- 1Professor Emeritus of Pediatrics, Università degli Studi di Milano, Milan, Italy
- 2Department of Surgical and Biomedical Sciences, Pediatric Clinic, Università degli Studi di Perugia, Perugia, Italy
Bacteriophages (BPs) are viruses that can infect and kill bacteria without any negative effect on human or animal cells. For this reason, it is supposed that they can be used, alone or in combination with antibiotics, to treat bacterial infections. In this narrative review, the advantages and limitations of BPs for use in humans will be discussed. PubMed was used to search for all of the studies published from January 2008 to December 2018 using the key words: “BPs” or “phages” and “bacterial infection” or “antibiotic” or “infectious diseases.” More than 100 articles were found, but only those published in English or providing evidence-based data were included in the evaluation. Literature review showed that the rapid rise of multi-drug-resistant bacteria worldwide coupled with a decline in the development and production of novel antibacterial agents have led scientists to consider BPs for treatment of bacterial infection. Use of BPs to overcome the problem of increasing bacterial resistance to antibiotics is attractive, and some research data seem to indicate that it might be a rational measure. However, present knowledge seems insufficient to allow the use of BPs for this purpose. To date, the problem of how to prepare the formulations for clinical use and how to avoid or limit the risk of emergence of bacterial resistance through the transmission of genetic material are not completely solved problems. Further studies specifically devoted to solve these problems are needed before BPs can be used in humans.
Introduction
Bacteriophages (BPs) are viruses that can infect and kill bacteria without any negative effect on human or animal cells. For this reason, it is supposed that they can be used, alone or in combination with antibiotics, to treat bacterial infections (Domingo-Calap and Delgado-Martínez, 2018). Administration of BPs for this purpose dates to about a century ago, mainly based on the studies of a French researcher, Felix d’Herelle. Due to his collaboration with his Georgian colleagues, BP therapy was largely used in the Soviet Union in patients of any age suffering from a wide range of diseases. The results were considered very satisfactory and were published in several reports (d’Herelle, 1931; Summers, 2001). However, most of these publications were written in Russian and did not reach the Western world. Moreover, when some results were translated and diffused among English-speaking scientists, they were seen with skepticism, as most of the clinical trials did not follow the international standards (Chanishvili, 2001). The increasing availability of safe and effective antimicrobial drugs after the Second World War has further contributed to the low esteem in which BPs were held in the West until the 1980s (Chanishvili, 2016). However, mainly because most antibiotics were not available, BPs continued to be used in Russia and in Eastern Europe, particularly in those countries previously included in the Soviet Union (Abedon et al., 2011).
The situation has radically changed in the last 30 years, especially in the last decade, as a result of the rapid rise of multi-drug-resistant bacteria worldwide coupled with a decline in the development and production of novel antibacterial agents (Perros, 2015; World Health Organization, 2018). The difficulties in the treatment of many life-threatening bacterial infections have led scientists to reconsider BPs. Several studies regarding BP use in vitro, in experimental animals and in humans have been performed in both the United States and Europe. The new interest in BPs is exemplified by their wide use in food production and cattle raising and, regarding therapy in humans, by the activation of a project funded by the European Commission to evaluate phage cocktails to treat burn injuries infected by Escherichia coli and Pseudomonas aeruginosa (European Commission, 2018) and by the existence in the ClinicalTrials.gov website of 38 clinical studies on a wide range of diseases and conditions in which BPs are tested (U.S. National Library of Medicine, 2018). However, several problems concerning the use of BP to treat infections are not solved, and this explains why no preparation for human use has been licensed by the European Medicine Agency or the Food and Drug Administration. In this narrative review, the advantages and limitations of BPs for use in humans will be discussed. PubMed was used to search for all of the studies published from January 2008 to December 2018 using the key words: “BPs” or “phages” and “bacterial infection” or “antibiotic” or “infectious diseases”. More than 100 articles were found, but only those published in English or providing evidence-based data were included in the evaluation.
Biology of Bacteriophages (BPs)
Bacteriophages are the most common biological entity. They can be found in soil and seawater, oceanic and terrestrial surfaces and extreme environments, such as those characterized by very high or very low temperatures. Moreover, they have been detected in hospitals, in wastewater and where bacteria can live, including animal and human tissues (Clokie et al., 2011). Several thousand BPs have been described. They have been classified according to their morphological characteristics, their nucleic acid content, the site where they can mostly be found, and the bacterial species that they can kill (Table 1).
The most frequently used classification divides tail components of BPs according to their biological cycle (Fauquet and Pringle, 2000; Van Regenmortel, 2007). Two groups are identified, lytic or virulent and lysogenic or temperate BPs. Their biological cycle implies attachment to and invasion of the bacterium. However, to initiate binding, BP structures have to match strain-specific variants of bacterial receptors. Considering the large and continuous mutations of both BP and bacterial structures, frequently mediated by BPs themselves, a single BP can infect a limited number of bacterial strains and, in several cases, only a single strain (Young, 2013). This explains the absolute specificity of BP activity. Once the BP enters the cell, the bacterial synthetic machinery is redirected to the production of viral genome and proteins. Finally, assembly and packing of BPs occurs, and cells are lysed with the release of new virions that can infect other bacterial cells (Young, 2013). However, the burst size can significantly vary according to the phage characteristics, the pathogens against which the BP is directed, and the environments in which the BP-pathogen relationship occurs (Weinbauer, 2004). In contrast, the lysogenic cycle is characterized by integration of viral genetic material into the host genome and, when cell divides, the transmission of virus chromosomes to daughter cells. Only exceptionally can the viral genome be detached from the bacterial DNA and enter the lytic stage (Salmond and Fineran, 2015). Due to their specific ability to kill bacteria, only lytic BPs are used to treat bacterial infections.
Potential Advantage of Bacteriophage (BP) Use to Treat Bacterial Infections
Theoretically, there are no bacteria that cannot be lysed by at least one BP. In this regard, BPs are significantly more effective than antibiotics, as, although some antimicrobial drugs have a very large spectrum of activity, an antibiotic able to kill all the bacterial species does not exist. However, the most attractive characteristic of BPs is their specificity of action, i.e., their ability to kill only the pathogen that they can recognize.
They have a very narrow spectrum of activity, which avoids the most important problem strictly related to the antibiotic administration, i.e., the influence on the entire microbiome with elimination of potentially beneficial bacteria, the overgrowth of secondary pathogens and the emergence of resistant bacteria (Domingo-Calap and Delgado-Martínez, 2018). Use of BPs without modification of the microbiota has been reported by several studies in both animals and humans. In mice, oral administration of four T4-like BPs effective against diarrhea-associated E. coli did not lead to any collateral damage of non-pathogenic bacteria of the same species (Chibani-Chennoufi et al., 2004). In humans, data confirming the specificity of BP action were shown in the study conducted by Sarker et al. (2012). These authors administered for 2 days an oral cocktail of nine T4-like E. coli BPs to 15 healthy adults. After a wash-out of 5 days, even though the given BPs could be detected in the feces of almost all treated subjects, no modification of gut microbiota composition was evidenced.
