- 1Combat Trauma and Burn Injury Research, US Army Institute of Surgical Research, San Antonio, TX, United States
- 2Extremity Trauma and Regenerative Medicine, US Army Institute of Surgical Research, San Antonio, TX, United States
- 3Dental and Craniofacial Trauma Research, US Army Institute of Surgical Research, San Antonio, TX, United States
- 4Burn Flight Team, US Army Institute of Surgical Research, San Antonio, TX, United States
Burns are caused by several mechanisms including flame, scald, chemical, electrical, and ionizing and non-ionizing radiation. Approximately half a million burn cases are registered annually, of which 40 thousand patients are hospitalized and receive definitive treatment. Burn care is very resource intensive as the treatment regimens and length of hospitalization are substantial. Burn wounds are classified based on depth as superficial (first degree), partial-thickness (second degree), or full-thickness (third degree), which determines the treatment necessary for successful healing. The goal of burn wound care is to fully restore the barrier function of the tissue as quickly as possible while minimizing infection, scarring, and contracture. The aim of this review is to highlight how tissue engineering and regenerative medicine strategies are being used to address the unique challenges of burn wound healing and define the current gaps in care for both partial- and full-thickness burn injuries. This review will present the current standard of care (SOC) and provide information on various treatment options that have been tested pre-clinically or are currently in clinical trials. Due to the complexity of burn wound healing compared to other skin injuries, burn specific treatment regimens must be developed. Recently, tissue engineering and regenerative medicine strategies have been developed to improve skin regeneration that can restore normal skin physiology and limit adverse outcomes, such as infection, delayed re-epithelialization, and scarring. Our emphasis will be centered on how current clinical and pre-clinical research of pharmacological agents, biomaterials, and cellular-based therapies can be applied throughout the continuum of burn care by targeting the stages of wound healing: hemostasis, inflammation, cell proliferation, and matrix remodeling.
Purpose of the Review
Currently, multiple strategies exist for the management of burn wounds depending on both the depth and extent of the burn. Burn wound care strategies aim to modulate the inflammatory response, accelerate re-epithelialization, and improve overall wound healing. Furthermore, combinatorial approaches that incorporate cellular-based therapies, pharmacological agents, and biomaterials are utilized to minimize infection and serve as burn wound coverage adjuncts with the goal of restoration of skin function (i.e., barrier, range of motion, sensation, hair and sweat generation, and pigmentation). This review focuses on how therapies for burn injuries are currently being developed to address the array of issues that occur throughout the continuum of burn care. Specifically, this review investigates treatment modalities for thermal burns that are currently in clinical trials and pre-clinical animal testing. To accomplish this and ultimately illustrate the challenges that remain unmet, it is important to understand the current standard of care (SOC) for burn wound injuries. Next, the United States (US) Food and Drug Administration (FDA) approval process will be described to explain how current products have been approved in order to highlight the challenges that new ideas and technologies will encounter and how this affects the current design of new products. Finally, an analysis of clinical and pre-clinical studies utilizing the latest regenerative therapies will be presented that are addressing the different stages of burn wound healing.
Burn Injuries
Anatomy of Skin
Skin is the human body's largest organ, encompassing ~1.5–2.0 square meters for an average adult. It functions as a defensive barrier against foreign materials, assists in thermoregulation, prevents evaporative loss of fluids, acts as a sensory organ, and plays a role in Vitamin D production. It is composed of three layers: the epidermis, dermis, and hypodermis. The epidermis is the outermost layer, while the dermis is between the epidermis and hypodermis. The papillary dermis (i.e., upper dermal layer) consists of rete ridges, capillaries, and loosely arranged collagen fibers. The reticular dermis (i.e., lower dermal layer) contains blood vessels, nerves, roots of hair follicles, sebaceous and sweat glands, densely packed collagen fibers, and provides nutritional and structural support to the epidermis. The innermost layer is the hypodermis which consists of subcutaneous adipose tissue and associated blood and lymphatic vessels. This layer provides insulation, cushion from traumatic insults, buoyancy to the body, and possesses some endocrine functions (Marks and Miller, 2013; Fenner and Clark, 2016).
Burn Pathophysiology
Even though burn wounds directly affect the skin, severe burns (>20% total body surface area, TBSA) cause a systemic inflammatory response that results in damage throughout the entire body including the immune system, gastrointestinal system, and muscle. This systemic damage is much more pronounced in burn injuries compared to other forms of trauma (Tiwari, 2012). A hallmark indicator of this stress response is an increase in the metabolic rate or hyper-metabolism which can lead to an overall leaner body mass from the increased metabolic demands (Orgill, 2009; Porter et al., 2016). For this review, we only will be focusing on treatments for the primary burn injury. Management of severe burn injuries requires specialized burn centers staffed with burn specialists. Nutrition, pain control, and rehabilitation are important components of burn care, but will not be addressed here. Decades ago, it was understood that burn wounds were unique and healed slower than other traumatic wounds (Monsaingeon and Molimard, 1976). In contrast to excisional wounds, a burn injury occurs with varying degrees of cellular injury, and even viable tissue adjacent to the burn is affected with altered physiology (Monstrey et al., 2008). These physiologic differences translate to slower healing after burn injury compared to excisional injury. For these reasons, animal models with excisional wounds, even those that form hypertrophic scars (HTS), are difficult to extrapolate to burn wounds (Carlsson et al., 2016). For the scope of this review, we will be focusing on research and therapeutics which specifically target and were tested topically on thermal burn wounds.
Burn Incidence and Depth
The annual burn incidence in the United States is ~486,000 according to the National Burn Repository of the American Burn Association. Approximately 3,275 people lose their lives and 40,000 require hospitalization due to burn related injuries (American Burn Association, 2016). A superficial burn involves only the epidermis; a common example is a sunburn which will heal on its own within 7 days by keratinocyte proliferation and differentiation from the basal epithelial cells. Deeper burns can be distinguished based on characteristics including pain (high to none), color (pink/red to white/brown), and capillary refill (brisk to none). Superficial partial-thickness (SPT) burns involve the epidermis and papillary dermis and are very painful to the touch with brisk capillary refill. Deep partial-thickness (DPT) burns involve the reticular dermis including the adnexal structures while full-thickness (FT) burns involve all of the epidermis and dermis and may also affect the subcutaneous adipose tissue, muscle, or even bone. Burn injuries are considered acute wounds that heal via the wound healing cascade (Lazarus et al., 1994; Figure 1). A major clinical challenge is determining the burn depth, which correlates with the amount of time the wound will need to heal. This assessment is extremely important due to the fact that wounds that take longer than 3 weeks to heal on their own have a high risk of forming a HTS (Monstrey et al., 2008). It is estimated that clinical assessment is only accurate ~65% of the time with indeterminate PT burns, where the differentiation between SPT and DPT is difficult (Heimbach et al., 1984; Zuo et al., 2017). A myriad of non-invasive techniques to assess the burn depth have been extensively explored in the clinical and pre-clinical setting. Currently, laser-Doppler imaging, which measures the perfusion or lack thereof in burned tissue, is the only modality that has earned FDA approval for burn assessment (Monstrey et al., 2008).
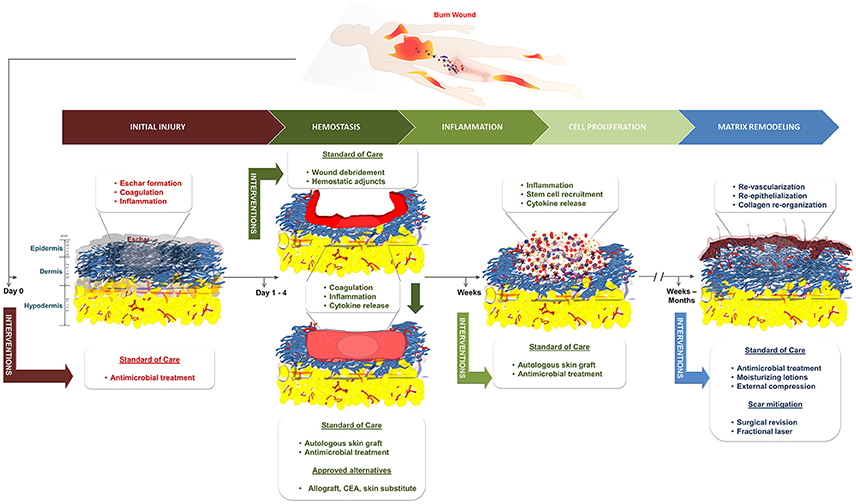
Figure 1. Schematic of the standard of care and the phases of healing for burn wounds. CEA, cultured epithelial autograft.
Standard of Care
Infection Prevention
After the initial burn injury, infection is the primary cause of death and morbidity, with 51% of deaths attributed to underlying sepsis (Greenhalgh et al., 2007; Krishnan et al., 2013). The wound rapidly becomes colonized by a number of pathogens immediately after injury due to the compromises in the barrier function of the skin. In addition, the bi-phasic immune response, acute hyperinflammation followed by immunosuppression, seen in burns leaves the patient unable to combat the infection. Also, the burn eschar provides an ideal environment that is rich in nutrients (i.e., denatured proteins and lipids) at an ideal temperature for microbial growth (Taneja et al., 2013). To prevent burn wound infection, topical antimicrobial agents have been the mainstay of therapy and include creams [e.g., bacitracin, mupirocin, and silver sulfadiazine (SSD)] and aqueous solutions (e.g., mafenide acetate aka Sulfamylon® and silver nitrate) (Palmieri and Greenhalgh, 2002; Storm-Versloot et al., 2010; Aziz et al., 2012; Heyneman et al., 2016; Afshari et al., 2017; Norman et al., 2017). In order to maintain their efficacy as prophylaxis for infection, these topical agents need to be applied at least daily, which can increase patient pain and interfere with wound healing. Compared to topical agents, silver-based dressings are advantageous as they require less frequent dressing changes due to the sustained release of silver ions to the wound bed. Additionally, clinical studies show that silver-based dressings minimize the incidence of infection, reduce eschar formation, and provide control of wound exudate (Aziz et al., 2012; Wasiak et al., 2013; Marx and Barillo, 2014; Lindberg et al., 2015; Munteanu et al., 2016). However, each topical therapy carries its own risk profile. SSD and silver-based wound dressings have been associated with delayed or incomplete re-epithelialization, generation of discolored scars, limited penetration of the burn eschar, hypersensitivity, neutropenia, and ineffectiveness against some pathogens (Hussain and Ferguson, 2006; Wang et al., 2009a,b). Additionally, silver-based dressings function only when moistened and are relatively expensive; although, these costs are partially mitigated by the need for less frequent dressing applications or changes. Nevertheless, further prospective, randomized control trials (RCT) are needed to determine the optimal wound dressing after burn injury (Storm-Versloot et al., 2010; Jull et al., 2015; Norman et al., 2017).
Due to prolonged hospitalizations after severe burn injury (Sarabahi et al., 2012), there has been a surge of fungal colonization and invasive infections which are linked to high mortality (Norbury et al., 2016; Sharma et al., 2016). Clinical guidelines recommend preventative measures by wound debridement and immediate autografting; otherwise, antifungal drugs such as the echinocandin drug caspofungin (Pappas et al., 2009), voriconazole, nystatin, or amphotericin B (Struck and Gille, 2013; Norbury et al., 2016) may be used. The increasing trend in fungal burn infections signifies an emergence of the next critical obstacle in burn care.
Surgical Management
Wound management of DPT and FT burn injuries is resource and labor intensive as it often requires multiple surgeries, repeated wound care, and can result in long hospital and intensive care unit stays (Sanchez et al., 2008). Burn wound management costs increase substantially with increasing TBSA primarily due to the length of hospital stay, generally estimated at 1 day per every 1% TBSA burned (Bessey et al., 2014; Kearns et al., 2014; Mathews et al., 2017). Clinically, burn wound management occurs during the acute phase (within days) of burn injury and involves tangential excision of necrotic tissue until punctate bleeding in the wound bed is visible, followed by immediate application of either a permanent autologous skin graft or temporary skin substitute. It is well accepted that early excision and immediate wound coverage help attenuate the inflammatory response of burn injury, decrease risk of infection, and lead to better healing outcomes. Unfortunately, burn wound excision is often accompanied by high amounts of blood loss and hypothermia, both of which limits the amount of tissue that can be excised per operation. Techniques to reduce blood loss include the topical use of thrombin spray, epinephrine soaked gauze, tumescence (subcutaneous infiltration of vasoconstrictors), fibrin sealants, extremity tourniquets, and cautery (Zuo et al., 2017). Complete hemostasis is necessary prior to application of a skin graft in order to prevent hematoma formation which could result in graft failure (Butts et al., 2017).
