- 1School of Biosciences, Taylor’s University, Subang Jaya, Malaysia
- 2Division of Genetics and Molecular Biology, Institute of Biological Sciences, Faculty of Science, University of Malaya, Kuala Lumpur, Malaysia
- 3International Genome Centre, Jiangsu University, Zhenjiang, China
- 4Biofunctional Molecule Exploratory Research Group, School of Pharmacy, Monash University Malaysia, Bandar Sunway, Malaysia
- 5Novel Bacteria and Drug Discovery Research Group, School of Pharmacy, Monash University Malaysia, Bandar Sunway, Malaysia
- 6Asian Centre for Evidence Synthesis in Population, Implementation and Clinical Outcomes, Health and Well-Being Cluster, Global Asia in the 21st Century Platform, Monash University Malaysia, Bandar Sunway, Malaysia
- 7Center of Health Outcomes Research and Therapeutic Safety, School of Pharmaceutical Sciences, University of Phayao, Phayao, Thailand
Cardiovascular diseases (CVDs) are closely linked to cellular oxidative stress and inflammation. This may be resulted from the imbalance generation of reactive oxygen species and its role in promoting inflammation, thereby contributing to endothelial dysfunction and cardiovascular complications. Nuclear factor erythroid 2-related factor 2 (Nrf2) is a transcription factor that plays a significant role in regulating expression of antioxidant and cytoprotective enzymes in response to oxidative stress. Natural products have emerged as a potential source of bioactive compounds which have shown to protect against atherogenesis development by activating Nrf2 signaling. This review aims to provide a comprehensive summary of the published data on the function, regulation and activation of Nrf2 as well as the molecular mechanisms of natural products in regulating Nrf2 signaling. The beneficial effects of using natural bioactive compounds as a promising therapeutic approach for the prevention and treatment of CVDs are reviewed.
Introduction
Cardiovascular disease (CVD) is a major health complication which accounts for 15.2 million deaths worldwide in 2016 (World Health Organization, 2018). Atherosclerosis, as characterized by the formation of plaques with bulks of modified low density lipoprotein (LDL), immune cells, smooth muscle cells and cellular debris in the arterial intima, is the primary cause of CVD. The molecular mechanisms underlying CVD have been extensively investigated over the past decades. It has been demonstrated that the involvement of oxidative stress and inflammation are associated with the pathogenesis of CVD. Oxidative stress which results from excessive generation of reactive oxygen species/reactive nitrogen species (ROS/RNS) can trigger inflammation, which contribute to LDL oxidation, endothelial dysfunction, atherosclerotic plaque formation, plaque rupture, vascular remodeling, and atherothrombosis (Pashkow, 2011; Hajjar and Gotto, 2013; Hussain et al., 2016). In response to increased ROS/RNS levels under oxidative stress condition, the cells will induce the expression of antioxidant proteins and phase II detoxification enzymes such as heme oxygenase 1 (HO-1), aldo-keto reductase (AKR), peroxiredoxin 1 (PRX), γ-glutamyl cysteine ligase (γ-GCL) glutamate-cysteine ligase modifier subunit (GCLM), superoxide dismutase (SOD), NADPH quinine oxidoreductase 1 (NQO1) and others (Menegon et al., 2016; Jeddi et al., 2017). Transcriptional regulation of these enzymes is mainly controlled by nuclear factor erythroid 2-related factor 2 (Nrf2), a transcription factor that plays as a central role in intracellular redox homeostasis. In addition, Nrf2 also protects against macrophage foam cells formation by regulating expression of scavenger receptors, ATP-binding cassette (ABC) transporters, and multidrug resistance-associated proteins (MRPs) (Jeddi et al., 2017; Ooi et al., 2017). For instance, deficiency of Nrf2 in the bone marrow has been shown to aggravate atherosclerosis in LDL receptor-null (LDLR-/-) mice (Collins et al., 2012; Ruotsalainen et al., 2013). These evidences support the notion that Nrf2 protects against atherosclerosis. Although some studies showed that Nrf2 exhibits pro-atherogenic effects, its molecular mechanisms remain unclear (Sussan et al., 2008; Barajas et al., 2011; Freigang et al., 2011; Harada et al., 2012; Ruotsalainen et al., 2018).
Natural products offer unique structural and chemical diversity that serve as a source of novel drug leads and therapeutic agents. Natural products have been shown to alleviate oxidative stress-induced diseases such as CVD, neurodegenerative diseases, cancer and metabolic disorders by regulating the Nrf2/antioxidant responsive element (ARE) pathway (Waltenberger et al., 2016; Basak et al., 2017; Matzinger et al., 2017). Natural products derived from olive oil (hydroxytyrosol) and red wine (resveratrol) have been demonstrated to inhibit ROS production. Meanwhile, both bioactive compounds have also been reported to enhance Nrf2 nuclear translocation and decrease miRNA-146a expression, a pro-inflammatory marker (Bigagli et al., 2017). Recent studies revealed that sulforaphane, an isothiocyanate derived from cruciferous vegetables, protects against CVD due to its antioxidant and anti-inflammatory effects mediated through the Nrf2 signaling pathway (Bai et al., 2015). These evidences suggested that natural products may serve as a promising therapeutic approach for the prevention and treatment of CVD associated with oxidative stress. This review will discuss on the current knowledge on the molecular mechanisms of cardioprotective bioactive compounds targeting the Nrf2/ARE signaling pathway (Figure 1).
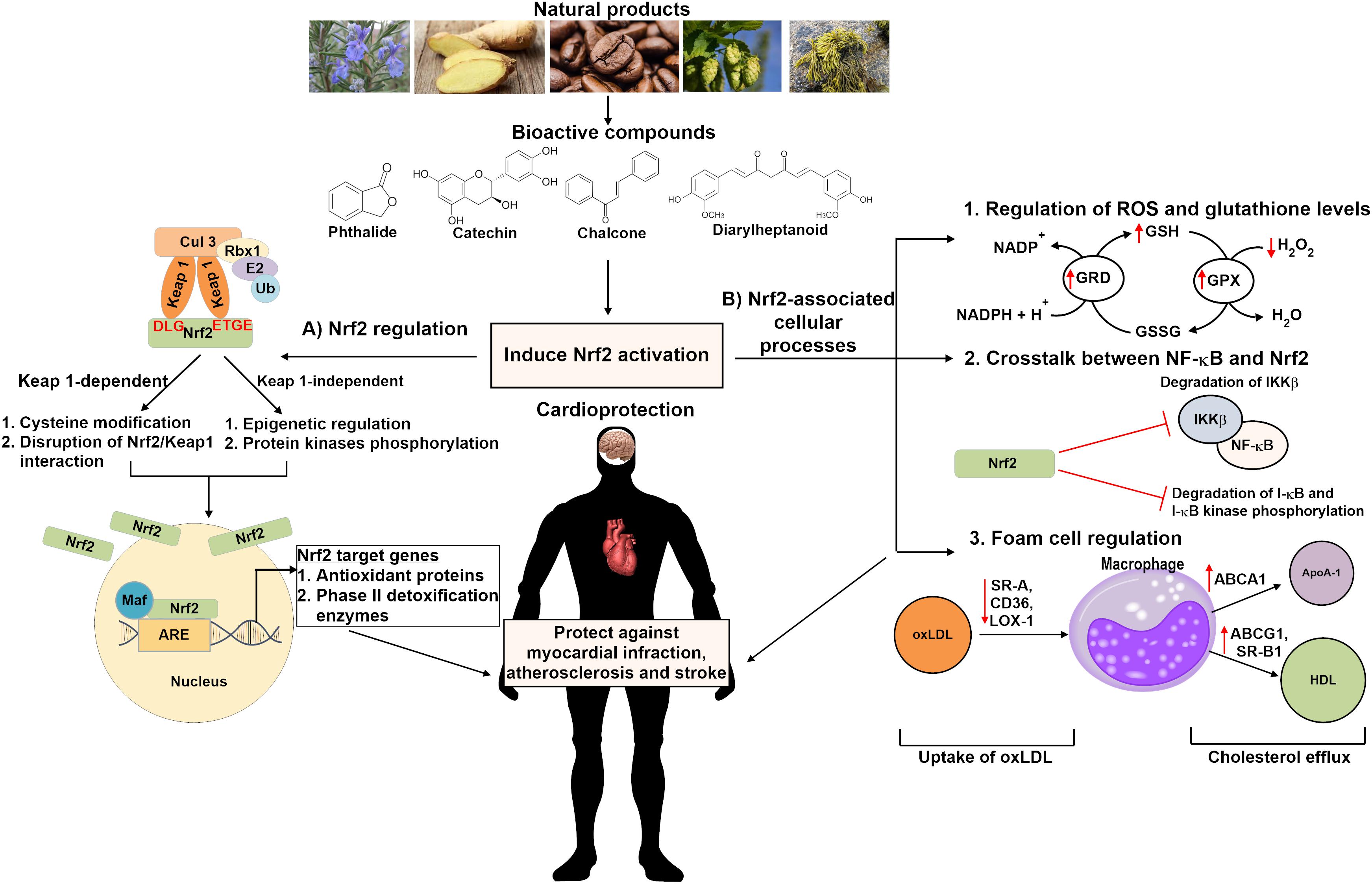
FIGURE 1. Schematic overview of the cardioprotective mechanisms of bioactive compounds derived from natural products in activating Nrf2 signaling pathway. Natural compounds may induce Nrf2 activation via the (A) Keap1-dependent or Keap1-independent pathway which involves cysteine modification, disruption of Nrf2/Keap1 interaction, epigenetic regulation and/or protein kinases phosphorylation. Nrf2 activation might also be induced through (B) Nrf2-associated cellular processes, including regulation of ROS and glutathione levels, inhibiting NF-κB inflammatory signaling pathway as well as controlling oxLDL uptake and cholesterol efflux in foam cells regulation. These bioactive compounds protect against cardiovascular diseases such as stroke, atherosclerosis and myocardial infraction. Nrf2 indicates nuclear factor erythroid 2-related factor 2; Keap 1, Kelch-like ECH-associated protein 1; Ub, ubiquitin; Maf, musculoaponeurotic fibrosarcoma; ARE, antioxidant response element; ROS, reactive oxygen species; GRD, glutathione reductase; GPX, glutathione peroxidase; GSH, glutathione; GSSG, glutathione disulfide; NF-κB, nuclear factor kappa-B kinase; IKK-β, inhibitor of nuclear factor kappa-B kinase subunit beta; oxLDL, oxidized low density lipoprotein; SR-A, scavenger receptor class A; CD36, scavenger receptor class B; LOX-1, lectin-type oxidized LDL receptor 1; ABC, ATP-binding cassette transporter; SR-B1, scavenger receptor class B type 1; ApoA-1, apolipoprotein A-1; HDL, high density lipoprotein.
Regulation of Nrf2 Signaling Pathway
Structural Features of Nrf2
Human Nrf2 (NFE2L2) protein comprises of 605 amino acid residues with molecular weight of 67.7 kDa (Cho, 2013). The Nrf2 gene consists of seven functional domains, also known as the Nrf2-ECH homology (Neh) domains (Namani et al., 2014; Canning et al., 2015). The position of each functional domain of Nrf2 is illustrated in Figure 2A. Neh 1 domain comprises of highly conserved basic region-leucine zipper (CNC-bZIP) region that dimerizes with small musculoaponeurotic fibrosarcoma (Maf) proteins and binds to ARE, a cis-acting enhancer sequence found in the promoter region of many genes encoding antioxidant and phase II detoxification enzymes or proteins. Neh 2 domain acts as a negative regulatory domain as it contains the two degrons, known as high-affinity ETGE motif and the lower-affinity DLG motif. These motifs specifically interact with Kelch-like ECH-associated protein 1 (Keap1) which mediate ubiquitination and degradation of Nrf2. The carboxy-terminal of Neh 3 domain is a transactivation domain that recruits the chromo-ATPase/helicase DNA-binding protein 6 (CHD 6) and drives ARE- gene expression. Both Neh 4 and Neh 5 also function as transactivation domains which are involved in the interaction with cAMP response element-binding protein (CREB)-binding protein (CBP) and receptor-associated coactivator 3 (RAC 3). Meanwhile, Neh 6 domain negatively regulates Nrf2 stability via glycogen synthase kinase-3 (GSK-3)/β-transducin repeat-containing protein (β-TrCP)-mediated degradation. It contains two highly conserved redox-independent degrons known as DSGIS and DSAPGS motifs. DSAPGS motif interacts with β-TrCP, which serves as a substrate receptor for the S-phase kinase-associated protein 1- Cullin 1- RING box protein-1/regulator of cullins-1 (Skp1–Cul1–Rbx1/Roc1) ubiquitin ligase complex. This results in ubiquitination and degradation of Nrf2 via the Keap1-independent pathway. Besides, suppression of Nrf2/ARE signaling pathway may be mediated via interacting with Neh 7 domain and the DNA-binding domain of retinoid X receptor α (RXRα).
