LaBar, K. S., Cabeza, R. (2005). Remembering one year later: role of the amygdala and the medial temporal lobe memory system in retrieving emotional memories. Proc. Natl. Acad. Sci. U. S. A. 102, 2626–2631. doi: 10.1073/pnas.0409848102
Domyancic, A. V., Morilak, D. A. (1997). Distribution of alpha1A adrenergic receptor mRNA in the rat brain visualized by in situ hybridization. J. Comp. Neurol. 386, 358–378. doi: 10.1002/(SICI)1096-9861(19970929)386:3<358::AID-CNE3>3.0.CO;2-0
Doze, V. A., Papay, R. S., Goldenstein, B. L., Gupta, M. K., Collette, K. M., Nelson, B. W., et al. (2011). Long-term α1A-adrenergic receptor stimulation improves synaptic plasticity, cognitive function, mood, and longevity. Mol. Pharmacol. 80, 747–758. doi: 10.1124/mol.111.073734
Dumont, E.C., Williams, J. T. (2004). Noradrenaline triggers GABAA inhibition of bed nucleus of the stria terminalis neurons projecting to the ventral tegmental area. J. Neurosci. 24 (38), 8198–8204.
Duteil, J., Rambert, F. A., Pessonnier, J., Hermant, J. F., Gombert, R., Assous, E. (1990). Central alpha 1-adrenergic stimulation in relation to the behaviour stimulating effect of modafinil; studies with experimental animals. Eur. J. Pharmacol. 180, 49–58. doi: 10.1016/0014-2999(90)90591-S
Dyer-Reaves, K., Goodman, A. M., Nelson, A. R., McMahon, L. L. (2019). Alpha1-Adrenergic Receptor Mediated Long-Term Depression at CA3-CA1 Synapses Can Be Induced via Accumulation of Endogenous Norepinephrine and Is Preserved Following Noradrenergic Denervation. Front. Synaptic. Neurosci. 11:27. doi: 10.3389/fnsyn.2019.00027
Eckmeier, D., Shea, S. D. (2014). Noradrenergic Plasticity of Olfactory Sensory Neuron Inputs to the Main Olfactory Bulb. J. Neurosci. 34 (46), 15234–15243. doi: 10.1523/JNEUROSCI.0551-14.2014
Enomoto, T., Tse, M. T., Floresco, S. B. (2011). Reducing prefrontal gamma-aminobutyric acid activity induces cognitive, behavioral, and dopaminergic abnormalities that resemble schizophrenia. Biol. Psychiatry 69, 432–441. doi: 10.1016/j.biopsych.2010.09.038
Erickson, J. C., Hollopeter, G., Thomas, S. A., Froelick, G. J., Palmiter, R. D. (1997). Disruption of the metallothionein-III gene in mice: analysis of brain zinc, behavior, and neuron vulnerability to metals, aging, and seizures. J. Neurosci. 17, 1271–1281. doi: 10.1523/JNEUROSCI.17-04-01271.1997
Evans, B. A., Broxton, N., Merlin, J., Sato, M., Hutchinson, D. S., Christopoulos, A., et al. (2011). Quantification of functional selectivity at the human α1A-adrenoceptor. Mol. Pharmacol. 79 (2), 298–307. doi: 10.1124/mol.110.067454
Ferry, B., McGaugh, J. L. (1999). Clenbuterol administration into the basolateral amygdala post training enhances retention in an inhibitory avoidance task. Neurobiol. Learn Mem. 72, 8–12. doi: 10.1006/nlme.1998.3904
Ferry, B., McGaugh, J. L. (2000). Role of amygdala norepinephrine in mediating stress hormone regulation of memory storage. Acta Pharmacol. Sin. 21 (6), 481–493. doi: CN 31-1347/R ISSN 1671-4083 EISSN 1745-7254
Ferry, B., Quirarte, G. (2012). “Role of norepinephrine in mediating inhibitory avoidance memory storage: a critical involvement of the basolateral amygdala,” in The Amygdala: A Discrete Multitasking Manager, 980-953-307-188-1. Ed. Ferry, B. (London, United Kingdom: InTech – Open Science/Open Mind – Press), 203–230.
Ferry, B., Roozendaal, B., McGaugh, J. L. (1999a). Involvement of α1-adrenergic receptors in the basolateral amygdala in modulation of memory storage. Eur. J. Pharmacol. 372 (1), 9–16. doi: 10.1016/S0014-2999(99)00169-7
Ferry, B., Roozendaal, B., McGaugh, J. L. (1999b). Basolateral amygdala noradrenergic influences on memory storage are mediated by an interaction between beta- and alpha1-adrenoceptors. J. Neurosci. 19 (12), 5119–5123. doi: 10.1523/JNEUROSCI.19-12-05119.1999
Fisher, S. A., Fischer, T. M., Carew, T. J. (1997). Multiple overlapping processes underlying short-term synaptic enhancement. Trends Neurosci. 20, 170–177. doi: 10.1016/S0166-2236(96)01001-6
Floresco, S. B., West, A. R., Ash, B., Moore, H., Grace, A. A. (2003). Afferent modulation of dopamine neuron firing differentially regulates tonic and phasic dopamine transmission. Nat. Neurosci. 6, 968–973. doi: 10.1038/nn1103
Forget, B., Hamon, M., Thiébot, M. (2009). Involvement of α1-adrenoceptors in conditioned place preference supported by nicotine in rats. Psychopharmacology. 205, 503–515. doi: 10.1007/s00213-009-1559-7
Forno, L. S. (1966). Pathology of parkinsonism-A preliminary report of 24 cases. J. Neurosurg. 24, 266–271. doi: 10.1016/B978-0-407-02295-9.50008-4
Foster, T. C., McNaughton, B. L. (1991). Long-term synaptic enhancement in CA1 is due to increased quanta1 size, not quanta1 content. Hippocampus. 1, 79–91. doi: 10.1002/hipo.450010108
Gazarini, L., Stern, C. A., Carobrez, A. P., Bertoglio, L. J. (2013). Enhanced noradrenergic activity potentiates fear memory consolidation and reconsolidation by differentially recruiting α1- and β-adrenergic receptors. Learn. Mem. 20 (4), 210–219. doi: 10.1101/lm.030007.112
Gibbs, M. E., Bowser, D. N. (2010). Astrocytic adrenoceptors and learning: alpha1-adrenoceptors. Neurochem. Int. 57 (4), 404–410. doi: 10.1016/j.neuint.2010.03.020
Gibbs, M. E., Summers, R. J. (2001). Stimulation of α1-adrenoceptors inhibits memory consolidation in the chick. Eur. J. Neurosci. 14, 1369–1376. doi: 10.1046/j.0953-816x.2001.01742.x
Gibbs, M. E., Hutchinson, D., Hertz, L. (2008). Astrocytic involvement in learning and memory consolidation. Neurosci. Biobehav. Rev. 32 (5), 927–944. doi: 10.1016/j.neubiorev.2008.02.001
Goertz, R. B., Wanat, M. J., Gomez, J. A., Brown, Z. J., Phillips, P. E., Paladini, C. A. (2015). Cocaine increases dopaminergic neuron and motor activity via midbrain α1 adrenergic signaling. Neuropsychopharmacology 40 (5), 1151–1162. doi: 10.1038/npp.2014.296
Gordon, G. R., Bains, J. S. (2003). Priming of excitatory synapses by alpha1 adrenoceptor-mediated inhibition of group III metabotropic glutamate receptors. J. Neurosci. 23, 6223–6231. doi: 10.1523/JNEUROSCI.23-15-06223.2003
Gordon, G. R., Iremonger, K. J., Kantevari, S., Ellis-Davies, G. C., MacVicar, B. A., Bains, J. S. (2009). Astrocyte-mediated distributed plasticity at hypothalamic glutamate synapses. Neuron 64 (3), 391–403. doi: 10.1016/j.neuron.2009.10.021
Greenwell, T. N., Walker, B. M., Cottone, P., Zorrilla, E. P., Koob, G. F. (2009). The alpha1 adrenergic receptor antagonist prazosin reduces heroin self-administration in rats with extended access to heroin administration. Pharmaco. Biochem. Behav. 91, 295–302. doi: 10.1016/j.pbb.2008.07.012
Grenhoff, J., Svensson, T. H. (1993). Prazosin modulates the firing pattern of dopamine neurons in rat ventral tegmental area. Eur. J. Pharmacol. 233, 79–84. doi: 10.1016/0014-2999(93)90351-H
Grenhoff, J., Nisell, M., Ferre, S., Aston-Jones, G., Svensson, T. H. (1993). Noradrenergic modulation of midbrain dopamine cell firing elicited by stimulation of the locus coeruleus in the rat. J. Neural. Transm. Gen. Sect. 93, 11–25. doi: 10.1007/BF01244934
Grenhoff, J., North, R. A., Johnson, S. W. (1995). Alpha1-adrenergic effects on dopamine neurons recorded intracellularly in the rat midbrain slice. Eur. J. Neurosci. 7, 1707–1713. doi: 10.1111/j.1460-9568.1995.tb00692.x
Grudzien, A., Shaw, P., Weintraub, S., Bigio, E., Mash, D. C., Mesulam, M. M. (2007). Locus coeruleus neurofibrillary degeneration in aging, mild cognitive impairment and early Alzheimer’s disease. Neurobiol. Aging. 28, 327–335. doi: 10.1016/j.neurobiolaging.2006.02.007
Guérin, D., Peace, S. T., Didier, A., Linster, C., Cleland, T. A. (2008). Noradrenergic neuromodulation in the olfactory bulb modulates odor habituation and spontaneous discrimination. Behav. Neurosci. 122, 816–826. doi: 10.1037/a0012522
Guerra-Gomes, S., Sousa, N., Pinto, L., Oliveira, J. F. (2018). Functional Roles of Astrocyte Calcium Elevations: From Synapses to Behavior. Front. Cell. Neurosci. 11, 427. doi: 10.3389/fncel.2017.00427
