- Instituto de Biología y Genética Molecular (IBGM), Universidad de Valladolid and Consejo Superior de Investigaciones Científicas (CSIC), Valladolid, Spain
Cancer, the second cause of death worldwide, is characterized by several common criteria, known as the “cancer hallmarks” such as unrestrained cell proliferation, cell death resistance, angiogenesis, invasion and metastasis. Calcium permeable channels are proteins present in external and internal biological membranes, diffusing Ca2+ ions down their electrochemical gradient. Numerous physiological functions are mediated by calcium channels, ranging from intracellular calcium homeostasis to sensory transduction. Consequently, calcium channels play important roles in human physiology and it is not a surprise the increasing number of evidences connecting calcium channels disorders with tumor cells growth, survival and migration. Multiple studies suggest that calcium signals are augmented in various cancer cell types, contributing to cancer hallmarks. This review focuses in the role of calcium permeable channels signaling in cancer with special attention to the mechanisms behind the remodeling of the calcium signals. Transient Receptor Potential (TRP) channels and Store Operated Channels (SOC) are the main extracellular Ca2+ source in the plasma membrane of non-excitable cells, while inositol trisphosphate receptors (IP3R) are the main channels releasing Ca2+ from the endoplasmic reticulum (ER). Alterations in the function and/or expression of these calcium channels, as wells as, the calcium buffering by mitochondria affect intracellular calcium homeostasis and signaling, contributing to the transformation of normal cells into their tumor counterparts. Several compounds reported to counteract several cancer hallmarks also modulate the activity and/or the expression of these channels including non-steroidal anti-inflammatory drugs (NSAIDs) like sulindac and aspirin, and inhibitors of polyamine biosynthesis, like difluoromethylornithine (DFMO). The possible role of the calcium permeable channels targeted by these compounds in cancer and their action mechanism will be discussed also in the review.
Introduction
Cancer is the second leading cause of death globally, causing 9.6 million deaths in 2018 with an increasing estimation of 70% over the next twenty years. There are more than 100 cancer types, affecting any part of the body in both sexes. However, the most common cancer types in women are: breast (25.4%), colorectal (9.6%), lung (8.8%), cervix (6.9%) and thyroid (6.3%), meanwhile lung (15.5%), prostate (14.5%), colorectal (11.4%), liver (6.8%) and bladder (4.8%) are the most common among men (https://www.wcrf.org); (https://www.who.int). Every type of cancer is characterized by its unique clinical features, molecular markers and a disease-specific profile of genes. Nevertheless, all cancer types meet several common criteria, known as the “cancer hallmarks” described in 2000 by Hanahan and Weinberg (Hanahan and Weinberg, 2000). Although, new emerging cancer hallmarks were added in the last decade, the most accepted hallmarks are: (1) sustained proliferation, (2) cell death resistance, (3) tissue invasion and metastasis and (4) persistent angiogenesis.
Since the first hypothesis by the mid-1800s, that “canalis” are present in biological membranes (Brücke, 1847), numerous physiological functions have emerged mediated by ion channels, ranging from control cellular ionic homeostasis to sensory transduction. Consequently, ion channels perform key roles in human physiology and it is not a surprise the increasing number of evidences connecting channels disorders with a broader human diseases. Nowadays, abundant evidence demonstrate that calcium, calcium permeable channels and calcium signals, play important functions in cancer cells proliferation, apoptosis resistance, invasion and drug resistance, common cancer hallmarks. The first direct evidences connecting calcium channels and cancer were published in the 1980s, when independent studies confirmed the inhibition of cancer growth using particular calcium channels blockers (Lee et al., 1988; Batra and Alenfall, 1991; Taylor and Simpson, 1992). The ion channels research not only confirm the calcium channels correlation with cancer. Other ion channels with important roles in membrane potential or cell volume regulation, including Na+, K+ and Cl− channels have been involved in the development of one or more cancer hallmarks (Pardo, 2004; Wang, 2004; Kunzelmann, 2005; Wulff et al., 2009; Schwab et al., 2012; Prevarskaya et al., 2018). At the present day, the relationship between altered expression and/or function of particular ion channels and cancer is well stablished, so well that the term “oncochannels” has been recently introduced (Huber, 2013).
This review focus in the role of calcium permeable channels signaling in cancer: from molecular mechanisms to therapeutics. Even though cancer therapies and drugs improved significantly in the last years, new and more efficient treatments are still needed to fight against such indiscriminate disease. Calcium channels and transporters are widely expressed in the plasma membrane of carcinoma cells where they may represent a good therapeutic target. For this reason, the study of ion channels role in both initial and advanced stages of the disease, should be useful to explore the possible clinical value of this membrane proteins as novel targets for therapy.
In this review we will consider the role of calcium permeable channels signaling in cancer with special attention to the mechanisms behind the remodeling of the calcium signals. The calcium channel modulators and their therapeutic use in treating cancer disease will be also discussed in the review.
Calcium Permeable Channels In Plasma Membrane, Endoplasmic Reticulum and Mitochondria
Calcium permeable channels are membrane proteins located in the external and internal cell membranes, including plasma membrane (PM), and endoplasmic reticulum (ER) or mitochondria, respectively. These channels diffuse passively Ca2+ ions down its electromechanical gradient from extracellular space and from intracellular calcium stores to the cytoplasm. At the PM there is a wide diversity of calcium channel types, characterized by their activation mechanism: (1) Voltage-gated calcium channels (VGCCs), (2) receptor-operated calcium channels (ROCCs), (3) store-operated calcium channels (SOCCs), (4) transient receptor potential channels (TRPs), (5) acid-sensing ion channels (ASICs) and (6) stretch-activated ion channels (SAICs). VGCCs are the major participants in the Ca2+ entry mechanism in excitable cells, including neurons, different types of muscle cells and some endocrine cells. In contrast, the main pathway for the Ca2+ influx in non-excitable cells such as epithelial cells from most carcinomas is performed by SOCCs (Figure 1). These channels generate the store-operated calcium entry (SOCE), originally called capacitative calcium entry. SOCCs open when ER Ca2+ stores are decreased from resting levels around 700 µM to about 200 µM (Alvarez and Montero, 2002; Villalobos et al., 2017). This partial depletion is sensed by the ER membrane-located Stromal Interaction Molecule 1 (STIM1) (Liou et al., 2005), when Ca2+ ions dissociate from its low affinity EF-Ca2+ binding domain. The decrease in ER-Ca2+ levels promotes STIM1 oligomerization and the interaction with the PM-localized calcium channel ORAI1 and/or TRP channels (Feske et al., 2006; Fahrner et al., 2017; Hernández-Morales et al., 2017; Secondo et al., 2018). The STIM1–ORAI1 interaction opens calcium release activated channels (CRAC), thus facilitating the calcium influx and the ER Ca2+-stores refilling for subsequent stimuli (Villalobos et al., 2017). In mammals, ORAI1 channel has two other isoforms, ORAI2 and ORAI3 encoded by homologous genes (Feske et al., 2006) and STIM1 only one STIM2 (Putney, 2018). In addition, there are two variants of ORAI1, the longer ORAI1α and the shorter ORAI1β, due to different translation points, with different membrane diffusion coefficients (Fukushima et al., 2012). The three ORAI isoforms can be activated by STIM1 with some variations in the pharmacology and biophysical properties of the channel (Lis et al., 2007; DeHaven et al., 2008).
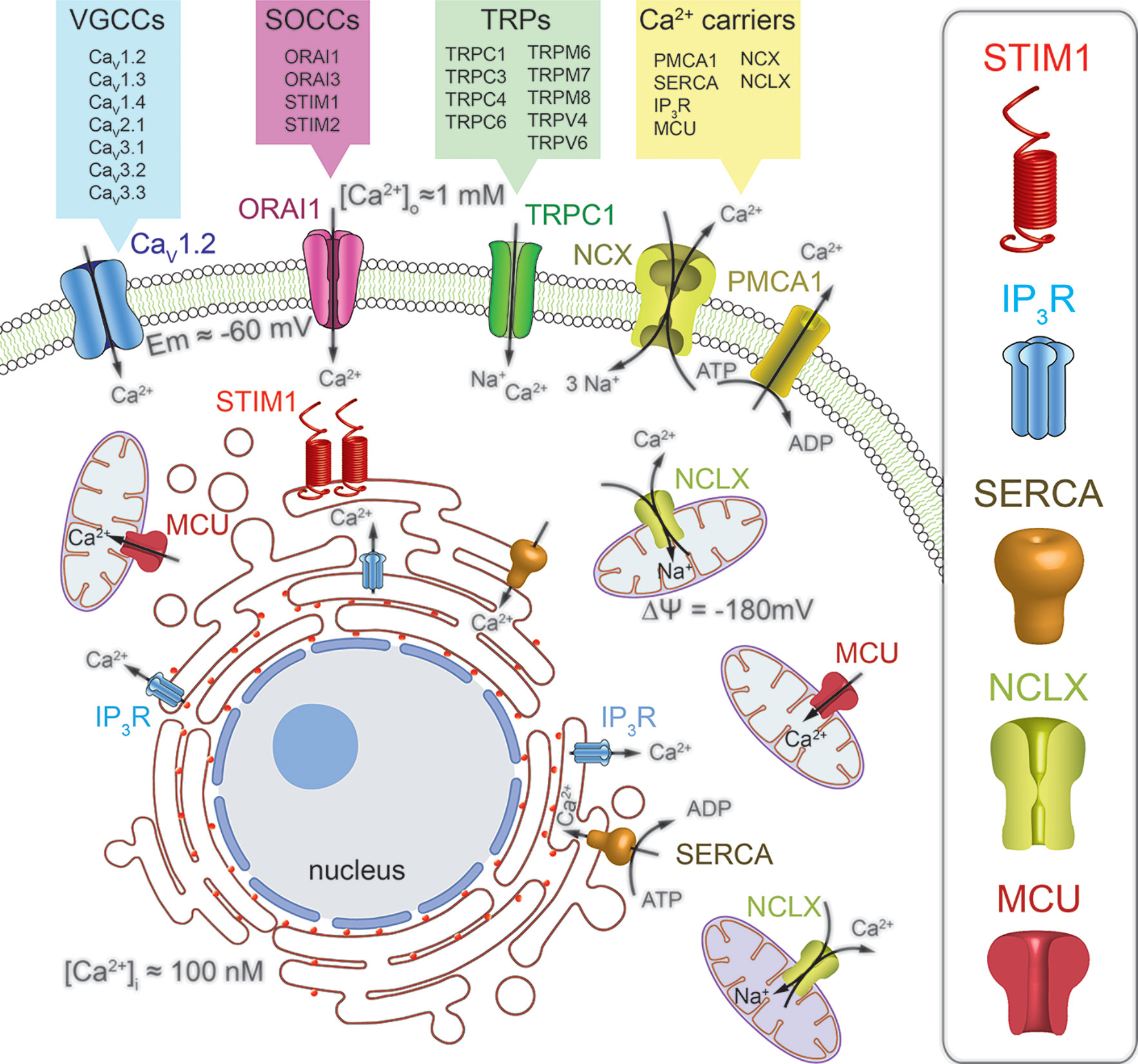
Figure 1 Schematic representation of the main calcium signaling pathways through Ca2+ channels and transporters involved in the regulation of calcium homeostasis in mammalian cells. The calcium influx pathway from the extracellular space is mediated by the combined action of the voltage gated calcium channels (VGCCs), the store-operated calcium channels (SOCCs) and associated proteins (STIM) and the transient receptor potential (TRPs) Ca2+ permeable channels. The calcium release pathway from the endoplasmic reticulum (ER) and mitochondria is mediated by the inositol triphosphate receptors (IP3R) and the mitochondrial Na+/Ca2+ exchanger (NCLX), respectively. Furthermore, the sarcoplasmic reticulum Ca2+-ATPase (SERCA) and the mitochondrial Ca2+ uniporter (MCU) are the responsible for the free cytoplasmic Ca2+ sequestration into organelles. Finally, the Ca2+ extrusion pathway from the cytoplasm is regulated by the plasma membrane Ca2+ATPase (PMCA1) and the Na+/Ca2+ exchanger (NCX).
Ca2+ release mechanisms in the ER are mediated by two major Ca2+ permeable channels: (1) ryanodine receptors (RyRs) (Fleischer et al., 1985) and (2) inositol trisphosphate (IP3) receptors (IP3Rs) (Berridge, 1983; Streb et al., 1983) (Figure 1). RyRs open mainly when intracellular Ca2+ rises, thus acting as a ligand in a mechanism called, Ca2+-induced Ca2+ release (CICR) (Endo et al., 1970). ER-Ca2+ release by IP3Rs starts at the PM, where agonist-induced G-protein-coupled receptors (GPCRs) activates the phospholipase C (PLC) enzyme. The PLC selectively induces the hydrolysis of the phosphatidylinositol 4,5-bisphosphate (PIP2), releasing diacylglycerol (DAG) and the second messenger IP3, which diffuses to the ER activating its receptor (Berridge, 1987).
In mitochondria, the principal calcium channel is the mitochondrial calcium uniporter (MCU), a Ca2+-activated, calcium channel, whose molecular machinery has been recently reported (Perocchi et al., 2010; Baughman et al., 2011; Sancak et al., 2013). MCU opens when large [Ca2+] microdomains develop at the mouth of this Ca2+-operated, calcium channel. The huge mitochondrial potential (ΔΨ), close to −180 mV, promotes the Ca2+ removal from cytosol into mitochondria (Valero et al., 2008; Villalobos et al., 2017), clearing large cytosolic Ca2+ loads formed during VGCCs opening in excitable cells (Villalobos et al., 2001) or during Ca2+ release from the ER (Rizzuto et al., 1998). In various cellular types, SOCE regulation by mitochondria depends on the Ca2+ buffering capability of these organelles, being able to prevent the slow, Ca2+-dependent inactivation of Ca2+ release activated calcium channels (CRAC) (Gilabert, 2000; Hernández-Morales et al., 2017).
Calcium Permeable Channels In Cancer
Voltage-Gated Calcium Channels
Recent data provide evidence of the importance of the calcium permeable channels in cancer. Regarding VGCCs, altered gene expression profiles has been related with different cancer types (Wang et al., 2015a) and it has been proved that different calcium channels blockers prevent cancer invasion (Buchanan and McCloskey, 2016; Jacquemet et al., 2016).
Experimental and bioinformatic analysis showed that different subunits of VGCCs such as CaV1.2, CaV1.3, CaV2.2, CaV3.1, Cav3.2 and CaV3.3 are involved in the development and progression of different types of cancer, showing a significantly overexpression in breast cancer. CaV1.4 only showed up-regulation in testis cancer, whereas CaV2.1, CaV1.2 and CaV1.3 were deeply overexpressed in a wide range of cancer types (Wang et al., 2015a). More specifically, the high voltage-activated CaV1.2 channel, commonly localized in cardiac, skeletal, smooth muscle, neurons, fibroblasts and pancreatic cells (Lipscombe et al., 2004; Tajada et al., 2013; Dixon et al., 2015), is overexpressed in most cancer types, including colorectal, gastric, leukemia, brain, uterus, breast, pancreatic, sarcoma, skin and prostate (Wang et al., 2015a). The CaV1.3 is expressed in most of the same cells that express CaV1.2, as well as, neuroendocrine, amacrine cells, auditory hair cells, photoreceptors and the sinoatrial node where it contributes to pacemaking (Platzer et al., 2000; Lipscombe et al., 2004; Moreno et al., 2016). The CaV1.3 is highly expressed in most types of cancer, including breast and prostate cancer, but also in brain, colorectal, gastric, bladder, lung, esophageal and uterine tumors (Chen et al., 2014; Wang et al., 2015a; Buchanan and McCloskey, 2016; Jacquemet et al., 2016). See Table 1 for a summary of calcium permeable channels involved in different cancer conditions. Furthermore, the carboxy terminus cleavage of both Cav1.2 and Cav1.3 promotes the altered expression of different proteins such as TRPV4 and small conductance potassium channels (SK3 and SK2) through the CCAT transcription factor or by direct translocation to the nucleus (Gomez-Ospina et al., 2006; Gomez-Ospina et al., 2013; Lu et al., 2015). Hence, additionally to the Ca2+ influx cancer-related signaling, both c-terminus can contribute to cancer hallmarks by modulation of other channels. It is also important to highlight the number of protein interaction sites described in the VGCCs, where different proteins modulate transcription factors such as CREB, NFAT and calmodulin, consequently linking ion channels with transcription factors involved in cancer progression (Mancini and Toker, 2009; Xiao et al., 2010; Berchtold and Villalobo, 2014).
Moreover, compelling studies point to the emerging role of VGCCs accessory subunits in cancer (Haworth and Brackenbury, 2019). The VGCCs are associated with these non-pore forming subunits that tune the biophysical properties of the channel, including activation, gating kinetics or trafficking to the PM (Dolphin, 2016). The increased expression of the CaV1.x and the CaV2.x accessory subunits, α2δ and β, have been related with different cancer hallmarks in liver, ovary, prostate, pancreas, lung, and colon tumors (Mitra et al., 2011; Cromer et al., 2015; Warnier et al., 2015; Yu et al., 2016; Badr et al., 2018; Gao et al., 2018). The involvement of these subunits in cancer adds an extra complexity to the VGCCs- cancer link mechanism.