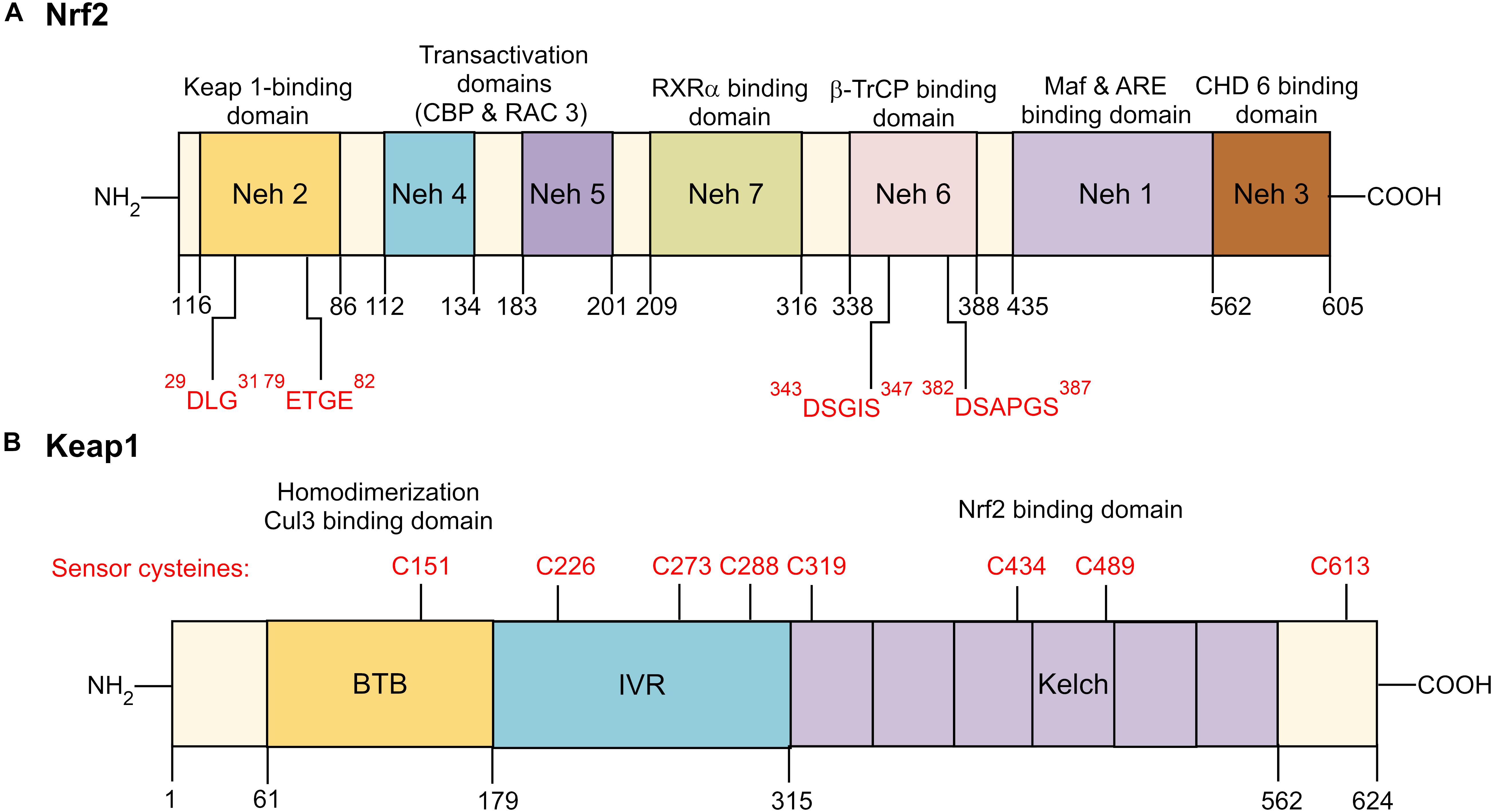
FIGURE 2. Domain structure of Nrf2 and Keap1. (A) Functional Nrf2-ECH homology (Neh) domains: Neh 1 is the binding site for small Maf proteins and ARE. Neh 2 serves as the binding site for Keap1 by interacting with low-affinity DLG and the high affinity ETGE motifs. Neh 3-5 are transactivation domains for Nrf2. Neh 6 is a serine-rich domain that negatively controls the Nrf2 stability by β-TrCP interacts with DSGIS and DSAPGS motifs. Neh 7 interacts with RXRα, a nuclear receptor responsible for suppression of Nrf2/ARE signaling pathway. (B) Functional Keap1 domains: N-terminal region, BTB dimerization domain, cysteine-rich IVR domain, six Kelch/DRG domain, and C-terminal region. BTB is responsible for Keap1 homodimerization and association with cullin (Cul3)-containing E3 ubiquitin ligase complex. IVR consists of reactive cysteine residues, including C226, C273 and C288. DRG domain is responsible for Nrf2 binding to DLG and ETGE motifs. Nrf2 indicates nuclear factor erythroid 2-related factor 2; Keap 1, Kelch-like ECH-associated protein 1; RXRα, retinoid X receptor α; β-TrCP, β-transducin repeat-containing protein; Maf, musculoaponeurotic fibrosarcoma; ARE, antioxidant response element; CHD 6, chromo-ATPase/helicase DNA-binding protein 6; BTB, Broad-Complex, Tramtrack, and Bric-a-Brac; IVR, intervening region; DRG, double glycine repeats.
Regulation of Nrf2 Activity
Keap1-Dependent Regulation of Nrf2 Activity
Keap1 is a cysteine rich adaptor protein for cullin (Cul3)-containing E3 ubiquitin ligase complex which mediates Nrf2 ubiquitination and degradation by 26S proteasomes. It consists of five sub-sections (Figure 2B), namely the N-terminal region, BTB dimerization domain (Broad-Complex, Tramtrack, and Bric-a-Brac), cysteine-rich intervening (IVR) domain, six Kelch/double glycine repeats (DRG) domain, and C-terminal region (Namani et al., 2014; Basak et al., 2017). Under normal homeostatic condition, Nrf2 has a short half-life of approximately 20 min. They are maintained at low level and constantly targeted for proteasomal degradation (Kobayashi and Yamamoto, 2006). The Keap1-dependent Nrf2 regulatory pathway supports the notion that exposure to ROS or Nrf2 inducers such as epigallocatechin-3-gallate (EGCG), sulforaphane, dimethyl fumarate (DMF), and tert-butylhydroquinone (tBHQ) will result in conformational changes in Keap1 cysteine residues, which interferes the interaction between Kelch domain and DLG motif where the ETGE motif still bound to Nrf2. Consequently, Keap1 fails to align with the E2 ubiquitin-conjugating enzyme and thus Nrf2 are no longer targeted for ubiquitination and degradation. The accumulation of free cytosolic Nrf2 is translocated into the nucleus where it dimerizes with Maf protein and binds to ARE sequences, resulting in the expression of downstream target genes (Figure 3) (Basak et al., 2017; Matzinger et al., 2017; Ooi et al., 2017).
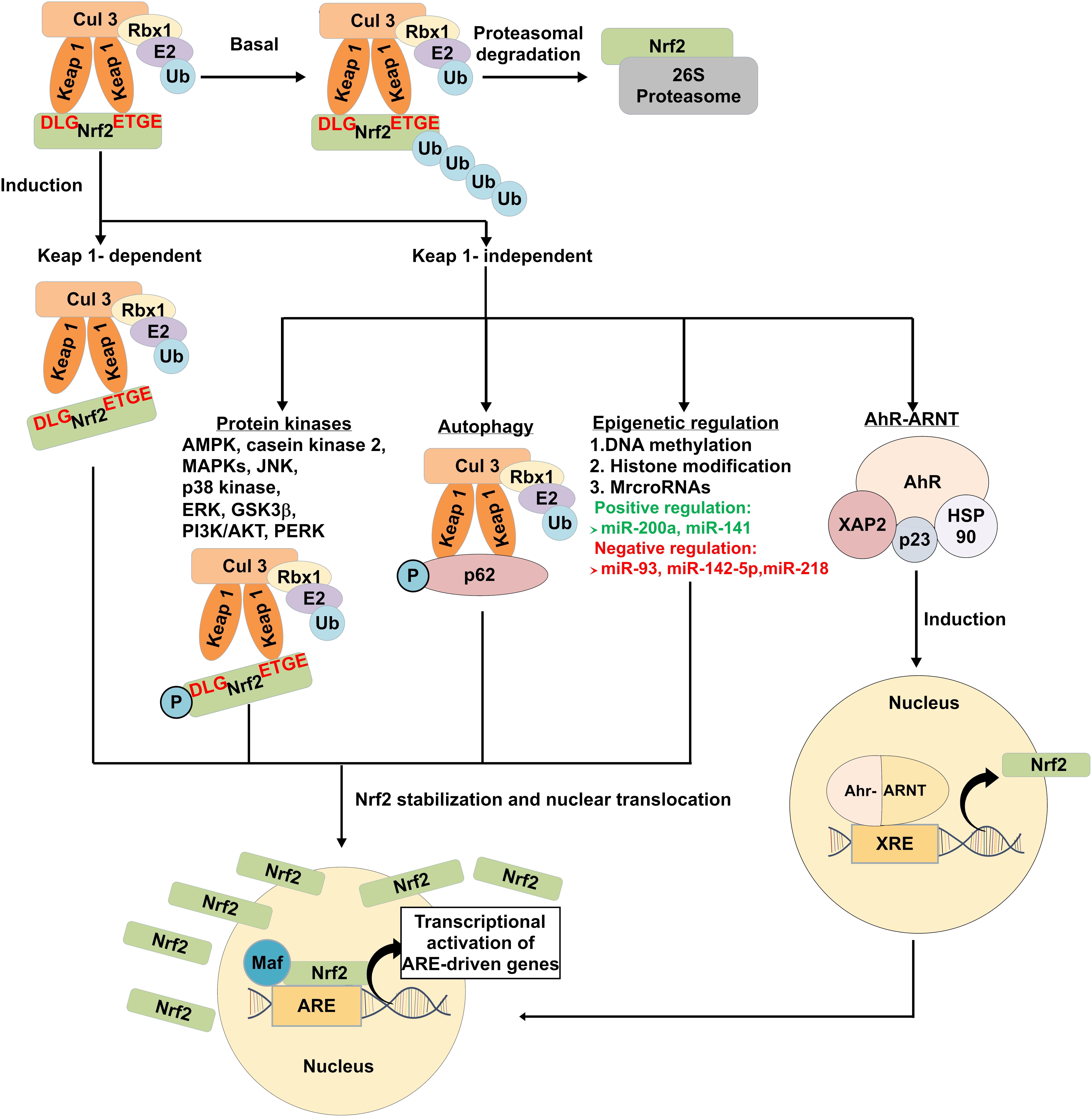
FIGURE 3. Modulation of Keap1-dependent and Keap1-independent mediated Nrf2 signaling pathways. Under basal condition, Nrf2 is constantly targeted by Keap1 for proteasomal degradation. Nrf2 may be induced by Keap1-dependent and Keap1-independent pathways. Under the Keap1-dependent pathway, exposure to oxidative stress and Nrf2 activators induce conformational change in the cysteine residues of Keap1, which disrupts the interaction between Kelch domain and DLG motif bound to Nrf2. This results in the release of Nrf2 from Keap1. Phosphorylation of protein kinases and autophagy adaptor proteins, epigenetic regulation and AhR-ARNT binding to XRE are associated with the release of Nrf2 in Keap1-independent-mediated pathways. Stabilized free cytosolic Nrf2 translocate into the nucleus, binds to ARE and induce transcription of antioxidant and detoxifying enzymes and proteins. Ub indicates ubiquitin; AMPK, AMP-activated protein kinase; MAPK, mitogen-activated protein kinase cascades; JNK, c-Jun N-terminal kinase; ERK, extracellular-signal-regulated kinase; GSK3β, glycogen synthase kinase-3β; PI3K/AKT, phosphatidylinositol-3-kinase; PERK, pancreatic endoplasmic reticulum kinase; p62, sequestosome-1; AhR-ARNT, aryl hydrocarbon receptor-aryl hydrocarbon receptor nuclear translocator; HSP90, heat shock protein 90; XAP2, X-associated protein 2; p23, HSP90 co-chaperone; ARE, antioxidant response element, and; XRE, xenobiotic response element.
Keap1-Independent Regulation of Nrf2 Activity
Apart from Keap1-dependent mechanism, emerging bodies of evidences revealed that Nrf2 can also be regulated through a number of mechanisms independent of Keap1. These mechanisms include transcriptional and epigenetic regulation, autophagy and other signaling pathways (Figure 3).
Transcriptional regulation of Nrf2
Binding of Aryl hydrocarbon receptor-aryl hydrocarbon receptor nuclear translocator (AhR-ARNT) to xenobiotic response element (XRE) sequences is known to regulate Nrf2 activation. AhR is a member of the basic helix-loop-helix Per-ARNT-Sim (bHLH-PAS) family of transcription factors that functions as xenobiotic chemical sensor in eukaryotes (Furue et al., 2017; Nebert, 2017). The inactive form of AhR is stabilized in the cytoplasm in a complex form with heat shock protein 90 (HSP90), X-associated protein 2 (XAP2), and HSP90 co-chaperone p23 (Mimura and Fujii-Kuriyama, 2003; Quintana, 2013; Furue et al., 2017). Upon exposure to polycyclic aromatic hydrocarbon, AhR ligand complex translocate into the nucleus where it dissociates from HSP90 complex and dimerizes with ARNT and binds to XRE sequences at the promoter region and upregulates the expression of phase I and II metabolic enzymes, cytochrome P450 family members, NQO1, Ya subunit of glutathione S-transferase (GST), δ-aminolevulinic acid synthase, UDP-glucuronosyltransferase and others (Beischlag et al., 2008; Furue et al., 2017). There are studies reporting the cross-talk between AhR and Nrf2 signaling pathway (Miao et al., 2005; Korashy and El-Kadi, 2006; Tsuji et al., 2012; Dietrich, 2016). It was shown that Nrf2 promoter contains three XRE-like elements (XREL) located at position -712 (XREL1), +755 (XREL2) and +850 (XREL3). The activity of 2, 3, 7, 8-tetrachlorodibenzo-p-dioxin (TCDD)-induced Nrf2 mRNA was abolished in transient AhR-silenced cell line tao (Miao et al., 2005). Besides, activation of AhR nuclear translocation by ketoconazole (KCZ) has shown to upregulate cytochrome P450 family 1 Subfamily A Member 1 (CYP1A1) expression. Meanwhile, it also induced the Nrf2 nuclear translocation, resulting in upregulation of NQO1 expression (Tsuji et al., 2012). These evidences suggest that activation of Nrf2 can be regulated by AhR-ARNT pathway.
Epigenetic regulation of Nrf2
Epigenetics modifications including DNA methylation, histone modification, and microRNAs (miRNAs) expressions are involved in Nrf2 regulation (Guo et al., 2015). For instance, the expression of Nrf2 and its downstream gene NQO1 were lower in transgenic adenocarcinoma of mouse prostate (TRAMP) C1 cells (Yu et al., 2010; Zhang et al., 2013). Treatment with DNA methyltransferases (DNMTs) inhibitor 5-aza-2′-deoxycytidine (5-Aza) and histone deacetylase (HDAC) inhibitor trichostatin A (TSA) has shown to restore the epigenetically silenced Nrf2 gene and increase NQO1 expression, which helps to prevent prostate cancer progression in TRAMP mice and protects against Alzheimer’s development in a mouse neuroblastoma N2a cellular model (Yu et al., 2010; Cao et al., 2016). In addition, TSA also increased Nrf2-regulated HO-1, NQO1, glutamate-cysteine ligase catalytic (GCLC) in neuron cultures and brain tissue by promoting Nrf2 dissociation from Keap1 and nuclear Nrf2 translocation (Wang et al., 2012).