In comparison to antibiotics, BPs are supposed to have several other advantages. It is thought that BPs are significantly safer and better tolerated, as they replicate only in the target bacterium but cannot infect mammalian cells. This conclusion seems supported by all the experiences gathered in the past in Eastern Europe and all the studies carried out more recently in experimental animals and humans, which have not reported significant adverse events following BP administration (Kakasis and Panitsa, 2018). Moreover, administration is easier, as BPs do not need repeated administrations shortly after one another over several days, as is commonly required for antibiotics because they can remain in the human body for relatively prolonged periods of time, i.e., up to several days (Bogovazova et al., 1991, 1992). In general, very few doses are needed because of the increase in BP concentration in the site of infection after the initial administration. Contrarily to antibiotics, their effect is limited to the site of infection that can be reached, even when bacteria are situated in a body organ or system in which antimicrobials can hardly penetrate. A lytic phage, EC200(PP), active against S242, a fatal neonatal meningitis E. coli strain, was evaluated in models of meningitis with 100% fatality. Though low titres of the BP were detected in the central nervous system, treatment 1 and 7 h post-infection rescued 100% of pups (Pouillot et al., 2012).
Using the new cost-effective, large-scale DNA sequencing and DNA synthesis technologies, BPs can be engineered to be able to overcome some limitations of antibiotic treatment. A good example of this is given by the evidence that BPs can disperse biofilm, a structure that makes infections difficult to eradicate with standard antibiotic therapy even if bacteria are sensitive to the administered drug. In an in vitro study, Lu and Collins engineered a BP affective against an E. coli producing biofilm to express a biofilm-degrading enzyme (Lu and Collins, 2007). A simultaneous attack to the bacterial cells and the biofilm matrix was possible. The results were very encouraging, as the engineered BP reduced bacterial biofilm cell count by approximately 99.9%. Moreover, BP genetic modifications can help to fight bacterial resistance to antibiotics. Edgar et al. (2012) introduced in lysogenic phages the genes rpsL and gyrA, which confer sensitivity in a dominant fashion to two antibiotics, streptomycin and nalidixic acid, respectively. They found that, after engineering, the minimal inhibitory concentrations of bacterial strains previously defined resistant to these drugs were significantly reduced to levels usually found in sensitive pathogens.
Finally, the use of BPs might be less expensive than that of antibiotics whose targets are multidrug-resistant pathogens. In a small group of patients suffering from methicillin-resistant Staphylococcus aureus infection, Miedzybrodzki et al. (2007) found that use of BPs significantly reduced healthcare costs.
Table 2 summarizes the main advantages of BPs in comparison to antibiotics.
Use of Bacteriophages (BPs) for Therapy
Table 3 shows the potential clinical applications of BPs observed in studies carried out in animals and humans.
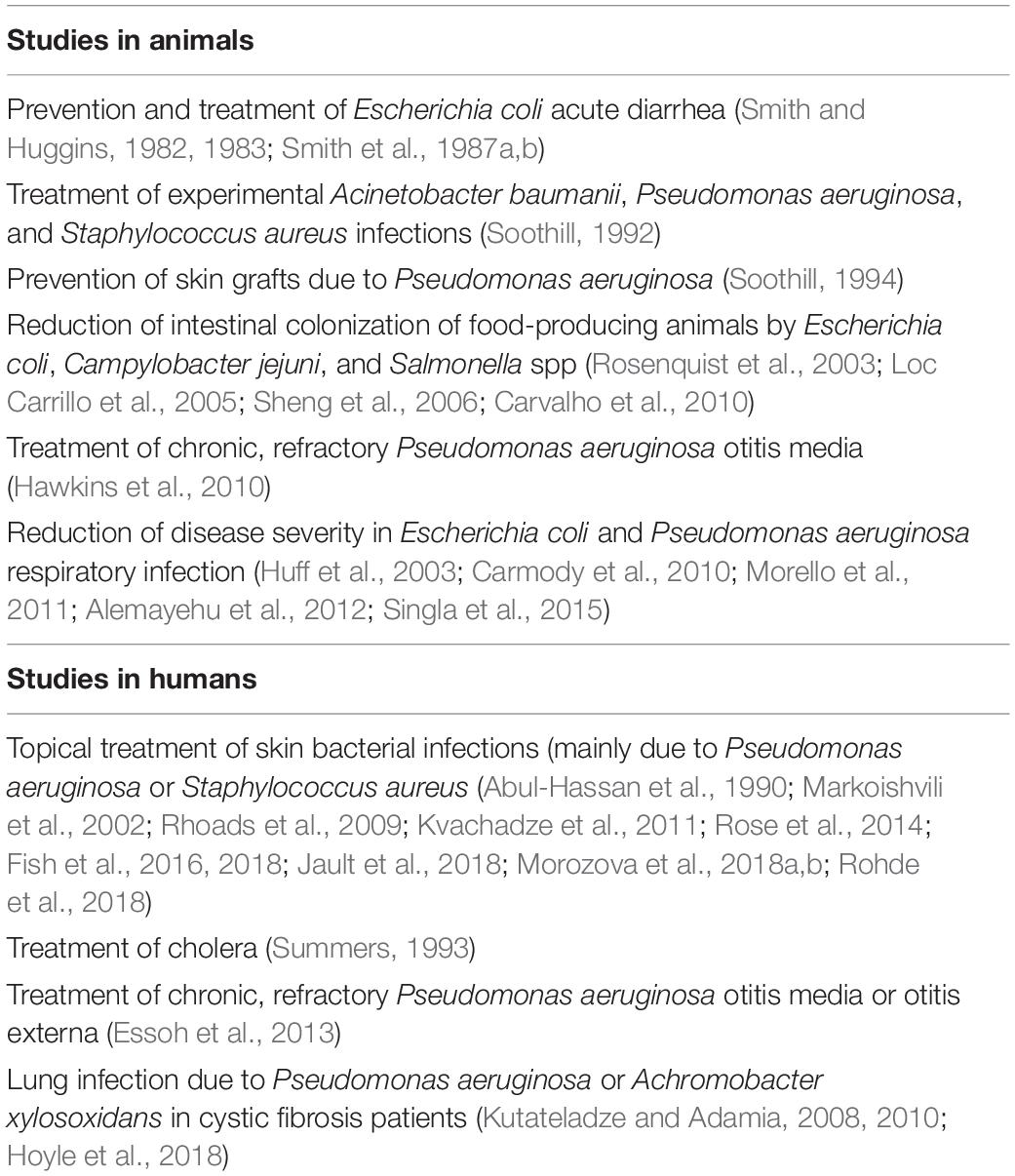
Table 3. Potential clinical applications of BPs observed in studies carried out in animals and humans (references in brackets).
Studies in Animals
The most important studies of BPs carried out in animals in the Western world date back to the 1980s, when Smith et al. made several attempts to evaluate whether the use of specific BPs against E. coli could prevent and treat acute diarrhea due to these pathogens (Smith and Huggins, 1982, 1983; Smith et al., 1987a, b). The results were encouraging, as infections due to oral inoculation of a mixture of 6 pathogenic E. coli strains were controlled by the administration of a single dose of a 105 pool of the six BPs active against them. A lower dose (102) was enough to prevent diarrhea. Further experimental evidence of the benefits deriving from the use of BPs was reported some years later by Soothill, who showed that specific BPs could control experimental Acinetobacter baumanii, P. aeruginosa, and S. aureus infections in mice (Soothill, 1992). Moreover, the same authors showed that BP administration prevented the destruction of skin grafts by P. aeruginosa in guinea pigs (Soothill, 1994). More recent studies have found that BP treatment of food-producing animals is associated with a reduction of the risk of contamination of the resulting food products during processing (Rosenquist et al., 2003; Loc Carrillo et al., 2005; Sheng et al., 2006; Carvalho et al., 2010). A 1- or 2-log reduction in the number of pathogens shed in feces of the slaughtered animals could reduce the risk by 45–75%, respectively. Moreover, several reports of reduced intestinal colonization of food-producing chickens and ruminants by E. coli, Campylobacter jejuni, and Salmonella spp. after BP administration have been published, and this has led to the wide use of BPs to prevent food contamination (Rosenquist et al., 2003; Loc Carrillo et al., 2005; Sheng et al., 2006; Carvalho et al., 2010).
