- 1Zhejiang Province Key Laboratory of Anti-Cancer Drug Research, College of Pharmaceutical Sciences, Zhejiang University, Hangzhou, China
- 2Center for Drug Safety Evaluation and Research, Zhejiang University, Hangzhou, China
Multiple sclerosis (MS) is a chronic inflammatory neurodegenerative disease of the central nervous system (CNS). The early stage is characterized by relapses and the later stage, by progressive disability. Results from experimental and clinical investigations have demonstrated that microglia and macrophages play a key part in the disease course. These cells actively initiate immune infiltration and the demyelination cascade during the early phase of the disease; however, they promote remyelination and alleviate disease in later stages. This review aims to provide a comprehensive overview of the existing knowledge regarding the neuromodulatory function of macrophages and microglia in the healthy and injured CNS, and it discusses the feasibility of harnessing microglia and macrophage physiology to treat MS. The review encourages further investigations into macrophage-targeted therapy, as well as macrophage-based drug delivery, for realizing efficient treatment strategies for MS.
Introduction
Multiple sclerosis, a CNS autoimmune and neurodegenerative disease, affects approximately 2.5 million people worldwide, seriously diminishing their quality of life and causing a significant financial burden (Compston and Coles, 2008; Lemus et al., 2018; Thompson et al., 2018). Studies using EAE, an animal model of MS, have revealed that microglia/macrophages actively participate in the pathogenesis of EAE progression (Jiang et al., 2014). The CNS contains several resident macrophages, such as microglia and non-parenchymal macrophages, in the choroid plexus, perivascular space, and meninges, which function to maintain CNS homeostasis (Prinz et al., 2011). Alterations in CNS homeostasis lead to the recruitment of peripheral blood-derived monocytes (known as monocyte-derived macrophages) into the CNS, the activation of microglia, and the presence of foamy macrophages (Bogie et al., 2014; Kuhlmann et al., 2017; Mrdjen et al., 2018; Zéphir, 2018). This phenomenon appears to be a type of self-protective mechanism designed to eliminate abnormalities and recover a steady state.
Monocyte-derived macrophages and CNS-resident phagocytic cells can promote neuroinflammation, thereby inducing MS, but they can also play neuroprotective and anti-inflammatory roles, depending on many factors. In this review, we discuss the protective and pathogenic mechanism of microglia and macrophages in the development of MS and EAE, and propose the use of macrophages in MS therapy for repairing CNS damage.
Microglia and Macrophages in a Healthy CNS
In a healthy CNS, microglia reside in the parenchyma, while non-parenchymal macrophages are present in boundary regions, including perivascular spaces, the meninges, and the choroid plexus. These cells have different developmental origins and serve distinct functions by specific subtypes.
Origin of CNS Macrophages and Microglia
The earlier view that CNS macrophages are derived from blood-borne myeloid cells during adulthood has now been challenged, because studies using novel transgenic mouse models have shown that macrophages other than choroid plexus macrophages originate exclusively from the yolk sac (Figure 1; Gomez Perdiguero et al., 2015; Goldmann et al., 2016). The application of tamoxifen to adult Cx3cr1CreER:R26-YFP mice has further demonstrated that not only microglia but also perivascular and meningeal macrophages retain the yfp label from embryonic day (E)16.0 to 8–9 weeks of age (Goldmann et al., 2016), indicating that non-parenchymal meningeal and perivascular CNS macrophages and microglia arise entirely from embryonic precursor cells. Fate-mapping tools have helped reveal that CNS macrophages arise from the primitive c-kit+ erythromyeloid precursors located in the yolk sac during E7.5∼8.0. Uncommitted erythromyeloid precursors subsequently disappear at E9.0 and develop into CD45+CX3CR1hiF4/80hi (A2) macrophage progenitors via immature CD45+CX3CR1loF4/80lo (A1) cells. Proliferating A2 cells then become CD11b+F4/80+ microglia and perivascular, meningeal, and choroid plexus macrophages (Bertrand et al., 2005; Kierdorf et al., 2013; Hoeffel et al., 2015; Matcovitch-Natan et al., 2016). Once established in the CNS, microglia and perivascular and meningeal macrophages persist throughout the life of the organism due to their longevity and their capacity of self-proliferation, rather than the infiltration of peripheral myeloid cells (Ajami et al., 2007, 2011). In contrast, choroid plexus macrophages mainly depend on blood-derived immigrating Ly6Chi monocytes after birth (Goldmann et al., 2016). The astonishing finding of such close ontogeny between microglia and the other non-parenchymal macrophages in the CNS prompts the question of whether they can transform into one another under specific physiological conditions.
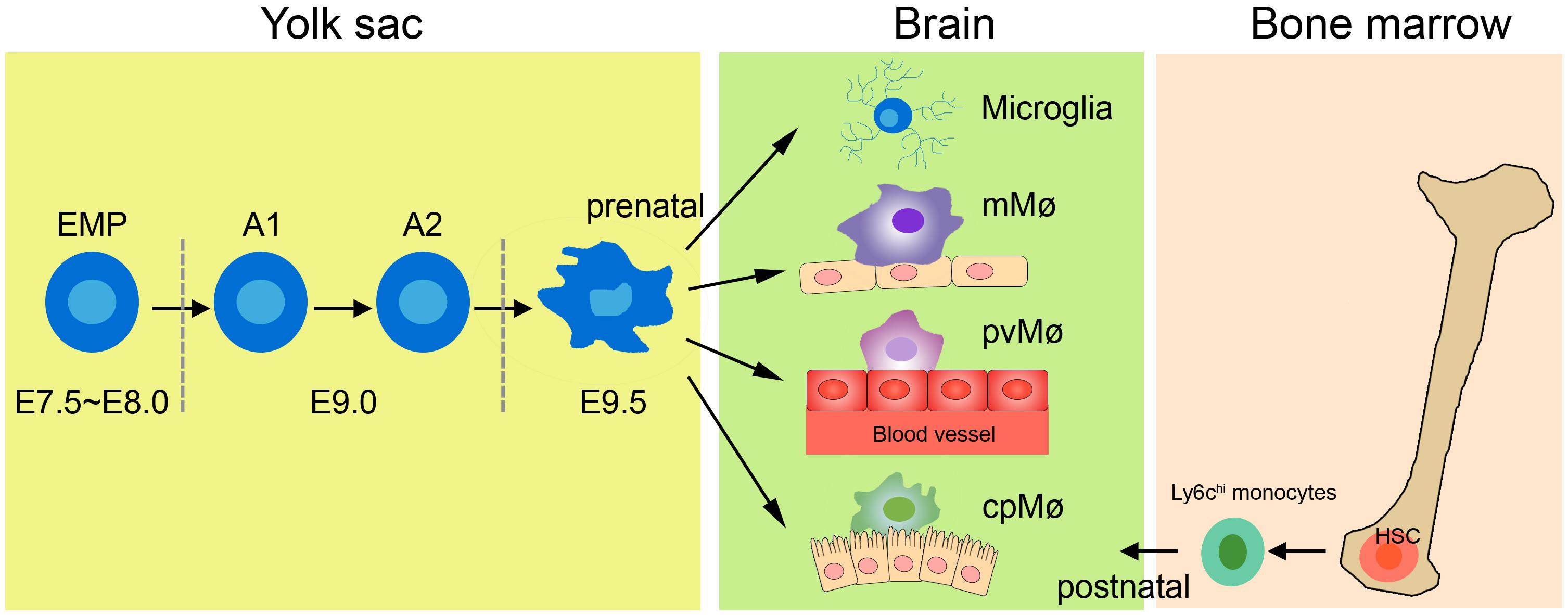
Figure 1. Origin of tissue macrophages in healthy CNS. Microglia and tissue-resident macrophages are derived from prenatal sources. The primitive erythromyeloid precursors (EMPs) located in the yolk sac during E7.5∼8.0 soon develop into A1 cells and finally become A2 cells. The matured A2 cells then differentiate into microglia, meningeal macrophages (mMø), perivascular macrophages (pvMø), and choroid-plexus macrophages (cpMø). However, after birth, cpMΦ originate exclusively from bone Ly6chi monocytes.
Functions of Microglia
Microglia are widely scattered throughout the brain and spinal cord, and they come into close contact with neurons, astrocytes, and oligodendrocytes (Ransohoff and Cardona, 2010; Szepesi et al., 2018). Studies have demonstrated that microglia mediate synaptic pruning and trophic-factor production through fractalkine-CX3CR1 signaling in neurodevelopment (Tremblay et al., 2010; Ransohoff and El Khoury, 2014; Schafer and Stevens, 2015; Miyamoto et al., 2016). Fractalkine receptor-deficient mice showed decreased neuron survival in layer V cortical neurons during the first postnatal week because of the reduced secretion of insulin-like growth factor from microglia (Ueno et al., 2013). Furthermore, microglia depletion by the Cre-Lox technique led to deficiencies in multiple learning tasks, as well as a significant reduction in learning-related synapse formation (Parkhurst et al., 2014). In addition, microglial-secreted BDNF could increase the activity of neuronal tropomyosin-related kinase receptor B, an important modulator in synaptic plasticity, prompting a crucial role of BDNF signaling in learning and memory-related synapse formation (Parkhurst et al., 2014). Microglia may communicate with neurons; changes in the physiological environment such as chronic stress and light deprivation can cause hyper-ramification of microglia and more frequent neuron–microglia contacts (Torres-Platas et al., 2014; Welberg, 2014; Yirmiya et al., 2015). Moreover, microglia act as scavengers in the adult CNS to remove damaged components, apoptotic cells, and misfolded proteins (Sierra et al., 2010). Once “danger” signals are detected in the surrounding environment, microglia rapidly change into a reactive phenotype, characterized by larger soma, and release various complement factors to facilitate neuron and tissue repair (Hanisch and Kettenmann, 2007; Graeber and Streit, 2010; Kettenmann et al., 2011).
Functions of Non-parenchymal Macrophages
Non-parenchymal brain macrophages have been largely ignored, but recent studies have refocused on their relevant functions in the perivascular space, meninges, and choroid plexus (Herz et al., 2017). Perivascular macrophages are critical in BBB establishment (Mendes-Jorge et al., 2009; He et al., 2016), and blockage of colony stimulating factor 1 receptor signaling leads to a decreased coverage of pericytes in brain vessels (Yamamoto et al., 2017). Perivascular macrophages continuously retract and protract along blood vessels in the adult brain (Daneman, 2012; Goldmann et al., 2016), suggesting that they may play a role in immune surveillance. Moreover, they can protect against bacterial infection by recruiting circulating leukocytes (Polfliet et al., 2001). The study of meningeal macrophages has been limited; however, it was reported that meninges possess a “glymphatic” system, which guides the clearance of waste products such as β-amyloid in the CNS (Raper et al., 2016). However, whether meningeal macrophages instruct lymphangiogenesis in the “glymphatic” system, similar to their role in the peripheral immune system, remains to be ascertained (Gordon et al., 2011; Louveau et al., 2015). In addition, meningeal macrophages function as APCs, detecting cell debris and antigens to maintain immune homeostasis (Kivisakk et al., 2009). Choroid plexus macrophages are located near the microvilli of the choroid plexus (Liddelow, 2015; Marques et al., 2017), and their main function may support CSF release and flux (Goldmann et al., 2016). In conclusion, considerable fundamental details about non-parenchymal macrophages remain to be determined.
Polarization of Microglia and Macrophages
Macrophages (including microglia) are often classified into M1 (inflammatory) and M2 (anti-inflammatory) phenotypes (Mikita et al., 2011; Liu et al., 2013). However, this simple classification does not reveal the full spectrum of macrophages under different stimulation conditions owing to their diversity and plasticity (Prinz and Priller, 2014). Transcriptome profiles have revealed at least nine different subtypes of human macrophages (Veremeyko et al., 2018). In this review, M1 and M2 represent the activation states of macrophages rather than specific cell subtypes.
Interferon-γ and lipopolysaccharide treatment can generate M1 cells, while IL-4, IL-10, or IL-13 are activators of M2 cells. M1 macrophages and microglia are characterized by an amoeboid shape, and they release pro-inflammatory cytokines, such as IL-6, IFN-γ, IL-23, TNF-α; inducible nitric oxide synthase; chemokines, including CCL 4, CCL5, and CCL8; and CXCL 2, CXCL4, and CXCL9 (Juhas et al., 2015; Kapellos and Iqbal, 2016). M1 macrophages and microglia are potent APCs, causing an adaptive immune response to clear foreign substances and abnormal proteins (Das et al., 2015). In contrast, M2 macrophages and microglia have smaller cell bodies and a branched structure (Ransohoff and Perry, 2009), and they express a variety of anti-inflammatory molecules, including IL-4, IL-10, IL-13, and transforming growth factor-β (David and Kroner, 2011). These contribute to immunoregulation by inhibiting inflammation, remodeling tissue, promoting angiogenesis, and clearing parasites (Banerjee et al., 2013). M2 microglia promote oligodendrocyte differentiation, and their depletion inhibits remyelination (Kotter et al., 2005; Miron et al., 2013). M2-polarized macrophages and microglia can be further subdivided into M2a, M2b, and M2c cells. These have different activation and functional mechanisms but have some biochemical overlap (Table 1; Murray et al., 2014). Increasing evidence suggests that M1 cells prefer an anaerobic environment, whereas oxygen consumption and oxidative phosphorylation benefit M2 macrophages (Shi et al., 2017; Wang et al., 2018).