MiRNAs are short, single-stranded, small non-coding RNA molecules of approximately 18–25 nucleotides long. It also has been implicated in regulation of Nrf2 at the post-transcriptional level (Lujambio and Lowe, 2012). Recent study showed that increased miR-200a expression leads to Keap1 degradation and Nrf2 protein stabilization, thereby protecting OB-6 osteoblastic cells from dexamethasone-induced oxidative stress and apoptosis (Zhao et al., 2017). A similar relationship was observed between miR-141 and Nrf2/Keap1 pathway in hepatocellular carcinoma cells. Increased levels of miR-141 expression in hepatocellular carcinoma cells such as HepG2, SMMC-7721, and HuH7 cell lines has shown to downregulate Keap1 expression via the Keap1 3′ untranslated region (3′ UTR), resulting in transcriptional activation of Nrf2-dependent HO-1 gene (Shi et al., 2015). Interestingly, an inverse correlation between miRNAs and Nrf2 has also been reported. Downregulation of miRNAs such as miR-93, miR-142-5p and miR-218 exhibited protective effects against cerebral ischemic injury and high glucose (HG)-induced apoptosis through upregulation of Nrf2/ARE signaling pathway (Wang et al., 2016, 2017; Liu et al., 2017).
Autophagy
Autophagy, a bulk-lysosomal degradation process that is responsible for the clearance of aggresomes and abnormal organelles, can enhance cell survival under stress condition. The functional role of autophagy adaptor proteins, also known as sequestosome-1 (p62/SQSTM1) in regulating Nrf2 and its downstream target genes has been elucidated (Bryan et al., 2013; Kapuy et al., 2018). Under oxidative stress condition, p62 is phosphorylated, which increases its binding affinity to Keap1. The binding of p62 to Keap1 results in the dissociation of Nrf2 from Keap1, thereby promoting Nrf2 stabilization and subsequent activation of downstream target genes. This is indicated in previous research showing that knockdown of p62 significantly promoted the accumulation of Keap1, thus enhanced Nrf2 degradation (Sun et al., 2016). In addition, depletion of SQSTM1 significantly doubled the half-life of Keap1 and lead to simultaneous decrease in Nrf2 protein and mRNA levels (Copple et al., 2010). Mammalian target of rapamycin complex 1 (mTORC1)-induced S351 phosphorylation in the Keap1-interacting region (KIR) motif of p62 markedly increase binding affinity of p62 for Keap1, thereby increasing the transcriptional activation of Nrf2 target genes (Ichimura et al., 2013). This suggests that p62 can compete with Nrf2 for binding to Keap1 via KIR motif, which has a sequence similar to the ETGE motif in Nrf2 (Jain et al., 2010). Apart from mTOR kinase-induced p62 phosphorylation, several other kinases such as class III phosphoinositide 3-kinase (PI3K) vacuolar protein sorting 34 (VPS34) (Jiang et al., 2017) and TGF-β-activated kinase 1 (TAK1) (Hashimoto et al., 2016) have also been demonstrated to phosphorylate p62 and facilitate the Keap1-p62 complex interaction, thus increasing Nrf2 expression levels. Overall, these studies revealed that p62/SQSTM1 creates a positive feedback loop for enhancing Nrf2 expression.
Other signaling pathways
Several protein kinases including AMP-activated protein kinase (AMPK), casein kinase 2, mitogen-activated protein kinase cascades (MAPKs): JUN-N-terminal kinase (JNK), p38 kinase, extracellular-signal-regulated kinase (ERK), GSK3β, phosphatidylinositol 3-kinase (PI3K/AKT) and pancreatic endoplasmic reticulum kinase (PERK) have been implicated in Nrf2/Keap1 interaction. Phosphorylation of the serine (Ser), threonine (Thr), and tyrosine (Tyr) residues could lead to enhanced Nrf2 stability, nuclear accumulation, and subsequent transactivation activity. Phosphorylation at Thr 172 and Ser 550 by AMPK (Zimmermann et al., 2015; Joo et al., 2016) and at Ser40 by casein kinase 2 (Apopa et al., 2008) could induce Nrf2 accumulation for ARE-driven gene transactivation. Furthermore, studies have shown that MAPKs signaling pathways have a role in the Nrf2 regulation. Recent research reported that Andrographolide, a labdane diterpenoid exerts a potential therapeutic effect against neuroinflammatory diseases through upregulation of Nrf2/HO-1 expression in astrocytes via p38 MAPK and ERK-dependent pathways (Wong et al., 2016). Similarly, it was shown that activation of p38 MAPK/Nrf2 pathway is required to induce the expression of HO-1 induction by fungal β-glucan-containing particles (β-GPs) (Ishida et al., 2018). Stimulation of p38 MAPK by anisomycin was found to phosphorylate Nrf2 protein, which promotes the interaction of Nrf2 with Keap1, thereby inhibiting nuclear translocation of Nrf2 (Keum et al., 2006). In addition, GSK3β has been reported to negatively regulate Nrf2 activity. Inhibition of GSK-3β can increase the nuclear accumulation of Nrf2 and antioxidant response in hepatocytes as well as rat with cerebral ischemia-reperfusion (Jiang Y. et al., 2015; Chen et al., 2016). Moreover, PI3K/AKT and PERK also have been reported positively regulate Nrf2 activation (Cullinan and Diehl, 2004; Zou et al., 2013).
Role of Nrf2 in Cardiovascular Diseases
A growing body of evidence showed that Nrf2 and its downstream target genes protect against CVD development, including oxidative stress-induced endothelial dysfunction and atherosclerosis. Endothelial dysfunction marks the early stages of atherosclerosis where oxidative stress enhances endothelium cell permeability, LDL oxidation, monocyte adherence, platelet activation, vascular inflammation, as well as proliferation and infiltration of vascular smooth muscle cells (VSMCs) from media to arterial intima (Hadi et al., 2005; Grover-Páez and Zavalza-Gómez, 2009). Transplantation of Nrf2-deficient bone marrow cells in LDLR-/- mice model showed a reduction in the expression levels of antioxidant enzymes [NAD(P)H dehydrogenase, NQO1, catalase and GPX1], increased macrophage migration, production of pro-inflammatory cytokines, and atherosclerotic lesions (Collins et al., 2012). Meanwhile, silencing of Nrf2 in U937 monocytic cells led to an elevation of pro-inflammatory cytokines including interleukin-1β (IL-1β), interleukin-6 (IL-6), tumor necrosis factor alpha (TNF-α), monocyte chemotactic protein-1 (MCP-1), and endoplasmic reticulum (ER) stress markers expression (Song et al., 2015). Further evidence showed that overexpression of Nrf2 in VSMCs of rabbit model showed increased expression of antioxidant enzymes (HO-1 and NQO1) and inhibition of VSMCs proliferation and vascular inflammation (Levonen et al., 2007). In addition, Nrf2 deficiency in macrophage promoted pro-inflammatory cytokines production (MCP-1, IL-6, and TNF-α) and enhanced oxidized low density lipoprotein (oxLDL) uptake, leading to foam cell formation (Ruotsalainen et al., 2013).
Interestingly, some studies reported that Nrf2-mediates pro-atherogenic effects (Sussan et al., 2008; Barajas et al., 2011; Freigang et al., 2011; Harada et al., 2012; Ruotsalainen et al., 2018). Nrf2 deficiency in ApoE-/- mice developed smaller atherosclerotic plaques by reducing CD36 expression, a type of scavenger receptor which is responsible for taking up modified LDLs (Sussan et al., 2008). Moreover, a reduction in pro-inflammatory cytokine IL-1-mediated vascular inflammation was observed in Nrf2-deficient ApoE-/- mice (Freigang et al., 2011). Recent studies have shown that deficiency of Nrf2 in LDLR-/- and LDLR-/- mice expressing apoB-100 only (LDLR-/-ApoB100/100) reduced atherosclerotic lesion development. However, Nrf2 deficiency in aged LDLR-/-ApoB100/100 mice exhibit enhanced plaque inflammation and calcification (Ruotsalainen et al., 2018). These evidences suggest that Nrf2 activation plays a dual role in CVD.
Protective Effects of Natural Products Against Cardiovascular Diseases
Natural products derived from plants, marine organisms and animals have been a reliable source of new structural leads for treatment of various diseases. Most bioactive compounds are produced as secondary metabolites, which can be classified as phenolics, flavonoids, chalcones, terpenoids, carotenoids, anthocyanins, quinones, and others. These bioactive compounds possess a wide range of biological activities including anti-tumor, anti-inflammatory, anti-carcinogenic, anti-viral, anti-microbial, anti-diarrheal, anti-oxidant, and other activities (Manivasagan et al., 2014; Hamed et al., 2015; Wang et al., 2018). Bioactive compounds have been shown to reduce atherosclerosis formation and risk of developing CVD (Rangel-Huerta et al., 2015; Dal and Sigrist, 2016). Recently, the PREvencion con DIeta MEDiterannea (PREDIMED) trial reported that dietary polyphenols intake such as extra-virgin olive oil and nuts were associated with improved CVD risk factors and decreased inflammatory biomarkers levels in high CVD risk participants. It was shown that polyphenol intake decreased blood pressure (BP), increased plasma high density lipoprotein (HDL) and decreased the inflammatory biomarkers of CVD, including vascular cell adhesion molecule 1 (VCAM-1), intercellular adhesion molecule 1 (ICAM-1), IL-6, TNF-α as well as MCP-1 (Medina-Remón et al., 2017). Similarly, Health, Alcohol and Psychosocial factors In Eastern Europe (HAPIEE) study also reported that dietary polyphenols (phenolic acids and stilbenes) intake were found to be inversely correlated to metabolic syndrome (MetS) which is closely link to risk factors of CVD including glucose intolerance, dyslipidemia, high BP and abdominal obesity (Grosso et al., 2017). Apart from dietary supplement human trials, accumulating evidence from both in vivo and in vitro studies are also supporting the cardioprotective effects of natural products. For instance, ApoE-/- mice fed with high cholesterol diet supplemented with ellagic acid (EA) exerts an atheroprotective effect by improving the antioxidant capacity, attenuated hypochlorous acid (HOCl)-induced endothelial dysfunction and increased the expression of HO-1 and Nrf2 (Ding et al., 2014). Treatment with quercetin ameliorated the high fat diet-induced MetS such as abdominal obesity, cardiovascular remodeling and liver complications in rats by increasing the expression of Nrf2, HO-1, carnitine palmitoyltransferase 1 (CPT1) and decreasing NF-κB (Panchal et al., 2012). Furthermore, cucurmin, a natural diarylheptanoids can prevent copper sulfate-induced LDL peroxidation, which is the earliest stage of atherosclerotic plaque that contributes to CVD (Mahfouz et al., 2009). Maslinic acid, a natural triterpenoid has also been shown to protect VSMCs against oxidative stress through activation of Akt/Nrf2/HO-1 pathway (Qin et al., 2014). Treatment with Tanshindiol C, a quinone derivative, attenuated oxLDL-induced macrophage foam cell formation by upregulating antioxidant peroxiredoxin 1 (Prdx1) and ATP-binding cassette transporter A1 (ABCA1) via Nrf2/Sirtuin 1 (Sirt1) signaling pathway (Yang et al., 2018b).
Molecular Mechanisms of Cardioprotective Natural Products Targeting the Nrf2 Signaling Pathway
The cardioprotective role of natural products targeting Nrf2 signaling pathway has been widely investigated. There are multiple mechanisms that are involved in activating Nrf2, including interaction with cysteine residues on Keap1, disruption of Nrf2/Keap1 interaction, epigenetic modification and activation of protein kinases. The molecular mechanisms of natural products targeting Nrf2 signaling pathway will be discussed and summarized as schematically outlined in Table 1.
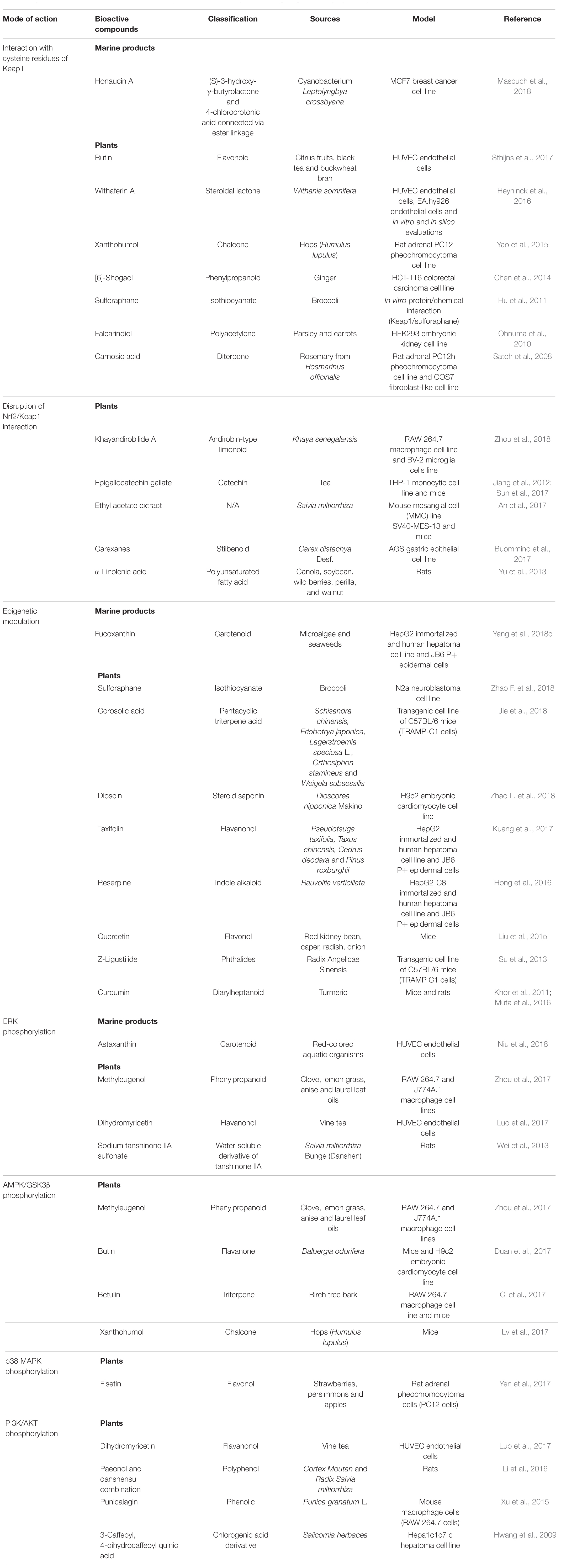
TABLE 1. Molecular mechanisms of bioactive compounds from natural products targeting Nrf2/Keap1 pathway.
