- 1Department of Cardiology, Renmin Hospital of Wuhan University, Wuhan, China
- 2Cardiovascular Research Institute, Wuhan University, Wuhan, China
- 3Hubei Key Laboratory of Cardiology, Wuhan, China
The transient receptor potential ankyrin 1 (TRPA1) channel is a calcium-permeable nonselective cation channel in the plasma membrane that belongs to the transient receptor potential (TRP) channel superfamily. Recent studies have suggested that the TRPA1 channel plays an essential role in the development and progression of several cardiovascular conditions, such as atherosclerosis, heart failure, myocardial ischemia–reperfusion injury, myocardial fibrosis, arrhythmia, vasodilation, and hypertension. Activation of the TRPA1 channel has a protective effect against the development of atherosclerosis. Furthermore, TRPA1 channel activation elicits peripheral vasodilation and induces a biphasic blood pressure response. However, loss of channel expression or blockade of its activation suppressed heart failure, myocardial ischemia–reperfusion injury, myocardial fibrosis, and arrhythmia. In this paper, we review recent research progress on the TRPA1 channel and discuss its potential role in the cardiovascular system.
Introduction
The mammalian transient receptor potential (TRP) superfamily of cation channels has six subfamilies, including the vanilloid (TRPV), canonical (TRPC), melastatin (TRPM), ankyrin (TRPA), polycystin (TRPP), and mucolipin (TRPML) subfamilies, which are separated based on sequence homology (Venkatachalam and Montell, 2007). Also known as ATKTM1, the TRPA1 channel represents the sole member of the TRPA subfamily and contains six transmembrane domain (S1–S6) polypeptide subunits. Its name is derived from the unusually high number of ankyrin repeats within the N-terminus and the pore-forming selectivity filter positions between the S5 and S6 transmembrane segments (Story et al., 2003; Nilius and Owsianik, 2011). Since it is a nonselective cation channel, Ca2+ and Na+ can cross the TRPA1 channel, which results in membrane depolarization and action potential discharge (Zygmunt and Hogestatt, 2014). The TRPA1 channel has been demonstrated to play a pivotal role in mediating a series of pathophysiological reactions, including pain, inflammation, itching, and tissue injury and repair (Bautista et al., 2006; Nilius and Szallasi, 2014; Wang et al., 2018a).
Initially, the TRPA1 channel was thought to be predominately expressed in the sensory neurons of the nodose ganglia, dorsal root ganglia, and trigeminal ganglia (Story et al., 2003; Nilius et al., 2012), as well as in hair cells (Corey et al., 2004; Nagata et al., 2005). In recent years, various researchers have demonstrated that the TRPA1 channel is widely expressed in nonneuronal cells, such as lung fibroblasts (Mukhopadhyay et al., 2011; Nassini et al., 2012), alveolar epithelial cells (Nassini et al., 2012), cardiomyocytes (Andrei et al., 2016), cardiac fibroblasts (Oguri et al., 2014), arterial endothelial cells (ECs) (Qian et al., 2013), pancreatic beta cells (Cao et al., 2012), enterochromaffin cells (Nozawa et al., 2009), odontoblasts (Egbuniwe et al., 2014), and T cells (Bertin et al., 2016).
The TRPA1 channel responds to a wide range of agonists, including pungent natural compounds, such as allyl isothiocyanate (AITC), cinnamaldehyde, and allicin (Bandell et al., 2004; Jordt et al., 2004; Macpherson et al., 2005); ambient toxins, such as acrolein and nicotine (Andre et al., 2008; Talavera et al., 2009); anesthetic agents, such as propofol and lidocaine (Leffler et al., 2011; Woll et al., 2017); chemical compounds, such as ASP-7663 and optovin (Kokel et al., 2013; Kojima et al., 2014); and a range of endogenous agonists, such as oxidized lipids, nitric oxide (NO), and hydrogen sulfide (H2S) (Andersson et al., 2008; Eberhardt et al., 2014). Both intracellular and extracellular Ca2+ can directly activate the channel, whose activation can be further strengthened by agonists (Zurborg et al., 2007; Wang et al., 2008). However, the effect of temperature on the activation of the TRPA1 channel is controversial. Some researchers have demonstrated that the TRPA1 channel could be activated by noxious cold stimuli (temperature of <17°C) (Story et al., 2003; Del et al., 2010), whereas other studies showed that cold does not activate the TRPA1 channel or that the cold-induced increase in intracellular Ca2+ indirectly activates the TRPA1 channel (Zurborg et al., 2007). Recent studies have indicated that the TRPA1 channel is activated by several substances that are produced during oxidative stress, such as hydrogen peroxide (H2O2), 4-hydroxynonenal (4-HNE), 4-oxononenal (4-ONE), 4-hydroxyhexenal (4-HHE), and 15-deoxy-delta(12,14)-prostaglandin J(2) ([15d-PGJ(2)]) (Trevisani et al., 2007; Andersson et al., 2008; Taylor-Clark et al., 2008). In addition, frequently used TRPA1 agonists are listed in Table 1.
Many chemicals, such as ruthenium red, amiloride, camphor, and menthol, have been shown to block this channel, but they lack specificity. Moreover, small-molecule compounds, such as HC-030031 (McNamara et al., 2007) and AP-18 (Petrus et al., 2007; Defalco et al., 2010), are primary inhibitors that specifically bind to the channel and have been widely used to study TRPA1 channel-mediated pharmacology in vitro and in vivo. Recently, some researchers have reported additional TRPA1 antagonists with highly selectivity and pharmaceutical properties, including A-967079 (Chen et al., 2011), TCS-5861528 (Wei et al., 2009), compound 10 (Copeland et al., 2014), and compound 31 (Rooney et al., 2014). These new-generation TRPA1 channel antagonists will be valuable for exploring the function and therapeutic utility of TRPA1. Frequently used TRPA1 inhibitors are listed in Table 2.
Previous studies have demonstrated that the TRPA1 channel is also widely expressed in the cardiovascular system and is involved in regulating intracellular Ca2+ concentrations (Oguri et al., 2014; Andrei et al., 2016). In this review, we summarize the potential involvement of the TRPA1 channel in modulating pathophysiologic conditions, including atherosclerosis, heart failure, myocardial ischemia–reperfusion injury (IRI), myocardial fibrosis, arrhythmia, vasodilation, and hypertension (Table 3).
Role of the Trpa1 Channel in the Cardiovascular System
Atherosclerosis
Atherosclerosis and its complications remain the leading causes of morbidity and mortality in developed countries (Momiyama et al., 2014; Solanki et al., 2018). Atherosclerosis, characterized by the hardening of the arterial wall and the narrowing of the arterial lumen, is considered a chronic inflammatory disease that results from lipid metabolism dysfunction, smooth muscle cell proliferation, and cholesterol-laden macrophage accumulation (Khera et al., 2011; Moore et al., 2013; Ye et al., 2017b). Evidence supports the idea that macrophages are the dominant immune cells and play critical roles in the development of atherosclerosis. During the early stages of atherosclerosis, the accumulation of lipid-laden macrophages and oxidized low-density lipoprotein (oxLDL) leads to lipid droplet generation in the subendothelial space. Macrophage accumulation and foam cell formation exacerbate the development of unstable plaques and plaque rupture (Libby, 2002; Khan et al., 2015).
As we know, cholesterol homeostasis in macrophage foam cells is regulated by the complex mechanisms underlying oxLDL internalization and cholesterol efflux. In macrophage foam cells, oxLDL internalization is mediated by scavenger receptor transporters, and several macrophage transporters, including ATP-binding cassette subfamily A member 1 (ABCA1) and ABCG1, are responsible for cholesterol efflux. In a mouse high-fat diet-induced atherosclerosis model, TRPA1 was localized mainly in macrophages; TRPA1 channel activation with AITC suppressed the progression of atherosclerosis in apolipoprotein E (apoE)−/− mice, while the protective effect was lost in apoE−/−TRPA1−/− mice. Moreover, inhibition of TRPA1 activity exacerbated atherosclerosis and abated cholesterol efflux by suppressing oxLDL-induced cholesterol efflux but did not alter oxLDL internalization (Zhao et al., 2016). This implied that TRPA1 activation suppressed excessive lipid accumulation by increasing ABC transporter-mediated cholesterol efflux. Inflammatory conditions are also a key event an implicated in the progression of atherosclerosis. The author also reported that AITC administration prevented TNF-α-induced macrophage inflammation. Thus, activation of the macrophage TRPA1 channel suppressed atherosclerosis by inhibiting cholesterol efflux and the inflammatory response. In all, these data indicate that pharmacological activation of the TRPA1 channel may have therapeutic value to prevent or treat atherosclerosis.
The existing research is very creative and interesting, but many crucial points still need consideration. Macrophage-mediated cholesterol metabolism and proinflammatory cytokine secretion are central steps in the initiation and progression of atherosclerosis. While there is considerable knowledge on the functions of Ca2+ influx from the endothelium and macrophages in atherosclerosis, less is known about the molecular mechanisms by which the TRPA1 channel regulates the formation of macrophage-derived foam cells that directly or indirectly depend on Ca2+ influx. Moreover, the macrophage number, macrophage phenotype, macrophage apoptosis, and lesion autophagy are also important determinants of atherosclerosis progression. Whether the TRPA1 channel modulates both the recruitment and polarization of macrophages toward a pro- or anti-inflammatory phenotype is unclear. Further studies require the generation of macrophage-specific TRPA1 knockout mice to examine the contribution of the TRPA1 channel to atherogenesis.