Gupta, M. K., Papay, R. S., Jurgens, C. W., Gaivin, R. J., Shi, T., Doze, V. A., et al. (2009). Alpha1-Adrenergic receptors regulate neurogenesis and gliogenesis. Mol. Pharmacol. 76 (2), 314–326. doi: 10.1124/mol.109.057307
Haack, K. K. V., McCarty, N. A. (2011). Functional Consequences of GPCR Heterodimerization: GPCRs as Allosteric Modulators. Pharmaceut. (Basel). 4 (3), 509–523. doi: 10.3390/ph4030509
Haist, F., Gore, J. B., Mao, H. (2001). Consolidation of human memory over decades revealed by functional magnetic resonance imaging. Nat. Neurosci. 4, 1139–1145. doi: 10.1038/nn739
Haj-Dahmane, S., Shen, R. Y. (2014). Chronic stress impairs α1-adrenoceptor-induced endocannabinoid-dependent synaptic plasticity in the dorsal raphe nucleus. J. Neurosci. 34 (44), 14560–14570. doi: 10.1523/JNEUROSCI.1310-14.2014
Hansen, N., Manahan-Vaughan, D. (2015). Hippocampal long-term potentiation that is elicited by perforant path stimulation or that occurs in conjunction with spatial learning is tightly controlled by beta-adrenoreceptors and the locus coeruleus. Hippocampus 25 (11), 1285–1298. doi: 10.1002/hipo.22436
Harley, C. W., Sara, S. J. (1992). Locus coeruleus bursts induced by glutamate trigger delayed perforant path spike amplitude potentiation in the dentate gyrus. Exp. Brain Res. 89, 581–587. doi: 10.1007/BF00229883
Harley, C. W. (1991). Noradrenergic and locus coeruleus modulation of the perforant path-evoked potential in rat dentate gyrus supports a role for the locus coeruleus in attentional and memorial processes. Prog. Brain. Res. 88, 307–321. doi: 10.1016/S0079-6123(08)63818-2
Harley, C. W. (2007). Norepinephrine and the dentate gyrus. Prog. Brain. Res. 163, 299–318. doi: 10.1016/S0079-6123(07)63018-0
Hartner, J. P., Schrader, L. A. (2018). Interaction of Norepinephrine and Glucocorticoids Modulate Inhibition of Principle Cells of Layer II Medial Entorhinal Cortex in Male Mice. Front. Synaptic. Neurosci. 10, 3. doi: 10.3389/fnsyn.2018.00003
Harvey, J. D., Heinbockel, T. (2018). Neuromodulation of Synaptic Transmission in the Main Olfactory Bulb. Int. J. Environ. Res. Public Health 15 (10), 2194. doi: 10.3390/ijerph15102194
Hassanpoor, H., Fallah, A., Raza, M. (2014). Mechanisms of hippocampal astrocytes mediation of spatial memory and theta rhythm by gliotransmitters and growth factors. Cell Biol. Int. 38, 1355–1366. doi: 10.1002/cbin.10326
Hayar, A., Heyward, P. M., Heinbockel, T., Shipley, M. T., Ennis, M. (2001). Direct excitation of mitral cells via activation of alpha1-noradrenergic receptors in rat olfactory bulb slices. J. Neurophysiol. 86 (5), 2173–2182. doi: 10.1152/jn.2001.86.5.2173
Heagerty, M. A., Ollerenshaw, J. D., Swales, J. D. (1986). Abnormal vascular phosphoinositide hydrolysis in the spontaneous hypertensive rat. Br. J. Pharmacol. 89, 803–807. doi: 10.1111/j.1476-5381.1986.tb11185.x
Hempel, P., Heinig, B., Jerosch, C., Decius, I., Karczewski, P., Kassner, U., et al. (2016). Immunoadsorption of Agonistic Autoantibodies Against α1-Adrenergic Receptors in Patients with Mild to Moderate Dementia. Ther. Apher. Dial. 20 (5), 523–529. doi: 10.1111/1744-9987.12415
Herold, S., Hecker, C., Deitmer, J. W., Brockhaus, J. (2005). Alpha1-Adrenergic modulation of synaptic input to Purkinje neurons in rat cerebellar brain slices. J. Neurosci. Res. 82, 571–579. doi: 10.1002/jnr.20660
Hertz, L., Lovatt, D., Goldman, S. A., Nedergaard, M. (2010). Adrenoceptors in brain: cellular gene expression and effects on astrocytic metabolism and [Ca(2+)]i. Neurochem. Int. 57 (4), 411–420. doi: 10.1016/j.neuint.2010.03.019
Heynen, A. J., Abraham, W. C., Bear, M. F. (1996). Bidirectional modification of CA1 synapses in the adult hippocampus in vivo. Nature. 381 (6578), 163–166. doi: 10.1038/381163a0
Hieble, J. P., DeMarinis, R. M., Matthews, W. D. (1986). Evidence for and against heterogeneity of α1-adrenoceptors Life. Sci. 38, 1339–1350. doi: 10.1016/0024-3205(86)90466-2
Hieble, J. P., Bylund, D. B., Clarke, D. E., Eikenburg, D. C., Langer, S. Z., Lefkowitz, R. J., et al. (1995). International Union of Pharmacology. X. Recommendation for nomenclature of alpha1-adrenoceptors: consensus update. Pharmacol. Rev. 47, 267–270.
Hilfiker, S., Augustine, G. J. (1999). Regulation of synaptic vesicle fusion by protein kinase C. J. Physiol. 515 ( Pt 1), 1. doi: 10.1111/j.1469-7793.1999.001ad.x
Hillman, K. L., Knudson, C. A., Carr, P. A., Doze, V. A., Porter, J. E. (2005). Adrenergic receptor characterization of CA1 hippocampal neurons using real time single cell RT-PCR. Brain. Res. Mol. Brain. Res. 139, 267–276. doi: 10.1016/j.molbrainres.2005.05.033
Hillman, K. L., Doze, V. A., Porter, J. E. (2007). Alpha1A-adrenergic receptors are functionally expressed by a subpopulation of cornu ammonis-1 interneurons in rat hippocampus. J. Pharmacol. Exp. Ther. 321, 1062–1068. doi: 10.1124/jpet.106.119297
Hillman, K. L., Lei, S., Doze, V. A., Porte,r, J. E. (2009). Alpha1A-adrenergic receptor activation increases inhibitory tone in CA1 hippocampus. Epilepsy. Res. 84, 97–109. doi: 10.1016/j.eplepsyres.2008.12.007
Hirono, M., Obata, K. (2006). Alpha-adrenoceptive dual modulation of inhibitory GABAergic inputs to Purkinje cells in the mouse cerebellum. J. Neurophysiol. 95, 700–708. doi: 10.1152/jn.00711.2005
Hong, C. J., Wang, Y. C., Liu, T. Y., Liu, H. C., Tsai, S. J. (2001). A study of α-adrenoceptor gene polymorphisms and Alzheimer disease. J. Neural. Transm. 108 (4), 445–450. doi: 10.1007/s007020170065
Hopkins, W. F., Johnston, D. (1984). Frequency-dependent noradrenergic modulation of long-term potentiation in the hippocampus. Science. 226, 350–352. doi: 10.1126/science.6091272
Huang, M., Daly, J. (1972). Accumulation of cyclic adenosine monophosphate in incubated slices of brain tissue. Structure-activity relationship of agonists of biogenic amines and tricyclic tranquilizers and antidepressants. J. Med. Chem. 15, 458–462. doi: 10.1021/jm00275a004
Huang, Y.-Y., Kandel, E. R. (1996). Modulation of both the early and the late phase of mossy fiber LTP by the activation of β-adrenergic receptors. Neuron. 16, 611–617. doi: 10.1016/S0896-6273(00)80080-X
Huang, Y. Y., Nguyen, P. V., Abel, T., Kandel, E. R. (1996). Long-lasting forms of synaptic potentiation in the mammalian hippocampus. Learn. Mem. 3, 74–85. doi: 10.1101/lm.3.2-3.74
Huzoor-Akbar, N., Chen, Y., Fossen, D. V., Wallace, D. (1989). Increased vascular contractile sensitivity to serotonin in spontaneously hypertensive rats is linked with increased turnover of phosphoinositides. Life. Sci. 45, 577–583. doi: 10.1016/0024-3205(89)90042-8
Hvoslef-Eide, M., Oomen, C. A., Fisher, B. M., Heath, C. J., Robbins, T. W., Saksida, L. M., et al. (2015). Facilitation of spatial working memory performance following intra-prefrontal cortical administration of the adrenergic alpha1 agonist phenylephrine. Psychopharmacol. (Berl). 232 (21-22), 4005–4016. doi: 10.1007/s00213-015-4038-3
Hyman, B. T., Van Hoesen, G. W., Damasio, A. R., Barnes, C. L. (1984). Alzheimer’s disease: cell-specific pathology isolates the hippocampal formation. Science. 225, 1168–1170. doi: 10.1126/science.6474172
Ikegaya, Y., Nakanishi, K., Saito, H., Abe, K. (1997). Amygdala beta-noradrenergic influence on hippocampal long-term potentiation in vivo. Neuroreport. 8, 3143–3146. doi: 10.1097/00001756-199709290-00027
Introini-Collison, I. B., Miyazaki, B., McGaugh, J. L. (1991). Involvement of the amygdala in the memory-enhancing effects of clenbuterol. Psychopharmacology. 104, 541–544. doi: 10.1007/BF02245663
Ishac, E. J. N., Lazar-Wesley, E., Kunos, G. (1992). Rapid inverse changes in α1B- and β2-adrenergic receptors and gene transcripts in acutely isolated rat liver cells. J. Cell Physiol. 152, 79–86. doi: 10.1002/jcp.1041520111
Iversen, L. L., Iversen, S. D., Bloom, F. E., Roth, R. H. (2009). Introduction to Neuropsychopharmacology (New York: Oxford University Press. Catecholamines), 150–213.
