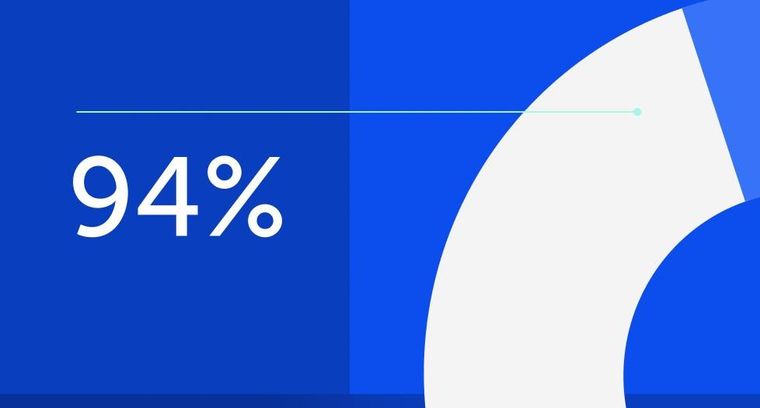
94% of researchers rate our articles as excellent or good
Learn more about the work of our research integrity team to safeguard the quality of each article we publish.
Find out more
REVIEW article
Front. Pharmacol., 23 July 2020
Sec. Pharmacology of Ion Channels and Channelopathies
Volume 11 - 2020 | https://doi.org/10.3389/fphar.2020.01111
This article is part of the Research TopicIon Channel Signalling in Cancer: From Molecular Mechanisms to TherapeuticsView all 17 articles
Voltage-gated sodium channels (VGSCs), which are abnormally expressed in various types of cancers such as breast cancer, prostate cancer, lung cancer, and cervical cancer, are involved in the metastatic process of invasion and migration. Nav1.5 is a pore-forming α subunit of VGSC encoded by SCN5A. Various studies have demonstrated that Nav1.5, often as its neonatal splice form, is highly expressed in metastatic breast cancer cells. Abnormal activation and expression of Nav1.5 trigger a variety of cellular mechanisms, including changing H+ efflux, promoting epithelial-to-mesenchymal transition (EMT) and the expression of cysteine cathepsin, to potentiate the metastasis and invasiveness of breast cancer cells in vitro and in vivo. Here, we systematically review the latest available data on the pro-metastatic effect of Nav1.5 and its underlying mechanisms in breast cancer. We summarize the factors affecting Nav1.5 expression in breast cancer cells, and discuss the potential of Nav1.5 blockers serving as candidates for breast cancer treatment.
Breast cancer accounts for the largest proportion of women’s mortality worldwide (Greaney et al., 2015). In the United States, approximately 12% of women will develop invasive breast cancer during their lifetime. The diagnosis of at least 276,480 new cases of invasive breast cancer and 42,170 breast cancer associated deaths are estimated in 2020 (Breastcancer.org). It is a complicated disease with inter- and intra-tumoral heterogeneity, and there are several different classifications of breast cancer according to advanced technical methods, including histological stratification and gene expression clustering (Sorlie et al., 2001; Margan et al., 2016; Yeo and Guan, 2017). The different molecular types of breast cancer correspond to different molecular mechanisms and require specific therapeutic strategies (Prat et al., 2015; Arciero et al., 2019). Therefore, appropriate management and therapies are necessary to achieve the best response to improve the prognosis and survival of patients. Notably, breast cancer cells have the potential to metastasize to distant organs such as the brain, lung, liver, and bone (Hoshino et al., 2015; Hurvitz et al., 2018; Park et al., 2018; Zhu et al., 2019). Cancer metastasis is a complex, multi-step process that is closely associated with cells’ local invasion, blood and lymphatic diffusion, and extravasation and colonization at distant sites. Metastatic cancer cells degrade the extracellular matrix, detach from their original location, and enter the circulation system through which they reach and colonize distant organs (Mego et al., 2010; Massague et al., 2017). Metastasis is the direct cause of death from cancer, accounting for nearly 90% of deaths related to breast cancer (Xie et al., 2017). Thus, there is an urgent need to identify the underlying molecular mechanisms of breast cancer and develop effective therapeutic strategies.
Voltage-gated sodium channels (VGSCs) are primarily expressed in excitable cells such as neurons. Na+ influx regulated by VGSCs produces action potential and propagates excitability. VGSCs also exist in non-excitable cells, including microglia, astrocytes, immune cells, fibroblasts, and cancer cells, where they regulate and influence an array of biological functions such as phagocytosis, motility, Na+/K+-ATPase activity, and metastatic activity (Black and Waxman, 2013). VGSCs are composed of pore-forming α subunits and auxiliary β subunits (Hull and Isom, 2018). The family Nav1 comprises nine genes (SCN1A-5A, SCN8A-11A) that encode α subunits, Nav1.1 to Nav1.9. Each α subunit comprises four homologous domains, and each domain consists of six transmembrane segments (Catterall, 2000). Five β subunits, including β1 and its splice variants β1B and β2–β4, are encoded by four genes (SCN1B-SCN4B) (Brackenbury and Isom, 2011). These β subunits have been found to modulate the bioelectrical characteristics of α subunits and function as cellular adhesion molecules. Both α and β subunits play a critical role in the development of the central nervous system (CNS), and altering the expression of specific subunits may cause developmental aberrations and CNS lesions. Furthermore, expression of α and β subunits is upregulated in various cancers such as breast cancer, prostate cancer, lung cancer, and cervical cancer, observed both in in vitro and in vivo systems. They are found to enhance metastasis progression, including invasion, migration, endocytosis, and gene expression, via the Na+ currents carried by α subunits and the adhesion interaction regulated by β subunits (Yang et al., 2012). The correlation between VGSCs-inhibiting drug uses and outcomes of cancer patients has been highlighted recently (Fairhurst et al., 2015; Reddy et al., 2015; Kao et al., 2018), even though the conclusions are still inconsistent. Hence, randomized controlled trials are required to exclude confounding factors and obtain a convincing conclusion in the future.
Nav1.5, a pore-forming α subunit of VGSC encoded by SCN5A, is expressed in lymphoma, neuroblastoma, breast and prostate cancer cells. Aberrant expression and activity of Nav1.5 are associated with cardiac excitability diseases such as arrhythmic dilated cardiomyopathy, Brugada syndrome, and long QT syndrome (Antzelevitch et al., 2005; Amin et al., 2013; Giudicessi and Ackerman, 2013; Beckermann et al., 2014). Nav1.5 is also overexpressed in metastatic breast cancer in vitro and in vivo and mainly exists in its DI: S3 5′ neonatal splice form (nNav1.5) in breast cancer cells, potentiating cell metastasis (Mohammed et al., 2016; Yamaci et al., 2017). Breast cancer cells treated with specific Nav1.5 inhibitors or siRNAs show decreased motility and metastatic capacity (Driffort et al., 2014).Therefore, Nav1.5 may be regarded as a promising target for the diagnosis and therapy of breast cancer.
With the high motility and metastatic capacity of breast cancer, it is imperative to determine the mechanisms of pro-metastatic effects of Nav1.5 and develop effective Nav1.5 inhibitors for breast cancer treatment. This review clarifies the role and mechanisms of Nav1.5 in metastatic breast cancer progression and summarizes some drugs with remarkable effects on reducing metastasis of breast cancer by acting on Nav1.5. All these evidence supports the idea that Nav1.5 as an anti-metastatic target for the treatment of metastatic breast cancer.
Nav1.5 in its neonatal DI:S3 5′ splice form is predominantly expressed in metastatic cancer cells (Fraser et al., 2005; Yamaci et al., 2017). This form has been found to participate in neonatal development, while it is absent in postnatal development. The overexpression of Nav1.5 in cancer cells suggests that embryonic genes are re-expressed during ontogenesis and participate in many cellular behaviors related to metastasis (Monk and Holding, 2001).
