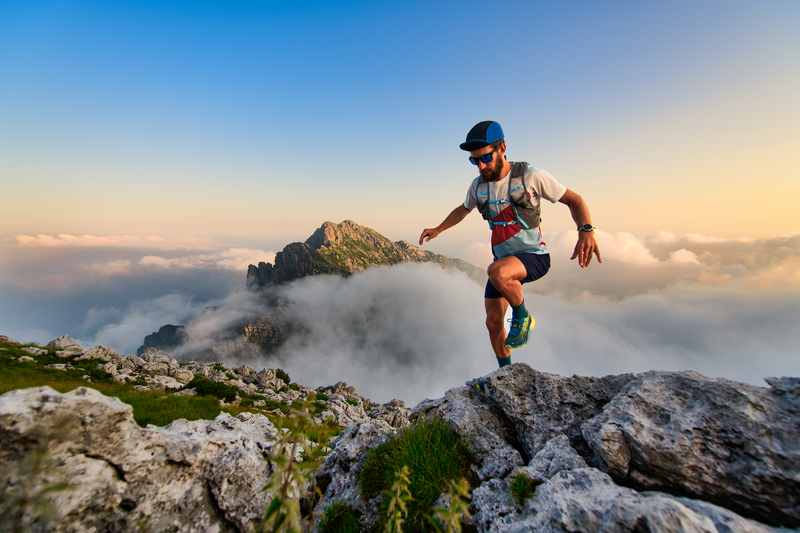
94% of researchers rate our articles as excellent or good
Learn more about the work of our research integrity team to safeguard the quality of each article we publish.
Find out more
MINI REVIEW article
Front. Pharmacol. , 21 August 2020
Sec. Cardiovascular and Smooth Muscle Pharmacology
Volume 11 - 2020 | https://doi.org/10.3389/fphar.2020.01314
This article is part of the Research Topic Cardiac Hypertrophy: From Compensation to Decompensation and Pharmacological Interventions View all 10 articles
Cardiac hypertrophy is an ongoing clinical challenge, as risk factors such as obesity, smoking and increasing age become more widespread, which lead to an increasing prevalence of developing hypertrophy. Pathological hypertrophy is a maladaptive response to stress conditions, such as pressure overload, and involve a number of changes in cellular mechanisms, gene expression and pathway regulations. Although several important pathways involved in the remodeling and hypertrophy process have been identified, further research is needed to achieve a better understanding and explore new and better treatment options. More recently discovered pathways showed the involvement of several non-coding RNAs, including micro RNAs (miRNAs), long non-coding RNAs (lncRNAs), and circular RNAs (circRNAs), which either promote or inhibit the remodeling process and pose a possible target for novel therapy approaches. In vitro modeling serves as a vital tool for this further pathway analysis and treatment testing and has vastly improved over the recent years, providing a less costly and labor-intensive alternative to in vivo animal models.
Left ventricular hypertrophy is a consequence of hypertension in up to 30% of patients (Cramariuc and Gerdts, 2016). Due to widespread risk factors such as obesity and smoking, the prevalence of hypertension and subsequent myocardial hypertrophy is rising, which poses a significant public health burden in an aging population (Benjamin et al., 2018). This development combined with the relative paucity of direct treatment options for cardiac hypertrophy makes continued research and the identification of novel therapeutic target molecules absolutely vital. Cardiac hypertrophy is an adaptive process which develops in response to physiological but also pathological processes, leading to heart muscle and cell hypertrophic growth with increased rigidity of the heart structures, and impaired diastolic function leading to heart failure with preserved ejection fraction (HFpEF) (Loonat et al., 2019; Zhao et al., 2020). Hypertrophic growth involves cardiomyocyte enlargement rather than division, as adult cardiomyocytes have lost the ability to divide (Porrello et al., 2013). Pathological hypertrophy leads to the loss of functional cardiomyocytes (Nakamura and Sadoshima, 2018) and can subsequently progress to heart failure with reduced ejection fraction (HFrEF) (Tham et al., 2015). The main focus in hypertrophy research lies in the investigation of signaling pathways, gene expression analysis, and production of certain proteins and transcription factors, which influence or are responsible for the remodeling process. Many pathways have been identified to be involved in the development of cardiac hypertrophy including calcineurin/nuclear factor of activated T cells (NFAT), mitogen-activated protein kinase ERK, small guanosine triphosphate (GTP)-binding proteins (Ras, Rho), PKC, transcriptional regulation, cell surface level control, miRNA, and many more (Stansfield et al., 2014). Hypertrophic signaling pathways are initiated through mechanical stimulation such as pressure overload and neurohumoral mechanisms including the release of signaling factors such as growth factors, hormones, cytokines, and chemokines (Heineke and Molkentin, 2006).