Interaction With Keap1 Cysteine Residues
Keap1 is a cysteine rich adaptor protein. Human Keap1 have a total of 27 cysteine residues which can be modified by oxidants and electrophiles. Among the cysteine residues in human Keap1, Cys 151, 273 and 288 are highly reactive and play an essential role for repression of Nrf2/ARE activation (Saito et al., 2016). Studies suggest that Keap1 cysteine residues modification by bioactive compounds derived from natural products are involved in activating Nrf2 antioxidant defense system. These bioactive compounds are reported to modify Keap1 cysteine residues via oxidation, alkylation or thiol disulfide interchange. A recent report investigating the effects of rutin, a flavonoid abundantly present in citrus fruit demonstrated that it protects against H2O2-induced oxidative stress in human umbilical vein endothelial cells (HUVECs). The study showed that rutin can target the Cys 151 of Keap1, and form an adduct with Keap1 which results in Nrf2 activation and upregulation of glutamate cysteine ligase, a glutathione biosynthesis rate-limiting enzyme which plays an important role in the endogenous antioxidant system (Sthijns et al., 2017). Honaucin A, an anti-inflammatory compound isolated from marine filamentous cyanobacterium Leptolyngbya crossbyana has been reported to induce alkylation of Keap1 cysteine thiols and thereby activating Nrf2/ARE pathway (Mascuch et al., 2018). Similarly, treatment with withaferin A, a steroidal lactone significantly increased expression of HO-1 in HUVECs and EA.hy926 endothelial cells by enhancing nuclear translocation of Nrf2. Analysis of the in vitro and in silico model suggested that withaferin A can interact with Cys 151, Cys 319, Cys 434, Cys 489, and Cys 613 (Heyninck et al., 2016). Apart from that, a number of bioactive compounds including xanthohumol, sulforaphane, falcarindiol, carnosic acid and (6)-shogaol have also been showed to interact with cysteine residues on Keap1, thereby stimulating its dissociation from Nrf2 and promoting Nrf2 nuclear accumulation which induces antioxidant proteins and phase II detoxification enzymes (Satoh et al., 2008; Ohnuma et al., 2010; Hu et al., 2011; Chen et al., 2014; Yao et al., 2015). The activation of Nrf2 and stimulation of downstream antioxidants and phase II detoxification enzymes expression suggest that these natural bioactive compounds represent a potential therapeutic source for prevention and treatment of CVDs.
Disruption of Nrf2/Keap1 Interaction
Apart from modification of Keap1 cysteine residues, studies have demonstrated that bioactive compounds from natural products can disrupt the Nrf2/Keap1 interaction, thereby promoting Nrf2 nuclear translocation. For instance, EGCG is a well-known Nrf2 activator that promotes the dissociation of Nrf2/Keap1 and activate ARE genes transcription, thereby inhibiting TNF-α-induced NF-κB activation in human THP-1 cells (Jiang et al., 2012). Similar study also found that ECGC protects mice model against diabetic nephropathy through inhibiting the function of Keap1 by forming hydrogen bonds with specific residues such as Ser 508, Ser 555, Ser 602, Tyr 525, Tyr 572, Gln 530, and Arg 483 (Sun et al., 2017). Besides, α-linolenic acid (ALA) has been found to protect against DOX-induced cardiotoxicity by exerting anti-oxidative and anti-apoptosis properties in rat model. The underlying mechanism is associated with the enhancement of antioxidant defense system through Nrf2/Keap1 pathway by promoting the degradation of Keap1 and thus facilitating nuclear translocation of Nrf2, as well as activation of AKT/ERK pathway (Yu et al., 2013). Similarly, khayandirobilide A (KLA) also exhibits anti-inflammatory effect via elevated expression of HO-1 by inducing Keap1 autophagic degradation and thus facilitating Nrf2 nuclear translocation (Zhou et al., 2018).
Epigenetic Modification
Epigenetic mechanisms have been reported to be associated with the pathogenesis of CVD. Epigenomics study in atherosclerotic human aorta demonstrated a genome-wide increase in DNA methylation during the onset and progression of atherosclerosis (Zaina et al., 2014). Studies have demonstrated that DNA demethylation and histone (de)acetylation can trigger or increase Nrf2 expression. Natural compounds derived from plants (such as sulforaphane, corosolic acid, taxifolin, reserpine, quercetin, Z-ligustilide and curcumin) and marine constituents (fucoxanthin) have been shown to activate Nrf2 signaling through epigenetic regulation (Khor et al., 2011; Su et al., 2013; Liu et al., 2015; Hong et al., 2016; Muta et al., 2016; Kuang et al., 2017; Jie et al., 2018; Yang et al., 2018c; Zhao F. et al., 2018). Quercetin, a natural flavonoid, significantly inhibited nickel-induced inflammation in mouse liver by decreasing Nrf2 DNA methylation and inhibiting the p38 MAPK signaling pathway (Liu et al., 2015). In addition, some natural compounds are involved in regulating miRNAs expression, which in turn activate Nrf2 pathway. For instance, treatment with dioscin, a natural steroid saponin markedly decreased the expression level of miRNA-140-5p, and subsequently activates Nrf2 and silent information regulator factor 2-related enzyme 2 (Sirt2) in cardiac H9c2 cells, thereby upregulating downstream target genes such as HO-1, NQO1, GST, GCLM, and forkhead box O3 (FOXO3a) (Zhao L. et al., 2018). However, the mechanism underlying epigenetic pathway responsible for the cardioprotective effects of natural product remains to be elucidated.
Protein Kinases Modulation
Protein kinases such as ERK, AMPK, GSK3β, p38 MAPK, and PI3K/AKT can mediate Nrf2 phosphorylation which enhance Nrf2 stability, thereby promoting nuclear Nrf2 translocation and transactivation activity (Nguyen et al., 2003; Bryan et al., 2013). Several natural compounds, including sodium tanshinone IIA sulfonate (STS), dihydromyricetin (DMY), methyleugenol (MLG), and astaxanthin have been shown to phosphorylate ERK, and upregulate Nrf2 expression (Wei et al., 2013; Luo et al., 2017; Zhou et al., 2017; Niu et al., 2018). STS, a water-soluble derivative of tanshione IIA, was found to protect against isoproterenol (ISO)-induced myocardial infarction (MI) in rat model. Pre-treatment with STS has dramatically increased ERK phosphorylation, and subsequently enhanced the expressions of Nrf2 and HO-1 (Wei et al., 2013). DMY has also been shown to ameliorate oxLDL-induced oxidative injury in HUVECs through activation of Akt/ERK/Nrf2/HO-1 (Luo et al., 2017). Similarly, MLG protects against t-BHP-triggered cytotoxicity and attenuated ROS generation by inducing antioxidant enzymes expression and ERK phosphorylation in RAW 264.7 and J774A.1 murine macrophage cell lines. Interestingly, MLG has also shown to phosphorylate AMPK and GSK3β, which leads to upregulation of Nrf2 and its downstream target genes (Zhou et al., 2017). Besides targeting ERK pathway, other cardioprotective natural products such as butin, xanthohumol, botulin, fisetin, paeonol and danshensu combination (PDSS), punicalagin and 3-caffeoyl, 4-dihydrocaffeoyl quinic acid can activate Nrf2 through phosphorylation AMPK, GSK3, p38 MAPK, and PI3K/AKT.
The Cardioprotective Mechanisms of Natural Products Regulating Nrf2-Associated Cellular Processes in Cardiovascular Diseases
Apart from targeting the Nrf2/Keap1 interaction, some cardioprotective natural products were found to regulate Nrf2-associated cellular processes. The mechanisms of natural products in regulating Nrf2-associated cellular processes were summarized and tabulated in Table 2.
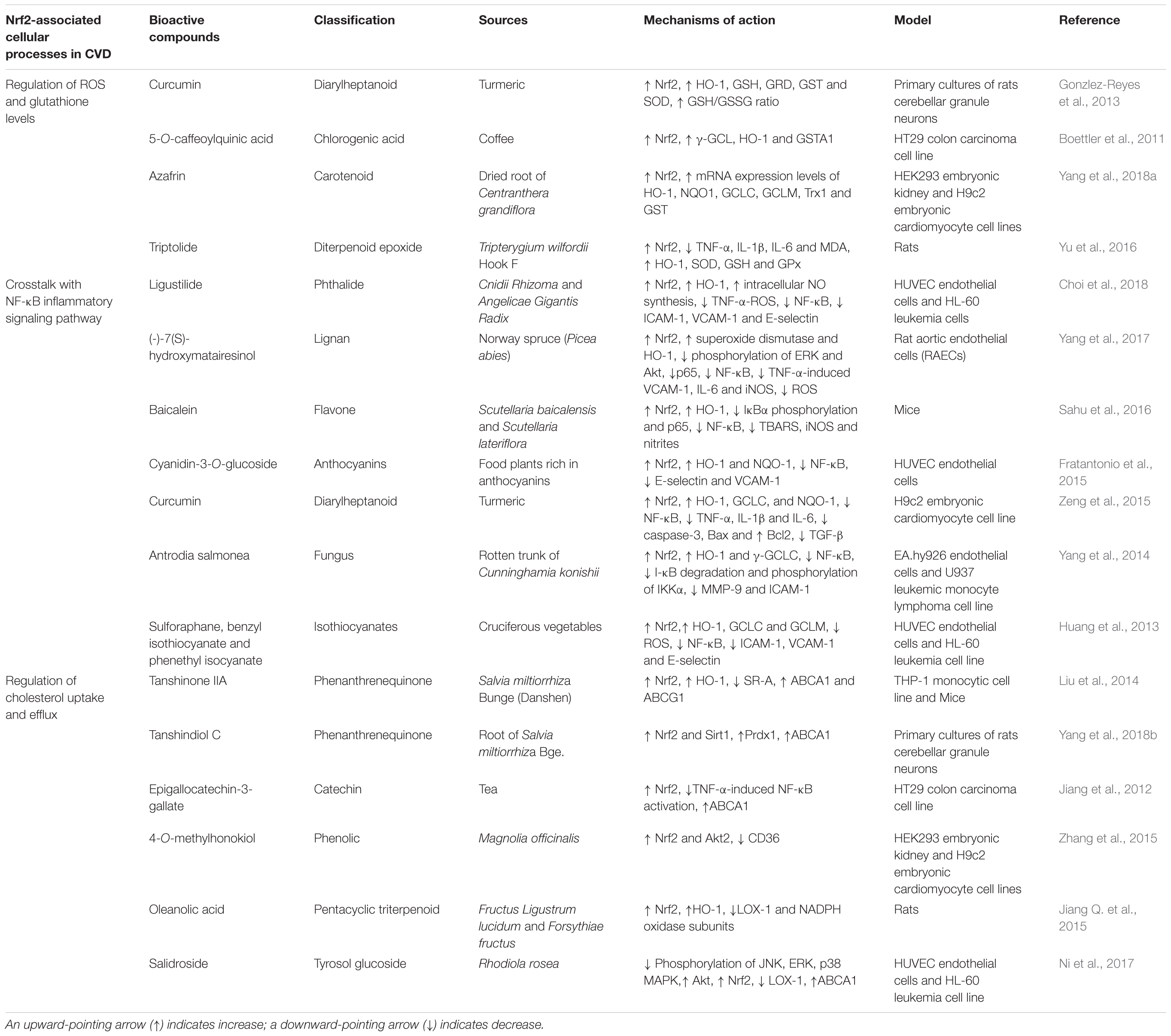
TABLE 2. Molecular mechanisms of bioactive compounds from natural products targeting Nrf2-associated cellular processes.
Regulation of ROS and Glutathione Levels
Glutathione (GSH) is a sulfhydryl group tripeptide composed of glutamate, cysteine and glycine. It plays an important role in cellular redox homeostasis. Several studies suggested that GSH can protect cells against oxidative stress due to its capability in interacting with antioxidant enzymes. Examples of GSH-linked defense enzymes include GSH peroxidase (GPX), GST, glutathione reductase (GRD), thioredoxins (Trx), glutaredoxin (GRX2), and PRX (Ribas et al., 2014; Ye et al., 2015). Glutathione is present mainly in reduced form and it is only oxidized into glutathione disulfide (GSSG) in the presence of oxidative stress. Thus, the ratio of GSH and GSSG within the cells is often used as the index for intracellular oxidative stress. Furthermore, clinical evidences have shown an association between GSH level and CVD. Low GSH level was observed in patients with the most severe cases of heart failure. Patients with cardiac diseases showed 21% depletion in blood GSH than healthy controls (Damy et al., 2009). Chronic depletion of myocardial GSH levels was reported in GCLM-deficient mice after transverse aortic constriction-induced pressure overload, and this condition leads to increased left ventricular dilation, myocardial fibrosis, and dysfunction (Watanabe et al., 2013).
Recent studies have reported the role of natural products at inducing glutathione-linked enzymes via Nrf2 signaling pathway. Azafrin, a natural carotenoid, have shown to induce Nrf2 downstream target genes such as HO-1, GCLC, GCLM, Trx1 and GST (Yang et al., 2018a). This is in consistent with another study showing that pre-treatment with triptolide, a diterpenoid epoxide protected Wistar rat from myocardial ischemia/reperfusion injuries by suppressing the production of pro-inflammatory cytokines (TNF-α, IL-1β, and IL-6) and inducing Nrf2-regulated antioxidant enzymes (SOD, GSH, GPx, and HO-1) (Yu et al., 2016). Besides, coffee constituent 5-O-caffeoylquinic acid (CGA) has also been shown to enhance Nrf2 nuclear translocation and increase the transcriptional expression of γ-GCL, HO-1 and GSTA1 (Boettler et al., 2011). Curcumin on the other hand has been found to attenuate hemin-induced ROS production and increase the ratio of GSH/GSSG in primary cultures of rat cerebellar granule neurons (CGNs). Furthermore, it also increased the cytoprotective enzymes such as HO-1, GR, GST, and SOD via inducing Nrf2 nuclear translocation (Gonzlez-Reyes et al., 2013).
Crosstalk With NF-κB Inflammatory Signaling Pathway
The transcription factor NF-κB plays a crucial role in regulating innate immunity and inflammatory responses, and it is also involved in the pathogenesis of atherosclerotic plaques formation (Maracle et al., 2018). Recent studies showed that there are potential crosstalk between NF-κB and Nrf2. Nrf2 deficient mice were found to have increased NF-κB activation, inflammatory cytokines TNF-α, IL-1β, and IL-6 production, and ICAM-1 expression in brain after traumatic brain injury compared to wild-type mice (Jin et al., 2008). Evidence suggests that Nrf2/Keap1 pathway can inactivate NF-κB activity through ubiquitin-mediated degradation of IKKβ (Lee et al., 2009). Besides, it has been reported that p65, a canonical NF-κB subunit antagonized the transcriptional activity of Nrf2 by depriving Nrf2 transcriptional co-activator CBP (Liu et al., 2008). These data suggest that there is a crosstalk between Nrf2 and NF-κB in regulating the transcription of its downstream target proteins.
