- 1Department of Biomedical Sciences, College of Graduate Studies, Midwestern University, Downers Grove, IL, United States
- 2Department of Ophthalmology & Visual Sciences, University of Illinois at Chicago, Chicago, IL, United States
- 3Chicago College of Pharmacy, Midwestern University, Downers Grove, IL, United States
- 4Chicago College of Osteopathic Medicine, Midwestern University, Downers Grove, IL, United States
- 5Department of Microbiology & Immunology, College of Graduate Studies, Midwestern University, Downers Grove, IL, United States
- 6Department of Medicinal Chemistry and Institute for Structural Biology, Drug Discovery and Development, Virginia Commonwealth University, Richmond, VA, United States
An extraordinary binding site generated in heparan sulfate (HS) structures, during its biosynthesis, provides a unique opportunity to interact with multiple protein ligands including viral proteins, and therefore adds tremendous value to this master molecule. An example of such a moiety is the sulfation at the C3 position of glucosamine residues in HS chain via 3-O sulfotransferase (3-OST) enzymes, which generates a unique virus-cell fusion receptor during herpes simplex virus (HSV) entry and spread. Emerging evidence now suggests that the unique patterns in HS sulfation assist multiple viruses in invading host cells at various steps of their life cycles. In addition, sulfated-HS structures are known to assist in invading host defense mechanisms and initiating multiple inflammatory processes; a critical event in the disease development. All these processes are detrimental for the host and therefore raise the question of how HS-sulfation is regulated. Epigenetic modulations have been shown to be implicated in these reactions during HSV infection as well as in HS modifying enzyme sulfotransferases, and therefore pose a critical component in answering it. Interestingly, heparanase (HPSE) activity is shown to be upregulated during virus infection and multiple other diseases assisting in virus replication to promote cell and tissue damage. These phenomena suggest that sulfotransferases and HPSE serve as key players in extracellular matrix remodeling and possibly generating unique signatures in a given disease. Therefore, identifying the epigenetic regulation of OST genes, and HPSE resulting in altered yet specific sulfation patterns in HS chain during virus infection, will be a significant a step toward developing potential diagnostic markers and designing novel therapies.
Introduction
Heparan sulfate (HS) is a linear polysaccharide ubiquitously present in all tissues. HS is attached to the cell surface or extracellular matrix proteins where it exists as heparan sulfate proteoglycans (HSPGs). Because of its unique structural capability, HSPGs serve as “heavy duty engines” by interacting with an array of multiple and diverse protein ligands to participate in virtually any given biological reaction (Yanagishita and Hascall, 1992; Sasisekharan and Venkataraman, 2000; Esko and Lindahl, 2001; Varki, 2002; Bishop et al., 2007). HS undergoes numerous modifications during its biosynthesis. One family of enzymes called sulfotransferases, which catalyzes sulfonation specific sites on HS and are recognized to play various important roles ranging from impacting cellular processes to microbial pathogenesis and associated inflammations. Infectious disease literature documents two-types of HS for their contributions in viral infections. In the first category, the plain-type or unmodified form of HS contains specific types or arrangements of sulfated residues, which assists multiple viruses for cell attachment or binding (WuDunn and Spear, 1989; Feldman et al., 2000; Shukla and Spear, 2001; Jiang et al., 2012; Richards et al., 2013; Tan et al., 2013). This is considered the first step toward establishing a successful infection. In the second category, an exceedingly specialized form of HS containing unique patterns of sulfation generated by sulfotransferase, is utilized by viruses to further facilitate infectious processes like virus-cell fusion, internalization, and trafficking etc (Shukla et al., 1999; Zautner et al., 2006; Borst et al., 2013; Connell and Lortat-Jacob, 2013; Makkonen et al., 2013).
Synthesis of Heparan Sulfate (HS)
HS synthesis is a dynamic process which initially begins with addition of tetrasaccharide linker region (GlcA-Gal-Gal- Xyl) on serine residues of the syndecans- the protein core (Esko and Lindahl, 2001). Following the initial addition of an N-acetylated GlcN (GlcNAc) residue to the beginning of the HS chain, polymerization continues with the addition of alternating GlcA and GlcNAc residues. The polymeric chain extension is also accompanied by a series of modifications in which multiple enzymes participate in a sequential order. These include glycosyltransferases, an epimerase, and sulfotransferases (de Agostini et al., 2008). During the initial process, N-deacetylation and N-sulfation of GlcNAc occur, transforming the glycosaminoglycan into N-sulfo-GlcN (GlcNS). Next C5 of GlcA is epimerized to IdoA, followed by O-sulfation, which is performed by 2-O-sulfotransferases (2-OSTs), 6-OST or 3-OST in the following order: Initially, 2-O-sulfation of IdoA and GlcA occurs followed by 6-O-sulfation of GlcNAc and GlcNS units, and lastly 3-O-sulfation of GlcN residues (Esko and Lindahl, 2001). The final step of 3-O sulfation is a rare modification that involves a finite number of chains (Marcum et al., 1986; Zhang et al., 2001; Thacker et al., 2014, 2016). Various arrangements of these modified residues result in a heterogeneous structure of HS which creates distinct binding motifs on the HS chains which are thought to regulate its functional specificity in distinct biological processes within the host. Concurrently, modifications of HS residues allow for distinct functions in pathogen–host interactions highlighting the impact of HS in disease development. For instance, the importance of HS-signaling in cancer biology, tumor development, metastasis, and differentiation are emerging (Figure 1) (Blackhall et al., 2001; Raman and Kuberan, 2010). Furthermore, a vast spectrum of pathogens ranging from viruses, bacteria, parasites, and fungi exploit the various moieties present on HS to signal and facilitate microbial pathogenesis (Sinnis et al., 2007; Gardner et al., 2011; Tiwari et al., 2012; Park and Shukla, 2013; Jinno and Park, 2015; Lin et al., 2015; Raman et al., 2016). HS promotes both, initial microbial attachment and associated inflammatory response, which may result in damaging outcomes for the host (Urbinati et al., 2009; Xu et al., 2011; Axelsson et al., 2012; Knelson et al., 2014; Yun et al., 2014; Kumar et al., 2015; Dyer et al., 2016).
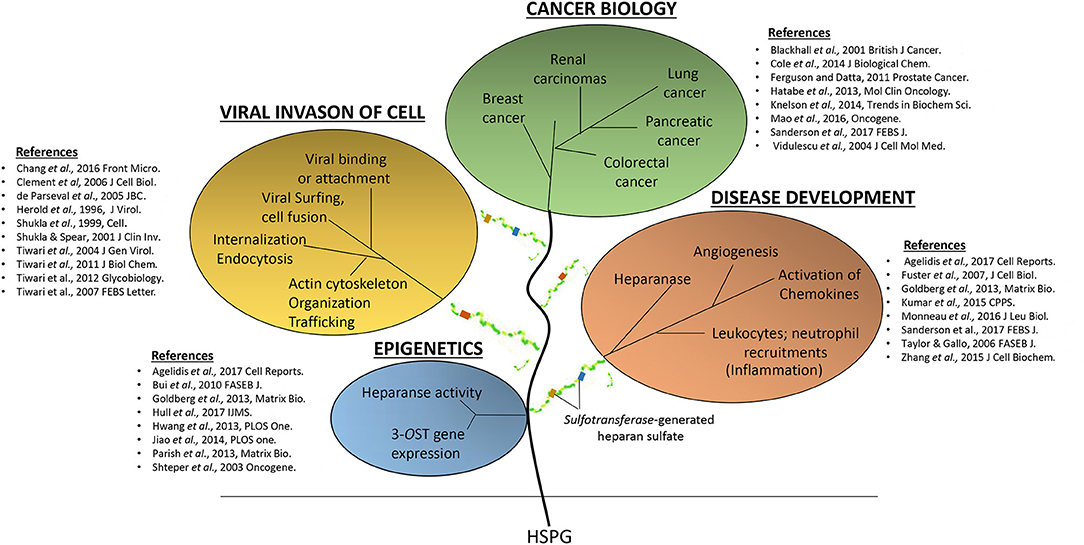
Figure 1. The detrimental effects of heparan sulfate (HS) and modified-forms of HS in various pathological events ranging from supporting virus invasions into host cell, multiple cancers, and disease development.
Sulfotransferase Generated Heparan Sulfate
Currently only three sulfotransferases: 2-O, 3-O, and 6-O sulfotransferase (2-OST, 3-OST, and 6-OST) enzymes are known to generate 2-O, 3-O, and 6-O sulfated heparan sulfate (2-OS HS, 3-OS HS, and 6-OS HS) respectively. These sulfated forms of HS play a role by generating high affinity receptors that allow viral and bacterial pathogens to have affinity for their natural target cell-types (Clement et al., 2006; Tiwari et al., 2006, 2007, 2011; Zautner et al., 2006; Trottein et al., 2009; Kobayashi et al., 2012; Hayashida et al., 2015; García et al., 2016) (Table 1 and Figure 2). Gene profiles for sulfotransferases have shown an enhanced expression in human monocytes and dendritic cells (DCs) during differentiation suggesting their role in responding to pathogens, stress, and during aging (Taylor and Gallo, 2006; Sikora et al., 2016). Since a large array of inflammatory and immunoregulatory mediators are known to interact with cell surface HS to target subsets of T lymphocytes and monocytes/macrophages (Zhou et al., 2015), dissecting such interactions could generate a valuable tool useful for understanding inflammatory and immune responses. Moreover, the impact of specific ligands on sulfotransferase enzymes and its turnover during pathogen invasion requires additional detailed investigation. In fact, a recent study offers oligosaccharides as a means by which to inhibit HS- sulfotransferases, adding new tools to probe the structural selectivity for HS-binding proteins (Raman et al., 2013).
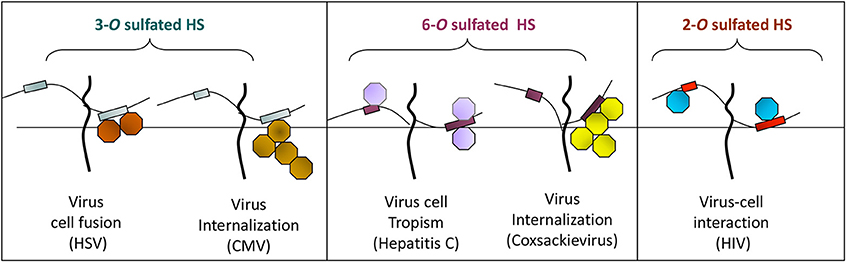
Figure 2. The O-sulfation in HS chain is known to generate binding sites for multiple viral proteins to facilitate viral entry. For instance, sulfation at 3-O position on glucosamine residues generates a HSV-1 glycoprotein D (gD) receptor for entry. In addition, 3-O sulfation in HS facilitates the spread of cytomegalovirus (CMV). Similarly, 6-O sulfation in HS chain promotes hepatitis C and coxackievirus entry. Interestingly, it has been demonstrated that HIV glycoprotein gp120 interacts with 2-O sulfated HS during cell entry.
Interestingly, these modified versions of HS are also increasingly recognized as potential markers for invasive diseases such as cancers and tumors. It has been proposed that 3-OS HS and 6-OS HS reinforce cancer cells to break down the extracellular matrix (ECM) to spread and highjack normal signaling pathways to facilitate cell spread (Brennan et al., 2016). In addition, HS structures containing unique subsets of sulfated domains are also proving to be facilitators for inflammatory responses making them specialized HS—a high-value molecule to be exploited for future drug development. In fact, engineered structure-specific HS-analogs or HS glycomimetics designed to modulate a specific function, such as to enhance protein interaction or to modulate the inflammatory process for overall higher efficacy and lower toxicity, are now routinely synthesized as promising new drugs for use against cancer and multiple other diseases (Esko and Selleck, 2002; Zhou et al., 2015; Afratis et al., 2017; Sanderson et al., 2017).
The unique chain that results from modifying HS enables it to bind specifically to its ligand to modulate angiogenesis, axonal sprouting, and insulin secretion (Liu and Thorp, 2002; Takahashi et al., 2012; van Wijk and van Kuppevelt, 2014; Zhang et al., 2015a). The many and diverse effects of the use of these drugs can results in better control of many different disease processes with minimal side effects and ultimately bring about better patient outcomes.
Sulfotransferase Generated Heparan Sulfate in HSV Entry
Modification also permits viral membrane fusion and penetration (Shukla et al., 1999; Xia et al., 2002; Chen et al., 2008). Unique sites within HS chains generated by 3-OSTs produce 3-OS HS, the newest family of HSV-1 glycoprotein D (gD) receptors, (Liu et al., 2002; Borst et al., 2013). The 3-OSTs act to modify HS further along in its biosynthesis, and each isoform recognizes glucosamine residues, as substrates, within regions of the HS chain having specific and unique, prior modifications, including epimerization and sulfation at other positions (Liu et al., 1999; Shworak et al., 1999). Thus, each 3-OST is capable of producing potentially unique protein-binding sites within HS. To date, seven different isoforms of human 3-OSTs (3-OST-1, 3-OST-2, 3-OST-3 A, 3- OST-3 B, 3-OST-4, 3-OST-5, 3-OST-6, and 3OST-7) are known. Although all the isoforms modify HS to generate 3-OS HS, it remains a mystery as to why seven isoforms are present. All, forms of 3-OST except 3-OST-1, produce HSV-1 entry receptors (Liu et al., 1999; Shukla et al., 1999). Interestingly, zebrafish embryo encodes 3-OST isoforms that are functionally similar in terms of recognizing HSV-1 glycoprotein D (gD) to promote viral entry. The gD receptors generated by other isoforms of 3-OST are very similar in structure, but likely not identical (Liu et al., 1999; Shukla et al., 1999; Chen et al., 2008; Borst et al., 2013). The isoform 3-OST-1 creates binding sites for antithrombin (Shworak et al., 1999; Mochizuki et al., 2003) but are unable to produce receptors for HSV-1 gD (Borst et al., 2013). Additionally, one or more isoforms of 3-OSTs have been found to be expressed in both human and mouse tissues that are relevant to HSV-1 infection examined thus far (Liu et al., 1999; Mochizuki et al., 2003; Lawrence et al., 2007) suggesting the significant role 3-OST play in contributing to disease development. The general distinction between plain-type of HS and the HS chain containing 3-O sulfation regarding their usage in viral entry is well-documented. Although the vast jungle of HS in the host cell assists in the capture and concentration of pathogens at a given site, it may not guarantee successful infection since HS mainly serves as an attachment receptor. Overall interactions between HS and pathogens are considered to be non-specific and may influence pathogenesis indirectly. On the other hand, target cells expressing 3-O or 6-O sulfated moieties in HS chains may guarantee that certain pathogens will be able to cross the host cell membrane and therefore the presence of the later form of specialized-HS may serve as a “Wi-Fi” zone for the targeting pathogens to easily invade the host cell. That is, pathogens in the vicinity of cells expressing modified HS can connect to “the internet” (host organism) by using modified HS as a means and signal to literally connect to host cells. To further the analogy, pathogens may even be able to detect modified HSPGs similar to how one can scan for Wi-Fi signals with a computer or a cellphone. Nonetheless, it remains to be investigated whether such “Wi-Fi” zones can be distinctly equipped with a particular signature of the 3-O-sulfated isoform that influences or contributes to establishing HSV tropism. Moreover, a HSV-1 gD-type 3-O HS specific to neurons or to ocular cells are well-documented (Clement et al., 2006; Deligny et al., 2010).