Permanent Wound Coverage
Autologous split-thickness skin grafts are harvested using a dermatome at a thickness of 0.008”−0.015” and consist of the epidermis and a small portion of the papillary dermis. They are the current SOC for permanent wound coverage for DPT and FT burns. Successful wound coverage with autograft necessitates sufficient donor site availability, which is an issue in larger TBSA burns. Due to the minimal amount of dermis in the autograft, the wounds typically heal with some degree of contraction (Bush and Gertzman, 2016). Meshing the autograft, which cuts slits to expand the skin, is routinely used from 1:1 for smaller burns to 6:1 for large TBSA burns. This process is performed when donor sites are limited in an effort to cover the wound with the minimal amount of required tissue (Finnerty et al., 2016). However, utilizing a higher meshed autograft increases the risk of infection, contraction, and scarring due to a longer time for complete re-epithelialization of the larger interstitial spaces (Finnerty et al., 2016). The hands, neck, and face are areas in which contraction creates dramatic quality of life issues, such as loss of function and poor cosmesis; therefore, unmeshed or sheet skin grafts are typically used to prevent these adverse outcomes. The thickness of the autograft inversely correlates with the amount of contraction that occurs (e.g., a thicker graft results in less contraction) (Carlsson et al., 2016); therefore, a FT graft also may be used in these areas to obtain the best functional and cosmetic outcome. However, utilization of a FT graft generates a secondary FT donor site wound which carries with it increased pain, greater risk of HTS, longer healing time, and its own need for wound closure (Stekelenburg et al., 2016).
Temporary Wound Coverage
Autografts provide the best permanent wound coverage and are always the clinician's first choice when available. However, large TBSA burns may not have autologous skin available due to a lack of donor sites. These cases require temporary wound coverage, such as coverage with fresh or cryopreserved allograft (see Table 7 for available allografts) or the use of a skin substitute until a donor site is ready for re-harvesting (see Table 8 for available substitutes). These temporary biological coverings protect the wound bed from desiccation, heat loss, microbial contamination, and promote the formation of granulation tissue (GT) favorable for autograft placement (Saffle, 2009). Allografts are available through tissue banks regulated by the American Association of Tissue Banks (AATB). Fresh allografts possess viable cells; however, the donor epidermis is not immune privileged and will ultimately be rejected due to the presence of class II antigens on the surface of the Langerhans cells. Since the dermis consists of mostly inert collagen, it can be incorporated into the GT of the wound bed, ultimately supporting a future autograft (Voigt et al., 2018). A wide variety of skin substitute products harvested either from cadaveric humans (allograft) or animals (xenograft) are available and consist of dermis and possibly even epidermis with the intent of replacing “like with like” (Tables 7, 8). Other than Epicel®, these substitutes are not autologous and do not provide permanent coverage of the wound but instead can provide temporary coverage with a benefit of augmenting the regeneration of the missing dermis. A few of these devices consist of an outer silicone layer to mimic a few of the epidermal functions such as preventing desiccation and bacterial contamination of the wound bed. A recent survey of 500 burn care specialists worldwide found that 51 and 28% frequently use allografts and xenografts, respectively, for temporary coverage of burn wounds with the intent of establishing an optimal wound bed to support wound closure with a meshed autograft (Wurzer et al., 2016). This two-stage method is commonly employed in which tangential excision and temporary coverage is performed during the first surgery and after a certain amount of time and improvement in the patient's condition, a skin graft is applied during a subsequent operation. The survey revealed that 61% of respondents use biological or synthetic materials in clinical practice but agreed that no ideal skin substitute exists that replaces all the characteristics of skin (Wurzer et al., 2016).
Hypertrophic Scar (HTS) Prevention
As stated above, if a wound has not healed in 3 weeks there is a high risk of developing HTS. Other risk factors of HTS formation include age (children), darker skin color (pigmentation), female gender, facial or neck injuries, and severity of injury (%TBSA and depth). Several studies report that from 32 to 72% of all burns result in HTS formation (Bombaro et al., 2003; Lawrence et al., 2012; Finnerty et al., 2016). Currently, no consensus exists as to the best method to prevent HTS formation. Commonly used techniques to mitigate HTS formation include massage therapy, moisturizers, pressure garments, silicone gel sheets, and exercise with varying results (Anthonissen et al., 2016). These methods are widely available, inexpensive, and low-risk but with controversial efficacy, as even large comprehensive reviews find only low quality evidence supporting their use in some cases (O'Brien and Jones, 2013). Nevertheless, these easy-to-use options have few adverse effects and remain a part of common clinical practice.
Hypertrophic Scar (HTS) Mitigation
Scar revision surgery remains the definitive method for managing HTS, particularly those with associated contractures. Surgeons employ many techniques to release the contracture with a complexity ranging from simple incision with a blade across the scar and application of skin substitute and/or autograft to local perforator flaps to more complex free flap reconstructions that may involve tissue expanders (Hudson and Renshaw, 2006; Hayashida and Akita, 2017). Other first-line treatments are also available that are less invasive. Intralesional steroid injection, for instance, inhibits fibroblast activity and alters transforming growth factor beta's (TGF-β1and TGF-β2) expression (Tziotzios et al., 2012). Similarly, intralesional 5-fluorouracil injections inhibit collagen production and fibroblast proliferation (de Waard et al., 1998), limiting the severity of HTS. These therapies are generally well tolerated with minimal side effects such as hypopigmentation or dermal atrophy. Cryotherapy is a safe office-based procedure often used in conjunction with or as a second-line treatment after steroid injection. Liquid nitrogen is carefully sprayed onto a scar, freezing and lysing underlying cells to alter fibrotic tissue, fibroblast activity, and collagen synthesis (Dalkowski et al., 2003), thereby reducing scar thickness. Lastly, clinicians are beginning to use a variety of medical lasers to treat HTS, which will be discussed later in section HTS Mitigation of the review. Many of these treatments are often combined over the course of a patient's care with synergistic results. Unfortunately, none of the available treatments have resulted in complete abatement of HTS.
FDA Regulations
Navigating the FDA system is complex and not a focus of this review but a basic understanding of the approval process is warranted since it impacts the development of future products. The FDA regulates most drugs, biologics, medical devices, and human cells and tissue products (HCT/P) by one of the following mechanisms with the goal of evaluating the safety and efficacy of a new product: (1) Investigational New Drug (IND) and New Drug Application (NDA) or Biological License Application (BLA), (2) 510(k) Submissions, (3) Investigational Device Exemption (IDE) and Premarket Approvals (PMA), (4) Humanitarian Device Exemptions (HDE), and (5) HCT/P. Table 1 explains each category, lists examples, outlines the steps during the approval process, and indicates if a clinical trial is required.
Drugs and biologics (i.e., stem cell therapies) take the longest to acquire approval that begins with pre-clinical animal studies followed by three phases of clinical trials, which are required components for an NDA or BLA submission to the FDA. This entire process can take 7–15 years and the latest estimated costs are ~$1B (Ciociola et al., 2014; Hung et al., 2017). Medical devices have 3 classes (I, II, and III) that correspond to increasing levels of risk for human use. Class I devices are regulated under general controls and are generally accepted as no risk to human life. Class II devices (i.e., some dressings and skin substitutes/acellular dermal matrices) require a 510(k) submission which is a review by the FDA to determine “substantial equivalence (SE).” This simply states that the new device has similar characteristics and intended use as a legally marketed device and is “cleared” for commercial distribution. It is noteworthy that most medical devices are “cleared” in this manner, which is not an actual “approval” of the device by the FDA and often does not require any human clinical data, with ~3,000–4,000 devices 510 (k) “cleared” annually (www.FDA.gov). Class III devices support or sustain human life thus must obtain a PMA after progressing through clinical trials to establish the device's safety. The HDE designation is for specialty diseases that affect a limited number of individuals (8,000) every year. The exemption is accompanied by very strict guidelines with the intent on getting these products to market in a quicker fashion. HCT/P products (i.e., allografts) are available through tissue banks much like blood through blood banks. The tissue banks must adhere to standards set forth by the FDA and the products must be screened and verified that no viral, such as human HIV and hepatitis, or bacterial contaminants are present (FDA, 2017, 2018a,b,c). In the following sections, we have listed products used in burn care that have been granted FDA approval via one of these mechanisms. The intention is to bring to light the plethora of available products with either a 510 (k) or HCT/P approval (Tables 5, 7, 8) but in some cases no thorough clinical evaluation has been performed showing true efficacy.
Wound Healing Cascade
When skin is injured as a result of trauma, surgery, or burn, it is considered an acute wound. Wound healing is an orchestrated sequence of events involving chemical signals, extracellular matrix (ECM) molecules, and a wide range of cell types. Acute wound healing follows a complex, overlapping cascade of events consisting of hemostasis, inflammation, cell proliferation, and matrix remodeling of the wound site (Lazarus et al., 1994). Table 2 lists the endogenous cells that are involved in this healing process and indicates what they secrete, the cells that those cytokines and growth factors target, and the subsequent cellular response. Each of these stages of wound healing are potential targets for tissue engineering and regenerative medicine (TERM) and regenerative pharmacological strategies.
TERM and Regenerative Pharmacology
As defined by the National Institute of Health, tissue engineered (TE) refers to “the practice of combining scaffolds, cells, and biologically active molecules into functional tissue….that restore, maintain, or improve damaged tissue or whole organs” (National Institute of Health, 2018). As with other target tissue, TE scaffolds for skin substitutes aim to be biomimetic through the use of native ECM proteins (e.g. collagen, elastin, hyaluronan, fibrin, fibronectin, and chondroitin sulfate) and cells (e.g. keratinocytes and fibroblasts). Regenerative pharmacology is “the application of pharmacological sciences to accelerate, optimize, and characterize (either in vitro or in vivo) the development, maturation, and function of bioengineered and regenerating tissues” (Christ et al., 2013). It can be used to accelerate wound healing through the delivery of pro-regenerative molecules such as immunomodulators, growth factors, gene therapy, and cell secretomes that can be delivered alone or by a TE construct. Together, TE and regenerative pharmacology fall under the broader umbrella of regenerative medicine, with the goal in which “the body uses its own systems, or sometimes help with foreign biological material to recreate cells and rebuild tissues and organs” (National Institute of Health, 2018). The following sections will indicate how current TERM techniques are being utilized throughout the phases of wound healing.
Hemostasis
Hemostasis is achieved by platelet accumulation at the site of injury and formation of a fibrin laden blood clot. Growth factors are released from the platelets after thrombin induced degranulation: platelet-derived growth factor (PDGF-α/β), TGF-α/β, and epidermal growth factor (EGF), which are trapped in the blood clot and recruit other cell types for wound repair (Werner and Grose, 2003). During burn surgery, significant amounts of blood loss occur during debridement and excision, estimated at ~200 ml/% TBSA that is tangentially excised (Allorto et al., 2015; Zuo et al., 2017). This presents a significant challenge for large TBSA wounds requiring debridement. For instance, a 50% TBSA patient could lose an estimated 5–10 liters of blood during surgery, thereby exceeding the blood volume of an adult and requiring replacement by transfusion (Zuo et al., 2017). The resulting massive transfusions can lead to a variety of complications such as hemorrhagic shock, infection, acute lung injury, multi-organ dysfunction, and even an increase in mortality (Sterling and Heimbach, 2011).
Hemostatic Adjuncts
A variety of topical hemostatic adjuncts are FDA approved to limit the amount of intraoperative blood loss that occurs during the excision surgery (Table 3) and have been recently reviewed (Sterling and Heimbach, 2011; Shander et al., 2014). In this section, therapies currently in clinical trials or that have recently completed a clinical trial will be discussed (Table 4).
