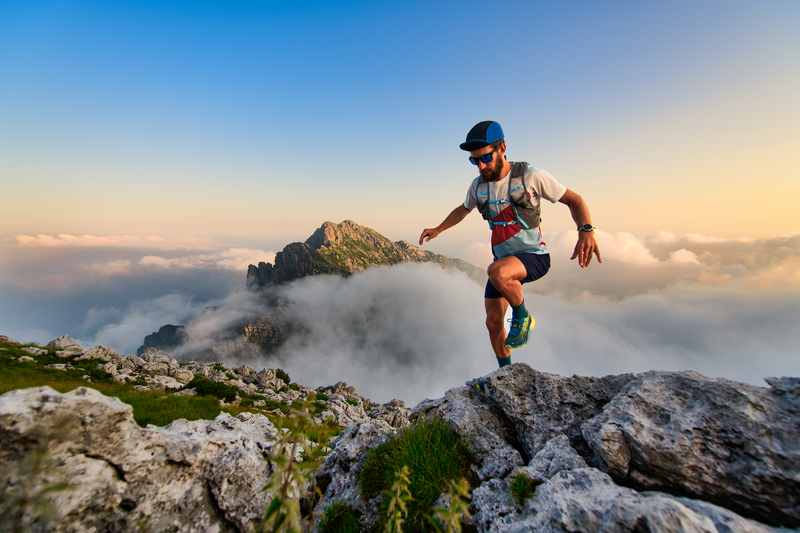
95% of researchers rate our articles as excellent or good
Learn more about the work of our research integrity team to safeguard the quality of each article we publish.
Find out more
OPINION article
Front. Pharmacol. , 10 December 2019
Sec. Translational Pharmacology
Volume 10 - 2019 | https://doi.org/10.3389/fphar.2019.01475
This article is part of the Research Topic Potential of Extracellular Matrix Molecules in Pharmaceutical Development View all 9 articles
As a leading cause of disability among adults, osteoarthritis (OA) leads to serious public health and economic burdens. Currently, treatment options for OA are generally based on symptom severity and duration, with the goals of symptom alleviation and improvement in functional status (Taruc-Uy and Lynch, 2013). Nonpharmacologic and pharmacologic strategies are used initially, while a surgical approach to OA is reserved for chronic cases when these treatments failed. Unfortunately, the currently available clinical pharmacologic treatments for OA, such as analgesia, glucocorticoids, non-steroidal anti-inflammatory drugs, and disease-modifying antirheumatic drugs, are not adequately effective (Chevalier et al., 2009; Scott, 2010; Verbruggen et al., 2012; Chevalier et al., 2015; Appleton, 2018; Li and Zheng, 2018; Li et al., 2018), and generally associated with a diversity of adverse side-effects (Habib et al., 2010; Cooper et al., 2016; Compston, 2018). For instance, analgesia does not reduce inflammation and cartilage damage (Appleton, 2018), glucocorticoids have been reported to induce severe damages in the musculoskeletal, cardiovascular, and gastrointestinal systems (Cooper et al., 2016; Compston, 2018), and non-steroidal anti-inflammatory drugs do not actively control arthritis progression (Appleton, 2018). Accumulating evidence demonstrates that an ideal OA-combating agent should be able to reduce inflammation and promote cartilage regeneration safely, which has long been desired. In responding to this demand, the current strategy for disease-modifying osteoarthritis drug seeking has shifted to biologoical molecules that promote chondorgenic development and regenration.
To date, a diversity of well-known pro-chondrogenic growth factors, such as bone morphogenetic proteins (BMPs) and transforming growth factors (TGFs), have been examined for OA treatment. However, the results are not optimistic since intra-articular injection of these growth factors could even enhance the inflammatory infiltration in damaged joints (Allen et al., 1990; Fava et al., 1991; Hong et al., 2009). Meanwhile, multiple transcriptional factors that potentially suppress inflammation, such as nuclear factor of activated T cells 1 (NFATc1), NFATc2, and runt-related transcription factor 1 (RUNX1), have also be introduced in this arena against OA, while they do not hold much promise presently. For example, the function of NFATc proteins in arthritis is controversial (Yaykasli et al., 2009; Miclea et al., 2011; Greenblatt et al., 2013).