TRP Calcium Permeable Channels
The Ca2+ permeable members of the TRP channel superfamily, which have a high diversity of gating mechanism (Nilius and Owsianik, 2010; Gees et al., 2012), are differently regulated in some cancer types. The remodeling of Ca2+ entrance and release pathways favors the phenotypic switch from normal to high proliferative state. For example, the expression of several TRP channels is elevated in different common carcinomas, e.g. TRPC1 in breast cancer (Dhennin-Duthille et al., 2011), TRPC3 in some breast and ovarian tumors (Aydar et al., 2009; Yang et al., 2009a; Tao et al., 2013), TRPC6 in breast, liver, stomach and glia cancers (El Boustany et al., 2008; Aydar et al., 2009; Cai et al., 2009; Ding et al., 2010; Dhennin-Duthille et al., 2011; Wen et al., 2016; Diez-Bello et al., 2019), TRPM7 in breast, pancreas, ovarian and gastric cancers (Hoshino et al., 1988; Kim et al., 2008; Kim et al., 2012; Middelbeek et al., 2012; Rybarczyk et al., 2012; Wang et al., 2014; Yee et al., 2015). TRPM8 is highly expressed at both mRNA and protein levels in tumor cells from different tissues: breast, pancreas, prostate, colorectal and lung (Schmidt et al., 2006; Chodon et al., 2010; Yee et al., 2010; Liu et al., 2014), but the expression is decreased during metastasis in prostate cancer cells via TCAF1 associated factor (Gkika et al., 2015). In this regard, a new pore-independent mechanism has been revealed, where TRPM8 inhibit endothelial cell migration via Rap1, a small GTPase, protein-protein interaction (Genova et al., 2017). TRPV6 expression levels are significantly high in different human carcinomas tissues, such as, breast, prostate, thyroid, colon and ovary (Wissenbach et al., 2001; Zhuang et al., 2002; Bolanz et al., 2008; Dhennin-Duthille et al., 2011). In contrast, the expression of other TRP channels appears to be decreased in cancer. For instance, TRPC4 channels in renal carcinoma (Veliceasa et al., 2007), TRPM6 in colorectal tumors (Xie et al., 2018), TRPV4 in skin and bladder tumor cells (Fusi et al., 2014; Mizuno et al., 2014; Yu et al., 2019) or the TRPV6 in lung carcinomas (Fan et al., 2014). In conclusion, the TRP channels superfamily expression levels have been described to be either up or down regulated in different tumors (Table 1). The specific molecular mechanism of these channels in cancer is not fully understood, but recent evidence suggests a key role for some of these channels in the cancer hallmarks (Villalobos et al., 2017; Prevarskaya et al., 2018). In addition, some members of the TRPC subfamily contribute to SOCE forming channel complexes with STIM1 and ORAI1 (Molnár et al., 2016; Villalobos et al., 2016). This set of proteins does not associate to form heterohexamers ORAI1/TRPC1/STIM1 complexes. Instead, ORAI1 and TRPC1 subunits form distinctive pore structures each responsible for its calcium selective permeability (Cheng et al., 2013). Further, TRPC1 activation is mediated by STIM1 binding, but TRPC1 trafficking to the membrane depends on ORAI1-based Ca2+ entry. Therefore, ORAI1/STIM1 and TRPC1/STIM1 assemble into two separate channels providing individual calcium signals for regulation of different cell functions (Ambudkar et al., 2017; Villalobos et al., 2018). In fact, there is a long-term controversy on the function of TRPC channels in SOCE. The final consensus is that they may play a role in some cell types but not in others (Ambudkar et al., 2017). In anterior pituitary cells from mice lacking either ORAI1 or the seven TRPC1–7 channels, it was recently shown that SOCE required only ORAI1 channels but not any of the TRPC channels (Núñez et al., 2019). In human colonic cells, it seems that SOCE is mediated only by ORAI1 channels in normal cells. However, in colon cancer cells, SOCE depends on both ORAI1 and TRPC1 channels (Sobradillo et al., 2014) as reviewed next. Other member of the TRP channel superfamily, which is translocated to the plasma membrane via ORIA1-mediated mechanism, is the TRPV6 channel controlling the cancer cell survival (Raphaël et al., 2014).
Store Operated Calcium Channels
The mechanisms through SOCCs members contribute to cancer progression differ according to the molecular entity of the protein and the cancer type-specificity. It is important to remember that SOCCs are not just a single molecular unit, but rather multiprotein complex which includes PM and ER-membrane components, ORAI and STIM proteins respectively. Although calcium signal remodeling involves changes of different Ca2+-permeable channels, Ca2+ entry through ORAI channels is particularly important, as its dysregulation contribute to cancer features such as sustained proliferation, apoptosis resistance and invasion.
ORAI1 key component of SOCE, is essential for human brain tumor glioblastoma invasion. In these cells, the ORAI1 expression is upregulated causing an increased ORAI1-based calcium influx (Motiani et al., 2013a). In this regard, some breast cancer cell lines exhibit augmented levels of ORAI1, SOCE and the remodeling of the calcium influx associated with invasive stimuli (McAndrew et al., 2011; Zhu et al., 2014b). The ORAI1 involvement occurs also in melanoma cells, playing a central role in cell migration and proliferation, most likely via ERK signaling (Umemura et al., 2014). Furthermore, the ORAI1 interaction with SK3, a small conductance calcium-activated potassium channel, has been correlated with invasion and metastasis in colon and breast cancer, respectively, via SigmaR1 chaperone (Gueguinou et al., 2017). In renal carcinomas, the ORAI1 expression levels in cancer tissues were statistically higher than the levels in the adjacent normal parenchymal tissues, promoting cell proliferation and migration (Kim et al., 2014). These results suggest that, regardless the feasible differences in the cancer type-specificity and mechanism, ORAI1 plays a pivotal role in proliferation and invasion. However, this assumption is controversial because other authors propose that proliferation and apoptosis resistance is due to the ORAI3 remodeling. For instance, in prostate cancer cells, the overexpression of ORAI3 promotes two cancer hallmarks at once, enhanced proliferation and apoptosis resistance (Dubois et al., 2014). The ORAI3 increased expression levels favors the formation of ORAI1-ORAI3 heterohexamers over the ORAI1 homohexamers. The shift in the ORAI1/ORAI3 ratio reduces the Ca2+ influx from the extracellular space, promoting the enhanced apoptosis resistance shown by these cells (Abeele et al., 2002). These results highlight the essential role of ORAI1 in death related process (Flourakis et al., 2010). The downregulation of the ORAI1-based SOCE is associated with increased store independent calcium entry (SICE), promoting cancer cells proliferation via the Ca2+/calcineurin-dependent transcription factor (Dubois et al., 2014). The ORAI3 expression is also elevated in breast cancer cells, where enhanced ORAI3-dependent SOCE result in apoptosis resistance, proliferation, and invasion in an estrogen receptor-dependent way (Motiani et al., 2013b). Association between elevated ORAI3 expression, enhanced ORAI3-based SOCE and high proliferation levels was also reported for non-small-cell lung adenocarcinoma (Ay et al., 2013) (Table 1).
The Ca2+ sensor STIM1 is overexpressed in different carcinomas, e.g., glioblastoma, cervix, breast, lung, liver, melanoma and colon (Scrideli et al., 2008; Chen et al., 2011; McAndrew et al., 2011; Li et al., 2013; Yang et al., 2013; Umemura et al., 2014; Wang et al., 2015b; Wang et al., 2016). In fact, STIM1 expression level is enhanced in early-stage cervical cancers, and using STIM1 siRNA, both SOCE and proliferation were inhibited in the cervical cancer cells. Furthermore, there is a positive correlation of local migration, tumor size and angiogenesis with STIM1 levels in cervical cancers cells (Chen et al., 2011). The abolition of STIM1 also prevents the cell proliferation and induces G0/G1 phase arrest of human glioblastoma cells (Li et al., 2013). In alveolar epithelial cells, STIM1 silencing attenuates the tumor growth, arresting cells in G1 phase in a p21 and cyclin D1-mediated pathway (Wang et al., 2016). In the same direction, STIM1 siRNA inhibits the migration and invasion abilities of the highly invasive tumor hepatic cells (Yang et al., 2013). Increased levels of STIM1 promotes growth and migration in colorectal cancer cells (CRC) and STIM1 elevated expression in CRC patients is associated with tumor size, invasion and metastasis (Wang et al., 2015b). Moreover, microarray analysis data show that, the basal-breast cancer, a breast cancer subtype with a very dismal prognosis, is correlated with an altered relationship between the ORAI1 activators STIM1 and STIM2, characterized by high STIM1/STIM2 ratios (McAndrew et al., 2011; Berna-Erro et al., 2017) (Table 1).
STIM1 homolog STIM2 senses smaller changes in ER-Ca2+ concentrations than STIM1, suggesting different cellular functions for these two Ca2+ sensors (Brandman et al., 2007). STIM1 detects deeper ER-Ca2+ depletions, due to its higher affinity for calcium, thus enabling the refilling of the depleted stores, while STIM2 detects lighter ER-Ca+2 depletions, keeping ER-Ca2+ concentrations within tight limits (Berna-Erro et al., 2017). Elevated STIM2 expression levels have been found in different cancer types, colorectal cancer (Aytes et al., 2012), human melanoma (Stanisz et al., 2014) and glioblastoma multiform tumors (Ruano et al., 2006). In colorectal cancer cells the high STIM2 expression levels has been related with a reduced invasive phenotype, suggesting a cancer cell growth suppressor function in contrast to STIM1 (Aytes et al., 2012; Berna-Erro et al., 2017). In the same vein, STIM2 overexpression was detected in human melanoma, where STIM2 siRNA treatment produced enhanced proliferation (Stanisz et al., 2014). These results correlate with the idea that, low STIM1/STIM2 ratios may be associated with less aggressive tumor conditions.
It is noteworthy that, while STIM2 gene expression levels are increased in CRC cells, STIM2 protein is practically absent in tumor cells, leading to the partial depletion of calcium stores (Sobradillo et al., 2014; Villalobos et al., 2016). The STIM2 loss in tumor cells moves internal Ca2+ store concentration near to the SOCE activation threshold and promotes apoptosis resistance and cell survival (Sobradillo et al., 2014). These discrepant results reveal the complexity role of STIM proteins in cancer and expand the classical function of controlling intracellular Ca2+ homeostasis. Additionally, the recent discovery of new STIM2 mRNA isoforms with opposite effects, might clarify these apparently contradictory reports. In these new reports, the novel variant STIM2.1 inhibits SOCE, whereas the former isoform STIM2.2 promotes it (Miederer et al., 2015) as detailed in (Berna-Erro et al., 2017).
Intracelular Calcium Homeostasis
Intracellular Ca2+ concentrations control many different physiological and cellular functions at global and local levels, such as gene transcription in the nucleus, cell respiration and ATP synthesis in mitochondria or exocytosis in plasma membrane. To regulate this wide range of physiological Ca2+-dependent processes, the cells maintain Ca2+ concentrations tightly controlled. This regulation is known as calcium homeostasis.
To keep calcium homeostasis and considering that Ca2+ is a cation and does not cross freely cell membranes, Ca2+ is transported through PM and internal membranes by particular transmembrane proteins, including Ca2+ permeable channels, Ca2+ pumps and ion transporters (Bong and Monteith, 2018; Villalobos et al., 2018). As we mentioned above, Ca2+ permeable channels enable Ca2+ entry into cells forced by the electrochemical gradient, formed by the difference in Ca2+ concentration between the internal (100 nM) and extracellular (1 mM) space and the membrane potential of about −60 mV. The calcium entry rises intracellular [Ca2+] triggering different cell process. Then, it is removed from the cytosol and transported back to the extracellular medium or uptake into the intracellular Ca2+ stores by Ca2+ pumps and transporters, restoring the basal Ca2+ levels (Villalobos et al., 2018) (Figure 1).
This removing-Ca2+ process requires energy provided by the ATP hydrolysis for Ca2+-pumps or the energy stored in the electrochemical gradient of other cation for transporters. For example, the Na+/Ca2+ exchanger transports three Na+ ions down its gradient in exchange of one Ca2+ cation against its electrochemical gradient (Reuter and Seitz, 1968). These dynamic Ca2+ signals differ with regard to their mechanisms of generation, spatial distributions and temporal properties, but are coordinated in space and time for a specific purpose. These calcium signals are characterized in different cell types and include calcium waves (Berridge et al., 2003; Parkash and Asotra, 2010), sparks (Cheng et al., 1993), calcium puffs (Yao et al., 1995), spikes (Meyer and Stryer, 1991), flickers (Wei et al., 2009), sparklets (Wang et al., 2001; Navedo et al., 2005; Tajada et al., 2017), etc. Compelling evidence show that variations in cellular Ca2+ dynamics participate in the normal and pathological signal transduction that controls cell proliferation and survival (Prevarskaya et al., 2018). Abnormal remodeling in Ca2+ homeostasis leads to the disruption of Ca2+ signaling resulting in a wide range of pathological conditions such as cell migration, excessive proliferation, invasion and programmed cell death resistance (Prevarskaya et al., 2011; Monteith et al., 2012; Prevarskaya et al., 2014).
Intracellular Ca2+-storage organelles like the ER, mitochondria and the recently emerged as a major intracellular Ca2+ storage, lysosomes (Lloyd-Evans and Waller-Evans, 2019), also hold their own Ca2+ transporters. The ER is the largest single organelle and the most important intracellular Ca2+ store (Lam and Galione, 2013). It operates as a dynamic Ca2+ store thanks to the combined action of Ca2+ channels and transporters in the ER-membrane, and the Ca2+-binding proteins in the ER-lumen, working as a high-capacity Ca2+ buffering system (Verkhratsky, 2005). Ca2+ is continuously leaking from the ER through leak channels still not identified. Nevertheless, Ca2+ concentration within the ER remains high due to the continuous activity of the endoplasmic reticulum Ca2+-ATPases (SERCA) that replenish Ca2+ stores (MacLennan, 1970) (Figure 1). On the other hand, activation of the IP3Rs, receptor-operated Ca2+ channels, release Ca2+ from intracellular store sites, contributing to the maintenance of a total amount of Ca2+ in the lumen similar to the extracellular space, in the mM range (Alvarez and Montero, 2002; Foskett et al., 2007). Because the lumen contains high concentrations of Ca+2 binding proteins (calreticulin, calsequestrin, annexin, calnexin, etc.) the concentration of free Ca+2 has been estimated to be between 100 and 700 µM (Foskett et al., 2007; Yáñez et al., 2012).
In the mitochondria, the Ca2+ uptake is conducted by the MCU and the Na+/Ca2+ (NCLX) and Ca2+/H+ (HCX) exchangers are responsible for the mitochondrial Ca2+ release in exchange for Na+ and H+, respectively (Bononi et al., 2012; Srinivasan et al., 2015) (Figure 1). A large increase in Ca2+, located near enough the MCU, triggers the influx of calcium to the mitochondrial matrix, rising the mitochondrial [Ca2+] to very high concentrations near the mM level (Pozzan et al., 2000; Contreras et al., 2010). Mitochondria are also in dynamic contact with other organelles including the ER. These transient contacts between ER and mitochondria are essential to generate the highly localized and concentrated Ca2+ microdomains, facilitating the Ca2+ transport into mitochondria (Paupe and Prudent, 2018). Through this mitochondrial-ER crosstalk, IP3Rs control the mitochondrial Ca2+ uptake and therefore metabolism and cell destiny (Cui et al., 2017). Although the mitochondria play an important role in Ca2+ homeostasis, the “in vivo” buffering capacity of this intracellular organelle is not particularly relevant (Pozzan et al., 2000).
Mitochondria not only modulate cytosolic Ca2+ signal. Mitochondrial calcium is also involved in the control of metabolism, ATP production and the regulation of cell death. Mitochondrial calcium regulates three important enzymes of the Krebs cycle: ketoglutarate, isocitrate, and pyruvate dehydrogenase. The effect of mitochondrial Ca2+ accumulation in Krebs cycle is the increase in the respiration rate and ATP synthesis (Mammucari et al., 2018). On the other hand, an excess of mitochondrial Ca2+ uptake, known as “mitochondrial calcium overload” triggers mitochondrial permeability transition pore (mPTP) opening. This is a high-conductance channel mediating mitochondrial swelling (Petronilli et al., 1993). This, together with other factors, triggers mitochondrial permeability transition, disruption of the mitochondrial potential and a massive release of cytochrome c, leading to the apoptosome activation and apoptosis (Giorgi et al., 2012; Mammucari et al., 2018).
The plasmalemma also displays many different calcium channels, ATP pumps and transporters to maintain the intracellular Ca2+ homeostasis. We have mentioned above the channels responsible of the Ca2+ influx. Now we will briefly describe the players involved in the transport of calcium out of the cell to maintain the Ca2+ electrochemical gradient. Two players in the Ca2+ outflow from cells have been described, the Na+/Ca2+ exchange transporter (NCX) and the plasma membrane Ca2+-ATPase (PMCA) (Figure 1). The NCX is in charge of Ca2+ extrusion from the cytoplasm against its electrochemical gradient without ATP consumption, in fact, the electrochemical gradient of sodium stores that energy. In addition, NCX can operate in reverse mode contributing to Ca2+ entry in different conditions, such as cellular activation (Blaustein and Lederer, 1999). The PMCA pump with a 1:1 Ca2+/ATP stoichiometry possesses a high-affinity but a low-capacity for Ca2+ transport, in total opposition to NCX (Noble and Herchuelz, 2007). This characteristic has conditioned the roles of these Ca2+ transporters. The PMCA performs a housekeeping role maintaining cytosolic Ca2+ around its basal level, whereas the NCX eliminates significant rises in intracellular Ca2+, but this model has been revised during the latest years. See (Brini and Carafoli, 2011) for review.