A series of evidence support the role of HS in viral infection as indicated in Figure 3. For example, enzymatic removal of specific regions in the HS chain in target cells by using heparanase significantly impairs the viral infection (Tiwari et al., 2004). Likewise, use of soluble heparin or HS-mimetic analogs inhibits viral infection through direct competition for cell surface HS (Gangji et al., 2018). Furthermore, a higher degree of viral entry was noticed in the cells that expressed HS compared to the mutant cells that were devoid of or weakly expressed HS. Lastly, the expression of viral envelop proteins which interact with HS in target cells, resulted in a resistance to viral entry by sequestering cell surface HS (Tiwari et al., 2006). As in the case of HSV-1, the expression of viral envelop glycoprotein B (gB) in target cells, which is known to bind HS, significantly impairs viral entry- a process also known as gB-mediated interference. Profoundly, our initial work made a unique discovery that viruses do in fact utilize HS beyond cell attachment and binding. Using cDNA library screening, direct evidence was presented for a modified version of HS; 3-OS HS generated by 3-OST-3 isoform as a receptor which promotes virus-cell membrane fusion (Shukla et al., 1999). Expression of the HS-modifying 3-OST-3 enzymes confers susceptibility for HSV-1 infection in previously HSV-1 resistant Chinese hamster ovary (CHO-K1) cells (Shukla et al., 1999). Additionally, an event with the protein receptors (nectin and HVEM) was observed that supports HSV-1 entry (Shukla and Spear, 2001). After identifying the significance of 3-OST-3 in HSV-1 entry, our group has cloned an additional six individual human and zebrafish isoforms of 3-OST enzymes and have characterized them against HSV-1 entry. Given the fact, that these enzymes are tightly regulated and are expressed in specific cells and tissues, it is tempting to speculate that 3-OST isoforms might be prime candidates for HSV localized infection either in brain or ocular cells and tissues, essentially contributing toward viral tropism. In this regard, our previous work has demonstrated the significance of one 3-OST-3 isoform type in primary cultures of human corneal stroma (CF) derived from eye donors- a natural target cell for HSV infection. Using si-RNA approaches together with the use of heparanase enzyme in cultured CF, we found a significant reduction in HSV-1 entry (Tiwari et al., 2006). Additional support for HSV use of the 3-OS HS receptor in CF was gained by using phage display-derived peptide targeting 3-OS HS receptor, which further resulted in a compelling inhibition in HSV-1 entry (Tiwari et al., 2011). To further strengthen our work, we used anti-3-OS HS peptides to successfully prevent HSV-1 infection in a mouse corneal model (Tiwari et al., 2011). With the above encouraging results, we have tested the clinical usage of anti-3-OS HS peptides by using them on a commercially available contact lens as a delivery vehicle for extended release as a potential and effective way to control corneal herpes infection (Jaishankar et al., 2016). Interestingly, a laboratory produced 3-O-sulfonated HS octasaccharide, was demonstrated to inhibit HSV-1 and host-cell interactions, suggesting the application of HS derived molecules as potential therapeutic tools against viral pathogens.
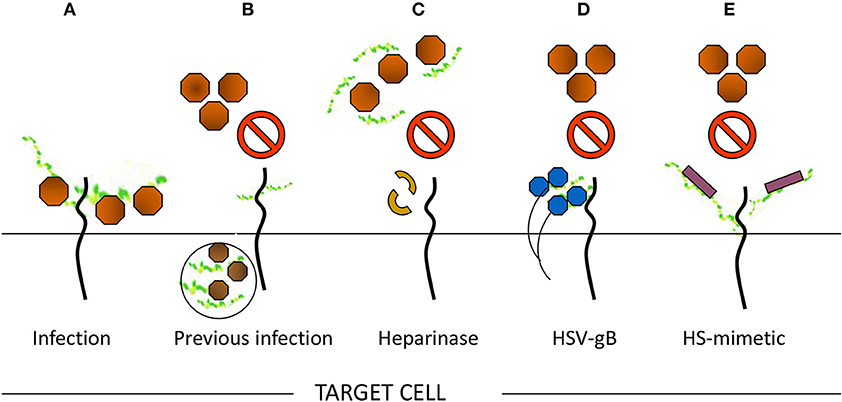
Figure 3. Multiple ways to demonstrate the significance of heparan sulfate (HS) in viral entry. (A) Moieties of HS chain provide initial docking sites for viral attachment, while sulfated HS chains generate receptors to promote virus-cell fusion. In contrast, the loss of HS may result in significant reduction of viral entry. For instance, (B) previous viral infection may result in resistance for incoming virus due to loss of HS. (C) In addition, enzymatic treatment of cells with heparanase may remove the critical moieties in HS chains required for viral entry and therefore results loss of viral infection. (D) Sequestering HS chain from the cell surface upon expressing viral protein renders resistance to in coming virus. (E) A competitive inhibition of viral entry by usage of HS mimetic on cell surface.
In the case of 3-OS HS, the ligands that are documented to interact are HSV-1 glycoprotein D (gD), antithrombin III, Fibroblast growth factor (FGF), Cyclophlin-B, and Neuropilin-1 (Liu et al., 1999; Datta et al., 2013; Baldwin et al., 2015; Zhang et al., 2015a). Emerging evidence suggests that multiple viruses have evolved with the interaction of O-sulfated domains in HS to promote infection. For instance, 3-OS HS although first recognized to be HSV-1 gD receptor, is now reported to be utilized by other members of the herpes virus family as well. A recent study by Baldwin et al. (2015) demonstrated that human cytomegalovirus interacts with 3-OS HS generated by 3-OST-3 isoforms to mediate virus spread (Zhang et al., 2010). This result emphasizes the symbolic role of 3-OS HS in co-infection or in superinfection models especially since herpes viruses are opportunistic by nature. On the other hand, it is also possible that viruses keep a secret and independent life in the infected cell by down-regulating the expression of 3-OS HS to make supportive habitats in the infected cells for their own distinct advantage. This question remains unresolved and needs to be further addressed. Similarly, 3-OS HS is exploited by viruses as an entry receptor, but at the line of duty, it primarily exists as a signaling transduction molecule. Therefore, it is possible that viruses intelligently sense the 3-OS HS environment and as such, they have no control over their turnover. Clearly, all the above questions are extremely vital as HS/3-OS HS widely participates in various steps of the virus life cycle.
Role of Sulfotransferase Generated Heparan Sulfate in Other Viruses
Interestingly among non-herpes viruses, expression of human 3-OST-3a may suppress Hepatitis B virus replication in hepatocytes (Hallak et al., 2000), while 6-O sulfation in HS chain potentially supports entry of human cytomegalovirus (Zautner et al., 2006). In addition, 6-OS HS has been shown to mediate internalization of coxackievirus B3 (Connell and Lortat-Jacob, 2013). Interestingly, 2-O sulfation is recognized by HIV glycoprotein gp120 during cell entry (Figure 2) (Makkonen et al., 2013; Matos et al., 2014). Conversely, N-sulfation of heparan is necessary for inhibition of Respiratory Syncytial Virus (RSV) infection (Fechtner et al., 2013) and is required for CHIKV infection (Tanaka et al., 2017). In case of Hepatitis C Virus (HCV), envelope glycoprotein E1/E2 requires both N and 6-O sulfate group to interact with HS (Trottein et al., 2009).
Besides viruses, other pathogens can also interact with the modified product of HS. For instance, an outer membrane protein of Chlamydia trachomatis known as OmcB interacts with 6-OS HS during infection (Tanaka et al., 2012). Seemingly, HS/3-OS HS becomes an ideal target for both viral and bacterial infection- a possibility that exists in many sexually transmitted infections. In the case of human T-cell leukemia virus type 1 (HTLV), it has been demonstrated that the combination of the number and the length of HS chains containing heparin-like regions is a critical factor in determining cell tropism of HTLV-1 (Monneau et al., 2016). It is hypothesized that shorter HS chains may be able to induce receptor complexes (HTLV-1 Env-HS-NRP-1-GLUT1) more efficiently than their longer counterparts by attracting HTLV-1 particles to the target cell surface. Nonetheless, the findings obtained in this study may provide the foundation for the development of effective therapies against HTLV-I infection and aid in the development of a metric for assessing the prognosis of HTLV-1 induced diseases. Whether the length of the HS chain influences virus infection in general still requires further investigation. Furthermore, whether the virus infection subverts the length of HS or effects HS-polymerization is also unrecognized. However, recent progress made in glycobiology has shown that glycosaminoglycan structural properties such as length, sulfation, and epimerization patterns, are cell, tissue, and developmental stage specific (Patel et al., 1993).
It is clear that a single type of sulfation in a HS chain can serve as an attachment point for multiple types of viruses. For instance, 3-O sulfation in HS generated by 3-OST-3 is utilized by both HSV and cytomegalovirus (CMV) to trigger virus entry. Moreover, an individual virus can exhibit the preference to two entirely different sulfation patterns in HS chains (Shukla et al., 1999; Baldwin et al., 2015). According to recent studies, CMV can facilitate both 6-OS HS and 3-OS HS for infectious entry (Borst et al., 2013; Baldwin et al., 2015). Though it is still unknown for many viruses, what specific sulfonation pattern is most preferred.
An exponential amount of evidence suggests that HSPGs play a significant role in the HIV lifecycle and disease development. Initially, it was demonstrated that viruses enter multiple T cells by using HS chains of HSPGs to facilitate entry (Roderiquez et al., 1995). Concomitantly, the presence of heparanase has been shown to competitively inhibit HIV entry into the target cells (Roderiquez et al., 1995; Ohshiro et al., 1996). In addition, multiple studies demonstrated that the presence of HSPG in various cell types enhances HIV entry (Ibrahim et al., 1999; Saphire et al., 2001; Guibinga et al., 2002; Zhang et al., 2002; Bobardt et al., 2004). For instance, primary human endothelial cells which are loaded with HSPG are extremely efficient in capturing viruses on the cell surface (Argyris et al., 2003). The overexpression of HS in primary endothelial cells near the blood-brain barrier and in the microvasculature, in addition to their ability to capture HIV-1, has been proposed as a novel mechanism to facilitate the invasion of the brain by HIV-1 (Argyris et al., 2003). Further prime target cells like DCs, macrophages, epithelial, and endothelial cells provide docking sites for HIV by expressing HS (Guibinga et al., 2002; Wu et al., 2003; Gallay, 2004; de Witte et al., 2007; Ceballos et al., 2009).
Additionally, HS-expressing spermatozoa play a crucial role in virus transformation into DCs, macrophage and T cells during HIV transmissions (de Parseval et al., 2005). In fact, four HS-binding domains have been identified in the V2 and V3 loops, the C-terminal domain, and the CD4-induced bridging sheet of the HIV gp120 (Crublet et al., 2008; Herrera et al., 2016). In contrast, one recent study depicts that HS combines with the innate protein human beta-defensin (hBD) and reduces HIV trans-epithelial transmission through inactivation of virus. Using an adult oral epithelial cell which expresses hBD, the authors showed the HSPG-mediated internalization of hBD and HIV-gp 120 in the endosome results in oligomerization and reduced infectivity of HIV (Vivès et al., 2005).
Remarkably, it has been demonstrated that HS not only facilitates viral attachment to host cells via HIV gp120 (Cladera et al., 2001) but also that HS mediates viral-host fusion by interacting with the fusion domain of gp41 (Teixé et al., 2008). On permissive cells, HIV binding to HS is thought to increase infectivity by concentrating viral particles at the cell surface (in the cis process). Additionally, for some cell types such as macrophages, HS may compensate for low CD4 expression (Guibinga et al., 2002). It has also been proven that the expression of HSPG on the CD4+ cell surface is regulated by virus infections and immune activation (Ibrahim et al., 1999; Alvarez Losada et al., 2002). HS may be directly involved in the infection of CD4+ and/or CD4− cells, making it an ideal target for new anti-HIV therapies (Wu et al., 2003; Somiya et al., 2016). Beside HS interaction to gp120, it also co-localizes with matrix protein p17 on activated human CD4+ T cells. Additionally, single particle tracking confirms that multivalent Tat protein transduction results in a domain-induced heparan sulfate proteoglycan cross-linkage, which activates Rac1 for virus internalization. HIV-1 Tat and HSPG interaction have been proposed as a novel mechanism of lymphocyte adhesion and migration across the endothelium (Xu et al., 2011).
Besides the symbolic role of HS for viral entry, HS plays other extensively documented roles in the viral lifecycle. Using a recombinant Hepatitis B virus (HBV) surface antigen L protein particles (bio-nanocapsules, BNCs), a HS-dependent mechanism of HBV uptake has been proposed (Dong et al., 2013). To study the hepatitis C virus (HCV), a gene silencing approach was used against enzymes involved in HS-biosynthesis to investigate structural determinants required by HCV during infection. The study found the N- and 6-O-sulfation, but not 2-O-sulfation, is required for HCV infection. It is also known that the minimum HS oligosaccharide length required for HCV infection is a decasaccharide. Overall, previous discoveries highlighted that HCV uses specific HS structure to initiate infectious cycle. Concurrently, another study implemented a different approach by using heparanase I, II, and III enzymes to cleave specific structures in HS to reach the same conclusion. The findings indicate that the heterogeneity of HS especially for rich N-sulfation and iduronic acids heavily participate in Respiratory Syncytial virus (RSV) infection in some mammalian cells (Cooper et al., 2005).
Besides the role of HS in viral entry, it is justified that HS is also involved in the induction of cytokines by HBV capsid in macrophages via TLR2 signaling (Tsidulko et al., 2015). A recent study has proposed that within various bovine tissues used for HS preparation, only liver-based HS can strongly bind to both E1 and E2 of Hepatitis C Virus (HCV). This study is very significant since HCV, which displays liver tropism, contains highly sulfated structures compared to HS from other tissues. This concludes that HS-proteoglycan on liver cell surfaces appear to be one of the molecules that define liver-specific tissue tropism of HCV (Trottein et al., 2009). Later, a study by Lawrence et al. (2007), Deligny et al. (2010) supports the notion that HS structures vary depending on the cell and tissue, and this can influence virus tropism. For instance, HS-modifying enzymes 3-OST-2 and 3-OST-4 are the predominant forms expressed in the neurons of the trigeminal ganglions while 3-OST-2 and 3-OST-4 generated HS have been shown to be of the principal gD-receptor type for HSV-1. Ultimately, all of these subtleties reinforce the presence of a selective interaction between HS and viruses. Although multiple viruses use HS proteoglycans which are ubiquitously expressed GAGs for binding and or attachment, it remains to be ruled out if virus interactions with HS are random or if they require structural specificity.