Another possibility for fighting OA is utilizing the extracellular matrix (ECM) molecules that naturally distribute in the articular cartilage. For example, fibromodulin (FMOD) is an ECM protein with multiple keratin sulfate side-chains that belongs to the small leucine-rich proteoglycan family (Plaas et al., 1990). It was first identified as a collagen-binding molecule broadly distributed in connective tissues, with particularly high expression in cartilage (Hedbom and Heinegard, 1989). In the past three decades, in addition to the broad attention of its effects on collagen fibrillogenesis (Chen et al., 2010), muscle development (Lee et al., 2018a; Lee et al., 2018b), cell reprogramming (Zheng et al., 2012; Li et al., 2016; Zheng et al., 2019), angiogenesis (Jian et al., 2013; Zheng et al., 2014; Ao et al., 2017), wound healing (Zheng et al., 2017), and tumorigenesis (Pourhanifeh et al., 2019), the involvement of FMOD in cartilage development and maintenance as well as arthritis progression, especially in temporomandibular joint (TMJ) OA, has been investigated through world-wide collaboration. Here, we review the current research investigating FMOD and arthritis, and aim to provide novel insight into the potential use of FMOD for OA management.
An investigation that focused on mouse glenohumeral joints demonstrated that, at 12–13 days post-coitus when the limb buds are just condensing mesenchymal cells, FMOD was not detectable at the protein level (Murphy et al., 1999). Intense FMOD staining was first noticed at the surface of the scapular and humeral anlage intracellularly and pericellularly in the interzone at 14–15 days post-coitus (Murphy et al., 1999). Starting from 17 days post-coitus, a strong FMOD signal was found in the ECM surrounding the chondrocytes at the surface of the joints and proliferating chondrocytes in the epiphyses of the humerus and scapula (Murphy et al., 1999). Meanwhile, during postnatal maturation until adulthood, FMOD was detected throughout the ECM of the developing articular surface and the growth plate but was more abundant in articular cartilage (Murphy et al., 1999). Since FMOD was associated with prechondrocytic mesenchymal cells in the interzone before joint cavitation and with developing articular chondrocytes in the maturing and young adult limbs, it has been proposed that FMOD may function in the early genesis of articular cartilage (Murphy et al., 1999).
It is worth noting that FMOD shared a similar temporospatial transcriptional pattern with type II collagen in mouse knee joints during postnatal development, while FMOD gene expression reached the maximum level at 1 month old (Saamanen et al., 2001). FMOD transcription was restricted to chondrocytes and peaked in the proliferating zone and the early articular cartilage (Saamanen et al., 2001), which had been confirmed at the protein level by immunostaining (Murphy et al., 1999). In mature animals, in situ hybridization revealed that both pericellular and interterritorial cartilage at knee joints had high FMOD expression with the highest intensity in the middle and deep zones of the uncalcified cartilage (Saamanen et al., 2001). At 6 months old, FMOD staining decreased in the uncalcified cartilage but increased in the calcified cartilage (Saamanen et al., 2001). FMOD was also detected in the hypertrophic chondrocytes of the secondary ossification centers and growth plate of mice at 10 days old, and transcription of FMOD was diminished and finally disappeared with maturation and aging of the trabecular epiphyses (Saamanen et al., 2001).
In addition to its spatiotemporal distribution, FMOD’s structural heterogeneity was also noticed during articular cartilage growth and development. For instance, FMOD isolated from young articular cartilage carries neither α(2-6)-linked N-acetylneuraminic acid nor α(1-3)-linked fucose in the N-linked keratan sulfate chains (Lauder et al., 1996). Meanwhile, an age-related increase has been observed in the abundance of both α(2-6)-linked N-acetylneuraminic acid and α(1-3)-linked fucose, but not the levels of galactose sulfation (Lauder et al., 1998). Western blot showed FMOD-derived from fetal and neonatal articular cartilage (f/n-FMOD) as a diffused region with a relative molecular weight of 70–110 kDa (Cs-Szabo et al., 1995; Roughley et al., 1996), while FMOD-derived from mature adult (a-FMOD) was a more discrete component with a relative molecular weight of 67 kDa (Cs-Szabo et al., 1995; Roughley et al., 1996)—larger than the FMOD core protein without post-translational modifications (46 kDa). Interestingly, digesting f/n-FMOD with keratanase II or endo β-galactosidase reduces its molecular weight to a similar level of a-FMOD (Cs-Szabo et al., 1995). Thus, Roughley et al. argued that FMOD might predominantly exist in the proteoglycan form in juvenile cartilage tissues but is mainly in a glycoprotein form in the adult counterparts (Roughley et al., 1996).