The expression levels of Nav1.5 and nNav1.5 in the highly metastatic MDA-MB-231 breast cancer cell line were significantly higher than those in weakly metastatic MCF-7 cells (Kamarulzaman et al., 2017; Zhang et al., 2018). Nav1.5 is specifically present on the membrane of MDA-MB-231 cells, but not in normal cell lines and weakly metastatic MCF-7 cells. In breast cancers, Nav1.5 α subunit mRNA and protein expression correlates with metastatic potential, and the neonatal SCN5A splice variant is expressed ~1,800-fold higher in metastatic MDA-MB-231 cells than in weakly metastatic MCF-7 cells. When voltage-gated membrane currents are examined in different cell lines, inward currents only occur in the highly metastatic breast cancer cell line MDA-MB-231 (Fraser et al., 2005). In vivo, it is widely acknowledged that Nav1.5 is present in breast cancer biopsies and is related to lymph node metastasis (Fraser et al., 2005). SCN5A expression is significantly elevated in breast cancer tissues and is an independent predictor of poor prognosis compared to its expression in normal breast tissue. SCN5A is overexpressed in tumor samples from patients who experience recurrence and death within 5 years; thus, SCN5A overexpression is associated with increased odds of developing metastasis (Yang et al., 2012). Nelson and his colleagues investigated the functional activity of Nav1.5 and its specific contribution to breast cancer tumor progression. SCN5A is upregulated at the mRNA and protein levels in metastatic breast tumors compared to that in normal, non-cancerous tissue (Nelson et al., 2015a; Nelson et al., 2015b; Yamaci et al., 2017)
Furthermore, some factors affect Nav1.5 expression in breast cancer cells. The β1 subunit mRNA and protein are strongly expressed in MCF-7 cells and are barely detectable in MDA-MB-231 cells. Inhibition of the β1 subunit reduces adhesion and enhances metastatic cell behavior by upregulating nNav1.5 expression (Chioni et al., 2009). It has been reported that the expression level of repressor element silencing transcription factor (REST) is significantly lower in MDA-MB-231 cells than in MCF-7 cells (Kamarulzaman et al., 2017). The inhibition of REST results in re-expression of various neonatal genes (Kuwahara, 2013), and REST recruits histone deacetylases (HDACs) for transcriptional repression activity (Roopra et al., 2000). It has been postulated that downregulation of REST and HDAC2 expression levels enhances the expression of Nav1.5 and nNav1.5, promoting aggressiveness of tumors (Kamarulzaman et al., 2017). The sigma-1 receptor is known as a protein located on the plasma membrane, endoplasmic reticulum, and perinuclear areas (Hayashi and Su, 2003; Hayashi and Su, 2005), and is up-regulated in breast cancer cells and tissues (Aydar et al., 2016). In breast cancer cells, the sigma-1 receptor combines with nNav1.5 protein to form a complex with a four-fold symmetry (Balasuriya et al., 2012), which translocate nNav1.5 protein to the plasma membrane, thereby increases the metastatic activity (Aydar et al., 2016). Salt-inducible kinase 1 (SIK1) has been identified as an important factor in regulating sodium homeostasis and as a tumor repressor that participates in the progression of cancer cells (Sjostrom et al., 2007; Selvik et al., 2014). Lower expression of SIK1 was observed in different breast tumor grades than in normal tissues (Gradek et al., 2019). Silencing SIK1 upregulates SCN5A expression and increases H+ outflow, thus, improving invasiveness (Gradek et al., 2019). TGF-β1 is a well-known inducer of epithelial-to-mesenchymal transition (EMT) and a multifunctional regulatory factor affecting cancer development (Jakowlew, 2006; Moustakas and Heldin, 2016). Weakly metastatic MCF-7 cells treated with TGF-β1 demonstrated increased expression of SCN5A and induction of invasion (Gradek et al., 2019). Epidermal growth factor (EGF) signals have been confirmed to be overexpressed and are involved in the development of breast cancer (Adams et al., 2009; Yao et al., 2012). Treatment with EGF promoted the migratory capacity of MDA-MB-231 cells by increasing the functional expression of the Nav1.5 channel (Gonzalez-Gonzalez et al., 2019). These results confirm that Nav1.5 channels play an important role in human breast cancer by affecting metastatic activity.
Furthermore, the importance of Nav1.5 expression in human breast cancer cells for the colonization of organs was assessed. ShCTL or shNav1.5 breast cancer cells injected into mice. ShCTL cells expressing Nav1.5 strongly colonized and invaded the chest area. All mice showed lung colonization and some showed rachis and rib colonization (Driffort et al., 2014). In addition, a high level of nNav1.5 expression is associated with the estrogen receptor (ERα) status of breast cancer. In all cases, lack of ERα was positive for nNav1.5 expression. In all cases of negative nNav1.5 expression, ERα is present (Yamaci et al., 2017). It is widely acknowledged that breast cancer cases lacking ERα protein are closely related to a more advanced stage and have a worse prognosis (Yi et al., 2014; Zhou and Slingerland, 2014). This is consistent with previous results showing that a high level of nNav1.5 expression is associated with aggressive breast cancer development. Consequently, nNav1.5 expression enhances growth and metastatic dissemination of breast cancer and is a potential prognostic marker for breast cancer.
Metastasis of breast cancer is a complex process, and there are a considerable number of studies focusing on this topic. Nav1.5 is involved in the metastatic cascade in breast cancer by acting on different targets.
Na+ currents carried by Nav1.5 have been found to promote the invasiveness of the breast cancer cell line MDA-MB-231 by regulating the H+ influx carried through Na+/H+ exchanger isoform-1 (NHE-1). NHE-1, which is an important regulator of H+ efflux, is co-expressed with Nav1.5 in lipid rafts within the caveolae of MDA-MB-231 cells. NHE-1 and Nav1.5 are coupled in the metastatic process of MDA-MB-231 cells (Brisson et al., 2011). Na+ inward currents can be found in highly metastatic MDA-MB-231 cells but are absent in weakly metastatic or normal cells. Similarly, highly metastatic MDA-MB-231 cells express a high level of NHE-1 mRNA, while weakly metastatic MCF-7 cells express a low level of NHE-1 mRNA, and normal cells express no NHE-1 (Chen et al., 2019). In MDA-MB-231 cells, Nav1.5 allosterically modulates the activity of NHE-1, rendering NHE-1 more active at physiological intracellular pH. This results in increased H+ efflux to the extracellular space, leading to acidification of the peri-membrane and intracellular alkalization (Brisson et al., 2013; Amith and Fliegel, 2017). The environment of the perimembrane favors the proteolytic activity of cysteine cathepsins (Cat) and matrix metalloproteinases (MMPs), which are major drivers of extracellular matrix (ECM) degradation and increase the invasiveness of breast cancer cells (Figure 1). Cat C, Cat B, Cat K, and Cat L are involved in Cat-dependent invasiveness in MDA-MB-231 cells. Apart from Cat K, the activity of this proteolytic protein can be potentiated by Nav1.5 (Gillet et al., 2009; Robey and Nesbit, 2013). NHE-1–modulated invasiveness can also be found in hepatoma cells (Yang et al., 2011).
Figure 1 Nav1.5 promotes the activity of NHE-1, resulting in the acidification of the peri-membrane, which favors the proteolytic activity of Cat and MMPs (yellow line). Nav1.5 also promotes invasiveness by the CD44-src-cortactin signaling pathway. After phosphorylation, cortactin releases actin-severing cofilin, promoting the formation of actin barbed ends of invadopodia (red line). Overexpression of CD44 upregulates the expression and activity of NHE-1, resulting in the expression level of MMPs (MMP2, MMP9, and MMP14) by increasing phosphorylated ERK1/2 (black line). Nav1.5 dependent Vm depolarization regulates Rac1 activation by its interaction with phosphatidylserine (blue solid line). Rac1 regulates the Arp2/3 complex and is closely associated with phosphorylation of cortactin and cofilin, promoting the promigratory phenotype (blue dotted line). High expression of NaV1.5 induces expression of SNAI1, triggering the EMT and pro-metastatic effect of breast cancer cells (purple line). (Dotted line indicates that the mechanism exists but has not been confirmed in breast cancer).
Nav1.5 expression enhances src kinase activity and controls cortactin phosphorylation, resulting in the release of actin-severing cofilin and the formation of actin barbed ends of invadopodias, which are actin-rich organelles that enable cells to stretch into the ECM and perform their proteolytic effect (Oser et al., 2009; Brisson et al., 2013). It is known that Nav1.5 expression in breast cancer cells is closely associated with the protein level of CD44, which correlates with poor outcomes of breast cancer (McFarlane et al., 2015; Nelson et al., 2015b). In turn, CD44 adheres to its ligand and leads to the activation of src and phosphorylation of cortactin (Bourguignon et al., 2001; Hill et al., 2006), thus, supporting that Nav1.5 may potentiate the invasion of breast cancer cells by the CD44-src-cortactin signaling axis. Apart from the CD44-src-cortactin signaling axis, CD44 targets NHE-1 to regulate the metastatic capacities of MDA-MB-231 cells through the mitogen-activated protein kinase pathway. Overexpression of CD44 upregulates the expression and activity of NHE-1, resulting in the increased expression of MMPs (MMP2, MMP9, and MMP14) by increasing phosphorylated ERK1/2 (Figure 1). Moreover, CD44 accelerates tumor growth and metastasis to the lung in MDA-MB-231 cells (Chang et al., 2014).