Several natural compounds have been reported to protect against CVDs by targeting both Nrf2 and NF-κB signaling pathways. For instance, Ligustilide, a phthalide compound inhibited VCAM-1, ICAM-1 and E-selectin expression by suppressing the NF-κB activation as well as inducing Nrf2-mediated HO-1 expression in TNF-α-stimulated HUVECs (Choi et al., 2018). Similarly, bioactive compounds such as cyanidin-3-O-glucoside and isothiocyanates have been reported to counteract the pathogenesis of endothelial dysfunction, including upregulation of Nrf2-dependent antioxidant response elements (HO-1, GCLC and GCLM) and downregulation of adhesion molecules (ICAM-1, VCAM-1, and E-selectin) via inhibition of NF-κB activation (Huang et al., 2013; Fratantonio et al., 2015). Furthermore, Antrodia salmonea, a medicinal fungal species exerts an anti-angiogenic and anti-atherogenic activity in human vascular endothelial cell line (EA.hy 926) and human leukemic monocyte lymphoma cell line (U937). It significantly suppressed TNF-α-induced matrix metalloproteinase-9 (MMP-9) and ICAM-1 expression via suppressing I-κB degradation and I-κB kinase phosphorylation, as well as upregulating the expression of HO-1 and γ-GCLC through Nrf2 signaling pathway (Yang et al., 2014).
Regulation of Cholesterol Uptake and Efflux (Foam Cell Formation)
Macrophage foam cell formation represents the early hallmarks of atherosclerosis lesion formation. Foam cells formation is closely associated with abnormal cholesterol metabolism that results from imbalanced cholesterol uptake and efflux. Macrophages may take up modified LDL via scavenger receptors or through the pinocytosis process. Scavenger receptors such as scavenger receptors class A (SR-A), scavenger receptor class B (CD36), and lectin-type oxidized LDL receptor (LOX-1) have been implicated in the pathogenesis of atherosclerosis (Kunjathoor et al., 2002; Schaeffer et al., 2009). Several studies suggested that SR-A and CD36 exert pro-atherogenic properties due to their ability to interact with modified LDL, thereby contributing to the foam cell formation. Silencing SR-A or CD36 alone in LDLR-/- ApoB100 mice was shown to profoundly protect against atherosclerosis (Mäkinen et al., 2010). Besides, studies have shown that cholesterol efflux transporters such as scavenger receptor class B type 1 (SR-B1), ABCA1 and ABCG1 promote efflux of free cholesterol to apolipoproteinA-1 (apoA-1) and HDL. Deficiency of either one or both efflux transporters (ABCA1 or ABCG1) have shown to enhance lipopolysaccharide (LPS)-induced inflammatory gene expression, reduce aortic endothelial NO synthase (eNOS), increase monocyte adhesion and infiltration into atherosclerotic plaque (Westerterp et al., 2016). Similarly, double-knockout ABCA1 and ABCG1 mice administered with a high cholesterol diet exhibited extensive infiltration of macrophage foam cells in the myocardium and spleen and have shown accelerated progression in atherosclerosis (Yvan-Charvet et al., 2007; Westerterp et al., 2013).
The role of Nrf2 in the transcriptional regulation of these scavenger receptors and cholesterol efflux transporters has been established. Many recent studies have reported the role of natural compounds in modulating cholesterol uptake and efflux receptors by targeting the Nrf2 signaling pathway. Oleanolic acid (OA), a natural pentacyclic triterpenoid, exerts anti-atherosclerotic effect in quail models and HUVECs where it was shown to inhibit oxLDL-induced LOX-1 and NADPH oxidase subunits expression while increasing the expression of Nrf2 and HO-1 (Jiang Q. et al., 2015). Furthermore, salidroside protects against foam cells formation by upregulating ABCA1 and downregulation of LOX-1 via activation of MAPK/Akt/Nrf2 pathways (Ni et al., 2017). This is consistent with another study which showed that Tanshinone IIA (Tan) inhibit atherosclerotic plaque formation in ApoE-/- mice and it is suggested that Tan regulates cholesterol metabolism by reducing the expression of SR-A, while further enhancing ABCA1 and ABCG1 expression in human THP-1 cells via activation of ERK/Nrf2/HO-1 pathway (Liu et al., 2014). Other plant constituents (as shown in Table 2) such as Tanshindiol C, epigallocatechin-3-gallate, and 4-O-methylhonokiol (MH) can regulate SR-A and CD36 receptors via Nrf2 activation and its downstream effects.
Recent Insights of the Clinical Investigation of Nrf2-Activators
Nrf2 is a potential therapeutic target for the treatment of multiple sclerosis, diabetes mellitus, Parkinson’s disease, cancer, and others diseases. Several Nrf2 activators were currently being tested in human clinical trials. For instance, bardoxolone methyl is an OA derivative tested in the Phase II/III clinical trials for the treatment of Alport syndrome, a genetic disorder characterized by glomerulonephritis, eye abnormalities, and hearing loss (Chin et al., 2018; Gross et al., 2018). Bardoxolone methyl activates Nrf2 by disrupting the Nrf2/Keap1 interaction and inhibits IKKβ kinase activity (Wang et al., 2014). It was also reported for its beneficial effect in Phase III clinical trial for type 2 diabetes and stage 4 chronic kidney disease patients, where it was shown to improve the estimated glomerular filtration rate (GFR) (Chin et al., 2018). It is suggested that bardoxolone methyl increases GFR by restoring endothelial dysfunction and reducing angiotensin II-induced glomerular mesangial cell contraction (Aminzadeh et al., 2013; Ding et al., 2013). In addition, Protandim®, a nutritional supplement containing five natural Nrf2 activators such as bacosides, silymarin, withanolides, ECGC and curcumin has been shown to reduce oxidative stress and increase antioxidant enzymes SOD and catalase expression (Nelson et al., 2006). Similarly, increased SOD expression was also observed after oral Protandim® supplementation in runners (Ueberschlag et al., 2016). Considering that many studies have reported the role of natural compounds in activating Nrf2 pathway, further investigations and validations in the clinical setting may help to accelerate its development as therapeutics protecting against CVD.
Conclusion
Transcription factor Nrf2 serves as the master regulator of cellular antioxidant defense system which has shown to protect against endothelial dysfunction, foam cells formation and atherosclerotic lesion development. Compelling evidences in this paper have demonstrated that a wide range of bioactive compounds derived from natural sources activate Nrf2/Keap1 signaling and protect against CVD development. These studies suggest that bioactive compounds may serve as new therapeutic strategies targeting CVD via Nrf2 pathway.
Author Contributions
This writing was performed by BO. BG and K-GC provided vital guidance and insight to the work. WY did some literature review and amended the review. K-GC, BG, and WY contributed to the funding of the project. The project was conceptualized by WY and BG.
Funding
This work was supported by the Ministry of Education (MOE) Fundamental Research Grant Scheme (FRGS/1/2015/SKK08/TAYLOR/03/1) awarded to WY; MOSTI eScience Fund (02-02-10-SF0215) awarded to BG, and University of Malaya Research Grants PG136-2016A and PG082-2015B awarded to K-GC.
Conflict of Interest Statement
The authors declare that the research was conducted in the absence of any commercial or financial relationships that could be construed as a potential conflict of interest.
References
Aminzadeh, M. A., Reisman, S. A., Vaziri, N. D., Shelkovnikov, S., Farzaneh, S. H., Khazaeli, M., et al. (2013). The synthetic triterpenoid RTA dh404 (CDDO-dhTFEA) restores endothelial function impaired by reduced Nrf2 activity in chronic kidney disease. Redox Biol. 1, 527–531. doi: 10.1016/j.redox.2013.10.007
An, L., Zhou, M., Marikar, F. M. M. T., Hu, X. W., Miao, Q. Y., Li, P., et al. (2017). Salvia miltiorrhiza lipophilic fraction attenuates oxidative stress in diabetic nephropathy through activation of nuclear factor erythroid 2-related factor 2. Am. J. Chin. Med. 45, 1441–1457. doi: 10.1142/s0192415x17500781
Apopa, P. L., He, X., and Ma, Q. (2008). Phosphorylation of Nrf2 in the transcription activation domain by casein kinase 2 (CK2) is critical for the nuclear translocation and transcription activation function of Nrf2 in IMR-32 neuroblastoma cells. J. Biochem. Mol. Toxicol. 22, 63–76. doi: 10.1002/jbt.20212
Bai, Y., Wang, X., Zhao, S., Ma, C., Cui, J., and Zheng, Y. (2015). Sulforaphane protects against cardiovascular disease via Nrf2 activation. Oxid. Med. Cell. Longev. 2015:407580. doi: 10.1155/2015/407580
Barajas, B., Che, N., Yin, F., Rowshanrad, A., Orozco, L. D., Gong, K. W., et al. (2011). NF-E2-related factor 2 promotes atherosclerosis by effects on plasma lipoproteins and cholesterol transport that overshadow antioxidant protection. Arterioscler. Thromb. Vasc. Biol. 31, 58–66. doi: 10.1161/ATVBAHA.110.210906
Basak, P., Sadhukhan, P., Sarkar, P., and Sil, P. C. (2017). Perspectives of the Nrf-2 signaling pathway in cancer progression and therapy. Toxicol. Rep. 4, 306–318. doi: 10.1016/j.toxrep.2017.06.002
Beischlag, T. V., Morales, J. L., Hollingshead, B. D., and Perdew, G. H. (2008). The aryl hydrocarbon receptor complex and the control of gene expression. Crit. Rev. Eukaryot. Gene Expr. 18, 207–250.
Bigagli, E., Cinci, L., Paccosi, S., Parenti, A., D’Ambrosio, M., and Luceri, C. (2017). Nutritionally relevant concentrations of resveratrol and hydroxytyrosol mitigate oxidative burst of human granulocytes and monocytes and the production of pro-inflammatory mediators in LPS-stimulated RAW 264.7 macrophages. Int. Immunopharmacol. 43, 147–155. doi: 10.1016/j.intimp.2016.12.012
Boettler, U., Sommerfeld, K., Volz, N., Pahlke, G., Teller, N., Somoza, V., et al. (2011). Coffee constituents as modulators of Nrf2 nuclear translocation and ARE (EpRE)-dependent gene expression. J. Nutr. Biochem. 22, 426–440. doi: 10.1016/j.jnutbio.2010.03.011
Bryan, H. K., Olayanju, A., Goldring, C. E., and Park, B. K. (2013). The Nrf2 cell defence pathway: Keap1-dependent and -independent mechanisms of regulation. Biochem. Pharmacol. 85, 705–717. doi: 10.1016/j.bcp.2012.11.016
Buommino, E., D’Abrosca, B., Donnarumma, G., Parisi, A., Scognamiglio, M., Fiorentino, A., et al. (2017). Evaluation of the antioxidant properties of carexanes in AGS cells transfected with the Helicobacter pylori’s protein HspB. Microb. Pathog. 108, 71–77. doi: 10.1016/j.micpath.2017.05.007
Canning, P., Sorrell, F. J., and Bullock, A. N. (2015). Structural basis of Keap1 interactions with Nrf2. Free Rad. Biol. Med. 88(Pt B), 101–107. doi: 10.1016/j.freeradbiomed.2015.05.034
Cao, H., Wang, L., Chen, B., Zheng, P., He, Y., Ding, Y., et al. (2016). DNA demethylation upregulated Nrf2 expression in Alzheimer’s disease cellular model. Front. Aging Neurosci. 7:244. doi: 10.3389/fnagi.2015.00244
Chen, H., Fu, J., Chen, H., Hu, Y., Soroka, D. N., Prigge, J. R., et al. (2014). Ginger compound [6]-shogaol and its cysteine-conjugated metabolite (M2) activate nrf2 in colon epithelial cells in vitro and in vivo. Chem. Res. Toxicol. 27, 1575–1585. doi: 10.1021/tx500211x
Chen, X., Liu, Y., Zhu, J., Lei, S., Dong, Y., Li, L., et al. (2016). GSK-3β downregulates Nrf2 in cultured cortical neurons and in a rat model of cerebral ischemia-reperfusion. Sci. Rep. 6:20196. doi: 10.1038/srep20196
Chin, M. P., Bakris, G. L., Block, G. A., Chertow, G. M., Goldsberry, A., Inker, L. A., et al. (2018). Bardoxolone methyl improves kidney function in patients with chronic kidney disease stage 4 and type 2 diabetes: post-Hoc analyses from bardoxolone methyl evaluation in patients with chronic kidney disease and type 2 diabetes study. Am. J. Nephrol. 47, 40–47. doi: 10.1159/000486398
Cho, H. (2013). Genomic structure and variation of nuclear factor (erythroid-derived 2)-like 2. Oxid. Med. Cell. Longev. 2013:24. doi: 10.1155/2013/286524
Choi, E. S., Yoon, J. J., Han, B. H., Jeong, D. H., Lee, Y. J., Kang, D. G., et al. (2018). Ligustilide attenuates vascular inflammation and activates Nrf2/HO-1 induction and, NO synthesis in HUVECs. Phytomedicine 38, 12–23. doi: 10.1016/j.phymed.2017.09.022
Ci, X., Zhou, J., Lv, H., Yu, Q., Peng, L., and Hua, S. (2017). Betulin exhibits anti-inflammatory activity in LPS-stimulated macrophages and endotoxin-shocked mice through an AMPK/AKT/Nrf2-dependent mechanism. Cell Death Amp. Dis. 8:e2798. doi: 10.1038/cddis.2017.39
Collins, A. R., Gupte, A. A., Ji, R., Ramirez, M. R., Minze, L. J., Liu, J. Z., et al. (2012). Myeloid deletion of nuclear factor erythroid 2-related factor 2 increases atherosclerosis and liver injury. Arterioscler. Thromb. Vasc. Biol. 32, 2839–2846. doi: 10.1161/ATVBAHA.112.300345