Izumi, Y., Zorumski, C. F. (1999). Norepinephrine promotes long-term potentiation in the adult rat hippocampus in vitro. Synapse 31 (3), 196–202. doi: 10.1002/(SICI)1098-2396(19990301)31:3<196::AID-SYN4>3.0.CO;2-K
Jackman, S. L., Regehr, W. G. (2017). The Mechanisms and Functions of Synaptic Facilitation. Neuron. 94 (3), 447–464. doi: 10.1016/j.neuron.2017.02.047
Jensen, B. C., Swigart, P. M., Simpson, P. C. (2009). Ten commercial antibodies for alpha1-adrenergic receptor subtypes are nonspecific. Naunyn. Schmiedebergs. Arch. Pharmacol. 379, 409–412. doi: 10.1007/s00210-008-0368-6
Jhou, T. C., Fields, H. L., Baxter, M. G., Saper, C. B., Holland, P. C. (2009a). The rostromedial tegmental nucleus (RMTg), a GABAergic afferent to midbrain dopamine neurons, encodes aversive stimuli and inhibits motor responses. Neuron. 61, 786–800. doi: 10.1016/j.neuron.2009.02.001
Jhou, T. C., Geisler, S., Marinelli, M., Degarmo, B. A., Zahm, D. S. (2009b). The mesopontine rostromedial tegmental nucleus: A structure targeted by the lateral habenula that projects to the ventral tegmental area of Tsai and substantia nigra compacta. J. Comp. Neurol. 513, 566–596. doi: 10.1002/cne.21891
Jiang, M., Griff, E. R., Ennis, M., Zimmer, L. A., Shipley, M. T. (1996). Activation of locus coeruleus enhances the responses of olfactory bulb mitral cells to weak olfactory nerve input. J. Neurosci. 16, 6319–6329. doi: 10.1523/JNEUROSCI.16-19-06319.1996
Jimenez-Rivera, C. A., Feliu-Mojer, M., Vazquez-Torres, R. (2006). Alpha-noradrenergic receptors modulate the development and expression of cocaine sensitization. Ann. NY. Acad. Sci. 1074, 390–402. doi: 10.1196/annals.1369.039
Joëls, M., Baram, T. Z. (2009). The neuro-symphony of stress. Nat. Rev. Neurosci. 10, 459–466. doi: 10.1038/nrn2632
Jones, L. S., Gauger, L. L., Davis, J. N., Slotkin, T. A., Bartolome, J. V. (1985). Postnatal development of brain alpha1-adrenergic receptors: in vitro autoradiography with [125I-]HEAT in normal rats and rats treated with alpha-difluoromethylornithine, a specific, irreversible inhibitor of ornithine decarboxylase. Neuroscience 15 (4), 1195–1202. doi: 10.1016/0306-4522(85)90262-3
Jucker, M., Walker, L. C. (2011). Pathogenic protein seeding in Alzheimer disease and other neurodegenerative disorders. Ann. Neurol. 70, 532–540. doi: 10.1002/ana.22615
Jurgens, C. W. D., Knudson, C. A., Carr, P. A., Perez, D. M., Doze, V. A. (2009). α1-Adrenergic receptor regulation of interneuron function. FASEB J. 23, 946.4. doi: 10.1096/fasebj.23.1_supplement.946.4
Kajiyama, Y., Ui, M. (1994). Switching from alpha1- to beta-subtypes in adrenergic response during primary culture of adult-rat hepatocytes as affected by the cell-to-cell interaction through plasma membranes. Biochem. J. 303, 313–321. doi: 10.1042/bj3030313
Karczewski, P., Hempel, P., Kunze, R., Bimmler, M. (2012a). Agonistic Autoantibodies to the α1-Adrenergic Receptor and the β2-Adrenergic Receptor in Alzheimer’s and Vascular Dementia. Scand. J. Immunol. 75, 524–530. doi: 10.1111/j.1365-3083.2012.02684.x
Karczewski, P., Pohlmann, A., Wagenhaus, B., Wisbrun, N., Hempel, P., Lemke, B., et al. (2012b). Antibodies to the alpha1-adrenergic receptor cause vascular impairments in rat brain as demonstrated by magnetic resonance angiography. PloS One 7, e41602. doi: 10.1371/journal.pone.0041602
Karczewski, P., Hempel, P., Bimmler, M. (2018). Role of alpha1-adrenergic receptor antibodies in Alzheimer’s disease. Front. Biosci (Landmark Ed). 23, 2082–2089. doi: 10.2741/4691
Katsuki, H., Izumi, Y., Zorumski, C. F. (1997). Noradrenergic regulation of synaptic plasticity in the hippocampal CA1 region. J. Neurophysiol. 77, 3013–3020. doi: 10.1152/jn.1997.77.6.3013
Kawaguchi, Y., Shindou, T. (1998). Noradrenergic excitation and inhibition of GABAergic cell types in rat frontal cortex. J. Neurosci. 18, 6963–6976. doi: 10.1523/JNEUROSCI.18-17-06963.1998
Keiflin, R., Janak, P. H. (2015). Dopamine prediction errors in reward learning and addiction: from theory to neural circuitry. Neuron. 88, 247–263. doi: 10.1016/j.neuron.2015.08.037
Kelly, S. C., He, B., Perez, S. E., Ginsberg, S. D., Mufson, E. J., Counts, S. E. (2017). Locus coeruleus cellular and molecular pathology during the progression of Alzheimer’s disease. Acta Neuropathol. Commun. 5, 8. doi: 10.1186/s40478-017-0411-2
Kemp, A., Manahan-Vaughan, D. (2004). Hippocampal long-term depression and long-term potentiation encode different aspects of novelty acquisition. Proc. Natl. Acad. Sci. U. S. A. 101, 8192–8197. doi: 10.1073/pnas.0402650101
Kendrick, K. M., Lévy, F., Keverne, E. B. (1991). Importance of vaginocervical stimulation for the formation of maternal bonding in primiparous and multiparous parturient ewes. Physiol. Behav. 50, 595–600. doi: 10.1016/0031-9384(91)90551-X
Khorchid, A., Cui, Q., Molina-Holgado, E., Almazan, G. (2002). Developmental regulation of alpha1A-adrenoceptor function in rat brain oligodendrocyte cultures. Neuropharmacology. 42 (5), 685–696. doi: 10.1016/S0028-3908(02)00013-8
Kirkwood, A., Rozas, C., Kirkwood, J., Perez, F., Bear, M. F. (1999). Modulation of long-term synaptic depression in visual cortex by acetylcholine and norepinephrine. J. Neurosci. 19, 1599–1609. doi: 10.1523/JNEUROSCI.19-05-01599.1999
Knauber, J., Müller, W. E. (2000a). Subchronic treatment with prazosin improves passive avoidance learning in aged mice: possible relationships to alpha1-receptor up-regulation. J. Neural. Transm. (Vienna). 107 (12), 1413–1426. doi: 10.1007/s007020070005
Knauber, J., Müller, W. E. (2000b). Decreased exploratory activity and impaired passive avoidance behaviour in mice deficient for the alpha(1b)-adrenoceptor. Eur. Neuropsychopharmacol. 10 (6), 423–427. doi: 10.1016/S0924-977X(00)00100-0
Knepper, S. M., Buckner, S. A., Brune, M. E., DeBernardis, J. F., Meyer, M. D., Hancock, A. A. (1995). A-61603, a potent α1-adrenergic receptor agonist, selective for the α1A receptor subtype. J. Pharmacol. Exp. Ther. 274 (1), 97–10.
Kobayashi, M., Sasabe, T., Shiohama, Y., Koshikawa, N. (2008). Activation of alpha1-adrenoceptors increases firing frequency through protein kinase C in pyramidal neurons of rat visual cortex. Neurosci. Lett. 430, 175–180. doi: 10.1016/j.neulet.2007.10.047
Kobayashi, M., Kojima, M., Koyanagi, Y., Adachi, K., Imamura, K., Koshikawa, N. (2009). Presynaptic and postsynaptic modulation of glutamatergic synaptic transmission by activation of alpha(1)- and beta-adrenoceptors in layer V pyramidal neurons of rat cerebral cortex. Synapse. 63 (4), 269–281. doi: 10.1002/syn.20604
Kotzbauer, P. T., Trojanowsk, J. Q., Lee, V. M. (2001). Lewy body pathology in Alzheimer’s disease. J. Mol. Neurosci. 17, 225–232. doi: 10.1385/JMN:17:2:225
Krugers, H. J., Karst, H., Joels, M. (2012). Interactions between noradrenaline and corticosteroids in the brain: from electrical activity to cognitive performance. Front. Cell. Neurosci. 6, 15. doi: 10.3389/fncel.2012.00015
Kulik, A., Haentzsch, A., Lückermann, M., Reichelt, W., Ballanyi, K. (1999). Neuron-glia signaling via alpha(1) adrenoceptor-mediated Ca(2+) release in Bergmann glial cells in situ. J. Neurosci. 19, 8401–8408. doi: 10.1523/JNEUROSCI.19-19-08401.1999
LaLumiere, R. T., McGaugh, J. L., McIntyre, C. K. (2017). Emotional Modulation of Learning and Memory: Pharmacological Implications. Pharmacol. Rev. 69 (3), 236–255. doi: 10.1124/pr.116.013474
Landfield, P. W., Lynch, G. (1977). Impaired monosynaptic potentiation in in vitro hippocampal slices from aged, memory-deficient rats. J. Geronto.l 32, 523–533. doi: 10.1093/geronj/32.5.523
Lapiz, M. D., Morilak, D. A. (2006). Noradrenergic modulation of cognitive function in rat medial prefrontal cortex as measured by attentional set shifting capability. Neuroscience. 137, 1039–1049. doi: 10.1016/j.neuroscience.2005.09.031
Laz, T. M., Forray, C., Smith, K. E., Bard, J. A., Vaysse, P. J., Branchek, T. A., et al. (1994). The rat homologue of the bovine alpha1c-adrenergic receptor shows the pharmacological properties of the classical alpha1A subtype. Mol. Pharmacol. 46, 414–422.
Lazou, A., Markou, T., Zioga, M., Vasara, E., Efstathiou, A., Gaitanak,i, C. (2006). Dopamine mimics the cardioprotective effect of ischemic preconditioning via activation of alpha1-adrenoceptors in the isolated rat heart. Physiol. Res. 55, 1–8.