A unique pattern of HS expression in Epstein Barr Virus (EBV) infected cells is established. Lymphoblastoid cell lines infected with EBV have shown specific proteoglycan expression with down-regulation of CD44 and ECM components and up-regulation of serglycin and perlecan/HSPG2. Nevertheless, in Burkitt's lymphoma cells (BL), serglycin was shown to be down-regulated. Fundamentally, the biosynthetic machinery for HS construction and modification was active in all cell lines, with the cavate that this machinery was down-regulated in BL cells (Shannon-Lowe and Rowe, 2011). A separate study documents the involvement of sulfated-HS during B cell/epithelial cell interactions during Epstein—Barr virus (EBV) transfer (Woodard et al., 2016). In fact, understanding HS-virus association has been investigated for many, entirely different reasons to directly help patients. In ocular gene therapy, a novel chimeric adenovirus-associated vector (AAV) has been developed, which binds to HS and hence displays better transduction ability. This result has generated AAV with higher accumulation and penetration to the retina and therefore offers a less invasive intravitreal injection route for ocular gene therapy (Kaever et al., 2016). Similarly, the significance of HS in developing antibodies against vaccinia virus A27, human papillomavirus (HPV) has been advocated (Silva et al., 2014; Xia et al., 2016).
In addition, a study tested two different strains (vaccine strain and clinical isolates) of Chikungunya virus (CHIKV) for GAG binding generated efficient virus replication. Although GAGs are necessary for efficient binding of both strains, they exhibit differential requirements for GAGs. However, the study delineated the critical amino acid residue 82 in the envelope glycoprotein E2 is a primary determinant of GAG utilization. A critical outcome for the future development of viral entry inhibitors against CHIKV infection (Richards et al., 2013). Additionally, the importance of HS structure for CHIKV infection was demonstrated in a study by Tanaka et al. (2017), Following genome screening the CRISPR/Cas9 system was deployed to create knockout models of haploid human cells (HAP1). These knockout models were created to assess the importance of various genes that modify HS in efficient CHIKV infection. Infectivity was not affected following disruption of genes encoding proteins that act sequentially after NDST1, an enzyme that catalyzes the N-sulfation step of HS (Tanaka et al., 2017). GLCE is one such protein that acts after the N-sulfation step. GLCE catalyzes the C5 epimerization of GlcA to IdoA which is a necessary step required to later produce the 2 and 3-O-sulfated forms of HS. This phenomenon stands in contrast to the mechanism of HSV infection, where these modifications have been shown to be necessary for infection. Concerning CHIKV infection, HS in the 2 and 3-O-sulfated forms are not required for productive infection but rather it is the N-sulfated HS that facilitates viral binding and host cell entry.
In the case of HPV, multiple HS-binding sites were found on the capsid protein L1 which bring sequential confirmation shifts during virus-receptor engagement assisting virus attachment and internalization. For instance, primary binding is regulated by site 1 (Lys278, Lys361). It can cause confirmation shift in L2, which mediates the display of the amino terminus. In the step after primary attachment, Site 2 (Lys54, Lys356) and site 3 (Asn57, Lys59, Lys442, Lys443) are essential for viral entry (Richards et al., 2013). Overall, the HS-binding mediates exposure of secondary binding sites aiding in the virus engulfment.
There is a study comparing the amino acid sequences of the E2 glycoprotein from natural North American eastern equine encephalitis virus (NA-EEEV) isolates that shows that the neurovirulence of EEEV is supported by the interaction with HS (Voss et al., 2010). For instance, mutations from lysine to glutamine at E2 71, from lysine to threonine at E2 71, or from threonine to lysine at E2 72 were found to have an altered virulence and interaction with HS. Using an electrostatic map, the HS-binding regions in the EEEV E1/E2 heterotrimer were predicted at the apical surface of E2. Basing this method upon the recent Chikungunya virus crystal structure, variants were detected to affect the electrochemical nature of the binding site (Ryman et al., 2007). The EEEV sylvatic cycle could be the starting point for these natural variations in the EEEV HS-binding domain, which may influence receptor interaction as well as the severity of EEEV disease (Voss et al., 2010).
Similarly, HS-binding has been proposed to be an important factor in neurovirulence of neuroadapted and non-neuroadapted Sindbis Viruses (SV) (Milho et al., 2012). Substantially, HS on olfactory neuroepithelium offers an essential and multifaceted site for HS-dependent murid herpesvirus-4 uptake. Therefore, olfactory neuroepithelium poses a critical entry point for heparan-dependent herpesvirus (Antoine et al., 2014).
Heparan Sulfate- a Gateway for the Emerging Diseases
Emerging highly pathogenic viruses continue to use cell surface HS for attachment. For instance, zoonotic viruses such as Nipah and Hendra viruses have shown to use to HS for viral entry during lethal infection (O'Hearn et al., 2015). Similarly, Filoviruses, including Ebola virus (EBOV) and Marburg virus (MARV), known to cause hemorrhagic fever with a high mortality rate are also known to use cell surface HS and enzyme EXT-1 involved in HS-biosynthesis (Riblett et al., 2016). In addition, Rift Valley fever virus (RVFV), also an emerging pathogen that can cause lethal hemorrhagic fever syndrome in humans, has been documented to use HS during the initial stages of infection (Mao et al., 2016).
Heparan Sulfate- a Platform for Infection
Viruses are consistently facing enormous challenges due to the jungle of HS chains on the cell surface which may hinder or prevent the virus from being released efficiently from the infected cell. Ironically this gives viruses an opportunity to get equipped with a strategy to overcome this problem. For instance, in hepatocytes hepatitis B the virus hides its envelope glycoprotein in its interior during release and becomes HSPG-non binding N-type. Then later it becomes HSPG-binding B-type followed by the translocation of its envelope protein back to the viral surface the latter of which, results in the virus's infectivity. In the case of HSV, it was recently discovered that upon infection the expression of heparanase enzyme is up-regulated via NF-kB mechanisms, which aid in cleaving off the HS/3OS HS chains resulting in virus release, supporting viral pathogenesis. Taken together, viruses find their way to leave the cells and prepare for next target cell by changing the landscape of their own HS-interacting envelope glycoproteins or by shedding the cell surface HS. Interestingly, an overexpression of heparanase is well-documented in multiple pathological conditions such as inflammation, angiogenesis, angiogenesis, tumor metastasis, and atherosclerosis. Again, heparanase mediated breaking or shedding of the basement membrane of epithelial and endothelial cells results in an increase in vascular permeability which in turns supports the leakiness and migration of the leukocytes in the surrounding tissues. In addition, actions of heparanase cause further damage by the release of HS-sequestered with cytokines and growth factors (e.g., vascular endothelial growth factor; VEGEF), which either on the spot or in the surrounding regions, ignite inflammatory processes and angiogenesis aggravating the issue. The later process results in the activation of GTPase signaling, which activates cytoskeleton proteins to promote cell migration and invasion. Again, these actions combined with the up-regulation of matrix metalloproteinase facilitate the intercellular tumor invasion and metastasis after the loss of the HS barrier in the ECM. Further, the released HS may bind to endogenous TLR4 which in turn-on activates the signaling cascade to release the pro-oncogenic signals. These reactions are too overwhelming for the cell and tissues to repair the damage and maintain their homeostasis. Therefore, the overall process may result in devastating disease. A similar situation exists during ocular HSV-1 infection when the virus initially mediates the infection in the layer of corneal epithelium which later brings an overwhelming immune response together with angiogenic cytokines. This immune-mediated response in the corneal stroma can compromise the corneal transparency ultimately resulting in vision loss.
The sulfated moieties present in a HS chain are key determinants for its biological significance. Analyzing HS-moieties for their structure and function is an important strategy for multiple reasons. For instance, multiple moieties in the HS structure remain “orphan” in the sense that the ligands they interact with are not yet fully discovered or if they exist, are poorly understood. In addition, the structural moieties in HS do have “good” and “ugly” components which are differentially expressed signifying a “biological clock” and further suggesting using expression pattern as a potential diagnostic tool. For example, expression of 3-OST and 6-OST has been shown to be up-regulated in certain cancers (Connell et al., 2012; Cole et al., 2014). Since the potential in human disease and associated pathologies including virus infection is now being recognized, identifying the vasculature of HS and associated signature of pro-inflammatory chemokines/cytokines in a diseased state is crucial for potential target development.
Interestingly, a study by Connell et al. (2012) demonstrated the concept of generating HS synthetic -mimetic peptide conjugated to a mini CD4 display high anti- HIV-1 activity independent of co-receptor usage (Whittall et al., 2013). The CD4 mimetic and the heparan sulfate derivative interact synergistically and allow potent antiviral activity against both CCR5- and CXCR4-tropic HIV-1 strains. Similarly, peptide-based molecules that directly recognize 3-OS HS or 6-OS HS or 2-OS HS will be of enormous value to understand multiple disease pathologies including virus pathogenesis. In this direction, we made an optimistic attempt by developing peptides which recognize HS or 3-OS HS. These peptides were found to be effective against a murine ocular HSV model. Further characterization of the anti-HS and anti-3-OS HS peptides to prevent inflammatory response need to be tested. In fact, there is a critical need to crack the code between the viruses and their affinity for specific forms of HS. We need to define their structural moieties. There is an emerging trend indicating that multiple viruses do use specific moieties present in HS chain suggesting association to the structural components. However, this remains to be determined if viruses have a preference for one type of sulfation pattern over other types. If true, it would give viruses an edge to establish tropism because cell and tissues exhibit specific expression of sulfated-HS. This could mean that viral use of a specific sulfate-HS moiety could be used as a marker for a more virulent strain. Further, is there is a unique sub-set of sulfated-HS that are expressed in disease states, which are either not present in healthy cells and tissues or present at low levels? A major gap in knowledge exists if the post virus infection influences the expression of certain types of HS-sulfotransferases or if their combinations further impact the additional variability and complexity in the existing HS structure. Developing a blueprint in the regions of the sulfated-moieties in HS during pre-and post-infection and identifying their binding partner's, especially the viral ligands, will boost the development of the targeted therapy. Our complete understanding of the structural components in HS interacting to ligands during various biological processes is very likely to advance our knowledge in the benefit of human health by preventing virus infection, serious diseases like cancer, and their associated pathologies. For instance, peptide-based formulations that directly target interactions between viruses envelop glycoprotein and respective sulfated-HS and affecting the immune response will be new exciting therapeutic options as a broad-spectrum virus inhibitor. Usage of cell surface HS moieties during viral binding and entry is a unique feature that is shared by medically important viruses including many herpes viruses. Similarly, multiple viruses exploit modified-HS differently to promote infection. For instance, HSV uses 3-OS HS to promote virus-cell fusion, while the presence of 3-OS HS significantly increases during CMV internalization, suggesting the possibility that 3-OS HS-mediates endocytosis (Baldwin et al., 2015). Further, use of multiple virus strains including clinical isolates and cell-types are also necessary to classify global or very specific-usage of sulfated-HS. These data will be highly informative and will allow in establishing HS-typing. One additional way is to screen the phage display library against 2-O, and 6-O sulfated-HS. Interestingly, certain structural forms of HS have been suggested to be involved in the uptake of ligands using endocytosis. Although, HS is a very versatile molecule; little information is available regarding the HS-epitope role in driving efficient trafficking of virus-cargo in endosomes, trapping cell signaling molecules which otherwise may diffuse, and controlling potential virus gene expression. Understanding the type of HS that is involved in loading and unloading virus cargo, the type of HS that effects cell-to-cell communication, and secondary messengers are emerging and fascinating science, which has tremendous treatment potential. For instance, the delivery of therapeutic agents such as HS recognizing particles conjugated with anti-HS/anti-3OS HS peptides in nanosomes may be given to the patient. Phage display based on anti-sulfated HS peptides will be useful in this regard as well to improve therapeutic, diagnostic options, and patient outcomes. Phage display library screening is now widely used as an antiviral approach to identify ligands that bind to virus glycoproteins or cellular receptors to reduce or block virus infection. It is tempting to speculate that designing peptides which delay or interfere with virus internalization, trafficking, or that potentially stop cell communication, could be very useful on various levels.
Heparan Sulfate- a Key Component in Actin Cytoskeleton and Inflammation
Cell surface HSPGs are known to influence the cell behavior and cytoskeletal organization via interaction with the numerous ligands (Martinho et al., 1996). It has been recently discovered that intercellular bridges or cytoplasmic bridges between cells expressing F-actin and HS/3-OS HS are indicators of cell-based oxidative stress, virus infection inflammation and cancer (Onfelt et al., 2004; Rustom et al., 2004; Vidulescu et al., 2004; Sowinski et al., 2008). Similarly, long bridges of tunneling nanotubes (TNTs) connecting two or more cells that express HS and 3-OS HS (Rustom et al., 2004; Chang et al., 2016) have been proposed to be heavily involved in organelles and vesicle transport as a part of cell to cell communication. Their usage in viral transport has gained momentum for retroviruses (Sowinski et al., 2008; Omsland et al., 2018). For instance, it was recently shown that HIV infection in the primary human macrophages results in an increased number of TNTs, which further gives the virus an opportunity to spread via a novel mechanism (Hashimoto et al., 2016). A similar mechanism may exist for multiple viruses. It is obvious that viruses use TNTs smartly and strategically to cover long distances quickly and efficiently while escaping the host immune response via compartmentalization. Again, HS may play a bigger role in the virus trafficking across TNTs by clumping or aggregating around the actin bundles followed by organizing the molecular machinery to interact with myosin binding ATPases to trigger the virus movement. HSV being a neurotropic virus may find similar TNTs between two neurons. Recently it has been shown that pathogenic α-synuclein fibrils, responsible for Parkinson disease, travel between neurons in culture inside lysosomal vesicles through tunneling nanotubes (TNTs)- a recently discovered mechanism of intercellular communication (Gallegos et al., 2015). HSV with the similar opportunity may exploit TNTs to move and find the new neighborhood to cause persistence, neuroinflammation, and potentially CNS associated neurodegenerative diseases. In regard to human metapneumovirus (HMPV), a cellular extension-based model recently has been proposed to be utilized by the virus. Interestingly, the virus has two modes of infection: cell-free infection, which is blocked by neutralizing antibodies and requires binding to HS moieties, and direct cell-to-cell infection, which is HS independent (El Najjar et al., 2016).
