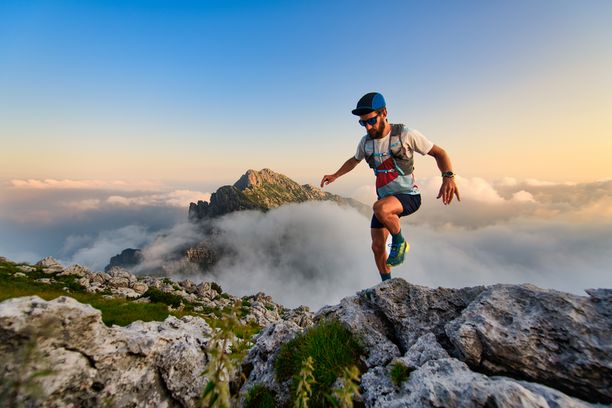
94% of researchers rate our articles as excellent or good
Learn more about the work of our research integrity team to safeguard the quality of each article we publish.
Find out more
ORIGINAL RESEARCH article
Front. Pharmacol., 14 November 2019
Sec. Predictive Toxicology
Volume 10 - 2019 | https://doi.org/10.3389/fphar.2019.01098
This article is part of the Research TopicThe Molecular Mechanism of the Toxicity and Metabolism of MycotoxinsView all 8 articles
Deoxynivalenol (DON) is a major fusarium toxin widely detected in cereal grains. The inadvertent exposure to this fungal secondary-metabolite gives rise to a myriad of adverse health effects including appetite loss, emesis, and suppression of the immune system. While most of the attention this mycotoxin has gained in the past four decades was related to its eukaryotic toxicity (monogastric animals and plants more precisely), recent studies have begun to reveal its negative influence on prokaryotes. Recently presented evidence indicates that DON can negatively affect many bacterial species, raising the possibility of DON-induced imbalances within the microbiota of the human and animal gut, in addition to other environmental niches. This in turn has led to a greater interest in understanding bacterial responses toward DON, and the involved mechanism(s) and metabolic pathways, in order to build a more comprehensive picture of DON-induced changes in both prokaryotes and eukaryotes alike. This study reveals the transcriptomic profiling of Devosia mutans strain 17-2-E-8 after the inclusion of DON within its growth medium. The results highlight three adaptive mechanisms involved in the response of D. mutans 17-2-E-8 to this mycotoxin, which include: (a) activation of adenosine 5’-triphosphate-binding cassette transporters; (b) engagement of a toxin-specific pyrroloquinoline quinone-dependent detoxification pathway; and finally (c) the upregulation of auxiliary coping proteins such as porins, glutathione S-transferases, and phosphotransferases. Some of the identified mechanisms are universal in nature and are shared with other bacterial genera and species.
Deoxynivalenol (DON) is a major fusarium mycotoxin widely present in cereal food/feed, which is produced by invasive fungal species during plant pathogenesis. Exposure of humans and animals to this fungal secondary-metabolite manifests in a myriad of short- and long-term adverse health effects, ranging from appetite loss, emesis, decreased weight gain, to the suppression of immune systems, depending on the exposure dose, its frequency, and the physiological stage of the exposed host (Hassan et al., 2015). Moreover, the ability of DON to interfere with the absorption of many nutrients, through the suppression of protein biosynthesis (Peng et al., 2017a) and/or interference with cellular membrane integrity (De Walle et al., 2010; Ghareeb et al., 2015), is well documented.
The past decade has witnessed a vast increase in our knowledge of gut microbiota and its role in human and animal health, which has led to greater scrutiny of environmental factors that may influence its composition. As most bacterial hosts can tolerate many-fold higher concentrations of DON than eukaryotes (Takakura et al., 2014), the toxicological effects of DON on most prokaryotes have been considered erroneously negligible (Suzuki and Iwahashi, 2015). However, several recent reports have revealed that exposure to DON can affect intestinal mucus production and the functional diversity of gut microbiota (Piotrowska et al., 2014; Peng et al., 2017b; Robert et al., 2017), as well as increasing the genotoxicity of certain chemicals in specific hosts (Payros et al., 2017), thereby highlighting the need for further investigations of DON effects in prokaryotes as well as in eukaryotes (Park et al., 2014; Ishikawa et al., 2017). A better understanding of the bacterial response to DON at the transcriptome level could also lead to the identification of more novel bacterial detoxification mechanisms, which can be applied to help address the growing problem of DON contamination in agricultural commodities.
The present work reports on the transcriptional response of Devosia mutans 17-2-E-8, an efficient DON detoxifier, to DON exposure probed by transcriptome profiling and connecting the observed changes with phenotype outcomes.
D. mutans 17-2-E-8 cells were grown and maintained as reported previously (He et al., 2016). The high-performance liquid chromatography (HPLC)-grade methanol used for extractions was obtained from Caledon Labs (Georgetown, ON, Canada), while DON was obtained either from Sigma-Aldrich (Oakville, ON, Canada) or TripleBond (Guelph, ON, Canada). Bacterial pellets were collected by centrifugation at 8,500 rpm for 30 min and stored frozen at -20°C until usage unless stated otherwise. 3-epi-DON, used as standard, was prepared as reported previously (He et al., 2015b).