Non-coding RNAs are RNAs that do not code for a protein and are not translated, however they have been shown to interfere with and regulate numerous physiological and pathophysiological pathways. There are different classes of non-coding RNAs, include microRNAs (miRNAs), long non-coding RNAs (lncRNAs) and circular RNAs (circRNAs), depending on their structure, length and function (Jaé and Dimmeler, 2020). MiRNAs are small RNAs with a length of approximately 22 nucleotides, which can inhibit mRNA translation and signal mRNA degradation (O’Brien et al., 2018). LncRNAs comprise of over 200 nucleotides and they can induce structural changes in DNA and chromatin, therefore regulating gene expression, and bind miRNAs thereby inhibiting their function (Dhanoa et al., 2018). Similarly, circRNAs also act as a sponge for miRNAs, however their structural properties are different to other non-coding RNAs, as they are circularized and are more robust resistant to RNAses (Yu and Kuo, 2019).
The purpose of this mini-review is to summarize the developments in myocardial hypertrophy models, new pathways, tissue engineering and non-coding RNAs relevant in myocardial hypertrophy induction and progression.
Cell culture models are essential for the investigation of pathological and physiological pathways in cardiac diseases. In contrast, in vivo animal models more closely simulate human physiological and pathological conditions. In cardiac research the most popular animal models are rodents such as rats and mice (Zuppinger, 2019). However, they differ from human physiology in some key aspects, such as faster heart rates and stem cell phenotypes (Ginis et al., 2004; Jochmans-Lemoine et al., 2015). Several large animal models using pigs, sheep, or dogs have been established to more accurately simulate human pathophysiology and cardiac hypertrophy with HFpEF and HFrEF, but very high cost and labor intensity limit their use for specialized research questions (Spannbauer et al., 2019). Sophisticated in vitro models are therefore an important tool to elucidate new remodeling and hypertrophy pathways and identify molecules of interest before proceeding to costly translational models.
The most widely used technique is a 2D monolayer cell culture, which allowed the discovery of several important hypertrophy pathways and cellular mechanisms, but lacks the complexity of interactivity between cells and cell types with electrical and paracrine factors (Zuppinger, 2016). To achieve a more realistic in vitro model of cardiac hypertrophy where native in vivo niche conditions can be imitated, cell culture techniques have vastly improved in recent years, giving rise to 3D cultures and organoids (Dutta et al., 2017). Cardiac organoids have been developed and have steadily improved, however the optimal ratio of cardiomyocytes, fibroblasts and endothelial cells is the subject of ongoing debate (Nugraha et al., 2019). The most prominent techniques for producing cardiac organoids include the use of hydrogels such as collagen, cell sheet technology where cells are cultured and subsequently stacked in different layers and hanging drop culture (Dutta et al., 2017; Nugraha et al., 2019). Despite their many advantages, it is important to note that cardiac organoids in their current state are still far from the complexity of the organs they are meant to represent and are also unable to capture organism-wide processes like immune response or neurohumoral feedback mechanisms (Iakobachvili and Peters, 2017), which also limit their use in modeling of in vivo myocardial hypertrophy.
In vitro cardiac hypertrophy can be induced by various methods such as chemical stimulation or mechanical stress induction. Mechanical stress induction is achieved by pressure overload in vivo and simulated by stretching of cardiomyocytes in vitro. On a cellular level, an activation of different oncogenes such as c-fos, c-myc, c-jun, and Egr-1, also called immediate-early genes, and activation of heat shock protein-70 are triggered and lead to subsequent upregulation of hypertrophy inducing genes ANP, BNP, and β-MHC (Rysä et al., 2018).
Pharmacological agents that are used for hypertrophy induction are for example phenylephrine (Jain et al., 2018), angiotensin II (Gelinas et al., 2018), noradrenaline (Guven, 2018), endothelin-1 (Zlabinger et al., 2019), and isoproterenol (Zhang et al., 2016). These agents interfere with a known pathway that is crucial in remodeling and subsequent cardiac hypertrophy. Angiotensin II was described to play a role in cardiac hypertrophy induction over 40 years ago, as it is part of the Renin-Angiotensin-Aldosterone-System. Endothelin-1 was discovered to have a higher protein and mRNA expression in dilated cardiomyopathy and influence matrix metalloproteinase 9 (Mmp9) expression levels (Hathaway et al., 2015). Further, a significant difference in mortality and pathogenesis has been reported in patients with a gene variation of the endothelin type A receptor gene (polymorphisms G231A and C1363T) (Herrmann et al., 2001; Telgmann et al., 2007). Noradrenaline is a stress induced neurotransmitter, which has been shown to be involved in cardiac hypertrophy and damage due to toxic effects on cardiomyocytes (Jain et al., 2015). Isoproterenol is a β-agonist and activates the Renin-Angiotensin-Aldosterone-System, thus inducing hypertrophic pathways (Leenen et al., 2001). All these pharmacologic agents induce hypertrophy by binding to specific G-coupled receptors on the cell membrane, which then induce diacylglycerol (DAG) and subsequently protein kinase C (PKC). PKC plays an important role in internal Ca2+ mobilization, which allows hypertrophic induction through NFAT or calmodulin-dependent kinase (CaMK) pathway interference (Heineke and Molkentin, 2006).