Epinephrine, a non-selective agonist of adrenergic receptors which activates α1, α2, β1, and β2 receptors, is part of the SOC and applied as a dilute solution in epi-soaked gauze but also infiltrated under the burn and donor site during the tumescent process. Phenylephrine, a selective agonist of adrenergic receptors which only activates α1 receptor, is being investigated as an alternative to epinephrine as a tumescent solution on the hypothesis of equal efficacy without the systemic side effects due to a lack of α2 and β-adrenergic activity. A recent phase 0 concentration finding study was completed that found vasoconstriction was achieved at a concentration of 5 ug/ml on donor sites in 6 burn patients (Mitchell et al., 2011). A phase 1 RCT is currently underway testing phenylephrine instead of epinephrine for tumescent infiltration of the injured site to decrease blood loss during tangential excision.
A new hemostatic hemafiber dressing, NuStat®, was recently cleared by the FDA that consists of a mixture of bamboo cellulose and continuous filament silica. This unique combination promotes hemostasis chemically by activating the coagulation cascade but also mechanically by compression. In a single institution RCT of burn patients requiring tangential excision, NuStat® was compared to the SOC administration of thrombin and epinephrine-soaked non-adherent dressings. Each patient was their own control with both therapies applied on roughly half of the burn and donor site. No statistically significant differences were observed in the amount of blood loss from either site indicating comparative efficacy to the SOC. Benefits of NuStat® reported were lower cost and ease of application due to no reagent preparation vs. the SOC (Butts et al., 2017).
TT-173 (Thrombotargets, Spain) is a new hemostatic agent that has been developed to modulate the coagulation pathway to induce clotting. It consists of a lipid microvesicle with a modified version of recombinant human tissue factor that is lyophilized and applied as a spray. A phase 2 RCT of 78 patients was recently completed evaluating this product's ability to reduce donor site bleeding duration. TT-173 was shown to stop bleeding faster than placebo. No adverse events were observed and donor sites healed as expected. Other benefits of TT-173 reported were reduced cost compared to fibrin sealants, ease of manufacturing, and no human or animal components which decreases the risk of pathogen transmission (Rojas et al., 2017).
Enzymatic debridement is an alternative debridement method that digests the proteins present in the necrotic tissue of the wound bed. NexoBrid® consists of a group of Bromelain enzymes that are extracted from the fruit and stems of pineapples. This product is currently approved in Europe and has been reported to selectively digest the necrotic tissue and work in as little as 4 h after application. Interestingly and more relevant to this section of the review, an additional benefit is a decrease in intraoperative blood loss, with reports of higher hemoglobin and hematocrit levels in patients treated with NexoBrid® vs. SOC (Rosenberg et al., 2015). A recent European consensus was published that had unanimous responses from surveyed clinicians stating enzymatic debridement with NexoBrid® reduced blood loss compared to SOC (Hirche et al., 2017). Seven clinical trials testing the safety and efficacy of this product have been completed with a US based multicenter RCT currently recruiting burn patients to demonstrate complete eschar removal and reduction in patients' surgical burden and its related blood loss as compared to SOC without long term cosmetic and functional issues (Rosenberg et al., 2014, 2015).
Recent clinical research evaluated the use of rThrombin (already FDA approved, ZymoGenetics, Inc.) as a plasma-free alternative produced from mammalian cells for use during burn surgeries. The initial study demonstrated efficacy in achieving hemostasis with 91.5% of patients attaining it in 20 min and safety with only 1.6% of the patients developing antibodies to the rThrombin (Greenhalgh et al., 2009). In a follow up study in 30 pediatric burn patients, topical rThrombin was applied as a hemostatic agent on day 1 and demonstrated no anti-rThrombin antibody production at day 29 (Foster et al., 2011).
Given the fact that these potential therapies are considered drugs or biologics, there is a long regulatory approval required before these newer TERM strategies can be implemented as SOC.
Inflammation
Inflammation, the second phase of wound healing, follows a general pattern of cellular infiltration following injury. Polymorphonuclear leukocytes (PMNs) (Table 2), a category of white blood cells which includes basophils, eosinophils, and neutrophils, infiltrate the wound within the first hours after injury and continue to do so for up to a week (Singer and Clark, 1999). These cells produce large quantities of reactive oxygen species and are responsible for ingestion and clearance of necrotic tissue and pathogens in the wound bed. Migration of PMNs to the wound is followed by macrophage infiltration within 1–2 days. Macrophages and Langerhans cells, dendritic cells (DCs) resident in the epidermis, are antigen presenting cells that are responsible for presenting antigens to T-cells in order to elicit an immune response. Macrophages are also responsible for producing nitric oxide (NO), an important regulator of collagen synthesis and angiogenesis, as well as many chemokines and cytokines such as Prostaglandin E2 (PGE2) and TGF-β, which induces cell proliferation and migration (Franz et al., 2007). Lastly, macrophages are essential for the initiation and propagation of new tissue formation at the wound site and facilitate the transition to the cell proliferation phase.
Thermal injury is associated with altered systemic immune function while the wound exhibits perturbed patterns of immune cell infiltration due to alterations in tissue permeability and lack of functioning vasculature in areas of coagulation (Rose and Chan, 2016). The precise alterations of immune cell infiltration in burns are not fully understood. Using murine models of flame and scald burns, Tschöp et al. demonstrated depletion of T-cells as well as decreases in the production of interferon gamma (IFN-γ) in the more severe burns, which contributed to immunosuppression by reducing the activity of the adaptive immune response. Eight days after burn, the observed immunosuppression was replaced by a predominance of a hyperinflammatory macrophage phenotype, as well as a three-fold increase in the number of IFN-γ producing T-cells. This suggests that increasing severity in burn correlates with both depressed innate and adaptive immune function (Tschöp et al., 2009). Another murine study of small TBSA (6%) burns was associated with fewer PMNs, as well as a reduced PMN respiratory burst (Calum et al., 2009).
Immunomodulation
It is well known that immune competence is vital to proper wound healing and immune cells play a major role in combating wound infection. They also have deleterious effects if their activity in the wound microenvironment delays or prevents healing, thus yielding a chronic wound (Szpaderska and DiPietro, 2005; Franz et al., 2007; Yan et al., 2012) with continued cell proliferation and scarring (Rosique et al., 2015). As such, manipulation of the immune system (e.g., immunomodulation), both systemically and locally to enhance healing while also avoiding infection is a tempting target, it must be approached with caution.
PMNs represent an early target for cellular immune modulation as they are present in the wound immediately after injury. Application of Biafine, a topical, trolamine-containing oil-in-water emulsion, to rat burn wounds was associated with improved healing outcomes through a reduced number of neutrophils and increased macrophage numbers. The authors hypothesized that this resulted in a significant increase in the production of NO in the burn wound microenvironment, thereby increasing the rate of cell proliferation and collagen deposition (Krausz et al., 2015). Another attractive target for immunomodulation is DCs. Vinish et al. were able to control the rate of wound closure through the transient depletion or enhancement of DCs in a murine model. Depletion of DCs with Diphtheria Toxin prior to burn delayed early wound closure and formation of GT, while lowering levels of TGF-β1 and CD31+ blood vessels. Conversely, enhancing DC numbers with recombinant fms-like tyrosine kinase-3 ligand (Flt-3), resulted in early wound closure, increased TGF-β1, and increased vascularization in the burn wound area, without excessive deposition of collagen (Vinish et al., 2016). Based upon these findings, stimulating TGF-β1 production appears to be a target for immunomodulatory therapy, but the timing of such therapy is critical. TGF-β1 induces inflammation early in the wound healing phase, leading to a self-limiting recruitment of immune cells, followed by cell proliferation, and re-epithelialization. However, once the wound has progressed to the remodeling phase, high TGF-β1 is associated with increased scar formation (Han et al., 2012; Gilbert et al., 2016).
Non-steroidal anti-inflammatory drugs (NSAIDs) and cyclooxygenase-2 (COX-2) inhibitors have been investigated as a method to attenuate the inflammatory response (Szpaderska and DiPietro, 2005); however, it appears that route of administration may drastically affect wound healing outcomes. When administered systemically, COX-2 inhibitors were shown to reduce epithelial cell proliferation and deposition of ECM and collagen which delayed wound healing (Fairweather et al., 2015). While there have been publications on the use of COX-2 inhibitors in both animal models and clinical studies, it is difficult to say whether systemic or local NSAID therapy would be beneficial to burn wound outcomes. In vivo rodent models utilizing NSAID therapy focused on survival following burn infection and sepsis (Shoup et al., 1998; Schwacha et al., 2002), while most rodent studies and human clinical studies investigating NSAIDs generally focus on pain alleviation and reduction of systemic inflammation following COX-2 inhibition (Chong et al., 2014; Rose and Chan, 2016).
Topical Therapeutics for Acute Bacterial Infection
Along with increasing rates of antibiotic resistance, the inability of systemic antibiotics to perfuse the compromised vasculature of burn wounds and penetrate the infected eschar has resulted in decades of research on novel agents and topical treatments. Comprehensive reviews of topical antimicrobial treatments for burn wounds have been recently published (Dai et al., 2010; Sevgi et al., 2013; Cartotto, 2017; Norman et al., 2017). Topical delivery of antibiotics directly to the site of injury is not a novel concept with many products already available on the market (Table 5). Topical antibiotic creams and ointments, antimicrobial impregnated dressings, and silver-based therapeutics dominate infected burn pre-clinical porcine models and clinical research (Table 6). Current research focuses on sustained delivery while maintaining bioactivity in order to reduce dressing changes, in turn reducing patient pain and burden on providers. Sustained delivery of antibiotics can be achieved by encapsulation into different hydrogel-based systems such as gelatin (Nunes et al., 2016), keratin (Roy et al., 2016), or chitosan (Hurler et al., 2012). Antibiotic incorporation into electrospun dressings (Chen et al., 2016; Dhand et al., 2016, 2017) and occlusive dressings (Steinstraesser et al., 2011) has also shown superior activity and accelerated wound healing when compared to current clinical silver-based products.
Natural antimicrobial products are once again taking the forefront of antimicrobial therapies (Newman and Cragg, 2012; Bitter and Erickson, 2016) due to their wide availability and inexpensiveness compared to current SOC. For instance, medicinal honey-based therapeutics are currently being investigated due to their antimicrobial and wound healing properties. In addition, overall patient satisfaction is reported to be higher when using medicinal honey compared to SSD (Nasir et al., 2010; Aziz and Abdul Rasool Hassan, 2017). There are a number of different varieties of honey based on the plant-derived active ingredients, but the most well-known is sourced from the Manuka tree in New Zealand (Carter et al., 2016; Duncan et al., 2016). In a RCT of 150 patients, two similar burn injuries were chosen on different parts of the patient's body and randomized to treatment with honey and the other with SSD. Honey accelerated re-epithelialization and had a lower infection rate compared to SSD (Malik et al., 2010). Another potential natural therapy uses medicinal herbs such as Centella asiatica incorporated into topical ointments (Centiderm®). The active triterpene glycosides within Centiderm® transforms by hydrolysis into asiatic acid, which has shown to reduce the incidence of wound infections. In a recent clinical study, no infections were observed in the Centiderm®-treated group of 40 burn patients while 4 of 35 patients in the SSD group developed infections at the treatment site (Saeidinia et al., 2017). In addition, topical oak bark ointment successfully reduced the quantity of Methicillin-resistant Staphylococcus aureus (MRSA) pathogens in a mature infection when applied twice daily when compared to SSD (Davis and Mertz, 2008).
The antimicrobial properties of silver-based dressings have been utilized for a number of FDA approved burn dressings. Unfortunately, a number of adverse outcomes are observed when using silver-based dressings such as delayed or incomplete re-epithelialization, scar discoloration, and hypersensitivity (Hussain and Ferguson, 2006; Wang et al., 2009a). For this reason, novel delivery systems for silver-based therapeutics have been developed including silver-loaded hydrogels (Boonkaew et al., 2014), which have found success in clinical applications (Glat et al., 2009). Even though there was no difference in infection rate between the silver-loaded hydrogel and SSD, there was a decrease in patient pain during dressing changes (Glat et al., 2009). In one report, a hydrofiber dressing coated with ionic silver reduced the incidence of burn wound infections, reduced pain, and accelerated wound closure when compared to SSD. The hydrofiber dressing was changed every 3 days unlike SSD cream which requires daily dressing changes (Muangman et al., 2010). Due to the limitations of silver-based products, a number of alternative metals with antimicrobial properties, such as copper and gallium, are currently being investigated as well (Sevgi et al., 2013).