In order to establish the inhibitory effect of DON on the growth of D. mutans 17-2-E-8 cells and their DON epimerization capacity, cultures (1.5 ml) of D. mutans 17-2-E-8 containing 1 × 106 colony forming units per milliliter were added as inoculum to cornmeal broth (He et al., 2016) (12.0 ml) supplemented with DON standards (1.5 ml) in sterile water. The final concentrations of DON spanned the 100–4,000 μg/ml range. These cultures were incubated at 28°C on a rotary shaker at 200 rpm up to 132 h. Bacterial cell numbers were enumerated every 12 h by plating serial dilutions onto cornmeal agar plates. The number of colony forming units of each dilution was determined after 72–96 h incubation at 28°C.
To evaluate the effect of DON on the ability of D. mutans 17-2-E-8 to enzymatically transform DON into 3-epi-DON (He et al., 2016), 150 μl of the liquid bacterial culture was collected at 6, 12, 24, 36, 48, 60, 72, 84, 96, 108, 120, and 132 h. The reactions were stopped by adding 150-μl methanol. Mixtures were allowed to stand for 2 h then centrifuged at 18,000×g for 5 min (Micromax® Microcentrifuge, Milford, MA, USA) before analyzing DON by the HPLC approach as outlined previously (He et al., 2016). An Agilent Technologies 1100 Series HPLC system equipped with a Luna C18 column (150 × 4.6 mm, 5 μm) (Phenomenex, Torrance, CA, USA) was used in the previously stated analyses. The binary mobile phase consisted of methanol (solvent A) and water (solvent B), and the gradient program started at 22% A, increased linearly to 41% A at 5 min, 100% A at 7 min, held at 100% A from 7 to 9 min, and returned to 22% A at 11 min. There was a post-run column reconditioning phase (2 min) under the starting conditions. The flow rate was held constant at 1.0 ml/min, and the detector was set at 218 nm. The identification and quantification of DON and 3-epi-DON were achieved through comparing the retention times, ultraviolet–visible spectra, and concentrations of pure standards. Bacterial growth and DON transformations were conducted in triplicate, and the mean values were reported.
An overnight D. mutans 17-2-E-8 culture grown in 60-ml lysogeny broth (supplemented with 50 μg/ml kanamycin) at OD600 > 1 was challenged with DON at a final concentration of 50 µg/ml. Samples (10 ml each) were collected before DON addition and at 1, 3, and 5 h after DON addition. Cells were harvested by centrifugation for 5–10 min at 13,000 rpm, and RNAlater Solution (Ambion, cat. # AM7021) was added for RNA stabilization during the cold storage.
Total RNA extractions were conducted using the RNeasy Mini kit (Qiagen, Toronto, ON; cat. #74104) according to the manufacturer’s recommendations. RNA integrity and concentrations were evaluated using the Bio-Rad Experion Automated Electrophoresis System (Bio-Rad Laboratories, Mississauga, ON). After ensuring the quality and integrity of the obtained RNA samples (Supplementary Figures 1A, B), the samples were treated with rDNase I (Ambion) for 1 h at 37°C (cat #: AM2235, Life Technologies Inc.) following the manufacturer’s instructions.
rRNA depletion was performed using RiboMinus Transcriptome Isolation Kit—bacteria module (Thermo Fisher Scientific, Ottawa, ON; cat. # K155004) according to the recommended protocol, and RNA-Seq libraries were prepared from 200 ng of RNA at the University Health Network/Mount Sinai Hospital—Gene Profiling Facility (Toronto, ON). The validated libraries were pooled and sequenced on HiSeq 2500 Sequencing System (Illumina, Inc.) with 100-bp paired-end sequencing runs. The resulting reads were analyzed using CLC Genomics Workbench 6.5.2 (CLC, http://www.clcbio.com/products/clc-genomics-workbench/) according to the supplier’s procedure. In essence, a transcriptomic analysis was initiated with unpaired two-group comparisons (control and DON treatment) using the existing expression values obtained from samples. Gene expression levels were normalized as reads per kilobase of transcript per million mapped reads, and the differentially expressed genes (up- or downregulated) between the control (time zero) and the sampling points (1, 3, and 5 h after DON addition) were presented as fold-changes (Supplementary Table 1).
One microgram of DNase-treated total RNA (the same sample submitted for the RNA-Seq analysis) was reverse transcribed to complementary DNA and amplified with gene-specific primers (Supplementary Table 2) using iTaq Universal SYBR green Supermix (Bio-Rad) on a ViiA™ 7 Real-Time PCR System (Applied Biosystems by Life Technologies, Austin, TX, USA). Samples were normalized to the expression of bacterial 16S rRNA methyltransferase using the ∆∆Ct method (Livak and Schmittgen, 2001; Cikos et al., 2007). These analyses were conducted in triplicate.
For bacterial cell counts, DON concentrations, and quantitative polymerase chain reaction (qPCR) analysis of transcripts, each sample was analyzed in triplicate, and mean values were determined. The relevant reduction of DON was calculated as follows:
Reduction of DON concentration (%) = (CDON added – CDON residual)/CDON added × 100.