During inflammation, chemokines stimulate the induction of endothelial filopodia and microvilli structures, which have high levels of HS/2-O/6-O/3-O sulfated HS (Sowinski et al., 2008). This signifies the role of sulfated-HS in helping in the sequestration or clustering of chemokines, their gradient and presentation of the chemokine to leukocytes, and further helping in leukocytes trans-endothelial migration. Interestingly, multiple studies have shown that viruses during cell entry often reorganize their actin cytoskeleton and produce filopodia (Chang et al., 2016). Although these structures have been proposed to aid in virus infections such as surfing and trafficking, it remains to be determined if such structures further participate in the recruitment of inflammatory cells and tissue invasion. On the one hand, a study by Whittall et al. (2013) clearly demonstrated co-localization of 2-O, 3-O, and 6-O heparan sulfate with chemokine (CXL8) on filopodial surfaces. On the other hand, the antagonizing chemotactic activity of pro-inflammatory cytokines and angiogenic activity by the usage of HS-mimetics are proving to be very useful (Mohamed and Coombe, 2017). Therefore, such small molecules can be valuable candidates in preventing not only HSV entry but also in recruiting inflammatory cells in response to HSV-1 in ocular cells and tissues (Gangji et al., 2018). This will bring a much-needed benefit for the patient suffering either from high tittered acute infection or low dosage chronic immune-mediated response to infection. The later phase is responsible for substantial immune cell infiltrates leading to scarring of the eye tissues.
Heparan Sulfate Structures That Interact With Viral Glycoproteins
Although biopolymer HS is massively diverse, specific sequences within it are likely to be critical for recognition of viral glycoproteins. An example of specific recognition is demonstrated by the HS–gD interaction, wherein a 3-O-sulfated GlcNp residue is required for HSV-1 to penetrate cells (Shukla et al., 1999; Xia et al., 2002; Tiwari et al., 2004). The site in HS to which gD binds is generated by an isoform of 3-O-sulfotransferase that yields an octasaccharide, ΔUA-(1➔4)-GlcNp2S-(1➔4)-IdoAp2S-(1➔4)-GlcNp2Ac-(1➔4)-GlcAp2S (or IdoAp2S)-(1➔4)-GlcNp2S-(1➔4)-IdoAp2S-(1➔4)-GlcNp3S6S (Liu et al., 2002). Although gB and gC are major components of the viral envelope that facilitates binding to HS, the structure(s) in HS which these glycoproteins recognize have not yet been determined. However, it appears likely that these glycoproteins recognize different HS sequence(s) as suggested by competition studies (Herold et al., 1995). In addition, different HS structures appear to be recognized by gC from HSV-1 and HSV-2 (Gerber et al., 1995). This raises the concept of HS mimetics as inhibitors of viral infection of host cells.
Mimics of HS as Inhibitors of Viral Entry Into Cells
Decades ago in 1964, it was discovered that heparin, a glycosaminoglycan related to heparan sulfate, inhibited HSV infection, which was possibly the origin for the idea that heparan sulfate is a viral receptor. Inhibition by heparin is a competitive process and, therefore, is most effective when the inhibitor is present during the attachment phase of viral entry. Heparin has been shown to bind to soluble forms of gB, gC, and gD (Nahmias and Kibrick, 1964; Herold et al., 1995; Tal-Singer et al., 1995; Feyzi et al., 1997; Trybala et al., 2000). Interestingly, whereas 6-O- and 3-O-sulfation is the primary determinant for HSV-1 infection, they appear to have a little role in HSV-2 infection, suggesting differences between the two types of viruses (Herold et al., 1996). Likewise, O-sulfation was found to be more important for binding to gB from HSV-1 than gB from HSV-2. Fractionation of polymeric heparin into discrete sulfated oligosaccharide mixtures suggests that nearly 10–12 monosaccharides are necessary for effective binding to gC (Feyzi et al., 1997).
Numerous other sulfated polysaccharides have been explored as mimics of HS in the inhibition of herpesvirus entry into cells. A principal source of these sulfated polysaccharides is sea algae, which biosynthesizes these molecules to retain K+ and Ca+2 ions from seawater and for enhanced resistance to desiccation. These polysaccharides include κ and λ carrageenans (Marchetti et al., 1995; Carlucci et al., 1997, 1999a,b; Zacharopoulos and Phillips, 1997), galactan sulfate (Damonte et al., 1996; Mazumder et al., 2002), galactofucan sulfate (Thompson and Dragar, 2004), fucan sulfate (Preeprame et al., 2001), spirulan (Hayashi K. et al., 1996; Hayashi T. et al., 1996), fucoidan (Ponce et al., 2003; Lee et al., 2004a), rhamnan sulfate (Lee et al., 1999), chitin sulfate (Ishihara et al., 1993), and other uncharacterized polymers (Hasui et al., 1995; Witvrouw and De Clercq, 1997; Lee et al., 2004b; Zhu et al., 2004). In addition, other polysaccharides investigated as mimics of heparan sulfate include chemically modified heparins (Herold et al., 1995, 1997; Feyzi et al., 1997), non-anticoagulated heparin (Herold et al., 1997), pentosan polysulfate (Herold et al., 1997), and dextran sulfate (Marchetti et al., 1995; Neyts et al., 1995; Dyer et al., 1997; Herold et al., 1997).
Non-saccharide mimetics of HS as Inhibitors of Viral Entry Into Cells
A growing a class of small molecules these days is called non-saccharide glycosaminoglycan (GAG) mimetics (NSGMs). NSGMs are much smaller than polymeric HS mimetics, which makes them more drug-like in nature in comparison to the oligomeric and polymeric HS mimetics. NSGMs are also much easier to prepare (synthesize) in comparison to oligomeric HS mimetics and they are highly water soluble. Most importantly, NSGMs are functional mimetics of polymeric GAGs, which arises from their ability to bind to sites on proteins that interact with GAGs. Many NSGMs have been discovered so far that modulate various processes in addition to viral infection (Desai, 2013). For example, sulfated flavonoids and xanthones have been found to work as anticoagulant and antiplatelet agents (Al-Horani et al., 2011; Correia-da-Silva et al., 2011); sulfated benzofurans have been found as inhibitors of thrombin (Sidhu et al., 2013); and sulfated benzylated glycosides have been discovered as inhibitors of human factor Xia (Al-Horani et al., 2013; Al-Horani and Desai, 2014).
In a recent work, Gangji et al. (2018) show for the first time NSGMs present an excellent alternative to polymeric HS agents for inhibiting HSV (Gangji et al., 2018). These authors screened a small library of synthetic NSGMs (MW in range of 500–2500) and identified a distinct group of NSGMs that bind glycoprotein D with high affinity (10 nM or so). More importantly, one specific NSGM, called SPGG, inhibited cellular entry of HSV-1 with IC50 in the range of 430 nM to 1.0 μM. This is greater than a 10-fold lower response than that reported for 3-O-sulfate containing heparin/heparan sulfate-derived octasaccharides (Liu et al., 2002; Copeland et al., 2008; Hu et al., 2011).
Overall, competitive inhibition of viral entry through the use of either oligosaccharidic or non-saccharidic agents is very exciting. Several poly/oligosaccharides and small aromatic agents have been discovered that present major opportunities for development of anti-virals. It appears that the category of NSGMs represents excellent agents for targeting viral glycoproteins.
Epigenetic Components
Equally important, growing evidence suggests that epigenetic regulation of HS-modifying enzymes (sulfotransferases) together with heparanase (HPSE; mammalian endoglycosidase which degrades HSPG) are important determinants in the pathogenesis of several inflammatory conditions (Figure 4). For example, Bui et al. (2010) showed upon analysis of chondrosarcoma cells the typical hypermethylation profile of 3-OST sulfotransferase genes, which contributed toward the invasive phenotype of cancer. A similar hypermethylation pattern in 3-OST-2 genes has recently been reported in a variety of cancers (Hwang et al., 2013; Hull et al., 2017). Several studies have shown that DNA methylation of the HPSE promoter influences HPSE expression in different stages of breast cancer and has a direct effect on tumor progression (Jiao et al., 2014). The knocking down and overexpression experiments with HPSE confirmed that HPSE regulates the transcription of a distinct cohort of immune response genes involved in T cell effector function and migration (Parish et al., 2013). An increase in HPSE levels result in NF-kB activation followed by the release of tumor-promoting substances, growth factors (GFs) and cytokines by tumor-associated macrophages (Goldberg et al., 2013). Further, the increased metastatic potential in vivo mice was inhibited with laminaran sulfate, a potent inhibitor of HPSE activity (Shteper et al., 2003). In addition, HPSE promotes cancer and related inflammatory pathologies by removing extracellular barriers for serve to limit invasion/extravasation. The release of HS-bound GFs and cytokines results in activation of anti-apoptotic signaling and stimulates angiogenesis (Goldberg et al., 2013). During ocular HSV-1 infection, it has been shown that upregulation of HPSE at the nucleus caused decreased interferon signaling and increased NF-κB activation, resulting neighboring cells to be more susceptible to infection and increased pro-inflammatory factor production (Agelidis et al., 2017). Therefore, HPSE represents an attractive target for the development of broad spectrum drugs which may have antimicrobial, anti-inflammatory to anti-tumor activities (Khachigian and Parish, 2004). Antimicrobial peptides and HS-mimetic, which target HPSE, are already gaining wide popularity to resolve life-threatening situations (Martin et al., 2015; Brennan et al., 2016).
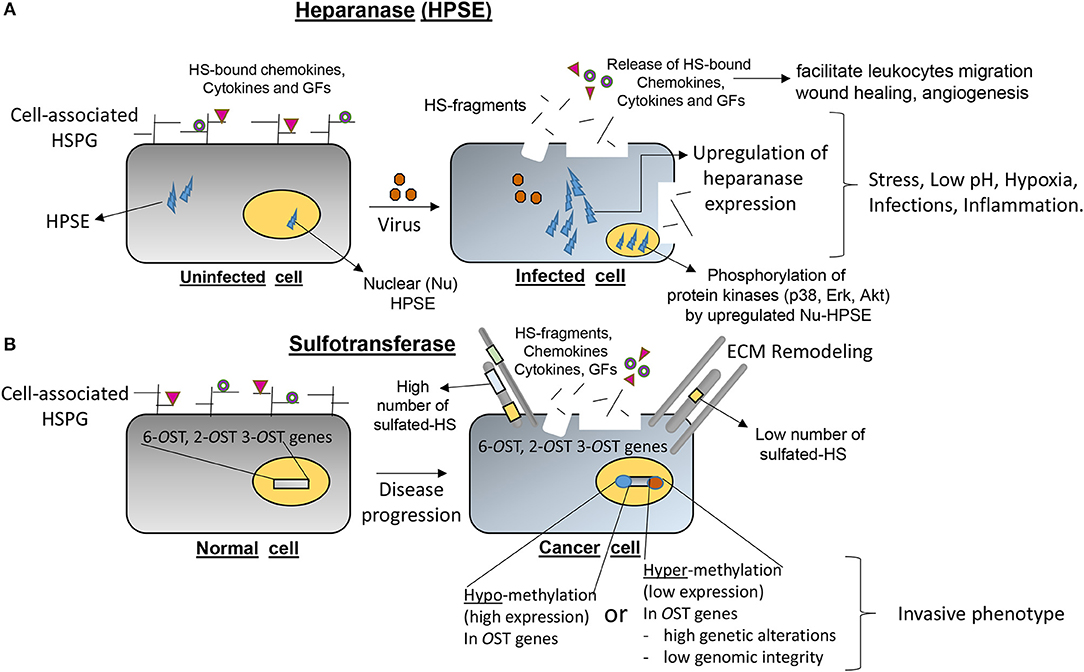
Figure 4. Emerging roles of heparanase (HPSE) and heparan sulfate (HS) modifying O-sulfotransferases (OSTs) in contributing directly in the onset of several disease pathologies. (A) An upregulation of HPSE under stress conditions such as low-pH, hypoxia, microbial infections, and inflammation degrades the normal architecture and basement membranes resulting the shedding of HSPG, cytokines/chemokines/growth factors (GFs) in ECM to initiate the cascade of inflammatory process. (B) Epigenetic regulations (hypo-methylation or hyper-methylation) of HS-modifying enzymes (2-, 6-, and 3-OSTs) resulting in either lower or over-expression of OST genes are widely documented in multiple diseases including cancer progression and certain invasive cancer phenotypes.
The ultimate benefit of understanding the regulation of the sulfotransferase and HPSE genes and their localization mechanisms during disease development will be instrumental in the discovery of novel biomarkers. This approach will be beneficial for the early detection of disease and therefore may offer a better prognosis. In addition, targeting genes responsible for abnormal phenotype by developing highly specific inhibitors may have limited side effects and hence bring novel interventions to prevent disease.
Conclusion
Growing evidence suggests that the interaction between viral components and their preferred modified HS-type variants is significantly important in our understanding toward the virus pathogenicity. Scientists of the past decade have developed sophisticated information on the molecular determinants of modified-HS and their counterpart ligands involved in various steps of microbial pathogenesis. Nonetheless, more exceedingly advanced works are required to demonstrate precise and more specialized work on the role of the modified forms of HS-variants in viral pathogenesis and in disease development. Heparan sulfate (HS) once stood for an ancient yet vital regulator of cell homeostasis, however with the discovery of the HS modifying enzymes, multiple roles of HS-variants (i.e., modified versions of HS) are turning out to be equally fascinating since the domains in the sulfated-HS carry very precise functions. One question remains to be addressed; do the pathogens including viruses have the ability to directly or indirectly control the expression of HS modifying enzymes? Further, the structure-function analysis of HS-variants in terms of their role in virus-infectivity, tropism, and in the disease-phenotype remains to be investigated. Since viral glycoproteins have unique structural motifs to bind HS and 3-OS HS, the possibility now exists to design HS mimetics, which selectively block viral protein interaction with the host cell to expand the selective therapeutic potential of HS mimetics (Gangji et al., 2018). The fact that HS, modified HS, together with HPSE activity plays a critical role during viral infection suggests that cell surface proteoglycans are a promising area for the development of reagents and understanding of the disease process. Overall, these studies certainly are worth pursuing to advance our approach to understanding disease and developing novel therapeutic interventions.
Author Contributions
DDK, DJ, MH, JB, MVV, and VT wrote the article. URD provided HS-mimetic section. VT, MH, JCB, and DDK prepared the figures and the table.
Funding
DDK was supported by the Biomedical Science Program (College of Graduate Studies) at Midwestern University (MWU). VT was supported by MWU's start-up funds.
Conflict of Interest Statement
The authors declare that the research was conducted in the absence of any commercial or financial relationships that could be construed as a potential conflict of interest.
Acknowledgments
Midwestern University start-up funds to VT is kindly acknowledged.