Induction of hypertrophy by all of these chemical agents show a similar modeling time of 24 h, which may be extended to 48 h depending on the used cell type (Zhang et al., 2016; Zlabinger et al., 2019; Zhao et al., 2020). The concentration needs to be optimized by testing different dilutions before the experiment, however the general used concentrations are 20 µM phenylephrine (Gelinas et al., 2018), 100 nM angiotensin II (Zlabinger et al., 2019), 10 μM isoproterenol (Zhang et al., 2016), and 10 nM endothelin-1 (Loonat et al., 2019).
Establishing a cardiac hypertrophy model needs to incorporate several decisions about induction models, cell sources, pathways of interest and cell culture types. Table 1 gives a broad overview about established approaches, while also mentioning the investigated pathway of the respective study. It is evident that a preferred choice of cell source are rat cardiomyocytes, which are mostly cultured in a 2D cell culture and the induction of hypertrophy is often performed chemically with pharmacological agents such as angiotensin II or isoproterenol. These approaches are based on availability of the cell source, as rat or also murine tissue for cell extraction is more easily accessible and animal care is cheaper to maintain than with larger animals. Further, chemical induction is a relatively easy method for hypertrophy induction compared to mechanical stress induction, which presumably is why it is often preferred.
Table 1 Overview over different approaches of hypertrophy induction methods and used cell sources in in vitro models and the investigated pathway of the study.
When considering which hypertrophy model to choose for a study, various factors need to be considered beforehand. The most prominent factor in research is often time and cost effectiveness, which acts in favor of 2D chemically stimulated cultures, however this is not the best option in quality of results in some cases. The analysis of certain genes, proteins and non-coding RNAs might be insufficient in chemical stimulated cell hypertrophy models, as these do not trigger all the pathways that would be activated in vivo, which can lead to a false read-out and interpretations (Carreño et al., 2006). 3D models, such as organoids, can be stimulated mechanically and show a better comparability to in vivo conditions. These also allow to investigate the crosstalk between different cell types, especially the three main types within the heart cardiomyocytes, fibroblast, and endothelial cells, which are often not considered in regular 2D models. Fibroblasts especially play an important role in fibrosis and remodeling during hypertrophy processes and are therefore important to investigate (Travers et al., 2016). Organoids appear as the most ideal option in regard to simulating hypertrophy conditions in vivo, while still considering the principles of 3R (replacement, reduction, and refinement). Improving and also simplifying organoid technology should be the goal in translational research, so it can be used more widely for disease modeling such as cardiac hypertrophy instead of animal models.
Among some well-known and established pathways in cardiac hypertrophy new signaling cascades have been identified over the last few years, especially with the rise of non-coding RNAs. Non-coding RNAs include amongst others miRNAs, lncRNAs, and circRNA. Some have been shown to play a role in remodeling and hypertrophy induction, either in promoting or inhibiting the process (Wehbe et al., 2019). These non-coding RNAs have also been investigated as potential treatment targets in cardiac hypertrophy, which may lead to new therapeutic approach for patients in the future (Li et al., 2018).
MiRNAs are small non-coding RNAs with a length of approximately 22 nucleotides and interfere with mRNAs through complementary binding causing degradation or inhibition of transcription (Fabian and Sonenberg, 2012; Wehbe et al., 2019).
Recently, Zhang et al. showed that miRNA-29 played a significant role, as overexpression seemed to inhibit angiotensin II induced LV hypertrophy in a mouse model. Collagen I and III secretion and TGFβ and pSMAD2/3 levels were downregulated indicating that hypertrophy was indeed hindered (Zhang et al., 2020). MiRNA-26a also seems to show anti-hypertrophic effects in rat models and in cell culture, as it targets the 3’-UTR of the GATA4 mRNA (Liu et al., 2016). Similar effects on remodeling can be seen with miRNA-101, as an overexpression in a rat hypertrophy model showed reduced gene expression and protein levels of known hypertrophy related genes like Rab1a, ANF, and b-MHC and reduced relative cell areas (Wei et al., 2015). MiRNA-133 downregulation has been found to aid hypertrophy development, however restoring the expression during hypertrophy induction only reduced fibrosis and apoptosis, but not hypertrophy signaling itself (Abdellatif, 2010).
On the other hand, miRNAs can also induce or help induce hypertrophy, as shown with, e.g., miRNA-22. MiRNA-22 overexpression has been shown to be able to induce hypertrophy in vitro by modulating PTEN levels without adding any other stimulating agent and further showed to be crucial for phenylephrine and angiotensin II hypertrophy induction (Xu et al., 2012; Huang et al., 2013). MiRNA-217 similarly promotes cardiac hypertrophy interfering with PTEN levels. Additionally, catalysis of histone 3 lysine 9 dimethylation (H3K9me2) and mRNA downregulation of euchromatic histone–lysine N-methyltransferases (EHMT1/2) have been described to imitate fetal heart associated conditions further leading to hypertrophy development (Inagawa et al., 2013; Thienpont et al., 2017). MiR-155 is another player that has been identified to promote cardiac hypertrophy, by causing an inflammatory response with macrophage migration and signaling (Heymans et al., 2013). Additionally, miRNA-200c has been identified to interfere in MAPK/ERK pathway upregulation by targeting dual-specific phosphatase-1 (DUSP-1) (Singh et al., 2017).