Heart Failure
Heart failure is a complex and multisystem clinical syndrome that results from impaired ventricular systolic and/or diastolic function (Katz and Rolett, 2016). Heart failure is a result of exposure to chronic cardiac stress or injury, including pressure overload, myocardial infarction or ischemia, myocarditis, and inherited diseases (Bacmeister et al., 2019; Ye et al., 2019). Ca2+ is a critical second messenger in cardiac function. It participates in not only in the excitation–contraction coupling and relaxation of the heart but also in a key signal transduction pathway responsible for various cardiovascular diseases (Goldhaber and Philipson, 2013). Altered Ca2+ homeostasis contributes notably to the pathophysiology of heart failure, and many models of heart failure exhibit deficient Ca2+ handling in cardiomyocytes (Bers, 2006; Roe et al., 2015). For example, the Ca2+-dependent Ca2+/calmodulin-dependent protein kinase II (CaMKII) and calcineurin pathways are centrally involved in mediating pathological hypertrophy and heart failure (Molkentin et al., 1998; Anderson et al., 2011). While the precise mechanism remains unclear, accumulating evidence suggests that targeting Ca2+ homeostasis in failing cardiomyocytes presents a promising new therapeutic approach for improving cardiac function.
Our previously study showed that TRPA1 expression is increased in failing human and mouse hearts (Wang et al., 2018b). Inhibition of the TRPA1 channel significantly ameliorated cardiac hypertrophy and heart failure by decreasing the increases in heart weight index and ventricular volume and improving cardiac function in transverse aortic constriction-induced pressure overload in mice. In addition, TRPA1 inhibition significantly reduced critical factors involved in heart failure, including calcineurin and CaMKII phosphorylation in mice induced by pressure overload. Another study demonstrated that that TRPA1 activation induced a dose-dependent increase in contractile function and peak [Ca2+]i in cardiomyocytes but not in cardiomyocytes obtained from TRPA1−/− mice (Andrei et al., 2017). In addition, the CaMKII inhibitor KN-93 reversed the AITC-induced increase in [Ca2+]i and contractile function. Based on these studies, it may be suggested that TRPA1 participates in the regulation of heart failure via a Ca2+-dependent mechanism.
In failing hearts, Ca2+ homeostasis is markedly altered, resulting in impaired systolic and diastolic function (Marks, 2013; Roe et al., 2015). Previous research revealed that the TRPA1 channel is present in cardiomyocytes and that TRPA1-mediated Ca2+ release contributed to nearly 40% of the overall [Ca2+]i increase in the physiological state (Andrei et al., 2016; Shang et al., 2016). The links between TRPA1 and heart failure are briefly summarized in Figure 1. Pressure overload activates the TRPA1 channel and increases Ca2+ influx. The activation of Ca2+-dependent pathways, including CaMKII and calcineurin, leads to hypertrophic gene expression and mediates heart failure.
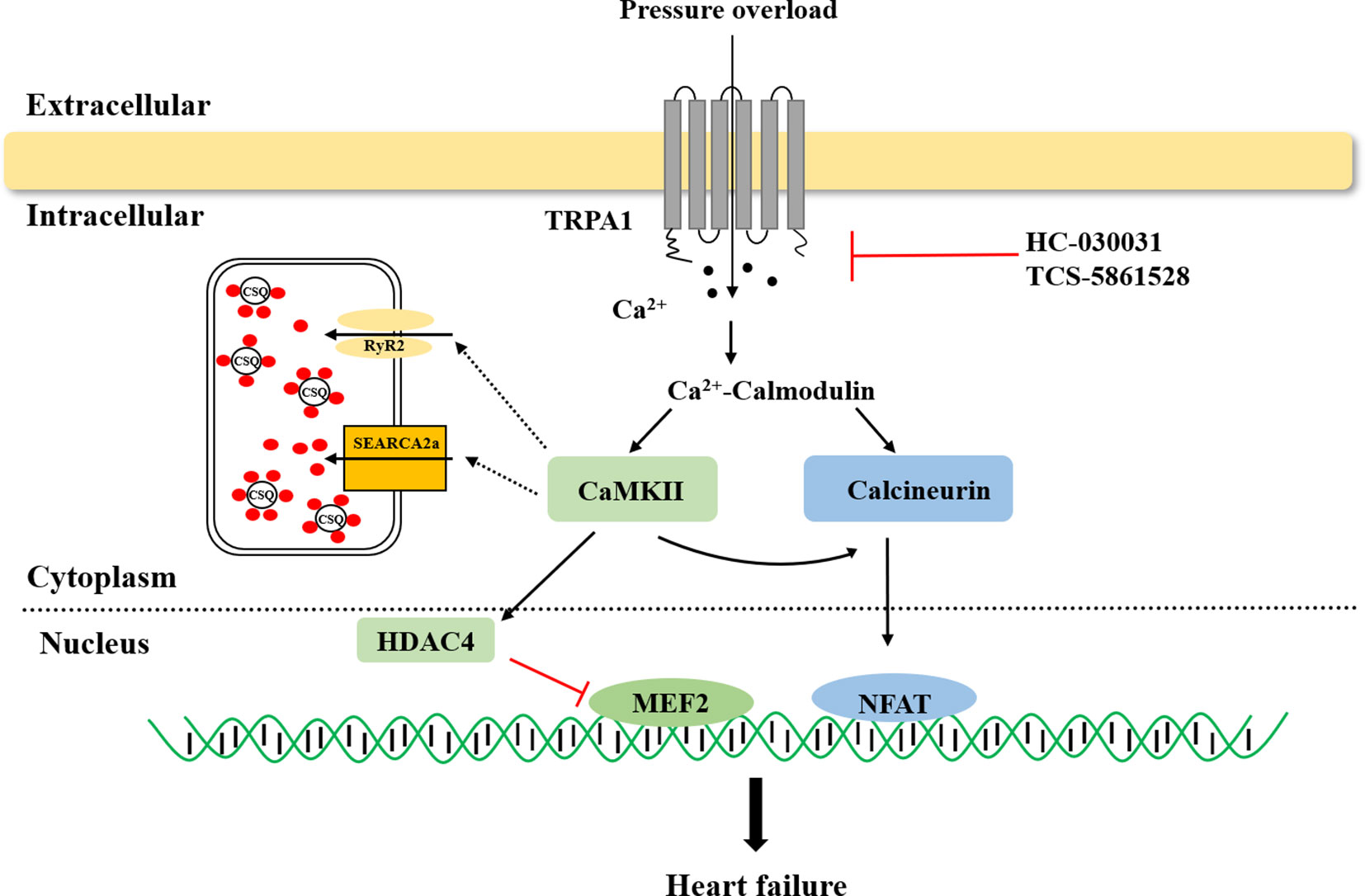
Figure 1 The role of transient receptor potential ankyrin 1 (TRPA1) in heart failure. TRPA1 channel inhibition decreased Ca2+ influx and inhibited Ca2+-dependent pathway activation, ultimately ameliorating pressure overload induced-heart failure. CaMKII, Ca2+/calmodulin-dependent protein kinase II; HDAC4, histone deacetylase 4; MEF2, myocyte enhancer factor 2; NFAT, nuclear factor of activated T cells.
Myocardial IRI
Myocardial IRI is a pathological condition that occurs after a critical ischemic period followed by blood supply restoration and reoxygenation that correlates with a deterioration of myocardial function and a marked inflammatory reaction (Ferdinandy et al., 2007; Eltzschig and Eckle, 2011). A variety of pathological processes and mediators, including intracellular Ca2+ overload and excess reactive oxygen species (ROS), are proposed to be crucial in myocardial IRI. During ischemia, ion pumps cannot function, resulting in a rise in Ca2+, which leads to intracellular Ca2+ overload, particularly during reperfusion when oxygen is reintroduced (Murphy and Steenbergen, 2008; Garcia-Dorado et al., 2012). ROS are produced physiologically by the mitochondrial electron transport chain during respiration, and increased ROS can result in mitochondrial matrix swelling and cell death (Murphy and Steenbergen, 2008; Zhou et al., 2015). Studies have shown substantial interest in developing therapies to prevent myocardial IRI. In particular, site-targeted treatments, such as inhibiting Ca2+ overload and reducing ROS accumulation, may improve the protective effect on the stressed myocardium (Raedschelders et al., 2012; Jennings, 2013).
The TRPA1 channel is a calcium-permeable nonselective cation channel in the plasma membrane; however, it also functions as a sensor that is activated by ROS and is modulated when intracellular changes in oxygen levels occur, and both factors are important for IRI (Viana, 2016; Pires and Earley, 2017). However, the role and mechanism of TRPA1 in myocardial IRI are conflicting and are still controversial. In an in vivo rat model of myocardial IRI (30 min of ischemia followed by 2 h of reperfusion), the administration of the TRPA1 agonist reduced the myocardial infarct size before ischemia and reperfusion, and TRPA1 channel inhibition also blocked the infarct size-sparing effects of morphine. In isolated cardiomyocytes, the activation of the TRPA1 channel reduced cardiomyocyte cell death and the release of lactate dehydrogenase when activated during reoxygenation (Lu et al., 2016). In contrast, global knockout of TRPA1 results in less myocardial injury in a mouse model of IRI (30 min of ischemia followed by 24 h of reperfusion) (Conklin et al., 2019). In addition, acrolein, an IRI-associated toxin, induced Ca2+ overload and hypercontraction in isolated cardiomyocytes, which were significantly ameliorated by TRPA1 inhibitor. These studies are contradictory, may be because of the exerting differential effects, TRPA1 in cardiac myocytes, vascular cells, and sensory neurons, which eliminates its function leads to variable effects on IRI. Therefore, a genetically modified mouse model with cell-specific deletion (or targeted inhibition) of TRPA1 in cardiomyocytes will be required to assess the role of myocardial TRPA1 in IRI.