References
Afratis, N. A., Karamanou, K., Piperigkou, Z., Vynios, D. H., and Theocharis, A. D. (2017). The role of heparins and nano-heparins as therapeutic tool in breast cancer. Glycoconj. J. 34, 299–307. doi: 10.1007/s10719-016-9742-7
Agelidis, A. M., Hadigal, S. R., Jaishankar, D., and Shukla, D. (2017). Viral activation of heparanase drives pathogenesis of herpes simplex virus-1. Cell Rep. 20, 439–450. doi: 10.1016/j.celrep.2017.06.041
Alhasan, A. A., Spielhofer, J., Kusche-Gullberg, M., Kirby, J. A., and Ali, S. (2014). Role of 6-O-sulfated heparan sulfate in chronic renal fibrosis. J. Biol. Chem. 289, 20295–20306. doi: 10.1074/jbc.M114.554691
Al-Horani, R. A., and Desai, U. R. (2014). Designing allosteric inhibitors of factor XIa. Lessons from the interactions of sulfated pentagalloylglucopyranosides. J. Med. Chem. 57, 4805–4818. doi: 10.1021/jm500311e
Al-Horani, R. A., Liang, A., and Desai, U. R. (2011) Designing nonsaccharide, allosteric activators of antithrombin for accelerated inhibition of factor Xa. J. Med. Chem. 6125–6138. doi: 10.1021/jm2008387
Al-Horani, R. A., Ponnusamy, P., Mehta, A. Y., Gailani, D., and Desai, U. R. (2013). Sulfated pentagalloylglucoside is a potent, allosteric, and selective inhibitor of factor XIa. J. Med. Chem. 56, 867–878. doi: 10.1021/jm301338q
Alvarez Losada, S., Cantó-Nogués, C., and Muñoz-Fernández, M. A. (2002). A new possible mechanism of human immunodeficiency virus type 1 infection of neural cells. Neurobiol. Dis. 11, 469–478. doi: 10.1006/nbdi.2002.0566
Antoine, T. E., Jones, K. S., Dale, R. M., Shukla, D., and Tiwari, V. (2014). Zebrafish: modeling for herpes simplex virus infections. Zebrafish 11, 17–25. doi: 10.1089/zeb.2013.0920
Argyris, E. G., Acheampong, E., Nunnari, G., Mukhtar, M., Williams, K. J., and Pomerantz, R. J. (2003). Human immunodeficiency virus type 1 enters primary human brain microvascular endothelial cells by a mechanism involving cell surface proteoglycans independent of lipid rafts. J. Virol. 77, 12140–12151. doi: 10.1128/JVI.77.22.12140-12151.2003
Axelsson, J., Xu, D., Kang, B. N., Nussbacher, J. K., Handel, T. M., Ley, K., et al. (2012). Inactivation of heparan sulfate 2-O-sulfotransferase accentuates neutrophil infiltration during acute inflammation in mice. Blood 120, 1742–1751. doi: 10.1182/blood-2012-03-417139
Baldwin, J., Maus, E., Zanotti, B., Volin, M. V., Tandon, R., Shukla, D., et al. (2015). A role for 3-O-sulfated heparan sulfate in promoting human cytomegalovirus infection in human iris cells. J. Virol. 89, 5185–5192. doi: 10.1128/JVI.00109-15
Bink, R. J., Habuchi, H., Lele, Z., Dolk, E., Joore, J., Rauch, G. J., et al. (2003). Heparan sulfate 6-o-sulfotransferase is essential for muscle development in zebrafish. J. Biol. Chem. 278, 31118–31127. doi: 10.1074/jbc.M213124200
Bishop, J. R., Schuksz, M., and Esko, J. D. (2007). Heparan sulphate proteoglycans fine-tune mammalian physiology. Nature 446, 1030–1037. doi: 10.1038/nature05817
Blackhall, F. H., Merry, C. L., Davies, E. J., and Jayson, G. C. (2001). Heparan sulfate proteoglycans and cancer. Br. J. Cancer 85, 1094–1098. doi: 10.1054/bjoc.2001.2054
Bobardt, M. D., Salmon, P., Wang, L., Esko, J. D., Gabuzda, D., Fiala, M., et al. (2004). Contribution of proteoglycans to human immunodeficiency virus type 1 brain invasion. J. Virol. 78, 6567–6584. doi: 10.1128/JVI.78.12.6567-6584.2004
Borst, E. M., Ständker, L., Wagner, K., Schulz, T. F., Forssmann, W. G., and Messerle, M. (2013). A peptide inhibitor of cytomegalovirus infection from human hemofiltrate. Antimicrob. Agents Chemother. 57, 4751–4760. doi: 10.1128/AAC.00854-13
Brennan, T. V., Lin, L., Brandstadter, J. D., Rendell, V. R., Dredge, K., Huang, X., et al. (2016). Heparan sulfate mimetic PG545-mediated antilymphoma effects require TLR9-dependent NK cell activation. J. Clin. Invest. 126, 207–219. doi: 10.1172/JCI76566
Bui, C., Ouzzine, M., Talhaoui, I., Sharp, S., Prydz, K., Coughtrie, M. W., et al. (2010). Epigenetics: methylation-associated repression of heparan sulfate 3-O-sulfotransferase gene expression contributes to the invasive phenotype of H-EMC-SS chondrosarcoma cells. FASEB J. 24, 436–450. doi: 10.1096/fj.09-136291
Cadwallander, A. B., and Yost, H. J. (2006). Combinatorial expression patterns of heparan sulfate sulfotransferases in zebrafish. I. The 3-O-sulfotransferase family. Dev. Dyn. 235, 3423–3431. doi: 10.1002/dvdy.20991
Carlucci, M. J., Ciancia, M., Matulewicz, M. C., Cerezo, A. S., and Damonte, E. B. (1999a). Antiherpetic activity and mode of action of natural carrageenans of diverse structural types. Antiviral Res. 43, 93–102. doi: 10.1016/S0166-3542(99)00038-8
Carlucci, M. J., Pujol, C. A., Ciancia, M., Noseda, M. D., Matulewicz, M. C., Damonte, E. B., et al. (1997). Antiherpetic and anticoagulant properties of carrageenans from the red seaweed Gigartina skottsbergii and their cyclized derivatives: correlation between structure and biological activity. Int. J. Biol. Macromol. 20, 97–105. doi: 10.1016/S0141-8130(96)01145-2
Carlucci, M. J., Scolaro, L. A., and Damonte, E. B. (1999b). Inhibitory action of natural carrageenans on Herpes simplex virus infection of mouse astrocytes. Chemotherapy 45, 429–436. doi: 10.1159/000007236
Ceballos, A., Remes Lenicov, F., Sabatté, J., Rodríguez Rodrígues, C., Cabrini, M., Jancic, C., et al. (2009). Spermatozoa capture HIV-1 through heparan sulfate and efficiently transmit the virus to dendritic cells. J. Exp. Med. 206, 2717–2733. doi: 10.1084/jem.20091579
Chang, K., Baginski, J., Hassan, S. F., Volin, M., Shukla, D., and Tiwari, V. (2016). Filopodia and viruses: an analysis of membrane processes in entry mechanisms. Front. Microbiol. 7:300. doi: 10.3389/fmicb.2016.00300
Chen, E., Stringer, S. E., Rusch, M. A., Selleck, S. B., and Ekker, S. C. (2005). A unique role for 6-O sulfation modification in zebrafish vascular development. Dev. Biol. 284, 364–376. doi: 10.1016/j.ydbio.2005.05.032
Chen, Y., Götte, M., Liu, J., and Park, P. W. (2008). Microbial subversion of heparan sulfate proteoglycans. Mol. Cells 26, 415–426.
Cladera, J., Martin, I., and O'Shea, P. (2001). The fusion domain of HIV gp41 interacts specifically with heparan sulfate on the T-lymphocyte cell surface. EMBO J. 20, 19–26. doi: 10.1093/emboj/20.1.19
Clement, C., Tiwari, V., Scanlan, P. M., Valyi-Nagy, T., Yue, B. Y., and Shukla, D. (2006). A novel role for phagocytosis-like uptake in herpes simplex virus entry. J. Cell Biol. 174, 1009–1021. doi: 10.1083/jcb.200509155
Cole, C. L., Rushton, G., Jayson, G. C., and Avizienyte, E. (2014). Ovarian cancer cell heparan sulfate 6-O-sulfotransferases regulate an angiogenic program induced by heparin-binding epidermal growth factor (EGF)-like growth factor/EGF receptor signaling. J. Biol. Chem. 289, 10488–10501. doi: 10.1074/jbc.M113.534263
Connell, B. J., Baleux, F., Coic, Y. M., Clayette, P., Bonnaffé, D., and Lortat-Jacob, H. (2012). A synthetic heparan sulfate-mimetic peptide conjugated to a mini CD4 displays very high anti- HIV-1 activity independently of coreceptor usage. Chem. Biol. 19, 131–139. doi: 10.1016/j.chembiol.2011.12.009
Connell, B. J., and Lortat-Jacob, H. (2013). Human immunodeficiency virus and heparan sulfate: from attachment to entry inhibition. Front. Immunol. 4:385. doi: 10.3389/fimmu.2013.00385
Cooper, A., Tal, G., Lider, O., and Shaul, Y. (2005). Cytokine induction by the hepatitis B virus capsid in macrophages is facilitated by membrane heparan sulfate and involves TLR2. J. Immunol. 175, 3165–3176.
Copeland, R., Balasubramaniam, A., Tiwari, V., Zhang, F., Bridges, A., Linhardt, R. J., et al. (2008). Using a 3-O-sulfated heparin octasaccharide to inhibit the entry of herpes simplex virus type 1. Biochemistry 47, 5774–5783. doi: 10.1021/bi800205t
Correia-da-Silva, M., Sousa, E., Duarte, B., Marques, F., Carvalho, F., Cunha-Ribeiro, L. M., et al. (2011). Polysulfated xanthones: multipathway development of a new generation of dual anticoagulant/antiplatelet agents. J. Med. Chem. 54, 5373–5384. doi: 10.1021/jm2006589
Crublet, E., Andrieu, J. P., Vivès, R. R., and Lortat-Jacob, H. (2008). The HIV-1 envelope glycoprotein gp120 features four heparan sulfate binding domains, including the co-receptor binding site. J. Biol. Chem. 283, 15193–15200. doi: 10.1074/jbc.M800066200
Damonte, E. B., Matulewicz, M. C., Cerezo, A. S., and Coto, C. E. (1996). Herpes simplex virus-inhibitory sulfated xylogalactans from the red seaweed Nothogenia fastigiata. Chemotherapy 42, 57–64. doi: 10.1159/000239422
Datta, P., Li, G., Yang, B., Zhao, X., Baik, J. Y., Gemmill, T. R., et al. (2013). Bioengineered Chinese hamster ovary cells with golgi-targeted 3-O-sulfotransferase-1 biosynthesize heparan sulfate with an antithrombin-binding site. J. Biol. Chem. 288, 37308–37318. doi: 10.1074/jbc.M113.519033
de Agostini, A. I., Dong, J. C., de Vantéry Arrighi, C., Ramus, M. A., Dentand-Quadri, I., Thalmann, S., et al. (2008). Human follicular fluid heparan sulfate contains abundant 3-O-sulfated chains with anticoagulant activity. J. Biol. Chem. 283, 28115–28124. doi: 10.1074/jbc.M805338200
de Parseval, A., Bobardt, M. D., Chatterji, A., Chatterji, U., Elder, J. H., David, G., et al. (2005). A highly conserved arginine in gp120 governs HIV-1 binding to both syndecans and CCR5 via sulfated motifs. J. Biol. Chem. 280, 39493–39504. doi: 10.1074/jbc.M504233200
de Witte, L., Zoughlami, Y., Aengeneyndt, B., David, G., van Kooyk, Y., Gissmann, L., et al. (2007). Binding of human papilloma virus L1 virus-like particles to dendritic cells is mediated through heparan sulfates and induces immune activation. Immunobiology 212, 679–691. doi: 10.1016/j.imbio.2007.09.006
Deligny, A., Denys, A., Marcant, A., Melchior, A., Mazurier, J., van Kuppevelt, T. H., et al. (2010). Synthesis of heparan sulfate with cyclophilin B-binding properties is determined by cell type-specific expression of sulfotransferases. J. Biol. Chem. 285, 1701–1715. doi: 10.1074/jbc.M109.018184
Desai, U. R. (2013). The promise of sulfated synthetic small molecules as modulators of glycosaminoglycan function. Future Med. Chem. 5, 1363–1366. doi: 10.4155/fmc.13.117
Dong, L. Q., Wang, X. Q., Guo, Y. N., Wu, J., Li, S., Yu, P., et al. (2013). HS N-sulfation and iduronic acids play an important role in the infection of respiratory syncytial virus in vitro. Eur. Rev. Med. Pharmacol. Sci. 17, 1864–1868.
Dyer, A. P., Banfield, B. W., Martindale, D., Spannier, D. M., and Tufaro, F. (1997). Dextran sulfate can act as an artificial receptor to mediate a type-specific herpes simplex virus infection via glycoprotein B. J. Virol. 71, 191–198.