Interestingly, FMOD is one of the small leucine-rich proteoglycans with the most significantly increased protein fragmentation in arthritis compared with macroscopically healthy articular cartilage from the age-matched donors (Melrose et al., 2008). In addition to the 59 kDa band, multiple small bands can be detected by Western blot when FMOD is isolated from articular cartilage of OA and rheumatoid arthritis patients (Cs-Szabo et al., 1995; Roughley et al., 1996; Melrose et al., 2008; Shu et al., 2019). Moreover, when using N-glycosidase to remove the sulfate chains from FMOD isolated from arthritic articular cartilage, several protein bands with the size of 43, 40, and 27 kDa were detected (Cs-Szabo et al., 1995). Therefore, arthritis progression may not only alter the degree and type of its carbohydrate substation but also lead to the breakage of the FMOD core protein.
Meanwhile, degradation of FMOD core protein was also observed in interleukin (IL)-1-challenged cartilage (Sztrolovics et al., 1999; Shu et al., 2019)—a representative model that elucidates the genetic and molecular pathogenesis of inflammation-related secondary OA (Kuyinu et al., 2016). The degradation of FMOD core protein was predominantly catalyzed by matrix metalloproteinases (MMPs) and ADAM metallopeptidases with thrombospondin type 1 motifs (ADAMTSs) (Kashiwagi et al., 2004; Shu et al., 2019). In vitro digestion of healthy human knee cartilage with MMP-13, ADAMTS-4, and ADAMTS-5 generated FMOD fragments of similar sizes as FMOD derived from OA cartilage without digestion (Shu et al., 2019). Notably, the fragmented FMOD is always detected by the antibody recognizing the N-terminal fragment of FMOD but not the one recognizing the C-terminal (Melrose et al., 2008; Shu et al., 2019). One possible explanation is that the C-terminus is vulnerable to the fragmentation and not stably retained in the tissue, and substantially lost into the synovial fluid (Melrose et al., 2008). Importantly, MMP-13 degradation of FMOD resulted in a fragment of 30 kDa, which was also detected in moderately and severely fibrillated cartilage, instead of healthy or slightly fibrillated cartilage (Monfort et al., 2006). These phenomena may support the hypothesis that the sensitivity of FMOD protein fragmentation is increased along with the severity of cartilage degradation.
FMOD-null (Fmod−/−) mice have distinct knee joints in comparison with their wildtype (WT) littermates at 36 weeks old (Gill et al., 2002), accompanied by a significantly higher histological arthritis score (Ameye et al., 2002). In addition, serial sections through FMOD-null mice knees showed degeneration and joint remodeling histologically. More severe incidences of degeneration occurred in the area of the tibial condyles that are uncovered by the menisci, as these sites experience the highest loading stress, resulting in considerable loss of cartilage and bone thickness (Gill et al., 2002). Moreover, the menisci of FMOD-null mice had a markedly less sharp profile with more rounded edges, similar to FMOD-null ligaments, which were also more likely to be damaged compared to WT ligaments. The area of tibial articular cartilage was even more exposed due to degenerated menisci compared to that of the WT littermates (Gill et al., 2002). Furthermore, knee joints of Fmod−/− mice at 80 weeks old displayed full-depth lesions of articular cartilage and clusters of cells that were not seen in the knee joints of WT littermates (Gill et al., 2002).
As biglycan (BGN) and FMOD have overlapping and possible compensatory functions in the joints (Shirakura et al., 2017), BGN and FMOD double-knockout (Bgn−/0/Fmod−/−) mice exhibit an earlier onset of OA than Fmod−/− mice. Bgn−/0/Fmod−/− mice presented with an abnormal gait characterized by the decreased flexibility of knee and ankle joints (dragging leg), which was observed as early as 3 weeks old. Additionally, at 3 months old, the histological arthritis score of the Bgn−/0/Fmod−/− knee joints was significantly higher than that of the WT knee joints. However, the abnormal gait phenomena were observed in neither BGN nor FMOD single knockout mice (Ameye et al., 2002).
Moreover, BGN and FMOD are also highly expressed in the disc and articular cartilage of the TMJ (Wadhwa et al., 2005a). Bgn−/0/Fmod−/− mice developed accelerated OA accompanied by small vertical clefts in the condylar cartilage and partial disruption of the disc as compared to WT animals at 6 months old (Wadhwa et al., 2005b). At 18 months old, extensive cartilage erosion was visible in the Bgn−/0/Fmod−/− mice TMJ (Wadhwa et al., 2005b).
There are several hypotheses about the possible roles of FMOD in arthritis. FMOD binds to collagens (Melching and Roughley, 1999), and fragmentation of FMOD during arthritis progression may destabilize collagen fibrils, rendering them more susceptible to tissue collagenases (Kashiwagi et al., 2004). However, such a difference between WT and FMOD-null mice may not necessarily have immediately visible effects at the ultrastructural level in adults (Ameye et al., 2002).