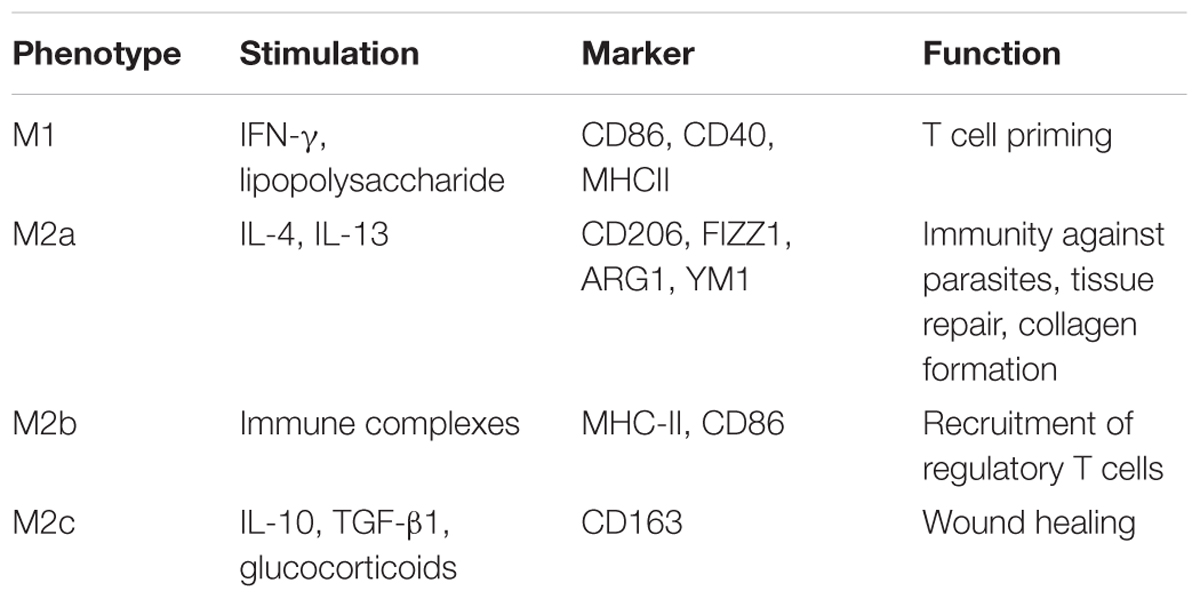
Table 1. Polarization of macrophages and microglia (Murray et al., 2014).
In conclusion, microglia and non-parenchymal macrophages are independent immune populations in the CNS. Besides different cell origins, they have their own functions in CNS development, homeostasis, and tissue healing (Lopez-Atalaya et al., 2018). Under steady state, microglia are primarily responsible for supporting the development of the healthy brain, they shape and maintain the neuronal synaptic network, interact with neurons and produce beneficial components (Hagemeyer et al., 2017). And non-parenchymal macrophages strictly limits access of peripheral immune cells (T cells, B cells, and monocytes) reach the CNS parenchyma (Polfliet et al., 2001). Upon detecting “danger” signals, both microglia and non-parenchymal macrophages produce large amounts of chemokines and cytokines to promote leukocyte infiltration into the CNS parenchyma and function as APCs to reactivate T cells in the CNS (Galli et al., 2011; Krausgruber et al., 2011). Moreover, microglia phagocytose damaged cell debris, as well as unnecessary neurons and synapses resulting from defective differentiation and/or migration (Paolicelli et al., 2011; Lopez-Atalaya et al., 2018).
Microglia and Macrophages in MS
After CNS injury and inflammation, while microglia situated in the parenchyma and macrophages in the choroid plexus, perivascular space, and the meninges are activated, monocytes from the blood cross the damaged BBB and become monocyte-derived macrophages in the CNS (Lassmann et al., 2001). These spatial differences may determine subtype-specific effector functions of these distinctive phagocytes in the diseased CNS. In this section, we compare and elaborate the functions of microglia and macrophages in the progression of MS and EAE.
Distinguishing Microglia From Other Macrophages
A lack of specific markers that distinguish resident microglia from other macrophages in MS lesions hampers the accurate evaluation of their contributions to human brain pathology. Originally, the expression profiles of CD11b and CD45 allowed the discrimination of CD11b+CD45med microglia from CD11b+CD45hi monocyte-derived macrophages (Martin et al., 2017). However, such a classification remains controversial (Koeniger and Kuerten, 2017). Studies have suggested TMEM119 (Bennett et al., 2016; Satoh et al., 2016; Li et al., 2019), Sall1 (Buttgereit et al., 2016), Siglec-H (Konishi et al., 2017), and P2Y12 (Mildner et al., 2017) as more valuable markers for distinguishing microglia from other macrophages. For instance, TMEM119 is expressed exclusively on Iba1+CD68+ microglia and not on infiltrated Iba1+CD68+ macrophages within demyelinating lesions of MS (Satoh et al., 2016). However, TMEM119 expression is absent in immature microglia (Satoh et al., 2016). Sall1 expression is abundant in neuronal and glial progenitor cells during CNS development (Harrison et al., 2012; Buttgereit et al., 2016), and P2Y12 shows decreased expression in activated microglia (Amadio et al., 2014; Mildner et al., 2017). Almost all microglia in the CNS parenchyma expressed Siglec-H, from developmental to mature stages, and the expression was maintained in activated microglia after CNS injuries (Koso et al., 2018). In contrast, Siglec-H expression was largely absent from other myeloid cells in the CNS (Koso et al., 2018). Overall, these findings suggest that Siglec-H is a promising specific marker for the specific identification of microglia from CNS-associated macrophages. However, these results are all from mice, and human Siglec-L2 is only ∼42% homologous with Siglec-H (Zhang et al., 2006). Whether Siglec-L2 might be a marker for microglia warrants further investigation.
Microglia
Pronounced microglial activation with high expression levels of pro-inflammatory genes can be found in lesion areas and normal-appearing white matter of MS patients (Lucchinetti et al., 2000; Singh et al., 2013; Zrzavy et al., 2017). These clusters of activated microglia are regarded as “pre-active lesions,” with an absence of leukocyte infiltration and demyelination; however, they may eventually develop into active demyelinating MS lesions (Singh et al., 2013). In contrast to healthy controls, the number of P2Y12+ homeostatic microglia is significantly reduced in the white matter of MS patients, and TMEM119+ microglia predominantly govern the edge of active lesions in MS (Zrzavy et al., 2017), indicating that microglial activation is related to disease development.
TSPO tracers have demonstrated inflammatory processes with microglia involvement in MS (Vas et al., 2008; Oh et al., 2011). These microglia are enriched with iron, express pro-inflammatory cytokines, and cause persistent tissue damage (Wisnieff et al., 2015). Although microglia are important in synaptic plasticity, this function is destroyed under pathological insults, which causes synaptic loss in MS and eventually, cognitive decline (Michailidou et al., 2015; Hong et al., 2016; Salter and Stevens, 2017; Di Filippo et al., 2018). Activated microglia in EAE mice may produce large amounts of TNF-α and thus induce excessive glutamate, leading to spontaneous and miniature excitatory post-synaptic currents (Centonze et al., 2009, 2010). Furthermore, astroglial ATP amplifies microglial signals and subsequently promotes glutamate release from astroglia, which directly inhibits synaptic transmission (Kettenmann et al., 2013). Classically activated neuroinflammatory microglia secrete TNF-α, and C1q, inducing A1 astrocyte dysfunction, thereby resulting in the dysfunction of both oligodendrocytes and neurons (Liddelow et al., 2017; Rothhammer et al., 2018). Importantly, mitochondrial disturbances have been demonstrated in modulating MS lesions, reactive oxygen species and reactive nitrogen species generated by the activated microglia also lead to intra-axonal mitochondrial injury (Balaban et al., 2005; Haile et al., 2017). Kinetics studies from EAE mice indicate that microglia are the first cell line to take up myelin antigens (Sosa et al., 2013), subsequently restimulating encephalitogenic T cells in the CNS through major histocompatibility complex (MHC) molecules and costimulatory molecules (Perry, 1998). Consistent with this, time-course studies in EAE mice showed that the appearance of inflammatory T cells in the CNS is consistent with the activation of CD11b+ microglia (Murphy et al., 2010). However, specific deletion of MHCII in microglia does not affect disease progression, suggesting that microglia are not important in reactivating efficient T cells in the CNS (Wolf et al., 2018). Microglia also secrete a series of pro-inflammatory cytokines such as IL-18, IL-6, and IL-1b and chemokines like CCL2 and CCL5 to aggravate both MS and EAE (Merson et al., 2010; Jiang et al., 2014). Moreover, activated microglia in white matter lesions may lead to greater brain atrophy in secondary progressive disease (Datta et al., 2017). In conclusion, microglia exert detrimental functions in MS and strongly damage myelin.
Microglial activation may also be beneficial for remyelination in MS due to the phagocytotic ability of microglia, as well as their secretion of neuroprotective molecules and anti-inflammatory cytokines (Du et al., 2017). Microglia-specific deletion of A20, the crucial protein in regulating microglial activation, makes microglia lose their ability to regulate neuronal synaptic function, thus exacerbating MS-like disease (Voet et al., 2018). Several receptors, including TREM2, complement receptor 3, and signal regulatory protein-α, invoke microglia to phagocytose myelin debris in lesions (Brendecke and Prinz, 2015). Microglia-specific ablation of TREM2 cannot upregulate genes associated with phagocytosis (Poliani et al., 2015), and the blockage of TREM2 results in increased demyelination in EAE (Piccio et al., 2007). Moreover, microglia secrete trypsinogen, insulin-like growth factor, and fibroblast growth factor during activation to promote neurogenesis (Pérez-Martín et al., 2010; Voss et al., 2012). Anti-inflammatory molecules from microglia also benefit remyelination in EAE, for example, IL-4 enhances oligodendrogenesis (Butovsky et al., 2006), and activin A drives oligodendrocyte differentiation (Miron et al., 2013). Interestingly, pro-inflammatory cytokine TNF has neuroprotective functions in EAE (Wolf et al., 2017), and deletion of microglial TNFR2 causing early disease onset, with increased leukocyte infiltration, demyelination and T cell activation in CNS (Gao et al., 2017). However, soluble TNF repair remyelination by suppressing clearance of myelin debris (Karamita et al., 2017). Other evidence indicated that microglia can prompt remyelination through P2X4R signaling, and that the blockade of P2X4R results in microglial activation to a pro-inflammatory phenotype and exacerbates the clinical symptoms of EAE mice (Zabala et al., 2018).
Monocyte-Derived Macrophages
During the effector stage of EAE, Ly6Chi monocytes rapidly infiltrate into the inflamed CNS with the help of matrix metalloproteinases (Ajami et al., 2011; Yamasaki et al., 2014). Monocytes then differentiate into macrophages, express MHC II and costimulatory molecules, and produce pro-inflammatory factors that actively contribute to the demyelination process (Jiang et al., 2014; Yamasaki et al., 2014). Consistently, the abundance of CCR1+/CCR5+ hematogenous monocytes has been found to appear in demyelination zones compared with the periplaque white matter of MS patients (Trapp et al., 1998; Trebst et al., 2001), and Kim1p staining shows abundant subcortical macrophage infiltration (Tobin et al., 2017).
The activated monocyte-derived macrophages is generally considered to be harmful in MS (Yamasaki et al., 2014). Infiltrating monocytes accumulate at the nodes of Ranvier during early stages of EAE and actively initiate the demyelination of axons (Yamasaki et al., 2014), similar to microglia, but they do not affect CNS-resident microglia (Ajami et al., 2011). Bone marrow chimeric mice show that TRPM2 expressed by CNS-infiltrating macrophages prompts the production of CXCL2, thus increasing neutrophil infiltration and contributing to the progression of EAE (Tsutsui et al., 2018). High F4/80+CD45hi macrophage/T-cell ratios were found in EAE mice (McMahon et al., 2005), indicating that recruited macrophages participate in T-cell proliferation after myelin antigen stimulation. Macrophages also secrete large amounts of pro-inflammatory cytokines, such as IL-6, IL-12, TNF-α, IL-1, and IL-23, to aggravate the inflammatory response (Valentin-Torres et al., 2016). IL-1β expression has been found to be pivotal in the polarization of Th17 cells and therefore for EAE induction (Sutton et al., 2006). IL-6 has also been demonstrated to be critical for CNS autoimmunity, as IL-6-deficient mice demonstrated alleviated EAE (Samoilova et al., 1998). Macrophages also express high levels of CCR4 during the disease, and CCR4 deletion leading to reduced accumulation of macrophages in CNS and attenuated EAE symptoms (Forde et al., 2011). These findings suggest that macrophages are responsible for demyelination, but their direct functions on axons remain to be determined.