Dyer, D. P., Salanga, C. L., Johns, S. C., Valdambrini, E., Fuster, M. M., Milner, C. M., et al. (2016). The anti-inflammatory protein TSG-6 regulates chemokine function by inhibiting chemokine/glycosaminoglycan interactions. J. Biol. Chem. 291, 12627–12640. doi: 10.1074/jbc.M116.720953
El Najjar, F., Cifuentes-Muñoz, N., Chen, J., Zhu, H., Buchholz, U. J., Moncman, C. L., et al. (2016). Human metapneumovirus induces reorganization of the actin cytoskeleton for direct cell-to-cell spread. PLoS Pathog. 12:e1005922. doi: 10.1371/journal.ppat.1005922
Esko, J. D., and Lindahl, U. (2001). Molecular diversity of heparan sulfate. J. Clin. Invest. 108, 169–173. doi: 10.1172/JCI200113530
Esko, J. D., and Selleck, S. B. (2002). Order out of chaos: assembly of ligand binding sites in heparan sulfate. Annu. Rev. Biochem. 71, 435–471. doi: 10.1146/annurev.biochem.71.110601.135458
Fechtner, T., Stallmann, S., Moelleken, K., Meyer, K. L., and Hegemann, J. H. (2013). Characterization of the interaction between the chlamydial adhesin OmcB and the human host cell. J. Bacteriol. 195, 5323–5333. doi: 10.1128/JB.00780-13
Feldman, S. A., Audet, S., and Beeler, J. A. (2000). The fusion glycoprotein of human respiratory syncytial virus facilitates virus attachment and infectivity via an interaction with cellular heparan sulfate. J. Virol. 74, 6442–6447. doi: 10.1128/JVI.74.14.6442-6447.2000
Ferguson, B. W., and Datta, S. (2011). Role of heparan sulfate 2-o-sulfotransferase in prostate cancer cell proliferation, invasion, and growth factor signaling. Prostate Cancer 2011:893208. doi: 10.1155/2011/893208
Ferreras, C., Rushton, G., Cole, C. L., Babur, M., Telfer, B. A., van Kuppevelt, T. H., et al. (2012). Endothelial heparan sulfate 6-O-sulfation levels regulate angiogenic responses of endothelial cells to fibroblast growth factor 2 and vascular endothelial growth factor. J. Biol. Chem. 287, 36132–36146. doi: 10.1074/jbc.M112.384875
Feyzi, E., Trybala, E., Bergström, T., Lindahl, U., and Spillmann, D. (1997). Structural requirement of heparan sulfate for interaction with herpes simplex virus type 1 virions and isolated glycoprotein C. J. Biol. Chem. 272, 24850–24857. doi: 10.1074/jbc.272.40.24850
Gallay, P. (2004). Syndecans and HIV-1 pathogenesis. Microbes Infect. 6, 617–622. doi: 10.1016/j.micinf.2004.02.004
Gallegos, S., Pacheco, C., Peters, C., Opazo, C. M., and Aguayo, L. G. (2015). Features of alpha-synuclein that could explain the progression and irreversibility of Parkinson's disease. Front. Neurosci. 9:59. doi: 10.3389/fnins.2015.00059
Gangji, R. N., Sankaranarayanan, N. V., Elste, J., Al-Horani, R. A., Afosah, D. K., Joshi, R., et al. (2018). Inhibition of herpes simplex virus-1 entry into human cells by nonsaccharide glycosaminoglycan mimetics. ACS Med. Chem. Lett. 9, 797–802. doi: 10.1021/acsmedchemlett.7b00364
García, B., Merayo-Lloves, J., Rodriguez, D., Alcalde, I., Garcia-Suarez, O., Alfonso, J. F., et al. (2016). Different use of cell surface glycosaminoglycans as adherence receptors to corneal cells by gram positive and gram negative pathogens. Front. Cell. Infect. Microbiol. 6:173. doi: 10.3389/fcimb.2016.00173
Gardner, C. L., Ebel, G. D., Ryman, K. D., and Klimstra, W. B. (2011). Heparan sulfate binding by natural eastern equine encephalitis viruses promotes neurovirulence. Proc. Natl. Acad. Sci. U.S.A. 108, 16026–16031. doi: 10.1073/pnas.1110617108
Gerber, S. I., Belval, B. J., and Herold, B. C. (1995). Differences in the role of glycoprotein C of HSV-1 and HSV-2 in viral binding may contribute to serotype differences in cell tropism. Virology 214, 29–39. doi: 10.1006/viro.1995.9957
Goldberg, R., Meirovitz, A., Hirshoren, N., Bulvik, R., Binder, A., Rubinstein, A. M., et al. (2013). Versatile role of heparanase in inflammation. Matrix Biol. 32, 234–240. doi: 10.1016/j.matbio.2013.02.008
Guibinga, G. H., Miyanohara, A., Esko, J. D., and Friedmann, T. (2002). Cell surface heparan sulfate is a receptor for attachment of envelope protein-free retrovirus-like particles and VSV-G pseudotyped MLV-derived retrovirus vectors to target cells. Mol. Ther. 5, 538–546. doi: 10.1006/mthe.2002.0578
Hallak, L. K., Spillmann, D., Collins, P. L., and Peeples, M. E. (2000). Glycosaminoglycan sulfation requirements for respiratory syncytial virus infection. J. Virol. 74, 10508–10513. doi: 10.1128/JVI.74.22.10508-10513.2000
Hashimoto, M., Bhuyan, F., Hiyoshi, M., Noyori, O., Nasser, H., Miyazaki, M., et al. (2016). Potential role of the formation of tunneling nanotubes in HIV-1 spread in macrophages. J. Immunol. 196, 1832–1841. doi: 10.4049/jimmunol.1500845
Hasui, M., Matsuda, M., Okutani, K., and Shigeta, S. (1995). In vitro antiviral activities of sulfated polysaccharides from a marine microalga (Cochlodinium polykrikoides) against human immunodeficiency virus and other enveloped viruses. Int. J. Biol. Macromol. 17, 293–297. doi: 10.1016/0141-8130(95)98157-T
Hatabe, S., Kimura, H., Arao, T., Kato, H., Hayashi, H., Nagai, T., et al. (2013). Overexpression of heparan sulfate 6-O-sulfotransferase-2 in colorectal cancer. Mol. Clin. Oncol. 1, 845–850. doi: 10.3892/mco.2013.151
Hayashi, K., Hayashi, T., and Kojima, I. (1996). A natural sulfated polysaccharide, calcium spirulan, isolated from Spirulina platensis: in vitro and ex vivo evaluation of anti-herpes simplex virus and anti-human immunodeficiency virus activities. AIDS Res. Hum. Retroviruses 12, 1463–1471. doi: 10.1089/aid.1996.12.1463
Hayashi, T., Hayashi, K., Maeda, M., and Kojima, I. (1996). Calcium spirulan, an inhibitor of enveloped virus replication, from a blue-green alga Spirulina platensis. J. Nat. Prod. 59, 83–87. doi: 10.1021/np960017o
Hayashida, A., Amano, S., Gallo, R. L., Linhardt, R. J., Liu, J., and Park, P. W. (2015). 2-O-sulfated domains in syndecan-1 heparan sulfate inhibit neutrophil cathelicidin and promote Staphylococcus aureus corneal infection. J. Biol. Chem. 290, 16157–16167. doi: 10.1074/jbc.M115.660852
Herold, B. C., Gerber, S. I., Belval, B. J., Siston, A. M., and Shulman, N. (1996). Differences in the susceptibility of herpes simplex virus types 1 and 2 to modified heparin compounds suggest serotype differences in viral entry. J. Virol. 70, 3461–3469.
Herold, B. C., Gerber, S. I., Polonsky, T., Belval, B. J., Shaklee, P. N., and Holme, K. (1995). Identification of structural features of heparin required for inhibition of herpes simplex virus type 1 binding. Virology 206, 1108–1116. doi: 10.1006/viro.1995.1034
Herold, B. C., Siston, A., Bremer, J., Kirkpatrick, R., Wilbanks, G., Fugedi, P., et al. (1997). Sulfated carbohydrate compounds prevent microbial adherence by sexually transmitted disease pathogens. Antimicrob. Agents Chemother. 41, 2776–2780. doi: 10.1128/AAC.41.12.2776
Herrera, R., Morris, M., Rosbe, K., Feng, Z., Weinberg, A., and Tugizov, S. (2016). Human beta-defensins 2 and−3 cointernalize with human immunodeficiency virus via heparan sulfate proteoglycans and reduce infectivity of intracellular virions in tonsil epithelial cells. Virology 487, 172–187. doi: 10.1016/j.virol.2015.09.025
Hu, Y. P., Lin, S. Y., Huang, C. Y., Zulueta, M. M., Liu, J. Y., Chang, W., et al. (2011). Synthesis of 3-O-sulfonated heparan sulfate octasaccharides that inhibit the herpes simplex virus type 1 host-cell interaction. Nat. Chem. 3, 557–563. doi: 10.1038/nchem.1073
Hull, E. E., Montgomery, M. R., and Leyva, K. J. (2017). Epigenetic regulation of the biosynthesis & enzymatic modification of heparan sulfate proteoglycans: implications for tumorigenesis and cancer biomarkers. Int. J. Mol. Sci. 18:1361. doi: 10.3390/ijms18071361
Hwang, J. A., Kim, Y., Hong, S. H., Lee, J., Cho, Y. G., Han, J. Y., et al. (2013). Epigenetic inactivation of heparan sulfate (glucosamine) 3-O-sulfotransferase 2 in lung cancer and its role in tumorigenesis. PLoS ONE 8:e79634. doi: 10.1371/journal.pone.0079634
Ibrahim, J., Griffin, P., Coombe, D. R., Rider, C. C., and James, W. (1999). Cell-surface heparan sulfate facilitates human immunodeficiency virus type 1 entry into some cell lines but not primary lymphocytes. Virus Res. 60, 159–169. doi: 10.1016/S0168-1702(99)00018-0
Irie, A., Yates, E. A., Turnbull, J. E., and Holt, C. E. (2002). Specific heparan sulfate structures involved in retinal axon targeting. Development 129, 61–70.
Ishihara, C., Yoshimatsu, K., Tsuji, M., Arikawa, J., Saiki, I., Tokura, S., et al. (1993). Anti-viral activity of sulfated chitin derivatives against Friend murine leukaemia and herpes simplex type-1 viruses. Vaccine 11, 670–674. doi: 10.1016/0264-410X(93)90315-O
Jaishankar, D., Buhrman, J. S., Valyi-Nagy, T., Gemeinhart, R. A., and Shukla, D. (2016). Extended release of an anti–heparan sulfate peptide from a contact lens suppresses corneal herpes simplex virus-1 infection. Invest. Ophthalmol. Vis. Sci. 57, 169–180. doi: 10.1167/iovs.15-18365
Jiang, J., Cun, W., Wu, X., Shi, Q., Tang, H., and Luo, G. (2012). Hepatitis C virus attachment mediated by apolipoprotein E binding to cell surface heparan sulfate. J. Virol. 86, 7256–7267. doi: 10.1128/JVI.07222-11
Jiao, F., Bai, S. Y., Ma, Y., Yan, Z. H., Yue, Z., Yu, Y., et al. (2014). DNA methylation of heparanase promoter influences its expression and associated with the progression of human breast cancer. PLoS ONE 9:e92190. doi: 10.1371/journal.pone.0092190
Jinno, A., and Park, P. W. (2015). Role of glycosaminoglycans in infectious disease. Methods Mol. Biol. 1229, 567–585. doi: 10.1007/978-1-4939-1714-3_45
Kaever, T., Matho, M. H., Meng, X., Crickard, L., Schlossman, A., Xiang, Y., et al. (2016). Linear epitopes in vaccinia virus A27 are targets of protective antibodies induced by vaccination against smallpox. J. Virol. 90, 4334–4345. doi: 10.1128/JVI.02878-15
Khachigian, L. M., and Parish, C. R. (2004). Phosphomannopentaose sulfate (PI-88): heparan sulfate mimetic with clinical potential in multiple vascular pathologies. Cardiovasc. Drug Rev. 22, 1–6. doi: 10.1111/j.1527-3466.2004.tb00127.x
Kinnunen, T., Huang, Z., Townsend, J., Gatdula, M. M., Brown, J. R., Esko, J. D., et al. (2005). Heparan 2-O-sulfotransferase, hst-2, is essential for normal cell migration in Caenorhabditis elegans. Proc. Natl. Acad. Sci. U.S.A. 102, 1507–1512. doi: 10.1073/pnas.0401591102
Knelson, E. H., Nee, J. C., and Blobe, G. C. (2014). Heparan sulfate signaling in cancer. Trends Biochem. Sci. 39, 277–288. doi: 10.1016/j.tibs.2014.03.001
Kobayashi, F., Yamada, S., Taguwa, S., Kataoka, C., Naito, S., Hama, Y., et al. (2012). Specific interaction of the envelope glycoproteins E1 and E2 with liver heparan sulfate involved in the tissue tropismatic infection by hepatitis C virus. Glycoconj. J. 29, 211–220. doi: 10.1007/s10719-012-9388-z
Kumar, A. V., Katakam, S. K., Urbanowitz, A. K., and Gotte, M. (2015). Heparan sulphate as a regulator of leukocyte recruitment in inflammation. Curr. Protein Pept. Sci. 16, 77–86. doi: 10.2174/1573402111666150213165054
Lawrence, R., Yabe, T., Hajmohammadi, S., Rhodes, J., McNeely, M., Liu, J., et al. (2007). The principal neuronal gD-type 3-O-sulfotransferases and their products in central and peripheral nervous system tissues. Matrix Biol. 26, 442–455. doi: 10.1016/j.matbio.2007.03.002
Lee, J. B., Hayashi, K., Hashimoto, M., Nakano, T., and Hayashi, T. (2004a). Novel antiviral fucoidan from sporophyll of Undaria pinnatifida (Mekabu). Chem. Pharm. Bull. 52, 1091–1094. doi: 10.1248/cpb.52.1091
Lee, J. B., Hayashi, K., Hayashi, T., Sankawa, U., and Maeda, M. (1999). Antiviral activities against HSV-1, HCMV, and HIV-1 of rhamnan sulfate from Monostroma latissimum. Planta Med. 65, 439–441. doi: 10.1055/s-2006-960804
Lee, J. B., Hayashi, K., Maeda, M., and Hayashi, T. (2004b). Antiherpetic activities of sulfated polysaccharides from green algae. Planta Med. 70, 813–817. doi: 10.1055/s-2004-827228
Lin, Y. P., Bhowmick, R., Coburn, J., and Leong, J. M. (2015). Host cell heparan sulfate glycosaminoglycans are ligands for OspF-related proteins of the Lyme disease spirochete. Cell. Microbiol. 17, 1464–1476. doi: 10.1111/cmi.12448
Liu, J., Shriver, Z., Pope, R. M., Thorp, S. C., Duncan, M. B., Copeland, R. J., et al. (2002). Characterization of a heparan sulfate octasaccharide that binds to herpes simplex virus type 1 glycoprotein D. J. Biol. Chem. 277, 33456–33467. doi: 10.1074/jbc.M202034200
Liu, J., Shworak, N. W., Sinaý, P., Schwartz, J. J., Zhang, L., Fritze, L. M., et al. (1999). Expression of heparan sulfate D-glucosaminyl 3-O-sulfotransferase isoforms reveals novel substrate specificities. J. Biol. Chem. 274, 5185–5192. doi: 10.1074/jbc.274.8.5185
Liu, J., and Thorp, S. C. (2002). Cell surface heparan sulfate and its roles in assisting viral infections. Med. Res. Rev. 22, 1–25. doi: 10.1002/med.1026
Makkonen, K. E., Turkki, P., Laakkonen, J. P., Ylä-Herttuala, S., Marjomäki, V., and Airenne, K. J. (2013). 6-o- and N-sulfated syndecan-1 promotes baculovirus binding and entry into mammalian cells. J. Virol. 87, 11148–11159. doi: 10.1128/JVI.01919-13
Mao, X., Gauche, C., Coughtrie, M. W., Bui, C., Gulberti, S., Merhi-Soussi, F., et al. (2016). The heparan sulfate sulfotransferase 3-OST3A (HS3ST3A) is a novel tumor regulator and a prognostic marker in breast cancer. Oncogene 35, 5043–5055. doi: 10.1038/onc.2016.44
Marchetti, M., Pisani, S., Pietropaolo, V., Seganti, L., Nicoletti, R., and Orsi, N. (1995). Inhibition of herpes simplex virus infection by negatively charged and neutral carbohydrate polymers. J. Chemother. 7, 90–96. doi: 10.1179/joc.1995.7.2.90
Marcum, J. A., Reilly, C. F., and Rosenberg, R. D. (1986). The role of specific forms of heparan sulfate in regulating blood vessel wall function. Prog. Hemost. Thromb. 8, 185–215.