Finally, several attempts to use BPs to treat respiratory infections of animals have been made. For instance, Hawkins et al. (2010) used BPs to treat 10 dogs with chronic, refractory P. aeruginosa otitis media. In each animal, a single dose of a topical preparation containing approximately 1 × 105 plaque-forming units (PFU) of each of 6 BPs, specific for these pathogens, was instilled directly into the auditory canal of one ear. Before therapy and 48 h later, conditions of the affected ear were assessed through the evaluation of a clinical score based on 5 indicators, and aural swabs were taken to measure BP and P. aeruginosa concentrations. Treatment was very effective and safe. Mean score was reduced by 30.1% (range 7.7–56.3%, p < 0.0001), and pathogen mean count declined by 67% [95% confidence interval (CI) 52–82, p < 0.001]. Improvement was accompanied by a significant increase in BP/swab counts (mean 5.9 × 107 PFU/swab, range 1.7 × 106 to 2.6 × 108 PFU/swab). In all the animals, BP counts were higher than those administered (mean rise: 99.1-fold, range 2.8–433.3-fold), showing significant viral replication. Treatment did not cause related inflammation or other adverse events, either local or systemic. Moreover, administration of BPs with an aerosol spray reduced the mortality of broiler chickens with E. coli respiratory infection (Huff et al., 2003) and limited the clinical problems of mice infected with P. aeruginosa (Morello et al., 2011; Alemayehu et al., 2012), Klebsiella pneumoniae (Singla et al., 2015), or Burkholderia cenocepacia (Carmody et al., 2010).
Studies in Humans
Bacteriophages have been used to treat bacterial infections involving different body sites with various preparations. The greatest number of these studies have investigated the use of BPs for topical treatment of skin bacterial infections. Beneficial effects of BP administration for treatment of localized infections in wounds, burns, and trophic ulcers, including diabetic foot ulcers, were reported during and after the Second World War in Eastern Europe (Morozova et al., 2018b). Similar results were later shown by a number of studies published in Russian with abstracts in English (Rohde et al., 2018) and finally confirmed, although with a few exceptions (Rose et al., 2014), by a series of trials carried out in the Western world and published in English in peer-reviewed journals (Markoishvili et al., 2002; Rhoads et al., 2009; Kvachadze et al., 2011; Fish et al., 2016, 2018; Jault et al., 2018; Morozova et al., 2018a).
The topical application of BPs cured burns infected by multidrug-resistant P. aeruginosa and allowed successful skin grafting in 18 out of 30 adult patients in whom this therapy was used (Abul-Hassan et al., 1990). Positive results were reported in several studies that used BPs in patients with infected ulcers (Rhoads et al., 2009; Fish et al., 2016, 2018; Morozova et al., 2018a), especially when BPs were included in a biodegradable wound dressing that released active antimicrobials, including BPs, for a long time (Kvachadze et al., 2011). For instance, patients with diabetic foot ulcers infected by S. aureus strains benefited from the administration of the Staphylococcus BP Sb-1 (Jault et al., 2018), even when BP administration therapy was the only antimicrobial treatment (Fish et al., 2018; Morozova et al., 2018b). Other BPs, specific for different bacterial pathogens, were effective in a number of diabetic foot ulcer infections previously treated unsuccessfully with antibiotics (Rhoads et al., 2009; Fish et al., 2016, 2018; Morozova et al., 2018a).
However, the positive effects of topical use of BPs to treat bacterial skin infections are partly questioned by the results of the study by Jault et al. (2018) the only randomized clinical trial carried out to evaluate impact of BPs on skin infections. These authors randomly assigned adult patients with a burn wound clinically infected with P. aeruginosa to receive a cocktail of 12 BPs (PP1131; 1 × 106 PFU/mL) or standard of care (1% sulfadiazine silver emulsion cream), both administered topically, once a day for 7 days, with 14 days of follow-up. Thirteen patients received BPs and 14 the standard therapy. Unfortunately, although BPs had an effect, the primary endpoint of the study, i.e., the median time to sustained reduction of bacterial burden, was reached significantly more slowly with BPs than with sulfadiazine cream (144 h vs. 47 h, p = 0.018). This can be explained by the evidence that the BP preparation was not stable, and patients received a 1,000-fold to 10,000-fold lower dose of active BPs than initially prescribed.
Oral administration of BPs was effective in prevention and treatment of cholera, but no randomized controlled trials enrolling patients with this disease have been conducted (Summers, 1993). The results on E. coli diarrhea have been partially disappointing. Studies carried out in both adults and children have found that 2 BP cocktails specifically active against some E. coli strains were safe and well tolerated and could be used to treat diarrhea due to these pathogens (Sarker et al., 2012; McCallin et al., 2013). The first contained eleven T4-like phages (AB2, 4, 6, 11, 46, 50, 55; JS34, 37, 98, D1.4) (Bourdin et al., 2014). The second, a Russian preparation, consisted of at least 17 different phage types, including T7 phage (McCallin et al., 2013). However, a prospective, randomized, placebo-controlled, parallel-group clinical trial carried out in Bangladesh in children aged 6–24 months did not find any positive effect on diarrhea evolution. With both preparations, no amelioration in quantitative diarrhea parameters over standard care was shown (Sarker et al., 2016). Moreover, an increase in gut content of Streptococcus gallolyticus and Streptococcus salivarius species groups strictly related to the quantitative diarrhea outcome was evidenced. These results suggested a potential modification of gut microbiota. The failure was explained by the evidence that, despite a careful selection of cases, only 60% of the 120 enrolled patients harbored an E. coli strain in the stool, as well as by the poor replication of BPs, as the BP content of the gut was never higher than the administered doses (Sarker et al., 2016).
Bacteriophages were also used to treat respiratory tract infections. Wright et al. (2009) conducted a randomized, double-blind, placebo-controlled phase I/II clinical trial administering a preparation containing 6 BPs active against P. aeruginosa or placebo to a group of 24 adult patients suffering from chronic, refractory aural discharge due to previous mastoid surgery, chronic perforations, or longstanding otitis externa. Only patients with P. aeruginosa infection due to strains susceptible to the used BPs were enrolled. BPs or placebo was instilled in the ear canal and each patient was re-examined at days 7, 21, and 42 after treatment. Relative to baseline, clinical improvement was more common among treated patients. Moreover, the deterioration seen in some placebo-receiving subjects was not evidenced in patients given BP. The reduction in P. aeruginosa concentration was significantly higher in treated patients than in controls at days 21 and 42 (p = 0.009 and p = 0.016, respectively), with a strict relationship between the disappearance of the pathogen and improvement in otoscopy. The mean recovery of BP in treated patients at the three study visits exceeded 200 times the input dose. No treatment-related adverse event was reported.