Increasing evidence shows that plasma membrane potential (Vm) is related to cell cycle progression and the level of differentiation, development, regeneration, and migration of cancer cells (Sundelacruz et al., 2009; Yang and Brackenbury, 2013). Emerging evidence confirms that proliferating tumor cells depolarizes Vm, while terminally differentiated non-cancer cells are characterized by hyperpolarized Vm (Binggeli and Cameron, 1980; Marino et al., 1994; Fraser et al., 2005). Yang et al. reported that a steady-state inward Na+ current carried by Nav1.5 contributes to depolarizing the resting Vm in MDA-MB-231 cells (Yang et al., 2020). Previous studies suggest that Vm depolarization promotes the redistribution of anionic phospholipids PIP2 and phosphatidylserine within the plasma membrane, resulting in GTPase K-Ras activation (Chen et al., 2011; Zhou et al., 2015; Remorino et al., 2017). Rac1 is one of the Rho GTPases that contribute to lamellipodia formation and migration (Nobes and Hall, 1995; Steffen et al., 2013). Similarly, Nav1.5 dependent Vm depolarization regulates Rac1 activation and localization in lamellipodia by its interaction with phosphatidylserine, regulating cell morphology and migration in breast cancer cells (Yang et al., 2020). Rac1 is a potential upstream regulator of the Arp2/3 complex, which is required for lamellipodia extension (Suraneni et al., 2012). Furthermore, Rac1 is closely associated with phosphorylation of cortactin and cofilin, promoting the acquisition of a promigratory phenotype (Head et al., 2003; Sumida and Yamada, 2015) (Figure 1).
Na+ currents carried by Nav1.5 stimulate phosphorylation of protein kinase A (PKA), which increases the Nav1.5 mRNA level but does not affect the total protein level. This changes the distribution of Nav1.5 in MDA-MB-231 cells, with the level of Nav1.5 increased on the plasma membrane and reduced in cytoplasm. The externalization of Nav1.5 potentiates the invasion and migration of breast cancer cells. The increase of Nav1.5 on the membrane in turn increases the Na+ currents, thus, forming a positive feedback (Figure 2) (Chioni et al., 2010). There is also positive feedback between Nav1.5 and GTPase RhoA, which is overexpressed in many cancers, including breast cancer. Silencing of RhoA decreases the expression of Nav1.5 mRNA, as well as Nav1.5-mediated Na+ currents. In turn, silencing of Nav1.5 decreased the protein level of RhoA (Dulong et al., 2014), which may be a result of the reduction in calcium concentration (Rao et al., 2001). In addition, suppressing both RhoA and Nav1.5 significantly reduced the invasion of MDA-MB-231 cells (Dulong et al., 2014).
Figure 2 Na+ currents carried by Nav1.5 stimulate phosphorylation of PKA, which increases the Nav1.5 mRNA level but does not affect the total protein level. This changes the distribution of Nav1.5 in MDA-MB-231 cells, such that Nav1.5 protein level increases on the membrane but that in the cytoplasm decreases. The externalization of Nav1.5 then potentiates the invasion and migration of breast cancer cells. The increase of Nav1.5 on the membrane in turn increases the Na+ currents, thus, forming a positive feedback.
Recent research has shown that Nav1.5 promotes invasiveness by taking part in EMT, a process involved in tissue formation during embryogenesis and repair processes, and promoting cancer cell dissemination from the primary tumor (Banyard and Bielenberg, 2015). MDA-MB-231 cells present typical spindle-shaped mesenchymal morphology and multiple filopodia. Upon knocking down the expression of SCN5A, these cells show decreased the number of filopodia that are shorter in length. The expression level of SNAI1, an EMT-inducing transcription factor, significantly decreased in MDA-MB-231 cells treated with a specific small-hairpin RNA (shNav1.5 cells). In contrast, MCF-7 cells overexpressing Nav1.5 display an increased invasive capacity, as well as an increased expression level of SNAI1, which provides evidence for the role of SNAI1 in Nav1.5 activity-mediated invasive process of breast cancer. Indeed, loss of expression of SIK1 upregulates Nav1.5 activity and expression (Gradek et al., 2019). Hence, it is that low expression levels of SIK1 in breast cancer cells induce the activity and expression of Nav1.5, followed by high expression of SNAI1, triggering the EMT and metastasis observed in breast cancer cells.
Nowadays, a variety of approaches, including specific antibodies, natural toxins, and pharmacological agents, are applied to reduce the metastasis of breast cancer cells by inhibiting Nav1.5 (Table 1).
Application of an anti-peptide polyclonal antibody, NESOpAb, blocks VGSC currents of EBNA-293 cells transfected with nNav1.5 and has no significant effect on adult Nav1.5 transfectants; thus, NESOpAb specifically targets nNav1.5, but not adult Nav1.5 (Chioni et al., 2005). NESOpAb inhibits the migration and invasion of MDA-MB-231 cells by suppressing the functional nNav1.5 activity but does not show similar results in human metastatic prostate cancer PC-3M cells, in which Nav1.7 is dominant (Diss et al., 2001; Brackenbury et al., 2007), which is consistent with the notion that NESOpAb specifically recognizes nNav1.5. E3Ab is a peptide-specific polyclonal antibody that recognizes the third extracellular region of Nav1.5, leading to specific inhibition of Nav1.5 activity (Xu et al., 2005). MDA-MB-231 cells treated with E3Ab show significantly decreased migration and invasive abilities. Indeed, a marked anti-proliferative effect was observed in E3Ab-treated MDA-MB-231 cells (Gao et al., 2019).
Phenytoin is an antiarrhythmic and antiepileptic agent that shows a high affinity toward Nav1.5 on the membrane of metastatic breast cancer cells in its inactivated state and reduces Na+ currents. The depolarization of MDA-MB-231 causes the opening of VGSCs, which then rapidly enter an incomplete inactivated state after a few milliseconds. This process produces transient and persistent Na+ currents, which play a key role in the metastasis of breast cancer cells (Yang et al., 2012). Phenytoin significantly reduces the mobility, migration, and invasion of metastatic breast cancer in vitro and in vivo (Nelson et al., 2015a; Mohammed et al., 2016). Blocking the Na+ current by phenytoin results in the downregulation of EGF expression and EGF-induced ERK1/2 phosphorylation, reducing the activity of MMP7, cathepsin E, and kallikrein-10, among which phenytoin showed the greatest inhibitory effect on cathepsin E (Mohammed et al., 2016). It has been proven that higher cathepsin E levels in the serum are positively correlated with poorer clinical prognosis in breast cancer patients (Kawakubo et al., 2014), thus, phenytoin might serve as an effective drug for improving therapeutic efficiency and survival of patients (Mohammed et al., 2016). Although phenytoin does not affect proliferation in vitro (Yang et al., 2012), it reduces the proliferation of metastatic breast cancer and slows tumor growth in vivo (Nelson et al., 2015a). The Notch signal inhibitor N-[N-(3,5-difluorophenacetyl)-L-alanyl]-S-phenylglycine-t-butyl ester (DAPT) has an anti-proliferative effect on MDA-MB-231 cells that can be decreased by phenytoin. Both DAPT and phenytoin reduce the lateral motility of breast cancer cells. It is reasonable to speculate that they have different effects on the proliferation of breast cancer cells. DAPT reduces the nNav1.5 mRNA expression level, which is not observed in the phenytoin treatment group. DAPT significantly reduces the ratio of MMP9 to tissue inhibitor of metalloproteinases-1 (TIMP1), which could be partly reversed by the combination of DAPT and phenytoin, indicating that the application of DAPT together with phenytoin is not a better choice than the single treatment of DAPT and phenytoin (Aktas et al., 2015). Furthermore, phenytoin inhibits the metastasis of breast cancer cells to other organs such as the lungs, liver, and spleen by reducing the density of MMP9-expressing cells (Nelson et al., 2015a). This suggests that the contribution of Nav1.5 to tumor growth in vivo is closely associated with adjacent tissues or the ECM.