As we known, the sensitivity of the TRPA1 channel to ROS was the greatest among the TRP channels (Yamamoto and Shimizu, 2016). Moreover, the TRPA1 channel also plays a pivotal role in the maintenance of O2 homeostasis (Takahashi et al., 2011). This study found that O2 could activate the TRPA1 channel through reversible covalent or oxidative modification of cysteine residues in hyperoxia directly. In addition, prolyl hydroxylases (PHDs) could inhibit TRPA1 channel activity under the condition of normoxia, whereas hypoxia could activate TRPA1 by diminishing the activity of PHDs. Accumulating research has shown that Ca2+ can enhance ROS output and generation, which correlates well with metabolic rate. Thus, it will be very interesting to determine the mechanism by which the TRPA1 channel mediates Ca2+ homeostasis and redox signaling in myocardial IRI (Figure 2).
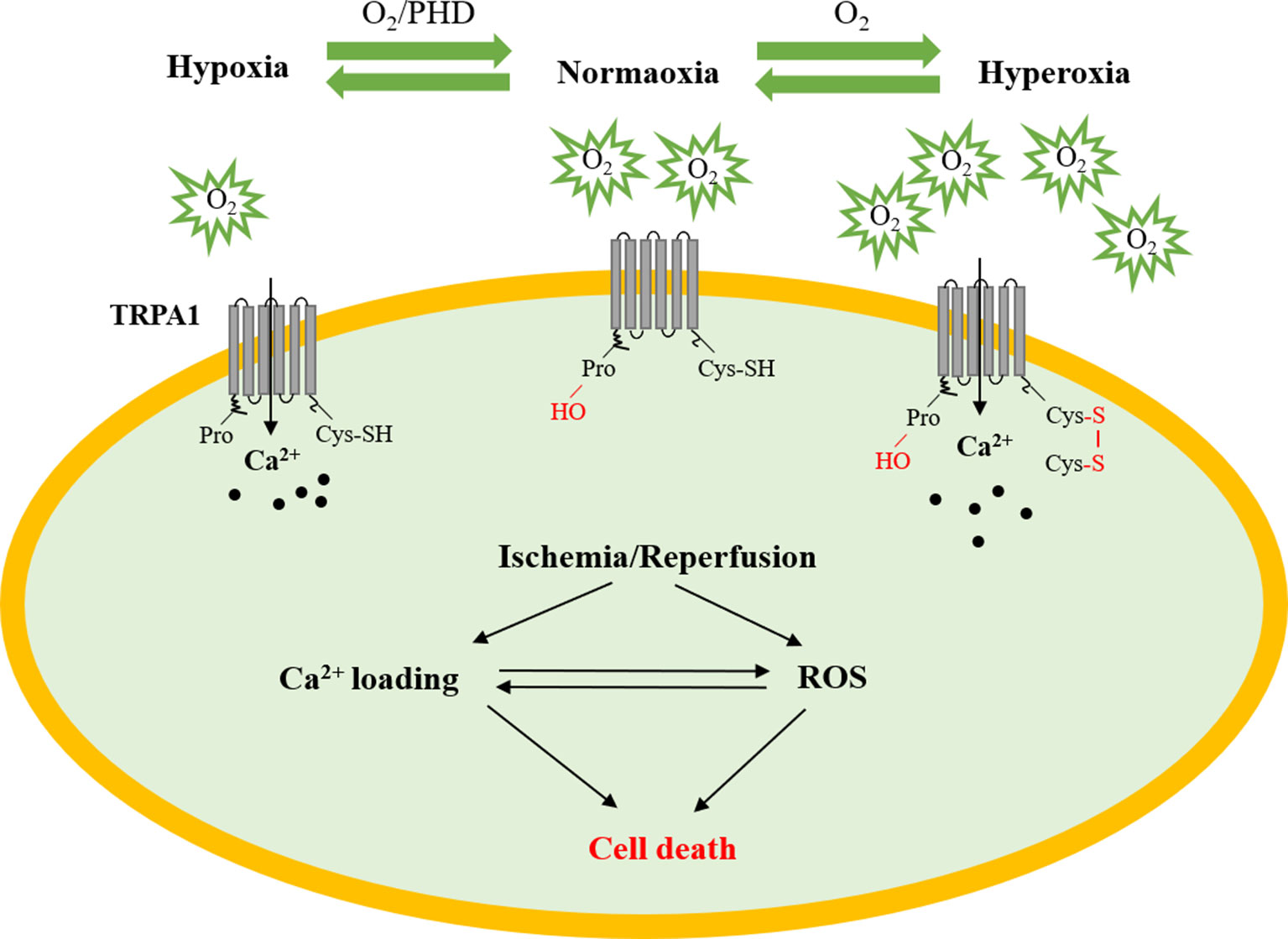
Figure 2 The role of TRPA1 in myocardial ischemia–reperfusion injury. Under normoxic conditions, PHDs inhibit TRPA1 channel activity. Under hyperoxic conditions, O2 directly activates the TRPA1 channel through reversible covalent or oxidative modification of cysteine residues. Under hypoxic conditions, the TRPA1 channel was activated by O2-dependent inhibition relief by PHD-mediated hydroxylation of a proline residue. The TRPA1 channel participates in the development of myocardial ischemia–reperfusion injury by regulating Ca2+ overload and ROS. PHD, prolyl hydroxylases; Cys, cysteine residue; ROS, reactive oxygen species.
Myocardial Fibrosis
Myocardial fibrosis is associated with cardiac fibroblast overproliferation and excessive extracellular matrix (ECM) protein accumulation in the myocardial interstitium (Schirone et al., 2017; Zhang et al., 2018). Cardiac fibroblasts are activated in response to a variety of pathological stimuli, such as myocardial injury, pressure overload, and failed repair. Cardiac fibroblasts are the main producers of ECM and play an important role in cell signaling and fibrotic responses (Yue et al., 2013; Moore-Morris et al., 2015). Accumulating evidence has demonstrated that Ca2+-dependent signaling is essential for the proliferation and differentiation of cardiac fibroblasts and ECM production (He et al., 2011; Yue et al., 2013). However, Ca2+-permeable channels in coordination with Ca2+-dependent signaling in cardiac fibroblasts are not fully understood. Thus, understanding the molecules responsible for Ca2+-dependent signaling in cardiac fibroblasts will provide novel targets for antifibrotic strategies.
Methylglyoxal (MG), a highly reactive dicarbonyl compound, is a metabolic intermediate of glycolysis that can activate TRPA1. MG can confer deleterious cardiovascular effects, which are associated with the activation of fibrosis (Desai and Wu, 2007; Subramanian and Nagarajan, 2017). In an in vitro study, TRPA1 was expressed in human adult ventricular cardiac fibroblasts, and inhibiting TRPA1 channel activity reduced MG-induced Ca2+ influx. Moreover, inhibition of TRPA1 suppressed MG-induced fibroblast proliferation and increased α-smooth muscle actin expression. In this study, the TRPA1-mediated Ca2+-dependent signaling pathway was suggested to be required for MG-induced cell cycle progression and differentiation in human cardiac fibroblasts (Oguri et al., 2014). Another study also demonstrated that inhibition of the TRPA1 channel attenuated fibrosis and inflammation by attenuating TGF-β1 signaling cascades in ocular fibroblasts (Okada et al., 2014). Taken together, these studies indicated that the TRPA1 channel may serve as a potential novel therapeutic target for fibrotic responses.
In recent years, the importance of TRP channels in regulating Ca2+ signaling and cardiac fibrogenesis has been recognized (Thodeti et al., 2013). Inhibition of the TRPM7 channel reduced the proliferation and differentiation of cardiac fibroblasts and ECM production (Du et al., 2010). In addition, the TRPC3 channel functions as a critical mediator in myocardial fibrosis in coordination with Ca2+ signaling and ROS production (Numaga-Tomita et al., 2017). Compared with most other TRP channels, the TRPA1 channel has high Ca2+ permeability (Zygmunt and Hogestatt, 2014). As the sole member of the TRPA subfamily, the TRPA1 channel could be important in causing cardiac fibrosis. Thus, further studies are required to clarify the molecular mechanisms underlying the regulation of myocardial fibrosis by the TRPA1 channel.
Arrhythmia
Common air pollution is composed of particulate matter and gaseous pollutants, such as ozone, nitrogen oxides, sulfur dioxide, aldehydes, and acrolein. Epidemiological studies have shown that the deleterious effects of noxious irritants and pollutant inhalation occur upon both short-term and long-term exposure. Air pollution can lead to millions of premature deaths worldwide, of which 60%–80% are cardiovascular diseases. In fact, exposure to air pollutants has been well established as a factor that increases the risk for cardiovascular events, particularly increasing the susceptibility to arrhythmias by altering autonomic nervous system (ANS) balance (Brook et al., 2004; Langrish et al., 2014).