Calcium Permeable Channel Modulators and Cancer
Voltage-Gated Ca2+ Channel Modulators and Cancer
Several epidemiological investigations report that, calcium channel blockers used for the medical therapy of other pathologies are related with a reduced prostate cancer risk (Debes et al., 2004; Rodriguez et al., 2009), or at least a significantly tumor aggressiveness reduction (Poch et al., 2013). There is more discrepancy in studies of other cancer types, ranging from significant diminution in breast cancer risk (Fitzpatrick et al., 1997; Taylor et al., 2008) to no association between VGCCs blockers and cancer in colorectal, lung and breast tumors (Michels et al., 1998; Boudreau et al., 2008; Devore et al., 2015; Brasky et al., 2017; Bowles et al., 2019), including higher lung cancer risk correlated with exposure to calcium channels blockers (Rotshild et al., 2018).
Although, the potential benefit of the VGCCs blockers needs further preclinical studies, specific VGCCs antagonists, already approved by the FDA and EMA and used in clinical treatments, may be interesting for cancer treatments where individual VGCCs are overexpressed.
CRAC Channel Modulators and Cancer
The identification of CRAC channels modulators has been an important challenge for researchers and pharmaceuticals, since their discovery in 1992 (Hoth and Penner, 1992), because they have been implicated in numerous human pathologies, including pancreatitis, immunodeficiency, occlusive vascular diseases and cancer (Gerasimenko et al., 2013; Tian et al., 2016; Stauderman, 2018).
Numerous compounds have been described as inhibitors of SOCE or CRAC channels, which can be classified into four groups: inorganics, aptamers, antibodies and organic molecules (Stauderman, 2018). The inorganic lanthanides, specifically the trivalent cations, Lanthanum (La+3) and Gadolinium (Gd+3) are potent but non-selective blockers of the CRAC current (Parekh and Putney, 2005). Both can block directly the CRAC pore formed by the loopI-II of ORAI1, but also block other Ca2+ channels and pumps, such as VGCCs, TRPs and PMCA (Trebak et al., 2002; Yeromin et al., 2006; Cui et al., 2017). However, Gd3+ is normally used in Ca2+ imaging experiments to discriminate endogenous SOCs from other Ca2+ permeable channels such as recombinant TRPs, because the concentration that efficiently blocks the endogenous pathway does not interfere with the TRPs (Trebak et al., 2002; Parekh and Putney, 2005). In addition, these chemical elements are not viable for therapeutic treatment due to toxicological concerns. Aptamer Y1, an artificial single-stranded oligonucleotide engineered to bind with high affinity the first extracellular domain of the ORAI1 protein, block SOCE in the nM range in mast cells (Sun et al., 2016). However, the oligonucleotides present a disadvantage to be consider a potential drug candidate, the administration route is restricted to intravenous, subcutaneous or local delivery, as happened with the antibodies (Stauderman, 2018). Among antibodies, 10F8 (Cox et al., 2013), Anti-CRACM1-IgG (Liu et al., 2017), hHG1/LG1 (Komai et al., 2017) and mAb 2C1.1 (Lin et al., 2013) have been reported to partial block SOCE or CRAC currents due to the low specificity against all ORAI isoforms. Considering the organic small agents, the 2-aminoethoxydiphenylborane (2-APB), a membrane permeable antagonist of the IP3-receptor activity, is widely used is SOCE experiments (Ma et al., 2000). Some studies reported that 2-APB inhibit cell proliferation and death resistance in gastric cancer cells (Sakakura et al., 2003; Cui et al., 2017) and cell migration in CRC cells (Abeele et al., 2002). Although, 2-APB is used as a reliable SOCE inhibitor (Bootman et al., 2002), it presents diverse effects on SOCE or CRAC currents. 2-APB is able to both, inhibit CRAC current without interference in the STIM1 and ORAI1 interaction (Yamashita et al., 2011) and directly activate ORAI3 channel independently of Ca2+ store depletion or STIM1 protein (Schindl et al., 2008). In addition, it presents a dose-dependent bimodal effect, stimulating CRAC currents at low concentration (less than 10 μM) and a transient increase followed by inhibition at higher (up to 50 μM) concentrations (Prakriya and Lewis, 2001). For this reason and because 2-APB modulates other proteins including SERCA (Missiaen et al., 2001), MagNum (Hermosura et al., 2002) and K+ channels (Wang et al., 2002), mitochondrial Ca2+ efflux (Prakriya and Lewis, 2001), gap junctions (Tao and Harris, 2007) and different TRP channels (Parekh and Putney, 2005; Derler et al., 2008), it should not be considered as a SOCE specific inhibitor (Parekh and Putney, 2005; Várnai et al., 2009). However, novel 2-APB derived compounds such as DBB-162AB and DPB-163AE with higher efficiency in SOCE inhibition and without unexpected interactions, are promising chemotherapeutic drugs (Goto et al., 2010).
Another class of small-molecules such as AnCoA4, ML-9, SB01990, KM06293 and RH01882 has been recently identified as SOCE inhibitors via different mechanism. AnCoA4 reduces the ORAI1 recruitment into puncta (Sadaghiani et al., 2014), ML-9 prevents STIM1 migration to ER-PM junctions (Smyth et al., 2008), SB01990, KM06293 and RH01882 modify the ORAI1 pore geometry, altering the Ca2+ selectivity and thereby reducing SOCE (Sampath and Sankaranarayanan, 2016; Cui et al., 2017).
Another compounds, imidazol, tenidap and SFK-96365 all classified as receptor-mediated Ca2+ entry blockers, were reported to inhibit CRAC currents in rat mast cells, but not in a specific manner (Franzius et al., 1994). For example, similar SKF96365 concentration used to block the CRAC currents, inhibits TRPC and TRPM channels with a comparable efficacy. The SKF-96365 was the first ORAI1 blocker used in cancer studies, specifically breast cancer. This imidazol derivative reduced tumor growth and invasion in vivo and inhibited cellular migration in vitro in breast cancer cells (Yang et al., 2009b). In other study, SKF-96365 significantly reduced tumor growth in esophageal cancer cells from immune deficient mouse, blocking ORAI-mediated SOCE and Ca2+ oscillations (Zhu et al., 2014a). Other compound targeting SOCE is the biomolecule Ohmline, a Edelfosine mimetic, that inhibits breast and colon cancer cells migration through the TRPC1/ORAI1/SK3 complex dissociation (Guéguinou et al., 2016).
Pyrazoles, heterocyclic organic compounds, specifically BTP1, BTP2 and BTP3, were identified by Abott and Astellas as SOCE blockers in Jurcat cells, using transcriptional and Ca2+ imaging assays (Djuric et al., 2000; Ishikawa et al., 2003; Stauderman, 2018). BTP2 (also known as Pyr2 or YM58483) is a pyrazole analogue that potently inhibits both TRPC-mediated and CRAC Ca2+ influx (He et al., 2005; Stauderman, 2018). However, similar to 2-APB and imidazole these compounds are not specific because they are involved in many other transport mechanisms. For instance, BTP2 also produces a PM depolarization via TRPM4 activation, therefore decreasing the Ca2+ driving force and consequently the Ca2+ influx inhibition (Takezawa et al., 2006). In contrast, other pyrazol-based compounds such as GSK-7975A (Derler et al., 2013), RO2959 (Chen et al., 2013) and the carboxamide GSK1349571A (Di Sabatino et al., 2009) seem to have reasonable selectivity with no affinity toward a wide range of receptors and ion channels and without affect TRPC1/5-mediated SOCE. Recently developed pyrazole analogues, such as, pyrazole-4-carboxamide (YW2065) and pyrimidine-2(1H)-thione derivative, have reported good anticancer activity in colorectal cancer cell lines compared to the standard drugs like sorafenib or oxaliplatin (Fahmy et al., 2016; Yang et al., 2019). More detailed information about the pharmacology of various CRAC channels modulators is revised in recent reports (Sweeney et al., 2009; Jairaman and Prakriya, 2013; Pevarello et al., 2014; Tian et al., 2016).
Other line of research is using small molecules compounds that modify the unbalance Ca2+ homeostasis in cancer cells. For example, the Nhr-BH4, a BCL-2 mimetic peptide that prevents the inhibition of the IP3R Ca2+ release and thus unblock the apoptosis resistance in breast cancer cells (Nougarede et al., 2018). The TAT-IDPs, other BCL-2 based peptide, potentiates the pro-apoptotic Ca2+ signaling in chronic lymphocytic leukemia cells avoiding the IP3R2 inhibition, an IP3R homolog, (Akl et al., 2013).
The importance of discovering new and specific inhibitors of CRAC channels and the development of new nanotechnology tools (Grolez et al., 2019), could be giant for the treatment of different human diseases. However, future pharmacology studies, and clinical trials will solve if these drugs are viable for therapeutic treatment.
Aspirin, Nsaids and Polyamine Synthesis Blockers as Calcium Channel Modulators In Cancer
Overwhelming evidence provided in the last decades strongly suggest that acetylsalicylic acid (ASA), or aspirin provide protection against several forms of cancer, particularly those in the gastrointestinal tract (Chan et al., 2012; Rothwell et al., 2012; Drew et al., 2016). Same evidence has been also provided for a series of non-steroidal anti-inflammatory drugs (NSAIDs) like ibuprofen, flurbiprofen and sulindac, either alone or, in combination with other compounds like difluoromethylornithin (DFMO), a suicide inhibitor of polyamine biosynthesis (Tegeder et al., 2001; Rostom et al., 2007; Meyskens et al., 2008; Thompson et al., 2010; Chan et al., 2012; Dolejs et al., 2016). Evidence includes multiple data in cell lines and model animals, but also tens of epidemiological studies. Aspirin and NSAIDs inhibit several characteristic cancer cell hallmarks, including unrestrained cell proliferation, cell migration and invasion and cell death resistance. Numerous series of epidemiological evidences suggested that regular use of aspirin and/or several NSAIDs provide significant reduction in the risk of developing several forms of cancer and, particularly colorectal cancer. However, recommendation to use these over the counter drugs for cancer chemoprevention is hindered by the well-known secondary effects and/or risks of the regular use of NSAIDs leading to the conclusion that if, for any other reason or condition, individuals use NSAIDs regularly, they are probably protected against several forms of cancer. For any other situation, recommendation remains at hands of Doctors, that must evaluate whether the risk of taking NSAIDs is worthy in some cases of high risk of cancer. In fact, several studies have concluded that individuals at high risk of developing some forms of hereditary cancer like Lynch Syndrome may benefit of taking aspirin and/or NSAIDs to help protecting them from cancer (Burn et al., 2011; Yurgelun and Hampel, 2018). This may also apply to those patients that have received surgery for removing of polyps and/or tumors in the gastrointestinal tract that are also at high risk of recurrence. In these particular cases, the benefits are supported by several clinical trials that have addressed specifically the treatment and dose. For example, a clinical trial based in the combination of sulindac with DFMO in patients with previous tumors indicate that this combination may reduce nearly 90% the risk of developing recurrences (Meyskens et al., 2008; Sporn and Hong, 2008), revised in (Laukaitis and Gerner, 2011).
The action mechanisms of these drugs involved in preventing cancer cell hallmarks and cancer itself have been addressed in a large series of studies but they remain obscure. Most studies have addressed the contribution of inflammation to carcinogenesis (Balkwill and Mantovani, 2001; Coussens and Werb, 2002) and the benefits of inhibiting inflammatory mechanisms in cancer cell (Hudes et al., 2013; Wu et al., 2013). However, although inflammation definitely contributes to cancer risk, several evidences suggest that additional and/or alternative mechanisms may contribute as well and some relevant mechanisms include modulation of the expression and/or activity of calcium permeable channels in cancer cells (Déliot and Constantin, 2015; Hoth, 2016; Prevarskaya et al., 2018).
Núñez et al. (2006) reported that salicylic acid, the most important metabolite of aspirin, inhibited store-operated Ca2+ entry (SOCE) and SOCE-dependent cell proliferation in colon cancer cells (Núñez et al., 2006; Valero et al., 2008). This effect cannot be attributed to inhibition of cyclooxygenase (COX) as salicylic acid lacks the acetyl moiety involved in COX acetylation, the mechanism involved in COX inhibition by aspirin. Also, it is achieved at concentrations far lower than the necessary to inhibit COX expression. Finally, the effects are similar in cells lacking COX2 expression implying that an alternative target is involved. On the other hand, same results are obtained by other NSAIDs including ibuprofen, indomethacin, sulindac and R-flubiprofen, the enantiomer unable to inhibit COX activity. Accordingly, salicylate and other NSAIDs inhibit SOCE and cell proliferation independently of COX activity and/or gene expression. Since SOCE is critical for cell proliferation, these drugs inhibit cell proliferation more likely because they inhibit SOCE rather than inflammatory pathways. This paradoxical view could be reconciled taken into account that SOCE may also contribute to inflammatory pathways as it is key in the activation of all cell types, including inflammatory cells. Importantly, inhibition of cell proliferation by salicylic acid is counteracted simply by enhancing extracellular Ca2+ just to restore Ca2+ entry in colon cancer cells. When taken together, these data indicate that salicylate (aspirin) and NSAIDs prevent cell proliferation in tumor and non-tumoral cells by inhibiting SOCE in a COX-independent manner.
In search for the mechanism of inhibition of SOCE by salicylate and other NSAIDs, Villalobos and cols. tested the effects of these drugs directly on Ca2+-release activated currents (CRAC) responsible for SOCE in rat basophilic leukemia cells (RBL) and other cell types (Muñoz et al., 2011). These currents display a strong Ca2+-dependent inactivation (Hoth et al., 1997; Fierro and Parekh, 2000) that render them unable to operate in a few seconds unless nearby mitochondria take up this calcium and sustain the current. In this sense, CRAC channels are dictated not only by the filling state of intracellular Ca2+ stores but also by mitochondria (Villalobos et al., 2018). Inhibition of the MCU, the calcium channel responsible of mitochondrial Ca2+ uptake, with ruthenium salts derivatives like RU386 or mitochondrial uncouplers like FCCP, that collapse the mitochondrial potential, the driving force for mitochondrial Ca2+ uptake, inhibit mitochondrial ability to take up Ca2+, thus leading to Ca2+-dependent inactivation of CRAC channels (Villalobos et al., 2019). Similar results have been obtained by MCU downregulation (Samanta et al., 2019). Salicylate and other NSAIDs are carboxylic acids bound to aromatic rings, the typical chemical structure of mitochondrial uncouplers. Accordingly, these drugs are able to decrease the mitochondrial potential or even collapsing it depending on concentration (Muñoz et al., 2011; Scatena, 2012; Hernández-Morales et al., 2017). As a consequence, at low concentrations they work as mild mitochondrial uncouplers that prevent mitochondrial Ca2+ uptake, leading to the Ca2+-dependent inactivation of CRAC channels and SOCE inhibition in RBL cells. In conditions of high intracellular concentration of the Ca2+ buffer, when the Ca2+-dependent inactivation cannot be achieved regardless of mitochondria, neither salicylate, NSAIDs nor MCU removal promote CRAC inactivation or SOCE inhibition. Therefore, salicylate and selected NSAIDs do not inhibit CRAC channels directly. Instead, these drugs promote the Ca2+-dependent inactivation of these channels by preventing the Ca2+ uptake by mitochondria exactly as if we remove the MCU (Villalobos et al., 2019). These same results have been obtained also in other cell types as well, including vascular smooth muscle cells (VSMCs) (Muñoz et al., 2011; Muñoz et al., 2013) and colonic cells both normal and tumoral (Hernández-Morales et al., 2017).
Interestingly, the effects of salicylate and NSAIDs are achieved at very low concentrations in normal VSMCs leading to a full arrest of cell proliferation. VSMCs undergo phenotypic remodeling in different physiopathological situations. For example, upon damage, VSMCs may transform from differentiated, contractile cells to a proliferative and migrating phenotype that restores injury and returns to the differentiated phenotype. This process helps restoring damaged vessels but it may not be as beneficial if excessive proliferation occludes vessels as it happens in restenosis. This transdifferentation process is mediated by a change in the prevalence of different Ca2+-permeable channels. In the differentiated phenotype, voltage-gated channels sensitive to dihydropyridines are prevalent. However, in the proliferative state, store-operated Ca2+ channels take the lead. These channels show strong Ca2+-dependent inactivation that is prevented by mitochondria. Accordingly, low concentrations of salicylate and NSAIDs inhibit SOCE and cell proliferation in these cells during the proliferative phenotype but have no effect on Ca2+ entry during the differentiated phenotype. In fact, they have been proposed for treatment of restenosis, or excessive VSMC proliferation after stent implantation in coronary arteries (Weber et al., 2000; Koo et al., 2007; Dannoura et al., 2014).