It is noteworthy that some dietary habits have been shown to protect against cancer. It has been reported that omega-3 long-chain polyunsaturated fatty acids (ω-3 LC-PUFAs) play a role in the prevention of cancer. The effects of docosahexaenoic acid (DHA), one of the most important ω-3 LC-PUFAs, have been studied in the field of breast cancer. Short- and long-term applications of DHA inhibit nNav1.5 activity in the metastatic human MDA-MB-231 breast cancer cell line by decreasing the peak VGSC current density. In the long-term application, DHA inhibits both mRNA and protein expression levels of nNav1.5 and reduces cell migration (Isbilen et al., 2006). Peroxisome proliferator-activated receptor β (PPARβ) regulates the expression of numerous genes, including SCN5A, by binding with PPAR response elements on the promoters of these genes, thus, regulating cell survival, inflammation, and metabolism (Peters et al., 2012). DHA, a natural ligand of PPAR, has shown the potential to suppress tumor growth in different kinds of models with no side effects (Forman et al., 1997; Yao et al., 2014). DHA has been shown to reduce PPARβ expression, which is overexpressed in breast cancer cells, and inhibits the invasiveness and growth of breast cancer cells (Wannous et al., 2013; Yao et al., 2014; Wannous et al., 2015). The downregulation of PPARβ reduces SCN5A expression at both the mRNA and protein levels, as well as nNav1.5 density at the plasma membrane, which finally leads to the reduction of Na+ currents. In addition, the downregulation of PPARβ reduces NHE-1–dependent H+ efflux by inhibiting the activity of Nav1.5 channels without altering NHE-1 expression. PPARβ is indispensable for DHA to reduce Nav1.5 expression and NHE-1 activity, giving rise to the invasiveness of breast cancer cells (Wannous et al., 2015).
Ranolazine has been approved by the US Food and Drug Administration for chronic angina (Antzelevitch et al., 2004). One of the most characterized pharmacological effects of ranolazine is its ability to selectively inhibit late Na+ currents. The inhibition of Na+ currents triggers a steeper Na+ gradient and activation of the Na+/Ca exchanger, which reduces the intracellular overloaded calcium and improves ventricular relaxation in cardiac ischemia conditions (Fraser et al., 2006). Ranolazine has also been shown to have anti-invasiveness potential in breast cancer. In vitro, ranolazine reduces the function of Nav1.5 and decreases Na+ influx in MDA-MB-231 cells, which results in decreased ECM degradative activity and pro-invasive morphology of cells. In vivo, ranolazine slows down tumor growth and inhibits the colonization of breast cancer cells to other organs without obvious toxic effects by reducing the Nav1.5 carried currents in tumor tissues (Driffort et al., 2014). The expressions of β‐adrenergic receptor (β‐AR) and Nav1.5 overlap substantially in MDA-MB-231 cells. Propranolol which is a blocker of β‐AR, coupled to with PKA activation, modulates Nav1.5 and takes part in reducing migration and invasion of MDA-MB-231 cells. Short-term treatment with propranolol tends to reduce peak Na+ currents carried by Nav1.5, while long-term treatment results in sustained changes of properties and expression of Nav1.5. Indeed, the role of factors contained in the serum cannot be ignored. Both propranolol and ranolazine reduced the motility and invasiveness of MDA-MB-231 cells, but the effect of their combination was not better than that of individual treatments (Lee et al., 2019).
Taxol and its derivatives, such as docetaxel, are widely used in the treatment of breast cancer (Dang and Hudis, 2006). The widely accepted explanation for their effects is that they stabilize the microtubules during mitosis, which leads to the inhibition of cell division (Camacho et al., 2000). Taxol also has a potential anti-invasive effect on breast cancer cells at low concentrations but has no effect on cell proliferation. The reduction of the current carried by Nav1.5 was not observed in cells pretreated with taxol, which demonstrates a significant leftward shift of the activation properties of Nav1.5. It is known that the activity of sodium channels is closely associated with perturbation of microtubules (Motlagh et al., 2002). Tran reported that a short-term and low-dose taxol treatment is sufficient to affect microtubule polymerization (Tran et al., 2009). It seems that the effects of taxol on cell invasiveness are complicated, and other proteins involved in the Nav1.5-regulated signaling pathway may be regulated by taxol, but the precise mechanisms remain unknown.
Recently, animal peptides have drawn considerable interest. FS50, the salivary protein from Xenopsylla cheopis, shows an inhibitory effect against the Nav1.5 channel (Xu et al., 2016). FS50 significantly reduced the peak VGSC currents and decreased the expression of Nav1.5 mRNA in MDA-MB-231 cells but had no effect on the total Nav1.5 protein levels. Notably, FS50 reduces the Nav1.5 protein expression in the plasma membrane, which means that FS50 only changes the distribution of the protein between the plasma membrane and the cytoplasm. The effect is identical to the effects of a PKA inhibitor; thus, FS50 may inhibit the PKA pathway, resulting in the reduced expression of Nav1.5. In the future, it is worth detecting PKA-related proteins after the treatment of FS50 (Brackenbury and Djamgoz, 2006; Chioni et al., 2010). FS50 inhibits the migration and invasion of MDA-MB-231 cells but has no effect on the proliferation of MCF-7 and MDA-MB-231 cells. The reduction of MMP9 activity and the ratio of MMP9 mRNA to TIMP1 mRNA were observed in MDA-MB-231 cells treated with FS50 (Zhang et al., 2018).
In addition to the known VGSC-blocking drugs, scientists have developed a highly predictive and comprehensive three-dimensional quantitative structure–activity relationship model for designing the compounds to bind with VGSC ligands (Zha et al., 2014). Five low micromolar, small molecule compounds acting as Nav1.5 blockers in MDA-MB-231 cells were designed, synthesized, and evaluated. Two of the compounds were identified to reduce peak Na+ currents and reduce the invasion of MDA-MB-231 cells without affecting cell viability (Dutta et al., 2018). Compared with known drugs, these compounds are more effective and have simpler chemical structures. Moreover, synthetic substances are designed to target Nav1.5, providing a new and potent direction to develop drugs for the treatment of breast cancer metastasis.
The membrane current generated by VGSCs has two distinct modes: transient (INaT) and persistent (INaP). INaP can last 100 ms to 1 s, while INaT lasts a millisecond (Djamgoz and Onkal, 2013). Hypoxia, which is commonly observed in cancer and promotes metastasis and invasion, significantly contributes to increasing INaP (Hammarstrom and Gage, 2002; Muz et al., 2015; Rankin and Giaccia, 2016; Guzel et al., 2019). Compared with INaT, INaP is resistant to inactivation even at depolarized potentials and will lead to significant changes in the global level of Na+ that affect Nav1.5-dependent mechanisms and play an important role in cancer progression (Saint, 2006). Indeed, the inhibition of INaP has been shown to produce a major anti-metastatic effect and has been proposed as a new target (Guzel et al., 2019). As mentioned above, MDA-MB-231 cells exhibit depolarized Vm. In addition, slower kinetics of activation, inactivation, and recovery from inactivation are observed in nNav1.5 than in the ‘adult’ form (Onkal et al., 2008). Thus, it is reasonable to assume that INaP is mainly responsible for Na+ elevation in breast cancer cells. At present, it is easy to identify INaT and INaP by whole-cell patch-clamp recordings, and a number of pharmacological agents have been shown to selectively block INaP (Urbani and Belluzzi, 2000; Maier, 2009). In the future, additional INaP blockers inhibiting Nav1.5-mediated persistent current should be considered as a new target to reduce metastasis of breast cancer. In addition, most agents listed above produce a major anti-metastatic effect by decreasing the current carried by Nav1.5 or changing the properties of Nav1.5. Specifically, decreasing the expression of nNav1.5 is another ideal target for specific anti-breast cancer treatment.
The increasing evidence is indicative of the role of aberrant Nav1.5 activation in the metastatic progression of breast cancer cells. Nav1.5 functions to trigger a variety of downstream mechanisms in breast cancer cells to regulate metastatic and invasive capacity. Considering the large and growing body of evidence, different approaches recognizing Nav1.5 are applied to inhibit metastasis of breast cancer in vitro and favor Nav1.5 as an anti-metastatic target. Although we reviewed several studies here, there are many unanswered questions that require further investigation: (i) Is it possible to make nNav1.5 a biomarker of early diagnosis of breast cancer; (ii) Can we develop additional agents specifically blocking INaP or nNav1.5 to improve efficacy and reduce side effects; and (iii) How can we accumulate the process of translational medicine and promote the application of these agents in the clinic to improve the outcomes for breast cancer patients.
QL and TW wrote the review and designed the figures and the table. WW and GC made contributions to searching related articles. XL, LJ, HT, MR and SK contributed to the conception of the study. MD supervised the whole work, contributed to writing, and critically revised the paper. All authors contributed to the article and approved the submitted version.
This work was supported by the National Natural Science Foundation of China under contract (Nos. 31672290, 31100764, 31971190, 30901874, 81503276), the Natural Science Foundation of Hunan Province, China (No. 2016JJ3180), the Valuable Instrument and Equipment Fund of Central South University (No. CSUZC2019046, CSUZC2020043).
The authors declare that the research was conducted in the absence of any commercial or financial relationships that could be construed as a potential conflict of interest.