The collected data were analyzed using SAS (SAS for Windows, Version 9.1, SAS institute, Cary, NC, USA) or Sigmaplot 12.5 (Systat Software, Inc). The data were tested for normality using the Kolmogorov–Smirnov method and equal variance (P value to reject was set for 0.05). Multiple group comparisons of normally distributed data were conducted by one-way analysis of variance, followed by Fisher’s protected least significant difference test. Treatments were arranged in a completely randomized fashion.
D. mutans 17-2-E-8 cells exhibited similar growth rates when grown in the complete absence or presence of low- to medium-range concentrations of DON (100 to 500 μg/ml) (Figure 1) indicating an effective adaptive/response mechanism(s), whereas growth was negatively affected only by high DON concentrations (2,000–4,000 μg/ml) (Figure 1). The reported high levels of DON exceed the maximum tolerated levels of DON in human food chain (2 μg/ml) and animal feed chain (5 μg/ml) by at least 400–2,000 times. Interestingly, the addition of 1,000 μg/ml DON resulted in a reduced growth only within the first 72 h following media inoculation, indicating that D. mutans 17-2-E-8 cells in the stationary phase may be more resistant to DON compared with actively dividing/growing cells.
Figure 1 The effect of increasing DON concentrations on growth patterns of D. mutans 17-2-E-8 in cornmeal broth cultures at 28°C and 200 rpm. The suppressive effect of DON was only noticed in concentrations that surpassed the 1,000 µg/ml indicating a very effective defense mechanism(s) of this bacterium against DON. The protected least significant difference [PLSD(0.05)] of the presented results was 0.32.
Figure 2 demonstrates the effect of DON concentrations on the ability of D. mutans 17-2-E-8 to metabolize DON, which has been previously shown to occur through the formation of 3-epi-DON using 3-keto-DON as an intermediate (Hassan et al., 2017). As expected, a reduction in DON concentrations was observed in cultures incubated with up to 1,000 µg/ml DON in parallel to our earlier reported studies (He et al., 2016; Hassan et al., 2017). This is despite the fact that a DON concentration of 1,000 µg/ml decreased the bacterial transformation rates of this mycotoxin in comparison with lower DON concentrations (in the 100–500 µg/ml range) within the 36–72-h time frame (Figure 2). Increasing DON concentrations beyond that point significantly and negatively correlated with the ability of D. mutans 17-2-E-8 to eliminate DON from the growth medium. The inhibitory effect of high DON concentrations on the transformation functionality was most evident at the 4,000 μg/ml, where the transformation activity was completely abrogated and could not be restored even with extended incubation times, compared with the 2,000 μg/ml dose, where the DON transformation capacity was restored partially by 84 h of incubation (Figure 2). This latter observation might be partially connected with the reduced sensitivity of bacterial cells (in their stationary phase) toward DON, resulting in an increased cell viability reported earlier, around the same time frame.
Figure 2 Deoxynivalenol biotransformation capacity of D. mutans 17-2-E-8. The ability of D. mutans 17-2-E-8 to transform DON (in growth media) is only saturated at extremely high concentrations of the toxin. Concentrations that ranged from 10 to 1,000 µg/ml had no negative effect on the bacterium’s ability to oxidize/epimerize DON (He et al., 2016) in cornmeal broth cultures at 28°C and 200 rpm. The protected least significant difference [PLSD(0.05)] of the test was 4.0.
To identify D. mutans 17-2-E-8 genes/enzymes that might play a role in this microbe response to DON, we profiled it's transcriptome over multiple time points after challenging overnight-grown D. mutans 17-2-E-8 cultures with 50 μg/ml DON.
The analysis of the depleted bacterial cultures confirmed DON reduction and its transformation to 3-epi-DON (Figure 3) as expected (Hassan et al., 2017). DON disappearance from the analyzed samples, coupled with 3-epi-DON accumulation as a final product, is particularly evident in Figure 3-D.
Figure 3 D. mutans 17-2-E-8 ability to biotransform DON. The obtained high-performance liquid chromatography results of overnight bacterial culture samples confirmed the ability of D. mutans 17-2-E-8 to epimerize DON and form the 3-epi-DON stereoisomer. The bacterial culture was utilized directly for total RNA preparation. Panel (A) represents DON standard in LB broth while panel (B) represents the fresh bacterial culture supplemented with DON (50 µg/ml) after one hour of growth. Panel (C) reflects the chromatogram of 3-epi-DON standard in fresh LB broth while the (D) panel shows the depleted bacterial culture after an overnight incubation with DON confirming DON disappearance and complete transformation into 3-epi-DON.
Transcriptional profiling of D. mutans 17-2-E-8 exposed to DON revealed 433 transcripts that were upregulated (≥threefold) within 5 h of DON addition (maximum response). The results are listed in Supplementary Table 1, along with the microbe’s response after 1 and 3 h. While this study focused solely on the upregulated transcripts (bearing in mind potential future industrial applications) rather than the downregulated ones, we have listed all downregulated and upregulated transcripts alike in Supplementary Table 1 due to their scientific importance.