Other studies have also defined the neuron-supportive activities of Ly6Chi monocytes in EAE. The activation of invariant natural killer T cells converts inflammatory Ly6Chi monocytes to M2 macrophages in the CNS, with associated improvement in neurological impairment (Denney et al., 2012; Jiang et al., 2017). Recent studies have showed that TG2 mRNA levels are increased in monocytes derived from MS patients and correlates with anti-inflammatory cytokine expression, proposing a more anti-inflammatory monocytes status in MS (Sestito et al., 2017). Monocyte-derived macrophages can also promote CNS repair in the injured spinal cord by clearing myelin debris through scavenger receptors (GrandPré et al., 2000; Kotter et al., 2006). In contrast to microglia, macrophages express TREM-1 rather than TREM-2, and although this isoform plays a role in inflammatory responses (Colonna and Facchetti, 2003), the functions of TREM-1 still need to be further studied in a CNS autoimmunity context. In addition, monocyte-derived macrophages secrete trophic factors and anti-inflammatory factors to alleviate sympathetic neuron dysfunction (Hikawa and Takenaka, 1996; Miron et al., 2013). The transcription factor Nr4a1 represses the autocrine production of norepinephrine in macrophages, and myeloid cells lacking Nr4a1 lead to more leukocyte infiltration within the CNS and more severe EAE clinical symptoms (Shaked et al., 2015). Consistently, expression levels of Nr4a were down-regulated in peripheral blood mononuclear cells from MS patients, and Fingolimod treatment could recovery MS from Nr4a2 deficit (Montarolo et al., 2018). Besides Ly6Chi monocytes, a small number of LyC6lo monocytes also recruited in the injured CNS (Saederup et al., 2010). Although studies have pointed toward a reparative role of infiltrated Ly6Clo monocytes in a spinal cord injury model (Arnold et al., 2007; Nahrendorf et al., 2007); however, more research is needed to determine whether Ly6Clo monocytes do promote repair processes in the pathogenesis of EAE.
Non-parenchymal Macrophages
Perivascular macrophages, typically CD163+HLADR+, are markedly increased in MS patients and EAE-affected animals (Zhang et al., 2011; Mammana et al., 2018). Substantial evidence indicates that perivascular macrophages have both supportive and neuroprotective roles in MS. Selective elimination of both perivascular and meningeal macrophages by treatment with clodronate-loaded liposomes reduces the progression of EAE in rats (Polfliet et al., 2002), suggesting that perivascular macrophages may act as competent APCs in EAE. EAE-related perivascular macrophages upregulate the expression of MHC II and can present peptides to myelin-specific T cells in the CNS, thereby accelerating disease progression (Perry, 1998; Fabriek et al., 2005; Greter et al., 2005; Vogel et al., 2013). Furthermore, perivascular macrophages express high levels of adhesion molecules such as VCAM-1 and ICAM-1 and chemokines like CCL2 and CCL3 (Hofmann et al., 2002; Vercellino et al., 2017), which may mediated by chondroitin sulfate proteoglycans (Stephenson et al., 2018), to promote leukocyte infiltration into the CNS. However, perivascular macrophages during the early stage of EAE are characterized as mannose receptor-positive M2 macrophages, produce leukemia inhibitory factors and neuroprotective factors, and secrete anti-inflammatory IL-6 and IL-1ra to protect glial and neuronal cells (Boven et al., 2006; Vanderlocht et al., 2006). Moreover, M2 perivascular macrophages can promote the differentiation of Th2 and Treg cells due to their anti-inflammatory characteristic (Weber et al., 2007).
An extensive number of meningeal immune cells in the CNS leads to a more severe clinical course of MS (Howell et al., 2011; Choi et al., 2012; Kilsdonk et al., 2017; Kolber et al., 2017; Bevan et al., 2018). In concordance, meningeal macrophages are equipped to present peptide antigens to T cells, triggering neuroinflammatory events (Bartholomäus et al., 2009; Kivisakk et al., 2009; Ransohoff and Cardona, 2010). In addition, meningeal macrophages can release inflammatory and toxic mediators or even invade the CNS parenchyma to sustain MS pathology (Maxwell et al., 1990; Garabedian et al., 2000; Reuter et al., 2001). In contrast, meningeal macrophages produce neurotrophic and anti-inflammatory factors to inhibit leukocyte activity and their infiltration into the CNS at particular disease stages (Bogie et al., 2014). Leptomeningeal macrophages increase the expression of transforming growth factor-β in glial cells and cortical neurons by releasing prostaglandin E2 during inflammation (Wu et al., 2007). However, other studies demonstrated that prostaglandin E2 could also exacerbate EAE (Lima et al., 2012), depending on the disease stage and microenvironment.
The choroid plexus, the site of CSF production, forms the interface between blood and the CNS (Wolburg and Paulus, 2010; Kaur et al., 2016). It is thus an important element in the communication between the vascular compartment and the CNS during inflammation (Breuer et al., 2018). The severity of MS coincides with enhanced levels of infiltrated leukocytes in the choroid plexus (Engelhardt et al., 2001; Vercellino et al., 2008; Mitchell et al., 2009), strongly indicating that inflammation at the choroid plexus contributes to the disease. Chemokines like CXCL9 and CXCL10 accumulate in large quantities in the CSF during MS progression (Edwards et al., 2013; Puthenparampil et al., 2017), but whether they are secreted by macrophages needs to be confirmed. Choroid plexus macrophages may release neurotoxic mediators and pro-inflammatory factors into the CSF during disease to impact perivascular and meningeal inflammation, as well as the activation and integrity of neurons and glial cells (Garabedian et al., 2000; Bragg et al., 2002a,b). However, remote brain choroid plexus can also recruit M2-like macrophages to an injured spinal cord, which might benefit myelin repair (Shechter et al., 2013).
Foamy Macrophages
Ample evidence indicates that abundant foamy macrophages are present in MS lesions (Grajchen et al., 2018). After disease initiation, both resident microglia and recruited monocytes ingest and accumulate vast amounts of myelin-derived lipids and thereby acquire their distinctive morphology (Li et al., 1996; Bogie et al., 2017), but how foamy macrophages function in the process of MS is still poorly understood. MHC II and costimulatory molecules expressed on foamy macrophages may present myelin antigens to autoreactive T cells (de Vos et al., 2002; Boven et al., 2006; van Zwam et al., 2011), thereby driving epitope spread and MS progression or even initiation (Stys et al., 2012). In agreement with this, numerous studies have found M1-like foamy macrophages present within MS lesions (Bogie et al., 2014). Interestingly, yet another study showed that microglia show the M1 phenotype after the first 6 h of myelin uptake, and they soon convert to the M2 phenotype after prolonged exposure (Liu et al., 2006), indicating that the polarization of foamy macrophages can be reversed. Both in vivo studies and in vitro cultures demonstrated that foamy macrophages prefer to express anti-inflammatory molecules rather than secrete pro-inflammatory cytokines (van Zwam et al., 2011), indicating that myelin macrophages may resolve the inflammation in MS demyelination lesions. Consistent with this, researchers found that mouse myelin macrophages could inhibit IFN-γ release by Th1 cells and suppress EAE severity (Bogie et al., 2014). Collectively, these studies imply that besides being aggressors in MS pathogenesis, foamy macrophages can also destroy T cell-induced autoimmunity. However, more research is warranted to confirm the specific role of foamy macrophages in MS progression.
In fact, among numerous phagocytic cells, only microglia and infiltrating macrophages play a crucial role in MS progression. Both of them participate in demyelination and remyelination through antigen presentation, phagocytosis, and inflammatory mediator secretion (Shechter et al., 2009; Ruckh et al., 2012; Li and Barres, 2018); however, microglia alone could affect neuron cells directly through fractalkine-CX3CR1 signaling (Eyo et al., 2016). Although microglia and infiltrating macrophages are potent APCs in EAE, the expression levels of MHC II and costimulatory molecules on microglia are lower (Brendecke and Prinz, 2015), indicating that inflammation in the CNS is mainly activated by infiltrating macrophages. The roles of perivascular macrophages, as well as macrophages, in the meninges and choroid plexus in MS are not yet completely understood. In conclusion, whether these macrophages are unique phagocyte subsets in MS, and if so, the underlying mechanism thereof, needs to be determined.
Therapeutic Perspectives
As discussed in the previous sections, any change in the physiological functions of macrophages and microglia can impact neuroinflammatory and neurodegenerative events. Therefore, selectively promoting macrophage polarization to the anti-inflammatory subtype is an attractive therapeutic option (Figure 2).
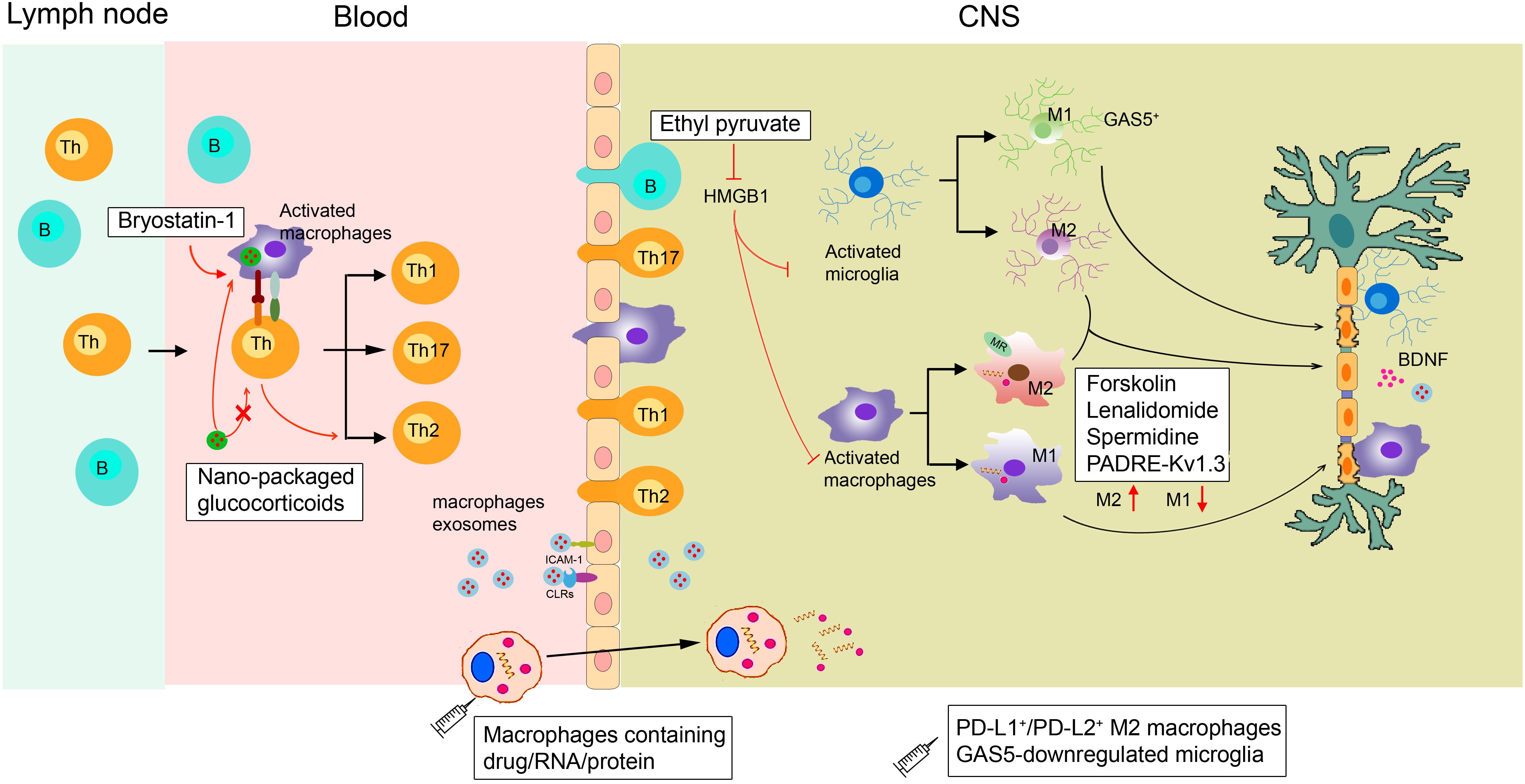
Figure 2. Proposed actions of disease-modifying multiple sclerosis therapies targeting macrophages. In the periphery, bryostatin-1 promotes the differentiation of lymphocytes into anti-inflammatory Th2 cells by acting on macrophages. Ethyl pyruvate prevents the activation of macrophages/microglia within the CNS. Lenalidomide, spermidine, forskolin, and the novel PADRE-Kv1.3 vaccine can promote macrophage/microglia M2 polarization. Transplanting GAS5-downregulated microglia into the lateral ventricles of EAE mice could promote remyelination. Exogenous supplementation of M2 macrophages such as PD-L1+/PD-L2+ M2 cells can also alleviate demyelination. Micro-based drug delivery systems for delivering GC to targeted macrophages have been widely used in EAE. As macrophages can easily migrate into the brain during MS, they can be used as cellular vehicles for the delivery of small drugs, RNAs, and proteins to the brain parenchyma. Macrophage exosomes could cross the BBB and deliver BDNF to recover injury. HMGB1, high-mobility group box 1; GC, glucocorticoids; MR, mineralocorticoid receptors; CLRs, C-type lectin receptors.