A number of in vitro studies have suggested that BPs could be effective in treating cystic fibrosis (CF) patients. Essoh et al. (2013) purified 6 BPs from a Russian cocktail, including 4 genera effective against P. aeruginosa, and tested them against the CF sputum. It was shown that 33 out of 47 P. aeruginosa strains were lysed. Saussereau et al. (2014) showed that the addition of a cocktail of 10 BPs infecting P. aeruginosa to sputum samples of CF patients led to a significant reduction of pathogens in comparison to controls (p = 0.024). In 5.8% of the cases, these results were associated with a relevant increase in BP concentration. In both these studies, BP therapy overcame the obstacles of the CF environment, mainly the mucus characteristics and the biofilm inhibition (European Commission, 2018). However, studies in humans are few and limited to case reports. No randomized, placebo-controlled trial is presently available. Single cases of CF patients with chronic, otherwise untreatable, lung infection due to P. aeruginosa (Kutateladze and Adamia, 2008) or Achromobacter xylosoxidans (Hoyle et al., 2018) that received BPs per aerosol with good results have been described. Moreover, aerosolized BPs were administered to 8 CF patients together with the conventional antibiotic treatment for 6–10 days. Both P. aeruginosa concentration in the respiratory secretions and antibiotic need were reduced (Kutateladze and Adamia, 2010). However, definitive conclusions on the relevance of BP treatment in patients with CF cannot be drawn.
Factors That Can Limit Bacteriophage (BP) Use and Development of New Preparations for Therapy
Data regarding the use of BPs to treat bacterial disease in humans are few, sometimes conflicting or negative and almost always collected in trials that are not randomized and placebo controlled. Moreover, the preparation of BPs for clinical use is difficult, and not all the problems strictly related with BP biology have been solved. Table 4 describes the main limitations associated with BP use.
Problems Related to the Choice of the BPs Effective for the Various Bacterial Infections
Identification of a therapeutic BP is very complicated. Isolation of BPs, generally from wastewater and sewage, is the first step and is per se relatively easy, although with differences between the various bacterial pathogens. For example, it is significantly easier if P. aeruginosa rather than S. aureus is the target of the BP (Mattila et al., 2015). However, before a BP is identified as a potential therapeutic agent, it has to be demonstrated that it is specific for a given bacterial strain. This is a relatively complicated problem, as evidence of lytic capacity of a BP can vary according to the interrelationships between the BP and bacterium and their modification with time together with the dose of virus used for the test. Moreover, the BP genome must be sequenced and not contain integrase genes, as in the lysogenic type, antibiotic resistant genes (ARG), genes for phage-encoded toxins or genes for other bacterial virulence factors. Finally, problems related to the formulation and stabilization of pharmaceutical preparations for clinical use are far from solved (Vandenheuvel et al., 2015). In this regard, it has to be highlighted that studies seem to indicate that stability of preparations for clinical use is strictly BP dependent and stabilization strategies should be optimized for each BP separately (Clark, 1962; Merabishvili et al., 2013). This can lead to costly and time-consuming clinical trials that could discourage the pharmaceutical industry from starting research and production of preparations for human use.
The Risk of Emergence of Bacterial Resistant to Bacteriophages (BPs)
Emergence of bacterial resistance against BPs is potentially possible, as bacteria possess or can develop several mechanisms to prevent viral infections. Among these are hiding, change or loss of receptor, secretion of substances that prevent phage adhesion to the bacterial pathogen, activation of measures for blocking phage DNA injection into the cell and inhibition of phage replication and release (Seed, 2015). Alteration or loss of receptor for membrane protein modifications has been demonstrated for E. coli (Riede and Eschbach, 1986), S. aureus (Nordström and Forsgren, 1974), Bordetella bronchiseptica (Liu et al., 2002), and Vibrio cholerae (Seed et al., 2012). Secretion of extracellular polymeric substances and glycoconjugates has been described for Pseudomonas spp. and Enterobacteriaceae (Drulis-Kawa et al., 2012), respectively. Development of bacterial resistance to BPs can be reduced with the use of BP cocktails, with the administration of a higher initial BP inoculum or the association with antibiotics. If BPs kill pathogens faster than they can replicate, a high inoculum is associated with a lower risk of development of BP-resistant bacteria. However, all these findings indicate that selection of a therapeutic BP must take into account the ability of each virus to induce bacterial resistance and the amount needed to avoid bacterial resistance development (Torres-Barceló, 2018a).
Risk That Bacteriophages (BPs) May Contribute to the Development of Antibiotic Resistance
Lysogenic phages incorporate their DNA into the bacterial genome. Consequently, they might be vehicles for horizontal exchange of genetic material and play a role in the diffusion of antibiotic resistance genes (ARGs). Theoretically, due to transduction, new microbes or even more resistant bacteria can develop (O’Shea and Boyd, 2002; Brabban et al., 2005; Maiques et al., 2007). However, the real contribution of phages to the diffusion of ARGs is not precisely defined. Most of the studies specifically planned to measure how often phages encode ARGs have suggested that these viruses are reservoirs of ARGs. Genes such as blaTEM (resistance to β-lactams), qnrS (reduced susceptibility to fluoroquinolones), ermB (resistance to macrolides), sulI (resistance to sulphonamides), and tetW (resistance to tetracyclines) have been found in the virome of activated sludge and environmental water samples (Parsley et al., 2010; Marti et al., 2014; Rodriguez-Mozaz et al., 2015; Subirats et al., 2016) and in the phage DNA fraction isolated from the intestinal mucus of wild freshwater fish species (Marti et al., 2018) and human fecal samples (Quirós et al., 2014). Moreover, diffusion of ARGs can be significantly favored by the presence in the environment of phage inducers, i.e., substances able to induce the expression of prophage gene products or lead to the excision and spread of temperate BPs. Some antibiotics, such as fluoroquinolones and some anticoagulants, are the most important (Torres-Barceló, 2018b). Treatment of wastewater samples with EDTA or sodium citrate activates the lytic cycle of lysogenic phages and leads to the generation of new phage particles, bacterial lyses, phage release outside the cell, and infection of a greater number of bacteria (Colomer-Lluch et al., 2014).
Finally, great numbers of phages carrying genes associated with antibiotic resistance have been detected in secretions and tissues of patients who suffer from recurrent infections due to antibiotic resistant pathogens and had been previously repeatedly treated with antimicrobial drugs. One of the best examples in this regard is CF, as evidenced by the study conducted by Fancello et al. (2011). These authors analyzed 1,031 short sequences in the CF virome putatively encoding antibiotic resistance and identified 66 efflux pump genes, 15 fluoroquinolone resistance genes and 9 β-lactamase genes. This and similar findings led to the supposition that BPs might be vehicles for the adaptation of bacteria to the CF lung environment and for the emergence and selection of multidrug-resistant pathogens with chimeric repertoires (Rolain et al., 2011). However, a recent reevaluation of previously collected data has suggested that AGR abundance in phages was vastly overestimated and that the risk of transduction, although possible, is lower than previously thought. In several studies, conclusions were misled by the excessive bacterial DNA content of the studied samples. Moreover, inadequate approaches to detect ARGs in phage genomes have been used (Enault et al., 2017). Partially in agreement with these findings were Lekunberri et al. (2017) who carried out an analysis of 33 viromes from human feces, pig feces, raw sewage, and freshwater and marine environments . The human-associated viromes did not carry or rarely carried ARGs, while those deriving from non-human sources harbored a significantly higher prevalence of ARGs.