Adams, B. D., Cowee, D. M., White, B. A. (2009). The role of miR-206 in the epidermal growth factor (EGF) induced repression of estrogen receptor-alpha (ERalpha) signaling and a luminal phenotype in MCF-7 breast cancer cells. Mol. Endocrinol. 23, 1215–1230. doi: 10.1210/me.2009-0062
Aktas, C. C., Zeybek, N. D., Piskin, A. K. (2015). In vitro effects of phenytoin and DAPT on MDA-MB-231 breast cancer cells. Acta Biochim. Biophys. Sin. (Shanghai) 47, 680–686. doi: 10.1093/abbs/gmv066
Amin, A. S., Pinto, Y. M., Wilde, A. A. (2013). Long QT syndrome: beyond the causal mutation. J. Physiol. 591, 4125–4139. doi: 10.1113/jphysiol.2013.254920
Amith, S. R., Fliegel, L. (2017). Na(+)/H(+) exchanger-mediated hydrogen ion extrusion as a carcinogenic signal in triple-negative breast cancer etiopathogenesis and prospects for its inhibition in therapeutics. Semin. Cancer Biol. 43, 35–41. doi: 10.1016/j.semcancer.2017.01.004
Antzelevitch, C., Belardinelli, L., Zygmunt, A. C., Burashnikov, A., Di Diego, J. M., Fish, J. M., et al. (2004). Electrophysiological effects of ranolazine, a novel antianginal agent with antiarrhythmic properties. Circulation 110, 904–910. doi: 10.1161/01.CIR.0000139333.83620.5D
Antzelevitch, C., Brugada, P., Borggrefe, M., Brugada, J., Brugada, R., Corrado, D., et al. (2005). Brugada syndrome: report of the second consensus conference. Heart Rhythm. 2, 429–440. doi: 10.1016/j.hrthm.2005.01.005
Arciero, C. A., Guo, Y., Jiang, R., Behera, M., O’Regan, R., Peng, L., et al. (2019). ER(+)/HER2(+) Breast Cancer Has Different Metastatic Patterns and Better Survival Than ER(-)/HER2(+) Breast Cancer. Clin. Breast Cancer 19, 236–245. doi: 10.1016/j.clbc.2019.02.001
Aydar, E., Stratton, D., Fraser, S. P., Djamgoz, M. B., Palmer, C. (2016). Sigma-1 receptors modulate neonatal Nav1.5 ion channels in breast cancer cell lines. Eur. Biophys. J. 45, 671–683. doi: 10.1007/s00249-016-1135-0
Balasuriya, D., Stewart, A. P., Crottes, D., Borgese, F., Soriani, O., Edwardson, J. M. (2012). The sigma-1 receptor binds to the Nav1.5 voltage-gated Na+ channel with 4-fold symmetry. J. Biol. Chem. 287, 37021–37029. doi: 10.1074/jbc.M112.382077
Banyard, J., Bielenberg, D. R. (2015). The role of EMT and MET in cancer dissemination. Connect Tissue Res. 56, 403–413. doi: 10.3109/03008207.2015.1060970
Beckermann, T. M., McLeod, K., Murday, V., Potet, F., George, A. L., Jr. (2014). Novel SCN5A mutation in amiodarone-responsive multifocal ventricular ectopy-associated cardiomyopathy. Heart Rhythm. 11, 1446–1453. doi: 10.1016/j.hrthm.2014.04.042
Binggeli, R., Cameron, I. L. (1980). Cellular potentials of normal and cancerous fibroblasts and hepatocytes. Cancer Res. 40, 1830–1835.
Black, J. A., Waxman, S. G. (2013). Noncanonical roles of voltage-gated sodium channels. Neuron 80, 280–291. doi: 10.1016/j.neuron.2013.09.012
Bourguignon, L. Y., Zhu, H., Shao, L., Chen, Y. W. (2001). CD44 interaction with c-Src kinase promotes cortactin-mediated cytoskeleton function and hyaluronic acid-dependent ovarian tumor cell migration. J. Biol. Chem. 276, 7327–7336. doi: 10.1074/jbc.M006498200
Brackenbury, W. J., Djamgoz, M. B. (2006). Activity-dependent regulation of voltage-gated Na+ channel expression in Mat-LyLu rat prostate cancer cell line. J. Physiol. 573, 343–356. doi: 10.1113/jphysiol.2006.106906
Brackenbury, W. J., Isom, L. L. (2011). Na Channel beta Subunits: Overachievers of the Ion Channel Family. Front. Pharmacol. 2, 53. doi: 10.3389/fphar.2011.00053
Brackenbury, W. J., Chioni, A. M., Diss, J. K., Djamgoz, M. B. (2007). The neonatal splice variant of Nav1.5 potentiates in vitro invasive behaviour of MDA-MB-231 human breast cancer cells. Breast Cancer Res. Treat 101, 149–160. doi: 10.1007/s10549-006-9281-1
Breastcancer.org. Breast Cancer Statistics. Available at: https://www.breastcancer.org/symptoms/understand_bc/statistics
Brisson, L., Gillet, L., Calaghan, S., Besson, P., Le Guennec, J. Y., Roger, S., et al. (2011). Na(V)1.5 enhances breast cancer cell invasiveness by increasing NHE1-dependent H(+) efflux in caveolae. Oncogene 30, 2070–2076. doi: 10.1038/onc.2010.574
Brisson, L., Driffort, V., Benoist, L., Poet, M., Counillon, L., Antelmi, E., et al. (2013). NaV1.5 Na(+) channels allosterically regulate the NHE-1 exchanger and promote the activity of breast cancer cell invadopodia. J. Cell Sci. 126, 4835–4842. doi: 10.1242/jcs.123901
Camacho, J., Sanchez, A., Stuhmer, W., Pardo, L. A. (2000). Cytoskeletal interactions determine the electrophysiological properties of human EAG potassium channels. Pflugers Arch. 441, 167–174. doi: 10.1007/s004240000420
Catterall, W. A. (2000). From ionic currents to molecular mechanisms: the structure and function of voltage-gated sodium channels. Neuron 26, 13–25. doi: 10.1016/S0896-6273(00)81133-2
Chang, G., Wang, J., Zhang, H., Zhang, Y., Wang, C., Xu, H., et al. (2014). CD44 targets Na(+)/H(+) exchanger 1 to mediate MDA-MB-231 cells’ metastasis via the regulation of ERK1/2. Br. J. Cancer 110, 916–927. doi: 10.1038/bjc.2013.809
Chen, X., Zhang, X., Jia, C., Xu, J., Gao, H., Zhang, G., et al. (2011). Membrane depolarization increases membrane PtdIns(4,5)P2 levels through mechanisms involving PKC betaII and PI4 kinase. J. Biol. Chem. 286, 39760–39767. doi: 10.1074/jbc.M111.289090
Chen, Q., Liu, Y., Zhu, X. L., Feng, F., Yang, H., Xu, W. (2019). Increased NHE1 expression is targeted by specific inhibitor cariporide to sensitize resistant breast cancer cells to doxorubicin in vitro and in vivo. BMC Cancer 19, 211. doi: 10.1186/s12885-019-5397-7
Chioni, A. M., Fraser, S. P., Pani, F., Foran, P., Wilkin, G. P., Diss, J. K., et al. (2005). A novel polyclonal antibody specific for the Na(v)1.5 voltage-gated Na(+) channel ‘neonatal’ splice form. J. Neurosci. Methods 147, 88–98. doi: 10.1016/j.jneumeth.2005.03.010
Chioni, A. M., Brackenbury, W. J., Calhoun, J. D., Isom, L. L., Djamgoz, M. B. (2009). A novel adhesion molecule in human breast cancer cells: voltage-gated Na+ channel beta1 subunit. Int. J. Biochem. Cell Biol. 41, 1216–1227. doi: 10.1016/j.biocel.2008.11.001
Chioni, A. M., Shao, D., Grose, R., Djamgoz, M. B. (2010). Protein kinase A and regulation of neonatal Nav1.5 expression in human breast cancer cells: activity-dependent positive feedback and cellular migration. Int. J. Biochem. Cell Biol. 42, 346–358. doi: 10.1016/j.biocel.2009.11.021
Dang, C., Hudis, C. (2006). Adjuvant taxanes in the treatment of breast cancer: no longer at the tip of the iceberg. Clin. Breast Cancer 7, 51–58. doi: 10.3816/CBC.2006.n.013
Diss, J. K., Archer, S. N., Hirano, J., Fraser, S. P., Djamgoz, M. B. (2001). Expression profiles of voltage-gated Na(+) channel alpha-subunit genes in rat and human prostate cancer cell lines. Prostate 48, 165–178. doi: 10.1002/pros.1095
Djamgoz, M. B., Onkal, R. (2013). Persistent current blockers of voltage-gated sodium channels: a clinical opportunity for controlling metastatic disease. Recent Pat. Anticancer Drug Discovery 8, 66–84. doi: 10.2174/1574892811308010066