MS Therapy by Regulating Macrophages
Although the crucial role of macrophages and microglia in neurodegenerative events has been proven, therapeutics solely targeting these phagocytes are still lacking. Currently, the clinical treatment of MS includes recombinant IFNβ-1a, glatiramer acetate, and natalizumab to mainly regulate T and B cells (Cheng et al., 2017). Studies have found that the neuroprotective effects of glatiramer acetate are mediated by activated M2 microglia (Ratchford et al., 2012). Furthermore, diverse new compounds that influence macrophage and microglial functions are undergoing non-clinical testing. These agents include ethyl pyruvate (Djedović et al., 2017), spermidine (Yang et al., 2016), bryostatin-1 (Kornberg et al., 2018), forskolin (Veremeyko et al., 2018), and lenalidomide (Weng et al., 2018). Ethyl pyruvate can reduce high-mobility group box 1 expression in activated ED1+ macrophages and Iba1+ microglia, thus inhibiting the activation of macrophages/microglia within the CNS to protect against EAE (Djedović et al., 2017). In addition, forskolin has been found to alleviate EAE by suppressing the expression of CD86 while enhancing the expression of ARG1 in macrophages (Veremeyko et al., 2018). Bryostatin-1 provides marked benefits in mice with EAE by acting on APCs, including macrophages, to promote the differentiation of lymphocytes into Th2 cells (Kornberg et al., 2018).
The novel PADRE-Kv1.3 vaccine has been applied to treat EAE. After vaccination, infiltrated microglia/macrophages significantly shift toward the M2 subtype in the CNS (Fan et al., 2018). Mineralocorticoid receptors expressed on macrophages promote their polarization toward M2 cells, and mice selectively lacking mineralocorticoid receptors in their myeloid cells show diminished clinical symptoms of EAE (Montes-Cobos et al., 2017b). Transferring M2 macrophages to eliminate EAE has been widely applied. The long non-coding RNA GAS5, a suppresser of microglial M2 polarization, is expressed at a high level within microglia in MS patients. Transplanting GAS5-downregulated microglia into the lateral ventricles of EAE mice could promote remyelination, similar to a lysolecithin-induced demyelination model (Sun et al., 2017). In addition, Ly6C+ monocytes could give rise to PD-L1+/PD-L2+ M2 cells, and transferring PD-L1+/PD-L2+ M2 macrophages might reduce the incidence of EAE (Terrazas et al., 2017).
Macrophage-Targeted Therapeutics via Micro-Based Drug Delivery Systems in Neurodegenerative Diseases
Because most drugs do not effectively reach macrophages at therapeutic levels, micro-based drug delivery systems for targeting macrophages have been widely used in treating CNS diseases. For example, glucocorticoids are used extensively to treat acute relapses in MS patients (Pato-Pato et al., 2003; Cohen et al., 2009), but their therapeutic effect is accompanied by serious side effects due to their broad spectrum of immunosuppressive actions (Baschant and Tuckermann, 2010). Interestingly, in vivo, glucocorticoids packaged with specific nanoparticles vs. free glucocorticoids are preferentially taken up by phagocytic cells, including macrophages, rather than T cells. This approach allows their therapeutic efficacy to be retained in EAE mice (Montes-Cobos et al., 2017a). Other research showed that infiltrated CD163+ macrophages in brains from a Parkinson’s disease model modulate local microglia to promote neuroprotection. Specifically designed liposomes can load dexamethasone solely to CD163+ macrophages. This modification leads to decreased dopaminergic cell death and better motor performance (Tentillier et al., 2016). However, we cannot ignore that several nanoparticles themselves also induce macrophage polarization toward various phenotypes and modulate macrophage reprogramming (Miao et al., 2017).
Macrophages as Drug Carriers for MS Therapy
Many CNS diseases are thought to be untreatable because drugs rarely pass through the BBB at therapeutic levels (Fischbach et al., 2013). Chemokine gradients secreted from the CNS parenchyma can induce macrophage migration to the brain, making macrophages potential carriers for drug/nano formulations across the BBB to reach target sites (Ye et al., 2018). Macrophages transporting superparamagnetic iron oxide nanoparticles and exogenous genes were injected intravenously into a lipopolysaccharide-induced acute neuroinflammatory mouse model. The differentiated macrophages demonstrated an excellent ability to spread into the brain, and the number of transported macrophages positively correlated with the number of intravenous cells (Tong et al., 2016). Moreover, macrophage exosomes can interact with brain microvessel endothelial cells that comprise the BBB by binding to carbohydrate-binding C-type lectin receptors. BBB cells can absorb more macrophage exosomes when inflammation occurs due to the upregulated secretion of ICAM-1. After intravenous administration, macrophage exosomes have been shown to cross the BBB and deliver a cargo protein, BDNF, in the presence of CNS inflammation (Yuan et al., 2017). Overall, these results support the concept of using monocytes/macrophages or macrophage exosomes as cellular vehicles for the delivery of small drugs, RNAs, and proteins to the brain parenchyma.
Perspective
It is becoming increasingly clear that microglia and macrophages play critical roles in healthy, inflamed, injured, and recovering CNS due to their dual natures. We have summarized the origin, subtypes, and functions of these phagocytic cells in MS. Therapeutic interventions that block the pro-inflammatory effects of macrophages/microglia during disease progression, while preserving their anti-inflammatory functions on EAE, have achieved great success. However, as infiltrating inflammatory monocytes and microglia contribute differentially to EAE pathophysiology, strategies that allow the differential manipulation of CNS-resident microglia and infiltrating macrophages to optimally target different mechanisms operating in MS need to be developed. Moreover, extensive additional research is needed before utilizing the physiology of microglia and macrophage subsets for MS therapy.
Author Contributions
QW and QH conceived the review article and made the corrections in the manuscript. BY provided some critical comments. JyW wrote the manuscript. JjW and JcW collected the related research articles.
Funding
This study was supported by the National Natural Science Foundation of China (81872878) and the Zhejiang Provincial Natural Science Foundation of China (LGF18H310001).
Conflict of Interest Statement
The authors declare that the research was conducted in the absence of any commercial or financial relationships that could be construed as a potential conflict of interest.
Abbreviations
APCs, antigen-presenting cells; BBB, blood–brain barrier; BDNF, brain-derived neurotrophic factor; CCL, C-C motif chemokine ligands; CNS, central nervous system; CSF, cerebrospinal fluid; CXCL, C-X-C motif chemokine ligands; EAE, experimental autoimmune encephalomyelitis; IFN, interferon; IL, interleukin; MHC, major histocompatibility complex; MS, multiple sclerosis; TNF, tumor necrosis factor.
References
Ajami, B., Bennett, J. L., Krieger, C., McNagny, K. M., and Rossi, F. M. (2011). Infiltrating monocytes trigger EAE progression, but do not contribute to the resident microglia pool. Nat. Neurosci. 14, 1142–1150. doi: 10.1038/nn.2887
Ajami, B., Bennett, J. L., Krieger, C., Tetzlaff, W., and Rossi, F. M. (2007). Local self-renewal can sustain CNS microglia maintenance and function throughout adult life. Nat. Neurosci. 10, 1538–1543. doi: 10.1038/nn2014
Amadio, S., Parisi, C., Montilli, C., Carrubba, A. S., Apolloni, S., and Volonté, C. (2014). P2Y(12) receptor on the verge of a neuroinflammatory breakdown. Mediators Inflamm. 2014:975849. doi: 10.1155/2014/975849
Arnold, L., Henry, A., Poron, F., Baba-Amer, Y., van Rooijen, N., Plonquet, A., et al. (2007). Inflammatory monocytes recruited after skeletal muscle injury switch into antiinflammatory macrophages to support myogenesis. J. Exp. Med. 204, 1057–1069. doi: 10.1084/jem.20070075
Balaban, R. S., Nemoto, S., and Finkel, T. (2005). Mitochondria, oxidants, and aging. Cell 120, 483–495. doi: 10.1016/j.cell.2005.02.001
Banerjee, S., Cui, H., Xie, N., Tan, Z., Yang, S., Icyuz, M., et al. (2013). MiR-125a-5p regulates differential activation of macrophages and inflammation. J. Biol. Chem. 288, 35428–35436. doi: 10.1074/jbc.M112.426866
Bartholomäus, I., Kawakami, N., Odoardi, F., Schläger, C., Miljkovic, D., Ellwart, J. W., et al. (2009). Effector T cell interactions with meningeal vascular structures in nascent autoimmune CNS lesions. Nature 462, 94–98. doi: 10.1038/nature08478
Baschant, U., and Tuckermann, J. (2010). The role of the glucocorticoid receptor in inflammation and immunity. J. Steroid Biochem. Mol. Biol. 120, 69–75. doi: 10.1016/j.jsbmb.2010.03.058
Bennett, M. L., Bennett, F. C., Liddelow, S. A., Ajami, B., Zamanian, J. L., Fernhoff, N. B., et al. (2016). New tools for studying microglia in the mouse and human CNS. Proc. Natl. Acad. Sci. U.S.A. 113, E1738–E1746. doi: 10.1073/pnas.1525528113
Bertrand, J. Y., Jalil, A., Klaine, M., Jung, S., Cumano, A., and Godin, I. (2005). Three pathways to mature macrophages in the early mouse yolk sac. Blood 106, 3004–3011. doi: 10.1182/blood-2005-02-0461
Bevan, R. J., Evans, R., Griffiths, L., Watkins, L. M., Rees, M. I., Magliozzi, R., et al. (2018). Meningeal inflammation and cortical demyelination in acute multiple sclerosis. Ann. Neurol. 84, 829–842. doi: 10.1002/ana.25365
Bogie, J. F., Mailleux, J., Wouters, E., Jorissen, W., Grajchen, E., Vanmol, J., et al. (2017). Scavenger receptor collectin placenta 1 is a novel receptor involved in the uptake of myelin by phagocytes. Sci. Rep. 7:44794. doi: 10.1038/srep44794
Bogie, J. F., Stinissen, P., and Hendriks, J. J. (2014). Macrophage subsets and microglia in multiple sclerosis. Acta Neuropathol. 128, 191–213. doi: 10.1007/s00401-014-1310-2
Boven, L. A., Van Meurs, M., Van Zwam, M., Wierenga-Wolf, A., Hintzen, R. Q., Boot, R. G., et al. (2006). Myelin-laden macrophages are anti-inflammatory, consistent with foam cells in multiple sclerosis. Brain 129, 517–526. doi: 10.1093/brain/awh707
Bragg, D. C., Boles, J. C., and Meeker, R. B. (2002a). Destabilization of neuronal calcium homeostasis by factors secreted from choroid plexus macrophage cultures in response to feline immunodeficiency virus. Neurobiol. Dis. 9, 173–186. doi: 10.1006/nbdi.2001.0459
Bragg, D. C., Hudson, L. C., Liang, Y. H., Tompkins, M. B., Fernandes, A., and Meeker, R. B. (2002b). Choroid plexus macrophages proliferate and release toxic factors in response to feline immunodeficiency virus. J. Neurovirol. 8, 225–239. doi: 10.1080/13550280290049679
Brendecke, S. M., and Prinz, M. (2015). Do not judge a cell by its cover—diversity of CNS resident, adjoining and infiltrating myeloid cells in inflammation. Semin. Immunopathol. 37, 591–605. doi: 10.1007/s00281-015-0520-6
Breuer, J., Korpos, E., Hannocks, M. J., Schneider-Hohendorf, T., Song, J., Zondler, L., et al. (2018). Blockade of MCAM/CD146 impedes CNS infiltration of T cells over the choroid plexus. J. Neuroinflammation 15, 236. doi: 10.1186/s12974-018-1276-4
Butovsky, O., Landa, G., Kunis, G., Ziv, Y., Avidan, H., Greenberg, N., et al. (2006). Induction and blockage of oligodendrogenesis by differently activated microglia in an animal model of multiple sclerosis. J. Clin. Invest. 116, 905–915. doi: 10.1172/JCI26836
Buttgereit, A., Lelios, I., Yu, X., Vrohlings, M., Krakoski, N. R., Gautier, E. L., et al. (2016). Sall1 is a transcriptional regulator defining microglia identity and function. Nat. Immunol. 17, 1397–1406. doi: 10.1038/ni.3585