LncRNAs can regulate promotors, enhancers and insulators by conformation changes and forming secondary structures further influencing expression of other genes. Moreover, lncRNAs can modulate miRNAs by acting as a sponge and therefore influence genes post-transcriptionally. The lncRNA myosin heavy‐chain‐associated RNA transcripts (MHRT) has been described to influence hypertrophy induction, as it antagonizes chromatin‐remodeling factor Brg1, which further activates β‐MHC. MHRT is encoded in the same gene locus (beta-cardiac muscle myosin heavy chain gene) as miRNA-208, which promotes cardiac hypertrophy (Callis et al., 2009; Han et al., 2011; Han et al., 2014). Another prominent lncRNA, identified by Wang et al. (2014a), is cardiac hypertrophy related factor (CHRF). The authors showed that CHRF was upregulated in cardiac hypertrophy and heart failure. CHRF acts as a sponge for a miRNA‐489, therefore de‐represses the myeloid differentiation primary response gene 88 (Myd88) and subsequently induces remodeling (Wang et al., 2014a). Cardiac‐apoptosis‐related lncRNA (CARL) has also been shown be strongly expressed in hypertrophy remodeling by inhibiting miR‐539 (Muthusamy et al., 2014; Wang et al., 2014b).
CircRNAs are circularized RNAs which are covalently closed. They are more stable than linear RNAs due to this structural feature and have recently gained an increasing research attention. However, they have not been as excessively studied as miRNAs and lncRNAs, leaving room for further studies on circRNAs in hypertrophy remodeling and in cardiovascular diseases in general (Hansen et al., 2013; Ottaviani and Martins, 2017; Li et al., 2018). CircRNAs can, similarly to lncRNAs, act as a sponge for miRNAs, thereby inhibiting them. CircRNA ciRS-7/CDR1as has been identified as a sponge for miR-7, which has been shown to be upregulated in patients with left ventricular hypertrophy (Hansen et al., 2013; Kaneto et al., 2017). The heart-related circRNA (HRCR) has also been found to inhibit miRNA-233 in the same way, therefore de-repressing the activity-regulated cytoskeleton-associated protein (K. Wang et al., 2016).
CircRNAs and LncRNAs provide a new approach for treatment due to their sponging properties and they may present a new biomarker for predicting the severity of the remodeling process and in heart failure. However, some challenges for non-coding RNAs as therapeutic option must still be faced, including off-target effects and delivery (Dong et al., 2018).
Cardiac hypertrophy will remain an ever-present clinical challenge due to several widespread risk factors in an increasingly aging patient population. Advanced and refined tissue engineering approaches give an opportunity to study pathological pathways extensively without requiring in vivo animal studies, which are more costly, time- and labor-intensive. These approaches allow the identification of possible biomarkers and the testing of therapies in a setting that mimics in vivo conditions more closely, although not perfectly as some factors like immune interactions are still lacking. The induction of cardiac hypertrophy in vitro is predominantly performed with pharmacological agents, such as angiotensin II, as mechanical stress induction poses a greater challenge.
Several well-known pathways which are involved in hypertrophic remodeling have been identified, including newer additions mostly revolving around non-coding RNAs like miRNAs, lncRNAs, and circRNAs. Non-coding RNAs that aid or negatively interfere with cardiac hypertrophy have been identified by inhibiting other factors (supporting or inhibitory) in the hypertrophy signaling cascades. There have been dozens of studies on a great number of different factors involved in this complex pathological process. Further, also epigenetic modifications have been discovered to be regulated and influenced to some degree by non-coding RNAs, which are involved in cardiac hypertrophy (Dong et al., 2018). However, it is important to further investigate and develop even more advanced tissue engineering options to allow a more profound understanding of remodeling pathways and subsequently the development of new pharmacological targets and treatment approaches.
NK and KZ wrote the main body of the manuscript. AS, JM-T, EH, and DT assisted with literature research and comments regarding language and style. AS and MG revised the manuscript and assisted with structural conceptualization.
The authors declare that the research was conducted in the absence of any commercial or financial relationships that could be construed as a potential conflict of interest.