Driffort, V., Gillet, L., Bon, E., Marionneau-Lambot, S., Oullier, T., Joulin, V., et al. (2014). Ranolazine inhibits NaV1.5-mediated breast cancer cell invasiveness and lung colonization. Mol. Cancer 13, 264. doi: 10.1186/1476-4598-13-264
Dulong, C., Fang, Y. J., Gest, C., Zhou, M. H., Patte-Mensah, C., Mensah-Nyagan, A. G., et al. (2014). The small GTPase RhoA regulates the expression and function of the sodium channel Nav1.5 in breast cancer cells. Int. J. Oncol. 44, 539–547. doi: 10.3892/ijo.2013.2214
Dutta, S., Lopez Charcas, O., Tanner, S., Gradek, F., Driffort, V., Roger, S., et al. (2018). Discovery and evaluation of nNav1.5 sodium channel blockers with potent cell invasion inhibitory activity in breast cancer cells. Bioorg. Med. Chem. 26, 2428–2436. doi: 10.1016/j.bmc.2018.04.003
Fairhurst, C., Watt, I., Martin, F., Bland, M., Brackenbury, W. J. (2015). Sodium channel-inhibiting drugs and survival of breast, colon and prostate cancer: a population-based study. Sci. Rep. 5, 16758. doi: 10.1038/srep16758
Forman, B. M., Chen, J., Evans, R. M. (1997). Hypolipidemic drugs, polyunsaturated fatty acids, and eicosanoids are ligands for peroxisome proliferator-activated receptors alpha and delta. Proc. Natl. Acad. Sci. U.S.A. 94, 4312–4317. doi: 10.1073/pnas.94.9.4312
Fraser, S. P., Diss, J. K., Chioni, A. M., Mycielska, M. E., Pan, H., Yamaci, R. F., et al. (2005). Voltage-gated sodium channel expression and potentiation of human breast cancer metastasis. Clin. Cancer Res. 11, 5381–5389. doi: 10.1158/1078-0432.CCR-05-0327
Fraser, H., Belardinelli, L., Wang, L., Light, P. E., McVeigh, J. J., Clanachan, A. S. (2006). Ranolazine decreases diastolic calcium accumulation caused by ATX-II or ischemia in rat hearts. J. Mol. Cell Cardiol. 41, 1031–1038. doi: 10.1016/j.yjmcc.2006.08.012
Gao, R., Cao, T., Chen, H., Cai, J., Lei, M., Wang, Z. (2019). Nav1.5-E3 antibody inhibits cancer progression. Trans. Cancer Res. 8, 44–50. doi: 10.21037/tcr.2018.12.23
Gillet, L., Roger, S., Besson, P., Lecaille, F., Gore, J., Bougnoux, P., et al. (2009). Voltage-gated Sodium Channel Activity Promotes Cysteine Cathepsin-dependent Invasiveness and Colony Growth of Human Cancer Cells. J. Biol. Chem. 284, 8680–8691. doi: 10.1074/jbc.M806891200
Giudicessi, J. R., Ackerman, M. J. (2013). Genotype- and phenotype-guided management of congenital long QT syndrome. Curr. Probl. Cardiol. 38, 417–455. doi: 10.1016/j.cpcardiol.2013.08.001
Gonzalez-Gonzalez, L., Gonzalez-Ramirez, R., Flores, A., Avelino-Cruz, J. E., Felix, R., Monjaraz, E. (2019). Epidermal Growth Factor Potentiates Migration of MDA-MB 231 Breast Cancer Cells by Increasing NaV1.5 Channel Expression. Oncology 97, 373–382. doi: 10.1159/000501802
Gradek, F., Lopez-Charcas, O., Chadet, S., Poisson, L., Ouldamer, L., Goupille, C., et al. (2019). Sodium Channel Nav1.5 Controls Epithelial-to-Mesenchymal Transition and Invasiveness in Breast Cancer Cells Through its Regulation by the Salt-Inducible Kinase-1. Sci. Rep. 9, 18652. doi: 10.1038/s41598-019-55197-5
Greaney, M. L., Sprunck-Harrild, K., Ruddy, K. J., Ligibel, J., Barry, W. T., Baker, E., et al. (2015). Study protocol for Young & Strong: a cluster randomized design to increase attention to unique issues faced by young women with newly diagnosed breast cancer. BMC Public Health 15, 37. doi: 10.1186/s12889-015-1346-9
Guzel, R. M., Ogmen, K., Ilieva, K. M., Fraser, S. P., Djamgoz, M. B. A. (2019). Colorectal cancer invasiveness in vitro: Predominant contribution of neonatal Nav1.5 under normoxia and hypoxia. J. Cell Physiol. 234, 6582–6593. doi: 10.1002/jcp.27399
Hammarstrom, A. K., Gage, P. W. (2002). Hypoxia and persistent sodium current. Eur. Biophys. J. 31, 323–330. doi: 10.1007/s00249-002-0218-2
Hayashi, T., Su, T. P. (2003). Sigma-1 receptors (sigma(1) binding sites) form raft-like microdomains and target lipid droplets on the endoplasmic reticulum: roles in endoplasmic reticulum lipid compartmentalization and export. J. Pharmacol. Exp. Ther. 306, 718–725. doi: 10.1124/jpet.103.051284
Hayashi, T., Su, T. (2005). The sigma receptor: evolution of the concept in neuropsychopharmacology. Curr. Neuropharmacol. 3, 267–280. doi: 10.2174/157015905774322516
Head, J. A., Jiang, D., Li, M., Zorn, L. J., Schaefer, E. M., Parsons, J. T., et al. (2003). Cortactin tyrosine phosphorylation requires Rac1 activity and association with the cortical actin cytoskeleton. Mol. Biol. Cell 14, 3216–3229. doi: 10.1091/mbc.e02-11-0753
Hill, A., McFarlane, S., Mulligan, K., Gillespie, H., Draffin, J. E., Trimble, A., et al. (2006). Cortactin underpins CD44-promoted invasion and adhesion of breast cancer cells to bone marrow endothelial cells. Oncogene 25, 6079–6091. doi: 10.1038/sj.onc.1209628
Hoshino, A., Costa-Silva, B., Shen, T. L., Rodrigues, G., Hashimoto, A., Tesic Mark, M., et al. (2015). Tumour exosome integrins determine organotropic metastasis. Nature 527, 329–335. doi: 10.1038/nature15756
Hull, J. M., Isom, L. L. (2018). Voltage-gated sodium channel beta subunits: The power outside the pore in brain development and disease. Neuropharmacology 132, 43–57. doi: 10.1016/j.neuropharm.2017.09.018
Hurvitz, S., Singh, R., Adams, B., Taguchi, J. A., Chan, D., Dichmann, R. A., et al. (2018). Phase Ib/II single-arm trial evaluating the combination of everolimus, lapatinib and capecitabine for the treatment of HER2-positive breast cancer with brain metastases (TRIO-US B-09). Ther. Adv. Med. Oncol. 10, 1–13. doi: 10.1177/1758835918807339
Isbilen, B., Fraser, S. P., Djamgoz, M. B. (2006). Docosahexaenoic acid (omega-3) blocks voltage-gated sodium channel activity and migration of MDA-MB-231 human breast cancer cells. Int. J. Biochem. Cell Biol. 38, 2173–2182. doi: 10.1016/j.biocel.2006.06.014
Jakowlew, S. B. (2006). Transforming growth factor-beta in cancer and metastasis. Cancer Metastasis Rev. 25, 435–457. doi: 10.1007/s10555-006-9006-2
Kamarulzaman, N. S., Dewadas, H. D., Leow, C. Y., Yaacob, N. S., Mokhtar, N. F. (2017). The role of REST and HDAC2 in epigenetic dysregulation of Nav1.5 and nNav1.5 expression in breast cancer. Cancer Cell Int. 17, 74. doi: 10.1186/s12935-017-0442-6
Kao, L. T., Huang, C. C., Lin, H. C., Huang, C. Y. (2018). Antiarrhythmic drug usage and prostate cancer: a population-based cohort study. Asian J. Androl. 20, 37–42. doi: 10.4103/aja.aja_26_17
Kawakubo, T., Yasukochi, A., Toyama, T., Takahashi, S., Okamoto, K., Tsukuba, T., et al. (2014). Repression of cathepsin E expression increases the risk of mammary carcinogenesis and links to poor prognosis in breast cancer. Carcinogenesis 35, 714–726. doi: 10.1093/carcin/bgt373
Kuwahara, K. (2013). Role of NRSF/REST in the regulation of cardiac gene expression and function. Circ. J. 77, 2682–2686. doi: 10.1253/circj.CJ-13-1210