Alternatively, FMOD may sequester TGF-β/BMP superfamily members in the ECM and thereby prevent their binding to the cellular receptors (Wadhwa et al., 2005a). For example, when treating the TMJ with BMP2, both catabolic and anabolic markers were more profoundly upregulated in the Bgn−/0/Fmod−/− mice than WT animals (Shirakura et al., 2017). This observation suggests that BGN and FMOD could protect the condyle from BMP2-induced matric turnover (Shirakura et al., 2017). Additionally, the sequestration of TGF-β1 in mandibular condylar chondrocyte ECM decreased in Bgn−/0/Fmod−/− mice. The overactive TGF-β1 signal transduction in Bgn−/0/Fmod−/− mice accelerated both production and degradation of type II collagen and aggrecan, and subsequently led to an overall imbalance in ECM turnover that favors cartilage degradation and the onset of OA (Embree et al., 2010).
FMOD may also function as a barrier preventing cell adhesion and subsequent cartilage damage. For example, FMOD administration dramatically prevents the adhesion of polymorphonuclear neutrophils and fibroblasts on articular cartilage surfaces (Noyori and Jasin, 1994; Mitani et al., 2001). This inhibition of cellular attachment may be attributed to the capability of FMOD to mask epitopes of cartilage collagen that face the joint cavity (Noyori and Jasin, 1994).
Furthermore, FMOD may participate in arthritis progression by directly manipulating inflammatory reactions. For instance, C1q and complement inhibitor factor H can directly bind to FMOD but in different regions (Akimoto et al., 2006). However, the deposition of the membrane attack complex and C5a release were lower in the presence of FMOD, presumably due to the formation of the FMOD-factor H complex (Akimoto et al., 2006). Interestingly, IL-1 only stimulates the binding of C1q, but not factor H, to the N-terminal fragment of FMOD in cartilage (Akimoto et al., 2006). Thus, FMOD may balance the activation of the classical complement pathway: when maintained in its intact form, FMOD silences the complement cascade by binding factor H; on the other hand, when FMOD is degraded or fragmented, as seen in OA (Melrose et al., 2008; Shu et al., 2019), the N-terminal FMOD segment binds to C1q and in turn activates the complement system to eliminate pathogens and damaged cells for tissue recovery and reconstruction.
FMOD has been used as an early marker of chondrogenesis (Barry et al., 2001). The expression level of FMOD is inversely correlated with the passage number of human chondrocytes in monolayer cultivation (Lin et al., 2008). In the TMJ cartilage of 3-month-old Bgn−/0/Fmod−/− mice, fewer proliferative chondrocytes were noticed in comparison to that of their WT counterparts (Wadhwa et al., 2005b). Moreover, Bgn−/0/Fmod−/− mice presented with more chondrocyte apoptosis in the articular cartilage than WT mice at the same developmental stage (Wadhwa et al., 2005a). A recent study even showed that microRNA-340-5p negatively regulated OA chondrocyte proliferation while stimulating apoptosis by reducing FMOD expression (Zhang et al., 2018). Nevertheless, the exact function of FMOD in chondrogenesis has yet to be fully uncovered.
As aforementioned, FMOD is a critical ECM component involved in articular cartilage development, growth, aging, and arthritis; however, the exact functions of FMOD during arthritis are still unclear. Take advantage of the development of the Cre/Lox as well as CRISPR-Cas9 recombination system, the specific functions of FMOD during arthritis progression could be deciphered in detail with tissue-specific knockout animal models. Recently, it has been reported that FMOD can be successfully produced and purified from the cell culture supernatant of stable recombinant CHO-K1 cells transfected with a plasmid harboring the human FMOD gene (Zheng et al., 2012; Li et al., 2016; Pourhanifeh et al., 2019). Since FMOD whole protein is now easy to produce, further in-depth investigations are warranted to reveal the underlying mechanism of action of FMOD as a new generation disease-modifying osteoarthritis drug candidate. Last but not least, the plasmid- or virus-mediated expression, as well as directly synthesis, could be utilized to identify the functional sequence(s) of FMOD that regulate(s) cartilage development and pathology, which would further advance the pharmacology application of FMOD.
CL conceived the opinion. CL, PH, and ZZ wrote and revised the manuscript. WJ and CH edited and proofread the manuscript. CL, ZZ, and MZ supervised the writing process and approved the manuscript. All authors reviewed the final manuscript.
ZZ is an inventor on fibromodulin-related patents assigned to UCLA. ZZ is a founder of Scarless Laboratories Inc., which sublicenses fibromodulin-related patents from the UC Regents, who also hold equity in the company. ZZ is also a former officer of Scarless Laboratories Inc.
The handling editor is currently organizing a Research Topic with one of the authors ZZ and confirms the absence of any other collaboration.