Martin, L., De Santis, R., Koczera, P., Simons, N., Haase, H., Heinbockel, L., et al. (2015). The synthetic antimicrobial peptide 19-2.5 interacts with heparanase and heparan sulfate in murine and human sepsis. PLoS ONE 10:e0143583. doi: 10.1371/journal.pone.0143583
Martinho, R. G., Castel, S., Ureña, J., Fernández-Borja, M., Makiya, R., Olivecrona, G., et al. (1996). Ligand binding to heparan sulfate proteoglycans induces their aggregation and distribution along actin cytoskeleton. Mol. Biol. Cell 7, 1771–1788. doi: 10.1091/mbc.7.11.1771
Matos, P. M., Andreu, D., Santos, N. C., and Gutierrez-Gallego, R. (2014). Structural requirements of glycosaminoglycans for their interaction with HIV-1 envelope glycoprotein gp120. Arch. Virol. 159, 555–560. doi: 10.1007/s00705-013-1831-3
Mazumder, S., Ghosal, P. K., Pujol, C. A., Carlucci, M. J., Damonte, E. B., and Ray, B. (2002). Isolation, chemical investigation and antiviral activity of polysaccharides from Gracilaria corticata (Gracilariaceae, Rhodophyta). Int. J. Biol. Macromol. 31, 87–95. doi: 10.1016/S0141-8130(02)00070-3
Milho, R., Frederico, B., Efstathiou, S., and Stevenson, P. G. (2012). A heparan-dependent herpesvirus targets the olfactory neuroepithelium for host entry. PLoS Pathog. 8:e1002986. doi: 10.1371/journal.ppat.1002986
Miyamoto, K., Asada, K., Fukutomi, T., Okochi, E., Yagi, Y., Hasegawa, T., et al. (2003). Methylation-associated silencing of heparan sulfate D-glucosaminyl 3-O-sulfotransferase-2 (3-OST-2) in human breast, colon, lung and pancreatic cancers. Oncogene 22, 274–280. doi: 10.1038/sj.onc.1206146
Mochizuki, H., Yoshida, K., Gotoh, M., Sugioka, S., Kikuchi, N., Kwon, Y. D., et al. (2003). Characterization of a heparan sulfate 3-O-sulfotransferase-5, an enzyme synthesizing a tetrasulfated disaccharide. J. Biol. Chem. 278, 26780–26787. doi: 10.1074/jbc.M301861200
Mohamed, S., and Coombe, D. R. (2017). Heparin mimetics: their therapeutic potential. Pharmaceuticals (Basel). 10:E78. doi: 10.3390/ph10040078
Monneau, Y., Arenzana-Seisdedos, F., and Lortat-Jacob, H. (2016). The sweet spot: how GAGs help chemokines guide migrating cells. J. Leukoc. Biol. 99, 935–953. doi: 10.1189/jlb.3MR0915-440R
Nahmias, A. J., and Kibrick, S. (1964). Inhibitory effect of heparin on herpes simplex virus. J. Bacteriol. 87, 1060–1066.
Neyts, J., Reymen, D., Letourneur, D., Jozefonvicz, J., Schols, D., Este, J., et al. (1995). Differential antiviral activity of derivatized dextrans. Biochem. Pharmacol. 50, 743–751. doi: 10.1016/0006-2952(95)00193-4
Nishida, M., Kozakai, T., Nagami, K., Kanamaru, Y., and Yabe, T. (2014). Structural alteration of cell surface heparan sulfate through the stimulation of the signaling pathway for heparan sulfate 6-O-sulfotransferase-1 in mouse fibroblast cells. Biosci. Biotechnol. Biochem. 78, 770–779. doi: 10.1080/09168451.2014.905178
O'Hearn, A., Wang, M., Cheng, H., Lear-Rooney, C. M., Koning, K., Rumschlag-Booms, E., et al. (2015). Role of EXT1 and glycosaminoglycans in the early stage of filovirus entry. J. Virol. 89, 5441–5449. doi: 10.1128/JVI.03689-14
Ohshiro, Y., Murakami, T., Matsuda, K., Nishioka, K., Yoshida, K., and Yamamoto, N. (1996). Role of cell surface glycosaminoglycans of human T cells in human immunodeficiency virus type-1 (HIV-1) infection. Microbiol. Immunol. 40, 827–835. doi: 10.1111/j.1348-0421.1996.tb01148.x
Omsland, M., Pise-Masison, C., Fujikawa, D., Galli, V., Fenizia, C., Parks, R. W., et al. (2018). Inhibition of tunneling nanotube (TNT) formation and human T-cell lukemia vrus type 1 (HTLV-1) transmission by cytarabine. Sci. Rep. 8:11118. doi: 10.1038/s41598-018-29391-w
Onfelt, B., Nedvetzki, S., Yanagi, K., and Davis, D. M. (2004). Cutting edge: Membrane nanotubes connect immune cells. J. Immunol. 173, 1511–1513. doi: 10.4049/jimmunol.173.3.1511
Parish, C. R., Freeman, C., Ziolkowski, A. F., He, Y. Q., Sutcliffe, E. L., Zafar, A., et al. (2013). Unexpected new roles for heparanase in Type 1 diabetes and immune gene regulation. Matrix Biol. 32, 228–233. doi: 10.1016/j.matbio.2013.02.007
Park, P. J., and Shukla, D. (2013). Role of heparan sulfate in ocular diseases. Exp. Eye Res. 110, 1–9. doi: 10.1016/j.exer.2013.01.015
Patel, M., Yanagishita, M., Roderiquez, G., Bou-Habib, D. C., Oravecz, T., Hascall, V. C., et al. (1993). Cell-surface heparan sulfate proteoglycan mediates HIV-1 infection of T-cell lines. AIDS Res. Hum. Retroviruses 9, 167–174. doi: 10.1089/aid.1993.9.167
Ponce, N. M., Pujol, C. A., Damonte, E. B., Flores, M. L., and Stortz, C. A. (2003). Fucoidans from the brown seaweed Adenocystis utricularis: extraction methods, antiviral activity and structural studies. Carbohydr. Res. 338, 153–165. doi: 10.1016/S0008-6215(02)00403-2
Preeprame, S., Hayashi, K., Lee, J. B., Sankawa, U., and Hayashi, T. (2001). A novel antivirally active fucan sulfate derived from an edible brown alga, Sargassum horneri. Chem. Pharm. Bull. 49, 484–485. doi: 10.1248/cpb.49.484
Raman, K., and Kuberan, B. (2010). Chemical tumor biology of heparan sulfate proteoglycans. Curr. Chem. Biol. 4, 20–31. doi: 10.2174/187231310790226206
Raman, K., Mencio, C., Desai, U. R., and Kuberan, B. (2013). Sulfation patterns determine cellular internalization of heparin-like polysaccharides. Mol. Pharm. 10, 1442–1449. doi: 10.1021/mp300679a
Raman, R., Tharakaraman, K., Sasisekharan, V., and Sasisekharan, R. (2016). Glycan-protein interactions in viral pathogenesis. Curr. Opin. Struct. Biol. 40, 153–162. doi: 10.1016/j.sbi.2016.10.003
Riblett, A. M., Blomen, V. A., Jae, L. T., Altamura, L. A., Doms, R. W., Brummelkamp, T. R., et al. (2016). A haploid genetic screen identifies heparan sulfate proteoglycans supporting rift valley fever virus Infection. J. Virol. 90, 1414–1423. doi: 10.1128/JVI.02055-15
Richards, K. F., Bienkowska-Haba, M., Dasgupta, J., Chen, X. S., and Sapp, M. (2013). Multiple heparan sulfate binding site engagements are required for the infectious entry of human papillomavirus type 16. J. Virol. 87, 11426–11437. doi: 10.1128/JVI.01721-13
Roderiquez, G., Oravecz, T., Yanagishita, M., Bou-Habib, D. C., Mostowski, H., and Norcross, M. A. (1995). Mediation of human immunodeficiency virus type 1 binding by interaction of cell surface heparan sulfate proteoglycans with the V3 region of envelope gp120-gp41. J. Virol. 69, 2233–2239.
Rustom, A., Saffrich, R., Markovic, I., Walther, P., and Gerdes, H. H. (2004). Nanotubular highways for intercellular organelle transport. Science 303, 1007–1010. doi: 10.1126/science.1093133
Ryman, K. D., Gardner, C. L., Burke, C. W., Meier, K. C., Thompson, J. M., and Klimstra, W. B. (2007). Heparan sulfate binding can contribute to the neurovirulence of neuroadapted and nonneuroadapted sindbis viruses. J. Virol. 81, 3563–3573. doi: 10.1128/JVI.02494-06
Samson, S. C., Ferrer, T., Jou, C. J., Sachse, F. B., Shankaran, S. S., Shaw, R. M., et al. (2013). 3-OST-7 regulates BMP-dependent cardiac contraction. PLoS Biol. 11:e1001727. doi: 10.1371/journal.pbio.1001727
Sanderson, R. D., Elkin, M., Rapraeger, A. C., Ilan, N., and Vlodavsky, I. (2017). Heparanase regulation of cancer, autophagy and inflammation: new mechanisms and targets for therapy. FEBS J. 284, 42–55. doi: 10.1111/febs.13932
Saphire, A. C., Bobardt, M. D., Zhang, Z., David, G., and Gallay, P. A. (2001). Syndecans serve as attachment receptors for human immunodeficiency virus type 1 on macrophages. J. Virol. 75, 9187–9200. doi: 10.1128/JVI.75.19.9187-9200.2001
Sasisekharan, R., and Venkataraman, G. (2000). Heparin and heparan sulfate: biosynthesis, structure and function. Curr. Opin. Chem. Biol. 4, 626–631. doi: 10.1016/S1367-5931(00)00145-9
Sedita, J., Izvolsky, K., and Cardoso, W. V. (2004). Differential expression of heparan sulfate 6-O-sulfotransferase isoforms in the mouse embryo suggests distinctive roles during organogenesis. Dev. Dyn. 231, 782–794. doi: 10.1002/dvdy.20173
Shannon-Lowe, C., and Rowe, M. (2011). Epstein-Barr virus infection of polarized epithelial cells via the basolateral surface by memory B cell-mediated transfer infection. PLoS Pathog. 7:e1001338. doi: 10.1371/journal.ppat.1001338
Shteper, P. J., Zcharia, E., Ashhab, Y., Peretz, T., Vlodavsky, I., and Ben-Yehuda, D. (2003). Role of promoter methylation in regulation of the mammalian heparanase gene. Oncogene 22, 7737–7749. doi: 10.1038/sj.onc.1207056
Shukla, D., Liu, J., Blaiklock, P., Shworak, N. W., Bai, X., Esko, J. D., et al. (1999). A novel role for 3-O-sulfated heparan sulfate in herpes simplex virus 1 entry. Cell 99, 13–22. doi: 10.1016/S0092-8674(00)80058-6
Shukla, D., and Spear, P. G. (2001). Herpesviruses and heparan sulfate: an intimate relationship in aid of viral entry. J. Clin. Invest. 108, 503–510. doi: 10.1172/JCI200113799
Shworak, N. W., Liu, J., Petros, L. M., Zhang, L., Kobayashi, M., Copeland, N. G., et al. (1999). Multiple isoforms of heparan sulfate D-glucosaminyl 3-O-sulfotransferase. Isolation, characterization, and expression of human cdnas and identification of distinct genomic loci. J. Biol. Chem. 274, 5170–5184. doi: 10.1074/jbc.274.8.5170
Sidhu, P. S., Abdel Aziz, M. H., Sarkar, A., Mehta, A. Y., Zhou, Q., and Desai, U. R. (2013). Designing allosteric regulators of thrombin. Exosite 2 features multiple subsites that can be targeted by sulfated small molecules for inducing inhibition. J. Med. Chem. 56, 5059–5070. doi: 10.1021/jm400369q
Sikora, A. S., Delos, M., Martinez, P., Carpentier, M., Allain, F., and Denys, A. (2016). Regulation of the expression of heparan sulfate 3-O-sulfotransferase 3B (HS3ST3B) by inflammatory stimuli in human monocytes. J. Cell. Biochem. 117, 1529–1542. doi: 10.1002/jcb.25444
Silva, L. A., Khomandiak, S., Ashbrook, A. W., Weller, R., Heise, M. T., Morrison, T. E., et al. (2014). A single-amino-acid polymorphism in Chikungunya virus E2 glycoprotein influences glycosaminoglycan utilization. J. Virol. 88, 2385–2397. doi: 10.1128/JVI.03116-13
Sinnis, P., Coppi, A., Toida, T., Toyoda, H., Kinoshita-Toyoda, A., Xie, J., et al. (2007). Mosquito heparan sulfate and its potential role in malaria infection and transmission. J. Biol. Chem. 282, 25376–25384. doi: 10.1074/jbc.M704698200
Somiya, M., Liu, Q., Yoshimoto, N., Iijima, M., Tatematsu, K., Nakai, T., et al. (2016). Cellular uptake of hepatitis B virus envelope L particles is independent of sodium taurocholate cotransporting polypeptide, but dependent on heparan sulfate proteoglycan. Virology 497, 23–32. doi: 10.1016/j.virol.2016.06.024
Song, K., Li, Q., Jiang, Z. Z., Guo, C. W., and Li, P. (2011). Heparan sulfate D-glucosaminyl 3-O-sulfotransferase-3B1, a novel epithelial-mesenchymal transition inducer in pancreatic cancer. Cancer Biol. Ther. 12, 388–398. doi: 10.4161/cbt.12.5.15957
Sowinski, S., Jolly, C., Berninghausen, O., Purbhoo, M. A., Chauveau, A., Köhler, K., et al. (2008). Membrane nanotubes physically connect T cells over long distances presenting a novel route for HIV-1 transmission. Nat. Cell Biol. 10, 211–219. doi: 10.1038/ncb1682
Stanford, K. I., Wang, L., Castagnola, J., Song, D., Bishop, J. R., Brown, J. R., et al. (2010). Heparan sulfate 2-O-sulfotransferase is required for triglyceride-rich lipoprotein clearance. J. Biol. Chem. 285, 286–294. doi: 10.1074/jbc.M109.063701
Takahashi, I., Ohashi, K., and Nata, K. (2012). Involvement of heparan sulfate 3-O-sulfotransferase isoform-1 in the insulin secretion pathway. J. Diabetes Investig. 3, 362–370. doi: 10.1111/j.2040-1124.2012.00205.x
Tal-Singer, R., Peng, C., Ponce De Leon, M., Abrams, W. R., Banfield, B. W., Tufaro, F., et al. (1995). Interaction of herpes simplex virus glycoprotein gC with mammalian cell surface molecules. J. Virol. 69, 4471–4483.