Acrolein is a volatile, unsaturated aldehyde and a toxic combustion product present in tobacco smoke and fires (Stevens and Maier, 2008). Recent research reported that exposure to acrolein increased heart rate (HR) variability and the incidence of arrhythmias in WT mice, while these consequences were eliminated in TRPA1−/− mice (Kurhanewicz et al., 2016; Kurhanewicz et al., 2018; Thompson et al., 2019). Moreover, exposure to diesel exhaust (DE) and aconitine increased sympathetic activation in spontaneously hypertensive rats. Pretreatment of low DE-exposed rats with a TRPA1 inhibitor or sympathetic blockade reduced susceptibility to ventricular arrhythmias (Hazari et al., 2011). As we know, HR variability is considered to be a marker of ANS imbalance and a risk factor for cardiovascular events. These findings likely indicated that the TRPA1 channel may contribute to the proarrhythmic response by causing sympathetic activation and ANS imbalance.
Other studies showed that the activation of the TRPA1 channel evoked reflex-mediated increases in parasympathetic activity. The application of AITC increased HR and renal sympathetic nerve activity, which were blunted in rats with chronic heart failure (Adam et al., 2019). Exposure of conscious rats to AITC caused a significant increase in the incidence of arrhythmic events, which included bradycardia, atrioventricular blockade, and even prolonged PR intervals. Furthermore, such responses to AITC exposure were inhibited by the cholinergic antagonist atropine (Hooper et al., 2016).
The ANS is composed of the sympathetic and parasympathetic systems located throughout the body, including in the heart, lung, and vasculature system. These sensory nerves are sensitive to multiple stimuli and responsible for maintaining homeostasis by having either excitatory or inhibitory effects. The responses to air pollutants or irritant inhalation are associated with the activation of the sympathetic and parasympathetic systems, which do not function independently and are highly integrated (Middlekauff et al., 2014; Perez et al., 2015). Previous studies have shown that TRPA1 channels are expressed in primary sensory neurons and that TRPA1 activation results in both afferent and efferent signaling (Zygmunt and Hogestatt, 2014). Different air pollutants and irritants have different effects when TRPA1 is activated, related to the activation of the sympathetic or parasympathetic systems. Thus, considering the complexity of the composition of air pollution and irritants, further studies are needed to identify the roles of the TRPA1 channel in modulating autonomic control of the heart.
Vasodilation
The vascular system plays essential roles in the transport of gases (such as oxygen and carbon dioxide), nutrients (such as amino acids and electrolytes), and circulating cells in the organism. The vascular system is an exquisitely sculpted vascular network throughout the body and contains multiple components. Among them, ECs and smooth muscle cells act as vital mediators of vascular homeostasis maintenance. In addition, perivascular sensory neurons contribute to the regulation of vascular tone through the release of neuropeptides, such as calcitonin gene-related peptide (CGRP) and substance P (Aubdool et al., 2016).
Recent studies demonstrated the involvement of the TRPA1 channel in the regulation of vasodilation (Earley, 2012; Eberhardt et al., 2014). Intraplantar injection of 4-ONE triggered a significant vasodilation response, which was absent in TRPA1−/− and CGRP−/− mice (Graepel et al., 2011). Moreover, AITC and environmental irritants, such as formaldehyde and acrolein, stimulate CGRP release and increase cerebral blood flow, effects that were blocked by TRPA1 and a CGRP receptor antagonist (Kunkler et al., 2011). Topical application of cinnamaldehyde to mouse ears resulted in a marked increase in the blood flow in the skin but not in HC-030031- or nitric oxide synthase (NOS) antagonist-pretreated mice (Aubdool et al., 2016). Based on the findings of these studies, it may be proposed that the activation of the TRPA1 channel could mediate neurogenic vasodilation in the peripheral vasculature and could be mediated by the sensory neuropeptide CGRP and NOS-derived NO.
Other studies also demonstrated that the TRPA1 channel causes vasodilation through an endothelium-dependent mechanism involving Ca2+ influx in arterial ECs. Earley and colleagues showed that TRPA1 channel was present in arterial ECs, and the AITC-induced cerebral artery dilation was abolished by TRPA1 inhibition and endothelium disruption. In addition, TRPA1 activation-induced dilation was suppressed by treatment with the small and intermediate conductance Ca2+-activated K+ channel (KCa) and an inwardly rectifying K+ channel (KIR) blocker (Earley et al., 2009). Moreover, activation of the TRPA1 channel enhanced endothelial Ca2+ dynamics primarily through the recruitment of endothelial Ca2+ and evoked graded cerebral artery vasodilation (Qian et al., 2013).
In summary, the above-mentioned results demonstrated that activation of the TRPA1 channel can elicit peripheral vasodilation and that the main mechanisms underlying this process involve nerve-induced vasodilation and endothelium-dependent vasodilation (Figure 3). On the one hand, the increased Ca2+ influx via TRPA1 activation resulted in hyperpolarization of the endothelial cell plasma membrane. The change in membrane potential occurs to hyperpolarize the vascular smooth muscle cell plasma membrane, resulting in myocyte relaxation. On the other hand, activation of TRPA1 in sensory neurons induces an increase in [Ca2+]i, leading to the release of the neuropeptide CGRP and NOS-derived NO, thus mediating vasodilation.
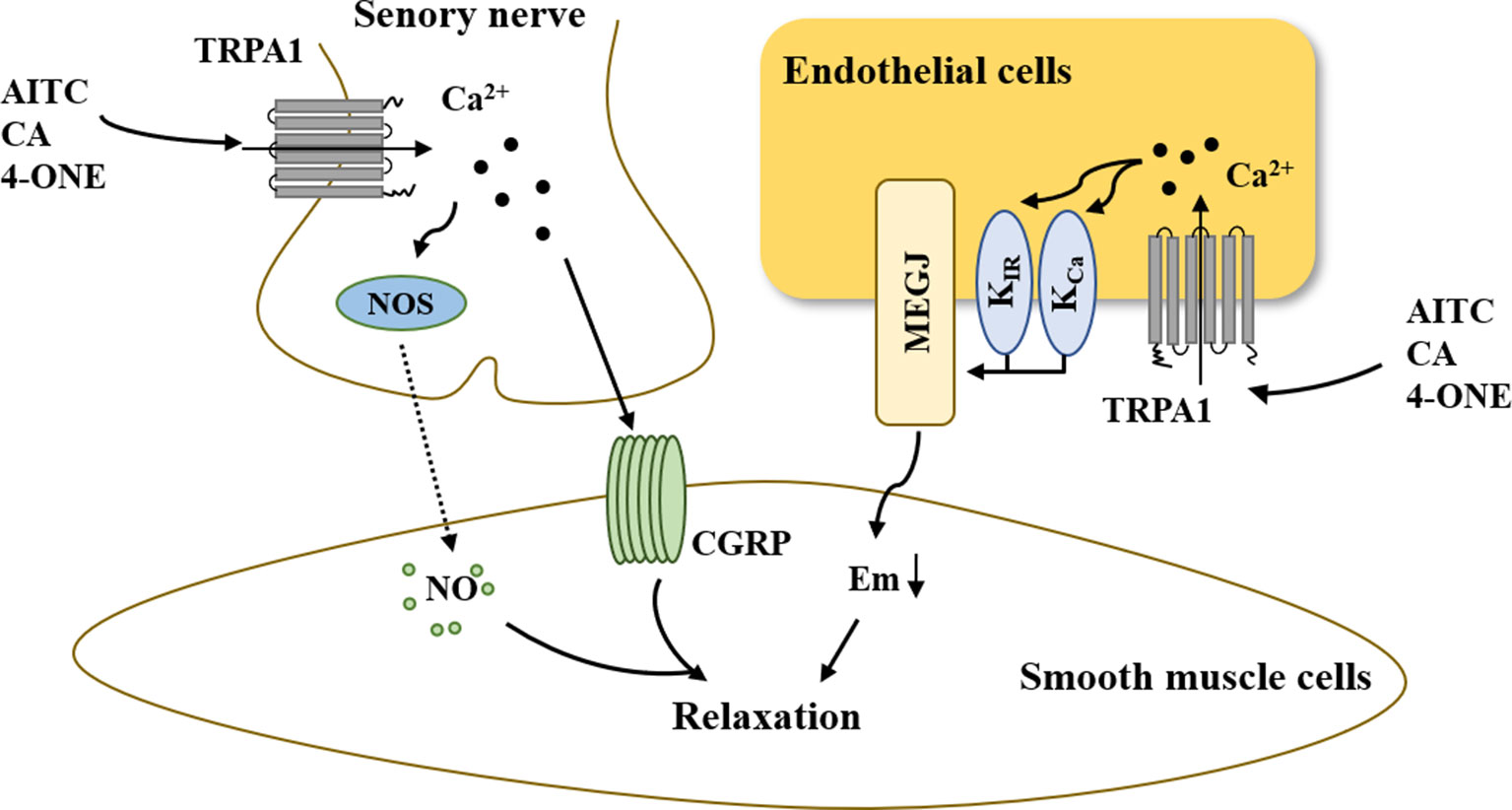
Figure 3 The role of TRPA1 in vasodilation. On the one hand, increased Ca2+ influx via TRPA1 activation results in hyperpolarization of the endothelial cell plasma membrane. The change in membrane potential is occurs to hyperpolarize the vascular smooth muscle cell plasma membrane, resulting in myocyte relaxation. On the other hand, the activation of TRPA1 in sensory neurons induces an increase in [Ca2+]i, leading to the release of the neuropeptide CGRP and NOS-derived NO, thus mediating vasodilation. AITC, allyl isothiocyanate; CA, cinnamaldehyde; 4-ONE, 4-oxo-2-nonenal; CGRP, calcitonin gene-related peptide; NOS, nitric oxide synthase; NO, nitric oxide; KCa, Ca2+-activated K+ channel; KIR, inwardly rectifying K+ channel; MEGJs, myoendothelial gap junctions.