The remaining authors declare that the research was conducted in the absence of any commercial or financial relationships that could be construed as a potential conflict of interest.
Akimoto, T., Nonaka, T., Kitamoto, Y., Ishikawa, H., Ninomiya, H., Chikamatsu, K., et al. (2006). Radiation therapy for T2N0 laryngeal cancer: a retrospective analysis for the impact of concurrent chemotherapy on local control. Int. J. Radiat. oncology biology Phys. 64 (4), 995–1001. doi: 10.1016/j.ijrobp.2005.10.003
Allen, J. B., Manthey, C. L., Hand, A. R., Ohura, K., Ellingsworth, L., Wahl, S. M. (1990). Rapid Onset Synovial inflammation and hyperplasia induced by transforming growth factor beta. J. Exp. Med. 171 (1), 231–247. doi: 10.1084/jem.171.1.231
Ameye, L., Aria, D., Jepsen, K., Oldberg, A., Xu, T., Young, M. F. (2002). Abnormal collagen fibrils in tendons of biglycan/fibromodulin-deficient mice lead to gait impairment, ectopic ossification, and osteoarthritis. FASEB J. 16 (7), 673–680. doi: 10.1096/fj.01-0848com
Ao, Z., Yu, S., Qian, P., Gao, W., Guo, R., Dong, X., et al. (2017). Tumor angiogenesis of SCLC inhibited by decreased expression of FMOD via downregulating angiogenic factors of endothelial cells. Biomedicine & Pharmacotherapy 87, 539–547. doi: 10.1016/j.biopha.2016.12.110
Appleton, C. T. (2018). Osteoarthritis year in review 2017: biology. Osteoarthritis Cartilage 26 (3), 296–303. doi: 10.1016/j.joca.2017.10.008
Barry, F., Boynton, R. E., Liu, B., Murphy, J. M. (2001). Chondrogenic differentiation of mesenchymal stem cells from bone marrow: differentiation-dependent gene expression of matrix components. Exp. Cell Res. 268 (2), 189–200. doi: 10.1006/excr.20015278
Chen, S., Oldberg, A., Chakravarti, S., Birk, D. E. (2010). Fibromodulin regulates collagen fibrillogenesis during peripheral corneal development. Dev. Dynamics : An Off. Publ. Am. Assoc. Anatomists 239 (3), 844–854. doi: 10.1002/dvdy.22216
Chevalier, X., Goupille, P., Beaulieu, A. D., Burch, F. X., Bensen, W. G., Conrozier, T., et al. (2009). Intraarticular injection of Anakinra in osteoarthritis of the Knee: a multicenter, randomized, double-blind, placebo-controlled study. Arthrit Rheum-Arthr 61 (3), 344–352. doi: 10.1002/art.24096
Chevalier, X., Ravaud, P., Maheu, E., Baron, G., Rialland, A., Vergnaud, P., et al. (2015). Adalimumab in patients with hand osteoarthritis refractory to analgesics and NSAIDs: a randomised, multicentre, double- blind, placebo-controlled trial. Ann. Rheum Dis. 74 (9), 1697–1705. doi: 10.1136/annrheumdis-2014-205348
Compston, J. (2018). Glucocorticoid-induced osteoporosis: an update. Endocrine 61 (1), 7–16. doi: 10.1007/s12020-018-1588-2
Cooper, C., Bardin, T., Brandi, M. L., Cacoub, P., Caminis, J., Civitelli, R., et al. (2016). Balancing benefits and risks of glucocorticoids in rheumatic diseases and other inflammatory joint disorders: new insights from emerging data. An expert consensus paper from the European Society for Clinical and Economic Aspects of Osteoporosis and Osteoarthritis (ESCEO). Aging Clin. Exp. Res. 28 (1), 1–16. doi: 10.1007/s40520-015-0522-1
Cs-Szabo, G., Roughley, P. J., Plaas, A. H., Glant, T. T. (1995). Large and small proteoglycans of osteoarthritic and rheumatoid articular cartilage. Arthritis Rheumatism 38 (5), 660–668. doi: 10.1002/art.1780380514
Embree, M. C., Kilts, T. M., Ono, M., Inkson, C. A., Syed-Picard, F., Karsdal, M. A., et al. (2010). Biglycan and fibromodulin have essential roles in regulating chondrogenesis and extracellular matrix turnover in temporomandibular joint osteoarthritis. Am. J. Pathol. 176 (2), 812–826. doi: 10.2353/ajpath.2010.090450
Fava, R. A., Olsen, N. J., Postlethwaite, A. E., Broadley, K. N., Davidson, J. M., Nanney, L. B., et al. (1991). Transforming growth factor beta 1 (TGF-beta 1) induced neutrophil recruitment to synovial tissues: implications for TGF-beta-driven synovial inflammation and hyperplasia. J. Exp. Med. 173 (5), 1121–1132. doi: 10.1084/jem.173.51121
Gill, M. R., Oldberg, A., Reinholt, F. P. (2002). Fibromodulin-null murine knee joints display increased incidences of osteoarthritis and alterations in tissue biochemistry. Osteoarthritis Cartilage 10 (10), 751–757. doi: 10.1053/joca.20020527
Greenblatt, M. B., Ritter, S. Y., Wright, J., Tsang, K., Hu, D., Glimcher, L. H., et al. (2013). NFATc1 and NFATc2 repress spontaneous osteoarthritis. Proc. Natl. Acad. Sci. U. S. A. 110 (49), 19914–19919. doi: 10.1073/pnas.1320036110
Habib, G. S., Saliba, W., Nashashibi, M. (2010). Local effects of intra-articular corticosteroids. Clin. Rheumatol 29 (4), 347–356. doi: 10.1007/s10067-009-1357-y
Hedbom, E., Heinegard, D. (1989). Interaction of a 59-kDa connective tissue matrix protein with collagen I and collagen II. J. Biol. Chem. 264 (12), 6898–6905.