Antimicrobial peptides (AMPs) are commonly cationic and have broad-spectrum antimicrobial activity, targeting bacterial cell membranes and disintegrating their lipid bilayer structure (Mahlapuu et al., 2016). Even though AMPs are currently not in clinical trials for burns, they have therapeutic potential in a range of infections, including those producing biofilms (Findlay et al., 2016; Ma et al., 2017). Topical application of epinecidin-1 to MRSA contaminated porcine burn wounds prevented infection, sepsis, and delayed wound healing (Huang et al., 2017). The treatment was administered 6 h after wound inoculation; therefore, further testing within a mature infection would highlight the potential of this modality as a therapy in addition to being a preventative measure.
Instead of focusing on antimicrobials to combat infectious microorganisms, current research also investigates the ability of predatory and probiotic bacteria to suppress colonization of pathogenic bacteria such as Pseudomonas (Kadouri et al., 2013). Recently, a clinical trial of 80 burned patients were treated with the probiotic bacteriotherapy through topical application of the Lactobacillus genus. When compared to SSD treatment, the probiotic decreased overall infection rates and promoted GT deposition (Peral et al., 2009). As we continue our understanding of the burn wound microbiome and the events to why some contaminations develop to be invasive infections, novel bacteriotherapies will arise.
The biofilm of a wound, much like an eschar, often prevents antimicrobial agents from reaching the wound bed (Phillips et al., 2015). For this reason, biofilm disrupting agents (synthetic and natural) are an emerging class of therapeutics used to penetrate and destabilize the biofilm microenvironment, leaving pathogens vulnerable to antimicrobial activity. Aryl-alkyl-lysines are small molecules that have been successful against both planktonic as well as the mature biofilm of an Acinetobacter baumannii burn wound infection (Ghosh et al., 2016). Also, a formulated garlic ointment has been capable of preventing biofilm development as well as disruption of immature biofilms with a spectrum of activity against many common burn pathogens (Nidadavolu et al., 2012).
Topical Therapeutics for Acute Fungal Infection
The increased prevalence of fungal wound infections has spurred research and development of novel antifungal treatments. Candida albicans is the most common fungus to infect burn wounds and represents the major target of current pre-clinical research. Silver-coated dressings and Nystatin have proven to be effective treatments (Acar et al., 2011). Electrospun SSD-containing nanofiber dressings were also shown to be effective against C. albicans infected burn eschar (Ciloglu et al., 2014). In many burn centers, systemic administration of Amphotericin B is used when invasive fungal infection is suspected; however, systemic administration of Amphotericin B is associated with a dose-dependent nephrotoxic effect (Hamill, 2013). This has led to the development of topically applied Amphotericin B encapsulated in polyethylene glycol and chitosan. Sustained release of Amphotericin B from the nanoparticle was able to clear fungal infections while having no adverse effect on wound healing (Sanchez et al., 2014). Even though topical application of Amphotericin B reduces the overall dose, Amphotericin B release from a carrier to the blood stream could potentially result in systemic dispersion and toxicity. For this reason, topical therapeutics using Amphotericin B must be evaluated for any signs of nephrotoxic effects.
The current approved therapies from the management of infection (Table 5) consists of either drugs that required a clinical trial or 510 (k) approved dressings that contain a well know antimicrobial (PHMB or silver). In current clinical trials are a wide range of new TERM strategies that are being explored that will require similar approval mechanisms (Table 6). What is still missing is comprehensive RCT of these agents comparing efficacy to each other (i.e., the silver dressings).
Cell Proliferation
Wound closure, generally accepted as complete re-epithelialization, is the purpose of the cell proliferation phase. Re-establishing the epidermal layer is paramount in regenerating the protective barrier of skin, preventing infection, and limiting fluid loss. Within days of injury, fibroblasts migrate into the wound and deposit large amounts of ECM consisting first of relatively disorganized type III collagen with wound collagen content reaching its peak 2–3 weeks after injury. These fibroblasts often differentiate into myofibroblasts which possess a contractile phenotype and are easy to identify due to their expression of α-SMA (alpha smooth muscle actin). New blood vessels are formed by invading endothelial cells throughout the ECM to supply nutrients to the newly forming GT. As the wound fills in with GT, keratinocytes at the wound edges migrate and proliferate over the top of the wound until wound closure takes place (Werner and Grose, 2003).
In uninjured skin, the basal epithelial cells self-renew and constantly differentiate into the epidermis every 2–3 weeks. The epidermis also contains endogenous stem cells that respond immediately to an injury and start the self-repair process. Without the basal epithelial cells in the epidermis, the hair follicle stem cells (HFSCs) that reside at the base of the hair follicle act as foci for re-epithelialization. Hair follicles extend through the entire depth of the dermis and some viable HFSCs are present even in DPT wounds. In the event of a PT or deeper burn, the entire epidermis is lacking and thus an epidermal replacement is needed. For FT burns, the entire dermis has also been lost and needs to be replaced; otherwise, the resulting quality of life may be impacted by contractures and scarring (Singer and Clark, 1999; Werner and Grose, 2003; Diegelmann and Evans, 2004).
Burn Wound Coverings
According to a panel of experts from American Burn Association, the burn wound coverings are classified under two broad categories, Skin replacement: defined as a tissue or graft that permanently replaces lost skin with healthy skin, and Skin Substitute: defined as a biomaterial, engineered tissue, or combination of materials and cells or tissues that can be substituted for skin autograft or allograft in a clinical procedure (Kagan et al., 2013). Currently available burn wound products fall under either of the above mentioned class of wound dressing categories. The burn wound coverings can be further divided into temporary biological coverings, epidermal, dermal or complete skin substitutes (Tables 7, 8).
Temporary Biological Coverings
Allografts are used as temporary biological coverings which serve as lifesaving treatments for patients with extensive burns and limited donor skin (Brown et al., 1953; Zuo et al., 2017). These coverings are utilized to provide barrier function to prevent bacterial infection and provide thermoregulation (Mohammadi et al., 2013). With advancements in preservation processes, human allografts (derived from amniotic membrane (AM) or skin) can be stored and banked sterilely, either with viable cells or as a decellularized product with a plethora of human -based products commercially available for burn wound coverage (Table 7). Human AM is considered an effective biological material due to its unique composition of substrate proteins, specifically collagen IV, laminin, integrin, and proteoglycans, and it has been proposed to benefit burn wound healing specifically (Kesting et al., 2008; Glat and Davenport, 2017; Mowry et al., 2017; Tenenhaus, 2017). Fresh AM demonstrated higher graft take compared to autograft on burn wounds (Mohammadi et al., 2013) while glycerol preserved or air-dried AM reduced the time to re-epithelialize on autograft donor sites (Zidan et al., 2015) and burn wounds (Singh and Chacharkar, 2011), respectively. A recent review shows a series of case studies using AM allograft, to treat PT and FT burns in various anatomical locations to promote wound re-epithelialization and vascular angiogenesis (Reilly et al., 2017). Current clinical trials further characterize donated amnion as a skin substitute for burn patients (Table 9); though the results are not posted, many anticipate AM will prove to be a safe and efficacious temporary biological covering.
Epidermal Substitutes
Epithelial cells applied either as sheets or as a spray represent the most common epidermal substitutes. Autologous epithelial cells obtained from a small biopsy of a patients' own skin have been successfully grown on a mouse irradiated fibroblast feeder layer and used to treat large TBSA burn injury. These sheets of cells are known as cultured epithelial autografts (CEA). The applicability of CEA to burn wounds was widely recognized following the introduction of Epicel™ in 1988. Since then, many studies show the benefits of CEA in providing coverage to extensive burn wounds (Wood et al., 2006; Sood et al., 2010; Cirodde et al., 2011). CEA success in the literature is variable, largely due to their delicate nature (only 7–10 cell layers of keratinocytes), need for an uninfected wound bed, expansion time for cells, and issues with transfer of the graft. To address these limitations, production of CEA on a chemically defined surface for easy and quick transfer to the wound bed has been developed (Myers et al., 1997; Wright et al., 1998; Horch et al., 2000; Hernon et al., 2006). Clinical usage of CEA with (1:6) widely meshed autografts demonstrated similar healing outcomes to (1:3) meshed autografts (Akita et al., 2018).
Similarly, suspensions of autologous cells (including epidermal progenitors and basal epithelial cells) applied with a spray device produce acceptable clinical outcomes (Yim et al., 2011; Esteban-Vives et al., 2016). A newer technology, “ReCell®,” which is an epithelial spray preparation device, has gained popularity applying autologous cells to the wound bed (Table 9). Using this device, epithelial cells can be isolated from a small biopsy of the patients' own skin and sprayed directly on the burn wound after excision or applied along with meshed autograft (Gravante et al., 2007; McHeik et al., 2014).
Dermal Substitutes
CEA and suspended epidermal cell technologies lack a vital component of skin—the dermis. Artificial dermal products were designed to significantly reduce the time needed to achieve final wound closure in the treatment of major burn wounds. This process typically requires a two-stage method with the first to apply the skin substitute in order to create a wound bed of GT, and the second to apply an autologous graft on the neodermis. One such clinical study used Integra® after early excision of burned tissue and was allowed to integrate. After 3 weeks, CEA was applied on top of the resulting wound bed. The reconstructed skin was durable with no signs of dehiscence (Matsumura et al., 2013). Recently, treatment of complex FT soft tissue injuries with Integra® combined with ReCell® reduced donor site skin requirements, permitted wider meshed autografts, and reduced time to complete healing (Hammer et al., 2017). Use of Integra® seeded with adipose tissue derived stem cells (ASCs) in porcine FT burns enhanced wound angiogenesis, blood vessel maturation, and matrix remodeling compared to Integra® without cells (Foubert et al., 2015). Currently, Integra® is being investigated as an adjunct to a meshed autograft in a single-stage surgery (Table 9). Newer techniques to incorporate stem cells or macromolecules, such as tropoelastin (Wang et al., 2015), may improve the efficiency of Integra®.
Parallel to Integra®'s development, Biobrane® was developed as a bilaminate membrane with an ultrathin layer of silicone rubber mechanically bonded to a knitted nylon fabric outer layer and porcine type I collagen inner layer into which GT grows (Frank et al., 1984; Yang et al., 1989). A recent retrospective study showed that application of Biobrane® maintains a healthy wound bed after burn excision and prior to grafting (Tan et al., 2015). Furthermore, Biobrane®, has been shown to decrease pain and hospitalization in PT burns (Lal et al., 2000). A recent study comparing Biobrane® to allograft for temporary coverage determined Biobrane® to have a lower cost and significantly reduced procedure time (Austin et al., 2015).
Many other synthetic dermal substitutes were introduced following the success of Integra® and Biobrane® (Table 8). In order to closely mimic the structural architecture and retain the biomolecular composition of dermis, specifically collagen, attempts were made to use completely decellularized skin tissue. The process of decellularization isolates the ECM scaffold of a tissue by chemically removing cells, yielding less immunogenic substrates for tissue regeneration (Chen et al., 2004; Gilbert et al., 2006). Many xenograft dermal substitutes are produced via such a process from animal skin (porcine, ovine, or bovine) and are indicated to treat PT burn injuries (Table 8). Apart from biological and biosynthetic dermal matrices, completely synthetic dressings like Suprathel®, were developed to cover burn wounds and has demonstrated equal efficacy as Biobrane® when applied over PT burns (Rahmanian-Schwarz et al., 2011). The concept of using dermal substrates to grow and deliver fibroblast was long realized when TransCyte™ (formerly marketed as Dermagraft-Transitional Covering) was introduced (Noordenbos et al., 1999). A prospective RCT using Dermagraft-TC™/TransCyte™ in patients with PT burns demonstrated faster re-epithelialization and fewer dressing changes compared to patients treated with Biobrane® or Silvadene® (Kumar et al., 2004).