Hypertension
Hypertension, characterized by increases in systolic blood pressure and/or diastolic blood pressure, is a common clinical disorder and a major public health issue (Oparil and Schmieder, 2015; Ye et al., 2017a; Taler, 2018). Hypertension is also a major risk factor for coronary artery disease, heart failure, stroke, and chronic kidney disease (Lau et al., 2017; Taler, 2018). Despite significant advances in antihypertensive therapy, a substantial proportion of patients have uncontrolled blood pressure. As such, there is a continued need to identify new targets to control blood pressure effectively.
Examination of whole-body TRPA1-deficient mice demonstrated that there was no difference in the baseline mean arterial pressure or HR of WT and TRPA1−/− mice under anesthesia (Pozsgai et al., 2010). Conscious C57BL/6 and TRPA1−/− mice have similar morphologic and hemodynamic parameters, including basal blood pressure and HR. In addition, similar blood pressure and HR were also observed after angiotensin II infusion (Bodkin et al., 2014). These studies suggest that mice can maintain basic blood pressure and compensate for TRPA1 deficiency.
Experimental and clinical investigations have tested the key role played by the ANS in modulating cardiovascular functions and controlling blood pressure. In humans and experimental animals, both the increased sympathetic nerve tension and reduced parasympathetic tone activity are associated with and responsible for the appearance and maintenance of hypertension and hypertension-related sequelae (Folkow, 1982; Mancia and Grassi, 2014). Intravenously injected TRPA1 agonist cinnamaldehyde induced a transient hypotensive response followed by a sustained hypertensive response in anesthetized mice. A lower dose of cinnamaldehyde (80 μM/kg) induced a hypotensive response that was significantly less than that in TRPA1−/− mice. Interestingly, the hypertensive response associated with a higher cinnamaldehyde dose (320 μM/kg) was blunted in TRPA1−/− mice. In addition, the cholinergic antagonist atropine significantly inhibited the hypotensive response to the low dose of cinnamaldehyde. The α-adrenergic blocker prazosin significantly inhibited both the hypotensive and hypertensive responses (Pozsgai et al., 2010). Another study showed that inhalation of AITC induced a transient hypertensive response followed by a prolonged hypotensive response in SD rats. Intraperitoneal injection of atropine accentuated the AITC-induced hypertensive response and prevented a hypotensive response. However, pretreatment with terazosin, an α1-adrenergic blocker, prevented the AITC-induced hypertensive response (Hooper et al., 2016). These data indicate that the TRPA1 channel can also influence changes in blood pressure via reflex modulation of the ANS.
Therefore, although mice are able to compensate for TRPA1 deficiency and maintain basic blood pressure, the TRPA1 channel can also influence changes in blood pressure via reflex modulation of the ANS. Given the diverse activation mechanisms of the TRPA1 channel, future studies may uncover more important functions of the channel in blood pressure regulation. Thus, defining the role of the TRPA1 channel in the regulation of blood pressure will provide a new target for future investigations of antihypertensive therapies.
Conclusion
The current review described the potential role of the TRPA1 channel in the regulation of the cardiovascular system. The TRPA1 channel is expressed in the cardiovascular system and involved in mediating a series of cardiovascular pathophysiologies. Activation of the TRPA1 channel has a protective effect against the development of atherosclerosis. Furthermore, TRPA1 channel activation elicits peripheral vasodilation and induces a biphasic blood pressure response. However, loss of channel expression or blockade of its activation suppressed heart failure, myocardial IRI, myocardial fibrosis, and arrhythmia. This finding indicates that the TRPA1 channel can modulate cardiovascular diseases in both positive and negative manners. Given the link between the TRPA1 channel and various cardiovascular diseases, it could be an attractive drug target for therapeutic interventions. However, we should also pay attention to the dual functions of the TRPA1 channel when designing clinical experiments. For this reason, more basic research on the function of the TRPA1 channel must be conducted before TRPA1 channel modulators are used in the clinical setting.
Author Contributions
ZW conceived the review and drafted the manuscript. ZW, DY, JY, MW, JL, HJ, YX, JZ, JC, and JW revised the manuscript critically for important intellectual content. All authors approved the final version of the manuscript submitted. In addition, ZW wants to thank, in particular, the patience, care, and support from Lin Tian over the past years: “Grow old along with me, the best is yet to be.”
Conflict of Interest
The authors declare that the research was conducted in the absence of any commercial or financial relationships that could be construed as a potential conflict of interest.
References
Adam, R. J., Xia, Z., Pravoverov, K., Hong, J., Case, A. J., Schultz, H. D., et al. (2019). Sympathoexcitation in response to cardiac and pulmonary afferent stimulation of TRPA1 channels is attenuated in rats with chronic heart failure. Am. J. Physiol. Heart Circ. Physiol. 316, H862–H872. doi: 10.1152/ajpheart.00696.2018
Anderson, M. E., Brown, J. H., Bers, D. M. (2011). CaMKII in myocardial hypertrophy and heart failure. J. Mol. Cell. Cardiol. 51, 468–473. doi: 10.1016/j.yjmcc.2011.01.012
Andersson, D. A., Gentry, C., Moss, S., Bevan, S. (2008). Transient receptor potential A1 is a sensory receptor for multiple products of oxidative stress. J. Neurosci. 28, 2485–2494. doi: 10.1523/JNEUROSCI.5369-07.2008
Andre, E., Campi, B., Materazzi, S., Trevisani, M., Amadesi, S., Massi, D., et al. (2008). Cigarette smoke-induced neurogenic inflammation is mediated by alpha,beta-unsaturated aldehydes and the TRPA1 receptor in rodents. J. Clin. Invest. 118, 2574–2582. doi: 10.1172/JCI34886
Andrei, S. R., Ghosh, M., Sinharoy, P., Dey, S., Bratz, I. N., Damron, D. S. (2017). TRPA1 ion channel stimulation enhances cardiomyocyte contractile function via a CaMKII-dependent pathway. Channels (Austin) 11, 587–603. doi: 10.1080/19336950.2017.1365206
Andrei, S. R., Sinharoy, P., Bratz, I. N., Damron, D. S. (2016). TRPA1 is functionally co-expressed with TRPV1 in cardiac muscle: Co-localization at z-discs, costameres and intercalated discs. Channels (Austin) 10, 395–409. doi: 10.1080/19336950.2016.1185579
Aubdool, A. A., Kodji, X., Abdul-Kader, N., Heads, R., Fernandes, E. S., Bevan, S., et al. (2016). TRPA1 activation leads to neurogenic vasodilatation: involvement of reactive oxygen nitrogen species in addition to CGRP and NO. Br. J. Pharmacol. 173, 2419–2433. doi: 10.1111/bph.13519
Bacmeister, L., Schwarzl, M., Warnke, S., Stoffers, B., Blankenberg, S., Westermann, D., et al. (2019). Inflammation and fibrosis in murine models of heart failure. Basic Res. Cardiol. 114, 19. doi: 10.1007/s00395-019-0722-5
Bandell, M., Story, G. M., Hwang, S. W., Viswanath, V., Eid, S. R., Petrus, M. J., et al. (2004). Noxious cold ion channel TRPA1 is activated by pungent compounds and bradykinin. Neuron 41, 849–857. doi: 10.1016/S0896-6273(04)00150-3
Bautista, D. M., Jordt, S. E., Nikai, T., Tsuruda, P. R., Read, A. J., Poblete, J., et al. (2006). TRPA1 mediates the inflammatory actions of environmental irritants and proalgesic agents. Cell 124, 1269–1282. doi: 10.1016/j.cell.2006.02.023