Hong, J. H., Lee, G. T., Lee, J. H., Kwon, S. J., Park, S. H., Kim, S. J., et al. (2009). Effect of bone morphogenetic protein-6 on macrophages. Immunology 128 (1 Suppl), e442–e450. doi: 10.1111/j.1365-2567.2008.02998.x
Jian, J., Zheng, Z., Zhang, K., Rackohn, T. M., Hsu, C., Levin, A., et al. (2013). Fibromodulin promoted in vitro and in vivo angiogenesis. Biochem. Biophys. Res. Commun. 436 (3), 530–535. doi: 10.1016/j.bbrc.2013.06.005
Kashiwagi, M., Enghild, J. J., Gendron, C., Hughes, C., Caterson, B., Itoh, Y., et al. (2004). Altered proteolytic activities of ADAMTS-4 expressed by C-terminal processing. J. Biol. Chem. 279 (11), 10109–10119. doi: 10.1074/jbc.M312123200
Kuyinu, E. L., Narayanan, G., Nair, L. S., Laurencin, C. T. (2016). Animal models of osteoarthritis: classification, update, and measurement of outcomes. J. Orthopaedic Surg. Res. 11. doi: 10.1186/s13018-016-0346-5
Lauder, R. M., Huckerby, T. N., Nieduszynski, I. A. (1996). The structure of the keratan sulphate chains attached to fibromodulin isolated from articular cartilage. Eur. J. Biochem. 242 (2), 402–409. doi: 10.1111/j.1432-1033.1996.0402r.x
Lauder, R. M., Huckerby, T. N., Nieduszynski, I. A., Plaas, A. H. (1998). Age-related changes in the structure of the keratan sulphate chains attached to fibromodulin isolated from articular cartilage. Biochem. J. 330 (Pt 2), 753–757. doi: 10.1042/bj3300753
Lee, E. J., Jan, A. T., Baig, M. H., Ahmad, K., Malik, A., Rabbani, G., et al. (2018a). Fibromodulin and regulation of the intricate balance between myoblast differentiation to myocytes or adipocyte-like cells. FASEB J. 32 (2), 768–781. doi: 10.1096/fj.201700665R
Lee, E. J., Nam, J. H., Choi, I. (2018b). Fibromodulin modulates myoblast differentiation by controlling calcium channel. Biochem. Biophys. Res. Commun. 503 (2), 580–585. doi: 10.1016/j.bbrc.2018.06.041
Li, C., Zheng, Z. (2018). What’s the future of osteoarthritis treatment. Acta Sci. Orthopaedics 1 (3), 01–02.
Li, C. S., Yang, P., Ting, K., Aghaloo, T., Lee, S., Zhang, Y., et al. (2016). Fibromodulin reprogrammed cells: a novel cell source for bone regeneration. Biomaterials 83, 194–206. doi: 10.1016/j.biomaterials.2016.01.013
Li, C., Zou, M., Zheng, Z. (2018). Current medication for osteoarthritis. Acta Sci. Orthopaedics 1 (3), 09–12.