The close inspection of the upregulated transcripts revealed the induction of several key stress and resistance genes/proteins that included, but not limited to, multiple adenosine 5’-triphosphate-binding cassette (ABC) transporters, ribosome-associated translation inhibitors, and porins in addition to various enzymes that could be implicated in xenobiotics detoxification(s) such as dehydrogenases, dioxygenases, a cytochrome P450, a chloramphenicol phosphotransferase, and a glutathione S-transferase. A short list of selected proteins previously reported to be part of microbial defense mechanisms (Pasternak et al., 1989; Niederweis, 2003; Harland et al., 2005; Piddock, 2006), coupled with their fold increases, is shown in Table 1. The majority of the upregulated transcripts within the 5-h exposure period belonged to the ABC-dependent transporters/effluxors (45%, 32 out of 71) with increases that spanned 3.04-fold (JP74_19625 ABC transporter permease) to ∞ (JP74_23460 sugar ABC transporter permease). The second protein group/response mechanism that was upregulated by large was cellular dehydrogenases (15%) and oxidoreductase (14%) (Table 1). The importance of some of these dehydrogenases [especially pyrroloquinoline quinone (PQQ)-dependent ones] is discussed later. Finally, multiple coping enzymes/proteins, including a cytochrome P450 (3.01-fold increase), a glutathione S-transferase (3.01-fold increase), a ribosome-associated translation inhibitor (7.9-fold increase), the JP74_12500 porin (3.24-fold increase), and a chloramphenicol phosphotransferase (3.15-fold increase), reflected increased upregulation in expression levels (Table 1).
Table 1 Devosia mutans 17-2-E-8 genes related to detoxification/defense mechanism(s) that were upregulated due to deoxynivalenol (50 µg/ml) inclusion in bacterial growth media.
Notably many PQQ-dependent transcripts were overexpressed as a result of DON inclusion within the growth medium (Figure 4 and Table 1). Multiple PQQ-dependent dehydrogenases including JP74_20580, JP74_15915, and JP74_18865 were upregulated up to 4.4-, 13.78-, and 15.25-fold increases, respectively, after 5-h growth in DON-supplemented medium, in comparison with the negative control (Table 1). At the same time, enzymes putatively involved in PQQ redox cofactor biosynthesis, such as PqqB, PqqD, and PqqE, each showed a gradual increase in expression levels spanning the 1–3-h time frame, with a maximum induction (5.03-, 5.15-, and 3.27-fold, respectively) after 5 h of continuous DON exposure (Table 1).
Figure 4 The horizontal comparisons of expression levels of RNA samples extracted from bacterial cells before and after challenging with DON (50 µg/ml). The graph clearly shows that multiple PQQ-dependent enzymes/defense mechanisms have increased due to DON treatment. The presented results were normalized using the reads per kilobase of transcript per million mapped reads. Points/dots above the diagonal gray line indicate upregulated transcripts, while points beneath that line indicate the downregulation/suppression of the involved transcript.
To validate these results, we used the qPCR screening to verify the expression levels of several genes, including two PQQ-dependent dehydrogenases (JP74_18865 and JP74_15915), an oxidoreductase (JP74_11190), and a hypothetical protein (JP74_20250). A close correlation was observed between the expression levels investigated by the qPCR approach and the obtained RNA-Seq data, thereby substantiating the RNA-Seq outcomes (Figure 5). Surprisingly, the qPCR data reflected higher fold increases in some cases (Figure 5D) in comparison with the results collected by RNA-Seq. Such a nonconformity between the two methodologies is well known and has been previously reported (Robinson et al., 2015; Everaert et al., 2017).
Figure 5 The tendencies observed in the RNA_Seq results were confirmed using a quantitative and semiquantitative polymerase chain reactions (qPCR): (A) the semiquantitative detection/amplification of the JP74_20250 gene to check the quality of the prepared complementary DNA strands, (B) the 16S rRNA methyltransferase (JP74_06180) gene was used as the housekeeping gene for the normalization and calculation of fold increases of transcripts amplified using the qPCR approach, (C) an example of the qPCR amplification/detection of a PQQ-dependent dehydrogenase (JP74_15915) and its relative expression in response to DON (D) in comparison to unchallenged bacterial cells (control).
Understanding how mycotoxins influence prokaryotes began to emerge as an important area of future research focus (Robert et al., 2017; Liao et al., 2018). This is particularly true in the light of the recent studies that implicated gut microbiota in DON-induced anorexia (Peng et al., 2017b), DON-induced immunotoxicity with reported changes in gut bacteria (Liao et al., 2018), and DON ability to modulate the genotoxicity of colibactin-producing Escherichia coli strains/isolates (Payros et al., 2017). The ability of even a single dose of mycotoxins to induce significant changes within the gut microbial communities (Ishikawa et al., 2017) establishes the urgency to fully engage in deciphering the effect of DON on as many prokaryotic species as possible in order to delineate the production and health-related ramifications of DON/microbial interactions.