The next generation skin graft may currently be in development in the form of genetically modified porcine skin [α-1, 3-galactosyltransferase knockout (GalT-KO)] which could significantly ease the availability of clinically acceptable xenografts (Leto Barone et al., 2015). The GalT-KO xenografts are tolerated similarly to the fresh or cryopreserved allografts (Leonard et al., 2017). If proven safe to be applied clinically, the cost may be considerably reduced and immediate availability of off-the-shelf xenograft for burn victims can be expected.
Complete Substitutes
To date, very few epidermal-dermal “complete” substitutes have been investigated. One example is Apligraf®, which is bovine type I collagen populated with neonatal fibroblasts and seeded by living human keratinocytes. In a multicenter RCT of 40 burn patients, Apligraf® placed over meshed autograft improved cosmetic and functional outcomes (Waymack et al., 2000). Another bilayer device, OrCel™, was introduced soon after Apligraf® to treat donor sites, where it accelerated healing and reduced scarring (Still et al., 2003). It is noteworthy, that despite allogeneic and synthetic origin of Apligraf®, OrCel™, and Dermagraft-TC™, rejection has not been an issue.
StrataGraft®, currently in clinical trials, is produced using NIKS® cells (human keratinocyte progenitor cell line), and is a viable FT product developed for treatment of severe burns after excision. StrataGraft® skin tissue consists of a stratified epidermal layer with a fibroblast laden collagen dermal component, is less fragile than CEA, and can be sutured, stapled or secured with an adhesive and remains intact on the wound bed, providing the critical barrier function during wound healing (Centanni et al., 2011; Table 9).
Cultured skin substitutes (CSS) contain collagen-glycosaminoglycan substrates with autologous fibroblasts and keratinocytes, and they are currently under clinical investigation as autologous engineered skin substitutes (ESS) (Table 9). CSS are proposed to provide permanent replacement of both dermal and epidermal layers in a single grafting procedure with similar mechanical properties as skin (Boyce et al., 2006; Sander et al., 2014). A recent RCT using CSS indicated that autologous ESS reduces mortality and requirements for donor skin to cover FT burns of greater than 50% TBSA (Boyce et al., 2017). Like CEA, though, CSS requires long culture times before application. In addition, CSS does not meet HCT/P designation because the cells are more than minimally manipulated, so no FDA approved indications exist presently (Table 1).
Use of dextran and fibrin hydrogels are currently being pre-clinically investigated as options to treat PT or FT burn wounds (Shen et al., 2015; Burmeister et al., 2018a). Bio-printing technology can develop three dimensional skin substitutes customized to individual patients (Ng et al., 2016). Still, the bio-printing process of skin requires autologous cells, a limitation in large TBSA burns. Despite successful skin substitute use in some arenas, further research into novel products is ongoing.
Stem Cells
Among the variety of available stem cells (Table 2), mesenchymal stem cells (MSCs) have multipotent potential and are easy to isolate, leading to their widespread adoption in wound healing literature. Recent reviews specifically address the key role of MSCs in burn wounds (Cheng et al., 2017; Maranda et al., 2017). Issues were identified that complicate comparing the efficacy of MSCs include the potential immune response, isolation procedures, culturing conditions, validation of differentiation potential, required therapeutic dose of cells, methods to deliver the cells, and the long-term viability of the cells.
Regardless of wound type, MSCs secrete anti-inflammatory factors such as IL-10 and tumor necrosis factor inducible gene siRNA6 (TSG-6) (Ennis et al., 2013). Recent studies show MSCs from different sources [bone marrow (BMSCs), ASCs, umbilical cord, and Wharton jelly] all reduced macrophage secreted pro-inflammatory cytokines IL-1α, IL-6, and IL-8 via PGE2 which potentially in burn wounds resolve the inflammatory phase (Najar et al., 2010; Yañez et al., 2010; Jin et al., 2013). In addition, it is worth remembering that elevated levels of NF-κB following tissue injury stimulates the secretion of PGE2 by MSCs which in turn significantly reduces the inflammatory cytokine surge following a burn injury.
BMSCs respond to the host chemokines: CXCR12/CXCR4, angiopoietin 1 (Ang-1), tyrosine kinase receptor, PDGF-β, and Tie-2 to facilitate MSCs-host endothelial cell interactions and wound vascularization (Lozito and Tuan, 2011; Hu et al., 2013). Further, BMSCs increase the stability of newly formed blood vessels by inhibiting high levels of exogenous matrix metalloproteinase 2 and 9 via tissue inhibitors of MMP-1 and−2 secretion (Kachgal and Putnam, 2011). Furthermore, BMSCs injected near the site of a burn wound differentiate into multiple skin cell types including keratinocytes, endothelial cells, pericytes, and monocytes. The BMSCs were traceable up to 3 months post-injury but not in 120 day mature scars, suggesting BMSCs play a role in wound healing and remodeling, but contribute less to long-term homeostasis, specifically scarring (Rea et al., 2009).
There is interest in purposing surgically discarded adipose tissue as immediate bed-side treatments. DPT and FT burn wounds treated with processed tissue improved GT formation, increased vascular endothelial growth factor (VEGF) levels at the wound site, and improved tissue re-vascularization (Atalay et al., 2014). Similarly, culture expanded ASCs implanted in the wound site responded to stromal derived factor one (SDF-1) and home to the perivascular space of nearby host blood vessels by binding to CXCR4 and CXCR7 (Stuermer et al., 2015; Kosaraju et al., 2016). To this end, a growing body of research has led to the discovery of MSCs from several different anatomical locations with each warranting further investigation into their wound healing potentials.
An alternative approach to delivering stem cells to the wounds involves collecting the products secreted from them and then applying this “secretome.” This secretome consists of extracellular vesicles, growth factors, and other proteins. The secretome profile of MSCs reveals that they express an array of pro-regenerative factors (Kilroy et al., 2007; Prockop and Oh, 2012; Phinney and Pittenger, 2017). Extracellular vesicles including exosomes and macrovesicles contain mRNA, microRNA (miRNA), and proteins which can be transferred between cells to regulate cell-to-cell communication, signaling, and altering cell or tissue metabolism. These molecules influence the response to injury and infection thereby highlighting their potential as therapeutics after burn injury (Levin and Sukhareva, 2016; O'Dea et al., 2016). In particular, MSC-derived exosomes reduced burn induced inflammation (Li et al., 2016). In a separate study, the paracrine factors from irradiated blood cells were collected. The secretome was then loaded into a commercially-available hydrogel and applied to a burn which was then covered with an autograft. Interestingly, the secretome from the irradiated cells led to improved angiogenesis in the wound when compared to the secretome from healthy cells (Hacker et al., 2016).
MSCs reduce HTS formation via constitutive paracrine effects of anti-inflammatory (PGE2) and anti-fibrotic factors, including hepatocyte growth factor, basic fibroblast growth factor (bFGF), and VEGF (Fang et al., 2016). Synergistically, these cytokines down-regulate expression of TGF-β1 and collagen (I and III) by fibroblasts (Zhang et al., 2006). MSCs have shown to improve long term scar outcomes when utilized early after burn injury; however, the use of stem cells after scar formation, specifically after a burn injury, still has to be explored.
A small number of trials have been conducted in the United States that utilize stem cells in the acute phase after burn with the main goal of establishing the safety of this treatment modality of which a phase 1, interventional clinical study is currently under investigation to determine if MSC treatment will improve healing and scarring of PT burns (Table 10). Thus far, most of the studies investigating the use of MSCs either applied them topically onto the wound or by injection at the wound site. New devices (e.g., hydrogels, nano-/micro-particles, nanofibers, ECMs, spheroids, and synthetic scaffolds) can deliver MSCs to maximize their potential at accelerating wound healing (Steffens et al., 2014; Chung et al., 2016).
According to the FDA, the use of homologous stem cells for skin regeneration—where donor cells or tissues match recipient cells or tissues and perform the same basic function(s)—is regulated separately from non-homologous cells or tissues. These non-homologous treatments are not regulated by the HCT/P exemption and instead are considered a “biologic,” requiring an IND, clinical trials showing both safety and efficacy, and BLA approval (Table 1) (FDA, 2017). With the implementation of the World Health Organization (WHO) mandate “WHO Guiding Principles on Transplantation,” it is expected that a global consensus on standard manufacturing protocols will be achieved for stem cells and future clinical trials will be performed with well-characterized cells under standardized conditions (http://www.who.int/transplantation/en/).
Growth Factors and Gene Therapy
To improve wound healing in burns, studies have investigated the application of growth factors derived from allogeneic sources, recombinant yeast, or bacteria. Treatment with these factors may occur alongside SOC (e.g., a skin graft). The existing body of literature on growth factor application to treat burn wounds is variable, though, with different burn pathologies and pre-clinical models studied. In contrast, a great deal of literature exists regarding the application of growth factors in other types of wounds that could be extrapolated to the burn wound (Moura et al., 2013; Picard et al., 2015; Zarei et al., 2018).
The most common method to deliver growth factors is the topical application of growth factor solutions, creams, or gels. In a pair of seminal studies, EGF produced a dose-dependent increase in epithelialization following a burn injury in a porcine model (Brown et al., 1986). TGF-α applied at low doses led to improved healing in a PT burn model (Schultz et al., 1987). In both studies, the growth factors were mixed into an antibiotic cream and then applied topically to the wound. Topical growth factor application has also been used to augment existing treatments. For instance, a series of growth factors were applied to a burn wound covered with a skin graft in a rat model. In this study, topical delivery of keratinocyte growth factor (KGF-2), bFGF, and TGF-β2 improved epithelialization rates compared to skin graft alone. Interestingly, KGF-1, IL-4, and macrophage colony stimulating factor (MCSF) did not significantly improve epithelialization rates (Smith et al., 2000). A recent meta-analysis suggested that the topical administration of granulocyte-macrophage colony stimulating factor (GM-CSF), bFGF, or EGF could shorten healing time in PT burns (Zhang et al., 2016). Despite this evidence, to date only one growth factor system has been approved by the FDA for a wound healing application: topical PDGF (Regranex® Gel) for treating chronic and not burn-related wounds (Bolton, 2016). Unfortunately, there have been no reported attempts to apply the Regranex® Gel in a burn wound. However, one US-based trial was identified that is investigating the use of recombinant PDGF in thermal burns (Table 11).
Instead of applying a single recombinant growth factor to treat a burn, other research has considered applying a combination of growth factors derived from allogeneic or autologous sources. Plasma-based treatments of burns are one such area of intense study. Platelet-rich plasma (PRP) contains extracted plasma purified with supraphysiological concentrations of platelets. Recent studies have yielded mixed results. A rodent burn model demonstrated that PRP accelerated wound closure and resulted in less GT compared to controls for PT burns but not FT burns (Venter et al., 2016). In contrast, a recent clinical trial failed to demonstrate any statistically significant improvement in burn healing when PRP was applied as an adjunct with an autograft (Marck et al., 2016). It should be noted that different definitions of PRP exist, and that the variable results in the literature concerning PRP might be due in part to inconsistent PRP formulations across different studies (Wasterlain et al., 2012).
In addition to the existing PRP-based studies, unique formulations of plasma for burn treatments have also been proposed. For instance, plasma was recently formulated with extremely high concentrations of growth factors, including PDGF at ~50-times the standard in vivo concentration (Araki et al., 2012). The potential of this formulation was demonstrated after no HTS formed after treatment of a FT burn on one finger compared to scarring on an adjacent finger with PT burns that were treated conservatively (Mashiko et al., 2016). Other efforts to prepare more durable plasma-based materials using different chemical cross-linkers have been reported. In a pair of recent studies, a polyethylene glycol (PEG)-reinforced fibrin hydrogel was used to treat burn wounds in a DPT porcine model. When applied post-debridement, the PEG-fibrin gel reduced the degree of contraction compared to untreated controls (Burmeister et al., 2018a). Using a similar porcine model, the PEG-fibrin gel was also used to deliver ASCs as an adjunct to a meshed autograft and led to improved angiogenesis (Burmeister et al., 2018b).