Tan, C. W., Poh, C. L., Sam, I. C., and Chan, Y. F. (2013). Enterovirus 71 uses cell surface heparan sulfate glycosaminoglycan as an attachment receptor. J. Virol. 87, 611–620. doi: 10.1128/JVI.02226-12
Tanaka, A., Jinno-Oue, A., Shimizu, N., Hoque, A., Mori, T., Islam, S., et al. (2012). Entry of human T-cell leukemia virus type 1 is augmented by heparin sulfate proteoglycans bearing short heparin-like structures. J. Virol. 86, 2959–2969. doi: 10.1128/JVI.05783-11
Tanaka, A., Tumkosit, U., Nakamura, S., Motooka, D., Kishishita, N., Priengprom, T., et al. (2017). Genome-wide screening uncovers the significance of N-sulfation of heparan sulfate as a host cell factor for Chikungunya virus Infection. J. Virol. 91, 1–22. doi: 10.1128/JVI.00432-17
Taylor, K. R., and Gallo, R. L. (2006). Glycosaminoglycans and their proteoglycans: host-associated molecular patterns for initiation and modulation of inflammation. FASEB J. 20, 9–22. doi: 10.1096/fj.05-4682rev
Teixé, T., Nieto-Blanco, P., Vilella, R., Engel, P., Reina, M., and Espel, E. (2008). Syndecan-2 and−4 expressed on activated primary human CD4+ lymphocytes can regulate T cell activation. Mol. Immunol. 45, 2905–2919. doi: 10.1016/j.molimm.2008.01.033
Thacker, B. E., Seamen, E., Lawrence, R., Parker, M. W., Xu, Y., Liu, J., et al. (2016). Expanding the 3-O-sulfate proteome–enhanced binding of neuropilin-1 to 3-O-sulfated heparan sulfate modulates its activity. ACS Chem. Biol. 11, 971–980. doi: 10.1021/acschembio.5b00897
Thacker, B. E., Xu, D., Lawrence, R., and Esko, J. D. (2014). Heparan sulfate 3-O-sulfation: a rare modification in search of a function. Matrix Biol. 35, 60–72. doi: 10.1016/j.matbio.2013.12.001
Thompson, K. D., and Dragar, C. (2004). Antiviral activity of Undaria pinnatifida against herpes simplex virus. Phytother. Res. 18, 551–555. doi: 10.1002/ptr.1487
Tillo, M., Charoy, C., Schwarz, Q., Maden, C. H., Davidson, K., Fantin, A., et al. (2016). 2- and 6-O-sulfated proteoglycans have distinct and complementary roles in cranial axon guidance and motor neuron migration. Development 143, 1907–1913. doi: 10.1242/dev.126854
Tiwari, V., Clement, C., Duncan, M. B., Chen, J., Liu, J., and Shukla, D. (2004). A role for 3-O-sulfated heparan sulfate in cell fusion induced by herpes simplex virus type 1. J. Gen. Virol. 85, 805–809. doi: 10.1099/vir.0.19641-0
Tiwari, V., Clement, C., Xu, D., Valyi-Nagy, T., Yue, B. Y., Liu, J., et al. (2006). Role for 3-O-sulfated heparan sulfate as the receptor for herpes simplex virus type 1 entry into primary human corneal fibroblasts. J. Virol. 80, 8970–8980. doi: 10.1128/JVI.00296-06
Tiwari, V., Liu, J., Valyi-Nagy, T., and Shukla, D. (2011). Anti-heparan sulfate peptides that block herpes simplex virus infection in vivo. J. Biol. Chem. 286, 25406–25415. doi: 10.1074/jbc.M110.201103
Tiwari, V., Maus, E., Sigar, I. M., Ramsey, K. H., and Shukla, D. (2012). Role of heparan sulfate in sexually transmitted infections. Glycobiology 22, 1402–1412. doi: 10.1093/glycob/cws106
Tiwari, V., ten Dam, G. B., Yue, B. Y., van Kuppevelt, T. H., and Shukla, D. (2007). Role of 3-O-sulfated heparan sulfate in virus-induced polykaryocyte formation. FEBS Lett. 581, 4468–4472. doi: 10.1016/j.febslet.2007.08.029
Trottein, F., Schaffer, L., Ivanov, S., Paget, C., Vendeville, C., Cazet, A., et al. (2009). Glycosyltransferase and sulfotransferase gene expression profiles in human monocytes, dendritic cells and macrophages. Glycoconj. J. 26, 1259–1274. doi: 10.1007/s10719-009-9244-y
Trybala, E., Liljeqvist, J. A., Svennerholm, B., and Bergström, T. (2000). Herpes simplex virus types 1 and 2 differ in their interaction with heparan sulfate. J. Virol. 74, 9106–9114. doi: 10.1128/JVI.74.19.9106-9114.2000
Tsidulko, A. Y., Matskova, L., Astakhova, L. A., Ernberg, I., and Grigorieva, E. V. (2015). Proteoglycan expression correlates with the phenotype of malignant and non-malignant EBV-positive B-cell lines. Oncotarget 6, 43529–43539. doi: 10.18632/oncotarget.5984
Urbinati, C., Nicoli, S., Giacca, M., David, G., Fiorentini, S., Caruso, A., et al. (2009). HIV-1 Tat and heparan sulfate proteoglycan interaction: a novel mechanism of lymphocyte adhesion and migration across the endothelium. Blood 114, 3335–3342. doi: 10.1182/blood-2009-01-198945
van Wijk, X. M. R., and van Kuppevelt, T. H. (2014). Heparan sulfate in angiogenesis: a target for therapy. Angiogenesis 17, 443–462. doi: 10.1007/s10456-013-9401-6
Varki, A. (2002). Six blind men and the elephant–the many faces of heparan sulfate. Proc. Natl. Acad. Sci. U.S.A. 99, 543–545. doi: 10.1073/pnas.022649499
Vidulescu, C., Clejan, S., and O'Connor, K. C. (2004). Vesicle traffic through intercellular bridges in DU 145 human prostate cancer cells. J. Cell. Mol. Med. 8, 388–396. doi: 10.1111/j.1582-4934.2004.tb00328.x
Vivès, R. R., Imberty, A., Sattentau, Q. J., and Lortat-Jacob, H. (2005). Heparan sulfate targets the HIV-1 envelope glycoprotein gp120 coreceptor binding site. J. Biol. Chem. 280, 21353–21357. doi: 10.1074/jbc.M500911200
Voss, J. E., Vaney, M. C., Duquerroy, S., Vonrhein, C., Girard-Blanc, C., Crublet, E., et al. (2010). Glycoprotein organization of chikungunya virus particles revealed by X-ray crystallography. Nature 468, 709–712. doi: 10.1038/nature09555
Wang, K. S., Wang, L., Liu, X., and Zeng, M. (2013). Association of HS6ST3 gene polymorphisms with obesity and triglycerides: gene x gender interaction. J. Genet. 92, 395–402. doi: 10.1007/s12041-013-0279-2
Wang, W., Ju, X., Sun, Z., Hou, W., Yang, L., and Zhang, R. (2015). Overexpression of heparan sulfate 6-O-sulfotransferase-2 enhances fibroblast growth factor-mediated chondrocyte growth and differentiation. Int. J. Mol. Med. 36, 825–832. doi: 10.3892/ijmm.2015.2272
Whittall, C., Kehoe, O., King, S., Rot, A., Patterson, A., and Middleton, J. (2013). A chemokine self-presentation mechanism involving formation of endothelial surface microstructures. J. Immunol. 190, 1725–1736. doi: 10.4049/jimmunol.1200867
Witvrouw, M., and De Clercq, E. (1997). Sulfated polysaccharides extracted from sea algae as potential antiviral drugs. Gen. Pharmacol. 29, 497–511. doi: 10.1016/S0306-3623(96)00563-0
Woodard, K. T., Liang, K. J., Bennett, W. C., and Samulski, R. J. (2016). Heparan sulfate binding promotes accumulation of intravitreally delivered adeno-associated viral vectors at the retina for enhanced transduction but weakly influences tropism. J. Virol. 90, 9878–9888. doi: 10.1128/JVI.01568-16
Wu, Z., Chen, Z., and Phillips, D. M. (2003). Human genital epithelial cells capture cell-free human immunodeficiency virus type 1 and transmit the virus to CD4+ Cells: implications for mechanisms of sexual transmission. J. Infect. Dis. 188, 1473–1482. doi: 10.1086/379248
WuDunn, D., and Spear, P. G. (1989). Initial interaction of herpes simplex virus with cells is binding to heparan sulfate. J. Virol. 63, 52–58.
Xia, G., Chen, J., Tiwari, V., Ju, W., Li, J. P., Malmstrom, A., et al. (2002). Heparan sulfate 3-O-sulfotransferase isoform 5 generates both an antithrombin-binding site and an entry receptor for herpes simplex virus, type 1. J. Biol. Chem. 277, 37912–37919. doi: 10.1074/jbc.M204209200
Xia, L., Xian, Y., Wang, D., Chen, Y., Huang, X., Bi, X., et al. (2016). A human monoclonal antibody against HPV16 recognizes an immunodominant and neutralizing epitope partially overlapping with that of H16.V5. Sci. Rep. 6:19042. doi: 10.1038/srep19042
Xu, D., Fuster, M. M., Lawrence, R., and Esko, J. D. (2011). Heparan sulfate regulates VEGF165- and VEGF121-mediated vascular hyperpermeability. J. Biol. Chem. 286, 737–745. doi: 10.1074/jbc.M110.177006
Xu, D., Olson, J., Cole, J. N., van Wijk, X. M., Brinkmann, V., Zychlinsky, A., et al. (2015). Heparan sulfate modulates neutrophil and endothelial function in antibacterial innate immunity. Infect. Immun. 83, 3648–3656. doi: 10.1128/IAI.00545-15
Yanagishita, M., and Hascall, V. C. (1992). Cell surface heparan sulfate proteoglycans. J. Biol. Chem. 267, 9451–9454.
Yun, S. I., Song, B. H., Kim, J. K., Yun, G. N., Lee, E. Y., Li, L., et al. (2014). A molecularly cloned, live-attenuated japanese encephalitis vaccine SA14-14-2 virus: a conserved single amino acid in the ij hairpin of the viral E glycoprotein determines neurovirulence in mice. PLoS Pathog. 10:e1004290. doi: 10.1371/journal.ppat.1004290
Zacharopoulos, V. R., and Phillips, D. M. (1997). Vaginal formulations of carrageenan protect mice from herpes simplex virus infection. Clin. Diagn. Lab. Immunol. 4, 465–468.
Zautner, A. E., Jahn, B., Hammerschmidt, E., Wutzler, P., and Schmidtke, M. (2006). N- and 6-O-sulfated heparan sulfates mediate internalization of coxsackievirus B3 variant PD into CHO-K1 cells. J. Virol. 80, 6629–6636. doi: 10.1128/JVI.01988-05
Zhang, L., Lawrence, R., Schwartz, J. J., Bai, X., Wei, G., Esko, J. D., et al. (2001). The effect of precursor structures on the action of glucosaminyl 3-O-sulfotransferase-1 and the biosynthesis of anticoagulant heparan sulfate. J. Biol. Chem. 276, 28806–28813. doi: 10.1074/jbc.M100204200
Zhang, L., Song, K., Zhou, L., Xie, Z., Zhou, P., Zhao, Y., et al. (2015a). Heparan sulfate D-glucosaminyl 3-O-sulfotransferase-3B1 (HS3ST3B1) promotes angiogenesis and proliferation by induction of VEGF in acute myeloid leukemia cells. J. Cell. Biochem. 116, 1101–1112. doi: 10.1002/jcb.25066
Zhang, W. W., Zhang, Y. B., Zhao, X. X., Hua, Y., Wu, Z. L., Yan, Y. C., et al. (2015b). Prmt7 regulates epiboly by facilitating 2-OST and modulating actin cytoskeleton. J. Mol. Cell Biol. 7, 489–491. doi: 10.1093/jmcb/mjv040
Zhang, Y. J., Hatziioannou, T., Zang, T., Braaten, D., Luban, J., Goff, S. P., et al. (2002). Envelope-dependent, cyclophilin-independent effects of glycosaminoglycans on human immunodeficiency virus type 1 attachment and infection. J. Virol. 76, 6332–6343. doi: 10.1128/JVI.76.12.6332-6343.2002
Zhang, Z., Liu, X., Chen, J., Su, H., Luo, Q., Ye, J., et al. (2010). Heparin sulphate D-glucosaminyl 3-O-sulfotransferase 3B1 plays a role in HBV replication. Virology 406, 280–285. doi: 10.1016/j.virol.2010.07.030
Zhao, S., Deng, C., Wang, Z., Teng, L., and Chen, J. (2015). Heparan sulfate 6-O-sulfotransferase 3 is involved in bone marrow mesenchymal stromal cell osteogenic differentiation. Biochemistry 80, 379–389. doi: 10.1134/S000629791503013X
Zhou, W., Hsieh, P. H., Xu, Y., O'Leary, T. R., Huang, X., and Liu, J. (2015). Design and synthesis of active heparan sulfate-based probes. Chem. Commun. 51, 11019–11021. doi: 10.1039/C5CC02008E
Keywords: heparan sulfate, herpes simplex virus, heparanse, sulfotranferases, heparan mimetic, viral entry
Citation: Kaltenbach DD, Jaishankar D, Hao M, Beer JC, Volin MV, Desai UR and Tiwari V (2018) Sulfotransferase and Heparanase: Remodeling Engines in Promoting Virus Infection and Disease Development. Front. Pharmacol. 9:1315. doi: 10.3389/fphar.2018.01315
Received: 10 September 2018; Accepted: 29 October 2018;
Published: 22 November 2018.
Edited by:
Chandravanu Dash, Meharry Medical College, United StatesReviewed by:
Yu Bai, Novo Nordisk, ChinaChunliang Li, St. Jude Children's Research Hospital, United States
Copyright © 2018 Kaltenbach, Jaishankar, Hao, Beer, Volin, Desai and Tiwari. This is an open-access article distributed under the terms of the Creative Commons Attribution License (CC BY). The use, distribution or reproduction in other forums is permitted, provided the original author(s) and the copyright owner(s) are credited and that the original publication in this journal is cited, in accordance with accepted academic practice. No use, distribution or reproduction is permitted which does not comply with these terms.
*Correspondence: Vaibhav Tiwari, dnRpd2FyQG1pZHdlc3Rlcm4uZWR1