Lazzaro, S. C., Hou, M., Cunha, C., LeDoux, J. E., Cain, C. K. (2010). Antagonism of lateral amygdala alpha1-adrenergic receptors facilitates fear conditioning and long-term potentiation. Learn. Mem. 17 (10), 489–493. doi: 10.1101/lm.1918210
LeDoux, J. E. (2000). Emotion circuits in the brain. Annu. Rev. Neurosci. 23, 155–184. doi: 10.1146/annurev.neuro.23.1.155
Leedham, J. A., Pennefather, J. N. (1986). Selectivities of some agonists acting at alpha1- and alpha2-adrenoreceptors in the rat vas deferens. J. Auton. Pharmacol. 6, 39–46. doi: 10.1111/j.1474-8673.1986.tb00629.x
Lei, S., Deng, P. Y., Porter, J. E., Shin, H. S. (2007). Adrenergic facilitation of GABAergic transmission in rat entorhinal cortex. J. Neurophysiol. 98, 2868–2877. doi: 10.1152/jn.00679.2007
Leppik, R. A., Mynett, A., Lazareno, S., Birdsall, N. J. (2000). Allosteric interactions between the antagonist prazosin and amiloride analogs at the human α1A-adrenergic receptor. Mo.l Pharmacol. 57, 436–445. doi: 10.1124/mol.57.3.436
Lewis, D. A., Morrison, J. H. (1989). Noradrenergic innervation of monkey prefrontal cortex: a dopamine-beta-hydroxylase immunohistochemical study. J. Comp. Neurol. 282, 317–330. doi: 10.1002/cne.902820302
Liang, K. C., Juler, R., McGaugh, J. L. (1986). Modulating effects of post training epinephrine on memory: involvement of the amygdala noradrenergic system. Brain Res. 368, 125–133. doi: 10.1016/0006-8993(86)91049-8
Liang, K. C., McGaugh, J. L., Yao, H.-Y. (1990). Involvement of amygdala pathways in the influence of post-training intra-amygdala norepinephrine and peripheral epinephrine on memory storage. Brain Res. 508, 225–233. doi: 10.1016/0006-8993(90)90400-6
Lin, Y., Quartermain, D., Dunn, A. J., Weinshenker, D., Stone, E. A. (2008). Possible dopaminergic stimulation of locus coeruleus alpha1-adrenoceptors involved in behavioral activation. Synapse. 62, 516–523. doi: 10.1002/syn.20517
Lindén, A., Rabe, K. F., Löfdahl, C. G. (1996). Pharmacological basis for duration of effect: formoterol and salmeterol versus short-acting beta 2-adrenoceptor agonists. Lung. 174 (1), 1–22. doi: 10.1007/BF00167947
Liu, X., Masoudi, A., Kahsai, A. W., Huang, L. Y., Pani, B., Staus, D. P., et al. (2019). Mechanism of β2AR regulation by an intracellular positive allosteric modulator. Science 364 (6447), 1283–1287. doi: 10.1126/science.aaw8981
Lobb, C. J., Wilson, C. J., Paladini, C. A. (2010). A dynamic role for GABA receptors on the firing pattern of midbrain dopaminergic neurons. J. Neurophysiol. 104, 403–413. doi: 10.1152/jn.00204.2010
Lohani, S., Martig, A. K., Underhill, S. M., DeFrancesco, A., Roberts, M. J., Rinaman, L., et al. (2018). Burst activation of dopamine neurons produces prolonged post-burst availability of actively released dopamine. Neuropsychopharmacology 43 (10), 2083–2092. doi: 10.1038/s41386-018-0088-7
Lucas, E. K., Wu, W. C., Roman-Ortiz, C., Clem, R. L. (2019). Prazosin during fear conditioning facilitates subsequent extinction in male C57Bl/6N mice. Psychopharmacol. (Berl). 236 (1), 273–279. doi: 10.1007/s00213-018-5001-x
Lukitsch, I., Kehr, J., Chaykovska, L., Wallukat, G., Nieminen-Kelhä, M., Batuman, V., et al. (2012). Renal ischemia and transplantation predics pose to vascular constriction mediated by angiotensin II type 1 receptor-activating antibodies. Transplantation. 94, 8–13. doi: 10.1097/TP.0b013e3182529bb7
Luo, F., Tang, H., Li, B. M., Li, S. H. (2014). Activation of alpha1-adrenoceptors enhances excitatory synaptic transmission via a pre- and postsynaptic protein kinase C-dependent mechanism in the medial prefrontal cortex of rats. Eur. J. Neurosci. 39, 1281–1293. doi: 10.1111/ejn.12495
Luo, F., Tang, H., Cheng, Z. Y. (2015a). Stimulation of α1-adrenoceptors facilitates GABAergic transmission onto pyramidal neurons in the medial prefrontal cortex. Neuroscience 300, 63–74. doi: 10.1016/j.neuroscience.2015.04.070
Luo, F., Li, S. H., Tang, H., Deng, W. K., Zhang, Y., Liu, Y. (2015b). Phenylephrine enhances glutamate release in the medial prefrontal cortex through interaction with N-type Ca2+ channels and release machinery. J. Neurochem. 132 (1), 38–50. doi: 10.1111/jnc.12941
Lv, J., Zhan, S. Y., Li, G. X., Wang, D., Li, Y. S., Jin, Q. H. (2016). α1-Adrenoceptors in the hippocampal dentate gyrus involved in learning-dependent long-term potentiation during active-avoidance learning in rats. Neuroreport. 27 (16), 1211–1216. doi: 10.1097/WNR.0000000000000679
Mahmoodi, G., Ahmadi, S., Pourmotabbed, A., Oryan, S., Zarrindast, M. R. (2010). Inhibitory avoidance memory deficit induced by scopolamine: Interaction of cholinergic and glutamatergic systems in the ventral tegmental area. Neurobiol. Learn. Mem. 94 (1), 83–90. doi: 10.1016/j.nlm.2010.04.004
Maity, S., Rah, S., Sonenberg, N., Gkogkas, C. G., Nguyen, P. V. (2015). Norepinephrine triggers metaplasticity of LTP by increasing translation of specific mRNAs. Learn. Memory 22 (10), 499–508. doi: 10.1101/lm.039222.115
Maity, S., Chandanathil, M., Millis, R. M., Connor, S. A. (2020). Norepinephrine stabilizes translation-dependent, homosynaptic long-term potentiation through mechanisms requiring the cAMP sensor Epac, mTOR and MAPK. Eur. J. Neurosci. 00, 1–10. doi: 10.1111/ejn.14735
Manahan-Vaughan, D., Braunewell, K. H. (1999). Novelty acquisition is associated with induction of hippocampal long-term depression. Proc. Natl. Acad. Sci. U. S. A. 96, 8739–8744. doi: 10.1073/pnas.96.15.8739
Mandairon, N., Peace, S., Karnow, A., Kim, J., Ennis, M., Linster, C. (2008). Noradrenergic modulation in the olfactory bulb influences spontaneous and reward-motivated discrimination, but not the formation of habituation memory. Eur. J. Neurosci. 27, 1210–1219. doi: 10.1111/j.1460-9568.2008.06101.x
Mao, Z. M., Arnsten, A. F., Li, B. M. (1999). Local infusion of an α1-adrenergic agonist into the prefrontal cortex impairs spatial working memory performance in monkeys. Biol. Psychiatry 46, 1259–1265. doi: 10.1016/S0006-3223(99)00139-0
Marek, G. J., Aghajanian, G. K. (1996). Alpha1B-adrenoceptor-mediated excitation of piriform cortical interneurons. Eur. J. Pharmacol. 305, 95–100. doi: 10.1016/0014-2999(96)00158-6
Marek, G. J., Aghajanian, G. K. (1999). 5-HT2A receptor or α1-adrenoceptor activation induces excitatory postsynaptic currents in layer V pyramidal cells of the medial prefrontal cortex. Eur. J. Pharmacol. 367, 197–206. doi: 10.1016/S0014-2999(98)00945-5
Marzo, A., Bai, J., Caboche, J., Vanhoutte, P., Otani, S. (2010). Cellular mechanisms of long-term depression induced by noradrenaline in rat prefrontal neurons. Neuroscience 169 (1), 74–86. doi: 10.1016/j.neuroscience.2010.04.046
McCormick, D. A., Pape, H. C., Williamson, A. (1991). Actions of norepinephrine in the cerebral cortex and thalamus: implications for function of the central noradrenergic system. Prog. Brain. Res. 88, 293–305. doi: 10.1016/S0079-6123(08)63817-0
McCune, S. K., Voigt, M. M., Hill, J. M. (1993). Expression of multiple alpha adrenergic receptor subtype messenger RNAs in the adult rat brain. Neuroscience. 57, 143–151. doi: 10.1016/0306-4522(93)90116-W
McElligott, Z. A., Winder, D. G. (2008). Alpha1-adrenergic receptor-induced heterosynaptic long-term depression in the bed nucleus of the stria terminalis is disrupted in mouse models of affective disorders. Neuropsychopharmacology 33 (10), 2313–2323. doi: 10.1038/sj.npp.1301635
McElligott, Z. A., Klug, J. R., Nobis, W. P., Patel, S., Grueter, B. A., Kash, T. L., et al. (2010). Distinct forms of Gq-receptor-dependent plasticity of excitatory transmission in the BNST are differentially affected by stress. Proc. Natl. Acad. Sci. U. S. A. 107 (5), 2271–2276. doi: 10.1073/pnas.0905568107
McLean, J. H., Shipley, M. T., Nickell, W. T., Aston-Jones, G., Reyher, C. K. (1989). Chemoanatomical organization of the noradrenergic input from locus coeruleus to the olfactory bulb of the adult rat. J. Comp. Neurol. 285 (3), 339–349. doi: 10.1002/cne.902850305
Mijares, A., Lebesgue, D., Wallukat, G., Hoebeke, J. (2000). From agonist to antagonist: Fab fragments of an agonist-like monoclonal anti-β2-adrenoceptor antibody behave as antagonists. Mol. Pharmacol. 58, 373–379. doi: 10.1124/mol.58.2.373
Miller, L. J. (2008). Prazosin for the treatment of posttraumatic stress disorder sleep disturbances. Pharmacotherapy 28, 656–666. doi: 10.1592/phco.28.5.656
Ming, G., Song, H. (2005). Adult neurogenesis in the mammalian central nervous system. Annu. Rev. Neurosci. 28, 223–250. doi: 10.1146/annurev.neuro.28.051804.101459
Minneman, K. P., Theroux, T. L., Hollinger, S., Han, C., Esbenshade, T. A. (1994). Selectivity of agonists for cloned α1-adrenergic receptor subtypes. Mol. Pharmacol. 46 (5), 929–936.