Advanced growth factor release strategies in TE often involve the use of microparticles, nanoparticles, or hydrogels in order to carefully control growth factor release rates or improve growth factor half-life. These approaches have been recently investigated for treating burn wounds by developing a biomatrix consisting of PDGF covalently bound to fibrin. PDGF was gradually released from fibrin via enzymatic degradation. The sustained release of PDGF from this matrix improved wound healing in a porcine grafted third degree burn model (Mittermayr et al., 2016). A more complex formulation was reported in which EGF was loaded into an artificial vesicle (termed “liposome”), which was in turn encapsulated into a chitosan gel. This liposome-in-chitosan formulation increased epithelialization rate more than a chitosan gel or EGF applied alone, possibly due to improved longevity of EGF within the liposomes (Degim et al., 2011). These efforts to modulate the release of growth factors into burn wounds can be expanded to include more advanced release strategies, such as the release of multiple factors from a single material or prolonged release of factors over time.
Plasmid DNA (pDNA) is non-chromosomal circular DNA which exploits the cell machinery to produce proteins for a transient amount of time (Scholz and Wagner, 2012). To enhance dermal regeneration after burn, a number of pDNAs that encode for pro-regenerative proteins have been investigated. For instance, a porous dermal equivalent loaded with pDNA-VEGF for local sustained production of VEGF was applied to a porcine FT burn wound. The pDNA-VEGF treated wound demonstrated faster regeneration and the development of a greater number of mature blood vessels compared to control groups (Guo et al., 2011). Intradermal injection of hypoxia inducible factor (HIF-1α) plasmid vector in combination with BMSCs improved wound healing in an elderly murine burn model which was characterized by impaired wound healing due to reduced levels of endogenous HIF-1α (Du et al., 2013).
This phase of wound healing spans the gamut on FDA approvals with allografts only requiring HCT/P, most skin substitutes receiving 510 (k) approved, and cell based substitutes requiring a clinical trial supported PMA (Tables 7, 8). These new TERM strategies are quite varied in their approach from TE skin, stem cells, growth factors, exosomes, secretomes, and gene therapy. Many if not all of these strategies will require a lengthy approval process with clinical trials (Tables 9–11 for current trials). This regulatory pathway is one of the major hurdles to eventually have these therapies in the clinical setting as SOC.
Matrix Remodeling
The final stage of wound healing is matrix remodeling which continues to progress for years after injury and when aberrant, can result in scar formation and contracture. As the proliferative phase of wound healing transitions into the remodeling phase, well organized type I collagen becomes more abundant and wound tensile strength improves, The remodeling of collagen fibers ultimately leads to ~80% wound strength by roughly 6-weeks after injury (Madden and Peacock, 1968; Diegelmann and Evans, 2004). Dependent on myofibroblasts, wound contraction occurs concurrently with remodeling and aids in wound closure. Under normal conditions, fibroblasts and myofibroblasts gradually disappear from the wound by apoptosis, but dysregulation of cell death and persistence of these cells after wound closure can lead to contractures and HTS (Sarrazy et al., 2011). Wound remodeling, specifically focusing on fibroblast/myofibroblast activity, are promising areas for improving wound healing outcomes.
The mechanisms of pathological scarring during remodeling are multifactorial and include exaggerated inflammation, prolonged re-epithelialization, overabundant ECM production, augmented neovascularization, atypical ECM remodeling, and reduced apoptosis (van der Veer et al., 2009). The molecular biology of pathologic scarring is likewise complex, with vast numbers of cytokines, growth factors, and other proteins interacting (Profyris et al., 2012). Rodent models have studied fetal regenerative healing along with adult wound healing to define the critical proteins involved. Among the most important of these molecules, TGF-β1 and TGF-β2 promote scar formation while TGF-β3 reduces scarring (Ferguson and O'Kane, 2004). Similarly, pro-inflammatory cytokines, namely IL-6 and IL-8, promote scarring (Liechty et al., 2000a) while anti-inflammatory cytokines, most importantly IL-10, reduce scarring (Liechty et al., 2000b). As this body of research continues to expand, the key molecular pathways and potential therapeutic targets to reduce scarring will be identified.
HTS Prevention
Currently, two FDA-approved treatments for idiopathic pulmonary fibrosis show some promise in wound healing. Nintedanib is a tyrosine kinase inhibitor that reduces myofibroblast differentiation and ECM production by dermal fibroblasts in animal models (Huang et al., 2016). Pirfenidone acts through an unknown mechanism to limit TGF-β signaling (Macías-Barragán et al., 2010). In clinical studies, this small molecule outperformed compression therapy in a trial of pediatric patients with established hypertrophic burn scars (Armendariz-Borunda et al., 2012; Janka-Zires et al., 2016). These novel therapies need to be further evaluated in the burn population in order to establish their safety and efficacy before widespread adoption is feasible (Table 13).
pDNA and RNA interference (RNAi) strategies have advanced significantly over the past 30 years. Small interfering RNA (siRNA) and miRNA play a role in RNAi pathways by post-transcriptional regulation of gene expression for a period of time (Lam et al., 2015). Currently, pDNA and RNAi strategies for HS treatment are still in their infancy and are primarily being developed in vitro and evaluated in the rabbit HS ear model (Li et al., 2011; Wang et al., 2014; Guo et al., 2017). However, Castleberry et al. developed a layer-by-layer siRNA delivery system to target the expression of connective tissue growth factor (CTGF), a key mediator of the TGF-β1 pro-fibrotic response. In a full-thickness rat burn model, knockdown of CTGF significantly altered the expression of αSMA, tissue inhibitor of metalloprotenase-1 (TIMP-1), and type 1 collagen. The RNAi treatment resulted in improved tissue remodeling and a reducing in total scar area and contraction (Castleberry et al., 2016).
HTS Mitigation
Despite a myriad of SOC scar treatment options including surgery, compression, silicone dressings, intralesional steroid or antimetabolite injection, laser therapy, cryotherapy, and others, published or ongoing clinical research for novel hypertrophic burn scar treatments are limited and frequently include case series or only small clinical trials. These novel therapies target specific molecular pathways vital to matrix remodeling and abnormal collagen deposition.
In a Phase II clinical trial of patients undergoing scar revision surgery, the recombinant TGF-β3 avotermin improved scar appearance when administered immediately following surgery (So et al., 2011). While use of Integra® as a dermal replacement is well established; recently, the use of Integra® in the form of a flowable powder demonstrated efficacy by reducing post-burn scars associated with joints (shoulders, hands, and arm) and improved their range of motion (Hirche et al., 2016). Injection of adipose tissue into burn scars was shown to downregulate TGF-β levels, reduce fibroblast numbers, and halt VEGF production 6 months after treatment, leading to improved scar texture and appearance (Bruno et al., 2013). In a porcine burn study, subcutaneous injections of ASCs or fresh lipoaspirate were delivered to the HTS and reduced scar thickness was demonstrated with both the purified stromal cells and fresh adipose tissue compared to control (Rapp et al., 2017). In other pre-clinical research, topical application of a TGF-β antagonist (Singer et al., 2009) or nitric oxide (Singer et al., 2017) improved healing time and reduced scar thickness in porcine PT burns. In another, porcine scars were treated subcutaneously with recombinant human tropoelastin, ultimately increasing tissue elastin but without a demonstrable effect on scar hardness, flexibility, or inflammation (Xie et al., 2017).
While the technology is not new, lasers are currently in 11 listed clinical trials to determine their safety and efficacy for scar mitigation (Table 12). Pulsed dye lasers administer light to target hemoglobin specifically and coagulate the abundant microvasculature that supports excessive cell and ECM proliferation in scars (Hultman et al., 2012). Fractional photothermolysis with the ablative CO2 or the erbium-doped yttrium aluminum garnet (Er:YAG) lasers create microscopic dermal injuries that renew the healing process on a smaller scale (Tierney et al., 2009). This new cycle of healing occurs with improved collagen deposition and fibroblast apoptosis, leading to improved scar texture and appearance. To achieve suitable results, though, multiple laser treatments may be needed, each carrying associated risks including pain, blistering, and long-term skin discoloration. The available literature reports these complication rates inconsistently, and robust clinical evidence with laser therapy, such as multi-center, randomized, controlled trials, is lacking (Zuccaro et al., 2017). In an effort to combine modalities, ablative lasers may be useful in enhancing the delivery of topical steroids into the scar tissue (Waibel et al., 2013).
The area of scar prevention and mitigation is becoming more important with the increase in survivability of severely burned patients. There is a paucity of approved therapies aimed at improving the long term outcomes of this debilitating injury. Tables 12, 13 are disproportionately tilted toward laser therapies. More therapies are needed from a prevention standpoint but the issue is that not every burn scars nor does every patient. To design an appropriate RCT is difficult in these situations.
Discussion
The current accepted practice for the treatment of burn wounds is early excision of the necrotic tissue followed by immediate autografting. This strategy reduces microbial colonization, improves survival, shortens hospital stay, and decreases HTS formation (Sterling and Heimbach, 2011). In the event of a large TBSA injury or in an attempt to reduce autograft requirements, a variety of epidermal and dermal products have been discussed in this article that may facilitate wound closure. Proper wound bed preparation including adequate debridement, hemostasis, and infection control is paramount prior to autografting in order to prevent graft failure; this is also true with the skin substitutes. The depth of burn and presence of dermis dictates which products can be used alone or in combination, such as a dermal substitute followed by an epidermal substitute. In addition to minimizing the use of donor skin, the research and development of TERM products aims to mitigate the incidence of infection, provide temporary and/or permanent coverage, and accelerate re-epithelialization during the acute stages of wound healing. What is still missing is comprehensive RCT of these products comparing efficacy to each other. One simple example would be a head-to-head comparison of all silver dressings but this can be extrapolated to other topics discussed in this review (i.e., dermal matrices). Exploiting the synergy of combined attributes is where TERM can provide the greatest benefit, such as the development of an antimicrobial full-thickness skin substitute. Moving forward, one of the greatest challenges for the use of TERM products is in deciding which therapies are compatible and also have complementary functions while successfully fitting into the FDA requirements. Definitive RCTs will answer these questions and determine the safety and efficacy of combinatorial approaches. With an understandable emphasis on acute care in the literature, current clinical gaps need to address the inflammatory response, as well as the proliferative and remodeling phases of wound healing. Stem cell secretome and other biological-based therapeutics working as an adjunct to skin substitutes can guide cell proliferation to enhance TERM product integration while potentially preventing scarring. Another major challenge is navigating the FDA approval process successfully. Novel TERM approaches that cannot rely on a “predicate” device to receive 510 (k) approval must undergo clinical trials prior to approval which is costly in terms of actual cost but also time. This inherently limits the advancement of new ideas and technologies that could significantly improve the functional and cosmetic outcomes of burn victims. Although TERM strategies have expanded our understanding of skin biology and physiology, no therapy is currently available that truly replaces skin, restores function (e.g., pigmentation, hair follicles, glands, elasticity, and nerves), and prevents scarring.
Author Contributions
RS: wound healing cascade, hemostasis section, discussion, compilation of article, tables; SN: burn wound coverings and stem cells, figures; CK: infection, gene therapy, tables; LM: immune modulation; RMC: burn wound coverings; NC: growth factors; AC, DT, and RKC: scar prevention and mitigation; JR: current standard of care and clinical gaps; RJC: participated in preparation and revision of manuscript. All authors approved the final version of the manuscript.
Funding
This research was funded by the U.S. Army Medical Research and Materiel Command. This research was supported in part by an appointment to the Postgraduate Research Participation Program at the U.S. Army Institute of Surgical Research administered by the Oak Ridge Institute for Science and Education through an interagency agreement between the U.S. Department of Energy and USAISR.
Disclaimer
The views expressed in this article are those of the authors and do not reflect the official policy or position of the U.S. Army Medical Department, Department of the Army, DoD, or the U.S. Government. The authors are employees of the U.S.Government, and this work was prepared as part of their official duties.
Conflict of Interest Statement
The authors declare that the research was conducted in the absence of any commercial or financial relationships that could be construed as a potential conflict of interest.