As stated above, sensitivity of store-operated channels to aspirin and NSAIDs depends on mitochondria. As mitochondria from normal and tumor cells differ because of the Warburg effect, perhaps mitochondrial control of store-operated channels may be different as well in normal and colon cancer cells. Hernández-Morales et al. (2017) reported recently that this is indeed the case, at least in normal and colon cancer cells. Mitochondria from normal cells are not powerful enough to remove completely the Ca2+-dependent inactivation of store-operated channels. However, in cancer cells, probably because of the Warburg effect, mitochondria display enhanced mitochondrial potential and remove efficiently Ca2+, thus preventing efficiently the Ca2+-dependent inactivation of store-operated channels and sustaining Ca2+ entry (Hernández-Morales et al., 2017). Supporting this idea, the RNA expression analysis of genes involved in mitochondrial Ca2+ transport indicate that, MCU positive-modulator genes are overexpressed while negative-modulators are underexpressed in colon cancer cells (Pérez-Riesgo et al., 2017).
As stated above in the review, another difference between normal and colon cancer cells is that SOCE in normal colonic cells involves only ORAI1-mediated CRAC channels. In contrast, in colon cancer cells, SOCE is mediated by both ORAI1 and TRPC1 channels. Whereas ORAI1 channels in normal cells are essentially inactivating, in colon cancer cells, perhaps the involvement of TRPC1 channels may contribute to sustain currents. A possibility recently pointed out is that Na+ influx mediated by TRPC1 channels may favor activity of the mitochondrial Na+/Ca2+ exchanger, in turn preventing mitochondrial Ca2+ overload and the ensuing formation of reactive oxygen species (ROS) and ROS-dependent inactivation of ORAI1 channels (Quintana et al., 2011; Villalobos et al., 2018). This form of ROS-dependent regulation of ORAI1 channels remains controversial (Samanta et al., 2018; Samanta et al., 2019; Parekh, 2019).
In addition to aspirin and NSAIDs, other drugs have been reported to provide chemoprotection against several forms of cancer, particularly colorectal cancer. The most critical compound is DFMO, also called eflornithine. DFMO is considered a suicide inhibitor of ornithine decarboxylase 1 (ODC1), the critical enzyme in the biosynthesis pathway of the main mammalian polyamines: putrescine, spermine and spermidine. This enzyme is usually overexpressed in cancer cells, thus explaining the increased levels of polyamines that may contribute to cancer cell hallmarks, including enhanced cell proliferation, migration and invasion (Luk and Baylin, 1984; Pegg, 1988; Giardiello et al., 1997; Weiss et al., 2002). Several authors have reported ODC1 polymorphisms and robust correlation between specific polyamines and tissue growth (Martínez et al., 2003; Pegg, 2016; Wang et al., 2017; Sánchez-Jiménez et al., 2019). Finally, different growth stimuli including tumor promoters are able to enhance ODC activity and tumor formation (Bachmann and Geerts, 2018; Gerner et al., 2018). These effects are inhibited by DFMO, including inhibition of colon carcinogenesis in cancer models, such as the ApcMin/+ mice with high ODC levels and polyamines in the gastrointestinal tract. Interestingly, combinations of DFMO and NSAIDs have synergistic effects and inhibit dramatically carcinogenesis in both the small and large intestines of these mice. Recent clinical trials indicate that DFMO prevents CRC, especially if combined with sulindac, a carboxylic NSAID (Meyskens et al., 2008; Thompson et al., 2010; Burke et al., 2016).
Recent data reported by Gutierrez et al., suggest that DFMO may inhibit carcinogenesis by partially reversing the remodeling of store-operated Ca2+ channels in colorectal cancer cells (Gutiérrez et al., 2019). As stated above, colorectal cancers undergo a remodeling of intracellular Ca2+ homeostasis consisting in enhanced store-operated Ca2+ entry and decreased Ca2+ store content that contributed to cancer cell hallmarks, including enhanced cell proliferation, invasion and survival (Sobradillo et al., 2014; Villalobos et al., 2016; Villalobos et al., 2017). Villalobos and cols. have proposed that, during carcinogenesis, colorectal cancer cells may switch from a small, transient SOCE based on ORAI1-dependent CRAC channels (normal cells) to large and sustained currents depending on both ORAI1 and TRPC1 channels (tumor cells) (Villalobos et al., 2016; Villalobos et al., 2017; Villalobos et al., 2019). Interestingly, DFMO treatment inhibits cancer cell hallmarks in these cells including cell proliferation and cell death resistance. These effects are associated to SOCE inhibition and enhancement of Ca2+ store content. Therefore, DFMO may inhibit cancer cell hallmarks by reversing Ca2+ remodeling in colorectal cancer cells (Gutiérrez et al., 2019). Further research revealed that DFMO treatment inhibits TRPC1 expression and eliminates the TRPC1 component of the store-operated currents in colon cancer cells. These effects are reversed in the presence of polyamine putrescine (Gutiérrez et al., 2019). Collectively, these data suggest that polyamines promote Ca2+ channel remodeling by inducing expression of TRPC1 channels and this change promotes cancer cell hallmarks. In fact, this process may simply represent the exacerbation of the physiological process known as epithelial restitution that is mediated by transient polyamine synthesis induce by epithelial damage (Rao et al., 2012). While in physiological conditions this is a transient process limited by the repair of the wound epithelium, in cancer cells this process may became chronic due to ODC overexpression associated to activation of c-myc oncogene secondary to mutations in APC.
As stated above, combination of DFMO plus sulindac prevents efficiently colorectal cancer in high risk patients. An ongoing, large scale, three phase clinical trial is presently testing this treatment for patients at high risk of CRC (S0820, Adenoma and Second Primary Prevention Trial—Full Text View—ClinicalTrials.gov). Interestingly, Gutierrez et al. have reported that the combination of DFMO plus sulindac removes not only the TRPC1 component of the store-operated currents but also the ORAI1-dependent, thus fully abolishing the current. The mechanisms are different. The TRPC1-dependent current is removed at the expression level. However, the ORAI1-dependent current is simply inactivated because the mitochondria-dependent potentiation of the current is prevented. In summary, these data suggest a critical role for Ca2+ channel remodeling in cancer and provide a molecular mechanism of cancer chemoprevention targeting the remodeling of ion channels.
Concluding Remarks
It is clearly evident in the literature, that Ca2+-permeable channels, transporters and pumps play important roles in a wide range of cancer-related process. The remodeling of these Ca2+-permeable channels contributes to the Ca2+-homeostasis dysregulation and both regulate several of the well-known cancer hallmarks. The influence of Ca2+ and other ion channels in carcinogenesis is so evident, that cancer was recently described as a channelopathy. This is a relative new field, however important advances in the identification of mechanism relating ion channels and cancer has been done. For this reason, ion channels represent a novel and possibly important clinical targets for some cancer types, with only a few channel blockers tested in clinical trials.
The use of ion channels modulators as chemotherapeutic agents has their pros and cons. Most of the Ca2+-permeable channels are ubiquitously expressed in different tissues, playing an important role in normal cell function. This is why, the pharmacological modulation of a specific cancer-related channel in tumor cells may produce significant toxicity in normal cells. Further research is required to take full advantage of channel modulators for cancer prevention, diagnosis and treatment.
Author Contributions
Both authors contributed equally to the writing of the manuscript.
Funding
This work has been supported by competitive grants RTI2018-099298-B-100 from Ministerio de Ciencia, Innovación y Universidades, Spain, BFU2015-70131 from Ministerio de Economía y Competitividad, Spain and VA294P18 from Junta de Castilla y León, Spain.
Conflict of Interest
The authors declare that the research was conducted in the absence of any commercial or financial relationships that could be construed as a potential conflict of interest.
References
Abeele, F., Vanden, Skryma, R., Shuba, Y., Van Coppenolle, F., Slomianny, C., Roudbaraki, M., et al. (2002). Bcl-2-dependent modulation of Ca2+ homeostasis and store-operated channels in prostate cancer cells. Cancer Cell 1, 169–179. doi: 10.1016/S1535-6108(02)00034-X
Akl, H., Monaco, G., La Rovere, R., Welkenhuyzen, K., Kiviluoto, S., Vervliet, T., et al. (2013). IP3R2 levels dictate the apoptotic sensitivity of diffuse large B-cell lymphoma cells to an IP3R-derived peptide targeting the BH4 domain of Bcl-2. Cell Death Dis. 4, 1–12. doi: 10.1038/cddis.2013.140
Alvarez, J., Montero, M. (2002). Measuring [Ca2+] in the endoplasmic rerticulum with aequorin. Cell Calcium 32, 251–260. doi: 10.1016/S0143416002001860
Ambudkar, I. S., de Souza, L. B., Ong, H. L. (2017). TRPC1, Orai1, and STIM1 in SOCE: Friends in tight spaces. Cell Calcium 63, 33–39. doi: 10.1016/j.ceca.2016.12.009
Anderson, K. J., Cormier, R. T., Scott, P. M. (2019). Role of ion channels in gastrointestinal cancer. World J. Gastroenterol. 25 (38), 5732–5772. doi: 10.3748/wjg.v25.i38.5732
Ay, A.-S., Benzerdjerb, N., Sevestre, H., Ahidouch, A., Ouadid-Ahidouch, H. (2013). Orai3 Constitutes a Native Store-Operated Calcium Entry That Regulates Non Small Cell Lung Adenocarcinoma Cell Proliferation. PloS One. 8 (9), 1–11. doi: 10.1371/journal.pone.0072889
Aydar, E., Yeo, S., Djamgoz, M., Palmer, C. (2009). Abnormal expression, localization and interaction of canonical transient receptor potential ion channels in human breast cancer cell lines and tissues: A potential target for breast cancer diagnosis and therapy. Cancer Cell Int. 9, 1–12. doi: 10.1186/1475-2867-9-23
Aytes, A., Molleví, D. G., Martinez-Iniesta, M., Nadal, M., Vidal, A., Morales, A., et al. (2012). Stromal interaction molecule 2 (STIM2) is frequently overexpressed in colorectal tumors and confers a tumor cell growth suppressor phenotype. Mol. Carcinog. 51, 746–753. doi: 10.1002/mc.20843
Bachmann, A. S., Geerts, D. (2018). Polyamine synthesis as a target of MYC oncogenes. J. Biol. Chem. 293, 18757–18769. doi: 10.1074/jbc.TM118.003336
Badr, S. A., Fahmi, M. W., Nomir, M. M., El-Shishtawy, M. M. (2018). Calcium channel α2δ1 subunit as a novel biomarker for diagnosis of hepatocellular carcinoma. Cancer Biol. Med. 15, 52–60. doi: 10.20892/j.issn.2095-3941.2017.0167
Balkwill, F., Mantovani, A. (2001). Inflammation and cancer: Back to Virchow? Lancet 357, 539–545. doi: 10.1016/S0140-6736(00)04046-0
Batra, S., Alenfall, J. (1991). Effect of diverse categories of drugs on human colon tumour cell proliferation. Anticancer Res. 11, 1221–1224.
Baughman, J. M., Perocchi, F., Girgis, H. S., Plovanich, M., Belcher-Timme, C. A., Sancak, Y., et al. (2011). Integrative genomics identifies MCU as an essential component of the mitochondrial calcium uniporter. Nature 476, 341–345. doi: 10.1038/nature10234
Berchtold, M. W., Villalobo, A. (2014). The many faces of calmodulin in cell proliferation, programmed cell death, autophagy, and cancer. Biochimica et Biophysica Acta - Molecular Cell Research 1843. Biochim. Biophys. Acta - Mol. Cell Res. 1843, 398–435. doi: 10.1016/j.bbamcr.2013.10.021
Berna-Erro, A., Jardin, I., Salido, G. M., Rosado, J. A. (2017). Role of STIM2 in cell function and physiopathology. J. Physiol. 595, 3111–3128. doi: 10.1113/JP273889
Berridge, M. J., Bootman, M. D., Roderick, H. L. (2003). Calcium signalling: Dynamics, homeostasis and remodelling. Nat. Rev. Mol. Cell Biol. 4, 517–529. doi: 10.1038/nrm1155
Berridge, M. J. (1983). Rapid accumulation of inositol trisphosphate reveals that agonists hydrolyse polyphosphoinositides instead of phosphatidylinositol. Biochem. J. 212, 849–858. doi: 10.1042/bj2120849
Berridge, M. J. (1987). Inositol Trisphosphate and Diacylglycerol: Two Interacting Second Messengers. Annu. Rev. Biochem. 56, 159–193. doi: 10.1146/annurev.bi.56.070187.001111
Blaustein, M. P., Lederer, W. J. (1999). Sodium/calcium exchange: Its physiological implications. Physiol. Rev. 79, 763–854. doi: 10.1152/physrev.1999.79.3.763
Bolanz, K. A., Hediger, M. A., Landowski, C. P. (2008). The role of TRPV6 in breast carcinogenesis. Mol. Cancer Ther. 7, 271–279. doi: 10.1158/1535-7163.MCT-07-0478
Bong, A. H. L., Monteith, G. R. (2018). Calcium signaling and the therapeutic targeting of cancer cells. Biochim. Biophys. Acta - Mol. Cell Res. 1865, 1786–1794. doi: 10.1016/j.bbamcr.2018.05.015
Bononi, A., Missiroli, S., Poletti, F., Suski, J. M., Agnoletto, C., Bonora, M., et al. (2012). Mitochondria-associated membranes (MAMs) as hotspot Ca(2+) signaling units. Adv. Exp. Med. Biol. 740, 411–437. doi: 10.1007/978-94-007-2888-2_17
Bootman, M. D., Collins, T. J., Mackenzie, L., Roderick, H. L., Berridge, M. J., Peppiatt, C. M. (2002). 2-aminoethoxydiphenyl borate (2-APB) is a reliable blocker of store-operated Ca2+ entry but an inconsistent inhibitor of InsP3-induced Ca2+ release. FASEB J. 16, 1145–1150. doi: 10.1096/fj.02-0037rev
Bose, T., Cies̈lar-Pobuda, A., Wiechec, E. (2015). Role of ion channels in regulating Ca2+ homeostasis during the interplay between immune and cancer cells. Cell Death Dis. 6, 1–11. doi: 10.1038/cddis.2015.23
Boudreau, D. M., Koehler, E., Rulyak, S. J., Haneuse, S., Harrison, R., Mandelson, M. T. (2008). Cardiovascular medication use and risk for colorectal cancer. Cancer Epidemiol. Biomarkers Prev. 17, 3076–3080. doi: 10.1158/1055-9965.EPI-08-0095
Bowles, E. J. A., Yu, O., Ziebell, R., Chen, L., Boudreau, D. M., Ritzwoller, D. P., et al. (2019). Cardiovascular medication use and risks of colon cancer recurrences and additional cancer events: A cohort study. BMC Cancer 19, 1–12. doi: 10.1186/s12885-019-5493-8
Brandman, O., Liou, J., Park, W. S., Meyer, T. (2007). STIM2 Is a Feedback Regulator that Stabilizes Basal Cytosolic and Endoplasmic Reticulum Ca2+ Levels. Cell 131, 1327–1339. doi: 10.1016/j.cell.2007.11.039
Brasky, T. M., Krok-Schoen, J. L., Liu, J., Chlebowski, R. T., Freudenheim, J. L., Lavasani, S., et al. (2017). Use of calcium channel blockers and breast cancer risk in the women’s health initiative. Cancer Epidemiol. Biomarkers Prev. 26, 1345–1348. doi: 10.1158/1055-9965.EPI-17-0096
Brini, M., Carafoli, E. (2011). The Plasma Membrane Ca 2þ ATPase and the Plasma Membrane Sodium Calcium Exchanger Cooperate in the Regulation of Cell Calcium. Cold Spring Harbor Perspect. Biol. 3, 1–15. doi: 10.1101/cshperspect.a004168
Buchanan, P. J., McCloskey, K. D. (2016). CaV channels and cancer: canonical functions indicate benefits of repurposed drugs as cancer therapeutics. Eur. Biophys. J. 45, 621–633. doi: 10.1007/s00249-016-1144-z
Burke, C. A., Dekker, E., Samadder, N. J., Stoffel, E., Cohen, A. (2016). Efficacy and safety of eflornithine (CPP-1X)/sulindac combination therapy versus each as monotherapy in patients with familial adenomatous polyposis (FAP): Design and rationale of a randomized, double-blind, Phase III trial. BMC Gastroenterol. 16, 87. doi: 10.1186/s12876-016-0494-4