The presented work is among the first to look into the changes induced in the global gene-regulation/expression levels (as a result of DON exposure) within a potent bacterial strain isolated mainly for its ability to transform/detoxify DON (He et al., 2016). While the studied bacterial isolate, D. mutans 17-2-E-8, did not originate from gut, the recently established role of Devosia species in gut’s health with links associating the decreased abundance of this genus with irritable bowel syndrome in humans (Ng et al., 2013) and gut microbiota dysbiosis (due to Salmonella infantis infections) in animals both justify looking into any transcriptomic changes induced in this genus due to DON exposure. Furthermore, the ability of multiple Devosia strains including D. mutans 17-2-E-8 (He et al., 2016), D. sp. ANSB714 (Zhao et al., 2016), D. RS1 and SS5 (Sato et al., 2012), and D. sp. strain A16 (Yin et al., 2016) to transform DON to less toxic metabolites, either 3-keto-DON and/or 3-epi-DON (He et al., 2015a; Hassan et al., 2016), with the possibility of incorporating of such detoxification pathways into animal feed applications (Yin et al., 2016; Zhao et al., 2016), necessitates a closer engagement with this genus to avoid any uncounted for detrimental effects in the future.
The collected results clearly show that the studied bacterium (pure culture) has the ability to withstand much higher concentrations of DON (up to 1,000 μg/ml), which fall significantly above the maximum permitted levels of DON (5–10 μg/ml) within any animal feed/human food. This in turn enhances the chances for incorporating the actively growing cells of this microorganism in future DON-mitigation strategies intended for the animal feed chain. At the same time, the influence of DON on D. mutans 17-2-E-8 needs to be further complemented with data related to mixed cultures and/or bio-niches that mimic the composition of natural microbiota within the animal gut, where other factors such as pH, low-oxygen levels, and bacterial competition(s) play fundamental roles and possibly render D. mutans 17-2-E-8 more/less susceptible toward DON.
The obtained results reflect a three-legged strategy that this bacterium utilizes to circumvent DON toxicity: (a) first, by upregulating multiple ABC-dependent membrane transporters and efflux pumps that remove many undesirable toxins/chemicals (including DON) from the cellular environment (Sharma et al., 2003; Barabote et al., 2011); (b) second, the bacterium engages a toxin-specific detoxification mechanism carried by certain dehydrogenases/oxidoreductases to reduce and eliminate the cellular toxicity of the involved toxin. In DON case, the 17-2-E-8 isolate specifically upregulates a PQQ-dependent glucose dehydrogenase that oxidizes DON to 3-keto-DON as a first-step detoxification followed by the subsequent reduction to the more stable/nontoxic 3-epi-DON isomer (Carere et al., 2018a; Hassan et al., 2017). The very recent isolation of the DON-C3 carbon oxidation/reduction enzymes using various protein fractionation techniques (Carere et al., 2018a; Carere et al., 2018b) supports this conclusion; (c) third, this microorganism employs an arsenal of auxiliary proteins including porins, glutathione S-transferases, ribosome-associated translation inhibitors, and chloramphenicol phosphotransferases, which is part of an efficient strategy that aims to block/minimize the entry of DON to the cytoplasm, introduce chemical modifications to minimize the overall toxicity, reduces translation errors/inhibitions (Agafonov and Spirin, 2004) associated with DON exposure (Pan et al., 2014), and/or increase toxin’s solubility to enhance toxin’s efflux/elimination. Although some of the mechanisms identified earlier are confined to DON-transforming Devosia (the PQQ-dependent pathway for example), the other mechanisms that are built upon the upregulation of adenosine 5’-triphosphate-dependent transporters are more universal in nature and indeed representative of widely speared response/resistance mechanisms in bacteria including multiple antibiotic resistance (Nikaido, 2009; Nikaido and Pages, 2012; Du et al., 2015; Wendlandt et al., 2015).
While our results are consistent with the ones reported by Park et al. (2014) in which the authors showed the ability of DON to influence ABC-dependent membrane transporters, bacterial chemotaxis, succinate dehydrogenases, and enzymes related to the glyoxylate cycle within E. coli K-12, they differ in the observed tendencies and magnitudes. The low concentrations that were used in Park’s study (0.2 and 2 µg/ml) did not lead to any upregulations of identified ABC transporters, while the upregulation of such transporters was certainly evident in our case. This can be attributed to the higher concentrations of DON that we have used (50 µg/ml). The facts that most prokaryotes/eukaryotes show a significant increase in a large number of ABC transporters in the due course of many mycotoxins exposures (Anzai et al., 2010; Dubey et al., 2014; Walter et al., 2015) validate our results and show the importance of this defense mechanism within its evolutionary context. In addition, the fact that DON levels can reach as high as 25–93 µg/g in plant materials and wheat kernels (Sinha and Savard, 1997) makes the use of high doses of DON (50 µg/ml in our RNA_Seq studies) more relevant to certain scenarios of animal/human exposure.
Finally, the results of this study can be useful not only in understanding how gram-negative bacteria respond to DON at the transcriptomic level but can also aid in developing and optimizing DON detoxification approaches (through the identification of novel enzymes) that can alleviate the toxicity of DON and possibly be utilized in a later stage within refined agricultural and industrial applications (Carere et al., 2018a).