Abdellatif, M. (2010). The Role of MicroRNA-133 in Cardiac Hypertrophy Uncovered. Circ. Res. 106 (1), 16–18. doi: 10.1161/CIRCRESAHA.109.212183
Benjamin, E. J., Virani, S. S., Callaway, C. W., Chamberlain, A. M., Chang, A. R., Cheng, S., et al. (2018). Heart Disease and Stroke Statistics-2018 Update: A Report From the American Heart Association. Circulation 137 (12), e67–492. doi: 10.1161/CIR.0000000000000558
Callis, T. E., Pandya, K., Seok, H. Y., Tang, R.-H., Tatsuguchi, M., Huang, Z.-P., et al. (2009). MicroRNA-208a Is a Regulator of Cardiac Hypertrophy and Conduction in Mice. J. Clin. Invest. 119 (9), 2772–2786. doi: 10.1172/JCI36154
Carreño, J. E., Apablaza, F., Ocaranza, M. P., Jalil, J. E. (2006). Cardiac hypertrophy: molecular and cellular events. Rev. Espanola Cardiol. 59 (5), 473–486. doi: 10.1016/S1885-5857(06)60796-2
Cramariuc, D., Gerdts, E. (2016). Epidemiology of Left Ventricular Hypertrophy in Hypertension: Implications for the Clinic. Expert Rev. Cardiovasc. Ther. 14 (8), 915–926. doi: 10.1080/14779072.2016.1186542
Dhanoa, J. K., Sethi, R. S., Verma, R., Arora, J. S., Mukhopadhyay, C. S. (2018). Long Non-Coding RNA: Its Evolutionary Relics and Biological Implications in Mammals: A Review. J. Anim. Sci. Technol. 60, 25. doi: 10.1186/s40781-018-0183-7
Dong, Y., Xu, S., Liu, J., Ponnusamy, M., Zhao, Y., Zhang, Y., et al. (2018). Non-Coding RNA-Linked Epigenetic Regulation in Cardiac Hypertrophy. Int. J. Biol. Sci. 14 (9), 1133–1141. doi: 10.7150/ijbs.26215
Dutta, D., Heo, I., Clevers, H. (2017). Disease Modeling in Stem Cell-Derived 3D Organoid Systems. Trends Mol. Med. 23 (5), 393–410. doi: 10.1016/j.molmed.2017.02.007
Fabian, M. R., Sonenberg, N. (2012). The Mechanics of MiRNA-Mediated Gene Silencing: A Look under the Hood of MiRISC. Nat. Struct. Mol. Biol. 19 (6), 586–593. doi: 10.1038/nsmb.2296
Gelinas, R., Mailleux, F., Dontaine, J., Bultot, L., Demeulder, B., Ginion, A., et al. (2018). AMPK Activation Counteracts Cardiac Hypertrophy by Reducing O-GlcNAcylation. Nat. Commun. 9 (1), 374. doi: 10.1038/s41467-017-02795-4
Ginis, I., Luo, Y., Miura, T., Thies, S., Brandenberger, R., Gerecht-Nir, S., et al. (2004). Differences between Human and Mouse Embryonic Stem Cells. Dev. Biol. 269 (2), 360–380. doi: 10.1016/j.ydbio.2003.12.034
Guven, C. (2018). The Effect of Diazoxide on Norepinephrine-Induced Cardiac Hypertrophy, in Vitro. Cell. Mol. Biol. (Noisy-Le-Grand France) 64 (10), 50–54. doi: 10.14715/cmb/2018.64.10.8
Han, P., Hang, C. T., Yang, J., Chang, C.-P. (2011). Chromatin Remodeling in Cardiovascular Development and Physiology. Circ. Res. 108 (3), 378–396. doi: 10.1161/CIRCRESAHA.110.224287
Han, P., Li, W., Lin, C.-H., Yang, J., Shang, C., Nuernberg, S. T., et al. (2014). A Long Noncoding RNA Protects the Heart from Pathological Hypertrophy. Nature 514 (7520), 102–106. doi: 10.1038/nature13596
Hansen, T. B., Jensen, T., II, Clausen, B. H., Bramsen, J. B., Finsen, B., Damgaard, C. K., et al. (2013). Natural RNA Circles Function as Efficient MicroRNA Sponges. Nature 495 (7441), 384–388. doi: 10.1038/nature11993
Hathaway, C. K., Grant, R., Hagaman, J. R., Hiller, S., Li, F., Xu, L., et al. (2015). Endothelin-1 Critically Influences Cardiac Function via Superoxide-MMP9 Cascade. Proc. Natl. Acad. Sci. U. S. A. 112 (16), 5141–5146. doi: 10.1073/pnas.1504557112
Heineke, J., Molkentin, J. D. (2006). Regulation of Cardiac Hypertrophy by Intracellular Signalling Pathways. Nat. Rev. Mol. Cell Biol. 7 (8), 589–600. doi: 10.1038/nrm1983
Herrmann, S., Schmidt-Petersen, K., Pfeifer, J., Perrot, A., Bit-Avragim, N., Eichhorn, C., et al. (2001). A Polymorphism in the Endothelin-A Receptor Gene Predicts Survival in Patients with Idiopathic Dilated Cardiomyopathy. Eur. Heart J. 22 (20), 1948–1953. doi: 10.1053/euhj.2001.2626