Reduced Activity Due to Immune System Response
Bacteriophages and their products are non-self-antigens, and it is not surprising that they can be recognized by the immune system and induce responses that can theoretically reduce the benefit of BP administration. Immune response to BPs has been demonstrated in both experimental animals and humans, although with differences according to the phage strain, the route of administration and the prior exposure. In animals, BPs were taken up by phagocytic cells a few minutes after the administration and might be destroyed by these cells within 2 h (Kaźmierczak et al., 2014). Moreover, examining the survival of T7 BP in the blood of healthy and immunocompromised mice, it was shown that, whereas in animals with severe combined immunodeficiency, BP titres were stable for a long time, in healthy mice 99% of BPs were eliminated within 60 min of the injection. As phage titres remained stable in the B-cell-deficient mouse, the greatest part of phage clearance from blood seemed to be due to specific antibody production (Srivastava et al., 2004).
In humans, Dąbrowska et al. (2014) who have studied the antigenicity of the proteins forming the E. coli T4 BP head surface, have reported that specific antibodies could be detected in more than 80% of enrolled individuals, although none of them had received phage therapy . However, even if not fully demonstrated, it seems likely that the immune response evoked by phages has a marginal or no impact on the potential bacterial killing of phage administration. Bacterial lysis occurs before a specific antibody is evoked. Moreover, with some exceptions, phage administration was generally not associated with tissue damage, an increase in pro-inflammatory cytokines or increased reactive oxygen species (ROS) production (Park et al., 2014). Miernikiewicz et al. (2013) have shown that phage T4 and its head proteins given intraperitoneally to mice did not affect the production of cytokines [interleukin (IL)-1α, IL-1β, IL-2, IL-6, IL-10, IL-12 p40/p70, interferon (IFN)-γ, tumour necrosis factor (TNF)-α, monocyte chemoattractant protein (MCP-1), monokine induced by gamma (MIG), RANTES, granulocyte colony-stimulating factor (GCSF), and granulocyte-macrophage colony-stimulating factor (GM-CSF)] or ROS. Similar findings were reported by Hwang et al. (2016) who studied the safety of an E. coli phage cocktail given by mouth to rats for 4 weeks. Carmody et al. (2010) studied the efficacy of phage therapy given by intranasal inhalation in a mouse model of B. cenocepacia pulmonary infection. Bacterial density, macrophage inflammatory protein 2 (MIP-2), and TNF-α were not increased but, on the contrary, were significantly reduced in lungs of treated mice compared to untreated controls (p < 0.05). On the other hand, data collected in humans that seem to indicate that BP administration is safe seem to support that, even if present, the immune response to BPs is not clinically important.
Conclusion
Use of BPs to overcome the problem of increasing microbial resistance to antibiotics is attractive, and some research data seem to indicate that it might be a rational measure. However, present knowledge seems insufficient to allow the use of BPs for this purpose. To date, properly designed clinical trials specifically planned to evaluate BP efficacy are very few and partially negative. Moreover, the problem of how to prepare the formulations for standardized and clinical use in bacterial control, how to avoid or limit the risk of emergence of bacterial resistance and the transmission of genetic material are not completely solved problems. In addition, the mechanisms concerning coevolution between BP and bacteria are unknown. Further studies specifically devoted to solve these problems are needed before BPs can be used in humans.
Author Contributions
NP and SE contributed to writing the manuscript. ES performed the literature review. All authors approved the final submitted version of the manuscript.
Funding
This study was supported by a grant from the World Association for Infectious Disease and Immunological Disorders (WAidid_2018_04).
Conflict of Interest Statement
The authors declare that the research was conducted in the absence of any commercial or financial relationships that could be construed as a potential conflict of interest.
References
Abedon, S. T., Kuhl, S. J., Blasdel, B. G., and Kutter, E. M. (2011). Phage treatment of human infections. Bacteriophage 1, 66–85. doi: 10.4161/bact.1.2.15845
Abul-Hassan, H. S., El-Tahan, K., Massoud, B., and Gomaa, R. (1990). Bacteriophage therapy of Pseudomonas burn wound sepsis. Ann. Mediterr Burn Club 3, 262–264.
Alemayehu, D., Casey, P. G., McAuliffe, O., Guinane, C. M., Martin, J. G., Shanahan, F., et al. (2012). Bacteriophages φMR299-2 and φNH-4 can eliminate Pseudomonas aeruginosa in the murine lung and on cystic fibrosis lung airway cells. mBio 3:e00029-12. doi: 10.1128/mBio.00029-12
Bogovazova, G. G., Voroshilova, N. N., and Bondarenko, V. M. (1991). The efficacy of Klebsiella pneumoniae bacteriophage in the therapy of experimental Klebsiella infection. Zh. Mikrobiol. Epidemiol. Immunobiol. 4, 5–8.
Bogovazova, G. G., Voroshilova, N. N., Bondarenko, V. M., Gorbatkova, G. A., Afanas’eva, E. V., Kazakova, T. B., et al. (1992). Immunobiological properties and therapeutic effectiveness of preparations from Klebsiella bacteriophages. Zh. Mikrobiol. Epidemiol. Immunobiol. 3, 30–33.
Bourdin, G., Schmitt, B., and Marvin Guy, L. (2014). Amplification and purification of T4-like Escherichia coli phages for phage therapy: from laboratory to pilot scale. Appl. Environ. Microbiol. 80, 1469–1476. doi: 10.1128/AEM.03357-13
Brabban, A. D., Hite, E., and Callaway, T. R. (2005). Evolution of foodborne pathogens via temperate bacteriophage-mediated gene transfer. Foodborne Pathog. Dis. 2:287303.
Carmody, L. A., Gill, J. J., Summer, E. J., Sajjan, U. S., Gonzalez, C. F., Young, R. F., et al. (2010). Efficacy of bacteriophage therapy in a model of Burkholderia cenocepacia pulmonary infection. J. Infect. Dis. 201, 264–271. doi: 10.1086/649227
Carvalho, C. M., Gannon, B. W., Halfhide, D. E., Santos, S. B., Hayes, C. M., Roe, J. M., et al. (2010). The in vivo efficacy of two administration routes of a phage cocktail to reduce numbers of Campylobacter coli and Campylobacter jejuni in chickens. BMC Microbiol. 10:232. doi: 10.1186/1471-2180-10-232
Chanishvili, N. (2001). Phage therapy–history from Twort and d’Herelle through Soviet experience to current approaches. Adv. Virus Res. 83, 3–40. doi: 10.1016/B978-0-12-394438-2.00001-3
Chanishvili, N. (2016). Bacteriophages as therapeutic and prophylactic means: summary of the Soviet and Post Soviet experiences. Curr. Drug Deliv. 13, 309–323. doi: 10.2174/156720181303160520193946
Chibani-Chennoufi, S., Sidoti, J., Bruttin, A., Kutter, E., Sarker, S., Brüssow, H., et al. (2004). In vitro and in vivo bacteriolytic activities of Escherichia coli phages: implications for phage therapy. Antimicrob. Agents Chemother. 48, 2558–2569. doi: 10.1128/aac.48.7.2558-2569.2004
Clark, W. A. (1962). Comparison of several methods for preserving bacteriophages. Appl. Microbiol. 10, 466–471.
Clokie, M. R., Millard, A. D., Letarov, A. V., and Heaphy, S. (2011). Phages in nature. Bacteriophage 1, 31–45.