Design of the work: YH, JH, DL, and TZ. Conducting experiments: YH and JH. Interpretation of data and analysis: YH, JH, DL, and TZ. Drafting the work: YH, JH, and TZ. Final approval: YH, JH, DL, and TZ.
The authors declare that the research was conducted in the absence of any commercial or financial relationships that could be construed as a potential conflict of interest.
The authors would like to thank Xiu-Zhen Li and Honghui Zhu for their valuable technical advice/assistance and Agriculture and Agri-Food Canada (AAFC Project #J-001498) for the financial support of this research work.
The Supplementary Material for this article can be found online at: https://www.frontiersin.org/articles/10.3389/fphar.2019.01098/full#supplementary-material
Supplementary Table 1 | A comprehensive list of Devosia mutans 17-2-E-8 transcripts that have been modulated (up or down-regulated) in response to deoxynivalenol (50 µg/ml) inclusion in bacterial growth media.
Agafonov, D. E., Spirin, A. S. (2004). The ribosome-associated inhibitor a reduces translation errors. Biochem. Biophys. Res. Commun. 320, 354–358. doi: 10.1016/j.bbrc.2004.05.171
Anzai, N., Jutabha, P., Endou, H. (2010). Molecular mechanism of ochratoxin a transport in the kidney. Toxins (Basel) 2, 1381–1398. doi: 10.3390/toxins2061381
Barabote, R. D., Thekkiniath, J., Strauss, R. E., Vediyappan, G., Fralick, J. A., San Francisco, M. J. (2011). Xenobiotic efflux in bacteria and fungi: a genomics update. Adv. Enzymol. Relat. Areas Mol. Biol. 77, 237–306. doi: 10.1002/9780470920541.ch6
Carere, J., Hassan, Y. I., Lepp, D., Zhou, T. (2018a). The enzymatic detoxification of the mycotoxin deoxynivalenol: identification of DepA from the DON epimerization pathway. Microb. Biotechnol. 11(6):1106-1111. doi: 10.1111/1751-7915.12874.
Carere, J., Hassan, Y. I., Lepp, D., Zhou, T. (2018b). The identification of DepB: an enzyme responsible for the final detoxification step in the deoxynivalenol epimerization pathway in Devosia mutans 17-2-E-8. Front. Microbiol. 9, 1573. doi: 10.3389/fmicb.2018.01573
Cikos, S., Bukovska, A., Koppel, J. (2007). Relative quantification of mRNA: comparison of methods currently used for real-time PCR data analysis. BMC Mol. Biol. 8, 113. doi: 10.1186/1471-2199-8-113
De Walle, J. V., Sergent, T., Piront, N., Toussaint, O., Schneider, Y. J., Larondelle, Y. (2010). Deoxynivalenol affects in vitro intestinal epithelial cell barrier integrity through inhibition of protein synthesis. Toxicol. Appl. Pharmacol. 245, 291–298. doi: 10.1016/j.taap.2010.03.012
Du, D., Van Veen, H. W., Murakami, S., Pos, K. M., Luisi, B. F. (2015). Structure, mechanism and cooperation of bacterial multidrug transporters. Curr. Opin. Struct. Biol. 33, 76–91. doi: 10.1016/j.sbi.2015.07.015
Dubey, M. K., Jensen, D. F., Karlsson, M. (2014). An ATP-binding cassette pleiotropic drug transporter protein is required for xenobiotic tolerance and antagonism in the fungal biocontrol agent Clonostachys rosea. Mol. Plant Microbe Interact. 27, 725–732. doi: 10.1094/MPMI-12-13-0365-R
Everaert, C., Luypaert, M., Maag, J. L. V., Cheng, Q. X., Dinger, M. E., Hellemans, J., et al. (2017). Benchmarking of RNA-sequencing analysis workflows using whole-transcriptome RT-qPCR expression data. Sci. Rep. 7, 1559. doi: 10.1038/s41598-017-01617-3
Ghareeb, K., Awad, W. A., Bohm, J., Zebeli, Q. (2015). Impacts of the feed contaminant deoxynivalenol on the intestine of monogastric animals: poultry and swine. J. Appl. Toxicol. 35, 327–337. doi: 10.1002/jat.3083
Harland, D. N., Garmory, H. S., Brown, K. A., Titball, R. W. (2005). An association between ATP binding cassette systems, genome sizes and lifestyles of bacteria. Res. Microbiol. 156, 434–442. doi: 10.1016/j.resmic.2004.12.002
Hassan, Y. I., He, J. W., Perilla, N., Tang, K., Karlovsky, P., Zhou, T. (2017). The enzymatic epimerization of deoxynivalenol by Devosia mutans proceeds through the formation of 3-keto-DON intermediate. Sci. Rep. 7, 6929. doi: 10.1038/s41598-017-07319-0
Hassan, Y. I., Watts, C., Li, X. Z., Zhou, T. (2015). A novel Peptide-binding motifs inference approach to understand deoxynivalenol molecular toxicity. Toxins (Basel) 7, 1989–2005. doi: 10.3390/toxins7061989