Centonze, D., Muzio, L., Rossi, S., Cavasinni, F., De Chiara, V., Bergami, A., et al. (2009). Inflammation triggers synaptic alteration and degeneration in experimental autoimmune encephalomyelitis. J. Neurosci. 29, 3442–3452. doi: 10.1523/JNEUROSCI.5804-08.2009
Centonze, D., Muzio, L., Rossi, S., Furlan, R., Bernardi, G., and Martino, G. (2010). The link between inflammation, synaptic transmission and neurodegeneration in multiple sclerosis. Cell Death Differ. 17, 1083–1091. doi: 10.1038/cdd.2009.179
Cheng, Y., Sun, L., Xie, Z., Fan, X., Cao, Q., Han, J., et al. (2017). Diversity of immune cell types in multiple sclerosis and its animal model: pathological and therapeutic implications. J. Neurosci. Res. 95, 1973–1983. doi: 10.1002/jnr.24023
Choi, S. R., Howell, O. W., Carassiti, D., Magliozzi, R., Gveric, D., Muraro, P. A., et al. (2012). Meningeal inflammation plays a role in the pathology of primary progressive multiple sclerosis. Brain 135, 2925–2937. doi: 10.1093/brain/aws189
Cohen, J. A., Imrey, P. B., Calabresi, P. A., Edwards, K. R., Eickenhorst, T., Felton, W. L., et al. (2009). Results of the avonex combination trial (ACT) in relapsing-remitting MS. Neurology 72, 535–541. doi: 10.1212/01.wnl.0000341934.12142.74
Colonna, M., and Facchetti, F. (2003). TREM-1 (triggering receptor expressed on myeloid cells): a new player in acute inflammatory responses. J. Infect. Dis. 187, S397–S401. doi: 10.1086/374754
Compston, A., and Coles, A. (2008). Multiple sclerosis. Lancet 372, 1502–1517. doi: 10.1016/S0140-6736(08)61620-7
Daneman, R. (2012). The blood-brain barrier in health and disease. Ann. Neurol. 72, 648–672. doi: 10.1002/ana.23648
Das, A., Sinha, M., Datta, S., Abas, M., Chaffee, S., Sen, C. K., et al. (2015). Monocyte and macrophage plasticity in tissue repair and regeneration. Am. J. Pathol. 185, 2596–2606. doi: 10.1016/j.ajpath.2015.06.001
Datta, G., Colasanti, A., Rabiner, E. A., Gunn, R. N., Malik, O., Ciccarelli, O., et al. (2017). Neuroinflammation and its relationship to changes in brain volume and white matter lesions in multiple sclerosis. Brain 140, 2927–2938. doi: 10.1093/brain/awx228
David, S., and Kroner, A. (2011). Repertoire of microglial and macrophage responses after spinal cord injury. Nat. Rev. Neurosci. 12, 388–399. doi: 10.1038/nrn3053
de Vos, A. F., van Meurs, M., Brok, H. P., Boven, L. A., Hintzen, R. Q., van der Valk, P., et al. (2002). Transfer of central nervous system autoantigens and presentation in secondary lymphoid organs. J. Immunol. 169, 5415–5423. doi: 10.4049/jimmunol.169.10.5415
Denney, L., Kok, W. L., Cole, S. L., Sanderson, S., McMichael, A. J., and Ho, L. P. (2012). Activation of invariant NKT cells in early phase of experimental autoimmune encephalomyelitis results in differentiation of Ly6Chi inflammatory monocyte to M2 macrophages and improved outcome. J. Immunol. 189, 551–557. doi: 10.4049/jimmunol.1103608
Di Filippo, M., Portaccio, E., Mancini, A., and Calabresi, P. (2018). Multiple sclerosis and cognition: synaptic failure and network dysfunction. Nat. Rev. Neurosci. 19, 599–609. doi: 10.1038/s41583-018-0053-9
Djedović, N., Stanisavljevic, S., Jevtić, B., Momčilović, M., Lavrnja, I., and Miljković, D. (2017). Anti-encephalitogenic effects of ethyl pyruvate are reflected in the central nervous system and the gut. Biomed. Pharmacother. 96, 78–85. doi: 10.1016/j.biopha.2017.09.110
Du, L., Zhang, Y., Chen, Y., Zhu, J., Yang, Y., and Zhang, H. L. (2017). Role of microglia in neurological disorders and their potentials as a therapeutic target. Mol. Neurobiol. 54, 7567–7584. doi: 10.1007/s12035-016-0245-0
Edwards, K. R., Goya, J., Plavina, T., Czerkowicz, J., Goelz, S., Ranger, A., et al. (2013). Feasibility of the use of combinatorial chemokine arrays to study blood and CSF in multiple sclerosis. PLoS One 8:e81007. doi: 10.1371/journal.pone.0081007
Engelhardt, B., Wolburg-Buchholz, K., and Wolburg, H. (2001). Involvement of the choroid plexus in central nervous system inflammation. Microsc. Res. Tech. 52, 112–129. doi: 10.1002/1097-0029(20010101)52:1<112::AID-JEMT13>3.0.CO;2-5
Eyo, U. B., Peng, J., Murugan, M., Mo, M., Lalani, A., Xie, P., et al. (2016). Regulation of physical microglia–neuron interactions by fractalkine signaling after status epilepticus. eNeuro 3:ENEURO.209–ENEURO.216. doi: 10.1523/ENEURO.0209-16.2016
Fabriek, B. O., Van Haastert, E. S., Galea, I., Polfliet, M. M., Döpp, E. D., Van Den Heuvel, M. M., et al. (2005). CD163-positive perivascular macrophages in the human CNS express molecules for antigen recognition and presentation. Glia 51, 297–305. doi: 10.1002/glia.20208
Fan, C., Long, R., You, Y., Wang, J., Yang, X., Huang, S., et al. (2018). A novel PADRE-Kv1.3 vaccine effectively induces therapeutic antibodies and ameliorates experimental autoimmune encephalomyelitis in rats. Clin. Immunol. 193, 98–109. doi: 10.1016/j.clim.2018.02.012
Fischbach, M. A., Bluestone, J. A., and Lim, W. A. (2013). Cell-based therapeutics: the next pillar of medicine. Sci. Transl. Med. 5:179s7. doi: 10.1126/scitranslmed.3005568
Forde, E. A., Dogan, R. N. E., and Karpus, W. J. (2011). CCR4 contributes to the pathogenesis of experimental autoimmune encephalomyelitis by regulating inflammatory macrophage function. J. Neuroimmunol. 236, 17–26. doi: 10.1016/j.jneuroim.2011.04.008
Galli, S. J., Borregaard, N., and Wynn, T. A. (2011). Phenotypic and functional plasticity of cells of innate immunity: macrophages, mast cells and neutrophils. Nat. Immunol. 12, 1035–1044. doi: 10.1038/ni.2109
Gao, H., Danzi, M. C., Choi, C. S., Taherian, M., Dalby-Hansen, C., Ellman, D. G., et al. (2017). Opposing functions of microglial and macrophagic TNFR2 in the pathogenesis of experimental autoimmune encephalomyelitis. Cell Rep. 18, 198–212. doi: 10.1016/j.celrep.2016.11.083
Garabedian, B. V., Lemaigre-Dubreuil, Y., and Mariani, J. (2000). Central origin of IL-1β produced during peripheral inflammation: role of meninges. Brain Res. Mol. Brain Res. 75, 259–263. doi: 10.1016/S0169-328X(99)00320-4
Goldmann, T., Wieghofer, P., Jordão, M. J., Prutek, F., Hagemeyer, N., Frenzel, K., et al. (2016). Origin, fate and dynamics of macrophages at CNS interfaces. Nat. Immunol. 17, 797–805. doi: 10.1038/ni.3423
Gomez Perdiguero, E., Klapproth, K., Schulz, C., Busch, K., Azzoni, E., Crozet, L., et al. (2015). Tissue-resident macrophages originate from yolk-sac-derived erythro-myeloid progenitors. Nature 518, 547–551. doi: 10.1038/nature13989
Gordon, E. J., Rao, S., Pollard, J. W., Nutt, S. L., Lang, R. A., and Harvey, N. L. (2011). Macrophages define dermal lymphatic vessel calibre during development by regulating lymphatic endothelial cell proliferation. Development 137, 3899–3910. doi: 10.1242/dev.050021
Graeber, M. B., and Streit, W. J. (2010). Microglia: biology and pathology. Acta Neuropathol. 119, 89–105. doi: 10.1007/s00401-009-0622-0
Grajchen, E., Hendriks, J. J. A., and Bogie, J. F. J. (2018). The physiology of foamy phagocytes in multiple sclerosis. Acta Neuropathol. Commun. 6:124. doi: 10.1186/s40478-018-0628-8
GrandPré, T., Nakamura, F., Vartanlan, T., and Strittmatter, S. M. (2000). Identification of the Nogo inhibitor of axon regeneration as a Reticulon protein. Nature 403, 439–444. doi: 10.1038/35000226
Greter, M., Heppner, F. L., Lemos, M. P., Odermatt, B. M., Goebels, N., Laufer, T., et al. (2005). Dendritic cells permit immune invasion of the CNS in an animal model of multiple sclerosis. Nat. Med. 11, 328–334. doi: 10.1038/nm1197
Hagemeyer, N., Hanft, K. M., Akriditou, M. A., Unger, N., Park, E. S., Stanley, E. R., et al. (2017). Microglia contribute to normal myelinogenesis and to oligodendrocyte progenitor maintenance during adulthood. Acta Neuropathol. 134, 441–458. doi: 10.1007/s00401-017-1747-1
Haile, Y., Deng, X., Ortiz-Sandoval, C., Tahbaz, N., Janowicz, A., Lu, J. Q., et al. (2017). Rab32 connects ER stress to mitochondrial defects in multiple sclerosis. J. Neuroinflammation 14:19. doi: 10.1186/s12974-016-0788-z
Hanisch, U. K., and Kettenmann, H. (2007). Microglia: active sensor and versatile effector cells in the normal and pathologic brain. Nat. Neurosci. 10, 1387–1394. doi: 10.1038/nn1997
Harrison, S. J., Nishinakamura, R., Jones, K. R., and Monaghan, A. P. (2012). Sall1 regulates cortical neurogenesis and laminar fate specification in mice: implications for neural abnormalities in Townes-Brocks syndrome. Dis. Model. Mech. 5, 351–365. doi: 10.1242/dmm.002873
He, H., Mack, J. J., Güç, E., Warren, C. M., Squadrito, M. L., Kilarski, W. W., et al. (2016). Perivascular macrophages limit permeability. Arterioscler. Thromb. Vasc. Biol. 36, 2203–2212. doi: 10.1161/ATVBAHA.116.307592
Herz, J., Filiano, A. J., Smith, A., Yogev, N., and Kipnis, J. (2017). Myeloid cells in the central nervous system. Immunity 46, 943–956. doi: 10.1016/j.immuni.2017.06.007
Hikawa, N., and Takenaka, T. (1996). Myelin-stimulated macrophages release neurotrophic factors for adult dorsal root ganglion neurons in culture. Cell. Mol. Neurobiol. 16, 517–528. doi: 10.1007/BF02150231
Hoeffel, G., Chen, J., Lavin, Y., Low, D., Almeida, F. F., See, P., et al. (2015). C-Myb(+) erythro-myeloid progenitor-derived fetal monocytes give rise to adult tissue-resident macrophages. Immunity 42, 665–678. doi: 10.1016/j.immuni.2015.03.011
Hofmann, N., Lachnit, N., Streppel, M., Witter, B., Neiss, W. F., Guntinas-Lichius, O., et al. (2002). Increased expression of ICAM-1, VCAM-1, MCP-1, and MIP-1α by spinal perivascular macrophages during experimental allergic encephalomyelitis in rats. BMC Immunol. 3:11. doi: 10.1186/1471-2172-3-11
Hong, S., Beja-Glasser, V. F., Nfonoyim, B. M., Frouin, A., Li, S., Ramakrishnan, S., et al. (2016). Complement and microglia mediate early synapse loss in Alzheimer mouse models. Science 352, 712–716. doi: 10.1126/science.aad8373
Howell, O. W., Reeves, C. A., Nicholas, R., Carassiti, D., Radotra, B., Gentleman, S. M., et al. (2011). Meningeal inflammation is widespread and linked to cortical pathology in multiple sclerosis. Brain 134, 2755–2771. doi: 10.1093/brain/awr182
Jiang, W., Li, D., Han, R., Zhang, C., Jin, W. N., Wood, K., et al. (2017). Acetylcholine-producing NK cells attenuate CNS inflammation via modulation of infiltrating monocytes/macrophages. Proc. Natl. Acad. Sci. U.S.A. 114, E6202–E6211. doi: 10.1073/pnas.1705491114
Jiang, Z., Jiang, J. X., and Zhang, G. X. (2014). Macrophages: a double-edged sword in experimental autoimmune encephalomyelitis. Immunol. Lett. 160, 17–22. doi: 10.1016/j.imlet.2014.03.006
Juhas, U., Ryba-Stanisławowska, M., Szargiej, P., and Myśliwska, J. (2015). Different pathways of macrophage activation and polarization. Postepy Hig. Med. Dosw. 69, 496–502. doi: 10.5604/17322693.1150133
Kapellos, T. S., and Iqbal, A. J. (2016). Epigenetic control of macrophage polarisation and soluble mediator gene expression during inflammation. Mediators Inflamm. 2016:6591703. doi: 10.1155/2016/6591703
Karamita, M., Barnum, C., Möbius, W., Tansey, M. G., Szymkowski, D. E., Lassmann, H., et al. (2017). Therapeutic inhibition of soluble brain TNF promotes remyelination by increasing myelin phagocytosis by microglia. JCI Insight 2:87455. doi: 10.1172/jci.insight.87455
Kaur, C., Rathnasamy, G., and Ling, E. A. (2016). The choroid plexus in healthy and diseased brain. J. Neuropathol. Exp. Neurol. 75, 198–213. doi: 10.1093/jnen/nlv030
Kettenmann, H., Hanisch, U. K., Noda, M., and Verkhratsky, A. (2011). Physiology of microglia. Physiol. Rev. 91, 461–553. doi: 10.1152/physrev.00011.2010
Kettenmann, H., Kirchhoff, F., and Verkhratsky, A. (2013). Microglia: new roles for the synaptic stripper. Neuron. 77, 10–18. doi: 10.1016/j.neuron.2012.12.023
Kierdorf, K., Erny, D., Goldmann, T., Sander, V., Schulz, C., Perdiguero, E. G., et al. (2013). Microglia emerge from erythromyeloid precursors via Pu.1-and Irf8-dependent pathways. Nat. Neurosci. 16, 273–280. doi: 10.1038/nn.3318