Minzenberg, M. J., Carter, C. S. (2008). Modafinil: a review of neurochemical actions and effects on cognition. Neuropsychopharmacology. 33 (7), 1477–1502. doi: 10.1038/sj.npp.1301534
Mishima, K., Tanoue, A., Tsuda, M., Hasebe, N., Fukue, Y., Egashira, N., et al. (2004). Characteristics of behavioral abnormalities in alpha1d-adrenoceptors deficient mice. Behav. Brain Res. 152, 365–373. doi: 10.1016/j.bbr.2003.10.038
Mitrano, D., Schroeder, J., Smith, Y., Cortright, J. J., Bubula, N., Vezina, P., et al. (2012). Alpha-1 Adrenergic Receptors are Localized on Presynaptic Elements in the Nucleus Accumbens and Regulate Mesolimbic Dopamine Transmission. Neuropsychopharmacol. 37, 2161–2172. doi: 10.1038/npp.2012.68
Morikawa, H., Paladini, C. A. (2011). Dynamic regulation of midbrain dopamine neuron activity: intrinsic, synaptic, and plasticity mechanisms. Neuroscience. 198, 95–111. doi: 10.1016/j.neuroscience.2011.08.023
Morrow, A. L., Creese, I. (1986). Characterization of alpha 1-adrenergic receptor subtypes in rat brain: a reevaluation of [3H]WB4104 and [3H]prazosin binding. Mol. Pharmacol. 29, 321–330.
Moshfegh, A., Babaei, P., Oryan, S., Soltani, B., Zarrindast, M. R., Moshfegh, A., et al. (2011). Involvement of dorsal hippocampal α1-adrenergic receptors in the effect of WIN55,212-2 on memory retrieval in inhibitory avoidance task. Neurosci. Lett. 489 (2), 69–73. doi: 10.1016/j.neulet.2010.07.079
Mouly, A. M., Elaagouby, A., Ravel, N. (1995). A study of the effects of noradrenaline in the rat olfactory bulb using evoked field potential response. Brain Res. 681, 47–57. doi: 10.1016/0006-8993(95)00280-4
Mouradian, R. D., Sessler, F. M., Waterhouse, B. D. (1991). Noradrenergic potentiation of excitatory transmitter action in cerebrocortical slices: evidence for mediation by an alpha 1 receptor-linked second messenger pathway. Brain Res. 546, 83–95. doi: 10.1016/0006-8993(91)91162-T
Mulkey, R. M., Malenka, R. C. (1992). Mechanisms underlying induction of homo-synaptic long-term depression in area CA1 of the hippocampus. Neuron. 9, 967–975. doi: 10.1016/0896-6273(92)90248-C
Musselman, D. M., Ford, A. P., Gennevois, D. J., Harbison, M. L., Laurent, A. L., Mokatrin, A. S., et al. (2004). A randomized crossover study to evaluate Ro 115-1240, a selective α1A/L -adrenoceptor partial agonist in women with stress urinary incontinence. BJU. Int. 93, 78–83. doi: 10.1111/j.1464-410X.2004.04560.x
Nai, Q., Dong, H. W., Hayar, A., Linster, C., Ennis, M. (2009). Noradrenergic regulation of GABAergic inhibition of main olfactory bulb mitral cells varies as a function of concentration and receptor subtype. J. Neurophysiol. 101 (5), 2472–2484. doi: 10.1152/jn.91187.2008
Nai, Q., Dong, H. W., Linster, C., Ennis, M. (2010). Activation of alpha1 and alpha2 noradrenergic receptors exert opposing effects on excitability of main olfactory bulb granule cells. Neuroscience 169 (2), 882–892. doi: 10.1016/j.neuroscience.2010.05.010
Newman, L. A., Korol, D. L., Gold, P. E. (2011). Lactate Produced by Glycogenolysis in Astrocytes Regulates Memory Processing. PloS One 6 (12), e28427. doi: 10.1371/journal.pone.0028427
Nguyen, P. V., Connor, S. A. (2019). Noradrenergic Regulation of Hippocampus-Dependent Memory. Cent. Nerv. Syst. Agents Med. Chem. 19 (3), 187–196. doi: 10.2174/1871524919666190719163632
Nguyen, P. V., Gelinas, J. N. (2018). Noradrenergic gating of long-lasting synaptic potentiation in the hippocampus: from neurobiology to translational biomedicine. J. Neurogenet. 32 (3), 171–182. doi: 10.1080/01677063.2018.1497630
Nicholas, A. P., Pieribone, V. A., Hokfelt, T. (1993). Cellular localization of messenger RNA for beta-1 and beta-2 adrenergic receptors in rat brain: an in situ hybridization study. Neuroscience 56, 1023–1039.
Nicholson, R., Dixon, A. K., Spanswick, D., Lee, K. (2005). Noradrenergic receptor mRNA expression in adult rat superficial dorsal horn and dorsal root ganglion neurons. Neurosci. Lett. 380 (3), 316–321. doi: 10.1016/j.neulet.2005.01.079
Oe, Y., Wang, X., Patriarchi, T., Konno, A., Ozawa, K., Yahagi, K., et al. (2020). Distinct temporal integration of noradrenaline signaling by astrocytic second messengers during vigilance. Nat. Commun. 11, 471. doi: 10.1038/s41467-020-14378-x
Okutani, F., Kaba, H., Takahash,i, S., Seto, K. (1998). The biphasic effects of locus coeruleus noradrenergic activation on dendrodendritic inhibition in the rat olfactory bulb. Brain Res. 783, 272–279. doi: 10.1016/S0006-8993(97)01371-1
Ordway, G. A., Schwartz, M. A., Frazer, A. (Eds.) (2007a). “Brain Norepinephrine,” in Part III. Norepinephrine and behavioral (Cambridge: Cambridge University Press), pp.157–pp.298.
Ordway, G. A., Schwartz, M. A., Frazer, A. (Eds.) (2007b). “Brain Norepinephrine,” in Part III. The biology of norepinephrine in CNS pathology (Cambridge: Cambridge University Press), 299–514.
Palacios, J. M., Hoyer, D., Cortés, R. (1987). Alpha 1-Adrenoceptors in the mammalian brain: similar pharmacology but different distribution in rodents and primates. Brain Res. 419, 65–75. doi: 10.1016/0006-8993(87)90569-5
Paladini, C. A., Tepper, J. M. (1999). GABA(A) and GABA(B) antagonists differentially affect the firing pattern of substantia nigra dopaminergic neurons in vivo. Synapse. 32, 165–176. doi: 10.1002/(SICI)1098-2396(19990601)32:3<165::AID-SYN3>3.0.CO;2-N
Paladini, C. A., Williams, J. T. (2004). Noradrenergic inhibition of midbrain dopamine neurons. J. Neurosci. 24, 4568–4575. doi: 10.1523/JNEUROSCI.5735-03.2004
Paladini, C. A., Fiorillo, C. D., Morikawa, H., Williams, J. T. (2001). Amphetamine selectively blocks inhibitory glutamate transmission in dopamine neurons. Nat. Neurosci. 4, 275–281. doi: 10.1038/85124
Paladini, C. A., Robinson, S., Morikawa, H., Williams, J. T., Palmiter, R. D. (2003). Dopamine controls the firing pattern of dopamine neurons via a network feedback mechanism. Proc. Natl. Acad. Sci. U. S. A. 100 (5), 2866–2871. doi: 10.1073/pnas.0138018100
Pankratov, Y., Lalo, U. (2015). Role for astroglial α1-adrenoreceptors in gliotransmission and control of synaptic plasticity in the neocortex. Front. Cell Neurosci. 9:230. doi: 10.3389/fncel.2015.00230
Panzanelli, P., Bardy, C., Nissant, A., Pallotto, M., Sassoè-Pognetto, M., Lledo, P. M., et al. (2009). Early synapse formation in developing interneurons of the adult olfactory bulb. J. Neurosci. 29 (48), 15039–15052. doi: 10.1523/JNEUROSCI.3034-09.2009
Papay, R., Gaivin, R., McCune, D. F., Rorabaugh, B. R., Macklin, W. B., McGrath, J. C., et al. (2004). Mouse alpha1B-adrenergic receptor is expressed in neurons and NG2 oligodendrocytes. J. Comp. Neurol. 478, 1–10. doi: 10.1002/cne.20215
Papay, R., Gaivin, R., Jha, A., McCune, D. F., McGrath, J. C., Rodrigo, M. C., et al. (2006). Localization of the mouse alpha1A-adrenergic receptor (AR) in the brain: alpha1AAR is expressed in neurons, GABAergic interneurons, and NG2 oligodendrocyte progenitors. J. Comp. Neurol. 497, 209–222. doi: 10.1002/cne.20992
Parpura, V., Grubišic, V., Verkhratsky, A. (2011). Ca(2+) sources for the exocytotic release of glutamate from astrocytes. Biochim. Biophys. Acta 1813, 984–991. doi: 10.1016/j.bbamcr.2010.11.006
Pascual, O., Casper, K. B., Kubera, C., Zhang, J., Revilla-Sanchez, R., Sul, J.-Y., et al. (2005). Astrocytic purinergic signaling coordinates synaptic networks. Science. 310 (5745), 113–116. doi: 10.1126/science.1116916
Pastoll, H., Solanka, L., van Rossum, M. C., Nolan, M. F. (2013). Feedback inhibition enables theta-nested gamma oscillations and grid firing fields. Neuron. 77, 141–154. doi: 10.1016/j.neuron.2012.11.032
Paukert, M., Agarwal, A., Cha, J., Doze, V. A., Kang, J. U., Bergles, D. E. (2014). Norepinephrine controls astroglial responsiveness to local circuit activity. Neuron. 82 (6), 1263–1270. doi: 10.1016/j.neuron.2014.04.038
Pearson-Leary, J., Osborne, D. M., McNay, E. C. (2016). Role of Glia in Stress-Induced Enhancement and Impairment of Memory. Front. Integr. Neurosci. 9, 63. doi: 10.3389/fnint.2015.00063
Pedarzani, P., Storm, J. F. (1996). Interaction between alpha- and beta- 2+ adrenergic receptor agonists modulating the slow Ca -activated K+ current IAHP in hippocampal neurons. Eur. J. Neurosci. 8 (10), 2098–2110. doi: 10.1111/j.1460-9568.1996.tb00731.x
Perez, H., Hernandez, A., Almli, C. R. (1987). Locus coeruleus stimulation modulates olfactory bulb evoked potentials. Brain Res. Bull. 18, 767–770. doi: 10.1016/0361-9230(87)90213-9
Perez, D. M., Piascik, M. T., Graham, R. M. (1991). Solution-phase library screening for the identification of rare clones: isolation of an alpha 1D-adrenergic receptor cDNA. Mol. Pharmacol. 40, 876–883.