Lee, A., Fraser, S. P., Djamgoz, M. B. A. (2019). Propranolol inhibits neonatal Nav1.5 activity and invasiveness of MDA-MB-231 breast cancer cells: Effects of combination with ranolazine. J. Cell Physiol. 234, 23066–23081. doi: 10.1002/jcp.28868
Maier, L. S. (2009). A novel mechanism for the treatment of angina, arrhythmias, and diastolic dysfunction: inhibition of late I(Na) using ranolazine. J. Cardiovasc. Pharmacol. 54, 279–286. doi: 10.1097/FJC.0b013e3181a1b9e7
Margan, M. M., Jitariu, A. A., Cimpean, A. M., Nica, C., Raica, M. (2016). Molecular Portrait of the Normal Human Breast Tissue and Its Influence on Breast Carcinogenesis. J. Breast Cancer 19, 99–111. doi: 10.4048/jbc.2016.19.2.99
Marino, A. A., Morris, D. M., Schwalke, M. A., Iliev, I. G., Rogers, S. (1994). Electrical potential measurements in human breast cancer and benign lesions. Tumour Biol. 15, 147–152. doi: 10.1159/000217885
Massague, J., Batlle, E., Gomis, R. R. (2017). Understanding the molecular mechanisms driving metastasis. Mol. Oncol. 11, 3–4. doi: 10.1002/1878-0261.12024
McFarlane, S., Coulter, J. A., Tibbits, P., O’Grady, A., McFarlane, C., Montgomery, N., et al. (2015). CD44 increases the efficiency of distant metastasis of breast cancer. Oncotarget 6, 11465–11476. doi: 10.18632/oncotarget.3410
Mego, M., Mani, S. A., Cristofanilli, M. (2010). Molecular mechanisms of metastasis in breast cancer–clinical applications. Nat. Rev. Clin. Oncol. 7, 693–701. doi: 10.1038/nrclinonc.2010.171
Mohammed, F. H., Khajah, M. A., Yang, M., Brackenbury, W. J., Luqmani, Y. A. (2016). Blockade of voltage-gated sodium channels inhibits invasion of endocrine-resistant breast cancer cells. Int. J. Oncol. 48, 73–83. doi: 10.3892/ijo.2015.3239
Monk, M., Holding, C. (2001). Human embryonic genes re-expressed in cancer cells. Oncogene 20, 8085–8091. doi: 10.1038/sj.onc.1205088
Motlagh, D., Alden, K. J., Russell, B., Garcia, J. (2002). Sodium current modulation by a tubulin/GTP coupled process in rat neonatal cardiac myocytes. J. Physiol. 540, 93–103. doi: 10.1113/jphysiol.2001.013474
Moustakas, A., Heldin, C. H. (2016). Mechanisms of TGFbeta-Induced Epithelial-Mesenchymal Transition. J. Clin. Med. 5, 63. doi: 10.3390/jcm5070063
Muz, B., de la Puente, P., Azab, F., Azab, A. K. (2015). The role of hypoxia in cancer progression, angiogenesis, metastasis, and resistance to therapy. Hypoxia (Auckl) 3, 83–92. doi: 10.2147/HP.S93413
Nelson, M., Yang, M., Dowle, A. A., Thomas, J. R., Brackenbury, W. J. (2015a). The sodium channel-blocking antiepileptic drug phenytoin inhibits breast tumour growth and metastasis. Mol. Cancer 14, 13. doi: 10.1186/s12943-014-0277-x
Nelson, M., Yang, M., Millican-Slater, R., Brackenbury, W. J. (2015b). Nav1.5 regulates breast tumor growth and metastatic dissemination in vivo. Oncotarget 6, 32914–32929. doi: 10.18632/oncotarget.5441
Nobes, C. D., Hall, A. (1995). Rho, rac, and cdc42 GTPases regulate the assembly of multimolecular focal complexes associated with actin stress fibers, lamellipodia, and filopodia. Cell 81, 53–62. doi: 10.1016/0092-8674(95)90370-4
Onkal, R., Mattis, J. H., Fraser, S. P., Diss, J. K., Shao, D., Okuse, K., et al. (2008). Alternative splicing of Nav1.5: an electrophysiological comparison of ‘neonatal’ and ‘adult’ isoforms and critical involvement of a lysine residue. J. Cell Physiol. 216, 716–726. doi: 10.1002/jcp.21451
Oser, M., Yamaguchi, H., Mader, C. C., Bravo-Cordero, J. J., Arias, M., Chen, X., et al. (2009). Cortactin regulates cofilin and N-WASp activities to control the stages of invadopodium assembly and maturation. J. Cell Biol. 186, 571–587. doi: 10.1083/jcb.200812176
Park, S. B., Chung, C. K., Gonzalez, E., Yoo, C. (2018). Causal Inference Network of Genes Related with Bone Metastasis of Breast Cancer and Osteoblasts Using Causal Bayesian Networks. J. Bone Metab. 25, 251–266. doi: 10.11005/jbm.2018.25.4.251
Peters, J. M., Shah, Y. M., Gonzalez, F. J. (2012). The role of peroxisome proliferator-activated receptors in carcinogenesis and chemoprevention. Nat. Rev. Cancer 12, 181–195. doi: 10.1038/nrc3214
Prat, A., Pineda, E., Adamo, B., Galvan, P., Fernandez, A., Gaba, L., et al. (2015). Clinical implications of the intrinsic molecular subtypes of breast cancer. Breast 24 Suppl 2, S26–S35. doi: 10.1016/j.breast.2015.07.008
Rankin, E. B., Giaccia, A. J. (2016). Hypoxic control of metastasis. Science 352, 175–180. doi: 10.1126/science.aaf4405
Rao, J. N., Li, L., Golovina, V. A., Platoshyn, O., Strauch, E. D., Yuan, J. X., et al. (2001). Ca2+-RhoA signaling pathway required for polyamine-dependent intestinal epithelial cell migration. Am. J. Physiol. Cell Physiol. 280, C993–1007. doi: 10.1152/ajpcell.2001.280.4.C993
Reddy, J. P., Dawood, S., Mitchell, M., Debeb, B. G., Bloom, E., Gonzalez-Angulo, A. M., et al. (2015). Antiepileptic drug use improves overall survival in breast cancer patients with brain metastases in the setting of whole brain radiotherapy. Radiother. Oncol. 117, 308–314. doi: 10.1016/j.radonc.2015.10.009
Remorino, A., De Beco, S., Cayrac, F., Di Federico, F., Cornilleau, G., Gautreau, A., et al. (2017). Gradients of Rac1 Nanoclusters Support Spatial Patterns of Rac1 Signaling. Cell Rep. 21, 1922–1935. doi: 10.1016/j.celrep.2017.10.069
Robey, I. F., Nesbit, L. A. (2013). Investigating mechanisms of alkalinization for reducing primary breast tumor invasion. BioMed. Res. Int. 2013, 485196. doi: 10.1155/2013/485196
Roopra, A., Sharling, L., Wood, I. C., Briggs, T., Bachfischer, U., Paquette, A. J., et al. (2000). Transcriptional repression by neuron-restrictive silencer factor is mediated via the Sin3-histone deacetylase complex. Mol. Cell Biol. 20, 2147–2157. doi: 10.1128/MCB.20.6.2147-2157.2000
Saint, D. A. (2006). The role of the persistent Na(+) current during cardiac ischemia and hypoxia. J Cardiovasc. Electrophysiol 17 Suppl 1, S96–s103. doi: 10.1111/j.1540-8167.2006.00390.x
Selvik, L. K., Rao, S., Steigedal, T. S., Haltbakk, I., Misund, K., Bruland, T., et al. (2014). Salt-inducible kinase 1 (SIK1) is induced by gastrin and inhibits migration of gastric adenocarcinoma cells. PloS One 9, e112485. doi: 10.1371/journal.pone.0112485
Sjostrom, M., Stenstrom, K., Eneling, K., Zwiller, J., Katz, A., II, Takemori, H., et al. (2007). SIK1 is part of a cell sodium-sensing network that regulates active sodium transport through a calcium-dependent process. Proc. Natl. Acad. Sci. U.S.A. 104, 16922–16927. doi: 10.1073/pnas.0706838104
Sorlie, T., Perou, C. M., Tibshirani, R., Aas, T., Geisler, S., Johnsen, H., et al. (2001). Gene expression patterns of breast carcinomas distinguish tumor subclasses with clinical implications. Proc. Natl. Acad. Sci. U.S.A. 98, 10869–10874. doi: 10.1073/pnas.191367098
Steffen, A., Ladwein, M., Dimchev, G. A., Hein, A., Schwenkmezger, L., Arens, S., et al. (2013). Rac function is crucial for cell migration but is not required for spreading and focal adhesion formation. J. Cell Sci. 126, 4572–4588. doi: 10.1242/jcs.118232