Colomer-Lluch, M., Jofre, J., and Muniesa, M. (2014). Quinolone resistance genes (qnrA and qnrS) in bacteriophage particles from wastewater samples and the effect of inducing agents on packaged antibiotic resistance genes. J. Antimicrob. Chemother. 69, 1265–1274. doi: 10.1093/jac/dkt528
Dąbrowska, K., Miernikiewicz, P., Piotrowicz, A., Hodyra, K., Owczarek, B., Lecion, D., et al. (2014). Immunogenicity studies of proteins forming the T4 phage head surface. J. Virol. 88, 12551–12557. doi: 10.1128/JVI.02043-14
d’Herelle, F. (1931). Bacteriophage as a treatment in acute medical and surgical infections. Bull. N. Y. Acad. Med. 7, 329–348.
Domingo-Calap, P., and Delgado-Martínez, J. (2018). Bacteriophages: protagonists of a post-antibiotic era. Antibiotics 7:E66. doi: 10.3390/antibiotics7030066
Drulis-Kawa, Z., Majkowska-Skrobek, G., Maciejewska, B., Delattre, A. S., and Lavigne, R. (2012). Learning from bacteriophages - advantages and limitations of phage and phage-encoded protein applications. Curr. Protein Pept. Sci. 13, 699–722. doi: 10.2174/138920312804871193
Edgar, R., Friedman, N., Molshanski-Mor, S., and Qimron, U. (2012). Reversing bacterial resistance to antibiotics by phage-mediated delivery of dominant sensitive genes. Appl. Environ. Microbiol. 78, 744–751. doi: 10.1128/AEM.05741-11
Enault, F., Briet, A., Bouteille, L., Roux, S., Sullivan, M. B., and Petit, M. A. (2017). Phages rarely encode antibiotic resistance genes: a cautionary tale for virome analyses. ISME J. 11, 237–247. doi: 10.1038/ismej.2016.90
Essoh, C., Blouin, Y., Loukou, G., Cablanmian, A., Lathro, S., Kutter, E., et al. (2013). The susceptibility of Pseudomonas aeruginosa strains from cystic fibrosis patients to bacteriophages. PLoS One 8:e60575. doi: 10.1371/journal.pone.0060575
European Commission (2018). Community Research and Development Information Service. Phagoburn Report Summary. .
Fancello, L., Desnues, C., Raoult, D., and Rolain, J. M. (2011). Bacteriophages and diffusion of genes encoding antimicrobial resistance in cystic fibrosis sputum microbiota. J. Antimicrob. Chemother. 66, 2448–2454. doi: 10.1093/jac/dkr315
Fauquet, C. M., and Pringle, C. R. (2000). Abbreviations for bacterial and fungal virus species names. Arch. Virol. 145, 197–203. doi: 10.1007/s007050050017
Fish, R., Kutter, E., Wheat, G., Blasdel, B., Kutateladze, M., and Kuhl, S. (2016). Bacteriophage treatment of intransigent diabetic toe ulcers: a case series. J. Wound Care 25, S27–S33.
Fish, R., Kutter, E., Wheat, G., Blasdel, B., Kutateladze, M., and Kuhl, S. (2018). Compassionate use of bacteriophage therapy for foot ulcer treatment as an effective step for moving towards clinical trials. Methods Mol. Biol. 1693, 159–170. doi: 10.1007/978-1-4939-7395-8_14
Hawkins, C., Harper, D., Burch, D., Anggård, E., and Soothill, J. (2010). Topical treatment of P. aeruginosa otitis of dogs with a bacteriophage mixture: a before/after clinical trial. Vet. Microbiol. 146, 309–313. doi: 10.1016/j.vetmic.2010.05.014
Hoyle, N., Zhvaniya, P., Balarjishvili, N., Bolkvadze, D., Nadareishvili, L., Nizharadze, D., et al. (2018). Phage therapy against Achromobacter xylosoxidans lung infection in a patient with cystic fibrosis: a case report. Res. Microbiol. 169, 540–542. doi: 10.1016/j.resmic.2018.05.001
Huff, W. E., Huff, G. R., Rath, N. C., Balog, J. M., and Donoghue, A. M. (2003). Evaluation of aerosol spray and intramuscular injection of bacteriophage to treat an Escherichia coli respiratory infection. Poult. Sci. 82, 1108–1112. doi: 10.1093/ps/82.7.1108
Hwang, J. Y., Kim, J. E., Song, Y. J., and Park, J. H. (2016). Safety of using Escherichia coli bacteriophages as a sanitizing agent based on inflammatory responses in rats. Food Sci. Biotechnol. 25, 355–360. doi: 10.1007/s10068-016-0050-6
Jault, P., Leclerc, T., Jennes, S., Pirnay, J. P., Que, Y. A., Resch, G., et al. (2018). Efficacy and tolerability of a cocktail of bacteriophages to treat burn wounds infected by Pseudomonas aeruginosa (PhagoBurn): a randomised, controlled, double-blind phase 1/2 trial. Lancet Infect. Dis. 19, 35–45. doi: 10.1016/S1473-3099(18)30482-1
Kakasis, A., and Panitsa, G. (2018). Bacteriophage therapy as an alternative treatment for human infections. A comprehensive review. Int. J. Antimicrob. Agents [Epub ahead of print].
Kaźmierczak, Z., Piotrowicz, A., Owczarek, B., Hodyra, K., Miernikiewicz, P., Lecion, D., et al. (2014). Molecular imaging of T4 phage in mammalian tissues and cells. Bacteriophage 4:e2836.