Burn, J., Gerdes, A. M., MacRae, F., Mecklin, J. P., Moeslein, G., Olschwang, S., et al. (2011). Long-term effect of aspirin on cancer risk in carriers of hereditary colorectal cancer: An analysis from the CAPP2 randomised controlled trial. Lancet 378, 2081–2087. doi: 10.1016/S0140-6736(11)61049-0
Cai, R., Ding, X., Zhou, K., Shi, Y., Ge, R., Ren, G., et al. (2009). Blockade of TRPC6 channels induced G2/M phase arrest and suppressed growth in human gastric cancer cells. Int. J. Cancer 125, 2281–2287. doi: 10.1002/ijc.24551
Chan, A. T., Arber, N., Burn, J., Chia, W. K., Elwood, P., Hull, M. A., et al. (2012). Aspirin in the chemoprevention of colorectal neoplasia: An overview. Cancer Prev. Res. 5, 164–178. doi: 10.1158/1940-6207.CAPR-11-0391
Chen, Y. F., Chiu, W. T., Chen, Y. T., Lin, P. Y., Huang, H. J., Chou, C. Y., et al. (2011). Calcium store sensor stromal-interaction molecule 1-dependent signaling plays an important role in cervical cancer growth, migration, and angiogenesis. Proc. Natl. Acad. Sci. U. S. A. 108, 15225–15230. doi: 10.1073/pnas.1103315108
Chen, G., Panicker, S., Lau, K. Y., Apparsundaram, S., Patel, V. A., Chen, S. L., et al. (2013). Characterization of a novel CRAC inhibitor that potently blocks human T cell activation and effector functions. Mol. Immunol. 54, 355–367. doi: 10.1016/j.molimm.2012.12.011
Chen, R., Zeng, X., Zhang, R., Huang, J., Kuang, X., Yang, J., et al. (2014). Cav1.3 channel α1D protein is overexpressed and modulates androgen receptor transactivation in prostate cancers. Urol. Oncol. Semin. Orig. Investig. 32, 524–536. doi: 10.1016/j.urolonc.2013.05.011
Cheng, H., Lederer, W. J., Cannell, M. B. (1993). Calcium sparks: Elementary events underlying excitation-contraction coupling in heart muscle. Sci. (80-.). 262, 740–744. doi: 10.1126/science.8235594
Cheng, K. T., Ong, H. L., Liu, X., Ambudkar, I. S. (2013). “Contribution and Regulation of TRPC Channels in Store-Operated Ca2+ Entry,” in Current Topics in Membranes (Cambridge, Massachusetts, Estados Unidos: Academic Press Inc.), 149–179. doi: 10.1016/B978-0-12-407870-3.00007-X
Chodon, D., Guilbert, A., Dhennin-Duthille, I., Gautier, M., Telliez, M. S., Sevestre, H., et al. (2010). Estrogen regulation of TRPM8 expression in breast cancer cells. BMC Cancer 10, 1–8. doi: 10.1186/1471-2407-10-212
Contreras, L., Drago, I., Zampese, E., Pozzan, T. (2010). Mitochondria: The calcium connection. Biochim. Biophys. Acta - Bioenerg. 1797, 607–618. doi: 10.1016/j.bbabio.2010.05.005
Coussens, L. M., Werb, Z. (2002). Inflammation and cancer. Nature 420, 860–867. doi: 10.1038/nature01322
Cox, J. H., Hussell, S., Søndergaard, H., Roepstorff, K., Bui, J.-V., Deer, J. R., et al. (2013). Antibody-Mediated Targeting of the Orai1 Calcium Channel Inhibits T Cell Function. PloS One 8, e82944. doi: 10.1371/journal.pone.0082944
Cromer, M. K., Choi, M., Nelson-Williams, C., Fonseca, A. L., Kunstman, J. W., Korah, R. M., et al. (2015). Neomorphic effects of recurrent somatic mutations in yin yang 1 in insulin-producing adenomas. Proc. Natl. Acad. Sci. U. S. A. 112, 4062–4067. doi: 10.1073/pnas.1503696112
Cui, C., Merritt, R., Fu, L., Pan, Z. (2017). Targeting calcium signaling in cancer therapy. Acta Pharm. Sin. B 7, 3–17. doi: 10.1016/j.apsb.2016.11.001
Déliot, N., Constantin, B. (2015). Plasma membrane calcium channels in cancer: Alterations and consequences for cell proliferation and migration. Biochim. Biophys. Acta - Biomembr. 1848, 2512–2522. doi: 10.1016/j.bbamem.2015.06.009
Dannoura, A., Giraldo, A., Pereira, I., Gibbins, J. M., Dash, P. R., Bicknell, K. A., et al. (2014). Ibuprofen inhibits migration and proliferation of human coronary artery smooth muscle cells by inducing a differentiated phenotype: Role of peroxisome proliferator-activated receptor γ. J. Pharm. Pharmacol. 66, 779–792. doi: 10.1111/jphp.12203
Debes, J. D., Roberts, R. O., Jacobson, D. J., Girman, C. J., Lieber, M. M., Tindall, D. J., et al. (2004). Inverse Association between Prostate Cancer and the Use of Calcium Channel Blockers. Cancer Epidemiol. Biomarkers Prev. 13, 255–259. doi: 10.1158/1055-9965.EPI-03-0093
DeHaven, W., II, Smyth, J. T., Boyles, R. R., Bird, G. S., Putney, J. W. (2008). Complex actions of 2-aminoethyldiphenyl borate on store-operated calcium entry. J. Biol. Chem. 283, 19265–19273. doi: 10.1074/jbc.M801535200
Derler, I., Fritsch, R., Schindl, R., Romanin, C. (2008). CRAC inhibitors: Identification and potential. Expert Opin. Drug Discovery 3, 787–800. doi: 10.1517/17460441.3.7.787
Derler, I., Schindl, R., Fritsch, R., Heftberger, P., Riedl, M. C., Begg, M., et al. (2013). The action of selective CRAC channel blockers is affected by the Orai pore geometry. Cell Calcium 53, 139–151. doi: 10.1016/j.ceca.2012.11.005
Devore, E. E., Kim, S., Ramin, C. A., Wegrzyn, L. R., Massa, J., Holmes, M. D., et al. (2015). Antihypertensive medication use and incident breast cancer in women. Breast Cancer Res. Treat. 150, 219–229. doi: 10.1007/s10549-015-3311-9
Dhennin-Duthille, I., Gautier, M., Faouzi, M., Guilbert, A., Brevet, M., Vaudry, D., et al. (2011). High Expression of Transient Receptor Potential Channels in Human Breast Cancer Epithelial Cells and Tissues: Correlation with Pathological Parameters. Cell. Physiol. Biochem. 28, 813–822. doi: 10.1159/000335795
Di Sabatino, A., Rovedatti, L., Kaur, R., Spencer, J. P., Brown, J. T., Morisset, V. D., et al. (2009). Targeting Gut T Cell Ca 2+ Release-Activated Ca 2+ Channels Inhibits T Cell Cytokine Production and T-Box Transcription Factor T-Bet in Inflammatory Bowel Disease. J. Immunol. 183, 3454–3462. doi: 10.4049/jimmunol.0802887
Diez-Bello, R., Jardin, I., Lopez, J. J., El Haouari, M., Ortega-Vidal, J., Altarejos, J., et al. (2019). (–)□Oleocanthal inhibits proliferation and migration by modulating Ca2+ entry through TRPC6 in breast cancer cells. Biochim. Biophys. Acta - Mol. Cell Res. 1866, 474–485. doi: 10.1016/j.bbamcr.2018.10.010
Ding, X., He, Z., Zhou, K., Cheng, J., Yao, H., Lu, D., et al. (2010). Essential role of TRPC6 channels in G2/M phase transition and development of human glioma. J. Natl. Cancer Inst. 102, 1052–1068. doi: 10.1093/jnci/djq217
Dixon, R. E., Moreno, C. M., Yuan, C., Opitz-Araya, X., Binder, M. D., Navedo, M. F., et al. (2015). Graded Ca2+/calmodulin-dependent coupling of voltage-gated CaV1.2 channels. Elife 2015, 1–21. doi: 10.7554/eLife.05608
Djuric, S. W., Wiedeman, P. E., Zhou, X., Ballaron, S., Bauch, J., Chen, Y. W., et al. (2000). 3,5-Bis(trifluoromethyl)pyrazoles: A novel class of NFAT transcription factor regulator. J. Med. Chem. 43, 2975–2981. doi: 10.1021/jm990615a
Dolejs, S. C., Gayed, B., Fajardo, A. (2016). Prevention of Colorectal Neoplasia. Clin. Colon Rectal Surg. 29, 353–362. doi: 10.1055/s-0036-1584086
Dolphin, A. C. (2016). Voltage-gated calcium channels and their auxiliary subunits: physiology and pathophysiology and pharmacology. J. Physiol. 594, 5369–5390. doi: 10.1113/JP272262
Drew, D. A., Cao, Y., Chan, A. T. (2016). Aspirin and colorectal cancer: The promise of precision chemoprevention. Nat. Rev. Cancer 16, 173–186. doi: 10.1038/nrc.2016.4
Dubois, C., Vanden Abeele, F., Lehen’kyi, V., Gkika, D., Guarmit, B., Lepage, G., et al. (2014). Remodeling of Channel-Forming ORAI Proteins Determines an Oncogenic Switch in Prostate Cancer. Cancer Cell 26, 19–32. doi: 10.1016/j.ccr.2014.04.025
El Boustany, C., Bidaux, G., Enfissi, A., Delcourt, P., Prevarskaya, N., Capiod, T. (2008). Capacitative calcium entry and transient receptor potential canonical 6 expression control human hepatoma cell proliferation. Hepatology 47, 2068–2077. doi: 10.1002/hep.22263
Endo, M., Tanaka, M., Ogawa, Y. (1970). Calcium Induced release of calcium from the Sarcoplasmic Reticulum of Skinned Skeletal Muscle Fibres. Nature 228, 34–36. doi: 10.1038/228034a0
Fahmy, H., Khalifa, N., Ismail, M., El-Sahrawy, H., Nossier, E. (2016). Biological Validation of Novel Polysubstituted Pyrazole Candidates with in Vitro Anticancer Activities. Molecules 21, 271. doi: 10.3390/molecules21030271
Fahrner, M., Schindl, R., Muik, M., Derler, I., Romanin, C. (2017). “The STIM-orai pathway: The interactions between STIM and orai,” in Advances in Experimental Medicine and Biology (New York City (USA): Springer New York LLC), 59–81. doi: 10.1007/978-3-319-57732-6_4
Fan, H., Shen, Y. X., Yuan, Y. F. (2014). Expression and prognostic roles of TRPV5 and TRPV6 in non-small cell lung cancer after curative resection. Asian Pacific J. Cancer Prev. 15, 2559–2563. doi: 10.7314/APJCP.2014.15.6.2559
Feske, S., Gwack, Y., Prakriya, M., Srikanth, S., Puppel, S. H., Tanasa, B., et al. (2006). A mutation in Orai1 causes immune deficiency by abrogating CRAC channel function. Nature 441, 179–185. doi: 10.1038/nature04702
Fierro, L., Parekh, A. B. (2000). Substantial depletion of the intracellular Ca2+ stores is required for macroscopic activation of the Ca2+ release-activated Ca2+ current in rat basophilic leukaemia cells. J. Physiol. 522, 247–257. doi: 10.1111/j.1469-7793.2000.t01-1-00247.x
Fitzpatrick, A. L., Daling, J. R., Furberg, C. D., Kronmal, R. A., Weissfeld, J. L. (1997). Use of calcium channel blockers and breast carcinoma risk in postmenopausal women. Cancer 80, 1438–1447. doi: 10.1002/(sici)1097-0142(19971015)80:8<1438::aid-cncr11>3.0.co;2-6
Fleischer, S., Ogunbunmi, E. M., Dixon, M. C., Fleer, E. A. M. (1985). Localization of Ca2+ release channels with ryanodine in junctional terminal cisternae of sarcoplasmic reticulum of fast skeletal muscle. Proc. Natl. Acad. Sci. U. S. A. 82, 7256–7259. doi: 10.1073/pnas.82.21.7256
Flourakis, M., Lehen’kyi, V., Beck, B., Raphaël, M., Vandenberghe, M., Abeele, F. V., et al. (2010). Orai1 contributes to the establishment of an apoptosis-resistant phenotype in prostate cancer cells. Cell Death Dis. 1, 1–9. doi: 10.1038/cddis.2010.52
Foskett, J. K., White, C., Cheung, K. H., Mak, D. O. D. (2007). Inositol trisphosphate receptor Ca2+ release channels. Physiol. Rev. 87, 593–658. doi: 10.1152/physrev.00035.2006
Franzius, D., Hoth, M., Penner, R. (1994). Non-specific effects of calcium entry antagonists in mast cells. Pflügers Arch. Eur. J. Physiol. 428, 433–438. doi: 10.1007/BF00374562
Fukushima, M., Tomita, T., Janoshazi, A., Putney, J. W. (2012). Alternative translation initiation gives rise to two isoforms of Orai1 with distinct plasma membrane mobilities. J. Cell Sci. 125, 4354–4361. doi: 10.1242/jcs.104919
Fusi, C., Materazzi, S., Minocci, D., Maio, V., Oranges, T., Massi, D., et al. (2014). Transient receptor potential vanilloid 4 (TRPV4) is downregulated in keratinocytes in human non-melanoma skin cancer. J. Invest. Dermatol. 134, 2408–2417. doi: 10.1038/jid.2014.145
Gackière, F., Bidaux, G., Delcourt, P., Van Coppenolle, F., Katsogiannou, M., Dewailly, E., et al. (2008). CaV3.2 T-type calcium channels are involved in calcium-dependent secretion of neuroendocrine prostate cancer cells. J. Biol. Chem. 283, 10162–10173. doi: 10.1074/jbc.M707159200
Gao, P., He, M., Zhang, C., Geng, C. (2018). Integrated analysis of gene expression signatures associated with colon cancer from three datasets. Gene 654, 95–102. doi: 10.1016/j.gene.2018.02.007
Gees, M., Owsianik, G., Nilius, B., Voets, T. (2012). TRP channels. Compr. Physiol. 2, 563–608. doi: 10.1002/cphy.c110026
Genova, T., Grolez, G. P., Camillo, C., Bernardini, M., Bokhobza, A., Richard, E., et al. (2017). TRPM8 inhibits endothelial cell migration via a nonchannel function by trapping the small GTPase Rap1. J. Cell Biol. 216, 2107–2130. doi: 10.1083/jcb.201506024
Gerasimenko, J. V., Gryshchenko, O., Ferdek, P. E., Stapleton, E., Hébert, T. O. G., Bychkova, S., et al. (2013). Ca2+ release-activated Ca2+ channel blockade as a potential tool in antipancreatitis therapy. Proc. Natl. Acad. Sci. U. S. A. 110, 13186–13191. doi: 10.1073/pnas.1300910110
Gerner, E. W., Bruckheimer, E., Cohen, A. (2018). Cancer pharmacoprevention: Targeting polyamine metabolism to manage risk factors for colon cancer. J. Biol. Chem. 293, 18770–18778. doi: 10.1074/jbc.TM118.003343
Giardiello, F. M., Hamilton, S. R., Hylind, L. M., Yang, V. W., Tamez, P., Casero, R. A. (1997). Ornithine decarboxylase and polyamines in familial adenomatous polyposis. Cancer Res. 57, 199–201.
Gilabert, J. A. (2000). Respiring mitochondria determine the pattern of activation and inactivation of the store-operated Ca2+ current ICRAC. EMBO J. 19, 6401–6407. doi: 10.1093/emboj/19.23.6401
Giorgi, C., Baldassari, F., Bononi, A., Bonora, M., De Marchi, E., Marchi, S., et al. (2012). Mitochondrial Ca2+ and apoptosis. Cell Calcium 52, 36–43. doi: 10.1016/j.ceca.2012.02.008
Gkika, D., Lemonnier, L., Shapovalov, G., Gordienko, D., Poux, C., Bernardini, M., et al. (2015). TRP channel-associated factors are a novel protein family that regulates TRPM8 trafficking and activity. J. Cell Biol. 208, 89–107. doi: 10.1083/jcb.201402076
Gomez-Ospina, N., Tsuruta, F., Barreto-Chang, O., Hu, L., Dolmetsch, R. (2006). The C Terminus of the L-Type Voltage-Gated Calcium Channel CaV1.2 Encodes a Transcription Factor. Cell 127, 591–606. doi: 10.1016/j.cell.2006.10.017
Gomez-Ospina, N., Panagiotakos, G., Portmann, T., Pasca, S. P., Rabah, D., Budzillo, A., et al. (2013). A Promoter in the Coding Region of the Calcium Channel Gene CACNA1C Generates the Transcription Factor CCAT. PloS One 8, 1–11. doi: 10.1371/journal.pone.0060526
Goto, J.-I., Suzuki, A. Z., Ozaki, S., Matsumoto, N., Nakamura, T., Ebisui, E., et al. (2010). Two novel 2-aminoethyl diphenylborinate (2-APB) analogues differentially activate and inhibit store-operated Ca(2+) entry via STIM proteins. Cell Calcium 47, 1–10. doi: 10.1016/j.ceca.2009.10.004
Grasso, C. S., Wu, Y. M., Robinson, D. R., Cao, X., Dhanasekaran, S. M., Khan, A. P., et al. (2012). The mutational landscape of lethal castration-resistant prostate cancer. Nature 487, 239–243. doi: 10.1038/nature11125
Grolez, G. P., Hammadi, M., Barras, A., Gordienko, D., Slomianny, C., Völkel, P., et al. (2019). Encapsulation of a TRPM8 Agonist, WS12, in Lipid Nanocapsules Potentiates PC3 Prostate Cancer Cell Migration Inhibition through Channel Activation. Sci. Rep. 9, 1–15. doi: 10.1038/s41598-019-44452-4
Guéguinou, M., Harnois, T., Crottes, D., Uguen, A., Deliot, N., Gambade, A., et al. (2016). SK3/TRPC1/Orai1 complex regulates SOCE-dependent colon cancer cell migration: A novel opportunity to modulate anti- EGFR mAb action by the alkyl-lipid Ohmline. Oncotarget 7, 36168–36184. doi: 10.18632/oncotarget.8786
Gueguinou, M., Crottès, D., Chantôme, A., Rapetti-Mauss, R., Potier-Cartereau, M., Clarysse, L., et al. (2017). The SigmaR1 chaperone drives breast and colorectal cancer cell migration by tuning SK3-dependent Ca2+ homeostasis. Oncogene 36, 3640–3647. doi: 10.1038/onc.2016.501
Guilbert, A., Dhennin-Duthille, I., Hiani, Y. E. L., Haren, N., Khorsi, H., Sevestre, H., et al. (2008). Expression of TRPC6 channels in human epithelial breast cancer cells. BMC Cancer 8, 1–11. doi: 10.1186/1471-2407-8-125
Gutiérrez, L. G., Hernández-Morales, M., Núñez, L., Villalobos, C. (2019). Inhibition of polyamine biosynthesis reverses Ca 2+ channel remodeling in colon cancer cells. Cancers (Basel). 11, 1–17. doi: 10.3390/cancers11010083
Hanahan, D., Weinberg, R. A. (2000). The hallmarks of cancer. Cell 100, 57–70. doi: 10.1016/S0092-8674(00)81683-9
Hao, Y., Triadafilopoulos, G., Sahbaie, P., Young, H. S., Omary, M. B., Lowe, A. W. (2006). Gene Expression Profiling Reveals Stromal Genes Expressed in Common Between Barrett’s Esophagus and Adenocarcinoma. Gastroenterology 131, 925–933. doi: 10.1053/j.gastro.2006.04.026.