Lin, Z., Fitzgerald, J. B., Xu, J., Willers, C., Wood, D., Grodzinsky, A. J., et al. (2008). Gene expression profiles of human chondrocytes during passaged monolayer cultivation. J. Orthopaedic Res. : Off. Publ. Orthopaedic Res. Soc. 26 (9), 1230–1237. doi: 10.1002/jor.20523
Melching, L. I., Roughley, P. J. (1999). Modulation of keratan sulfate synthesis on lumican by the action of cytokines on human articular chondrocytes. Matrix Biol. J. Int. Soc. Matrix Biol. 18 (4), 381–390. doi: 10.1016/S0945-053X(99)00033-5
Melrose, J., Fuller, E. S., Roughley, P. J., Smith, M. M., Kerr, B., Hughes, C. E., et al. (2008). Fragmentation of decorin, biglycan, lumican and keratocan is elevated in degenerate human meniscus, knee and hip articular cartilages compared with age-matched macroscopically normal and control tissues. Arthritis Res. Ther. 10 (4), R79. doi: 10.1186/ar2453
Miclea, R. L., Siebelt, M., Finos, L., Goeman, J. J., Lowik, C. W., Oostdijk, W., et al. (2011). Inhibition of Gsk3beta in cartilage induces osteoarthritic features through activation of the canonical Wnt signaling pathway. Osteoarthritis cartilage 19 (11), 1363–1372. doi: 10.1016/j.joca.2011.07.014
Mitani, Y., Honda, A., Jasin, H. E. (2001). Polymorphonuclear leukocyte adhesion to articular cartilage is inhibited by cartilage surface macromolecules. Rheumatol. Int. 20 (5), 180–185. doi: 10.1007/s002960000098
Monfort, J., Tardif, G., Reboul, P., Mineau, F., Roughley, P., Pelletier, J. P., et al. (2006). Degradation of small leucine-rich repeat proteoglycans by matrix metalloprotease-13: identification of a new biglycan cleavage site. Arthritis Res. Ther. 8 (1), R26. doi: 10.1186/ar1873
Murphy, J. M., Heinegard, R., McIntosh, A., Sterchi, D., Barry, F. P. (1999). Distribution of cartilage molecules in the developing mouse joint. Matrix Biol. J. Int. Soc. Matrix Biol. 18 (5), 487–497. doi: 10.1016/S0945-053X(99)00042-6
Noyori, K., Jasin, H. E. (1994). Inhibition of human fibroblast adhesion by cartilage surface proteoglycans. Arthritis Rheumatism 37 (11), 1656–1663. doi: 10.1002/art.1780371115
Plaas, A. H., Neame, P. J., Nivens, C. M., Reiss, L. (1990). Identification of the keratan sulfate attachment sites on bovine fibromodulin. J. Biol. Chem. 265 (33), 20634–20640.
Pourhanifeh, M. H., Mohammadi, R., Noruzi, S., Hosseini, S. A., Fanoudi, S., Mohamadi, Y., et al. (2019). The role of fibromodulin in cancer pathogenesis: implications for diagnosis and therapy. Cancer Cell Int. 19. doi: 10.1186/s12935-019-0870-6
Roughley, P. J., White, R. J., Cs-Szabo, G., Mort, J. S. (1996). Changes with age in the structure of fibromodulin in human articular cartilage. Osteoarthritis cartilage 4 (3), 153–161. doi: 10.1016/S1063-4584(96)80011-2
Saamanen, A. M., Salminen, H. J., Rantakokko, A. J., Heinegard, D., Vuorio, E. I. (2001). Murine fibromodulin: cDNA and genomic structure, and age-related expression and distribution in the knee joint. Biochem. J. 355 (Pt 3), 577–585. doi: 10.1042/bj3550577
Scott, D. L. (2010). “Arthritis in the Elderly,” in Brocklehurst’s Textbook of Geriatric Medicine and Gerontology. Eds. Fillit, H. M., Rockwood, K., Woodhouse, K. (Phiadelphia: Saunders, Elservier), 566–576. doi: 10.1016/B978-1-4160-6231-8.10070-4
Shirakura, M., Kram, V., Robinson, J., Sikka, S., Kilts, T. M., Wadhwa, S., et al. (2017). Extracellular matrix mediates BMP-2 in a model of temporomandibular joint osteoarthritis. Cells Tissues Organs 204 (2), 84–92. doi: 10.1159/000464102
Shu, C. C., Flannery, C. R., Little, C. B., Melrose, J. (2019). Catabolism of Fibromodulin in developmental rudiment and pathologic articular cartilage demonstrates novel roles for MMP-13 and ADAMTS-4 in C-terminal processing of SLRPs. Int. J. Mol. Sci. 20 (3). doi: 10.3390/ijms20030579
Sztrolovics, R., White, R. J., Poole, A. R., Mort, J. S., Roughley, P. J. (1999). Resistance of small leucine-rich repeat proteoglycans to proteolytic degradation during interleukin-1-stimulated cartilage catabolism. Biochem. J. 339 (Pt 3), 571–577. doi: 10.1042/bj3390571