Heymans, S., Corsten, M. F., Verhesen, W., Carai, P., van Leeuwen, R. E. W., Custers, K., et al. (2013). Macrophage MicroRNA-155 Promotes Cardiac Hypertrophy and Failure. Circulation 128 (13), 1420–1432. doi: 10.1161/CIRCULATIONAHA.112.001357
Huang, Z.-P., Chen, J., Seok, H. Y., Zhang, Z., Kataoka, M., Hu, X., et al. (2013). MicroRNA-22 Regulates Cardiac Hypertrophy and Remodeling in Response to Stress. Circ. Res. 112 (9), 1234–1243. doi: 10.1161/CIRCRESAHA.112.300682
Iakobachvili, N., Peters, P. J. (2017). Humans in a Dish: The Potential of Organoids in Modeling Immunity and Infectious Diseases. Front. Microbiol. 8, 2402. doi: 10.3389/fmicb.2017.02402
Inagawa, M., Nakajima, K., Makino, T., Ogawa, S., Kojima, M., Ito, S., et al. (2013). Histone H3 Lysine 9 Methyltransferases, G9a and GLP Are Essential for Cardiac Morphogenesis. Mech. Dev. 130 (11–12), 519–531. doi: 10.1016/j.mod.2013.07.002
Jaé, N., Dimmeler, S. (2020). Noncoding RNAs in Vascular Diseases. Circ. Res. 126 (9), 1127–1145. doi: 10.1161/CIRCRESAHA.119.315938
Jain, A., Atale, N., Kohli, S., Bhattacharya, S., Sharma, M., Rani, V. (2015). An Assessment of Norepinephrine Mediated Hypertrophy to Apoptosis Transition in Cardiac Cells: A Signal for Cell Death. Chem. Biol. Interact. 225, 54–62. doi: 10.1016/j.cbi.2014.11.017
Jain, A., Hasan, J., Desingu, P. A., Sundaresan, N. R., Chatterjee, K. (2018). Engineering an in Vitro Organotypic Model for Studying Cardiac Hypertrophy. Colloids Surf. B. Biointerf. 165, 355–362. doi: 10.1016/j.colsurfb.2018.02.036
Jiang, J., Lan, C., Li, L., Yang, D., Xia, X., Liao, Q., et al. (2018). A Novel Porcupine Inhibitor Blocks WNT Pathways and Attenuates Cardiac Hypertrophy. Biochim. Biophys. Acta Mol. Basis Dis. 1864 (10), 3459–3467. doi: 10.1016/j.bbadis.2018.07.035
Jochmans-Lemoine, A., Villalpando, G., Gonzales, M., Valverde, I., Soria, R., Joseph, V. (2015). Divergent Physiological Responses in Laboratory Rats and Mice Raised at High Altitude. J. Exp. Biol. 218 (Pt 7), 1035–1043. doi: 10.1242/jeb.112862
Kaneto, C. M., Nascimento, J. S., Moreira, M. C. R., Ludovico, N. D., Santana, A. P., Silva, R. A. A., et al. (2017). MicroRNA Profiling Identifies MiR-7-5p and MiR-26b-5p as Differentially Expressed in Hypertensive Patients with Left Ventricular Hypertrophy. Braz. J. Med. Biol. Res. 50 (12), e6211. doi: 10.1590/1414-431X20176211
Leenen, F. H., White, R., Yuan, B. (2001). Isoproterenol-Induced Cardiac Hypertrophy: Role of Circulatory versus Cardiac Renin-Angiotensin System. Am. J. Physiol. Heart Circulatory Physiol. 281 (6), H2410–H2416. doi: 10.1152/ajpheart.2001.281.6.H2410
Li, Y., Liang, Y., Zhu, Y., Zhang, Y., Bei, Y. (2018). Noncoding RNAs in Cardiac Hypertrophy. J. Cardiovasc. Trans. Res. 11 (6), 439–449. doi: 10.1007/s12265-018-9797-x
Liu, Y., Wang, Z., Xiao, W. (2016). MicroRNA-26a Protects against Cardiac Hypertrophy via Inhibiting GATA4 in Rat Model and Cultured Cardiomyocytes. Mol. Med. Rep. 14 (3), 2860–2866. doi: 10.3892/mmr.2016.5574
Loonat, A. A., Curtis, M.K., Richards, M. A., Nunez-Alonso, G., Michl, J., Swietach, P. (2019). A High-Throughput Ratiometric Method for Imaging Hypertrophic Growth in Cultured Primary Cardiac Myocytes. J. Mol. Cell. Cardiol. 130, 184–196. doi: 10.1016/j.yjmcc.2019.04.001
Muthusamy, S., DeMartino, A. M., Watson, L. J., Brittian, K. R., Zafir, A., Dassanayaka, S., et al. (2014). MicroRNA-539 Is up-Regulated in Failing Heart, and Suppresses O-GlcNAcase Expression. J. Biol. Chem. 289 (43), 29665–29676. doi: 10.1074/jbc.M114.578682
Nakamura, M., Sadoshima, J. (2018). Mechanisms of Physiological and Pathological Cardiac Hypertrophy. Nat. Rev. Cardiol. 15 (7), 387–407. doi: 10.1038/s41569-018-0007-y