Hassan, Y. I., Zhu, H., Zhu, Y., Zhou, T. (2016). Beyond ribosomal binding: the increased polarity and aberrant molecular interactions of 3-epi-deoxynivalenol. Toxins (Basel) 8(9): pii: E261. doi: 10.3390/toxins8090261
He, J. W., Bondy, G. S., Zhou, T., Caldwell, D., Boland, G. J., Scott, P. M. (2015a). Toxicology of 3-epi-deoxynivalenol, a deoxynivalenol-transformation product by Devosia Mutans, 17–2-E-8, Food Chem. Toxicol. 84:250–259. doi: 10.1016/j.fct.2015.09.003
He, J. W., Hassan, Y. I., Perilla, N., Li, X. Z., Boland, G. J., Zhou, T. (2016). Bacterial epimerization as a route for deoxynivalenol detoxification: the influence of growth and environmental conditions. Front. Microbiol. 7, 572. doi: 10.3389/fmicb.2016.00572
He, J. W., Yang, R., Zhou, T., Boland, G. J., Scott, P. M., Bondy, G. S. (2015b). An epimer of deoxynivalenol: purification and structure identification of 3-epi-deoxynivalenol. Food Addit. Contam. Part A Chem. Anal. Control Expo. Risk Assess. 32, 1523–1530. doi: 10.1080/19440049.2015.1072771
Ishikawa, A. T., Weese, J. S., Bracarense, A.P.F.R.L., Alfieri, A. A., Oliveira, G. G., Kawamura, O., et al. (2017). Single aflatoxin B1 exposure induces changes in gut microbiota community in C57Bl/6 mice. World Mycotoxin. J. 10, 249–254. doi: 10.3920/WMJ2017.2190
Liao, Y., Peng, Z., Chen, L., Nussler, A. K., Liu, L., Yang, W. (2018). Deoxynivalenol, gut microbiota and immunotoxicity: a potential approach? Food Chem. Toxicol. 112, 342–354. doi: 10.1016/j.fct.2018.01.013
Livak, K. J., Schmittgen, T. D. (2001). Analysis of relative gene expression data using real-time quantitative PCR and the 2(-Delta Delta C(T)) Method. Methods 25, 402–408. doi: 10.1006/meth.2001.1262
Ng, S. C., Lam, E. F., Lam, T. T., Chan, Y., Law, W., Tse, P. C., et al. (2013). Effect of probiotic bacteria on the intestinal microbiota in irritable bowel syndrome. J. Gastroenterol. Hepatol. 28, 1624–1631. doi: 10.1111/jgh.12306
Niederweis, M. (2003). Mycobacterial porins–new channel proteins in unique outer membranes. Mol. Microbiol. 49, 1167–1177. doi: 10.1046/j.1365-2958.2003.03662.x
Nikaido, H. (2009). Multidrug resistance in bacteria. Annu. Rev. Biochem. 78, 119–146. doi: 10.1146/annurev.biochem.78.082907.145923
Nikaido, H., Pages, J. M. (2012). Broad-specificity efflux pumps and their role in multidrug resistance of Gram-negative bacteria. FEMS Microbiol. Rev. 36, 340–363. doi: 10.1111/j.1574-6976.2011.00290.x
Pan, X., Whitten, D. A., Wilkerson, C. G., Pestka, J. J. (2014). Dynamic changes in ribosome-associated proteome and phosphoproteome during deoxynivalenol-induced translation inhibition and ribotoxic stress. Toxicol. Sci. 138, 217–233. doi: 10.1093/toxsci/kft270
Park, J., Lee, H. H., Youn, K., Kim, S., Jung, B., Lee, J., et al. (2014). Transcriptome analyses to understand effects of the fusarium deoxynivalenol and nivalenol mycotoxins on Escherichia coli. J. Biotechnol. 192 Pt A, 231–239. doi: 10.1016/j.jbiotec.2014.10.018
Pasternak, C. A., Bashford, C. L., Menestrina, G. (1989). Mechanisms of attack and defence at the cell surface: the use of phospholipid bilayers as models for cell membrane. Biosci. Rep. 9, 503–507. doi: 10.1007/BF01117054
Payros, D., Dobrindt, U., Martin, P., Secher, T., Bracarense, A. P., Boury, M., et al. (2017). The food contaminant deoxynivalenol exacerbates the genotoxicity of gut microbiota. MBio 8(2), e00007-17. doi: 10.1128/mBio.00007-17
Peng, Z., Chen, L., Nussler, A. K., Liu, L., Yang, W. (2017a). Current sights for mechanisms of deoxynivalenol-induced hepatotoxicity and prospective views for future scientific research: a mini review. J. Appl. Toxicol. 37, 518–529. doi: 10.1002/jat.3428
Peng, Z., Chen, L., Xiao, J., Zhou, X., Nussler, A. K., Liu, L., et al. (2017b). Review of mechanisms of deoxynivalenol-induced anorexia: the role of gut microbiota. J. Appl. Toxicol. 37, 1021–1029. doi: 10.1002/jat.3475
Piddock, L. J. (2006). Multidrug-resistance efflux pumps—not just for resistance. Nat. Rev. Microbiol. 4, 629–636. doi: 10.1038/nrmicro1464