Bers, D. M. (2006). Altered cardiac myocyte Ca regulation in heart failure. Physiology (Bethesda) 21, 380–387. doi: 10.1152/physiol.00019.2006
Bertin, S., Aoki-Nonaka, Y., Lee, J., de Jong, P. R., Kim, P., Han, T., et al. (2016). The TRPA1 ion channel is expressed in CD4+ T cells and restrains T-cell-mediated colitis through inhibition of TRPV1. Gut. 66, 1584–1596 doi: 10.1136/gutjnl-2015-310710
Bodkin, J. V., Thakore, P., Aubdool, A. A., Liang, L., Fernandes, E. S., Nandi, M., et al. (2014). Investigating the potential role of TRPA1 in locomotion and cardiovascular control during hypertension. Pharmacol. Res. Perspect 2, e00052. doi: 10.1002/prp2.52
Brook, R. D., Franklin, B., Cascio, W., Hong, Y., Howard, G., Lipsett, M., et al. (2004). Air pollution and cardiovascular disease: a statement for healthcare professionals from the Expert Panel on Population and Prevention Science of the American Heart Association. Circulation 109, 2655–2671. doi: 10.1161/01.CIR.0000128587.30041.C8
Cao, D. S., Zhong, L., Hsieh, T. H., Abooj, M., Bishnoi, M., Hughes, L., et al. (2012). Expression of transient receptor potential ankyrin 1 (TRPA1) and its role in insulin release from rat pancreatic beta cells. PLoS One 7, e38005. doi: 10.1371/journal.pone.0038005
Chen, J., Joshi, S. K., DiDomenico, S., Perner, R. J., Mikusa, J. P., Gauvin, D. M., et al. (2011). Selective blockade of TRPA1 channel attenuates pathological pain without altering noxious cold sensation or body temperature regulation. Pain 152, 1165–1172. doi: 10.1016/j.pain.2011.01.049
Conklin, D. J., Guo, Y., Nystoriak, M. A., Jagatheesan, G., Obal, D., Kilfoil, P. J., et al. (2019). TRPA1 channel contributes to myocardial ischemia–reperfusion injury. Am. J. Physiol. Heart Circ. Physiol. 316, H889–H899. doi: 10.1152/ajpheart.00106.2018
Copeland, K. W., Boezio, A. A., Cheung, E., Lee, J., Olivieri, P., Schenkel, L. B., et al. (2014). Development of novel azabenzofuran TRPA1 antagonists as in vivo tools. Bioorg. Med. Chem. Lett. 24, 3464–3468. doi: 10.1016/j.bmcl.2014.05.069
Corey, D. P., Garcia-Anoveros, J., Holt, J. R., Kwan, K. Y., Lin, S. Y., Vollrath, M. A., et al. (2004). TRPA1 is a candidate for the mechanosensitive transduction channel of vertebrate hair cells. Nature 432, 723–730. doi: 10.1038/nature03066
Defalco, J., Steiger, D., Gustafson, A., Emerling, D. E., Kelly, M. G., Duncton, M. A. (2010). Oxime derivatives related to AP18: Agonists and antagonists of the TRPA1 receptor. Bioorg. Med. Chem. Lett. 20, 276–279. doi: 10.1016/j.bmcl.2009.10.113
Del, C. D., Murphy, S., Heiry, M., Barrett, L. B., Earley, T. J., Cook, C. A., et al. (2010). TRPA1 contributes to cold hypersensitivity. J. Neurosci. 30, 15165–15174. doi: 10.1523/JNEUROSCI.2580-10.2010
Desai, K., Wu, L. (2007). Methylglyoxal and advanced glycation endproducts: new therapeutic horizons? Recent Pat. Cardiovasc. Drug Discov. 2, 89–99. doi: 10.2174/157489007780832498
Du, J., Xie, J., Zhang, Z., Tsujikawa, H., Fusco, D., Silverman, D., et al. (2010). TRPM7-mediated Ca2+ signals confer fibrogenesis in human atrial fibrillation. Circ. Res. 106, 992–1003. doi: 10.1161/CIRCRESAHA.109.206771
Earley, S. (2012). TRPA1 channels in the vasculature. Br. J. Pharmacol. 167, 13–22. doi: 10.1111/j.1476-5381.2012.02018.x
Earley, S., Gonzales, A. L., Crnich, R. (2009). Endothelium-dependent cerebral artery dilation mediated by TRPA1 and Ca2+-Activated K+ channels. Circ. Res. 104, 987–994. doi: 10.1161/CIRCRESAHA.108.189530
Eberhardt, M., Dux, M., Namer, B., Miljkovic, J., Cordasic, N., Will, C., et al. (2014). H2S and NO cooperatively regulate vascular tone by activating a neuroendocrine HNO-TRPA1-CGRP signalling pathway. Nat. Commun. 5, 4381. doi: 10.1038/ncomms5381
Egbuniwe, O., Grover, S., Duggal, A. K., Mavroudis, A., Yazdi, M., Renton, T., et al. (2014). TRPA1 and TRPV4 activation in human odontoblasts stimulates ATP release. J. Dent. Res. 93, 911–917. doi: 10.1177/0022034514544507
Eltzschig, H. K., Eckle, T. (2011). Ischemia and reperfusion–from mechanism to translation. Nat. Med. 17, 1391–1401. doi: 10.1038/nm.2507
Ferdinandy, P., Schulz, R., Baxter, G. F. (2007). Interaction of cardiovascular risk factors with myocardial ischemia/reperfusion injury, preconditioning, and postconditioning. Pharmacol. Rev. 59, 418–458. doi: 10.1124/pr.107.06002
Folkow, B. (1982). Physiological aspects of primary hypertension. Physiol. Rev. 62, 347–504. doi: 10.1152/physrev.1982.62.2.347
Garcia-Dorado, D., Ruiz-Meana, M., Inserte, J., Rodriguez-Sinovas, A., Piper, H. M. (2012). Calcium-mediated cell death during myocardial reperfusion. Cardiovasc. Res. 94, 168–180. doi: 10.1093/cvr/cvs116
Goldhaber, J. I., Philipson, K. D. (2013). Cardiac sodium-calcium exchange and efficient excitation–contraction coupling: implications for heart disease. Adv. Exp. Med. Biol. 961, 355–364. doi: 10.1007/978-1-4614-4756-6_30
Graepel, R., Fernandes, E. S., Aubdool, A. A., Andersson, D. A., Bevan, S., Brain, S. D. (2011). 4-oxo-2-nonenal (4-ONE): evidence of transient receptor potential ankyrin 1-dependent and -independent nociceptive and vasoactive responses in vivo. J. Pharmacol. Exp. Ther. 337, 117–124. doi: 10.1124/jpet.110.172403
Hazari, M. S., Haykal-Coates, N., Winsett, D. W., Krantz, Q. T., King, C., Costa, D. L., et al. (2011). TRPA1 and sympathetic activation contribute to increased risk of triggered cardiac arrhythmias in hypertensive rats exposed to diesel exhaust. Environ. Health Perspect. 119, 951–957. doi: 10.1289/ehp.1003200
He, M. L., Liu, W. J., Sun, H. Y., Wu, W., Liu, J., Tse, H. F., et al. (2011). Effects of ion channels on proliferation in cultured human cardiac fibroblasts. J. Mol. Cell. Cardiol. 51, 198–206. doi: 10.1016/j.yjmcc.2011.05.008
Hooper, J. S., Hadley, S. H., Morris, K. F., Breslin, J. W., Dean, J. B., Taylor-Clark, T. E. (2016). Characterization of cardiovascular reflexes evoked by airway stimulation with allylisothiocyanate, capsaicin, and ATP in Sprague-Dawley rats. J. Appl. Physiol. 120, 580–591. doi: 10.1152/japplphysiol.00944.2015
Jennings, R. B. (2013). Historical perspective on the pathology of myocardial ischemia/reperfusion injury. Circ. Res. 113, 428–438. doi: 10.1161/CIRCRESAHA.113.300987
Jordt, S. E., Bautista, D. M., Chuang, H. H., McKemy, D. D., Zygmunt, P. M., Hogestatt, E. D., et al. (2004). Mustard oils and cannabinoids excite sensory nerve fibres through the TRP channel ANKTM1. Nature 427, 260–265. doi: 10.1038/nature02282
Katz, A. M., Rolett, E. L. (2016). Heart failure: when form fails to follow function. Eur. Heart J. 37, 449–454. doi: 10.1093/eurheartj/ehv548
Khan, R., Spagnoli, V., Tardif, J. C., L’Allier, P. L. (2015). Novel anti-inflammatory therapies for the treatment of atherosclerosis. Atherosclerosis 240, 497–509. doi: 10.1016/j.atherosclerosis.2015.04.783
Khera, A. V., Cuchel, M., de la Llera-Moya, M., Rodrigues, A., Burke, M. F., Jafri, K., et al. (2011). Cholesterol efflux capacity, high-density lipoprotein function, and atherosclerosis. N. Engl. J. Med. 364, 127–135. doi: 10.1056/NEJMoa1001689
Kojima, R., Nozawa, K., Doihara, H., Keto, Y., Kaku, H., Yokoyama, T., et al. (2014). Effects of novel TRPA1 receptor agonist ASP7663 in models of drug-induced constipation and visceral pain. Eur. J. Pharmacol. 723, 288–293. doi: 10.1016/j.ejphar.2013.11.020
Kokel, D., Cheung, C. Y., Mills, R., Coutinho-Budd, J., Huang, L., Setola, V., et al. (2013). Photochemical activation of TRPA1 channels in neurons and animals. Nat. Chem. Biol. 9, 257–263. doi: 10.1038/nchembio.1183
Kunkler, P. E., Ballard, C. J., Oxford, G. S., Hurley, J. H. (2011). TRPA1 receptors mediate environmental irritant-induced meningeal vasodilatation. Pain 152, 38–44. doi: 10.1016/j.pain.2010.08.021