Kutateladze, M., and Adamia, R. (2008). Phage therapy experience at the Eliava Institute. Med. Mal. Infect. 38, 426–430. doi: 10.1016/j.medmal.2008.06.023
Kutateladze, M., and Adamia, R. (2010). Bacteriophages as potential new therapeutics to replace or supplement antibiotics. Trends Biotechnol. 28, 591–595. doi: 10.1016/j.tibtech.2010.08.001
Kvachadze, L., Balarjishvili, N., Meskhi, T., Tevdoradze, E., Skhirtladze, N., and Pataridze, T. (2011). Evaluation of lytic activity of staphylococcal bacteriophage Sb-1 against freshly isolated clinical pathogens. Microb. Biotechnol. 4, 643–650. doi: 10.1111/j.1751-7915.2011.00259.x
Lekunberri, I., Subirats, J., Borrego, C. M., and Balcázar, J. L. (2017). Exploring the contribution of bacteriophages to antibiotic resistance. Environ. Pollut. 220, 981–984. doi: 10.1016/j.envpol.2016.11.059
Liu, M., Deora, R., Doulatov, S. R., Gingery, M., Eiserling, F. A., Preston, A., et al. (2002). Reverse transcriptase-mediated tropism switching in Bordetella bacteriophage. Science 295, 2091–2094. doi: 10.1126/science.1067467
Loc Carrillo, C., Atterbury, R. J., el-Shibiny, A., Connerton, P. L., Dillon, E., Scott, A., et al. (2005). Bacteriophage therapy to reduce Campylobacter jejuni colonization of broiler chickens. Appl. Environ. Microbiol. 71, 6554–6563. doi: 10.1128/aem.71.11.6554-6563.2005
Lu, T. K., and Collins, J. J. (2007). Dispersing biofilms with engineered enzymatic bacteriophage. Proc. Natl. Acad. Sci. U.S.A. 104, 11197–11202. doi: 10.1073/pnas.0704624104
Maiques, E., Ubeda, C., Tormo, M. A., Ferrer, M. D., Lasa, I., Novick, R. P., et al. (2007). Role of staphylococcal phage and SaPI integrase in intra- and interspecies SaPI transfer. J. Bacteriol. 189, 5608–5616. doi: 10.1128/jb.00619-07
Markoishvili, K., Tsitlanadze, G., Katsarava, R., Morris, J. G., and Sulakvelidze, A. (2002). A novel sustained-release matrix based on biodegradable poly(ester amide)s and impregnated with bacteriophages and an antibiotic shows promise in management of infected venous stasis ulcers and other poorly healing wounds. Int. J. Dermatol. 41, 453–458. doi: 10.1046/j.1365-4362.2002.01451.x
Marti, E., Huerta, B., Rodríguez-Mozaz, S., Barceló, D., Marcé, R., and Balcázar, J. L. (2018). Abundance of antibiotic resistance genes and bacterial community composition in wild freshwater fish species. Chemosphere 196, 115–119. doi: 10.1016/j.chemosphere.2017.12.108
Marti, E., Variatza, E., and Balcázar, J. L. (2014). Bacteriophages as a reservoir of extended-spectrum β-lactamase and fluoroquinolone resistance genes in the environment. Clin. Microbiol. Infect. 20, O456–O459. doi: 10.1111/1469-0691.12446
Mattila, S., Ruotsalainen, P., and Jalasvuori, M. (2015). On-Demand Isolation of Bacteriophages against drug-resistant bacteria for personalized phage therapy. Front. Microbiol. 6:1271. doi: 10.3389/fmicb.2015.01271
McCallin, S., Sarker, S. A., and Barretto, C. (2013). Safety analysis of a Russian phage cocktail: from metagenomic analysis to oral application in healthy human subjects. Virology 443, 187–196. doi: 10.1016/j.virol.2013.05.022
Merabishvili, M., Vervaet, C., Pirnay, J. P., De Vos, D., Verbeken, G., Mast, J., et al. (2013). Stability of Staphylococcus aureus phage ISP after freeze-drying (lyophilization). PLoS One 8:e68797. doi: 10.1371/journal.pone.0068797
Miedzybrodzki, R., Fortuna, W., Weber-Dabrowska, B., and Górski, A. (2007). Phage therapy of staphylococcal infections (including MRSA) may be less expensive than antibiotic treatment. Postepy Hig. Med. Dosw. 61, 461–465.
Miernikiewicz, P., Da̧browska, K., Piotrowicz, A., Owczarek, B., Wojas-Turek, J., Kicielińska, J., et al. (2013). T4 phage and its head surface proteins do not stimulate inflammatory mediator production. PLoS One 8:e71036. doi: 10.1371/journal.pone.0071036
Morello, E., Saussereau, E., Maura, D., Huerre, M., Touqui, L., and Debarbieux, L. (2011). Pulmonary bacteriophage therapy on Pseudomonas aeruginosa cystic fibrosis strains: first steps towards treatment and prevention. PLoS One 6:e16963. doi: 10.1371/journal.pone.0016963
Morozova, V. V., Kozlova, Y., Ganichev, D., and Tikunova, N. (2018a). Bacteriophage treatment of infected diabetic foot ulcers. Methods Mol. Biol. 1693, 151–158. doi: 10.1007/978-1-4939-7395-8_13
Morozova, V. V., Vlassov, V. V., and Tikunova, N. V. (2018b). Applications of Bacteriophages in the treatment of localized infections in humans. Front. Microbiol. 9:1696. doi: 10.3389/fmicb.2018.01696
Nordström, K., and Forsgren, A. (1974). Effect of protein A on adsorption of bacteriophages to Staphylococcus aureus. J. Virol. 14, 198–202.
O’Shea, Y. A., and Boyd, E. F. (2002). Mobilization of the Vibrio pathogenicity island between Vibrio cholerae isolates mediated by CP-T1 generalized transduction. FEMS Microbiol. Lett. 214, 153–157. doi: 10.1016/s0378-1097(02)00880-7
Park, K., Cha, K. E., and Myung, H. (2014). Observation of inflammatory responses in mice orally fed with bacteriophage T7. J. Appl. Microbiol. 117, 627–633. doi: 10.1111/jam.12565
Parsley, L. C., Consuegra, E. J., Kakirde, K. S., Land, A. M., Harper, W. F. Jr., and Liles, M. R. (2010). Identification of diverse antimicrobial resistance determinants carried on bacterial, plasmid, or viral metagenomes from an activated sludge microbial assemblage. Appl. Environ. Microbiol. 76, 3753–3757. doi: 10.1128/AEM.03080-09
Perros, M. (2015). A sustainable model for antibiotics. Science 347, 1062–1064. doi: 10.1126/science.aaa3048
Pouillot, F., Chomton, M., Blois, H., Courroux, C., Noelig, J., Bidet, P., et al. (2012). Efficacy of bacteriophage therapy in experimental sepsis and meningitis caused by a clone O25b:H4-ST131 Escherichia coli strain producing CTX-M-15. Antimicrob. Agents Chemother. 56, 3568–3575. doi: 10.1128/AAC.06330-11
Quirós, P., Colomer-Lluch, M., Martínez-Castillo, A., Miró, E., Argente, M., and Jofre, J. (2014). Antibiotic resistance genes in the bacteriophage DNA fraction of human fecal samples. Antimicrob. Agents Chemother. 58, 606–609. doi: 10.1128/AAC.01684-13
Rhoads, D. D., Wolcott, R. D., Kuskowski, M. A., Wolcott, B. M., Ward, L. S., and Sulakvelidze, A. (2009). Bacteriophage therapy of venous leg ulcers in humans: results of a phase I safety trial. J. Wound Care 18, 240–243.
Riede, I., and Eschbach, M. L. (1986). Evidence that TraT interacts with OmpA of Escherichia coli. FEBS Lett. 205, 241–245. doi: 10.1016/0014-5793(86)80905-x
Rodriguez-Mozaz, S., Chamorro, S., Marti, E., Huerta, B., Gros, M., and Sànchez-Melsió, A. (2015). Occurrence of antibiotics and antibiotic resistance genes in hospital and urban wastewaters and their impact on the receiving river. Water Res. 69, 234–242. doi: 10.1016/j.watres.2014.11.021
Rohde, C., Resch, G., Pirnay, J. P., Blasdel, B. G., Debarbieux, L., Gelman, D., et al. (2018). Expert opinion on three phage therapy related topics: bacterial phage resistance, phage training and prophages in bacterial production strains. Viruses 10:E178. doi: 10.3390/v10040178
Rolain, J. M., Fancello, L., Desnues, C., and Raoult, D. (2011). Bacteriophages as vehicles of the resistome in cystic fibrosis. J. Antimicrob. Chemother. 66, 2444–2447. doi: 10.1093/jac/dkr318
Rose, T., Verbeken, G., Vos, D. D., Merabishvili, M., Vaneechoutte, M., Lavigne, R., et al. (2014). Experimental phage therapy of burn wound infection: difficult first steps. Int. J. Burns Trauma 4, 66–73.