Perez, D. M., Piascik, M. T., Malik, N., Gaivin, R., Graham, R. M. (1994). Cloning, expression, and tissue distribution of the rat homolog of the bovine alpha 1C-adrenergic receptor provide evidence for its classification as the alpha 1A subtype. Mol. Pharmacol. 46, 823–831.
Perez, D. M., Stauffer, S., Macdonald, J. (2019). Allosteric Activators of the Alpha1A-Adrenergic Receptor. U.S. Provisional Patent Application No. 62/837,565 (Washington, DC: U.S. Patent and Trademark Office).
Petrakis, I. L., Desai, N., Gueorguieva, R., Arias, A., O’Brien, E., Jane, J. S., et al. (2016). Prazosin for Veterans with Posttraumatic Stress Disorder and Comorbid Alcohol Dependence: A Clinical Trial. Alcoholism Clin. Exp. Res. 40 (1), 178–186. doi: 10.1111/acer.12926
Petrasek, T., Doulames, V., Prokopova, I., Vales, K., Stuchlik, A., Petrasek, T., et al. (2010). Combined administration of alpha1-adrenoceptor antagonist prazosin and beta-blocker propranolol impairs spatial avoidance learning on a dry arena. Behav. Brain Res. 208 (2), 402–407. doi: 10.1016/j.bbr.2009.12.025
Pieribone, V. A., Nicholas, A. P., Dagerlind, A., Hokfelt, T. (1994). Distribution of α1 adrenoceptors in rat brain revealed by in situ hybridization experiments utilizing subtype-specific probes. J. Neurosci. 14, 4252–4268. doi: 10.1523/JNEUROSCI.14-07-04252.1994
Pissonnier, D., Thiery, J. C., Fabre-Nys, C., Poindron, P., Keverne, E. B. (1985). The importance of olfactory bulb noradrenalin for maternal recognition in sheep. Physiol. Behav. 35, 361–363. doi: 10.1016/0031-9384(85)90309-9
Pohlmann, A., Karczewski, P., Ku, C. M., Dieringer, B., Waiczies, H., Wisbrund, N., et al. (2014). Cerebral blood volume estimation by ferumoxytol-enhanced steady-state MRI at 9.4. T reveals microvascular impact of α1-adrenergic receptor antibodies. NMR. Biomed. 27, 1085–1093. doi: 10.1002/nbm.3160
Poirel, O., Mella, S., Videau, C., Ramet, L., Davoli, M. A., Herzog, E., et al. (2018). Moderate decline in select synaptic markers in the prefrontal cortex (BA9) of patients with Alzheimer’s disease at various cognitive stages. Sci. Rep. 8, 938. doi: 10.1038/s41598-018-19154-y
Porter-Stransky, K. A., Centanni, S. W., Karne, S. L., Odil, L. M., Fekir, S., Wong, J. C., et al. (2019). Noradrenergic Transmission at Alpha1-Adrenergic Receptors in the Ventral Periaqueductal Gray Modulates Arousal. Biol. Psychiatry 85 (3), 237–247. doi: 10.1016/j.biopsych.2018.07.027
Pussinen, R., Nieminen, S., Koivisto, E., Haapalinna, A., Riekkinen, P., Sirvio, J. (1997). Enhancement of intermediate-term memory by an α-1 agonist or a partial agonist at the glycine site of the NMDA receptor. Neurobiol. Learn. Mem. 67, 69–74. doi: 10.1006/nlme.1996.3738
Puumala, T., Greijus, S., Narinen, K., Haapalinna, A., Riekkinen, P., Sirviö, J. (1998). Stimulation of α-1 adrenergic receptors facilitates spatial learning in rats. Eur. Neuropsychopharmacol. 8, 17–26. doi: 10.1016/S0924-977X(97)00040-0
Quilichini, P., Sirota, A., Buzsaki, G. (2010). Intrinsic circuit organization and theta-gamma oscillation dynamics in the entorhinal cortex of the rat. J. Neurosci. 30, 11128–11142. doi: 10.1523/JNEUROSCI.1327-10.2010
Rajagopal, S., Shenoy, S. K. (2018). GPCR desensitization: Acute and prolonged phases. Cell Signal. 41, 9–16. doi: 10.1016/j.cellsig.2017.01.024
Rangel, S., Leon, M. (1995). Early odor preference training increases olfactory bulb norepinephrine. Brain Res. Dev. Brain Res. 85, 187–191. doi: 10.1016/0165-3806(94)00211-H
Raskind, M. A., Peskind, E. R., Kanter, E. D., Petrie, E. C., Radant, A., Thompson, C. E., et al. (2003). Reduction of nightmares and other PTSD symptoms in combat veterans by prazosin: a placebo-controlled study. Am. J. Psychiatry 160, 371–373. doi: 10.1176/appi.ajp.160.2.371
Raskind, M. A., Peskind, E. R., Hoff, D. J., Hart, K. L., Holmes, H. A., Warren, D., et al. (2007). A parallel group placebo-controlled study of prazosin for trauma nightmares and sleep disturbance in combat veterans with post-traumatic stress disorder. Biol. Psychiatry 61, 928–934. doi: 10.1016/j.biopsych.2006.06.032
Rey, E., Hernandez-Diaz, F. J., Abreu, P., Alonso, R., Tabares, L. (2001). Dopamine induces intracellular Ca2+ signals mediated by alpha1B-adrenoceptors in rat pineal cells. Eur. J. Pharmacol. 430, 9–17. doi: 10.1016/S0014-2999(01)01250-X
Riekkinen, M., Kemppainen, S., Riekkinen, P., Jr. (1997). Effects of stimulation of alpha 1-adrenergic and NMDA/glycine-B receptors on learning defects in aged rats. Psychopharmacol. (Berl). 131 (1), 49–56. doi: 10.1007/s002130050264
Robbins, T. W., Arnsten, A. F. (2009). The neuropsychopharmacology of fronto-executive function: monoaminergic modulation. Annu. Rev. Neurosci. 32, 267–287. doi: 10.1146/annurev.neuro.051508.135535
Rokosh, D. G., Simpson, P. C. (2002). Knockout of the alpha1A/C-adrenergic receptor subtype: the alpha1A/C is expressed in resistance arteries and is required to maintain arterial blood pressure. Proc. Natl. .Acad. Sci. USA. 99, 9474–9479. doi: 10.1073/pnas.132552699
Rorabaugh, J. M., Chalermpalanupap, T., Botz-Zapp, C. A., Fu, V. M., Lembeck, N. A., Cohen, R. M., et al. (2017). Chemogenetic locus coeruleus activation restores reversal learning in a rat model of Alzheimer’s disease. Brain 140, 3023–3038. doi: 10.1093/brain/awx232
Ruffolo, R. R., Jr, Waddell, J. E. (1982). Receptor interactions of imidazolines. IX. Cirazoline is an α 1 adrenergic agonist and an α 2 adrenergic antagonist. J. Pharmacol. Exp. Ther. 222 (1), 29–36.
Ruffolo, R. R., Jr, Waddell, J. E. (1983). Aromatic and benzylic hydroxyl substitution of imidazolines and phenethylamines: differences in activity at α1 and α2-adrenergic receptors J. Pharmacol. Exp. Ther. 224, 559–566.
Ruffolo, R. R., Jr, Yaden, E. L., Waddell, J. E., Dillard, R. D. (1980). Receptor interactions of imidazolines. VI. Significance of carbon bridge separating phenyl and imidazoline rings of tolazoline-like α-adrenergic imidazolines. J. Pharmacol. Exp. Ther. 214, 535–540.
Rutecki, P. A. (1995). Noradrenergic modulation of epileptiform activity in the hippocampus. Epilepsy Res. 20, 125–136. doi: 10.1016/0920-1211(94)00078-B
Sadalge, A., Coughlin, L., Fu, H., Wang, B., Valladares, O., Valentino, R., et al. (2003). α1d Adrenoceptor signaling is required for stimulus induced locomotor activity. Mol. Psychiatry 8, 664–672. doi: 10.1038/sj.mp.4001351
Salgado, H., Garcia-Oscos, F., Patel, A., Martinolich, L., Nichols, J. A., Dinh, L., et al. (2011). Layer-specific noradrenergic modulation of inhibition in cortical layer II/III. Cereb. Cortex. 21, 212–221. doi: 10.1093/cercor/bhq081
Santana, N., Mengod, G., Artigas, F. (2013). Expression of α1-adrenergic receptors in rat prefrontal cortex: cellular co-localization with 5-HT2A receptors. Int. J. Neuropsychopharmacol. 16, 1139–1151. doi: 10.1017/S1461145712001083
Scanziani, M., Gähwiler, B. H., Thompson, S. M. (1993). Presynaptic inhibition of excitatory synaptic transmission mediated by α-adrenergic receptors in area CA3 of the rat hippocampus in vitro. J. Neurosci. 13, 5393–5401. doi: 10.1523/JNEUROSCI.13-12-05393.1993
Schambra, U. B., Mackensen, G. B., Stafford-Smith, M., Haines, D. E., Schwinn, D. A. (2005). Neuron specific alpha-adrenergic receptor expression in human cerebellum: implications for emerging cerebellar roles in neurologic disease. Neuroscience 135 (2), 507–523. doi: 10.1016/j.neuroscience.2005.06.021
Scheiderer, C. L., Dobrunz, L. E., McMahon, L. L. (2004). Novel form of long-term synaptic depression in rat hippocampus induced by activation of alpha 1 adrenergic receptors. J. Neurophysiol. 91, 1071–1077. doi: 10.1152/jn.00420.2003
Scheiderer, C. L., Smith, C. C., McCutchen, E., McCoy, P. A., Thacker, E. E., Kolasa, K., et al. (2008). Coactivation of M(1) muscarinic and alpha1 adrenergic receptors stimulates extracellular signal-regulated protein kinase and induces long-term depression at CA3-CA1 synapses in rat hippocampus. J. Neurosci. 28 (20), 5350–5358. doi: 10.1523/JNEUROSCI.5058-06.2008
Schultz, J., Daly, J. W. (1973). Accumulation of cyclic adenosin 3′,5′-monophosphate in cerebral cortical slices from rat and mouse: stimulatory effect of α- and β-adrenergic agents and adenosine. J. Neurochem. 21, 1319–1326. doi: 10.1111/j.1471-4159.1973.tb07585.x
Schultz, P. E., Cook, E. P., Johnston, D. (1994). Changes in Paired-Pulse Facilitation Suggest Presynaptic Involvement in Long-Term Potentiation. J. Neurosci. 14 (9), 5325–5337. doi: 10.1523/JNEUROSCI.14-09-05325.1994
Schultz, W. (2015). Neuronal reward and decision signals: from theories to data. Physiol. Rev. 95, 853–951. doi: 10.1152/physrev.00023.2014
Schwarz, K. R., Lanier, S. M., Carter, E. A., Homcy, C. J., Graham, R. M. (1985). Rapid reciprocal changes in adrenergic receptors in intact isolated hepatocytes during primary cell culture. Mol. Pharmacol. 27, 200–209.