Copple, I. M., Lister, A., Obeng, A. D., Kitteringham, N. R., Jenkins, R. E., Layfield, R., et al. (2010). Physical and functional interaction of sequestosome 1 with Keap1 regulates the Keap1-Nrf2 cell defense pathway. J. Biol. Chem. 285, 16782–16788. doi: 10.1074/jbc.M109.096545
Cullinan, S. B., and Diehl, J. A. (2004). PERK-dependent activation of Nrf2 contributes to redox homeostasis and cell survival following endoplasmic reticulum stress. J. Biol. Chem. 279, 20108–20117. doi: 10.1074/jbc.M314219200
Dal, S., and Sigrist, S. (2016). The protective effect of antioxidants consumption on diabetes and vascular complications. Diseases 4:24. doi: 10.3390/diseases4030024
Damy, T., Kirsch, M., Khouzami, L., Caramelle, P., Le Corvoisier, P., Roudot-Thoraval, F., et al. (2009). Glutathione deficiency in cardiac patients is related to the functional status and structural cardiac abnormalities. PLoS One 4:e4871. doi: 10.1371/journal.pone.0004871
Dietrich, C. (2016). Antioxidant functions of the aryl hydrocarbon receptor. Stem Cells Int. 2016:10. doi: 10.1155/2016/7943495
Ding, Y., Stidham, R., Bumeister, R., Trevino, I., Winters, A., Sprouse, M., et al. (2013). The synthetic triterpenoid, RTA405, increases glomerular filtration rate and reduces angiotensin II-induced contraction of glomerular mesangial cells. Kidney Int. 83, 845–854. doi: 10.1038/ki.2012.393
Ding, Y., Zhang, B., Zhou, K., Chen, M., Wang, M., Jia, Y., et al. (2014). Dietary ellagic acid improves oxidant-induced endothelial dysfunction and atherosclerosis: Role of Nrf2 activation. Int. J. Cardiol. 175, 508–514. doi: 10.1016/j.ijcard.2014.06.045
Duan, J., Guan, Y., Mu, F., Guo, C., Zhang, E., Yin, Y., et al. (2017). Protective effect of butin against ischemia/reperfusion-induced myocardial injury in diabetic mice: involvement of the AMPK/GSK-3β/Nrf2 signaling pathway. Sci. Rep. 7:41491. doi: 10.1038/srep41491
Fratantonio, D., Speciale, A., Ferrari, D., Cristani, M., Saija, A., and Cimino, F. (2015). Palmitate-induced endothelial dysfunction is attenuated by cyanidin-3-O-glucoside through modulation of Nrf2/Bach1 and NF-κB pathways. Toxicol. Lett. 239, 152–160. doi: 10.1016/j.toxlet.2015.09.020
Freigang, S., Ampenberger, F., Spohn, G., Heer, S., Shamshiev, A. T., Kisielow, J., et al. (2011). Nrf2 is essential for cholesterol crystal-induced inflammasome activation and exacerbation of atherosclerosis. Eur. J. Immunol. 41, 2040–2051. doi: 10.1002/eji.201041316
Furue, M., Uchi, H., Mitoma, C., Hashimoto-Hachiya, A., Chiba, T., Ito, T., et al. (2017). Antioxidants for healthy skin: the emerging role of aryl hydrocarbon receptors and nuclear factor-erythroid 2-related factor-2. Nutrients 9:223. doi: 10.3390/nu9030223
Gonzlez-Reyes, S., Guzmn-Beltrn, S., Medina-Campos, O. N., and Pedraza-Chaverri, J. (2013). Curcumin pretreatment induces Nrf2 and an antioxidant response and prevents hemin-induced toxicity in primary cultures of cerebellar granule neurons of rats. Oxid. Med. Cell. Longev. 2013:14. doi: 10.1155/2013/801418
Gross, O., Appel, G., Block, G., Chin, M., Goldsberry, A., Inker, L., et al. (2018). A phase 2/3 study of the efficacy and safety of bardoxolone methyl in patients with Alport syndrome. Nephrol. Dialys. Trans. 33(Suppl._1), i384–i385. doi: 10.1093/ndt/gfy104.SP121
Grosso, G., Stepaniak, U., Micek, A., Stefler, D., Bobak, M., and Pająk, A. (2017). Dietary polyphenols are inversely associated with metabolic syndrome in Polish adults of the HAPIEE study. Eur. J. Nutr. 56, 1409–1420. doi: 10.1007/s00394-016-1187-z
Grover-Páez, F., and Zavalza-Gómez, A. B. (2009). Endothelial dysfunction and cardiovascular risk factors. Diabetes. Res. Clin. Pract 84, 1–10. doi: 10.1016/j.diabres.2008.12.013
Guo, Y., Yu, S., Zhang, C., and Kong, A.-N. T. (2015). Epigenetic regulation of Keap1-Nrf2 signaling. Free Radic. Biol. Med. 88(Pt B), 337–349. doi: 10.1016/j.freeradbiomed.2015.06.013
Hadi, H. A. R., Carr, C. S., and Al Suwaidi, J. (2005). Endothelial dysfunction: cardiovascular risk factors, therapy, and outcome. Vasc. Health Risk Manag. 1, 183–198.
Hajjar, D. P., and Gotto, A. M. (2013). Biological relevance of inflammation and oxidative stress in the pathogenesis of arterial diseases. Am. J. Pathol. 182, 1474–1481. doi: 10.1016/j.ajpath.2013.01.010
Hamed, I., Özogul, F., Özogul, Y., and Regenstein, J. M. (2015). Marine bioactive compounds and their health benefits: a review. Compr. Rev. Food Sci. Food Saf. 14, 446–465. doi: 10.1111/1541-4337.12136
Harada, N., Ito, K., Hosoya, T., Mimura, J., Maruyama, A., Noguchi, N., et al. (2012). Nrf2 in bone marrow-derived cells positively contributes to the advanced stage of atherosclerotic plaque formation. Free Rad. Biol. Med. 53, 2256–2262. doi: 10.1016/j.freeradbiomed.2012.10.001
Hashimoto, K., Simmons, A. N., Kajino-Sakamoto, R., Tsuji, Y., and Ninomiya-Tsuji, J. (2016). TAK1 regulates the Nrf2 antioxidant system through modulating p62/ SQSTM1. Antioxid. Redox Signal. 25, 953–964. doi: 10.1089/ars.2016.6663
Heyninck, K., Sabbe, L., Chirumamilla, C. S., Szarc, vel Szic, K., Vander Veken, P., et al. (2016). Withaferin A induces heme oxygenase (HO-1) expression in endothelial cells via activation of the Keap1/Nrf2 pathway. Biochem. Pharmacol. 109, 48–61. doi: 10.1016/j.bcp.2016.03.026
Hong, B., Su, Z., Zhang, C., Yang, Y., Guo, Y., Li, W., et al. (2016). Reserpine inhibit the JB6 P + cell transformation through epigenetic reactivation of Nrf2-mediated anti-oxidative stress pathway. AAPS J. 18, 659–669. doi: 10.1208/s12248-016-9901-6
Hu, C., Eggler, A. L., Mesecar, A. D., and van Breemen, R. B. (2011). Modification of Keap1 cysteine residues by sulforaphane. Chem. Res. Toxicol. 24, 515–521. doi: 10.1021/tx100389r
Huang, C. S., Lin, A. H., Liu, C. T., Tsai, C. W., Chang, I. S., Chen, H. W., et al. (2013). Isothiocyanates protect against oxidized LDL-induced endothelial dysfunction by upregulating Nrf2-dependent antioxidation and suppressing NFκB activation. Mol. Nutr. Food Res. 57, 1918–1930. doi: 10.1002/mnfr.201300063
Hussain, T., Tan, B., Yin, Y., Blachier, F., Tossou, M. C. B., and Rahu, N. (2016). Oxidative stress and inflammation: What polyphenols can do for us? Oxid. Med. Cell. Longev. 2016:7432797. doi: 10.1155/2016/7432797
Hwang, Y. P., Yun, H. J., Chun, H. K., Chung, Y. C., Kim, H. K., Jeong, M. H., et al. (2009). Protective mechanisms of 3-caffeoyl, 4-dihydrocaffeoyl quinic acid from Salicornia herbacea against tert-butyl hydroperoxide-induced oxidative damage. Chem. Biol. Interact 181, 366–376. doi: 10.1016/j.cbi.2009.07.017
Ichimura, Y., Waguri, S., Sou, Y.-S., Kageyama, S., Hasegawa, J., Ishimura, R., et al. (2013). Phosphorylation of p62 activates the Keap1-Nrf2 pathway during selective autophagy. Mol. Cell 51, 618–631. doi: 10.1016/j.molcel.2013.08.003
Ishida, Y., Ohta, K., Naruse, T., Kato, H., Fukui, A., Shigeishi, H., et al. (2018). Candida albicans β-glucan-containing particles increase HO-1 expression in oral keratinocytes via a reactive oxygen species/p38 mitogen-activated protein kinase/Nrf2 pathway. Infect. Immun. 86, e575–e517. doi: 10.1128/iai.00575-17
Jain, A., Lamark, T., Sjøttem, E., Bowitz Larsen, K., Atesoh Awuh, J., Øvervatn, A., et al. (2010). p62/SQSTM1 is a target gene for transcription factor NRF2 and creates a positive feedback loop by inducing antioxidant response element-driven gene transcription. J. Biol. Chem. 285, 22576–22591. doi: 10.1074/jbc.M110.118976
Jeddi, F., Soozangar, N., Sadeghi, M. R., Somi, M. H., and Samadi, N. (2017). Contradictory roles of Nrf2/Keap1 signaling pathway in cancer prevention/promotion and chemoresistance. DNA Repair. 54, 13–21. doi: 10.1016/j.dnarep.2017.03.008
Jiang, J., Mo, Z., Yin, K., Zhao, G., Lv, Y., Ouyang, X., et al. (2012). Epigallocatechin-3-gallate prevents TNF-α-induced NF-κB activation thereby upregulating ABCA1 via the Nrf2/Keap1 pathway in macrophage foam cells. Int. J. Mol. Med. 29, 946–956. doi: 10.3892/ijmm.2012.924
Jiang, Q., Wang, D., Han, Y., Han, Z., Zhong, W., and Wang, C. (2015). Modulation of oxidized-LDL receptor-1 (LOX1) contributes to the antiatherosclerosis effect of oleanolic acid. Int. J. Biochem. Cell Biol. 69, 142–152. doi: 10.1016/j.biocel.2015.10.023
Jiang, Y., Bao, H., Ge, Y., Tang, W., Cheng, D., Luo, K., et al. (2015). Therapeutic targeting of GSK3β enhances the Nrf2 antioxidant response and confers hepatic cytoprotection in hepatitis C. Gut 64, 168–179. doi: 10.1136/gutjnl-2013-306043
Jiang, X., Bao, Y., Liu, H., Kou, X., Zhang, Z., Sun, F., et al. (2017). VPS34 stimulation of p62 phosphorylation for cancer progression. Oncogene 36:6850. doi: 10.1038/onc.2017.295
Jie, Y., Renyi, W., Wenji, L., Linbo, G., Yuqing, Y., Ping, L., et al. (2018). The triterpenoid corosolic acid blocks transformation and epigenetically reactivates Nrf2 in TRAMP-C1 prostate cells. Mol. Carcinog. 57, 512–521. doi: 10.1002/mc.22776
Jin, W., Wang, H., Yan, W., Xu, L., Wang, X., Zhao, X., et al. (2008). Disruption of Nrf2 enhances upregulation of nuclear factor-κB activity, proinflammatory cytokines, and intercellular adhesion molecule-1 in the brain after traumatic brain injury. Mediat. Inflamm. 2008:725174. doi: 10.1155/2008/725174
Joo, M. S., Kim, W. D., Lee, K. Y., Kim, J. H., Koo, J. H., and Kim, S. G. (2016). AMPK facilitates nuclear accumulation of nrf2 by phosphorylating at serine 550. Mol. Cell. Biol. 36, 1931–1942. doi: 10.1128/mcb.00118-16
Kapuy, O., Papp, D., Vellai, T., Bánhegyi, G., and Korcsmáros, T. (2018). Systems-level feedbacks of Nrf2 controlling autophagy upon oxidative stress response. Antioxidants 7:39. doi: 10.3390/antiox7030039
Keum, Y.-S., Yu, S., Chang, P. P.-J., Yuan, X., Kim, J.-H., Xu, C., et al. (2006). Mechanism of action of sulforaphane: Inhibition of p38 mitogen-activated protein kinase isoforms contributing to the induction of antioxidant response element–mediated heme oxygenase-1 in human hepatoma HepG2 cells. Cancer Res. 66, 8804–8813. doi: 10.1158/0008-5472.can-05-3513
Khor, T. O., Huang, Y., Wu, T.-Y., Shu, L., Lee, J., and Kong, A.-N. T. (2011). Pharmacodynamics of curcumin as DNA hypomethylation agent in restoring the expression of Nrf2 via promoter CpGs demethylation. Biochem. Pharmacol. 82, 1073–1078. doi: 10.1016/j.bcp.2011.07.065
Kobayashi, M., and Yamamoto, M. (2006). Nrf2–Keap1 regulation of cellular defense mechanisms against electrophiles and reactive oxygen species. Adv. Enzyme Regul. 46, 113–140. doi: 10.1016/j.advenzreg.2006.01.007
Korashy, H. M., and El-Kadi, A. O. S. (2006). The role of aryl hydrocarbon receptor in the pathogenesis of cardiovascular diseases. Drug Metab. Rev. 38, 411–450. doi: 10.1080/03602530600632063
Kuang, H., Tang, Z., Zhang, C., Wang, Z., Li, W., Yang, C., et al. (2017). Taxifolin activates the Nrf2 anti-oxidative stress pathway in mouse skin epidermal JB6 P + cells through epigenetic modifications. Int. J. Mol. Sci. 18:1546. doi: 10.3390/ijms18071546
Kunjathoor, V. V., Febbraio, M., Podrez, E. A., Moore, K. J., Andersson, L., Koehn, S., et al. (2002). Scavenger receptors class A-I/II and CD36 are the principal receptors responsible for the uptake of modified low density lipoprotein leading to lipid loading in macrophages. J. Biol. Chem. 277, 49982–49988. doi: 10.1074/jbc.M209649200
Lee, D.-F., Kuo, H.-P., Liu, M., Chou, C.-K., Xia, W., Du, Y., et al. (2009). Keap1 E3 ligase-mediated down-regulation of NF-κB signaling by targeting IKKβ. Mol. Cell. 36, 131–140. doi: 10.1016/j.molcel.2009.07.025
Levonen, A., Inkala, M., Heikura, T., Jauhiainen, S., Jyrkkänen, H., Kansanen, E., et al. (2007). Nrf2 gene transfer induces antioxidant enzymes and suppresses smooth muscle cell growth in vitro and reduces oxidative stress in rabbit aorta in vivo. Arterioscler. Thromb. Vasc. Biol. 27, 741–747. doi: 10.1161/01.ATV.0000258868.80079.4d
Li, H., Song, F., Duan, L.-R., Sheng, J.-J., Xie, Y.-H., Yang, Q., et al. (2016). Paeonol and danshensu combination attenuates apoptosis in myocardial infarcted rats by inhibiting oxidative stress: Roles of Nrf2/HO-1 and PI3K/Akt pathway. Sci. Rep. 6:23693. doi: 10.1038/srep23693
Liu, C. M., Ma, J. Q., Xie, W. R., Liu, S. S., Feng, Z. J., Zheng, G. H., et al. (2015). Quercetin protects mouse liver against nickel-induced DNA methylation and inflammation associated with the Nrf2/HO-1 and p38/STAT1/NF-κB pathway. Food Chem. Toxicol. 82, 19–26. doi: 10.1016/j.fct.2015.05.001
Liu, G. H., Qu, J., and Shen, X. (2008). NF-κB/p65 antagonizes Nrf2-ARE pathway by depriving CBP from Nrf2 and facilitating recruitment of HDAC3 to MafK. Biochimica et Biophysica Acta Mol. Cell Res. 1783, 713–727. doi: 10.1016/j.bbamcr.2008.01.002
Liu, X., Zhang, Y., Wu, H., Zhu, P., Mo, X., and Ying, J. (2017). Suppression of microRNA-128 attenuates high glucose-induced podocyte apoptosis through activation of Nrf2-ARE signaling pathway. Int. J. Clin. Exp. Pathol. 10, 922–931.