Rosenquist, H., Nielsen, N. L., Sommer, H. M., Nørrung, B., and Christensen, B. B. (2003). Quantitative risk assessment of human campylobacteriosis associated with thermophilic Campylobacter species in chickens. Int. J. Food Microbiol. 83, 87–103. doi: 10.1016/s0168-1605(02)00317-3
Salmond, G. P., and Fineran, P. C. (2015). A century of the phage: past, present and future. Nat. Rev. Microbiol. 13, 777–786. doi: 10.1038/nrmicro3564
Sarker, S. A., McCallin, S., Barretto, C., Berger, B., Pittet, A. C., Sultana, S., et al. (2012). Oral T4-like phage cocktail application to healthy adult volunteers from Bangladesh. Virology 434, 222–232. doi: 10.1016/j.virol.2012.09.002
Sarker, S. A., Sultana, S., Reuteler, G., Moine, D., Descombes, P., Charton, F., et al. (2016). Oral phage therapy of acute bacterial diarrhea with two coliphage preparations: a randomized trial in children from Bangladesh. EBioMedicine 4, 124–137. doi: 10.1016/j.ebiom.2015.12.023
Saussereau, E., Vachier, I., Chiron, R., Godbert, B., Sermet, I., Dufour, N., et al. (2014). Effectiveness of bacteriophages in the sputum of cystic fibrosis patients. Clin. Microbiol. Infect. 20, 983–990. doi: 10.1111/1469-0691.12712
Seed, K. D. (2015). Battling phages: how bacteria defend against viral attack. PLoS Pathog. 11:e1004847. doi: 10.1371/journal.ppat.1004847
Seed, K. D., Faruque, S. M., Mekalanos, J. J., Calderwood, S. B., Qadri, F., and Camilli, A. (2012). Phase variable O antigen biosynthetic genes control expression of the major protective antigen and bacteriophage receptor in Vibrio cholerae O1. PLoS Pathog. 8:e1002917. doi: 10.1371/journal.ppat.1002917
Sheng, H., Knecht, H. J., Kudva, I. T., and Hovde, C. J. (2006). Application of bacteriophages to control intestinal Escherichia coli O157:H7 levels in ruminants. Appl. Environ. Microbiol. 72, 5359–5366. doi: 10.1128/aem.00099-06
Singla, S., Harjai, K., Katare, O. P., and Chhibber, S. (2015). Bacteriophage-loaded nanostructured lipid carrier: improved pharmacokinetics mediates effective resolution of Klebsiella pneumoniae-induced lobar pneumonia. J. Infect. Dis. 212, 325–334. doi: 10.1093/infdis/jiv029
Smith, H. W., and Huggins, M. B. (1982). Successful treatment of experimental Escherichia coli infections in mice using phage: its general superiority over antibiotics. J. Gen. Microbiol. 128, 307–318. doi: 10.1099/00221287-128-2-307
Smith, H. W., and Huggins, M. B. (1983). Effectiveness of phages in treating experimental Escherichia coli diarrhoea in calves, piglets and lambs. J. Gen. Microbiol. 129, 2659–2675. doi: 10.1099/00221287-129-8-2659
Smith, H. W., Huggins, M. B., and Shaw, K. M. (1987a). Factors influencing the survival and multiplication of bacteriophages in calves and in their environment. J. Gen. Microbiol. 133, 1127–1135. doi: 10.1099/00221287-133-5-1127
Smith, H. W., Huggins, M. B., and Shaw, K. M. (1987b). The control of experimental Escherichia coli diarrhoea in calves by means of bacteriophages. J. Gen. Microbiol. 133, 1111–1126. doi: 10.1099/00221287-133-5-1111
Soothill, J. S. (1992). Treatment of experimental infections of mice with bacteriophages. J. Med. Microbiol. 37, 258–261. doi: 10.1099/00222615-37-4-258
Soothill, J. S. (1994). Bacteriophage prevents destruction of skin grafts by Pseudomonas aeruginosa. Burns 20, 209–211. doi: 10.1016/0305-4179(94)90184-8
Srivastava, A. S., Kaido, T., and Carrier, E. (2004). Immunological factors that affect the in vivo fate of T7 phage in the mouse. J. Virol. Methods 115, 99–104. doi: 10.1016/j.jviromet.2003.09.009
Subirats, J., Sànchez-Melsió, A., Borrego, C. M., Balcázar, J. L., and Simonet, P. (2016). Metagenomic analysis reveals that bacteriophages are reservoirs of antibiotic resistance genes. Int. J. Antimicrob. Agents 48, 163–167. doi: 10.1016/j.ijantimicag.2016.04.028
Summers, W. C. (1993). Cholera and plague in India: the bacteriophage inquiry of 1927-1936. J. Hist. Med. Allied Sci. 48, 275–301. doi: 10.1093/jhmas/48.3.275
Summers, W. C. (2001). Bacteriophage therapy. Annu. Rev. Microbiol. 55, 437–451. doi: 10.1016/B978-0-12-394438-2.00001-3
Torres-Barceló, C. (2018b). The disparate effects of bacteriophages on antibiotic-resistant bacteria. Emerg. Microbes Infect. 7:168. doi: 10.1038/s41426-018-0169-z
U.S. National Library of Medicine (2018). ClinicalTrials.gov. Bacteriophages. Available at: https://clinicaltrials.gov/ct2/results?cond=&term=bacteriophages&cntry=&state=&city=&dist (accessed October 10).
Van Regenmortel, M. H. (2007). Virus species and virus identification: past and current controversies. Infect. Genet. Evol. 7, 133–144. doi: 10.1016/j.meegid.2006.04.002
Vandenheuvel, D., Lavigne, R., and Brüssow, H. (2015). Bacteriophage therapy: advances in formulation strategies and human clinical trials. Annu. Rev. Virol. 2, 599–618. doi: 10.1146/annurev-virology-100114-054915
Weinbauer, M. G. (2004). Ecology of prokaryotic viruses. FEMS Microbiol. Rev. 28, 127–181. doi: 10.1016/j.femsre.2003.08.001
Wright, A., Hawkins, C. H., Anggård, E. E., and Harper, D. R. (2009). A controlled clinical trial of a therapeutic bacteriophage preparation in chronic otitis due to antibiotic-resistant Pseudomonas aeruginosa; a preliminary report of efficacy. Clin. Otolaryngol. 34, 349–357. doi: 10.1111/j.1749-4486.2009.01973.x
Keywords: antibiotics, antimicrobial resistance, bacterial infection, bacteriophages, multi-drug resistance
Citation: Principi N, Silvestri E and Esposito S (2019) Advantages and Limitations of Bacteriophages for the Treatment of Bacterial Infections. Front. Pharmacol. 10:513. doi: 10.3389/fphar.2019.00513
Received: 28 February 2019; Accepted: 24 April 2019;
Published: 08 May 2019.
Edited by:
Roberto Paganelli, Università degli Studi G. d’Annunzio Chieti e Pescara, ItalyReviewed by:
Han Qiao, Shanghai Jiao Tong University School of Medicine, ChinaErzsébet Bartolák-Suki, Boston University, United States
Copyright © 2019 Principi, Silvestri and Esposito. This is an open-access article distributed under the terms of the Creative Commons Attribution License (CC BY). The use, distribution or reproduction in other forums is permitted, provided the original author(s) and the copyright owner(s) are credited and that the original publication in this journal is cited, in accordance with accepted academic practice. No use, distribution or reproduction is permitted which does not comply with these terms.
*Correspondence: Susanna Esposito, susanna.esposito@unimi.it