Haworth, A. S., Brackenbury, W. J. (2019). Emerging roles for multifunctional ion channel auxiliary subunits in cancer. Cell Calcium 80, 125–140. doi: 10.1016/j.ceca.2019.04.005
He, L.-P., Hewavitharana, T., Soboloff, J., Spassova, M. A., Gill, D. L. (2005). A functional link between store-operated and TRPC channels revealed by the 3,5-bis(trifluoromethyl)pyrazole derivative, BTP2. J. Biol. Chem. 280, 10997–11006. doi: 10.1074/jbc.M411797200
Hermosura, M. C., Monteilh-Zoller, M. K., Scharenberg, A. M., Penner, R., Fleig, A. (2002). Dissociation of the store-operated calcium current/CRAC and the Mg-nucleotide-regulated metal ion current MagNuM. J. Physiol. 539, 445–458. doi: 10.1113/jphysiol.2001.013361
Hernández-Morales, M., Sobradillo, D., Valero, R. A., Muñoz, E., Ubierna, D., Moyer, M. P., et al. (2017). Mitochondria sustain store-operated currents in colon cancer cells but not in normal colonic cells: Reversal by non-steroidal anti-inflammatory drugs. Oncotarget 8, 55332–55352. doi: 10.18632/oncotarget.19430
Hoshino, Y., Obara, H., Kusunoki, M., Fujii, Y., Iwai, S. (1988). Hypoxic contractile response in isolated human pulmonary artery: role of calcium ion. J. Appl. Physiol. 65, 2468–2474. doi: 10.1152/jappl.1988.65.6.2468
Hoth, M., Penner, R. (1992). Depletion of intracellular calcium stores activates a calcium current in mast cells. Nature 355, 353–356. doi: 10.1038/355353a0
Hoth, M., Fanger, C. M., Lewis, R. S. (1997). Mitochondrial regulation of store-operated calcium signaling in T lymphocytes. J. Cell Biol. 137, 633–648. doi: 10.1083/jcb.137.3.633
Hoth, M. (2016). CRAC channels, calcium, and cancer in light of the driver and passenger concept. Biochim. Biophys. Acta - Mol. Cell Res. 1863, 1408–1417. doi: 10.1016/j.bbamcr.2015.12.009
Hudes, G., Tagawa, S. T., Whang, Y. E., Qi, M., Qin, X., Puchalski, T. A., et al. (2013). A phase 1 study of a chimeric monoclonal antibody against interleukin-6, siltuximab, combined with docetaxel in patients with metastatic castration-resistant prostate cancer. Invest. New Drugs 31, 669–676. doi: 10.1007/s10637-012-9857-z
Ishikawa, J., Ohga, K., Yoshino, T., Takezawa, R., Ichikawa, A., Kubota, H., et al. (2003). A Pyrazole Derivative, YM-58483, Potently Inhibits Store-Operated Sustained Ca 2+ Influx and IL-2 Production in T Lymphocytes. J. Immunol. 170, 4441–4449. doi: 10.4049/jimmunol.170.9.4441
Jacquemet, G., Baghirov, H., Georgiadou, M., Sihto, H., Peuhu, E., Cettour-Janet, P., et al. (2016). L-type calcium channels regulate filopodia stability and cancer cell invasion downstream of integrin signalling. Nat. Commun. 7, 1–17. doi: 10.1038/ncomms13297
Jairaman, A., Prakriya, M. (2013). Molecular pharmacology of store-operated CRAC channels. Channels 7, 402–414. doi: 10.4161/chan.25292
Kale, V. P., Amin, S. G., Pandey, M. K. (2015). Targeting ion channels for cancer therapy by repurposing the approved drugs. Biochim. Biophys. Acta - Biomembr. 1848, 2747–2755. doi: 10.1016/j.bbamem.2015.03.034
Kim, B. J., Park, E. J., Lee, J. H., Jeon, J. H., Kim, S. J., So, I. (2008). Suppression of transient receptor potential melastatin 7 channel induces cell death in gastric cancer. Cancer Sci. 99, 2502–2509. doi: 10.1111/j.1349-7006.2008.00982.x
Kim, B. J., Kim, S. Y., Lee, S., Jeon, J. H., Matsui, H., Kwon, Y. K., et al. (2012). The role of transient receptor potential channel blockers in human gastric cancer cell viability. Can. J. Physiol. Pharmacol. 90, 175–186. doi: 10.1139/Y11-114
Kim, J. H., Lkhagvadorj, S., Lee, M. R., Hwang, K. H., Chung, H. C., Jung, J. H., et al. (2014). Orai1 and STIM1 are critical for cell migration and proliferation of clear cell renal cell carcinoma. Biochem. Biophys. Res. Commun. 448, 76–82. doi: 10.1016/j.bbrc.2014.04.064
Komai, T., Kimura, T., Baba, D. Y., O.-U, P., 2019, U. (2017). Anti-Orai1 antibody. US 2017/0226203.
Koo, B. K., Kim, Y. S., Park, K. W., Yang, H. M., Kwon, D. A., Chung, J. W., et al. (2007). Effect of celecoxib on restenosis after coronary angioplasty with a Taxus stent (COREA-TAXUS trial): an open-label randomised controlled study. Lancet 370, 567–574. doi: 10.1016/S0140-6736(07)61295-1
Kunzelmann, K. (2005). Ion channels and cancer. J. Membr. Biol. 205, 159–173. doi: 10.1007/s00232-005-0781-4
Lam, A. K. M., Galione, A. (2013). The endoplasmic reticulum and junctional membrane communication during calcium signaling. Biochim. Biophys. Acta - Mol. Cell Res. 1833, 2542–2559. doi: 10.1016/j.bbamcr.2013.06.004
Latour, I., Louw, D. F., Beedle, A. M., Hamid, J., Sutherland, G. R., Zamponi, G. W. (2004). Expression of T-type calcium channel splice variants in human glioma. Glia 48, 112–119. doi: 10.1002/glia.20063
Laukaitis, C. M., Gerner, E. W. (2011). DFMO: Targeted risk reduction therapy for colorectal neoplasia. Best Pract. Res. Clin. Gastroenterol. 25, 495–506. doi: 10.1016/j.bpg.2011.09.007
Lee, S. C., Deutsch, C., Beck, W. T. (1988). Comparison of ion channels in multidrug-resistant and -sensitive human leukemic cells. Proc. Natl. Acad. Sci. U. S. A. 85, 2019–2023. doi: 10.1073/pnas.85.6.2019
Li, G., Zhang, Z., Wang, R., Ma, W., Yang, Y., Wei, J., et al. (2013). Suppression of STIM1 inhibits human glioblastoma cell proliferation and induces G0/G1 phase arrest. J. Exp. Clin. Cancer Res. 32, 1–9. doi: 10.1186/1756-9966-32-20
Lin, F. F., Elliott, R., Colombero, A., Gaida, K., Kelley, L., Moksa, A., et al. (2013). Generation and characterization of fully human monoclonal antibodies against human orai1 for autoimmune diseases. J. Pharmacol. Exp. Ther. 345, 225–238. doi: 10.1124/jpet.112.202788
Liou, J., Kim, M. L., Won, D. H., Jones, J. T., Myers, J. W., Ferrell, J. E., et al. (2005). STIM is a Ca2+ sensor essential for Ca2+-store- depletion-triggered Ca2+ influx. Curr. Biol. 15, 1235–1241. doi: 10.1016/j.cub.2005.05.055
Lipscombe, D., Helton, T. D., Xu, W. (2004). L-type calcium channels: The low down. J. Neurophysiol. 92, 2633–2641. doi: 10.1152/jn.00486.2004
Lis, A., Peinelt, C., Beck, A., Parvez, S., Monteilh-Zoller, M., Fleig, A., et al. (2007). CRACM1, CRACM2, and CRACM3 Are Store-Operated Ca2+ Channels with Distinct Functional Properties. Curr. Biol. 17, 794–800. doi: 10.1016/j.cub.2007.03.065
Liu, J., Chen, Y., Shuai, S., Ding, D., Li, R., Luo, R. (2014). TRPM8 promotes aggressiveness of breast cancer cells by regulating EMT via activating AKT/GSK-3β pathway. Tumor Biol. 35, 8969–8977. doi: 10.1007/s13277-014-2077-8
Liu, S., Hasegawa, H., Takemasa, E., Suzuki, Y., Oka, K., Kiyoi, T., et al. (2017). Efficiency and Safety of CRAC Inhibitors in Human Rheumatoid Arthritis Xenograft Models. J. Immunol. 199, 1584–1595. doi: 10.4049/jimmunol.1700192
Lloyd-Evans, E., Waller-Evans, H. (2019). Lysosomal Ca2+ Homeostasis and Signaling in Health and Disease. Cold Spring Harb. Perspect. Biol. 12, 1–12. doi: 10.1101/cshperspect.a035311
Lu, L., Sirish, P., Zhang, Z., Woltz, R. L., Li, N., Timofeyev, V., et al. (2015). Regulation of gene transcription by voltage-gated L-type calcium channel, Cav1.3. J. Biol. Chem. 290, 4663–4676. doi: 10.1074/jbc.M114.586883
Luk, G. D., Baylin, S. B. (1984). Ornithine Decarboxylase as a Biologic Marker in Familial Colonic Polyposis. N. Engl. J. Med. 311, 80–83. doi: 10.1056/NEJM198407123110202
Ma, H. T., Patterson, R. L., Van Rossum, D. B., Birnbaumer, L., Mikoshiba, K., Gill, D. L. (2000). Requirement of the inositol trisphosphate receptor for activation of store-operated Ca2+ channels. Sci. (80-. ). 287, 1647–1651. doi: 10.1126/science.287.5458.1647
MacLennan, D. H. (1970). Purification and properties of an adenosine triphosphatase from sarcoplasmic reticulum. J. Biol. Chem. 245, 4508–4518.
Mammucari, C., Raffaello, A., Vecellio Reane, D., Gherardi, G., De Mario, A., Rizzuto, R. (2018). Mitochondrial calcium uptake in organ physiology: from molecular mechanism to animal models. Pflugers Archiv. Eur. J. Physiol. 470, (8) 1165–1179. doi: 10.1007/s00424-018-2123-2
Mancini, M., Toker, A. (2009). NFAT proteins: Emerging roles in cancer progression. Nat. Rev. Cancer 9, 810–820. doi: 10.1038/nrc2735
Martínez, M. E., O’Brien, T. G., Fultz, K. E., Babbar, N., Yerushalmi, H., Qu, N., et al. (2003). Pronounced reduction in adenoma recurrence associated with aspirin use and a polymorphism in the ornithine decarboxylase gene. Proc. Natl. Acad. Sci. U. S. A. 100, 7859–7864. doi: 10.1073/pnas.1332465100
McAndrew, D., Grice, D. M., Peters, A. A., Davis, F. M., Stewart, T., Rice, M., et al. (2011). ORAI1-mediated calcium influx in lactation and in breast cancer. Mol. Cancer Ther. 10, 448–460. doi: 10.1158/1535-7163.MCT-10-0923
Meyer, T., Stryer, L. (1991). Calcium spiking. Annu. Rev. Biophys. Biophys. Chem. 20, 153–174. doi: 10.1146/annurev.bb.20.060191.001101
Meyskens, F. L., McLaren, C. E., Pelot, D., Fujikawa-Brooks, S., Carpenter, P. M., Hawk, E., et al. (2008). Difluoromethylornithine plus sulindac for the prevention of sporadic colorectal adenomas: A randomized placebo-controlled, double-blind trial. Cancer Prev. Res. 1, 32–38. doi: 10.1158/1940-6207.CAPR-08-0042
Michels, K. B., Rosner, B. A., Walker, A. M., Stampfer, M. J., Manson, J. E., Colditz, G. A., et al. (1998). Calcium channel blockers, cancer incidence, and cancer mortality in a cohort of U.S. Women: The nurses’ health study. Cancer 83, 2003–2007. doi: 10.1002/(SICI)1097-0142(19981101)83:9<2003::AID-CNCR17>3.0.CO;2-3
Middelbeek, J., Kuipers, A. J., Henneman, L., Visser, D., Eidhof, I., Van Horssen, R., et al. (2012). TRPM7 is required for breast tumor cell metastasis. Cancer Res. 72, 4250–4261. doi: 10.1158/0008-5472.CAN-11-3863
Miederer, A. M., Alansary, D., Schwär, G., Lee, P. H., Jung, M., Helms, V., et al. (2015). A STIM2 splice variant negatively regulates store-operated calcium entry. Nat. Commun. 6, 1–12. doi: 10.1038/ncomms7899
Missiaen, L., Callewaert, G., De Smedt, H., Parys, J. B. (2001). 2-Aminoethoxydiphenyl borate affects the inositol 1,4,5-trisphosphate receptor, the intracellular Ca2+ pump and the non-specific Ca2+ leak from the non-mitochondrial Ca2+ stores in permeabilized A7r5 cells. Cell Calcium 29, 111–116. doi: 10.1054/ceca.2000.0163
Mitra, R., Lee, J., Jo, J., Milani, M., McClintick, J. N., Edenberg, H. J., et al. (2011). Prediction of postoperative recurrence-free survival in non-small cell lung cancer by using an internationally validated gene expression model. Clin. Cancer Res. 17, 2934–2946. doi: 10.1158/1078-0432.CCR-10-1803
Mizuno, H., Suzuki, Y., Watanabe, M., Sokabe, T., Yamamoto, T., Hattori, R., et al. (2014). Potential role of transient receptor potential (TRP) channels in bladder cancer cells. J. Physiol. Sci. 64, 305–314. doi: 10.1007/s12576-014-0319-6
Molnár, T., Yarishkin, O., Iuso, A., Barabas, P., Jones, B., Marc, R. E., et al. (2016). Store-operated calcium entry in müller glia is controlled by synergistic activation of TRPC and orai channels. J. Neurosci. 36, 3184–3198. doi: 10.1523/JNEUROSCI.4069-15.2016
Monteith, G. R., Davis, F. M., Roberts-Thomson, S. J. (2012). Calcium channels and pumps in cancer: changes and consequences. J. Biol. Chem. 287, 31666–31673. doi: 10.1074/jbc.R112.343061
Moreno, C. M., Dixon, R. E., Tajada, S., Yuan, C., Opitz-Araya, X., Binder, M. D., et al. (2016). Ca2+ entry into neurons is facilitated by cooperative gating of clustered Cav1.3 channels. Elife 5, 1–26. doi: 10.7554/eLife.15744
Motiani, R. K., Hyzinski-García, M. C., Zhang, X., Henkel, M. M., Abdullaev, I. F., Kuo, Y. H., et al. (2013a). STIM1 and Orai1 mediate CRAC channel activity and are essential for human glioblastoma invasion. Pflugers Arch. Eur. J. Physiol. 465, 1249–1260. doi: 10.1007/s00424-013-1254-8
Motiani, R. K., Zhang, X., Harmon, K. E., Keller, R. S., Matrougui, K., Bennett, J. A., et al. (2013b). Orai3 is an estrogen receptor α-regulated Ca2+ channel that promotes tumorigenesis. FASEB J. 27, 63–75. doi: 10.1096/fj.12-213801
Muñoz, E., Valero, R. A., Quintana, A., Hoth, M., Núñez, L., Villalobos, C. (2011). Nonsteroidal anti-inflammatory drugs inhibit vascular smooth muscle cell proliferation by enabling the Ca2+-dependent inactivation of calcium release-activated calcium/orai channels normally prevented by mitochondria. J. Biol. Chem. 286, 16186–16196. doi: 10.1074/jbc.M110.198952
Muñoz, E., Hernández-Morales, M., Sobradillo, D., Rocher, A., Núñez, L., Villalobos, C. (2013). Intracellular Ca2+ remodeling during the phenotypic journey of human coronary smooth muscle cells. Cell Calcium 54, 375–385. doi: 10.1016/j.ceca.2013.08.006
Núñez, L., Valero, R. A., Senovilla, L., Sanz-Blasco, S., García-Sancho, J., Villalobos, C. (2006). Cell proliferation depends on mitochondrial Ca2+ uptake: inhibition by salicylate. J. Physiol. 571, 57–73. doi: 10.1113/jphysiol.2005.100586
Núñez, L., Bird, G. S., Hernando-Pérez, E., Pérez-Riesgo, E., Putney, J. W., Villalobos, C. (2019). Store-operated Ca2+ entry and Ca2+ responses to hypothalamic releasing hormones in anterior pituitary cells from Orai1-/- and heptaTRPC knockout mice. Biochim. Biophys. Acta Mol. Cell Res. 1866, 1124–1136. doi: 10.1016/j.bbamcr.2018.11.006
Navedo, M. F., Amberg, G. C., Votaw, V. S., Santana, L. F. (2005). Constitutively active L-type Ca2+ channels. Proc. Natl. Acad. Sci. 102, 11112–11117. doi: 10.1073/pnas.0500360102
Nilius, B., Owsianik, G. (2010). Transient receptor potential channelopathies. Pflugers Arch. Eur. J. Physiol. 460, 437–450. doi: 10.1007/s00424-010-0788-2
Noble, D., Herchuelz, A. (2007). meeting report Role of Na/Ca exchange and the plasma membrane Ca 2+-ATPase in cell function Conference on Na/Ca Exchange. EMBO Rep. 8, 228–232. doi: 10.1038/sj.embor.7400914
Nougarede, A., Popgeorgiev, N., Kassem, L., Omarjee, S., Borel, S., Mikaelian, I., et al. (2018). Breast cancer targeting through inhibition of the endoplasmic reticulum-based apoptosis regulator Nrh/BCL2L10. Cancer Res. 78, 1404–1417. doi: 10.1158/0008-5472.CAN-17-0846
Pardo, L. A. (2004). Voltage-gated potassium channels in cell proliferation. Physiology 19, 285–292. doi: 10.1152/physiol.00011.2004
Parekh, A. B., Putney, J. W. (2005). Store-operated calcium channels. Physiol. Rev. 85, 757–810. doi: 10.1152/physrev.00057.2003
Parekh, A. B. (2019). Calcium signalling in health and disease. Semin. Cell Dev. Biol. 94, 1–2. doi: 10.1016/j.semcdb.2019.05.030
Parkash, J., Asotra, K. (2010). Calcium wave signaling in cancer cells. Life Sci. 87, 587–595. doi: 10.1016/j.lfs.2010.09.013
Paupe, V., Prudent, J. (2018). New insights into the role of mitochondrial calcium homeostasis in cell migration. Biochem. Biophys. Res. Commun. 500, 75–86. doi: 10.1016/j.bbrc.2017.05.039
Pegg, A. E. (1988). Polyamine Metabolism and Its Importance in Neoplastic Growth and as a Target for Chemotherapy. Cancer Res. 48, 759–774.