Taruc-Uy, R. L., Lynch, S. A. (2013). Diagnosis and treatment of osteoarthritis. Primary Care 40 (4), 821–836. doi: 10.1016/j.pop.2013.08.003
Verbruggen, G., Wittoek, R., Cruyssen, B. V., Elewaut, D. (2012). Tumour necrosis factor blockade for the treatment of erosive osteoarthritis of the interphalangeal finger joints: a double blind, randomised trial on structure modification. Ann. Rheum Dis. 71 (6), 891–898. doi: 10.1136/ard.2011.149849
Wadhwa, S., Embree, M., Ameye, L., Young, M. F. (2005a). Mice deficient in biglycan and fibromodulin as a model for temporomandibular joint osteoarthritis. Cells Tissues Organs 181 (3-4), 136–143. doi: 10.1159/000091375
Wadhwa, S., Embree, M. C., Kilts, T., Young, M. F., Ameye, L. G. (2005b). Accelerated osteoarthritis in the temporomandibular joint of biglycan/fibromodulin double-deficient mice. Osteoarthritis Cartilage 13 (9), 817–827. doi: 10.1016/j.joca.2005.04.016
Yaykasli, K. O., Oohashi, T., Hirohata, S., Hatipoglu, O. F., Inagawa, K., Demircan, K., et al. (2009). ADAMTS9 activation by interleukin 1 beta via NFATc1 in OUMS-27 chondrosarcoma cells and in human chondrocytes. Mol. Cell. Biochem. 323 (1-2), 69–79. doi: 10.1007/s11010-008-9965-4
Zhang, W., Cheng, P., Hu, W., Yin, W., Guo, F., Chen, A., et al. (2018). Downregulated microRNA-340-5p promotes proliferation and inhibits apoptosis of chondrocytes in osteoarthritis mice through inhibiting the extracellular signal-regulated kinase signaling pathway by negatively targeting the FMOD gene. J. Cell. Physiol. 234 (1), 927–939. doi: 10.1002/jcp.26921
Zheng, Z., Jian, J., Zhang, X., Zara, J. N., Yin, W., Chiang, M., et al. (2012). Reprogramming of human fibroblasts into multipotent cells with a single ECM proteoglycan, fibromodulin. Biomaterials 33 (24), 5821–5831. doi: 10.1016/j.biomaterials.2012.04.049
Zheng, Z., Jian, J., Velasco, O., Hsu, C. Y., Zhang, K., Levin, A., et al. (2014). Fibromodulin enhances angiogenesis during cutaneous wound healing. Plast. Reconstructive Surg. Global Open 2 (12), e275. doi: 10.1097/GOX.0000000000000243
Zheng, Z., James, A. W., Li, C., Jiang, W., Wang, J. Z., Chang, G. X., et al. (2017). Fibromodulin reduces scar formation in adult cutaneous wounds by eliciting a fetal-like phenotype. Signal Transduction Targeted Ther. 2. doi: 10.1038/sigtrans.2017.50
Keywords: fibromodulin, arthritis, osteoarthritis, collagen, inflammation, keratan sulfate
Citation: Li C, Ha P, Jiang W, Haveles CS, Zheng Z and Zou M (2019) Fibromodulin – A New Target of Osteoarthritis Management? Front. Pharmacol. 10:1475. doi: 10.3389/fphar.2019.01475
Received: 16 August 2019; Accepted: 13 November 2019;
Published: 10 December 2019.
Edited by:
Feng Chen, Peking University, ChinaReviewed by:
Jingtan Su, University of Southern California, United StatesCopyright © 2019 Li, Ha, Jiang, Haveles, Zheng and Zou. This is an open-access article distributed under the terms of the Creative Commons Attribution License (CC BY). The use, distribution or reproduction in other forums is permitted, provided the original author(s) and the copyright owner(s) are credited and that the original publication in this journal is cited, in accordance with accepted academic practice. No use, distribution or reproduction is permitted which does not comply with these terms.
*Correspondence: Zhong Zheng; bGVveno5NUBnbWFpbC5jb20=; Min Zou, em91bWluQG1haWwueGp0dS5lZHUuY24=
Disclaimer: All claims expressed in this article are solely those of the authors and do not necessarily represent those of their affiliated organizations, or those of the publisher, the editors and the reviewers. Any product that may be evaluated in this article or claim that may be made by its manufacturer is not guaranteed or endorsed by the publisher.
Research integrity at Frontiers
Learn more about the work of our research integrity team to safeguard the quality of each article we publish.