Abbreviations
α-SMA, alpha smooth muscle actin; AM, amniotic membrane; AMP, Antimicrobial peptides; ASC, adipose tissue derived mesenchymal stem cells; AATB, American Association of Tissue Banks; bFGF, basic fibroblast growth factor; BLA, Biological License Application; BMSC, bone marrow derived mesenchymal stem cells; CEA, cultured epithelial autograft; COX-2, cyclooxygenase-2; CSS, cultured skin substitutes; CXCL2, chemokine (C-X-C motif) ligand 2; DCs, dendritic cells; DPT, deep partial-thickness; ECM, extracellular matrix; EGF, epidermal growth factor; ESS, engineered skin substitutes; FDA, Food and Drug Administration; FLT-3, fms-like tyrosine kinase-3 ligand; FT, full-thickness; GM-CSF, granulocyte-macrophage colony stimulating factor; GT, Granulation Tissue; HCT/Ps, Human cells, tissues, and Cellular and Tissue-based Products; HDE, Humanitarian Device Exemption; HFSC, hair follicle stem cells; HIF, hypoxia inducible factor; HTS, Hypertrophic scars; IDE, Investigational Device Exemption; IL, interleukin; IFN-γ, interferon gamma; IND, Investigational New Drug; KGF, keratinocyte growth factor; MCSF, macrophage colony stimulating factor; miRNA, microRNA; MMP, matrix metalloproteinase; MRSA, Methicillin-resistant Staphylococcus aureus; MSC, mesenchymal stem cells; NDA, New Drug Application; NO, nitric oxide; NSAIDS, non-steroidal anti-inflammatory drugs; PDGF, platelet derived growth factor; pDNA, Plasmid DNA; PGE2, Prostaglandin E2; PMA, Premarket Approval; PMNs, polymorphonuclear leukocytes or neutrophils; PRP, platelet rich plasma; PT, partial-thickness; RCT, randomized control trials; RNAi, RNA interference; SDF, stromal derived factor; siRNA, Small interfering RNA; SOC, standard of care; SPT, superficial partial-thickness; SSD, silver sulfadiazine; SVF, stromal vascular fraction; TBSA, total body surface area; TE, tissue engineering; TERM, tissue engineering and regenerative medicine; TGF-β, transforming growth factor beta; TIMP, tissue inhibitor of matrix metalloproteinase; TNF-α, tumor necrosis factor alpha; VEGF, vascular endothelial growth factor.
References
Acar, A., Uygur, F., Diktas, H., Evinc, R., Ulkur, E., Oncul, O., et al. (2011). Comparison of silver-coated dressing (Acticoat®), chlorhexidine acetate 0.5% (Bactigrass®) and nystatin for topical antifungal effect in Candida albicans-contaminated, full-skin-thickness rat burn wounds. Burns 37, 882–885. doi: 10.1016/j.burns.2011.01.024
Afshari, A., Nguyen, L., Kahn, S. A., and Summitt, B. (2017). 2.5% mafenide acetate: a cost-effective alternative to the 5% solution for burn wounds. J. Burn Care Res. 38, e42–e47. doi: 10.1097/BCR.0000000000000425
Akita, S., Hayashida, K., Yoshimoto, H., Fujioka, M., Senju, C., Morooka, S., et al. (2018). Novel application of cultured epithelial autografts (CEA) with expanded mesh skin grafting over an artificial dermis or dermal wound bed preparation. Int. J. Mol. Sci. 19:57. doi: 10.3390/ijms19010057
Allorto, N. L., Bishop, D. G., and Rodseth, R. N. (2015). Vasoconstrictor clysis in burn surgery and its impact on outcomes: systematic review and meta-analysis. Burns 41, 1140–1146. doi: 10.1016/j.burns.2015.03.010
American Burn Association (2016). Burn Incidence Fact Sheet. Chicago, IL: The ABA. Available online at: http://ameriburn.org/who-we-are/media/burn-incidence-fact-sheet/
Anthonissen, M., Daly, D., Janssens, T., and Van den Kerckhove, E. (2016). The effects of conservative treatments on burn scars: a systematic review. Burns 42, 508–518. doi: 10.1016/j.burns.2015.12.006
Araki, J., Jona, M., Eto, H., Aoi, N., Kato, H., Suga, H., et al. (2012). Optimized preparation method of platelet-concentrated plasma and noncoagulating platelet-derived factor concentrates: maximization of platelet concentration and removal of fibrinogen. Tissue Eng. Part C Methods 18, 176–185. doi: 10.1089/ten.tec.2011.0308
Armendariz-Borunda, J., Lyra-Gonzalez, I., Medina-Preciado, D., Gonzalez-Garcia, I., Martinez-Fong, D., Miranda, R. A., et al. (2012). A controlled clinical trial with pirfenidone in the treatment of pathological skin scarring caused by burns in pediatric patients. Ann. Plast. Surg. 68, 22–28. doi: 10.1097/SAP.0b013e31821b6d08
Atalay, S., Coruh, A., and Deniz, K. (2014). Stromal vascular fraction improves deep partial thickness burn wound healing. Burns 40, 1375–1383. doi: 10.1016/j.burns.2014.01.023
Austin, R. E., Merchant, N., Shahrokhi, S., and Jeschke, M. G. (2015). A comparison of Biobrane and cadaveric allograft for temporizing the acute burn wound: cost and procedural time. Burns 41, 749–753. doi: 10.1016/j.burns.2014.10.003
Aziz, Z., and Abdul Rasool Hassan, B. (2017). The effects of honey compared to silver sulfadiazine for the treatment of burns: a systematic review of randomized controlled trials. Burns 43, 50–57. doi: 10.1016/j.burns.2016.07.004
Aziz, Z., Abu, S. F., and Chong, N. J. (2012). A systematic review of silver-containing dressings and topical silver agents (used with dressings) for burn wounds. Burns 38, 307–318. doi: 10.1016/j.burns.2011.09.020
Bessey, P. Q., Phillips, B. D., Lentz, C. W., Edelman, L. S., Faraklas, I., Finocchiaro, M. A., et al. (2014). Synopsis of the 2013 annual report of the national burn repository. J. Burn Care Res. 35 (Suppl. 2), S218–S234. doi: 10.1097/BCR.0000000000000080
Bitter, C. C., and Erickson, T. B. (2016). Management of burn injuries in the wilderness: lessons from low-resource settings. Wilderness Environ. Med. 27, 519–525. doi: 10.1016/j.wem.2016.09.001
Bolton, L. L. (2016). Quality randomized clinical trials of topical diabetic foot ulcer healing agents. Adv. Wound Care 5, 137–147. doi: 10.1089/wound.2014.0571
Bombaro, K. M., Engrav, L. H., Carrougher, G. J., Wiechman, S. A., Faucher, L., Costa, B. A., et al. (2003). What is the prevalence of hypertrophic scarring following burns? Burns 29, 299–302. doi: 10.1016/S0305-4179(03)00067-6
Boonkaew, B., Barber, P. M., Rengpipat, S., Supaphol, P., Kempf, M., He, J., et al. (2014). Development and characterization of a novel, antimicrobial, sterile hydrogel dressing for burn wounds: single-step production with gamma irradiation creates silver nanoparticles and radical polymerization. J. Pharm. Sci. 103, 3244–3253. doi: 10.1002/jps.24095
Boyce, S. T., Kagan, R. J., Greenhalgh, D. G., Warner, P., Yakuboff, K. P., Palmieri, T., et al. (2006). Cultured skin substitutes reduce requirements for harvesting of skin autograft for closure of excised, full-thickness burns. J. Trauma 60, 821–829. doi: 10.1097/01.ta.0000196802.91829.cc
Boyce, S. T., Simpson, P. S., Rieman, M. T., Warner, P. M., Yakuboff, K. P., Bailey, J. K., et al. (2017). Randomized, paired-site comparison of autologous engineered skin substitutes and split-thickness skin graft for closure of extensive, full-thickness burns. J. Burn Care Res. 38, 61–70. doi: 10.1097/BCR.0000000000000401
Brown, G. L., Curtsinger, L. III., Brightwell, J. R., Ackerman, D. M., Tobin, G. R., Polk, H. C. Jr., et al. (1986). Enhancement of epidermal regeneration by biosynthetic epidermal growth factor. J. Exp. Med. 163, 1319–1324. doi: 10.1084/jem.163.5.1319
Brown, J. B., Fryer, M. P., Randall, P., and Lu, M. (1953). Postmortem homografts as biological dressings for extensive burns and denuded areas; immediate and preserved homografts as life-saving procedures. Ann. Surg. 138, 618–630. doi: 10.1097/00000658-195310000-00015
Bruno, A., Delli Santi, G., Fasciani, L., Cempanari, M., Palombo, M., and Palombo, P. (2013). Burn scar lipofilling: immunohistochemical and clinical outcomes. J. Craniofac. Surg. 24, 1806–1814. doi: 10.1097/SCS.0b013e3182a148b9
Burmeister, D. M., Roy, D. C., Becerra, S. C., Natesan, S., and Christy, R. J. (2018a). In situ delivery of fibrin-based hydrogels prevents contraction and reduces inflammation. J. Burn Care Res. 39, 40–53. doi: 10.1097/BCR.0000000000000576
Burmeister, D. M., Stone, R. II., Wrice, N., Laborde, A., Becerra, S. C., Natesan, S., et al. (2018b). Delivery of allogeneic adipose stem cells in polyethylene glycol-fibrin hydrogels as an adjunct to meshed autografts after sharp debridement of deep partial thickness burns. Stem Cells Transl. Med. 7, 360–372. doi: 10.1002/sctm.17-0160
Bush, K., and Gertzman, A. A. (2016). “Chapter 5 - process development and manufacturing of human and animal acellular dermal matrices,” in Skin Tissue Engineering and Regenerative Medicine, eds J. H. H. Iv (Boston, MA: Academic Press), 83–108.