Nugraha, B., Buono, M. F., von Boehmer, L., Hoerstrup, S. P., Emmert, M. Y. (2019). Human Cardiac Organoids for Disease Modeling. Clin. Pharmacol. Ther. 105 (1), 79–85. doi: 10.1002/cpt.1286
O’Brien, J., Hayder, H., Zayed, Y., Peng, C. (2018). Overview of MicroRNA Biogenesis, Mechanisms of Actions, and Circulation. Front. Endocrinol. 9, 402. doi: 10.3389/fendo.2018.00402
Ottaviani, L., Martins, P. A. C. (2017). Non-Coding RNAs in Cardiac Hypertrophy. J. Physiol. 595 (12), 4037–4050. doi: 10.1113/JP273129
Porrello, E. R., Mahmoud, A., II, Simpson, E., Johnson, B. A., Grinsfelder, D., Canseco, D., et al. (2013). Regulation of Neonatal and Adult Mammalian Heart Regeneration by the MiR-15 Family. Proc. Natl. Acad. Sci. U. S. A. 110 (1), 187–192. doi: 10.1073/pnas.1208863110
Rysä, J., Tokola, H., Ruskoaho, H. (2018). Mechanical Stretch Induced Transcriptomic Profiles in Cardiac Myocytes. Sci. Rep. 8 (1), 4733. doi: 10.1038/s41598-018-23042-w
Singh, G. B., Raut, S. K., Khanna, S., Kumar, A., Sharma, S., Prasad, R., et al. (2017). MicroRNA-200c Modulates DUSP-1 Expression in Diabetes-Induced Cardiac Hypertrophy. Mol. Cell. Biochem. 424 (1–2), 1–11. doi: 10.1007/s11010-016-2838-3
Spannbauer, A., Traxler, D., Zlabinger, K., Gugerell, A., Winkler, J., Mester-Tonczar, J., et al. (2019). Large Animal Models of Heart Failure With Reduced Ejection Fraction (HFrEF). Front. Cardiovasc. Med. 6, 117. doi: 10.3389/fcvm.2019.00117
Stansfield, W. E., Ranek, M., Pendse, A., Schisler, J. C., Wang, S., Pulinilkunnil, T. (2014). “Chapter 4—The Pathophysiology of Cardiac Hypertrophy and Heart Failure,” in Cellular and Molecular Pathobiology of Cardiovascular Disease. Eds. Willis, M. S., Homeister, J. W., Stone, J. R. (San Diego, CA, USA: Academic Press), 51–78. doi: 10.1016/B978-0-12-405206-2.00004-1
Telgmann, R., Harb, B. A., Ozcelik, C., Perrot, A., Schönfelder, J., Nonnenmacher, A., et al. (2007). The G-231A Polymorphism in the Endothelin-A Receptor Gene Is Associated with Lower Aortic Pressure in Patients with Dilated Cardiomyopathy. Am. J. Hypertension 20 (1), 32–37. doi: 10.1016/j.amjhyper.2006.06.016
Tham, Y. K., Bernardo, B. C., Ooi, J. Y.Y., Weeks, K. L., McMullen, J. R. (2015). Pathophysiology of Cardiac Hypertrophy and Heart Failure: Signaling Pathways and Novel Therapeutic Targets. Arch. Toxicol. 89 (9), 1401–1438. doi: 10.1007/s00204-015-1477-x
Thienpont, B., Aronsen, J. M., Robinson, E. L., Okkenhaug, H., Loche, E., Ferrini, A., et al. (2017). The H3K9 Dimethyltransferases EHMT1/2 Protect against Pathological Cardiac Hypertrophy. J. Clin. Invest. 127 (1), 335–348. doi: 10.1172/JCI88353
Travers, J. G., Kamal, F. A., Robbins, J., Yutzey, K. E., Blaxall, B. C. (2016). Cardiac Fibrosis: The Fibroblast Awakens. Circ. Res. 118 (6), 1021–1040. doi: 10.1161/CIRCRESAHA.115.306565
Wang, K., Liu, F., Zhou, L.-Y., Long, B., Yuan, S.-M., Wang, Y., et al. (2014a). The Long Noncoding RNA CHRF Regulates Cardiac Hypertrophy by Targeting MiR-489. Circ. Res. 114 (9), 1377–1388. doi: 10.1161/CIRCRESAHA.114.302476
Wang, K., Long, B., Zhou, L.-Y., Liu, F., Zhou, Q.-Y., Liu, C.-Y., et al. (2014b). CARL LncRNA Inhibits Anoxia-Induced Mitochondrial Fission and Apoptosis in Cardiomyocytes by Impairing MiR-539-Dependent PHB2 Downregulation. Nat. Commun. 5, 3596. doi: 10.1038/ncomms4596
Wang, K., Long, B., Liu, F., Wang, J.-X., Liu, C.-Y., Zhao, B., et al. (2016). A Circular RNA Protects the Heart from Pathological Hypertrophy and Heart Failure by Targeting MiR-223. Eur. Heart J. 37 (33), 2602–2611. doi: 10.1093/eurheartj/ehv713
Wang, L., Kryshtal, D. O., Kim, K., Parikh, S., Cadar, A. G., Bersell, K. R., et al (2017). Myofilament Calcium-Buffering Dependent Action Potential Triangulation in Human-Induced Pluripotent Stem Cell Model of Hypertrophic Cardiomyopathy. J. Am. Coll. Cardiol. U. S. 70 (20), 2600–2602. doi: 10.1016/j.jacc.2017.09.033