Kurhanewicz, N., Ledbetter, A., Farraj, A., Hazari, M. (2018). TRPA1 mediates the cardiac effects of acrolein through parasympathetic dominance but also sympathetic modulation in mice. Toxicol. Appl. Pharmacol. 347, 104–114. doi: 10.1016/j.taap.2018.03.027
Kurhanewicz, N., Intosh-Kastrinsky, R., Tong, H., Ledbetter, A., Walsh, L., Farraj, A., et al. (2016). TRPA1 mediates changes in heart rate variability and cardiac mechanical function in mice exposed to acrolein. Toxicol. Appl. Pharmacol. 324, 51–60 doi: 10.1016/j.taap.2016.10.008
Langrish, J. P., Watts, S. J., Hunter, A. J., Shah, A. S., Bosson, J. A., Unosson, J., et al. (2014). Controlled exposures to air pollutants and risk of cardiac arrhythmia. Environ. Health Perspect. 122, 747–753. doi: 10.1289/ehp.1307337
Lau, D. H., Nattel, S., Kalman, J. M., Sanders, P. (2017). Modifiable Risk Factors and Atrial Fibrillation. Circulation 136, 583–596. doi: 10.1161/CIRCULATIONAHA.116.023163
Leffler, A., Lattrell, A., Kronewald, S., Niedermirtl, F., Nau, C. (2011). Activation of TRPA1 by membrane permeable local anesthetics. Mol. Pain 7, 62. doi: 10.1186/1744-8069-7-62
Lu, Y., Piplani, H., McAllister, S. L., Hurt, C. M., Gross, E. R. (2016). Transient Receptor Potential Ankyrin 1 Activation within the Cardiac Myocyte Limits Ischemia–reperfusion Injury in Rodents. Anesthesiology 125, 1171–1180. doi: 10.1097/ALN.0000000000001377
Macpherson, L. J., Geierstanger, B. H., Viswanath, V., Bandell, M., Eid, S. R., Hwang, S., et al. (2005). The pungency of garlic: activation of TRPA1 and TRPV1 in response to allicin. Curr. Biol. 15, 929–934. doi: 10.1016/j.cub.2005.04.018
Mancia, G., Grassi, G. (2014). The autonomic nervous system and hypertension. Circ. Res. 114, 1804–1814. doi: 10.1161/CIRCRESAHA.114.302524
Marks, A. R. (2013). Calcium cycling proteins and heart failure: mechanisms and therapeutics. J. Clin. Invest. 123, 46–52. doi: 10.1172/JCI62834
McNamara, C. R., Mandel-Brehm, J., Bautista, D. M., Siemens, J., Deranian, K. L., Zhao, M., et al. (2007). TRPA1 mediates formalin-induced pain. Proc. Natl. Acad. Sci. U. S. A. 104, 13525–13530. doi: 10.1073/pnas.0705924104
Middlekauff, H. R., Park, J., Moheimani, R. S. (2014). Adverse effects of cigarette and noncigarette smoke exposure on the autonomic nervous system: mechanisms and implications for cardiovascular risk. J. Am. Coll. Cardiol. 64, 1740–1750. doi: 10.1016/j.jacc.2014.06.1201
Molkentin, J. D., Lu, J. R., Antos, C. L., Markham, B., Richardson, J., Robbins, J., et al. (1998). A calcineurin-dependent transcriptional pathway for cardiac hypertrophy. Cell 93, 215–228. doi: 10.1016/S0092-8674(00)81573-1
Momiyama, Y., Adachi, H., Fairweather, D., Ishizaka, N., Saita, E. (2014). Inflammation, Atherosclerosis and Coronary Artery Disease. Clin. Med. Insights Cardiol. 8, 67–70. doi: 10.4137/CMC.S39423
Moore, K. J., Sheedy, F. J., Fisher, E. A. (2013). Macrophages in atherosclerosis: a dynamic balance. Nat. Rev. Immunol. 13, 709–721. doi: 10.1038/nri3520
Moore-Morris, T., Guimaraes-Camboa, N., Yutzey, K. E., Puceat, M., Evans, S. M. (2015). Cardiac fibroblasts: from development to heart failure. J. Mol. Med. (Berl.) 93, 823–830. doi: 10.1007/s00109-015-1314-y
Mukhopadhyay, I., Gomes, P., Aranake, S., Shetty, M., Karnik, P., Damle, M., et al. (2011). Expression of functional TRPA1 receptor on human lung fibroblast and epithelial cells. J. Recept. Signal Transduct. Res. 31, 350–358. doi: 10.3109/10799893.2011.602413
Murphy, E., Steenbergen, C. (2008). Mechanisms underlying acute protection from cardiac ischemia–reperfusion injury. Physiol. Rev. 88, 581–609. doi: 10.1152/physrev.00024.2007
Nagata, K., Duggan, A., Kumar, G., Garcia-Anoveros, J. (2005). Nociceptor and hair cell transducer properties of TRPA1, a channel for pain and hearing. J. Neurosci. 25, 4052–4061. doi: 10.1523/JNEUROSCI.0013-05.2005
Nassini, R., Pedretti, P., Moretto, N., Fusi, C., Carnini, C., Facchinetti, F., et al. (2012). Transient receptor potential ankyrin 1 channel localized to non-neuronal airway cells promotes non-neurogenic inflammation. PLoS One 7, e42454. doi: 10.1371/journal.pone.0042454
Nilius, B., Appendino, G., Owsianik, G. (2012). The transient receptor potential channel TRPA1: from gene to pathophysiology. Pflugers Arch. 464, 425–458. doi: 10.1007/s00424-012-1158-z
Nilius, B., Owsianik, G. (2011). The transient receptor potential family of ion channels. Genome Biol. 12, 218. doi: 10.1186/gb-2011-12-3-218
Nilius, B., Szallasi, A. (2014). Transient receptor potential channels as drug targets: from the science of basic research to the art of medicine. Pharmacol. Rev. 66, 676–814. doi: 10.1124/pr.113.008268
Nozawa, K., Kawabata-Shoda, E., Doihara, H., Kojima, R., Okada, H., Mochizuki, S., et al. (2009). TRPA1 regulates gastrointestinal motility through serotonin release from enterochromaffin cells. Proc. Natl. Acad. Sci. U. S. A. 106, 3408–3413. doi: 10.1073/pnas.0805323106
Numaga-Tomita, T., Oda, S., Shimauchi, T., Nishimura, A., Mangmool, S., Nishida, M. (2017). TRPC3 Channels in Cardiac Fibrosis. Front. Cardiovasc. Med. 4, 56. doi: 10.3389/fcvm.2017.00056
Oguri, G., Nakajima, T., Yamamoto, Y., Takano, N., Tanaka, T., Kikuchi, H., et al. (2014). Effects of methylglyoxal on human cardiac fibroblast: roles of transient receptor potential ankyrin 1 (TRPA1) channels. Am. J. Physiol. Heart Circ. Physiol. 307, H1339–H1352. doi: 10.1152/ajpheart.01021.2013
Okada, Y., Shirai, K., Reinach, P. S., Kitano-Izutani, A., Miyajima, M., Flanders, K. C., et al. (2014). TRPA1 is required for TGF-beta signaling and its loss blocks inflammatory fibrosis in mouse corneal stroma. Lab. Invest. 94, 1030–1041. doi: 10.1038/labinvest.2014.85
Oparil, S., Schmieder, R. E. (2015). New approaches in the treatment of hypertension. Circ. Res. 116, 1074–1095. doi: 10.1161/CIRCRESAHA.116.303603
Perez, C. M., Hazari, M. S., Farraj, A. K. (2015). Role of autonomic reflex arcs in cardiovascular responses to air pollution exposure. Cardiovasc. Toxicol. 15, 69–78. doi: 10.1007/s12012-014-9272-0
Petrus, M., Peier, A. M., Bandell, M., Hwang, S. W., Huynh, T., Olney, N., et al. (2007). A role of TRPA1 in mechanical hyperalgesia is revealed by pharmacological inhibition. Mol. Pain 3, 40. doi: 10.1186/1744-8069-3-40
Pires, P. W., Earley, S. (2017). Redox regulation of transient receptor potential channels in the endothelium. Microcirculation 24. doi: 10.1111/micc.12329
Pozsgai, G., Bodkin, J. V., Graepel, R., Bevan, S., Andersson, D. A., Brain, S. D. (2010). Evidence for the pathophysiological relevance of TRPA1 receptors in the cardiovascular system in vivo. Cardiovasc. Res. 87, 760–768. doi: 10.1093/cvr/cvq118
Qian, X., Francis, M., Solodushko, V., Earley, S., Taylor, M. S. (2013). Recruitment of dynamic endothelial Ca2+ signals by the TRPA1 channel activator AITC in rat cerebral arteries. Microcirculation 20, 138–148. doi: 10.1111/micc.12004
Raedschelders, K., Ansley, D. M., Chen, D. D. (2012). The cellular and molecular origin of reactive oxygen species generation during myocardial ischemia and reperfusion. Pharmacol. Ther. 133, 230–255. doi: 10.1016/j.pharmthera.2011.11.004
Roe, A. T., Frisk, M., Louch, W. E. (2015). Targeting cardiomyocyte Ca2+ homeostasis in heart failure. Curr. Pharm. Des. 21, 431–448. doi: 10.2174/138161282104141204124129
Rooney, L., Vidal, A., D’Souza, A. M., Devereux, N., Masick, B., Boissel, V., et al. (2014). Discovery, optimization, and biological evaluation of 5-(2-(trifluoromethyl)phenyl) indazoles as a novel class of transient receptor potential A1 (TRPA1) antagonists. J. Med. Chem. 57, 5129–5140. doi: 10.1021/jm401986p