Liu, Z., Wang, J., Huang, E., Gao, S., Li, H., Lu, J., et al. (2014). Tanshinone IIA suppresses cholesterol accumulation in human macrophages: role of heme oxygenase-1. J. Lipid Res. 55, 201–213. doi: 10.1194/jlr.M040394
Lujambio, A., and Lowe, S. W. (2012). The microcosmos of cancer. Nature 482:347. doi: 10.1038/nature10888
Luo, Y., Lu, S., Dong, X., Xu, L., Sun, G., and Sun, X. (2017). Dihydromyricetin protects human umbilical vein endothelial cells from injury through ERK and Akt mediated Nrf2/HO-1 signaling pathway. Apoptosis 22, 1013–1024. doi: 10.1007/s10495-017-1381-3
Lv, H., Liu, Q., Wen, Z., Feng, H., Deng, X., and Ci, X. (2017). Xanthohumol ameliorates lipopolysaccharide (LPS)-induced acute lung injury via induction of AMPK/GSK3β-Nrf2 signal axis. Redox Biol. 12, 311–324. doi: 10.1016/j.redox.2017.03.001
Mahfouz, M. M., Zhou, S. Q., and Kummerow, F. A. (2009). Curcumin prevents the oxidation and lipid modification of LDL and its inhibition of prostacyclin generation by endothelial cells in culture. Prostagland. Other Lipid Mediat. 90, 13–20. doi: 10.1016/j.prostaglandins.2009.06.005
Mäkinen, P. I., Lappalainen, J. P., Heinonen, S. E., Leppänen, P., Lähteenvuo, M. T., Aarnio, J. V., et al. (2010). Silencing of either SR-A or CD36 reduces atherosclerosis in hyperlipidaemic mice and reveals reciprocal upregulation of these receptors. Cardiovasc. Res. 88, 530–538. doi: 10.1093/cvr/cvq235
Manivasagan, P., Venkatesan, J., Sivakumar, K., and Kim, S. K. (2014). Pharmaceutically active secondary metabolites of marine actinobacteria. Microbiol. Res. 169, 262–278. doi: 10.1016/j.micres.2013.07.014
Maracle, C. X., Agca, R., Helder, B., Meeuwsen, J. A. L., Niessen, H. W. M., Biessen, E. A. L., et al. (2018). Noncanonical NF-κB signaling in microvessels of atherosclerotic lesions is associated with inflammation, atheromatous plaque morphology and myocardial infarction. Atherosclerosis 270, 33–41. doi: 10.1016/j.atherosclerosis.2018.01.032
Mascuch, S. J., Boudreau, P. D., Carland, T. M., Pierce, N. T., Olson, J., Hensler, M. E., et al. (2018). Marine natural product Honaucin A attenuates inflammation by activating the Nrf2-ARE pathway. J. Nat. Prod. 81, 506–514. doi: 10.1021/acs.jnatprod.7b00734
Matzinger, M., Fischhuber, K., and Heiss, E. H. (2017). Activation of Nrf2 signaling by natural products-can it alleviate diabetes. Biotechnol. Adv. 36, 1738–1767. doi: 10.1016/j.biotechadv.2017.12.015
Medina-Remón, A., Casas, R., Tressserra-Rimbau, A., Ros, E., Martínez-González, M. A., Fitó, M., et al. (2017). Polyphenol intake from a Mediterranean diet decreases inflammatory biomarkers related to atherosclerosis: a substudy of the PREDIMED trial. Br. J. Clin. Pharmacol. 83, 114–128. doi: 10.1111/bcp.12986
Menegon, S., Columbano, A., and Giordano, S. (2016). The dual roles of Nrf2 in cancer. Trends Mol. Med. 22, 578–593. doi: 10.1016/j.molmed.2016.05.002
Miao, W., Hu, L., Scrivens, P. J., and Batist, G. (2005). Transcriptional regulation of NF-E2 p45-related factor (NRF2) expression by the aryl hydrocarbon receptor-xenobiotic response element signaling pathway: direct cross-talk between phase I and II drug-metabolizing enzymes. J. Biol. Chem. 280, 20340–20348. doi: 10.1074/jbc.M412081200
Mimura, J., and Fujii-Kuriyama, Y. (2003). Functional role of AhR in the expression of toxic effects by TCDD. Biochimica et Biophysica Acta General Subjects 1619, 263–268. doi: 10.1016/S0304-4165(02)00485-3
Muta, K., Obata, Y., Oka, S., Abe, S., Minami, K., Kitamura, M., et al. (2016). Curcumin ameliorates nephrosclerosis via suppression of histone acetylation independent of hypertension. Nephrol. Dialys. Transplant. 31, 1615–1623. doi: 10.1093/ndt/gfw036
Namani, A., Li, Y., Wang, X. J., and Tang, X. (2014). Modulation of Nrf2 signaling pathway by nuclear receptors: implications for cancer. Biochimica et Biophysica Acta Mol. Cell Res. 1843, 1875–1885. doi: 10.1016/j.bbamcr.2014.05.003
Nebert, D. W. (2017). Aryl hydrocarbon receptor (AHR): “Pioneer member” of the basic-helix/loop/helix per-Arnt-sim (bHLH/PAS) family of “sensors” of foreign and endogenous signals. Prog. Lipid Res. 67, 38–57. doi: 10.1016/j.plipres.2017.06.001
Nelson, S. K., Bose, S. K., Grunwald, G. K., Myhill, P., and McCord, J. M. (2006). The induction of human superoxide dismutase and catalase in vivo: a fundamentally new approach to antioxidant therapy. Free Rad. Biol. Med. 40, 341–347. doi: 10.1016/j.freeradbiomed.2005.08.043
Nguyen, T., Sherratt, P. J., Huang, H. C., Yang, C. S., and Pickett, C. B. (2003). Increased protein stability as a mechanism that enhances Nrf2-mediated transcriptional activation of the antioxidant response element: degradation of Nrf2 by the 26 S proteasome. J. Biol. Chem. 278, 4536–4541. doi: 10.1074/jbc.M207293200
Ni, J., Li, Y., Li, W., and Guo, R. (2017). Salidroside protects against foam cell formation and apoptosis, possibly via the MAPK and AKT signaling pathways. Lipids Health Dis. 16:198. doi: 10.1186/s12944-017-0582-7
Niu, T., Xuan, R., Jiang, L., Wu, W., Zhen, Z., Song, Y., et al. (2018). Astaxanthin induces the Nrf2/HO-1 antioxidant pathway in human umbilical vein endothelial cells by generating trace amounts of ROS. J. Agric. Food Chem. 66, 1551–1559. doi: 10.1021/acs.jafc.7b05493
Ohnuma, T., Nakayama, S., Anan, E., Nishiyama, T., Ogura, K., and Hiratsuka, A. (2010). Activation of the Nrf2/ARE pathway via S-alkylation of cysteine 151 in the chemopreventive agent-sensor Keap1 protein by falcarindiol, a conjugated diacetylene compound. Toxicol. Appl. Pharmacol. 244, 27–36. doi: 10.1016/j.taap.2009.12.012
Ooi, B. K., Goh, B. H., and Yap, W. H. (2017). Oxidative stress in cardiovascular diseases: Involvement of Nrf2 antioxidant redox signaling in macrophage foam cells formation. Int. J. Mol. Sci. 18:2336. doi: 10.3390/ijms18112336
Panchal, S. K., Poudyal, H., and Brown, L. (2012). Quercetin ameliorates cardiovascular, hepatic, and metabolic changes in diet-induced metabolic syndrome in rats. J. Nutr. 142, 1026–1032. doi: 10.3945/jn.111.157263
Pashkow, F. J. (2011). Oxidative stress and inflammation in heart disease: do antioxidants have a role in treatment and/or prevention? Int. J. Inflamm. 2011:9. doi: 10.4061/2011/514623
Qin, X., Qiu, C., and Zhao, L. (2014). Maslinic acid protects vascular smooth muscle cells from oxidative stress through Akt/Nrf2/HO-1 pathway. Mol. Cell. Biochem. 390, 61–67. doi: 10.1007/s11010-013-1956-4
Quintana, F. J. (2013). The aryl hydrocarbon receptor: a molecular pathway for the environmental control of the immune response. Immunology 138, 183–189. doi: 10.1111/imm.12046
Rangel-Huerta, O., Pastor-Villaescusa, B., Aguilera, C., and Gil, A. (2015). A systematic review of the efficacy of bioactive compounds in cardiovascular disease: Phenolic compounds. Nutrients 7, 5177–5216. doi: 10.3390/nu7075177
Ribas, V., García-Ruiz, C., and Fernández-Checa, J. C. (2014). Glutathione and mitochondria. Front. Pharmacol. 5:151. doi: 10.3389/fphar.2014.00151
Ruotsalainen, A. K., Inkala, M., Partanen, M. E., Lappalainen, J. P., Kansanen, E., Mäkinen, P. I., et al. (2013). The absence of macrophage Nrf2 promotes early atherogenesis. Cardiovasc. Res. 98, 107–115. doi: 10.1093/cvr/cvt008
Ruotsalainen, A. K., Lappalainen, J. P., Heiskanen, E., Merentie, M., Sihvola, V., Näpänkangas, J., et al. (2018). Nuclear factor E2-related factor 2 deficiency impairs atherosclerotic lesion development but promotes features of plaque instability in hypercholesterolaemic mice. Cardiovasc. Res. 36:cvy143. doi: 10.1093/cvr/cvy143
Sahu, B. D., Kumar, J. M., Kuncha, M., Borkar, R. M., Srinivas, R., and Sistla, R. (2016). Baicalein alleviates doxorubicin-induced cardiotoxicity via suppression of myocardial oxidative stress and apoptosis in mice. Life Sci. 144, 8–18. doi: 10.1016/j.lfs.2015.11.018
Saito, R., Suzuki, T., Hiramoto, K., Asami, S., Naganuma, E., Suda, H., et al. (2016). Characterizations of three major cysteine sensors of Keap1 in stress response. Mol. Cell. Biol. 36, 271–284. doi: 10.1128/MCB.00868-15
Satoh, T., Kosaka, K., Itoh, K., Kobayashi, A., Yamamoto, M., Shimojo, Y., et al. (2008). Carnosic acid, a catechol-type electrophilic compound, protects neurons both in vitro and in vivo through activation of the Keap1/Nrf2 pathway via S-alkylation of targeted cysteines on Keap1. J. Neurochem. 104, 1116–1131. doi: 10.1111/j.1471-4159.2007.05039.x
Schaeffer, D. F., Riazy, M., Parhar, K. S., Chen, J. H., Duronio, V., Sawamura, T., et al. (2009). LOX-1 augments oxLDL uptake by lysoPC-stimulated murine macrophages but is not required for oxLDL clearance from plasma. J. Lipid Res. 50, 1676–1684. doi: 10.1194/jlr.M900167-JLR200
Shi, L., Wu, L., Chen, Z., Yang, J., Chen, X., Yu, F., et al. (2015). MiR-141 activates Nrf2-dependent antioxidant pathway via down-regulating the expression of Keap1 conferring the resistance of hepatocellular carcinoma cells to 5-fluorouracil. Cell Physiol. Biochem. 35, 2333–2348. doi: 10.1159/000374036
Song, M. G., Ryoo, I. G., Choi, H. Y., Choi, B. H., Kim, S. T., Heo, T. H., et al. (2015). NRF2 signaling negatively regulates phorbol-12-myristate-13-acetate (PMA)-induced differentiation of human monocytic U937 cells into pro-inflammatory macrophages. PLoS One 10:e0134235. doi: 10.1371/journal.pone.0134235
Sthijns, M. M. J. P. E., Schiffers, P. M., Janssen, G. M., Lemmens, K. J. A., Ides, B., Vangrieken, P., et al. (2017). Rutin protects against H2O2-triggered impaired relaxation of placental arterioles and induces Nrf2-mediated adaptation in human umbilical vein endothelial cells exposed to oxidative stress. Biochimica et Biophysica Acta General Sub. 1861(5 Part A), 1177–1189. doi: 10.1016/j.bbagen.2017.03.004
Su, Z. Y., Khor, T. O., Shu, L., Lee, J. H., Saw, C. L. L., Wu, T. Y., et al. (2013). Epigenetic reactivation of Nrf2 in murine prostate cancer TRAMP C1 cells by natural phytochemicals Z-ligustilide and Radix angelica sinensis via promoter CpG demethylation. Chem. Res. Toxicol. 26, 477–485. doi: 10.1021/tx300524p
Sun, W., Liu, X., Zhang, H., Song, Y., Li, T., Liu, X., et al. (2017). Epigallocatechin gallate upregulates Nrf2 to prevent diabetic nephropathy via disabling Keap1. Free Rad. Biol. Med. 108, 840–857. doi: 10.1016/j.freeradbiomed.2017.04.365
Sun, X., Ou, Z., Chen, R., Niu, X., Chen, D., Kang, R., et al. (2016). Activation of the p62-Keap1-NRF2 pathway protects against ferroptosis in hepatocellular carcinoma cells. Hepatology 63, 173–184. doi: 10.1002/hep.28251
Sussan, T. E., Jun, J., Thimmulappa, R., Bedja, D., Antero, M., Gabrielson, K. L., et al. (2008). Disruption of Nrf2, a key inducer of antioxidant defenses, attenuates ApoE-mediated atherosclerosis in mice. PLoS One 3:e3791. doi: 10.1371/journal.pone.0003791
Tsuji, G., Takahara, M., Uchi, H., Matsuda, T., Chiba, T., Takeuchi, S., et al. (2012). Identification of ketoconazole as an Ahr-Nrf2 activator in cultured human keratinocytes: the basis of its anti-inflammatory effect. J. Investigat. Dermatol. 132, 59–68. doi: 10.1038/jid.2011.194
Ueberschlag, S. L., Seay, J. R., Roberts, A. H., DeSpirito, P. C., Stith, J. M., Folz, R. J., et al. (2016). The effect of Protandim(®) supplementation on athletic performance and oxidative blood markers in runners. PLoS One 11:e0160559. doi: 10.1371/journal.pone.0160559
Waltenberger, B., Mocan, A., Šmejkal, K., Heiss, E., and Atanasov, A. (2016). Natural products to counteract the epidemic of cardiovascular and metabolic disorders. Mol. 21:807. doi: 10.3390/molecules21060807
Wang, B., Zhu, X., Kim, Y., Li, J., Huang, S., Saleem, S., et al. (2012). Histone deacetylase inhibition activates transcription factor Nrf2 and protects against cerebral ischemic damage. Free Rad. Biol. Med. 52, 928–936. doi: 10.1016/j.freeradbiomed.2011.12.006
Wang, N., Zhang, L., Lu, Y., Zhang, M., Zhang, Z., Wang, K., et al. (2017). Down-regulation of microRNA-142-5p attenuates oxygen-glucose deprivation and reoxygenation-induced neuron injury through up-regulating Nrf2/ARE signaling pathway. Biomed. Pharmacother. 89, 1187–1195. doi: 10.1016/j.biopha.2017.03.011
Wang, P., Liang, X., Lu, Y., Zhao, X., and Liang, J. (2016). MicroRNA-93 downregulation ameliorates cerebral ischemic injury through the Nrf2/HO-1 defense pathway. Neurochem. Res. 41, 2627–2635. doi: 10.1007/s11064-016-1975-0
Wang, T. Y., Li, Q., and Bi, K. S. (2018). Bioactive flavonoids in medicinal plants: structure, activity and biological fate. Asian J. Pharmaceut. Sci. 13, 12–23. doi: 10.1016/j.ajps.2017.08.004
Wang, Y.-Y., Yang, Y.-X., Zhe, H., He, Z.-X., and Zhou, S.-F. (2014). Bardoxolone methyl (CDDO-Me) as a therapeutic agent: An update on its pharmacokinetic and pharmacodynamic properties. Drug Design Dev. Ther. 8, 2075–2088. doi: 10.2147/DDDT.S68872
Watanabe, Y., Watanabe, K., Kobayashi, T., Saito, Y., Fujioka, D., Nakamura, T., et al. (2013). Chronic depletion of glutathione exacerbates ventricular remodelling and dysfunction in the pressure-overloaded heart. Cardiovasc. Res. 97, 282–292. doi: 10.1093/cvr/cvs333
Wei, B., You, M. G., Ling, J. J., Wei, L. L., Wang, K., Li, W. W., et al. (2013). Regulation of antioxidant system, lipids and fatty acid β-oxidation contributes to the cardioprotective effect of sodium tanshinone IIA sulphonate in isoproterenol-induced myocardial infarction in rats. Atherosclerosis 230, 148–156. doi: 10.1016/j.atherosclerosis.2013.07.005
Westerterp, M., Murphy, A. J., Wang, M., Pagler, T. A., Vengrenyuk, Y., Kappus, M. S., et al. (2013). Deficiency of ABCA1 and ABCG1 in macrophages increases inflammation and accelerates atherosclerosis in mice. Circ. Res 112, 1456–1465.