Scoriels, L., Jones, P. B., Sahakian, B. J. (2013). Modafinil effects on cognition and emotion in schizophrenia and its neurochemical modulation in the brain. Neuropharmacology. 64, 168–184. doi: 10.1016/j.neuropharm.2012.07.011
Shao, Y., Sutin, J. (1992). Expression of adrenergic receptors in individual astrocytes and motor neurons isolated from the adult rat brain. Glia. 6 (2), 108–117. doi: 10.1002/glia.440060205
Sharpe, I. A., Thomas, L., Loughnan, M., Motin, L., Palant, E., Croker, D. E., et al. (2003). Allosteric α1-adrenoreceptor antagonism by the conopeptide ρ-TIA. J. Biol. Chem. 278, 34451–34457. doi: 10.1074/jbc.M305410200
Shimazaki, Y., Nishiki, T., Omori, A., Sekiguchi, M., Kamata, Y., Kozaki, S., et al. (1996). Phosphorylation of 25-kDa synaptosome-associated protein. Possible involvement in protein kinase C-mediated regulation of neurotransmitter release. J. Biol. Chem. 271, 14548–14553. doi: 10.1074/jbc.271.24.14548
Shimohama, S., Taniguchi, T., Fujiwara, M., Kameyama, M. (1986). Biochemical characterization of α1-adrenergic receptors in human brain and changes in Alzheimer-type dementia. J. Neurochem. 47 (4), 1295–1301. doi: 10.1111/j.1471-4159.1986.tb00753.x
Simard, M., Couldwell, W. T., Zhang, W., Song, H., Liu, S., Cotrina, M. L., et al. (1999). Glucocorticoids-potent modulators of astrocytic calcium signaling. Glia 28, 1–12. doi: 10.1002/(SICI)1098-1136(199910)28:1<1::AID-GLIA1>3.0.CO;2-4
Sirviö, J., MacDonald, E. (1999). Central alpha1-adrenoceptors: their role in the modulation of attention and memory formation. Pharmacol. Ther. 83, 49–65. doi: 10.1016/s0163-7258(99)00017-0
Skelly, M. J., Ariwodola, O. J., Weiner, J. L. (2017). Fear conditioning selectively disrupts noradrenergic facilitation of GABAergic inhibition in the basolateral amygdala. Neuropharmacology 113 (Pt A), 231–240. doi: 10.1016/j.neuropharm.2016.10.003
Spreng, M., Cotecchia, S., Schenk, F. (2001). A behavioral study of alpha-1b adrenergic receptor knockout mice: increased reaction to novelty and selectively reduced learning capacities. Neurobiol. Learn Mem. 75, 214–229. doi: 10.1006/nlme.2000.3965
Squire, L. R., Stark, C. E., Clark, R. E. (2004). The medial temporal lobe. Annu. Rev. Neurosci. 27, 279–306. doi: 10.1146/annurev.neuro.27.070203.144130
Stanasila, L., Perez, J. B., Vogel, H., Cotecchia, S. (2003). Oligomerization of the alpha 1a- and alpha 1b-adrenergic receptor subtypes. Potential implications in receptor internalization. J. Biol. Chem. 278 (41), 40239–40251.
Stanton, P. K., Jones, R. S., Mody, I., Heinemann, U. (1987). Epileptiform activity induced by lowering extracellular [Mg2+] in combined hippocampal-entorhinal cortex slices: modulation by receptors for norepinephrine and N-methyl-D-aspartate. Epilepsy Res. 1, 53–62. doi: 10.1016/0920-1211(87)90051-9
Steffenach, H. A., Witter, M., Moser, M. B., Moser, E. I. (2005). Spatial memory in the rat requires the dorsolateral band of the entorhinal cortex. Neuron. 45, 301–313. doi: 10.1016/j.neuron.2004.12.044
Stevens, C. F., Sullivan, J. M. (1998). Regulation of the readily releasable vesicle pool by protein kinase C. Neuron. 21 (4), 885–893. doi: 10.1016/S0896-6273(00)80603-0
Stone, E. A., Platt, J. E., Herrera, A. S., Kirk, K. L. (1986). Effect of repeated restraint stress, desmethylimipramine or adrenocorticotropin on the alpha and beta adrenergic components of the cyclic AMP response to norepinephrine in rat brain slices. J. Pharmacol. Exp. Ther. 237 (3), 702–707.
Stone, E. A., McEwen, B. S., Herrera, A. S., Carr, K. D. (1987). Regulation of α and β components of noradrenergic cyclic AMP response in cortical slices. Eur. J. Pharmacol. 141, 347–356. doi: 10.1016/0014-2999(87)90551-6
Stone, E. A., Cotecchia, S., Lin, Y., Quartermain, D. (2002). Role of brain alpha 1B-adrenoceptors in modafinil-induced behavioral activity. Synapse. 46, 269–270. doi: 10.1002/syn.10127
Stuchlik, A., Vales, K. (2008a). Role of alpha1- and alpha2-adrenoceptors in the regulation of locomotion and spatial behavior in the active place avoidance task: a dose-response study. Neurosci. Lett. 3), 235–240. doi: 10.1016/j.neulet.2008.01.013
Stuchlik, A., Petrasek, T., Vales, K. (2008b). Dopamine D2 receptors and alpha1-adrenoceptors synergistically modulate locomotion and behavior of rats in a place avoidance task. Behav. Brain Res. 189 (1), 139–144. doi: 10.1016/j.bbr.2007.12.025
Sullivan, R. M., Wilson, D. A., Leon, M. (1989). Norepinephrine and learning-induced plasticity in infant rat olfactory system. J. Neurosci. 9, 3998–4006. doi: 10.1523/JNEUROSCI.09-11-03998.1989
Sullivan, R. M., Zyzak, D. R., Skierkowsk,i, P., Wilson, D. A. (1992). The role of olfactory bulb norepinephrine in early olfactory learning. Brain Res. Dev. Brain Res. 70, 279–282. doi: 10.1016/0165-3806(92)90207-D
Szot, P., White, S. S., Greenup, J. L., Leverenz, J. B., Peskind, E. R., Raskind, M. A. (2005). Alpha1-adrenoreceptor in human hippocampus: binding and receptor subtype mRNA expression. Brain Res. Mol. Brain Res. 139 (2), 367–371. doi: 10.1016/j.molbrainres.2005.06.013
Szot, P., White, S. S., Greenup, J. L., Leverenz, J. B., Peskind, E. R., Raskind, M. A. (2006). Compensatory changes in the noradrenergic nervous system in the locus ceruleus and hippocampus of postmortem subjects with Alzheimer’s disease and dementia with Lewy bodies. J. Neurosci. 26, 467–478. doi: 10.1523/JNEUROSCI.4265-05.2006
Szot, P., White, S. S., Greenup, J. L., Leverenz, J. B., Peskind, E. R., Raskind, M. A. (2007). Changes in adrenoreceptors in the prefrontal cortex of subjects with dementia: evidence of compensatory changes. Neuroscience 146 (1), 471–480. doi: 10.1016/j.neuroscience.2007.01.031
Tamagnini, F., Burattini, C., Casoli, T., Balietti, M., Fattoretti, P., Aicardi, G. (2012). Early impairment of long-term depression in the perirhinal cortex of a mouse model of Alzheimer’s disease. Rejuv. Res. 15 (2), 231–234. doi: 10.1089/rej.2011.1311
Tanoue, A., Nasa, Y., Koshimizu, T., Shinoura, H., Oshikawa, S., Kawai, T., et al. (2002). The alpha(1D)-adrenergic receptor directly regulates arterial blood pressure via vasoconstriction. J. Clin. Invest. 109, 765–775. doi: 10.1172/JCI200214001
Taylor, H. R., Freeman, M. K., Cates, M. E. (2008). Prazosin for treatment of nightmares related to posttraumatic stress disorder. Am. J. Health Syst. Pharm. 65, 716–722. doi: 10.2146/ajhp070124
Theofilas, P., Ehrenberg, A. J., Dunlop, S., DiLorenzo, Alho, A. T., Nguy, A., et al. (2017). Locus coeruleus volume and cell population changes during Alzheimer’s disease progression: a stereological study in human postmortem brains with potential implication for early-stage biomarker discovery. Alzheimers Dement. 13, 236–246. doi: 10.1016/j.jalz.2016.06.2362
Thomas, S. A., Palmiter, R. D. (1997a). Disruption of the dopamine B-hydroxylase gene in mice suggests roles for norepinephrine in motor function, learning, and memory. Behav. Neurosci. 111, 579–589. doi: 10.1037/0735-7044.111.3.579
Thomas, S. A., Palmiter, R. D. (1997b). Impaired maternal behavior in mice lacking norepinephrine and epinephrine. Cell. 91, 583–592. doi: 10.1016/S0092-8674(00)80446-8
Thomas, S. A., Palmiter, R. D. (1997c). Thermoregulatory and metabolic phenotypes of mice lacking noradrenaline and adrenaline. Nature 387, 94–97. doi: 10.1038/387094a0
Thyrian, J. R., Hertel, J., Schulze, L. N., Dörr, M., Prüss, H., Hempel, P., et al. (2018). Prevalence and Determinants of Agonistic Autoantibodies Against α1-Adrenergic Receptors in Patients Screened Positive for Dementia: Results from the Population-Based DelpHi-Study. J. Alzheimers Dis. 64 (4), 1091–1097. doi: 10.3233/JAD-171096
Torkaman-Boutorabi, A., Danyali, F., Oryan, S., Ebrahimi-Ghiri, M., Zarrindast, M. R. (2014). Hippocampal α-adrenoceptors involve in the effect of histamine on spatial learning. Physiol. Behav. 129, 17–24. doi: 10.1016/j.physbeh.2014.02.009
Tovar-Díaz, J., Pomrenze, J., Kan, M. B., Pahlavan, R., Morikawa, B., Tovar-Díaz J, H., et al. (2018). Cooperative CRF and α1 Adrenergic Signaling in the VTA Promotes NMDA Plasticity and Drives Social Stress Enhancement of Cocaine Conditioning. Cell Rep. 22 (10), 2756–2766. doi: 10.1016/j.celrep.2018.02.039
Treviño, M., Frey, S., Köhr, G. (2012). Alpha-1 adrenergic receptors gate rapid orientation-specific reduction in visual discrimination. Cereb. Cortex. 22 (11), 2529–2541. doi: 10.1093/cercor/bhr333
Tzschentke, T. M. (2000). The medial prefrontal cortex as a part of the brain reward system. Amino Acids 19 (1), 211–219. doi: 10.1007/s007260070051
Ul Haq, R., Liotta, A., Kovacs, R., Rösler, A., Jarosch, M. J., Heinemann, U., et al. (2012). Adrenergic modulation of sharp wave-ripple activity in rat hippocampal slices. Hippocampus. 22, 516–533. doi: 10.1002/hipo.20918
Unnerstall, J. R., Fernandez, I., Orensanz, L. M. (1985). The alpha-adrenergic receptor: radiohistochemical analysis of functional characteristics and biochemical differences. Pharmacol. Biochem. Behav. 22, 859–874. doi: 10.1016/0091-3057(85)90538-6
Valley, M. T., Henderson, L. G., Inverso, S. A., Lledo, P. M. (2013). Adult neurogenesis produces neurons with unique GABAergic synapses in the olfactory bulb. J. Neurosci. 33 (37), 14660–14665.