Kilsdonk, I. D., Schoonheim, M., and Wattjes, M. P. (2017). In-vivo imaging of meningeal inflammation in multiple sclerosis: presence of evidence or evidence of presence? Mult. Scler. 23, 1169–1171. doi: 10.1177/1352458517704924
Kivisakk, P., Imitola, J., Rasmussen, S., Elyaman, W., Zhu, B., Ransohoff, R. M., et al. (2009). Localizing central nervous system immune surveillance: meningeal antigen-presenting cells activate T cells during experimental autoimmune encephalomyelitis. Ann. Neurol. 65, 457–469. doi: 10.1002/ana.21379
Koeniger, T., and Kuerten, S. (2017). Splitting the “unsplittable”: dissecting resident and infiltrating macrophages in experimental autoimmune encephalomyelitis. Int. J. Mol. Sci. 18:E2072. doi: 10.3390/ijms18102072
Kolber, P., Droby, A., Roebroeck, A., Goebel, R., Fleischer, V., Groppa, S., et al. (2017). A “kissing lesion”: in-vivo 7T evidence of meningeal inflammation in early multiple sclerosis. Mult. Scler. 23, 1167–1169. doi: 10.1177/1352458516683267
Konishi, H., Kobayashi, M., Kunisawa, T., Imai, K., Sayo, A., Malissen, B., et al. (2017). Siglec-H is a microglia-specific marker that discriminates microglia from CNS-associated macrophages and CNS-infiltrating monocytes. Glia 65, 1927–1943. doi: 10.1002/glia.23204
Kornberg, M. D., Smith, M. D., Shirazi, H. A., Calabresi, P. A., Snyder, S. H., and Kim, P. M. (2018). Bryostatin-1 alleviates experimental multiple sclerosis. Proc. Natl. Acad. Sci. U.S.A. 115, 2186–2191. doi: 10.1073/pnas.1719902115
Koso, H., Nishinakamura, R., and Watanabe, S. (2018). Sall1 regulates microglial morphology cell autonomously in the developing retina. Adv. Exp. Med. Biol. 1074, 209–215. doi: 10.1007/978-3-319-75402-4_26
Kotter, M. R., Li, W. W., Zhao, C., and Franklin, R. J. (2006). Myelin impairs CNS remyelination by inhibiting oligodendrocyte precursor cell differentiation. J. Neurosci. 26, 328–332. doi: 10.1523/JNEUROSCI.2615-05.2006
Kotter, M. R., Zhao, C., Van Rooijen, N., and Franklin, R. J. (2005). Macrophage-depletion induced impairment of experimental CNS remyelination is associated with a reduced oligodendrocyte progenitor cell response and altered growth factor expression. Neurobiol. Dis. 18, 166–175. doi: 10.1016/j.nbd.2004.09.019
Krausgruber, T., Blazek, K., Smallie, T., Alzabin, S., Lockstone, H., Sahgal, N., et al. (2011). IRF5 promotes inflammatory macrophage polarization and TH1-TH17 responses. Nat. Immunol. 12, 231–238. doi: 10.1038/ni.1990
Kuhlmann, T., Ludwin, S., Prat, A., Antel, J., Brück, W., and Lassmann, H. (2017). An updated histological classification system for multiple sclerosis lesions. Acta Neuropathol. 133, 13–24. doi: 10.1007/s00401-016-1653-y
Lassmann, H., Brück, W., and Lucchinetti, C. (2001). Heterogeneity of multiple sclerosis pathogenesis: implications for diagnosis and therapy. Trends Mol. Med. 7, 115–121. doi: 10.1016/S1471-4914(00)01909-2
Lemus, H. N., Warrington, A. E., and Rodriguez, M. (2018). Multiple sclerosis: mechanisms of disease and strategies for myelin and axonal repair. Neurol. Clin. 36, 1–11. doi: 10.1016/j.ncl.2017.08.002
Li, H., Cuzner, M. L., and Newcombe, J. (1996). Microglia-derived macrophages in early multiple sclerosis plaques. Neuropathol. Appl. Neurobiol. 22, 207–215. doi: 10.1111/j.1365-2990.1996.tb00896.x
Li, Q., and Barres, B. A. (2018). Microglia and macrophages in brain homeostasis and disease. Nat. Rev. Immunol. 18, 225–242. doi: 10.1038/nri.2017.125
Li, Q., Lan, X., Han, X., and Wang, J. (2019). Expression of Tmem119/Sall1 and Ccr2/CD69 in FACS-sorted microglia- and monocyte/macrophage-enriched cell populations after intracerebral hemorrhage. Front. Cell. Neurosci. 12:520. doi: 10.3389/fncel.2018.00520
Liddelow, S. A. (2015). Development of the choroid plexus and blood-CSF barrier. Front. Neurosci. 9:32. doi: 10.3389/fnins.2015.00032
Liddelow, S. A., Guttenplan, K. A., Clarke, L. E., Bennett, F. C., Bohlen, C. J., Schirmer, L., et al. (2017). Neurotoxic reactive astrocytes are induced by activated microglia. Nature 541, 481–487. doi: 10.1038/nature21029
Lima, I. V., Bastos, L. F., Limborço-Filho, M., Fiebich, B. L., and de Oliveira, A. C. (2012). Role of prostaglandins in neuroinflammatory and neurodegenerative diseases. Mediators Inflamm. 2012:946813. doi: 10.1155/2012/946813
Liu, C., Li, Y., Yu, J., Feng, L., Hou, S., Liu, Y., et al. (2013). Targeting the shift from M1 to M2 macrophages in experimental autoimmune encephalomyelitis mice treated with fasudil. PLoS One 8:e54841. doi: 10.1371/journal.pone.0054841
Liu, Y., Hao, W., Letiembre, M., Walter, S., Kulanga, M., Neumann, H., et al. (2006). Suppression of microglial inflammatory activity by myelin phagocytosis: role of p47-PHOX-mediated generation of reactive oxygen species. J. Neurosci. 26, 12904–12913. doi: 10.1523/JNEUROSCI.2531-06.2006
Lopez-Atalaya, J. P., Askew, K. E., Sierra, A., and Gomez-Nicola, D. (2018). Development and maintenance of the brain’s immune toolkit: microglia and non-parenchymal brain macrophages. Dev. Neurobiol. 78, 561–579. doi: 10.1002/dneu.22545
Louveau, A., Smirnov, I., Keyes, T. J., Eccles, J. D., Rouhani, S. J., Peske, J. D., et al. (2015). Structural and functional features of central nervous system lymphatic vessels. Nature 523, 337–341. doi: 10.1038/nature14432
Lucchinetti, C., Brück, W., Parisi, J., Scheithauer, B., Rodriguez, M., and Lassmann, H. (2000). Heterogeneity of multiple sclerosis lesions: implications for the pathogenesis of demyelination. Ann. Neurol. 47, 707–717. doi: 10.1002/1531-8249(200006)47:6<707::AID-ANA3>3.0.CO;2-Q
Mammana, S., Fagone, P., Cavalli, E., Basile, M. S., Petralia, M. C., Nicoletti, F., et al. (2018). The role of macrophages in neuroinflammatory and neurodegenerative pathways of Alzheimer’s disease, amyotrophic lateral sclerosis, and multiple sclerosis: pathogenetic cellular effectors and potential therapeutic targets. Int. J. Mol. Sci. 19:E831. doi: 10.3390/ijms19030831
Marques, F., Sousa, J. C., Brito, M. A., Pahnke, J., Santos, C., Correia-Neves, M., et al. (2017). The choroid plexus in health and in disease: dialogues into and out of the brain. Neurobiol. Dis. 107, 32–40. doi: 10.1016/j.nbd.2016.08.011
Martin, E., El-Behi, M., Fontaine, B., and Delarasse, C. (2017). Analysis of microglia and monocyte-derived macrophages from the central nervous system by flow cytometry. J. Vis. Exp. 124:e55781. doi: 10.3791/55781
Matcovitch-Natan, O., Winter, D. R., Giladi, A., Vargas Aguilar, S., Spinrad, A., Sarrazin, S., et al. (2016). Microglia development follows a stepwise program to regulate brain homeostasis. Science 353:aad8670. doi: 10.1126/science.aad8670
Maxwell, W. L., Follows, R., Ashhurst, D. E., and Berry, M. (1990). The response of the cerebral hemisphere of the rat to injury. II. The neonatal rat. Philos. Trans. R. Soc. Lond. B. Biol. Sci. 328, 501–513. doi: 10.1098/rstb.1990.0122
McMahon, E. J., Bailey, S. L., Castenada, C. V., Waldner, H., and Miller, S. D. (2005). Epitope spreading initiates in the CNS in two mouse models of multiple sclerosis. Nat. Med. 11, 335–339. doi: 10.1038/nm1202
Mendes-Jorge, L., Ramos, D., Luppo, M., Llombart, C., Alexandre-Pires, G., Nacher, V., et al. (2009). Scavenger function of resident autofluorescent perivascular macrophages and their contribution to the maintenance of the blood-retinal barrier. Invest. Ophthalmol. Vis. Sci. 50, 5997–6005. doi: 10.1167/iovs.09-3515
Merson, T. D., Binder, M. D., and Kilpatrick, T. J. (2010). Role of cytokines as mediators and regulators of microglial activity in inflammatory demyelination of the CNS. Neuromolecular Med. 12, 99–132. doi: 10.1007/s12017-010-8112-z
Miao, X., Leng, X., and Zhang, Q. (2017). The current state of nanoparticle-induced macrophage polarization and reprogramming research. Int. J. Mol. Sci. 18:E336. doi: 10.3390/ijms18020336
Michailidou, I., Willems, J. G., Kooi, E. J., Van Eden, C., Gold, S. M., Geurts, J. J., et al. (2015). Complement C1q-C3-associated synaptic changes in multiple sclerosis hippocampus. Ann. Neurol. 77, 1007–1026. doi: 10.1002/ana.24398
Mikita, J., Dubourdieu-Cassagno, N., Deloire, M. S., Vekris, A., Biran, M., Raffard, G., et al. (2011). Altered M1/M2 activation patterns of monocytes in severe relapsing experimental rat model of multiple sclerosis. Amelioration of clinical status by M2 activated monocyte administration. Mult. Scler. 17, 2–15. doi: 10.1177/1352458510379243
Mildner, A., Huang, H., Radke, J., Stenzel, W., and Priller, J. (2017). P2Y12 receptor is expressed on human microglia under physiological conditions throughout development and is sensitive to neuroinflammatory diseases. Glia 65, 375–387. doi: 10.1002/glia.23097
Miron, V. E., Boyd, A., Zhao, J. W., Yuen, T. J., Ruckh, J. M., Shadrach, J. L., et al. (2013). M2 microglia and macrophages drive oligodendrocyte differentiation during CNS remyelination. Nat. Neurosci. 16, 1211–1218. doi: 10.1038/nn.3469
Mitchell, K., Yang, H. Y., Berk, J. D., Tran, J. H., and Iadarola, M. J. (2009). Monocyte chemoattractant protein-1 in the choroid plexus: a potential link between vascular pro-inflammatory mediators and the CNS during peripheral tissue inflammation. Neuroscience 158, 885–895. doi: 10.1016/j.neuroscience.2008.10.047
Miyamoto, A., Wake, H., Ishikawa, A. W., Eto, K., Shibata, K., Murakoshi, H., et al. (2016). Microglia contact induces synapse formation in developing somatosensory cortex. Nat. Commun. 7:12540. doi: 10.1038/ncomms12540
Montarolo, F., Perga, S., Martire, S., Brescia, F., Caldano, M., Lo Re, M., et al. (2018). Study of the NR4A family gene expression in patients with multiple sclerosis treated with Fingolimod. Eur. J. Neurol. 26, 667–672. doi: 10.1111/ene.13875
Montes-Cobos, E., Ring, S., Fischer, H. J., Heck, J., Strauß, J., Schwaninger, M., et al. (2017a). Targeted delivery of glucocorticoids to macrophages in a mouse model of multiple sclerosis using inorganic-organic hybrid nanoparticles. J. Control. Release 245, 157–169. doi: 10.1016/j.jconrel.2016.12.003
Montes-Cobos, E., Schweingruber, N., Li, X., Fischer, H. J., Reichardt, H. M., and Lühder, F. (2017b). Deletion of the mineralocorticoid receptor in myeloid cells attenuates central nervous system autoimmunity. Front. Immunol. 8:1319. doi: 10.3389/fimmu.2017.01319
Mrdjen, D., Pavlovic, A., Hartmann, F. J., Schreiner, B., Utz, S. G., Leung, B. P., et al. (2018). High-dimensional single-cell mapping of central nervous system immune cells reveals distinct myeloid subsets in health, aging, and disease. Immunity 48, 380–395.e6. doi: 10.1016/j.immuni.2018.01.011
Murphy, A. C., Lalor, S. J., Lynch, M. A., and Mills, K. H. (2010). Infiltration of Th1 and Th17 cells and activation of microglia in the CNS during the course of experimental autoimmune encephalomyelitis. Brain. Behav. Immun. 24, 641–651. doi: 10.1016/j.bbi.2010.01.014
Murray, P. J., Allen, J. E., Biswas, S. K., Fisher, E. A., Gilroy, D. W., Goerdt, S., et al. (2014). Macrophage activation and polarization: nomenclature and experimental guidelines. Immunity 41, 14–20. doi: 10.1016/j.immuni.2014.06.008
Nahrendorf, M., Swirski, F. K., Aikawa, E., Stangenberg, L., Wurdinger, T., Figueiredo, J. L., et al. (2007). The healing myocardium sequentially mobilizes two monocyte subsets with divergent and complementary functions. J. Exp. Med. 204, 3037–3047. doi: 10.1084/jem.20070885
Oh, U., Fujita, M., Ikonomidou, V. N., Evangelou, I. E., Matsuura, E., Harberts, E., et al. (2011). Translocator protein PET imaging for glial activation in multiple sclerosis. J. Neuroimmune Pharmacol. 6, 354–361. doi: 10.1007/s11481-010-9243-6
Paolicelli, R. C., Bolasco, G., Pagani, F., Maggi, L., Scianni, M., Panzanelli, P., et al. (2011). Synaptic pruning by microglia is necessary for normal brain development. Science 333, 1456–1458.