Pegg, A. E. (2016). Functions of polyamines in mammals. J. Biol. Chem. 291, 14904–14912. doi: 10.1074/jbc.R116.731661
Pérez-Riesgo, E., Gutiérrez, L. G., Ubierna, D., Acedo, A., Moyer, M. P., Núñez, L., et al. (2017). Transcriptomic Analysis of Calcium Remodeling in Colorectal Cancer. Int. J. Mol. Sci. 18, 1–23. doi: 10.3390/ijms18050922
Perocchi, F., Gohil, V. M., Girgis, H. S., Bao, X. R., McCombs, J. E., Palmer, A. E., et al. (2010). MICU1 encodes a mitochondrial EF hand protein required for Ca2+ uptake. Nature 467, 291–296. doi: 10.1038/nature09358
Petronilli, V., Cola, C., Bernardi, P. (1993). Modulation of the mitochondrial cyclosporin A-sensitive permeability transition pore. II. The minimal requirements for pore induction underscore a key role for transmembrane electrical potential, matrix pH, and matrix Ca2+. J. Biol. Chem. 268, 1011–1016.
Pevarello, P., Cainarca, S., Liberati, C., Tarroni, P., Piscitelli, F., Severi, E. (2014). Ca2+ release-activated Ca2+ channel inhibitors. Pharm. Pat. Anal. 3, 171–182. doi: 10.4155/ppa.14.7
Phan, N. N., Wang, C. Y., Chen, C. F., Sun, Z., Lai, M. D., Lin, Y. C. (2017). Voltage-gated calcium channels: Novel targets for cancer therapy. Oncol. Lett. 14, 2059–2074. doi: 10.3892/ol.2017.6457
Platzer, J., Engel, J., Schrott-Fischer, A., Stephan, K., Bova, S., Chen, H., et al. (2000). Congenital deafness and sinoatrial node dysfunction in mice lacking class D L-type Ca2+ channels. Cell 102, 89–97. doi: 10.1016/S0092-8674(00)00013-1
Poch, M. A., Mehedint, D., Green, D. J., Payne-Ondracek, R., Fontham, E. T. H., Bensen, J. T., et al. (2013). The association between calcium channel blocker use and prostate cancer outcome. Prostate 73, 865–872. doi: 10.1002/pros.22632
Pozzan, T., Magalhães, P., Rizzuto, R. (2000). The comeback of mitochondria to calcium signalling. Cell Calcium 28, 279–283. doi: 10.1054/ceca.2000.0166
Prakriya, M., Lewis, R. S. (2001). Potentiation and inhibition of Ca(2+) release-activated Ca(2+) channels by 2-aminoethyldiphenyl borate (2-APB) occurs independently of IP(3) receptors. J. Physiol. 536, 3–19. doi: 10.1111/j.1469-7793.2001.t01-1-00003.x
Prevarskaya, N., Flourakis, M., Bidaux, G., Thebault, S., Skryma, R. (2007). Differential role of TRP channels in prostate cancer. in. Biochem. Soc. Trans. 35, 133–135. doi: 10.1042/BST0350133
Prevarskaya, N., Skryma, R., Shuba, Y. (2011). Calcium in tumour metastasis: new roles for known actors. Nat. Rev. Cancer 11, 609–618. doi: 10.1038/nrc3105
Prevarskaya, N., Ouadid-Ahidouch, H., Skryma, R., Shuba, Y. (2014). Remodelling of Ca2+ transport in cancer: how it contributes to cancer hallmarks? Philos. Trans. R. Soc Lond. B. Biol. Sci. 369, 20130097. doi: 10.1098/rstb.2013.0097
Prevarskaya, N., Skryma, R., Shuba, Y. (2018). Ion channels in cancer: Are cancer hallmarks oncochannelopathies? Physiol. Rev. 98, 559–621. doi: 10.1152/physrev.00044.2016
Putney, J. W. (2018). Forms and Functions of Store-operated Calcium Entry Mediators, STIM and Orai. Adv. Bio. Regul. 68, 88–96. doi: 10.1016/j.jbior.2017.11.006
Quintana, A., Pasche, M., Junker, C., Al-Ansary, D., Rieger, H., Kummerow, C., et al. (2011). Calcium microdomains at the immunological synapse: how ORAI channels, mitochondria and calcium pumps generate local calcium signals for efficient T-cell activation. EMBO J. 30, 3895–3912. doi: 10.1038/emboj.2011.289
Rao, J. N., Rathor, N., Zhuang, R., Zou, T., Liu, L., Xiao, L., et al. (2012). Polyamines regulate intestinal epithelial restitution through TRPC1-mediated Ca2+ signaling by differentially modulating STIM1 and STIM2. Am. J. Physiol. Cell Physiol. 303, C308–C317. doi: 10.1152/ajpcell.00120.2012
Raphaël, M., Lehen’kyi, V., Vandenberghe, M., Beck, B., Khalimonchyk, S., Abeele, F. V., et al. (2014). TRPV6 calcium channel translocates to the plasma membrane via Orai1-mediated mechanism and controls cancer cell survival. Proc. Natl. Acad. Sci. U. S. A. 111, E3870–E3879. doi: 10.1073/pnas.1413409111
Reuter, H., Seitz, N. (1968). The dependence of calcium efflux from cardiac muscle on temperature and external ion composition. J. Physiol. 195, 451–470. doi: 10.1113/jphysiol.1968.sp008467
Rizzuto, R., Pinton, P., Carrington, W., Fay, F. S., Fogarty, K. E., Lifshitz, L. M., et al. (1998). Close contacts with the endoplasmic reticulum as determinants of mitochondrial Ca2+ responses. Sci. (80-. ). 280, 1763–1766. doi: 10.1126/science.280.5370.1763
Rodriguez, C., Jacobs, E. J., Deka, A., Patel, A. V., Bain, E. B., Thun, M. J., et al. (2009). Use of blood-pressure-lowering medication and risk of prostate cancer in the Cancer Prevention Study II Nutrition Cohort. Cancer Causes Control 20, 671–679. doi: 10.1007/s10552-008-9280-0
Rostom, A., Dubé, C., Lewin, G., Tsertsvadze, A., Barrowman, N., Code, C., et al. (2007). Nonsteroidal anti-inflammatory drugs and cyclooxygenase-2 inhibitors for primary prevention of colorectal cancer: a systematic review prepared for the U.S. Preventive Services Task Force. Ann. Intern. Med. 146, 376–389. doi: 10.7326/0003-4819-146-5-200703060-00010
Rothwell, P. M., Price, J. F., Fowkes, F. G. R., Zanchetti, A., Roncaglioni, M. C., Tognoni, G., et al. (2012). Short-term effects of daily aspirin on cancer incidence, mortality, and non-vascular death: Analysis of the time course of risks and benefits in 51 randomised controlled trials. Lancet 379, 1602–1612. doi: 10.1016/S0140-6736(11)61720-0
Rotshild, V., Azoulay, L., Zarifeh, M., Masarwa, R., Hirsh-Raccah, B., Perlman, A., et al. (2018). The Risk for Lung Cancer Incidence with Calcium Channel Blockers: A Systematic Review and Meta-Analysis of Observational Studies. Drug Saf. 41, 555–564. doi: 10.1007/s40264-018-0644-4
Ruano, Y., Mollejo, M., Ribalta, T., Fiaño, C., Camacho, F., II, Gómez, E., et al. (2006). Identification of novel candidate target genes in amplicons of Glioblastoma multiforme tumors detected by expression and CGH microarray profiling. Mol. Cancer 5, 1–12. doi: 10.1186/1476-4598-5-39
Rybarczyk, P., Gautier, M., Hague, F., Dhennin-Duthille, I., Chatelain, D., Kerr-Conte, J., et al. (2012). Transient receptor potential melastatin-related 7 channel is overexpressed in human pancreatic ductal adenocarcinomas and regulates human pancreatic cancer cell migration. Int. J. Cancer. 131, E851–E861. doi: 10.1002/ijc.27487
Sánchez-Jiménez, F., Medina, M.Á, Villalobos-Rueda, L., Urdiales, J. L. (2019). Polyamines in mammalian pathophysiology. Cell. Mol. Life Sci. 76, 3987–4008. doi: 10.1007/s00018-019-03196-0
Sabates-Bellver, J., Van Der Flier, L. G., De Palo, M., Cattaneo, E., Maake, C., Rehrauer, H., et al. (2007). Transcriptome profile of human colorectal adenomas. Mol. Cancer Res. 5, 1263–1275. doi: 10.1158/1541-7786.MCR-07-0267
Sadaghiani, A. M., Lee, S. M., Odegaard, J., II, Leveson-Gower, D. B., McPherson, O. M., Novick, P., et al. (2014). Identification of Orai1 channel inhibitors by using minimal functional domains to screen small molecule microarrays. Chem. Biol. 21, 1278–1292. doi: 10.1016/j.chembiol.2014.08.016
Sakakura, C., Hagiwara, A., Fukuda, K., Shimomura, K., Takagi, T., Kin, S., et al. (2003). Possible involvement of inositol 1,4,5-trisphosphate receptor type 3 (IP3R3) in the peritoneal dissemination of gastric cancers. Anticancer Res. 23, 3691–3697.
Samanta, K., Mirams, G. R., Parekh, A. B. (2018). Sequential forward and reverse transport of the Na+ Ca2+ exchanger generates Ca2+ oscillations within mitochondria. Nat. Commun. 9, 1–10. doi: 10.1038/s41467-017-02638-2
Samanta, K., Bakowski, D., Amin, N., Parekh, A. B. (2019). The whole-cell Ca 2+ release-activated Ca 2+ current, I CRAC , is regulated by the mitochondrial Ca 2+ uniporter channel and is independent of extracellular and cytosolic Na +. J. Physiol. 598, 1753–1773. doi: 10.1113/JP276551
Sampath, B., Sankaranarayanan, K. (2016). Glu106 targeted inhibitors of ORAI1 as potential Ca2+ release-activated Ca2+ (CRAC) channel blockers - molecular modeling and docking studies. J. Recept. Signal Transduction Res. 36, 572–585. doi: 10.3109/10799893.2016.1141956
Sancak, Y., Markhard, A. L., Kitami, T., Kovács-Bogdán, E., Kamer, K. J., Udeshi, N. D., et al. (2013). EMRE is an essential component of the mitochondrial calcium uniporter complex. Sci. (80-. ). 342, 1379–1382. doi: 10.1126/science.1242993
Scatena, R. (2012). Mitochondria and drugs. Adv. Exp. Med. Biol. 942, 329–346. doi: 10.1007/978-94-007-2869-1_15
Schindl, R., Bergsmann, J., Frischauf, I., Derler, I., Fahrner, M., Muik, M., et al. (2008). 2-aminoethoxydiphenyl borate alters selectivity of Orai3 channels by increasing their pore size. J. Biol. Chem. 283, 20261–20267. doi: 10.1074/jbc.M803101200
Schmidt, U., Fuessel, S., Koch, R., Baretton, G. B., Lohse, A., Tomasetti, S., et al. (2006). Quantitative multi-gene expression profiling of primary prostate cancer. Prostate 66, 1521–1534. doi: 10.1002/pros.20490
Schwab, A., Fabian, A., Hanley, P. J., Stock, C. (2012). Role of ion channels and transporters in cell migration. Physiol. Rev. 92, 1865–1913. doi: 10.1152/physrev.00018.2011
Scrideli, C. A., Carlotti, C. G., Okamoto, O. K., Andrade, V. S., Cortez, M. A. A., Motta, F. J. N., et al. (2008). Gene expression profile analysis of primary glioblastomas and non-neoplastic brain tissue: Identification of potential target genes by oligonucleotide microarray and real-time quantitative PCR. J. Neurooncol. 88, 281–291. doi: 10.1007/s11060-008-9579-4
Secondo, A., Bagetta, G., Amantea, D. (2018). On the role of store-operated calcium entry in acute and chronic neurodegenerative diseases. Front. Mol. Neurosci. 11, 1–14. doi: 10.3389/fnmol.2018.00087
Smyth, J. T., DeHaven, W., II, Bird, G. S., Putney, J. W. (2008). Ca2+-store-dependent and -independent reversal of Stim1 localization and function. J. Cell Sci. 121, 762–772. doi: 10.1242/jcs.023903
Sobradillo, D., Hernández-Morales, M., Ubierna, D., Moyer, M. P., Núñez, L., Villalobos, C. (2014). A reciprocal shift in transient receptor potential channel 1 (TRPC1) and stromal interaction molecule 2 (STIM2) contributes to Ca2+ remodeling and cancer hallmarks in colorectal carcinoma cells. J. Biol. Chem. 289, 28765–28782. doi: 10.1074/jbc.M114.581678
Sporn, M. B., Hong, W. K. (2008). Concomitant DFMO and sulindac chemoprevention of colorectal adenomas: A major clinical advance. Nat. Clin. Pract. Oncol. 5, 628–629. doi: 10.1038/ncponc1221
Srinivasan, S., Chitalia, V., Meyer, R. D., Hartsough, E., Mehta, M., Harrold, I., et al. (2015). Hypoxia-induced expression of phosducin-like 3 regulates expression of VEGFR-2 and promotes angiogenesis. Angiogenesis. 18, 449–462. doi: 10.1007/s10456-015-9468-3
Stanisz, H., Saul, S., Müller, C. S. L., Kappl, R., Niemeyer, B. A., Vogt, T., et al. (2014). Inverse regulation of melanoma growth and migration by Orai1/STIM2-dependent calcium entry. Pigment Cell Melanoma Res. 27, 442–453. doi: 10.1111/pcmr.12222
Stauderman, K. A. (2018). CRAC channels as targets for drug discovery and development. Cell Calcium 74, 147–159. doi: 10.1016/j.ceca.2018.07.005
Streb, H., Irvine, R. F., Berridge, M. J., Schulz, I. (1983). Release of Ca2+ from a nonmitochondrial intracellular store in pancreatic acinar cells by inositol-1,4,5-trisphosphate. Nature 306, 67–69. doi: 10.1038/306067a0
Sun, L., Hui, A. M., Su, Q., Vortmeyer, A., Kotliarov, Y., Pastorino, S., et al. (2006). Neuronal and glioma-derived stem cell factor induces angiogenesis within the brain. Cancer Cell 9, 287–300. doi: 10.1016/j.ccr.2006.03.003
Sun, R., Yang, Y., Ran, X., Yang, T. (2016). Calcium Influx of Mast Cells Is Inhibited by Aptamers Targeting the First Extracellular Domain of Orai1. PloS One 11, e0158223. doi: 10.1371/journal.pone.0158223
Sweeney, Z. K., Minatti, A., Button, D. C., Patrick, S. (2009). Small-Molecule Inhibitors of Store-Operated Calcium Entry. ChemMedChem 4, 706–718. doi: 10.1002/cmdc.200800452
Tajada, S., Cidad, P., Colinas, O., Santana, L. F., López-López, J. R., Pérez-García, M. T. (2013). Down-regulation of CaV1.2 channels during hypertension: How fewer CaV1.2 channels allow more Ca2+ into hypertensive arterial smooth muscle. J. Physiol. 591, 6175–6191. doi: 10.1113/jphysiol.2013.265751
Tajada, S., Moreno, C. M., O’Dwyer, S., Woods, S., Sato, D., Navedo, M. F., et al. (2017). Distance constraints on activation of TRPV4 channels by AKAP150-bound PKCα in arterial myocytes. J. Gen. Physiol. 149, 639–659. doi: 10.1085/jgp.201611709
Takezawa, R., Cheng, H., Beck, A., Ishikawa, J., Launay, P., Kubota, H., et al. (2006). A pyrazole derivative potently inhibits lymphocyte Ca2+ influx and cytokine production by facilitating transient receptor potential melastatin 4 channel activity. Mol. Pharmacol. 69, 1413–1420. doi: 10.1124/mol.105.021154
Tao, L., Harris, A. L. (2007). 2-Aminoethoxydiphenyl borate directly inhibits channels composed of connexin26 and/or connexin32. Mol. Pharmacol. 71, 570–579. doi: 10.1124/mol.106.027508
Tao, X., Zhao, N., Jin, H., Zhang, Z., Liu, Y., Wu, J., et al. (2013). FSH enhances the proliferation of ovarian cancer cells by activating transient receptor potential channel C3. Endocr. Relat. Cancer 20, 415–429. doi: 10.1530/ERC-12-0005
Taylor, J. M., Simpson, R. U. (1992). Inhibition of Cancer Cell Growth by Calcium Channel Antagonists in the Athymic Mouse. Cancer Res. 52, 2413–2418.