Vanhoose, A. M., Emery, M., Jimenez, L., Winder, D. G. (2002). ERK activation by G-protein-coupled receptors in mouse brain is receptor identity-specific. J. Biol. Chem. 277, 9049–9053. doi: 10.1074/jbc.M108309200
Velásquez-Martinez, M. C., Vázquez-Torres, R., Jiménez-Rivera, C. A. (2012). Activation of alpha1-adrenoceptors enhances glutamate release onto ventral tegmental area dopamine cells. Neuroscience. 216, 18–30. doi: 10.1016/j.neuroscience.2012.03.056
Verkhratsky, A., Parpura, V., Vardjan, N., Zorec, R. (2019). Physiology of Astroglia. Adv. Exp. Med. Biol. 1175, 45–91. doi: 10.1007/978-981-13-9913-8_3
Wada, S., Yanagida, J., Sasase, H., Zhang, T., Li, X., Kamii, H., et al. (2020). Acute restraint stress augments the rewarding memory of cocaine through activation of α1 adrenoceptors in the medial prefrontal cortex of mice. Neuropharmacology 166, 107968. doi: 10.1016/j.neuropharm.2020.107968
Walling, S. G., Harley, C. W. (2004). Locus ceruleus activation initiates delayed synaptic potentiation of perforant path input to the dentate gyrus in awake rats: a novel beta-adrenergic- and protein synthesis-dependent mammalian plasticity mechanism. Neurosci. 24, 598–604. doi: 10.1523/JNEUROSCI.4426-03.2004
Wallukat, G., Wollenberger, A. (1987). “Involvement of β2-adrenergic receptors in the potentaion of the chronotropic action of isoprenaline evoked in rocker-cultured neonatal rat heart cells by pyruvate and L (+) lactate,” in Parmacological aspects of heart disease. Eds. Beamisch, R. E., Panagia, V., Dhalla, N. S. (Boston: Martinius Nijhoff Publishing), pp 217–pp 231.
Wang, M., Ramos, B. P., Paspalas, C. D., Shu, Y., Simen, A., Duque, A., et al. (2007). Alpha2A-adrenoceptors strengthen working memory networks by inhibiting cAMP-HCN channel signaling in prefrontal cortex. Cell. 129, 397–410. doi: 10.1016/j.cell.2007.03.015
Wang, C., Liu, Y., Cao, J. M. (2014). G protein-coupled receptors: extranuclear mediators for the non-genomic actions of steroids. Int. J. Mol. Sci. 15 (9), 15412–15425. doi: 10.3390/ijms150915412
Watabe, A. M., Zaki, P. A., O’Dell, T. J. (2000). Coactivation of beta-adrenergic and cholinergic receptors enhances the induction of long-term potentiation and synergistically activates mitogen-activated protein kinase in the hippocampal CA1 region. J. Neurosci. 20, 5924–5931. doi: 10.1523/JNEUROSCI.20-16-05924.2000
Webb, D. R., Handel, T. M., Kretz-Rommel, A., Stevens, R. C. (2013). Opportunities for functional selectivity in GPCR antibodies. Biochem. Pharmacol. 85 (2), 147–152. doi: 10.1016/j.bcp.2012.08.021
Williams, A. M., Nguyen, M. L. D., Morilak, D. A. (1997). Co-localization of α1D Adrenergic Receptor mRNA with Mineralocorticoid and Glucocorticoid Receptor mRNA in Rat Hippocampus. J. Neuroendo. 9, 113–119. doi: 10.1046/j.1365-2826.1997.00522.x
Winder-Rhodes, S. E., Chamberlain, S. R., Idris, M. I., Robbins, T. W., Sahakian, B. J., Müller, U. (2010). Effects of modafinil and prazosin on cognitive and physiological functions in healthy volunteers. J. Psychopharmacol. Oxf. Engl. 24, 1649–1657. doi: 10.1177/0269881109105899
Wu, X. S., Wu, L. G. (2001). Protein kinase c increases the apparent affinity of the release machinery to Ca2+ by enhancing the release machinery downstream of the Ca2+ sensor. J. Neurosci. 21, 7928– 7936. doi: 10.1523/JNEUROSCI.21-20-07928.2001
Xie, J., Ho Lee, Y., Wang, C., Mo Chung, J., Chung, K. (2001). Differential expression of alpha1-adrenoceptor subtype mRNAs in the dorsal root ganglion after spinal nerve ligation. Brain Res. Mol. Brain Res. 93 (2), 164–172. doi: 10.1016/S0169-328X(01)00201-7
Xu, L., Anwyl, R., Rowan, M. J. (1998). Spatial exploration induces a persistent reversal of long-term potentiation in rat hippocampus. Nature. 394, 891–894. doi: 10.1038/29783
Yamada, M., Mehraein, P. (1977). Distribution of Senile Changes in Brain Stem Nuclei. Psychiatry Clin. Neurosci. 31, 219–224. doi: 10.1111/j.1440-1819.1977.tb02722.x
Yuan, W. X., Chen, S. R., Chen, H., Pan, H. L. (2009). Stimulation of alpha(1)-adrenoceptors reduces glutamatergic synaptic input from primary afferents through GABA(A) receptors and T-type Ca(2+) channels. Neuroscience. 158, 1616–1624. doi: 10.1016/j.neuroscience.2008.11.022
Zarow, C., Lyness, S. A., Mortimer, J. A., Chui, H. C. (2003). Neuronal loss is greater in the locus coeruleus than nucleus basalis and substantia nigra in Alzheimer and Parkinson diseases. Arch. Neurol. 60, 337–341. doi: 10.1001/archneur.60.3.337
Zhang, W. P., Ouyang, M., Thomas, S. A. (2004). Potency of catecholamines and other L-tyrosine derivatives at the cloned mouse adrenergic receptors. Neuropharmacology. 47, 438–449. doi: 10.1016/j.neuropharm.2004.04.017
Zhang, Z., Cordeiro Matos, S., Jego, S., Adamantidis, A., Seguela, P. (2013). Norepinephrine drives persistent activity in prefrontal cortex via synergistic alpha1 and alpha2 adrenoceptors. PloS One 8, e66122. doi: 10.1371/journal.pone.0066122
Zhou, Z., Liao, Y., Li, L., Wei, F., Wang, B., Wei, Y., et al. (2008). Vascular damages in rats immunized by alpha1-adrenoceptor peptides. Cell Mol. Immunol. 5, 349–356. doi: 10.1038/cmi.2008.43
Zilles, K., Gross, G., Schleicher, A., Schildgen, S., Bauer, A., Bahro, M., et al. (1991). Regional and laminar distributions of alpha1-adrenoceptors and their subtypes in human and rat hippocampus. Neuroscience 40, 307–320. doi: 10.1016/0306-4522(91)90122-5
Zimnik, N. C., Treadway, T., Smith, R. S., Araneda, R. C. (2013). α(1A)-Adrenergic regulation of inhibition in the olfactory bulb. J. Physiol. 591 (7), 1631–1643. doi: 10.1113/jphysiol.2012.248591
Keywords: adrenergic receptor, G-protein coupled receptor, cognition, neurotransmission, synaptic plasticity
Citation: Perez DM (2020) α1-Adrenergic Receptors in Neurotransmission, Synaptic Plasticity, and Cognition. Front. Pharmacol. 11:581098. doi: 10.3389/fphar.2020.581098
Received: 07 July 2020; Accepted: 11 September 2020;
Published: 29 September 2020.
Edited by:
Ashok Kumar, University of Florida, United StatesReviewed by:
Dan Monaghan, University of Nebraska Medical Center, United StatesCopyright © 2020 Perez. This is an open-access article distributed under the terms of the Creative Commons Attribution License (CC BY). The use, distribution or reproduction in other forums is permitted, provided the original author(s) and the copyright owner(s) are credited and that the original publication in this journal is cited, in accordance with accepted academic practice. No use, distribution or reproduction is permitted which does not comply with these terms.
*Correspondence: Dianne M. Perez, UGVyZXpkQGNjZi5vcmc=
Disclaimer: All claims expressed in this article are solely those of the authors and do not necessarily represent those of their affiliated organizations, or those of the publisher, the editors and the reviewers. Any product that may be evaluated in this article or claim that may be made by its manufacturer is not guaranteed or endorsed by the publisher.
Research integrity at Frontiers
Learn more about the work of our research integrity team to safeguard the quality of each article we publish.