Parkhurst, C. N., Yang, G., Ninan, I., Savas, J. N., Yates, J. R., Lafaille, J. J., et al. (2014). Microglia promote learning-dependent synapse formation through BDNF. Cell 155, 1596–1609. doi: 10.1016/j.cell.2013.11.030
Pato-Pato, A., Prieto, J. M., Lema, M., Dapena-Bolaño, D., Abella-Corral, J., and Pumar, J. M. (2003). [Cerebral atrophy in multiple sclerosis patients treated periodically with boluses of methylprednisolone]. Rev. Neurol. 37, 501–506.
Pérez-Martín, M., Cifuentes, M., Grondona, J. M., López-Avalos, M. D., Gómez-Pinedo, U., García-Verdugo, J. M., et al. (2010). IGF-I stimulates neurogenesis in the hypothalamus of adult rats. Eur. J. Neurosci. 31, 1533–1548. doi: 10.1111/j.1460-9568.2010.07220.x
Perry, V. H. (1998). A revised view of the central nervous system microenvironment and major histocompatibility complex class II antigen presentation. J. Neuroimmunol. 90, 113–121. doi: 10.1016/S0165-5728(98)00145-3
Piccio, L., Buonsanti, C., Mariani, M., Cella, M., Gilfillan, S., Cross, A. H., et al. (2007). Blockade of TREM-2 exacerbates experimental autoimmune encephalomyelitis. Eur. J. Immunol. 37, 1290–1301. doi: 10.1002/eji.200636837
Polfliet, M. M., van de Veerdonk, F., Döpp, E. A., van Kesteren-Hendrikx, E. M., van Rooijen, N., Dijkstra, C. D., et al. (2002). The role of perivascular and meningeal macrophages in experimental allergic encephalomyelitis. J. Neuroimmunol. 122, 1–8. doi: 10.1016/S0165-5728(01)00445-3
Polfliet, M. M., Zwijnenburg, P. J., van Furth, A. M., van der Poll, T., Döpp, E. A., Renardel de Lavalette, C., et al. (2001). Meningeal and perivascular macrophages of the central nervous system play a protective role during bacterial meningitis. J. Immunol. 167, 4644–4650. doi: 10.4049/jimmunol.167.8.4644
Poliani, P. L., Wang, Y., Fontana, E., Robinette, M. L., Yamanishi, Y., Gilfillan, S., et al. (2015). TREM2 sustains microglial expansion during aging and response to demyelination. J. Clin. Invest. 125, 2161–2170. doi: 10.1172/JCI77983
Prinz, M., and Priller, J. (2014). Microglia and brain macrophages in the molecular age: from origin to neuropsychiatric disease. Nat. Rev. Neurosci. 15, 300–312. doi: 10.1038/nrn3722
Prinz, M., Priller, J., Sisodia, S. S., and Ransohoff, R. M. (2011). Heterogeneity of CNS myeloid cells and their roles in neurodegeneration. Nat. Neurosci. 14, 1227–1235. doi: 10.1038/nn.2923
Puthenparampil, M., Federle, L., Miante, S., Zito, A., Toffanin, E., Ruggero, S., et al. (2017). BAFF Index and CXCL13 levels in the cerebrospinal fluid associate respectively with intrathecal IgG synthesis and cortical atrophy in multiple sclerosis at clinical onset. J. Neuroinflammation 14:11. doi: 10.1186/s12974-016-0785-2
Ransohoff, R. M., and Cardona, A. E. (2010). The myeloid cells of the central nervous system parenchyma. Nature 468, 253–262. doi: 10.1038/nature09615
Ransohoff, R. M., and El Khoury, J. (2014). Microglia in health and disease. Cold Spring Harb. Perspect. Biol. 8:a020560. doi: 10.1101/cshperspect.a020560
Ransohoff, R. M., and Perry, V. H. (2009). Microglial physiology: unique stimuli, specialized responses. Annu. Rev. Immunol. 27, 119–145. doi: 10.1146/annurev.immunol.021908.132528
Raper, D., Louveau, A., and Kipnis, J. (2016). How do meningeal lymphatic vessels drain the CNS? Trends Neurosci. 39, 581–586. doi: 10.1016/j.tins.2016.07.001
Ratchford, J. N., Endres, C. J., Hammoud, D. A., Pomper, M. G., Shiee, N., McGready, J., et al. (2012). Decreased microglial activation in MS patients treated with glatiramer acetate. J. Neurol. 259, 1199–1205. doi: 10.1007/s00415-011-6337-x
Reuter, U., Bolay, H., Jansen-Olesen, I., Chiarugi, A., Sanchez del Rio, M., Letourneau, R., et al. (2001). Delayed inflammation in rat meninges: implications for migraine pathophysiology. Brain 124, 2490–2502. doi: 10.1093/brain/124.12.2490
Rothhammer, V., Borucki, D. M., Tjon, E. C., Takenaka, M. C., Chao, C. C., Ardura-Fabregat, A., et al. (2018). Microglial control of astrocytes in response to microbial metabolites. Nature 557, 724–728. doi: 10.1038/s41586-018-0119-x
Ruckh, J. M., Zhao, J. W., Shadrach, J. L., van wijngaarden, P., Rao, T. N., Wagers, A. J., et al. (2012). Rejuvenation of regeneration in the aging central nervous system. Cell Stem Cell 10, 96–103. doi: 10.1016/j.stem.2011.11.019
Saederup, N., Cardona, A. E., Croft, K., Mizutani, M., Cotleur, A. C., Tsou, C. L., et al. (2010). Selective chemokine receptor usage by central nervous system myeloid cells in CCR2-red fluorescent protein knock-in mice. PLoS One 5:e13693. doi: 10.1371/journal.pone.0013693
Salter, M. W., and Stevens, B. (2017). Microglia emerge as central players in brain disease. Nat. Med. 23, 1018–1027. doi: 10.1038/nm.4397
Samoilova, E. B., Horton, J. L., Liu, T. S., and Chen, Y. (1998). IL-6-deficient mice are resistant to experimental autoimmune encephalomyelitis: roles of IL-6 in the activation and differentiation of autoreactive T cells. J. Immunol. 161, 6480–6486.
Satoh, J., Kino, Y., Asahina, N., Takitani, M., Miyoshi, J., Ishida, T., et al. (2016). TMEM119 marks a subset of microglia in the human brain. Neuropathology 36, 39–49. doi: 10.1111/neup.12235
Schafer, D. P., and Stevens, B. (2015). Microglia function in central nervous system development and plasticity. Cold Spring Harb. Perspect. Biol. 7:a020545. doi: 10.1101/cshperspect.a020545
Sestito, C., Brevé, J. J. P., van Eggermond, M. C. J. A., Killestein, J., Teunissen, C. E., van Rossum, J., et al. (2017). Monocyte-derived tissue transglutaminase in multiple sclerosis patients: reflecting an anti-inflammatory status and function of the cells? J. Neuroinflammation 14, 257. doi: 10.1186/s12974-017-1035-y
Shaked, I., Hanna, R. N., Shaked, H., Chodaczek, G., Nowyhed, H. N., Tweet, G., et al. (2015). Transcription factor Nr4a1 couples sympathetic and inflammatory cues in CNS-recruited macrophages to limit neuroinflammation. Nat. Immunol. 16, 1228–1234. doi: 10.1038/ni.3321
Shechter, R., London, A., varol, C., Raposo, C., Cusimano, M., Yovel, G., et al. (2009). Infiltrating blood-derived macrophages are vital cells playing an anti-inflammatory role in recovery from spinal cord injury in mice. PLoS Med. 6:e1000113. doi: 10.1371/journal.pmed.1000113
Shechter, R., Miller, O., Yovel, G., Rosenzweig, N., London, A., Ruckh, J., et al. (2013). Recruitment of beneficial M2 macrophages to injured spinal cord is orchestrated by remote brain choroid plexus. Immunity 38, 555–569. doi: 10.1016/j.immuni.2013.02.012
Shi, Y., Pan, C., Auckloo, B. N., Chen, X., Chen, C. T. A., Wang, K., et al. (2017). Stress-driven discovery of a cryptic antibiotic produced by Streptomyces sp. WU20 from Kueishantao hydrothermal vent with an integrated metabolomics strategy. Appl. Microbiol. Biotechnol. 101, 1395–1408. doi: 10.1007/s00253-016-7823-y
Sierra, A., Encinas, J. M., Deudero, J. J., Chancey, J. H., Enikolopov, G., Overstreet-Wadiche, L. S., et al. (2010). Microglia shape adult hippocampal neurogenesis through apoptosis-coupled phagocytosis. Cell Stem Cell 7, 483–495. doi: 10.1016/j.stem.2010.08.014
Singh, S., Metz, I., Amor, S., van der Valk, P., Stadelmann, C., and Brück, W. (2013). Microglial nodules in early multiple sclerosis white matter are associated with degenerating axons. Acta Neuropathol. 125, 595–608. doi: 10.1007/s00401-013-1082-0
Sosa, R. A., Murphey, C., Ji, N., Cardona, A. E., and Forsthuber, T. G. (2013). The kinetics of myelin antigen uptake by myeloid cells in the central nervous system during experimental autoimmune encephalomyelitis. J. Immunol. 191, 5848–5857. doi: 10.4049/jimmunol.1300771
Stephenson, E. L., Mishra, M. K., Moussienko, D., Laflamme, N., Rivest, S., Ling, C. C., et al. (2018). Chondroitin sulfate proteoglycans as novel drivers of leucocyte infiltration in multiple sclerosis. Brain 141, 1094–1110. doi: 10.1093/brain/awy033
Stys, P. K., Zamponi, G. W., Van Minnen, J., and Geurts, J. J. G. (2012). Will the real multiple sclerosis please stand up? Nat. Rev. Neurosci. 13, 507–514. doi: 10.1038/nrn3275
Sun, D., Yu, Z., Fang, X., Liu, M., Pu, Y., Shao, Q., et al. (2017). LncRNA GAS5 inhibits microglial M2 polarization and exacerbates demyelination. EMBO Rep. 18, 1801–1816. doi: 10.15252/embr.201643668
Sutton, C., Brereton, C., Keogh, B., Mills, K. H., and Lavelle, E. C. (2006). A crucial role for interleukin (IL)-1 in the induction of IL-17–producing T cells that mediate autoimmune encephalomyelitis. J. Exp. Med. 203, 1685–1691. doi: 10.1084/jem.20060285
Szepesi, Z., Manouchehrian, O., Bachiller, S., and Deierborg, T. (2018). Bidirectional microglia–neuron communication in health and disease. Front. Cell. Neurosci. 12:323. doi: 10.3389/fncel.2018.00323
Tentillier, N., Etzerodt, A., Olesen, M. N., Rizalar, F. S., Jacobsen, J., Bender, D., et al. (2016). Anti-inflammatory modulation of microglia via CD163-targeted glucocorticoids protects dopaminergic neurons in the 6-OHDA Parkinson’s disease model. J. Neurosci. 36, 9375–9390. doi: 10.1523/JNEUROSCI.1636-16.2016
Terrazas, C., de Dios Ruiz-Rosado, J., Amici, S. A., Jablonski, K. A., Martinez-Saucedo, D., Webb, L. M., et al. (2017). Helminth-induced Ly6Chi monocyte-derived alternatively activated macrophages suppress experimental autoimmune encephalomyelitis. Sci. Rep. 7:40814. doi: 10.1038/srep40814
Thompson, A. J., Baranzini, S. E., Geurts, J., Hemmer, B., and Ciccarelli, O. (2018). Multiple sclerosis. Lancet 391, 1622–1636. doi: 10.1016/S0140-6736(18)30481-1
Tobin, W. O., Costanzi, C., Guo, Y., Parisi, J. E., Weigand, S. D., and Lucchinetti, C. F. (2017). Clinical-radiological-pathological spectrum of central nervous system-idiopathic inflammatory demyelinating disease in the elderly. Mult. Scler. 23, 1204–1213. doi: 10.1177/1352458516675748
Tong, H. I., Kang, W., Shi, Y., Zhou, G., and Lu, Y. (2016). Physiological function and inflamed-brain migration of mouse monocyte-derived macrophages following cellular uptake of superparamagnetic iron oxide nanoparticles-Implication of macrophage-based drug delivery into the central nervous system. Int. J. Pharm. 505, 271–282. doi: 10.1016/j.ijpharm.2016.03.028