Westerterp, M., Tsuchiya, K., Tattersall, I. W., Fotakis, P., Bochem, A. E., Molusky, M. M., et al. (2016). Deficiency of ATP-binding cassette transporters A1 and G1 in endothelial cells accelerates atherosclerosis in mice. Arterioscler. Thromb. Vasc. Biol. 36, 1328–1337. doi: 10.1161/ATVBAHA.115.306670
Wong, S. Y., Tan, M. G. K., Wong, P. T. H., Herr, D. R., and Lai, M. K. P. (2016). Andrographolide induces Nrf2 and heme oxygenase 1 in astrocytes by activating p38 MAPK and ERK. J. Neuroinflamm. 13:251. doi: 10.1186/s12974-016-0723-3
World Health Organization (2018). Global Health Estimates 2016: Deaths by Cause, Age, Sex, by Country and By Region, 2000-2016 Available: http://www.who.int/healthinfo/global_burden_disease/estimates/en/ [accessed May 28, 2018].
Xu, X., Li, H., Hou, X., Li, D., He, S., Wan, C., et al. (2015). Punicalagin induces Nrf2/HO-1 expression via upregulation of PI3K/AKT pathway and inhibits LPS-induced oxidative stress in RAW264.7 macrophages. Mediat. Inflamm. 2015:380218. doi: 10.1155/2015/380218
Yang, D., Xiao, C. X., Su, Z. H., Huang, M. W., Qin, M., Wu, W. J., et al. (2017). (-)-7(S)-hydroxymatairesinol protects against tumor necrosis factor-α-mediated inflammation response in endothelial cells by blocking the MAPK/NF-κB and activating Nrf2/HO-1. Phytomedicine 32, 15–23. doi: 10.1016/j.phymed.2017.04.005
Yang, H. L., Chang, H. C., Lin, S. W., Senthil Kumar, K. J., Liao, C. H., Wang, H. M., et al. (2014). Antrodia salmonea inhibits TNF-α-induced angiogenesis and atherogenesis in human endothelial cells through the down-regulation of NF-κB and up-regulation of Nrf2 signaling pathways. J. Ethnopharmacol. 151, 394–406. doi: 10.1016/j.jep.2013.10.052
Yang, S., Chou, G., and Li, Q. (2018a). Cardioprotective role of azafrin in against myocardial injury in rats via activation of the Nrf2-ARE pathway. Phytomedicine 123, 92–100. doi: 10.1016/j.phymed.2018.04.042
Yang, Y., Li, X., Peng, L., An, L., Sun, N., Hu, X., et al. (2018b). Tanshindiol C inhibits oxidized low-density lipoprotein induced macrophage foam cell formation via a peroxiredoxin 1 dependent pathway. Biochimica et Biophysica Acta Mol. Basis Dis. 1864, 882–890. doi: 10.1016/j.bbadis.2017.12.033
Yang, Y., Yang, I., Cao, M., Su, Z.-Y., Wu, R., Guo, Y., et al. (2018c). Fucoxanthin elicits epigenetic modifications, Nrf2 activation and blocking transformation in mouse skin JB6 P + cells. AAPS J. 20:32. doi: 10.1208/s12248-018-0197-6
Yao, J., Zhang, B., Ge, C., Peng, S., and Fang, J. (2015). Xanthohumol, a polyphenol chalcone present in hops, activating Nrf2 enzymes to confer protection against oxidative damage in PC12 cells. J. Agric. Food Chem. 63, 1521–1531. doi: 10.1021/jf505075n
Ye, Z. W., Zhang, J., Townsend, D. M., and Tew, K. D. (2015). Oxidative stress, redox regulation and diseases of cellular differentiation. Biochim. Biophys. Acta 1850, 1607–1621. doi: 10.1016/j.bbagen.2014.11.010
Yen, J., Wu, P., Chen, S., and Wu, M. (2017). Fisetin protects PC12 cells from tunicamycin-mediated cell death via reactive oxygen species scavenging and modulation of Nrf2-driven gene expression, SIRT1 and MAPK signaling in PC12 cells. Int. J. Mol. Sci. 18:852. doi: 10.3390/ijms18040852
Yu, H., Shi, L., Zhao, S., Sun, Y., Gao, Y., Sun, Y., et al. (2016). Triptolide attenuates myocardial ischemia/reperfusion injuries in rats by inducing the activation of Nrf2/HO-1 defense pathway. Cardiovasc. Toxicol. 16, 325–335. doi: 10.1007/s12012-015-9342-y
Yu, S., Khor, T. O., Cheung, K. L., Li, W., Wu, T. Y., Huang, Y., et al. (2010). Nrf2 expression is regulated by epigenetic mechanisms in prostate cancer of TRAMP mice. PLoS One 5:e8579. doi: 10.1371/journal.pone.0008579
Yu, X., Cui, L., Zhang, Z., Zhao, Q., and Li, S. (2013). α-Linolenic acid attenuates doxorubicin-induced cardiotoxicity in rats through suppression of oxidative stress and apoptosis. Acta Biochimica et Biophysica Sinica 45, 817–826. doi: 10.1093/abbs/gmt082
Yvan-Charvet, L., Ranalletta, M., Wang, N., Han, S., Terasaka, N., Li, R., et al. (2007). Combined deficiency of ABCA1 and ABCG1 promotes foam cell accumulation and accelerates atherosclerosis in mice. J. Clin. Invest. 117, 3900–3908. doi: 10.1172/JCI33372
Zaina, S., Heyn, H., Carmona, F. J., Varol, N., Sayols, S., Condom, E., et al. (2014). DNA methylation map of human atherosclerosis. Circulation 7, 692–700. doi: 10.1161/circgenetics.113.000441
Zeng, C., Zhong, P., Zhao, Y., Kanchana, K., Zhang, Y., Khan, Z. A., et al. (2015). Curcumin protects hearts from FFA-induced injury by activating Nrf2 and inactivating NF-κB both in vitro and in vivo. J. Mol. Cell Cardiol. 79, 1–12. doi: 10.1016/j.yjmcc.2014.10.002
Zhang, C., Su, Z. Y., Khor, T. O., Shu, L., and Kong, A. N. T. (2013). Sulforaphane enhances Nrf2 expression in prostate cancer TRAMP C1 cells through epigenetic regulation. Biochem. Pharmacol. 85, 1398–1404. doi: 10.1016/j.bcp.2013.02.010
Zhang, Z., Chen, J., Zhou, S., Wang, S., Cai, X., Conklin, D. J., et al. (2015). Magnolia bioactive constituent 4-O-methylhonokiol prevents the impairment of cardiac insulin signaling and the cardiac pathogenesis in high-fat diet-induced obese mice. Int. J. Biol. Sci. 11, 879–891. doi: 10.7150/ijbs.12101
Zhao, F., Zhang, J., and Chang, N. (2018). Epigenetic modification of Nrf2 by sulforaphane increases the antioxidative and anti-inflammatory capacity in a cellular model of Alzheimer’s disease. Eur. J. Pharmacol 824, 1–10. doi: 10.1016/j.ejphar.2018.01.046
Zhao, L., Tao, X., Qi, Y., Xu, L., Yin, L., and Peng, J. (2018). Protective effect of dioscin against doxorubicin-induced cardiotoxicity via adjusting microRNA-140-5p-mediated myocardial oxidative stress. Redox Biol. 16, 189–198. doi: 10.1016/j.redox.2018.02.026
Zhao, S., Mao, L., Wang, S.-G., Chen, F.-L., Ji, F., and Fei, H.-D. (2017). MicroRNA-200a activates Nrf2 signaling to protect osteoblasts from dexamethasone. Oncotarget 8, 104867–104876. doi: 10.18632/oncotarget.20452
Zhou, J., Ma, X., Cui, Y., Song, Y., Yao, L., Liu, Y., et al. (2017). Methyleugenol protects against t-BHP-triggered oxidative injury by induction of Nrf2 dependent on AMPK/GSK3β and ERK activation. J. Pharmacol. Sci. 135, 55–63. doi: 10.1016/j.jphs.2017.09.003
Zhou, M. M., Zhang, W. Y., Li, R. J., Guo, C., Wei, S. S., Tian, X. M., et al. (2018). Anti-inflammatory activity of Khayandirobilide A from Khaya senegalensis via NF-κB, AP-1 and p38 MAPK/Nrf2/HO-1 signaling pathways in lipopolysaccharide-stimulated RAW 264.7 and BV-2 cells. Phytomedicine 42, 152–163. doi: 10.1016/j.phymed.2018.03.016
Zimmermann, K., Baldinger, J., Mayerhofer, B., Atanasov, A. G., Dirsch, V. M., and Heiss, E. H. (2015). Activated AMPK boosts the Nrf2/HO-1 signaling axis—A role for the unfolded protein response. Free Radic. Biol. Med. 88, 417–426. doi: 10.1016/j.freeradbiomed.2015.03.030
Keywords: nuclear factor erythroid 2-related factor 2 (Nrf2), cardiovascular diseases (CVDs), natural products, oxidative stress, nuclear factor-κB (NF-κB)
Citation: Ooi BK, Chan K-G, Goh BH and Yap WH (2018) The Role of Natural Products in Targeting Cardiovascular Diseases via Nrf2 Pathway: Novel Molecular Mechanisms and Therapeutic Approaches. Front. Pharmacol. 9:1308. doi: 10.3389/fphar.2018.01308
Received: 21 August 2018; Accepted: 25 October 2018;
Published: 15 November 2018.
Edited by:
Águeda González Rodríguez, Instituto de Investigación Sanitaria del Hospital Universitario de La Princesa, SpainReviewed by:
Gloria Virginia López, Universidad de la República, UruguayClaudio Ferrante, Università degli Studi G. d’Annunzio Chieti e Pescara, Italy
Copyright © 2018 Ooi, Chan, Goh and Yap. This is an open-access article distributed under the terms of the Creative Commons Attribution License (CC BY). The use, distribution or reproduction in other forums is permitted, provided the original author(s) and the copyright owner(s) are credited and that the original publication in this journal is cited, in accordance with accepted academic practice. No use, distribution or reproduction is permitted which does not comply with these terms.
*Correspondence: Kok-Gan Chan, a29rZ2FuQHVtLmVkdS5teQ== Wei Hsum Yap, d2VpaHN1bS55YXBAdGF5bG9ycy5lZHUubXk=