Wehbe, N., Nasser, S. A., Pintus, G., Badran, A., Eid, A. H., Baydoun, E. (2019). MicroRNAs in Cardiac Hypertrophy. Int. J. Mol. Sci. 20 (19), 4714. doi: 10.3390/ijms20194714
Wei, L., Yuan, M., Zhou, R., Bai, Q., Zhang, W., Zhang, M., et al. (2015). MicroRNA-101 Inhibits Rat Cardiac Hypertrophy by Targeting Rab1a. J. Cardiovasc. Pharmacol. 65 (4), 357–363. doi: 10.1097/FJC.0000000000000203
Xu, X.-D., Song, X.-W., Li, Q., Wang, G.-K., Jing, Q., Qin, Y.-W. (2012). Attenuation of MicroRNA-22 Derepressed PTEN to Effectively Protect Rat Cardiomyocytes from Hypertrophy. J. Cell. Physiol. 227 (4), 1391–1398. doi: 10.1002/jcp.22852
Yu, C.-Y., Kuo, H.-C. (2019). The Emerging Roles and Functions of Circular RNAs and Their Generation. J. Biomed. Sci. 26 (1):29. doi: 10.1186/s12929-019-0523-z
Zhang, Y., Xu, J., Long, Z., Wang, C., Wang, L., Sun, P., et al. (2016). Hydrogen (H(2)) Inhibits Isoproterenol-Induced Cardiac Hypertrophy via Antioxidative Pathways. Front. Pharmacol. 7:392:392. doi: 10.3389/fphar.2016.00392
Zhang, S.-J., Yun, C.-J., Liu, J., Yao, S.-Y., Li, Y., Wang, M., et al. (2020). MicroRNA-29a Attenuates Angiotensin-II Induced-Left Ventricular Remodeling by Inhibiting Collagen, TGF-β and SMAD2/3 Expression. J. Geriatric Cardiol. 17 (2), 96–104. doi: 10.11909/j.issn.1671-5411.2020.02.008
Zhao, D., Gao, Y., Wei, W., Pei, H., Xu, C., Zhao, Z. (2020). PKD Deletion Promotes Autophagy and Inhibits Hypertrophy in Cardiomyocyte. Exp. Cell Res. 386 (2):111742. doi: 10.1016/j.yexcr.2019.111742
Zlabinger, K., Spannbauer, A., Traxler, D., Gugerell, A., Lukovic, D., Winkler, J., et al. (2019). MiR-21, MiR-29a, GATA4, and MEF2c Expression Changes in Endothelin-1 and Angiotensin II Cardiac Hypertrophy Stimulated Isl-1(+)Sca-1(+)c-Kit(+) Porcine Cardiac Progenitor Cells In Vitro. Cells 8 (11), 1416. doi: 10.3390/cells8111416
Zuppinger, C. (2016). 3D Culture for Cardiac Cells. Biochim. Biophys. Acta (BBA) 1863 (7, Part B), 1873–1881. doi: 10.1016/j.bbamcr.2015.11.036
Keywords: cardiac hypertrophy, disease modeling, hypertrophy induction, non-coding RNA, tissue engineering
Citation: Kastner N, Zlabinger K, Spannbauer A, Traxler D, Mester-Tonczar J, Hašimbegović E and Gyöngyösi M (2020) New Insights and Current Approaches in Cardiac Hypertrophy Cell Culture, Tissue Engineering Models, and Novel Pathways Involving Non-Coding RNA. Front. Pharmacol. 11:1314. doi: 10.3389/fphar.2020.01314
Received: 04 June 2020; Accepted: 07 August 2020;
Published: 21 August 2020.
Edited by:
Hai-Gang Zhang, Army Medical University, ChinaReviewed by:
Yong Zhang, Harbin Medical University, ChinaCopyright © 2020 Kastner, Zlabinger, Spannbauer, Traxler, Mester-Tonczar, Hašimbegović and Gyöngyösi. This is an open-access article distributed under the terms of the Creative Commons Attribution License (CC BY). The use, distribution or reproduction in other forums is permitted, provided the original author(s) and the copyright owner(s) are credited and that the original publication in this journal is cited, in accordance with accepted academic practice. No use, distribution or reproduction is permitted which does not comply with these terms.
*Correspondence: Mariann Gyöngyösi, bWFyaWFubi5neW9uZ3lvc2lAbWVkdW5pd2llbi5hYy5hdA==
†These authors have contributed equally to this work
Disclaimer: All claims expressed in this article are solely those of the authors and do not necessarily represent those of their affiliated organizations, or those of the publisher, the editors and the reviewers. Any product that may be evaluated in this article or claim that may be made by its manufacturer is not guaranteed or endorsed by the publisher.
Research integrity at Frontiers
Learn more about the work of our research integrity team to safeguard the quality of each article we publish.