Taylor, J. T., Huang, L., Pottle, J. E., Liu, K., Yang, Y., Zeng, X., et al. (2008). Selective blockade of T-type Ca2+ channels suppresses human breast cancer cell proliferation. Cancer Lett. 267, 116–124. doi: 10.1016/j.canlet.2008.03.032
Tegeder, I., Pfeilschifter, J., Geisslinger, G. (2001). Cyclooxygenase-independent actions of cyclooxygenase inhibitors. FASEB J. 15, 2057–2072. doi: 10.1096/fj.01-0390rev
Thompson, P. A., Wertheim, B. C., Zell, J. A., Chen, W. P., McLaren, C. E., Lafleur, B. J., et al. (2010). Levels of rectal mucosal polyamines and prostaglandin E2 predict ability of DFMO and sulindac to prevent colorectal adenoma. Gastroenterology 139, 797–805, 805.e1. doi: 10.1053/j.gastro.2010.06.005
Tian, C., Du, L., Zhou, Y., Li, M. (2016). Store-operated CRAC channel inhibitors: Opportunities and challenges. Future Med. Chem. 8, 817–832. doi: 10.4155/fmc-2016-0024
Trebak, M., Bird, G. S. J., McKay, R. R., Putney, J. W. (2002). Comparison of human TRPC3 channels in receptor-activated and store-operated modes. Differential sensitivity to channel blockers suggests fundamental differences in channel composition. J. Biol. Chem. 277, 21617–21623. doi: 10.1074/jbc.M202549200
Umemura, M., Baljinnyam, E., Feske, S., De Lorenzo, M. S., Xie, L. H., Feng, X., et al. (2014). Store-operated Ca2+ entry (SOCE) regulates melanoma proliferation and cell migration. PloS One 9, 1–11. doi: 10.1371/journal.pone.0089292
Várnai, P., Hunyady, L., Balla, T. (2009). STIM and Orai: the long-awaited constituents of store-operated calcium entry. Trends Pharmacol. Sci. 30, 118–128. doi: 10.1016/j.tips.2008.11.005
Valero, R. A., Senovilla, L., Núñez, L., Villalobos, C. (2008). The role of mitochondrial potential in control of calcium signals involved in cell proliferation. Cell Calcium. 44, 259–269. doi: 10.1016/j.ceca.2007.12.002
Veliceasa, D., Ivanovic, M., Hoepfner, F. T.-S., Thumbikat, P., Volpert, O. V., Smith, N. D. (2007). Transient potential receptor channel 4 controls thrombospondin-1 secretion and angiogenesis in renal cell carcinoma. FEBS J. 274, 6365–6377. doi: 10.1111/j.1742-4658.2007.06159.x
Verkhratsky, A. (2005). Physiology and pathophysiology of the calcium store in the endoplasmic reticulum of neurons. Physiol. Rev. 85, 201–279. doi: 10.1152/physrev.00004.2004
Villalobos, C., Núñez, L., Chamero, P., Alonso, M. T., García-Sancho, J. (2001). Mitochondrial [Ca2+] Oscillations Driven by Local High [Ca 2+] Domains Generated by Spontaneous Electric Activity. J. Biol. Chem. 276, 40293–40297. doi: 10.1074/jbc.C100465200
Villalobos, C., Sobradillo, D., Hernández-Morales, M., Núñez, L. (2016). “Remodeling of calcium entry pathways in cancer,” in Advances in Experimental Medicine and Biology (New York City (USA): Springer New York LLC), 449–466. doi: 10.1007/978-3-319-26974-0_19
Villalobos, C., Sobradillo, D., Hernández-Morales, M., Núñez, L. (2017). Calcium remodeling in colorectal cancer. Biochim. Biophys. Acta - Mol. Cell Res. 1864, 843–849. doi: 10.1016/j.bbamcr.2017.01.005
Villalobos, C., Gutiérrez, L. G., Hernández-Morales, M., del Bosque, D., Núñez, L. (2018). Mitochondrial control of store-operated Ca 2+ channels in cancer: Pharmacological implications. Pharmacol. Res. 135, 136–143. doi: 10.1016/j.phrs.2018.08.001
Villalobos, C., Hernández-Morales, M., Gutiérrez, L. G., Núñez, L. (2019). TRPC1 and ORAI1 channels in colon cancer. Cell Calcium 81, 59–66. doi: 10.1016/j.ceca.2019.06.003
Wang, S. Q., Song, L. S., Lakatta, E. G., Cheng, H. (2001). Ca2+ signalling between single L-type Ca2+ channels and ryanodine receptors in heart cells. Nature 410, 592–596. doi: 10.1038/35069083
Wang, Y., Deshpande, M., Payne, R. (2002). 2-Aminoethoxydiphenyl borate inhibits phototransduction and blocks voltage-gated potassium channels in Limulus ventral photoreceptors. Cell Calcium 32, 209–216. doi: 10.1016/S0143416002001562
Wang, J., Liao, Q. J., Zhang, Y., Zhou, H., Luo, C. H., Tang, J., et al. (2014). TRPM7 is required for ovarian cancer cell growth, migration and invasion. Biochem. Biophys. Res. Commun. 454, 547–553. doi: 10.1016/j.bbrc.2014.10.118
Wang, C. Y., Lai, M. D., Phan, N. N., Sun, Z., Lin, Y. C. (2015a). Meta-analysis of public microarray datasets reveals voltage-gated calcium gene signatures in clinical cancer patients. PloS One. 10. doi: 10.1371/journal.pone.0125766
Wang, J. Y., Sun, J., Huang, M. Y., Wang, Y. S., Hou, M. F., Sun, Y., et al. (2015b). STIM1 overexpression promotes colorectal cancer progression, cell motility and COX-2 expression. Oncogene 34, 4358–4367. doi: 10.1038/onc.2014.366
Wang, Y., Wang, H., Li, L., Li, J., Pan, T., Zhang, D., et al. (2016). Elevated expression of STIM1 is involved in lung tumorigenesis. Oncotarget 7, 86584–86593. doi: 10.18632/oncotarget.13359
Wang, C., Ruan, P., Zhao, Y., Li, X., Wang, J., Wu, X., et al. (2017). Spermidine/spermine N1-acetyltransferase regulates cell growth and metastasis via AKT/β-catenin signaling pathways in hepatocellular and colorectal carcinoma cells. Oncotarget 8, 1092–1109. doi: 10.18632/oncotarget.13582
Wang, Z. (2004). Roles of K+ channels in regulating tumour cell proliferation and apoptosis. Pflugers Arch. Eur. J. Physiol. 448, 274–286. doi: 10.1007/s00424-004-1258-5
Warnier, M., Roudbaraki, M., Derouiche, S., Delcourt, P., Bokhobza, A., Prevarskaya, N., et al. (2015). CACNA2D2 promotes tumorigenesis by stimulating cell proliferation and angiogenesis. Oncogene 34, 5383–5394. doi: 10.1038/onc.2014.467
Weber, A. A., Yildirim, H., Schrör, K. (2000). Cyclooxygenase-independent inhibition of smooth muscle cell mitogenesis by ibuprofen. Eur. J. Pharmacol. 389, 67–69. doi: 10.1016/S0014-2999(99)00868-7
Wei, C., Wang, X., Chen, M., Ouyang, K., Song, L. S., Cheng, H. (2009). Calcium flickers steer cell migration. Nature 457, 901–905. doi: 10.1038/nature07577
Weiss, T. S., Bernhardt, G., Buschauer, A., Thasler, W. E., Dolger, D., Zirngibl, H., et al. (2002). Polyamine levels of human colorectal adenocarcinomas are correlated with tumor stage and grade. Int. J. Colorectal Dis. 17, 381–387. doi: 10.1007/s00384-002-0394-7
Wen, L., Liang, C., Chen, E., Chen, W., Liang, F., Zhi, X., et al. (2016). Regulation of Multi-drug Resistance in hepatocellular carcinoma cells is TRPC6/Calcium Dependent. Sci. Rep. 6, 1–14. doi: 10.1038/srep23269
Wissenbach, U., Niemeyer, B. A., Fixemer, T., Schneidewind, A., Trost, C., Cavalié, A., et al. (2001). Expression of CaT-like, a Novel Calcium-selective Channel, Correlates with the Malignancy of Prostate Cancer. J. Biol. Chem. 276, 19461–19468. doi: 10.1074/jbc.M009895200
Wu, C., Fernandez, S. A., Criswell, T., Chidiac, T. A., Guttridge, D., Villalona-Calero, M., et al. (2013). Disrupting cytokine signaling in pancreatic cancer: a phase I/II study of etanercept in combination with gemcitabine in patients with advanced disease. Pancreas 42, 813–818. doi: 10.1097/MPA.0b013e318279b87f
Wulff, H., Castle, N. A., Pardo, L. A. (2009). Voltage-gated potassium channels as therapeutic targets. Nat. Rev. Drug Discovery 8, 982–1001. doi: 10.1038/nrd2983
Xiao, X., Li, B. X., Mitton, B., Ikeda, A., Sakamoto, K. M. (2010). Targeting CREB for Cancer Therapy: Friend or Foe. Curr. Cancer Drug Targets 10, 384–391. doi: 10.2174/156800910791208535
Xie, B., Zhao, R., Bai, B., Wu, Y., Xu, Y., Lu, S., et al. (2018). Identification of key tumorigenesis-related genes and their microRNAs in colon cancer. Oncol. Rep. 40, 3551–3560. doi: 10.3892/or.2018.6726
Yáñez, M., Gil-Longo, J., Campos-Toimil, M. (2012). Calcium binding proteins. Adv. Exp. Med. Biol. 740, 461–482. doi: 10.1007/978-94-007-2888-2_19
Yamashita, M., Somasundaram, A., Prakriya, M. (2011). Competitive modulation of Ca2+ release-activated Ca2+ channel gating by STIM1 and 2-aminoethyldiphenyl borate. J. Biol. Chem. 286, 9429–9442. doi: 10.1074/jbc.M110.189035
Yang, S. L., Cao, Q., Zhou, K. C., Feng, Y. J., Wang, Y. Z. (2009a). Transient receptor potential channel C3 contributes to the progression of human ovarian cancer. Oncogene 28, 1320–1328. doi: 10.1038/onc.2008.475
Yang, S., Zhang, J. J., Huang, X. Y. (2009b). Orai1 and STIM1 Are Critical for Breast Tumor Cell Migration and Metastasis. Cancer Cell 15, 124–134. doi: 10.1016/j.ccr.2008.12.019
Yang, N., Tang, Y., Wang, F., Zhang, H., Xu, D., Shen, Y., et al. (2013). Blockade of store-operated Ca2+ entry inhibits hepatocarcinoma cell migration and invasion by regulating focal adhesion turnover. Cancer Lett. 330, 163–169. doi: 10.1016/j.canlet.2012.11.040
Yang, W., Li, Y., Ai, Y., Obianom, O. N., Guo, D., Yang, H., et al. (2019). Pyrazole-4-Carboxamide (YW2065): A Therapeutic Candidate for Colorectal Cancer via Dual Activities of Wnt/β-Catenin Signaling Inhibition and AMP-Activated Protein Kinase (AMPK) Activation. J. Med. Chem. 62, 11151–11164. doi: 10.1021/acs.jmedchem.9b01252
Yao, Y., Choi, J., Parker, I. (1995). Quantal puffs of intracellular Ca2+ evoked by inositol trisphosphate in Xenopus oocytes. J. Physiol. 482, 533–553. doi: 10.1113/jphysiol.1995.sp020538
Yee, N. S., Zhou, W., Lee, M. (2010). Transient receptor potential channel TRPM8 is over-expressed and required for cellular proliferation in pancreatic adenocarcinoma. Cancer Lett. 297, 49–55. doi: 10.1016/j.canlet.2010.04.023
Yee, N. S., Kazi, A. A., Li, Q., Yang, Z., Berg, A., Yee, R. K. (2015). Aberrant over-expression of TRPM7 ion channels in pancreatic cancer: Required for cancer cell invasion and implicated in tumor growth and metastasis. Biol. Open. 4, 507–514. doi: 10.1242/bio.20137088
Yeromin, A. V., Zhang, S. L., Jiang, W., Yu, Y., Safrina, O., Cahalan, M. D. (2006). Molecular identification of the CRAC channel by altered ion selectivity in a mutant of Orai. Nature 443, 226–229. doi: 10.1038/nature05108
Yu, D., Holm, R., Goscinski, M. A., Trope, C. G., Nesland, J. M., Suo, Z. (2016). Prognostic and clinicopathological significance of Cacna2d1 expression in epithelial ovarian cancers: A retrospective study. Am. J. Cancer Res. 6, 2088–2097.
Yu, S., Huang, S., Ding, Y., Wang, W., Wang, A., Lu, Y. (2019). Transient receptor potential ion-channel subfamily V member 4: a potential target for cancer treatment. Cell Death Dis. 10, 1–17. doi: 10.1038/s41419-019-1708-9
Yurgelun, M. B., Hampel, H. (2018). Recent Advances in Lynch Syndrome: Diagnosis, Treatment, and Cancer Prevention. Am. Soc Clin. Oncol. Educ. B. 38, 101–109. doi: 10.1200/edbk_208341
Zhu, H., Zhang, H., Jin, F., Fang, M., Huang, M., Yang, C. S., et al. (2014a). Elevated Orai1 expression mediates tumor-promoting intracellular Ca 2+ oscillations in human esophageal squamous cell carcinoma. Oncotarget. 5, 3455–3471. doi: 10.18632/oncotarget.1903
Zhu, M., Chen, L., Zhao, P., Zhou, H., Zhang, C., Yu, S., et al. (2014b). Store-operated Ca2+ entry regulates glioma cell migration and invasion via modulation of Pyk2 phosphorylation. J. Exp. Clin. Cancer Res. 33, 98. doi: 10.1186/s13046-014-0098-1
Zhuang, L., Peng, J., Tou, L., Takanaga, H., Adam, R. M., Hediger, M. A., et al. (2002). Calcium-selective ion channel, CaT1, is apically localized in gastrointestinal tract epithelia and is aberrantly expressed in human malignancies. Lab. Investig. 82, 1755–1764. doi: 10.1097/01.LAB.0000043910.41414.E7
Keywords: Ca2+ channels, cancer hallmarks, store-operated Ca2+ entry, TRP channels, calcium channel modulators in cancer
Citation: Tajada S and Villalobos C (2020) Calcium Permeable Channels in Cancer Hallmarks. Front. Pharmacol. 11:968. doi: 10.3389/fphar.2020.00968
Received: 10 March 2020; Accepted: 15 June 2020;
Published: 07 July 2020.
Edited by:
Sébastien Roger, Université de Tours, FranceReviewed by:
Juan Carlos Gomora, National Autonomous University of Mexico, MexicoDimitra Gkika, Lille University of Science and Technology, France
Copyright © 2020 Tajada and Villalobos. This is an open-access article distributed under the terms of the Creative Commons Attribution License (CC BY). The use, distribution or reproduction in other forums is permitted, provided the original author(s) and the copyright owner(s) are credited and that the original publication in this journal is cited, in accordance with accepted academic practice. No use, distribution or reproduction is permitted which does not comply with these terms.
*Correspondence: Sendoa Tajada, sendoa.tajada@uva.es