Sumida, G. M., Yamada, S. (2015). Rho GTPases and the downstream effectors actin-related protein 2/3 (Arp2/3) complex and myosin II induce membrane fusion at self-contacts. J. Biol. Chem. 290, 3238–3247. doi: 10.1074/jbc.M114.612168
Sundelacruz, S., Levin, M., Kaplan, D. L. (2009). Role of membrane potential in the regulation of cell proliferation and differentiation. Stem Cell Rev. Rep. 5, 231–246. doi: 10.1007/s12015-009-9080-2
Suraneni, P., Rubinstein, B., Unruh, J. R., Durnin, M., Hanein, D., Li, R. (2012). The Arp2/3 complex is required for lamellipodia extension and directional fibroblast cell migration. J. Cell Biol. 197, 239–251. doi: 10.1083/jcb.201112113
Tran, T. A., Gillet, L., Roger, S., Besson, P., White, E., Le Guennec, J. Y. (2009). Non-anti-mitotic concentrations of taxol reduce breast cancer cell invasiveness. Biochem. Biophys. Res. Commun. 379, 304–308. doi: 10.1016/j.bbrc.2008.12.073
Urbani, A., Belluzzi, O. (2000). Riluzole inhibits the persistent sodium current in mammalian CNS neurons. Eur. J. Neurosci. 12, 3567–3574. doi: 10.1046/j.1460-9568.2000.00242.x
Wannous, R., Bon, E., Maheo, K., Goupille, C., Chamouton, J., Bougnoux, P., et al. (2013). PPARbeta mRNA expression, reduced by n-3 PUFA diet in mammary tumor, controls breast cancer cell growth. Biochim. Biophys. Acta 1831, 1618–1625. doi: 10.1016/j.bbalip.2013.07.010
Wannous, R., Bon, E., Gillet, L., Chamouton, J., Weber, G., Brisson, L., et al. (2015). Suppression of PPARbeta, and DHA treatment, inhibit NaV1.5 and NHE-1 pro-invasive activities. Pflugers Arch. 467, 1249–1259. doi: 10.1007/s00424-014-1573-4
Xie, H. Y., Shao, Z. M., Li, D. Q. (2017). Tumor microenvironment: driving forces and potential therapeutic targets for breast cancer metastasis. Chin. J. Cancer 36, 36. doi: 10.1186/s40880-017-0202-y
Xu, S. Z., Zeng, F., Lei, M., Li, J., Gao, B., Xiong, C., et al. (2005). Generation of functional ion-channel tools by E3 targeting. Nat. Biotechnol. 23, 1289–1293. doi: 10.1038/nbt1148
Xu, X., Zhang, B., Yang, S., An, S., Ribeiro, J. M., Andersen, J. F. (2016). Structure and Function of FS50, a salivary protein from the flea Xenopsylla cheopis that blocks the sodium channel NaV1.5. Sci. Rep. 6, 36574. doi: 10.1038/srep36574
Yamaci, R. F., Fraser, S. P., Battaloglu, E., Kaya, H., Erguler, K., Foster, C. S., et al. (2017). Neonatal Nav1.5 protein expression in normal adult human tissues and breast cancer. Pathol. Res. Pract. 213, 900–907. doi: 10.1016/j.prp.2017.06.003
Yang, M., Brackenbury, W. J. (2013). Membrane potential and cancer progression. Front. Physiol. 4, 185. doi: 10.3389/fphys.2013.00185
Yang, X., Wang, D., Dong, W., Song, Z., Dou, K. (2011). Suppression of Na+/H + exchanger 1 by RNA interference or amiloride inhibits human hepatoma cell line SMMC-7721 cell invasion. Med. Oncol. 28, 385–390. doi: 10.1007/s12032-010-9447-x
Yang, M., Kozminski, D. J., Wold, L. A., Modak, R., Calhoun, J. D., Isom, L. L., et al. (2012). Therapeutic potential for phenytoin: targeting Na(v)1.5 sodium channels to reduce migration and invasion in metastatic breast cancer. Breast Cancer Res. Treat 134, 603–615. doi: 10.1007/s10549-012-2102-9
Yang, M., James, A. D., Suman, R., Kasprowicz, R., Nelson, M., O’Toole, P. J., et al. (2020). Voltage-dependent activation of Rac1 by Nav 1.5 channels promotes cell migration. J. Cell Physiol. 235, 3950–3972. doi: 10.1002/jcp.29290
Yao, W., Feng, D., Bian, W., Yang, L., Li, Y., Yang, Z., et al. (2012). EBP50 inhibits EGF-induced breast cancer cell proliferation by blocking EGFR phosphorylation. Amino Acids 43, 2027–2035. doi: 10.1007/s00726-012-1277-z
Yao, P. L., Morales, J. L., Zhu, B., Kang, B. H., Gonzalez, F. J., Peters, J. M. (2014). Activation of peroxisome proliferator-activated receptor-beta/delta (PPAR-beta/delta) inhibits human breast cancer cell line tumorigenicity. Mol. Cancer Ther. 13, 1008–1017. doi: 10.1158/1535-7163.MCT-13-0836
Yeo, S. K., Guan, J. L. (2017). Breast Cancer: Multiple Subtypes within a Tumor? Trends Cancer 3, 753–760. doi: 10.1016/j.trecan.2017.09.001
Yi, M., Huo, L., Koenig, K. B., Mittendorf, E. A., Meric-Bernstam, F., Kuerer, H. M., et al. (2014). Which threshold for ER positivity? a retrospective study based on 9639 patients. Ann. Oncol. 25, 1004–1011. doi: 10.1093/annonc/mdu053
Zha, C., Brown, G. B., Brouillette, W. J. (2014). A highly predictive 3D-QSAR model for binding to the voltage-gated sodium channel: design of potent new ligands. Bioorg. Med. Chem. 22, 95–104. doi: 10.1016/j.bmc.2013.11.049
Zhang, B., Deng, Z., Zeng, B., Yang, S., Chen, X., Xu, X., et al. (2018). In-vitro effects of the FS50 protein from salivary glands of Xenopsylla cheopis on voltage-gated sodium channel activity and motility of MDA-MB-231 human breast cancer cells. Anticancer Drugs 29, 880–889. doi: 10.1097/CAD.0000000000000662
Zhou, W., Slingerland, J. M. (2014). Links between oestrogen receptor activation and proteolysis: relevance to hormone-regulated cancer therapy. Nat. Rev. Cancer 14, 26–38. doi: 10.1038/nrc3622
Zhou, Y., Wong, C. O., Cho, K. J., van der Hoeven, D., Liang, H., Thakur, D. P., et al. (2015). SIGNAL TRANSDUCTION. Membrane potential modulates plasma membrane phospholipid dynamics and K-Ras signaling. Science 349, 873–876. doi: 10.1126/science.aaa5619
Keywords: VGSCs, Nav1.5, breast cancer, metastasis, mechanism, inhibitors
Citation: Luo Q, Wu T, Wu W, Chen G, Luo X, Jiang L, Tao H, Rong M, Kang S and Deng M (2020) The Functional Role of Voltage-Gated Sodium Channel Nav1.5 in Metastatic Breast Cancer. Front. Pharmacol. 11:1111. doi: 10.3389/fphar.2020.01111
Received: 17 January 2020; Accepted: 08 July 2020;
Published: 23 July 2020.
Edited by:
Lin-Hua Jiang, University of Leeds, United KingdomReviewed by:
Mustafa Bilgin Djamgoz, Imperial College London, United KingdomCopyright © 2020 Luo, Wu, Wu, Chen, Luo, Jiang, Tao, Rong, Kang and Deng. This is an open-access article distributed under the terms of the Creative Commons Attribution License (CC BY). The use, distribution or reproduction in other forums is permitted, provided the original author(s) and the copyright owner(s) are credited and that the original publication in this journal is cited, in accordance with accepted academic practice. No use, distribution or reproduction is permitted which does not comply with these terms.
*Correspondence: Meichun Deng, ZGVuZ21jaEBjc3UuZWR1LmNu
†These authors have contributed equally to this work
Disclaimer: All claims expressed in this article are solely those of the authors and do not necessarily represent those of their affiliated organizations, or those of the publisher, the editors and the reviewers. Any product that may be evaluated in this article or claim that may be made by its manufacturer is not guaranteed or endorsed by the publisher.
Research integrity at Frontiers
Learn more about the work of our research integrity team to safeguard the quality of each article we publish.