Schirone, L., Forte, M., Palmerio, S., Yee, D., Nocella, C., Angelini, F., et al. (2017). A Review of the Molecular Mechanisms Underlying the Development and Progression of Cardiac Remodeling. Oxid. Med. Cell. Longev. 2017, 3920195. doi: 10.1155/2017/3920195
Shang, S., Zhu, F., Liu, B., Chai, Z., Wu, Q., Hu, M., et al. (2016). Intracellular TRPA1 mediates Ca2+ release from lysosomes in dorsal root ganglion neurons. J. Cell Biol. 215, 369–381. doi: 10.1083/jcb.201603081
Solanki, A., Bhatt, L. K., Johnston, T. P. (2018). Evolving targets for the treatment of atherosclerosis. Pharmacol. Ther. 187, 1–12. doi: 10.1016/j.pharmthera.2018.02.002
Stevens, J. F., Maier, C. S. (2008). Acrolein: sources, metabolism, and biomolecular interactions relevant to human health and disease. Mol. Nutr. Food Res. 52, 7–25. doi: 10.1002/mnfr.200700412
Story, G. M., Peier, A. M., Reeve, A. J., Eid, S. R., Mosbacher, J., Hricik, T. R., et al. (2003). ANKTM1, a TRP-like channel expressed in nociceptive neurons, is activated by cold temperatures. Cell 112, 819–829. doi: 10.1016/S0092-8674(03)00158-2
Subramanian, U., Nagarajan, D. (2017). All-Trans Retinoic Acid supplementation prevents cardiac fibrosis and cytokines induced by Methylglyoxal. Glycoconj. J. 34, 255–265. doi: 10.1007/s10719-016-9760-5
Takahashi, N., Kuwaki, T., Kiyonaka, S., Numata, T., Kozai, D., Mizuno, Y., et al. (2011). TRPA1 underlies a sensing mechanism for O2. Nat. Chem. Biol. 7, 701–711. doi: 10.1038/nchembio.640
Talavera, K., Gees, M., Karashima, Y., Meseguer, V. M., Vanoirbeek, J. A., Damann, N., et al. (2009). Nicotine activates the chemosensory cation channel TRPA1. Nat. Neurosci. 12, 1293–1299. doi: 10.1038/nn.2379
Taler, S. J. (2018). Initial Treatment of Hypertension. N. Engl. J. Med. 378, 636–644. doi: 10.1056/NEJMcp1613481
Taylor-Clark, T. E., McAlexander, M. A., Nassenstein, C., Sheardown, S. A., Wilson, S., Thornton, J., et al. (2008). Relative contributions of TRPA1 and TRPV1 channels in the activation of vagal bronchopulmonary C-fibres by the endogenous autacoid 4-oxononenal. J. Physiol. 586, 3447–3459. doi: 10.1113/jphysiol.2008.153585
Thodeti, C. K., Paruchuri, S., Meszaros, J. G. (2013). A TRP to cardiac fibroblast differentiation. Channels (Austin) 7, 211–214. doi: 10.4161/chan.24328
Thompson, L. C., Walsh, L., Martin, B. L., McGee, J., Wood, C., Kovalcik, K., et al. (2019). Ambient Particulate Matter and Acrolein Co-Exposure Increases Myocardial Dyssynchrony in Mice via TRPA1. Toxicol. Sci. 167, 559–572. doi: 10.1093/toxsci/kfy262
Trevisani, M., Siemens, J., Materazzi, S., Bautista, D. M., Nassini, R., Campi, B., et al. (2007). 4-Hydroxynonenal, an endogenous aldehyde, causes pain and neurogenic inflammation through activation of the irritant receptor TRPA1. Proc. Natl. Acad. Sci. U. S. A. 104, 13519–13524. doi: 10.1073/pnas.0705923104
Venkatachalam, K., Montell, C. (2007). TRP channels. Annu. Rev. Biochem. 76, 387–417. doi: 10.1146/annurev.biochem.75.103004.142819
Viana, F. (2016). TRPA1 channels: molecular sentinels of cellular stress and tissue damage. J. Physiol. 594, 4151–4169. doi: 10.1113/JP270935
Wang, Y. Y., Chang, R. B., Waters, H. N., McKemy, D. D., Liman, E. R. (2008). The nociceptor ion channel TRPA1 is potentiated and inactivated by permeating calcium ions. J. Biol. Chem. 283, 32691–32703. doi: 10.1074/jbc.M803568200
Wang, Z., Wang, M., Liu, J., Ye, J., Jiang, H., Xu, Y., et al. (2018a). Inhibition of TRPA1 Attenuates Doxorubicin-Induced Acute Cardiotoxicity by Suppressing Oxidative Stress, the Inflammatory Response, and Endoplasmic Reticulum Stress. Oxid. Med. Cell. Longev. 2018, 5179468. doi: 10.1155/2018/5179468
Wang, Z., Xu, Y., Wang, M., Ye, J., Liu, J., Jiang, H., et al. (2018b). TRPA1 inhibition ameliorates pressure overload-induced cardiac hypertrophy and fibrosis in mice. EBioMedicine. 36, 54–62. doi: 10.1016/j.ebiom.2018.08.022
Wei, H., Hamalainen, M. M., Saarnilehto, M., Koivisto, A., Pertovaara, A. (2009). Attenuation of mechanical hypersensitivity by an antagonist of the TRPA1 ion channel in diabetic animals. Anesthesiology 111, 147–154. doi: 10.1097/ALN.0b013e3181a1642b
Woll, K. A., Skinner, K. A., Gianti, E., Bhanu, N. V., Garcia, B. A., Carnevale, V., et al. (2017). Sites Contributing to TRPA1 Activation by the Anesthetic Propofol Identified by Photoaffinity Labeling. Biophys. J. 113, 2168–2172. doi: 10.1016/j.bpj.2017.08.040
Yamamoto, S., Shimizu, S. (2016). Significance of TRP channels in oxidative stress. Eur. J. Pharmacol. 793, 109–111. doi: 10.1016/j.ejphar.2016.11.007
Ye, J., Ji, Q., Liu, J., Liu, L., Huang, Y., Shi, Y., et al. (2017a). Interleukin 22 Promotes Blood Pressure Elevation and Endothelial Dysfunction in Angiotensin II-Treated Mice. J. Am. Heart Assoc. 6. doi: 10.1161/JAHA.117.005875
Ye, J., Wang, M., Xu, Y., Liu, J., Jiang, H., Wang, Z., et al. (2017b). Sestrins increase in patients with coronary artery disease and associate with the severity of coronary stenosis. Clin. Chim. Acta 472, 51–57. doi: 10.1016/j.cca.2017.07.020
Ye, J., Wang, Z., Ye, D., Wang, Y., Wang, M., Ji, Q., et al. (2019). Increased Interleukin-11 Levels Are Correlated with Cardiac Events in Patients with Chronic Heart Failure. Mediators Inflamm. 2019, 1575410. doi: 10.1155/2019/1575410
Yue, Z., Zhang, Y., Xie, J., Jiang, J., Yue, L. (2013). Transient receptor potential (TRP) channels and cardiac fibrosis. Curr. Top. Med. Chem. 13, 270–282. doi: 10.2174/1568026611313030005
Zhang, N., Wei, W. Y., Li, L. L., Hu, C., Tang, Q. Z. (2018). Therapeutic Potential of Polyphenols in Cardiac Fibrosis. Front. Pharmacol. 9, 122. doi: 10.3389/fphar.2018.00122
Zhao, J. F., Shyue, S. K., Kou, Y. R., Lu, T. M., Lee, T. S. (2016). Transient Receptor Potential Ankyrin 1 Channel Involved in Atherosclerosis and Macrophage-Foam Cell Formation. Int. J. Biol. Sci. 12, 812–823. doi: 10.7150/ijbs.15229
Zhou, T., Chuang, C. C., Zuo, L. (2015). Molecular Characterization of Reactive Oxygen Species in Myocardial Ischemia-Reperfusion Injury. Biomed Res. Int. 2015, 864946. doi: 10.1155/2015/864946
Zurborg, S., Yurgionas, B., Jira, J. A., Caspani, O., Heppenstall, P. A. (2007). Direct activation of the ion channel TRPA1 by Ca2+. Nat. Neurosci. 10, 277–279. doi: 10.1038/nn1843
Keywords: TRPA1 channel, atherosclerosis, heart failure, myocardial ischemia–reperfusion injury, myocardial fibrosis, arrhythmia, vasodilation, hypertension
Citation: Wang Z, Ye D, Ye J, Wang M, Liu J, Jiang H, Xu Y, Zhang J, Chen J and Wan J (2019) The TRPA1 Channel in the Cardiovascular System: Promising Features and Challenges. Front. Pharmacol. 10:1253. doi: 10.3389/fphar.2019.01253
Received: 07 July 2019; Accepted: 27 September 2019;
Published: 18 October 2019.
Edited by:
Martin C. Michel, Johannes Gutenberg, University Mainz, GermanyReviewed by:
Philip Aaronson, King’s College London, United KingdomJennifer Beth Stott, University of London, United Kingdom
Copyright © 2019 Wang, Ye, Ye, Wang, Liu, Jiang, Xu, Zhang, Chen and Wan. This is an open-access article distributed under the terms of the Creative Commons Attribution License (CC BY). The use, distribution or reproduction in other forums is permitted, provided the original author(s) and the copyright owner(s) are credited and that the original publication in this journal is cited, in accordance with accepted academic practice. No use, distribution or reproduction is permitted which does not comply with these terms.
*Correspondence: Jun Wan, d2FuanVuQHdodS5lZHUuY24=
†These authors have contributed equally to this work