Piotrowska, M., Slizewska, K., Nowak, A., Zielonka, L., Zakowska, Z., Gajecka, M., et al. (2014). The effect of experimental fusarium mycotoxicosis on microbiota diversity in porcine ascending colon contents. Toxins (Basel) 6, 2064–2081. doi: 10.3390/toxins6072064
Robert, H., Payros, D., Pinton, P., Theodorou, V., Mercier-Bonin, M., Oswald, I. P. (2017). Impact of mycotoxins on the intestine: are mucus and microbiota new targets? J. Toxicol. Environ. Health B. Crit. Rev. 20, 249–275. doi: 10.1080/10937404.2017.1326071
Robinson, D. G., Wang, J. Y., Storey, J. D. (2015). A nested parallel experiment demonstrates differences in intensity-dependence between RNA-seq and microarrays. Nucleic Acids Res. 43, e131. doi: 10.1093/nar/gkv636
Sato, I., Ito, M., Ishizaka, M., Ikunaga, Y., Sato, Y., Yoshida, S., et al. (2012). Thirteen novel deoxynivalenol-degrading bacteria are classified within two genera with distinct degradation mechanisms. FEMS Microbiol. Lett. 327, 110–117. doi: 10.1111/j.1574-6968.2011.02461.x
Sharma, R., Awasthi, Y. C., Yang, Y., Sharma, A., Singhal, S. S., Awasthi, S. (2003). Energy dependent transport of xenobiotics and its relevance to multidrug resistance. Curr. Cancer Drug Targets 3, 89–107. doi: 10.2174/1568009033482047
Sinha, R. C., Savard, M. E. (1997). Concentration of deoxynivalenol in single kernels and various tissues of wheat heads. Can. J. Plant Pathol. 19, 8–12. doi: 10.1080/07060669709500578
Suzuki, T., Iwahashi, Y. (2015). Low toxicity of deoxynivalenol-3-glucoside in microbial cells. Toxins (Basel) 7, 187–200. doi: 10.3390/toxins7010187
Takakura, N., Nesslany, F., Fessard, V., Le Hegarat, L. (2014). Absence of in vitro genotoxicity potential of the mycotoxin deoxynivalenol in bacteria and in human TK6 and HepaRG cell lines. Food Chem. Toxicol. 66, 113–121. doi: 10.1016/j.fct.2014.01.029
Walter, S., Kahla, A., Arunachalam, C., Perochon, A., Khan, M. R., Scofield, S. R., et al. (2015). A wheat ABC transporter contributes to both grain formation and mycotoxin tolerance. J. Exp. Bot. 66, 2583–2593. doi: 10.1093/jxb/erv048
Wendlandt, S., Shen, J., Kadlec, K., Wang, Y., Li, B., Zhang, W. J., et al. (2015). Multidrug resistance genes in staphylococci from animals that confer resistance to critically and highly important antimicrobial agents in human medicine. Trends Microbiol. 23, 44–54. doi: 10.1016/j.tim.2014.10.002
Yin, X., Zhu, Z., Zhou, Y., Ji, F., Yao, Z., Shi, J., et al. (2016). Complete genome sequence of deoxynivalenol-degrading bacterium Devosia sp. strain A16. J. Biotechnol. 218, 21–22. doi: 10.1016/j.jbiotec.2015.11.016
Keywords: Deoxynivalenol, Devosia, RNA_Seq, prokaryotes, detoxification
Citation: Hassan YI, He JW, Lepp D and Zhou T (2019) Understanding the Bacterial Response to Mycotoxins: The Transcriptomic Analysis of Deoxynivalenol-Induced Changes in Devosia mutans 17-2-E-8. Front. Pharmacol. 10:1098. doi: 10.3389/fphar.2019.01098
Received: 31 October 2018; Accepted: 26 August 2019;
Published: 14 November 2019.
Edited by:
Ursula Gundert-Remy, Charité Medical University of Berlin, GermanyReviewed by:
Michael Rychlik, Technical University of Munich, GermanyCopyright 2019 Her Majesty the Queen in Right of Canada, as represented by the Minister of Agriculture and Agri-Food Canada. This is an open-access article distributed under the terms of the Creative Commons Attribution License (CC BY). The use, distribution or reproduction in other forums is permitted, provided the original author(s) and the copyright owner(s) are credited and that the original publication in this journal is cited, in accordance with accepted academic practice. No use, distribution or reproduction is permitted which does not comply with these terms.
*Correspondence: Ting Zhou, VGluZy5aaG91QGNhbmFkYS5jYQ==
Disclaimer: All claims expressed in this article are solely those of the authors and do not necessarily represent those of their affiliated organizations, or those of the publisher, the editors and the reviewers. Any product that may be evaluated in this article or claim that may be made by its manufacturer is not guaranteed or endorsed by the publisher.
Research integrity at Frontiers
Learn more about the work of our research integrity team to safeguard the quality of each article we publish.