Torres-Platas, S. G., Cruceanu, C., Chen, G. G., Turecki, G., and Mechawar, N. (2014). Evidence for increased microglial priming and macrophage recruitment in the dorsal anterior cingulate white matter of depressed suicides. Brain Behav. Immun. 42, 50–59. doi: 10.1016/j.bbi.2014.05.007
Trapp, B. D., Peterson, J., Ransohoff, R. M., Rudick, R., Mörk, S., and Bö, L. (1998). Axonal transection in the lesions of multiple sclerosis. N. Engl. J. Med. 338, 278–285. doi: 10.1056/NEJM199801293380502
Trebst, C., Sørensen, T. L., Kivisäkk, P., Cathcart, M. K., Hesselgesser, J., Horuk, R., et al. (2001). CCR1+/CCR5+ mononuclear phagocytes accumulate in the central nervous system of patients with multiple sclerosis. Am. J. Pathol. 159, 1701–1710. doi: 10.1016/S0002-9440(10)63017-9
Tremblay, M. Ě, Lowery, R. L., and Majewska, A. K. (2010). Microglial interactions with synapses are modulated by visual experience. PLoS Biol. 8:e1000527. doi: 10.1371/journal.pbio.1000527
Tsutsui, M., Hirase, R., Miyamura, S., Nagayasu, K., Nakagawa, T., Mori, Y., et al. (2018). TRPM2 exacerbates central nervous system inflammation in experimental autoimmune encephalomyelitis by increasing production of CXCL2 chemokines. J. Neurosci. 38, 8484–8495. doi: 10.1523/JNEUROSCI.2203-17.2018
Ueno, M., Fujita, Y., Tanaka, T., Nakamura, Y., Kikuta, J., Ishii, M., et al. (2013). Layer v cortical neurons require microglial support for survival during postnatal development. Nat. Neurosci. 16, 543–551. doi: 10.1038/nn.3358
Valentin-Torres, A., Savarin, C., Hinton, D. R., Phares, T. W., Bergmann, C. C., and Stohlman, S. A. (2016). Sustained TNF production by central nervous system infiltrating macrophages promotes progressive autoimmune encephalomyelitis. J. Neuroinflammation 13:46. doi: 10.1186/s12974-016-0513-y
van Zwam, M., Samsom, J. N., Nieuwenhuis, E. E., Melief, M.-J., Wierenga-Wolf, A. F., Dijke, I. E., et al. (2011). Myelin ingestion alters macrophage antigen-presenting function in vitro and in vivo. J. Leukoc. Biol. 90, 123–132. doi: 10.1189/jlb.1209813
Vanderlocht, J., Hellings, N., Hendriks, J. J., Vandenabeele, F., Moreels, M., Buntinx, M., et al. (2006). Leukemia inhibitory factor is produced by myelin-reactive T cells from multiple sclerosis patients and protects against tumor necrosis factor-alpha-induced oligodendrocyte apoptosis. J. Neurosci. Res. 83, 763–774. doi: 10.1002/jnr.20781
Vas, A., Shchukin, Y., Karrenbauer, V. D., Cselényi, Z., Kostulas, K., Hillert, J., et al. (2008). Functional neuroimaging in multiple sclerosis with radiolabelled glia markers: preliminary comparative PET studies with [11C]vinpocetine and [11C]PK11195 in patients. J. Neurol. Sci. 264, 9–17. doi: 10.1016/j.jns.2007.07.018
Vercellino, M., Trebini, C., Capello, E., Mancardi, G. L., Giordana, M. T., and Cavalla, P. (2017). Inflammatory responses in Multiple Sclerosis normal-appearing white matter and in non-immune mediated neurological conditions with wallerian axonal degeneration: a comparative study. J. Neuroimmunol. 312, 49–58. doi: 10.1016/j.jneuroim.2017.09.004
Vercellino, M., Votta, B., Condello, C., Piacentino, C., Romagnolo, A., Merola, A., et al. (2008). Involvement of the choroid plexus in multiple sclerosis autoimmune inflammation: a neuropathological study. J. Neuroimmunol. 199, 133–141. doi: 10.1016/j.jneuroim.2008.04.035
Veremeyko, T., Yung, A. W. Y., Dukhinova, M., Kuznetsova, I. S., Pomytkin, I., Lyundup, A., et al. (2018). Cyclic AMP pathway suppress autoimmune neuroinflammation by inhibiting functions of encephalitogenic CD4 T cells and enhancing M2 macrophage polarization at the site of inflammation. Front. Immunol. 9:50. doi: 10.3389/fimmu.2018.00050
Voet, S., Prinz, M., and van Loo, G. (2018). Microglia in central nervous system inflammation and multiple sclerosis pathology. Trends Mol. Med. 25, 112–123. doi: 10.1016/j.molmed.2018.11.005
Vogel, D. Y., Vereyken, E. J., Glim, J. E., Heijnen, P. D., Moeton, M., van der Valk, P., et al. (2013). Macrophages in inflammatory multiple sclerosis lesions have an intermediate activation status. J. Neuroinflammation 10, 35. doi: 10.1186/1742-2094-10-35
Voss, E. V., Škuljec, J., Gudi, V., Skripuletz, T., Pul, R., Trebst, C., et al. (2012). Characterisation of microglia during de- and remyelination: can they create a repair promoting environment? Neurobiol. Dis. 45, 519–528. doi: 10.1016/j.nbd.2011.09.008
Wang, Q., He, Z., Huang, M., Liu, T., Wang, Y., Xu, H., et al. (2018). Vascular niche IL-6 induces alternative macrophage activation in glioblastoma through HIF-2α. Nat. Commun. 9:559. doi: 10.1038/s41467-018-03050-0
Weber, M. S., Prod’homme, T., Youssef, S., Dunn, S. E., Rundle, C. D., Lee, L., et al. (2007). Type II monocytes modulate T cell-mediated central nervous system autoimmune disease. Nat. Med. 13, 935–943. doi: 10.1038/nm1620
Welberg, L. (2014). A synaptic role for microglia. Nat. Rev. Neurosci. 15, 68–69. doi: 10.1038/nrn3678
Weng, Q., Wang, J., Wang, J., Wang, J., Sattar, F., Zhang, Z., et al. (2018). Lenalidomide regulates CNS autoimmunity by promoting M2 macrophages polarization article. Cell Death Dis. 9:251. doi: 10.1038/s41419-018-0290-x
Wisnieff, C., Ramanan, S., Olesik, J., Gauthier, S., Wang, Y., and Pitt, D. (2015). Quantitative susceptibility mapping (QSM) of white matter multiple sclerosis lesions: interpreting positive susceptibility and the presence of iron. Magn. Reson. Med. 74, 564–570. doi: 10.1002/mrm.25420
Wolburg, H., and Paulus, W. (2010). Choroid plexus: biology and pathology. Acta Neuropathol. 119, 75–88. doi: 10.1007/s00401-009-0627-8
Wolf, Y., Shemer, A., Levy-Efrati, L., Gross, M., Kim, J. S., Engel, A., et al. (2018). Microglial MHC class II is dispensable for experimental autoimmune encephalomyelitis and cuprizone-induced demyelination. Eur. J. Immunol. 48, 1308–1318. doi: 10.1002/eji.201847540
Wolf, Y., Shemer, A., Polonsky, M., Gross, M., Mildner, A., Yona, S., et al. (2017). Autonomous TNF is critical for in vivo monocyte survival in steady state and inflammation. J. Exp. Med. 214, 905–917. doi: 10.1084/jem.20160499
Wu, Z., Hayashi, Y., Zhang, J., and Nakanishi, H. (2007). Involvement of prostaglandin E2 released from leptomeningeal cells in increased expression of transforming growth factor-beta in glial cells and cortical neurons during systemic inflammation. J. Neurosci. Res. 85, 184–192. doi: 10.1002/jnr.21100
Yamamoto, S., Muramatsu, M., Azuma, E., Ikutani, M., Nagai, Y., Sagara, H., et al. (2017). A subset of cerebrovascular pericytes originates from mature macrophages in the very early phase of vascular development in CNS. Sci. Rep. 7:3855. doi: 10.1038/s41598-017-03994-1
Yamasaki, R., Lu, H., Butovsky, O., Ohno, N., Rietsch, A. M., Cialic, R., et al. (2014). Differential roles of microglia and monocytes in the inflamed central nervous system. J. Exp. Med. 211, 1533–1549. doi: 10.1084/jem.20132477
Yang, Q., Zheng, C., Cao, J., Cao, G., Shou, P., Lin, L., et al. (2016). Spermidine alleviates experimental autoimmune encephalomyelitis through inducing inhibitory macrophages. Cell Death Differ. 23, 1850–1861. doi: 10.1038/cdd.2016.71
Ye, Z. P., Ai, X. L., Faramand, A. M., and Fang, F. (2018). Macrophages as nanocarriers for drug delivery: novel therapeutics for central nervous system diseases. J. Nanosci. Nanotechnol. 18, 471–485. doi: 10.1166/jnn.2018.15218
Yirmiya, R., Rimmerman, N., and Reshef, R. (2015). Depression as a microglial disease. Trends Neurosci. 38, 637–658. doi: 10.1016/j.tins.2015.08.001
Yuan, D., Zhao, Y., Banks, W. A., Bullock, K. M., Haney, M., Batrakova, E., et al. (2017). Macrophage exosomes as natural nanocarriers for protein delivery to inflamed brain. Biomaterials 142, 1–12. doi: 10.1016/j.biomaterials.2017.07.011
Zabala, A., Vazquez-Villoldo, N., Rissiek, B., Gejo, J., Martin, A., Palomino, A., et al. (2018). P2X4 receptor controls microglia activation and favors remyelination in autoimmune encephalitis. EMBO Mol. Med. 10:e8743. doi: 10.15252/emmm.201708743
Zéphir, H. (2018). Progress in understanding the pathophysiology of multiple sclerosis. Rev. Neurol. 174, 358–363. doi: 10.1016/j.neurol.2018.03.006
Zhang, J., Raper, A., Sugita, N., Hingorani, R., Salio, M., Palmowski, M. J., et al. (2006). Characterization of Siglec-H as a novel endocytic receptor expressed on murine plasmacytoid dendritic cell precursors. Blood 107, 3600–3608. doi: 10.1182/blood-2005-09-3842
Zhang, Z., Zhang, Z. Y., Schittenhelm, J., Wu, Y., Meyermann, R., and Schluesener, H. J. (2011). Parenchymal accumulation of CD163+ macrophages/microglia in multiple sclerosis brains. J. Neuroimmunol. 237, 73–79. doi: 10.1016/j.jneuroim.2011.06.006
Keywords: multiple sclerosis, microglia, macrophages, central nervous system, targeted therapy
Citation: Wang J, Wang J, Wang J, Yang B, Weng Q and He Q (2019) Targeting Microglia and Macrophages: A Potential Treatment Strategy for Multiple Sclerosis. Front. Pharmacol. 10:286. doi: 10.3389/fphar.2019.00286
Received: 04 December 2018; Accepted: 08 March 2019;
Published: 22 March 2019.
Edited by:
Pietro Giusti, University of Padua, ItalyReviewed by:
Inge Huitinga, Netherlands Institute for Neuroscience (KNAW), NetherlandsIrena Lavrnja, University of Belgrade, Serbia
Copyright © 2019 Wang, Wang, Wang, Yang, Weng and He. This is an open-access article distributed under the terms of the Creative Commons Attribution License (CC BY). The use, distribution or reproduction in other forums is permitted, provided the original author(s) and the copyright owner(s) are credited and that the original publication in this journal is cited, in accordance with accepted academic practice. No use, distribution or reproduction is permitted which does not comply with these terms.
*Correspondence: Qinjie Weng, d2VuZ3FpbmppZUB6anUuZWR1LmNu Qiaojun He, cWlhb2p1bmhlQHpqdS5lZHUuY24=