Butts, C. C., Bose, K., Frotan, M. A., Hodge, J., and Gulati, S. (2017). Controlling intraoperative hemorrhage during burn surgery: a prospective, randomized trial comparing NuStat® hemostatic dressing to the historic standard of care. Burns 43, 374–378. doi: 10.1016/j.burns.2016.08.026
Calum, H., Moser, C., Jensen, P. Ø., Christophersen, L., Maling, D. S., Gennip, M. V., et al. (2009). Thermal injury induces impaired function in polymorphonuclear neutrophil granulocytes and reduced control of burn wound infection. Clin. Exp. Immunol. 156, 102–110. doi: 10.1111/j.1365-2249.2008.03861.x
Carlsson, A. H., Rose, L. F., Fletcher, J. L., Wu, J. C., Leung, K. P., and Chan, R. K. (2016). Antecedent thermal injury worsens split-thickness skin graft quality: a clinically relevant porcine model of full-thickness burn, excision and grafting. Burns 43, 223–231. doi: 10.1016/j.burns.2016.08.006
Carter, D. A., Blair, S. E., Cokcetin, N. N., Bouzo, D., Brooks, P., Schothauer, R., et al. (2016). Therapeutic manuka honey: no longer so alternative. Front. Microbiol. 7:569. doi: 10.3389/fmicb.2016.00569
Cartotto, R. (2017). Topical antimicrobial agents for pediatric burns. Burns Trauma 5:33. doi: 10.1186/s41038-017-0096-6
Castleberry, S. A., Golberg, A., Sharkh, M. A., Khan, S., Almquist, B. D., Austen, W. G. Jr., et al. (2016). Nanolayered siRNA delivery platforms for local silencing of CTGF reduce cutaneous scar contraction in third-degree burns. Biomaterials 95, 22–34. doi: 10.1016/j.biomaterials.2016.04.007
Centanni, J. M., Straseski, J. A., Wicks, A., Hank, J. A., Rasmussen, C. A., Lokuta, M. A., et al. (2011). StrataGraft skin substitute is well-tolerated and is not acutely immunogenic in patients with traumatic wounds: results from a prospective, randomized, controlled dose escalation trial. Ann. Surg. 253, 672–683. doi: 10.1097/SLA.0b013e318210f3bd
Chen, J., Liu, Z., Chen, M., Zhang, H., and Li, X. (2016). Electrospun gelatin fibers with a multiple release of antibiotics accelerate dermal regeneration in infected deep burns. Macromol. Biosci. 16, 1368–1380. doi: 10.1002/mabi.201600108
Chen, R. N., Ho, H. O., Tsai, Y. T., and Sheu, M. T. (2004). Process development of an acellular dermal matrix (ADM) for biomedical applications. Biomaterials 25, 2679–2686. doi: 10.1016/j.biomaterials.2003.09.070
Cheng, J. Z., Farrokhi, A., Ghahary, A., and Jalili, R. B. (2017). Therapeutic use of stem cells in treatment of burn injuries. J. Burn Care Res. 39, 175–182. doi: 10.1097/BCR.0000000000000571
Chong, S. J., Wong, Y. C., Wu, J., Tan, M. H., Lu, J., and Moochhala, S. M. (2014). Parecoxib reduces systemic inflammation and acute lung injury in burned animals with delayed fluid resuscitation. Int. J. Inflamm. 2014:972645. doi: 10.1155/2014/972645
Christ, G. J., Saul, J. M., Furth, M. E., and Andersson, K. E. (2013). The pharmacology of regenerative medicine. Pharmacol. Rev. 65, 1091–1133. doi: 10.1124/pr.112.007393
Chung, E., Rybalko, V. Y., Hsieh, P. L., Leal, S. L., Samano, M. A., Willauer, A. N., et al. (2016). Fibrin-based stem cell containing scaffold improves the dynamics of burn wound healing. Wound Repair Regen. 24, 810–819. doi: 10.1111/wrr.12459
Ciloglu, N. S., Mert, A. I., Dogan, Z., Demir, A., Cevan, S., Aksaray, S., et al. (2014). Efficacy of silver-loaded nanofiber dressings in Candida albicans-contaminated full-skin thickness rat burn wounds. J. Burn Care Res. 35, e317–e320. doi: 10.1097/BCR.0b013e3182aa7143
Ciociola, A. A., Cohen, L. B., and Kulkarni, P. (2014). How drugs are developed and approved by the FDA: current process and future directions. Am. J. Gastroenterol. 109, 620–623. doi: 10.1038/ajg.2013.407
Cirodde, A., Leclerc, T., Jault, P., Duhamel, P., Lataillade, J. J., and Bargues, L. (2011). Cultured epithelial autografts in massive burns: a single-center retrospective study with 63 patients. Burns 37, 964–972. doi: 10.1016/j.burns.2011.03.011
Dai, T., Huang, Y. Y., Sharma, S. K., Hashmi, J. T., Kurup, D. B., and Hamblin, M. R. (2010). Topical antimicrobials for burn wound infections. Recent Pat. Antiinfect. Drug Discov. 5, 124–151. doi: 10.2174/157489110791233522
Dalkowski, A., Fimmel, S., Beutler, C., and Zouboulis Ch, C. (2003). Cryotherapy modifies synthetic activity and differentiation of keloidal fibroblasts in vitro. Exp. Dermatol. 12, 673–681. doi: 10.1034/j.1600-0625.2003.00015.x
Davis, S. C., and Mertz, P. M. (2008). Determining the effect of an oak bark formulation on methicillin-resistant staphylococcus aureus and wound healing in porcine wound models. Ostomy Wound Manage 54, 16–18.
Degim, Z., Çelebi, N., Alemdaroglu, C., Deveci, M., Ozturk, S., and Ozogul, C. (2011). Evaluation of chitosan gel containing liposome-loaded epidermal growth factor on burn wound healing. Int. Wound J. 8, 343–354. doi: 10.1111/j.1742-481X.2011.00795.x
de Waard, J. W., de Man, B. M., Wobbes, T., van der Linden, C. J., and Hendriks, T. (1998). Inhibition of fibroblast collagen synthesis and proliferation by levamisole and 5-fluorouracil. Eur J Cancer 34, 162–167. doi: 10.1016/S0959-8049(97)00352-3
Dhand, C., Barathi, V. A., Ong, S. T., Venkatesh, M., Harini, S., Dwivedi, N., et al. (2016). Latent oxidative polymerization of catecholamines as potential cross-linkers for biocompatible and multifunctional biopolymer scaffolds. ACS Appl. Mater. Interfaces 8, 32266–32281. doi: 10.1021/acsami.6b12544
Dhand, C., Venkatesh, M., Barathi, V. A., Harini, S., Bairagi, S., Goh Tze Leng, E., et al. (2017). Bio-inspired crosslinking and matrix-drug interactions for advanced wound dressings with long-term antimicrobial activity. Biomaterials 138, 153–168. doi: 10.1016/j.biomaterials.2017.05.043
Diegelmann, R. F., and Evans, M. C. (2004). Wound healing: an overview of acute, fibrotic and delayed healing. Front. Biosci. 9, 283–289. doi: 10.2741/1184
Du, J., Liu, L., Lay, F., Wang, Q., Dou, C., Zhang, X., et al. (2013). Combination of HIF-1alpha gene transfection and HIF-1-activated bone marrow-derived angiogenic cell infusion improves burn wound healing in aged mice. Gene Ther. 20, 1070–1076. doi: 10.1038/gt.2013.32
Duncan, C. L., Enlow, P. T., Szabo, M. M., Tolchin, E., Kelly, R. W., Castanon, L., et al. (2016). A pilot study of the efficacy of active leptospermum honey for the treatment of partial-thickness facial burns. Adv. Skin Wound Care 29, 349–355. doi: 10.1097/01.ASW.0000484666.83140.b0
Ennis, W. J., Sui, A., and Bartholomew, A. (2013). Stem cells and healing: impact on inflammation. Adv Wound Care 2, 369–378. doi: 10.1089/wound.2013.0449
Esteban-Vives, R., Choi, M. S., Young, M. T., Over, P., Ziembicki, J., Corcos, A., et al. (2016). Second-degree burns with six etiologies treated with autologous noncultured cell-spray grafting. Burns 42, e99–e106. doi: 10.1016/j.burns.2016.02.020
Fairweather, M., Heit, Y. I., Buie, J., Rosenberg, L. M., Briggs, A., Orgill, D. P., et al. (2015). Celecoxib inhibits early cutaneous wound healing. J. Surg. Res. 194, 717–724. doi: 10.1016/j.jss.2014.12.026
Fang, F., Huang, R. L., Zheng, Y., Liu, M., and Huo, R. (2016). Bone marrow derived mesenchymal stem cells inhibit the proliferative and profibrotic phenotype of hypertrophic scar fibroblasts and keloid fibroblasts through paracrine signaling. J. Dermatol. Sci. 83, 95–105. doi: 10.1016/j.jdermsci.2016.03.003
FDA (2017). Regulatory Considerations for Human Cells, Tissues, and Cellular and Tissue Based Products: Minimal Manipulation and Homologous Use. Silver Spring, MD: FDA.
FDA (2018a). Humanitarian Device Exemption. Silver Spring, MD: FDA. Available online at: https://www.fda.gov/MedicalDevices/DeviceRegulationandGuidance/HowtoMarketYourDevice/PremarketSubmissions/HumanitarianDeviceExemption/default.htm
FDA (2018b). PMA approvals. Silver Springs, MD: FDA. Available online at: https://www.fda.gov/MedicalDevices/DeviceRegulationandGuidance/HowtoMarketYourDevice/PremarketSubmissions/PremarketApprovalPMA/default.htm
FDA (2018c). Premarket Notification 510(k). Silver Spring, MD: FDA. Available online at: https://www.fda.gov/MedicalDevices/DeviceRegulationandGuidance/HowtoMarketYourDevice/PremarketSubmissions/PremarketNotification510k/default.htm
Fenner, J., and Clark, R. A. F. (2016). “Chapter 1 - Anatomy, physiology, histology, and immunohistochemistry of human skin,” in Skin Tissue Engineering and Regenerative Medicine, eds J. H. H. Iv (Boston, MA: Academic Press), 1–17.
Ferguson, M. W., and O'Kane, S. (2004). Scar-free healing: from embryonic mechanisms to adult therapeutic intervention. Philos. Trans. R. Soc. Lond. B. Biol. Sci. 359, 839–850. doi: 10.1098/rstb.2004.1475
Findlay, F., Proudfoot, L., Stevens, C., and Barlow, P. G. (2016). Cationic host defense peptides; novel antimicrobial therapeutics against Category A pathogens and emerging infections. Pathog. Glob. Health 110, 137–147. doi: 10.1080/20477724.2016.1195036
Finnerty, C. C., Jeschke, M. G., Branski, L. K., Barret, J. P., Dziewulski, P., and Herndon, D. N. (2016). Hypertrophic scarring: the greatest unmet challenge after burn injury. Lancet 388, 1427–1436. doi: 10.1016/S0140-6736(16)31406-4
Foster, K. N., Mullins, R. F., Greenhalgh, D. G., Gamelli, R. L., Glat, P., Lentz, C. W., et al. (2011). Recombinant human thrombin: safety and immunogenicity in pediatric burn wound excision. J. Pediatr. Surg. 46, 1992–1999. doi: 10.1016/j.jpedsurg.2011.05.022
Foubert, P., Barillas, S., Gonzalez, A. D., Alfonso, Z., Zhao, S., Hakim, I., et al. (2015). Uncultured adipose-derived regenerative cells (ADRCs) seeded in collagen scaffold improves dermal regeneration, enhancing early vascularization and structural organization following thermal burns. Burns 41, 1504–1516. doi: 10.1016/j.burns.2015.05.004
Frank, D. H., Brahme, J., and Van de Berg, J. S. (1984). Decrease in rate of wound contraction with the temporary skin substitute biobrane. Ann. Plast. Surg. 12, 519–524. doi: 10.1097/00000637-198406000-00005
Franz, M. G., Steed, D. L., and Robson, M. C. (2007). Optimizing healing of the acute wound by minimizing complications. Curr. Probl. Surg. 44, 691–763. doi: 10.1067/j.cpsurg.2007.07.001
Ghosh, C., Manjunath, G. B., Konai, M. M., Uppu, D. S., Paramanandham, K., Shome, B. R., et al. (2016). Aryl-alkyl-lysines: membrane-active small molecules active against murine model of burn infection. ACS Infect. Dis. 2, 111–122. doi: 10.1021/acsinfecdis.5b00092
Gilbert, R., Vickaryous, M., and Viloria-Petit, A. (2016). Signalling by transforming growth factor beta isoforms in wound healing and tissue regeneration. J. Dev. Biol. 4:21. doi: 10.3390/jdb4020021
Gilbert, T. W., Sellaro, T. L., and Badylak, S. F. (2006). Decellularization of tissues and organs. Biomaterials 27, 3675–3683. doi: 10.1016/j.biomaterials.2006.02.014
Glat, P. M., and Davenport, T. (2017). Current techniques for burn reconstruction: using dehydrated human amnion/chorion membrane allografts as an adjunctive treatment along the reconstructive ladder. Ann Plast Surg. 78(2 Suppl. 1), S14–S18. doi: 10.1097/SAP.0000000000000980
Glat, P. M., Kubat, W. D., Hsu, J. F., Copty, T., Burkey, B. A., Davis, W., et al. (2009). Randomized clinical study of SilvaSorb gel in comparison to Silvadene silver sulfadiazine cream in the management of partial-thickness burns. J. Burn Care Res. 30, 262–267. doi: 10.1097/BCR.0b013e318198a2e8
Gravante, G., Di Fede, M. C., Araco, A., Grimaldi, M., De Angelis, B., Arpino, A., et al. (2007). A randomized trial comparing ReCell system of epidermal cells delivery versus classic skin grafts for the treatment of deep partial thickness burns. Burns 33, 966–972. doi: 10.1016/j.burns.2007.04.011
Greenhalgh, D. G., Gamelli, R. L., Collins, J., Sood, R., Mozingo, D. W., Gray, T. E., et al. (2009). Recombinant thrombin: safety and immunogenicity in burn wound excision and grafting. J. Burn Care Res. 30, 371–379. doi: 10.1097/BCR.0b013e3181a28979
Greenhalgh, D. G., Saffle, J. R., Holmes, J. H. T., Gamelli, R. L., Palmieri, T. L., Horton, J. W., et al. (2007). American Burn Association consensus conference to define sepsis and infection in burns. J. Burn Care Res. 28, 776–790. doi: 10.1097/BCR.0b013e3181599bc9
Guo, L., Xu, K., Yan, H., Feng, H., Wang, T., Chai, L., et al. (2017). MicroRNA expression signature and the therapeutic effect of the microRNA21 antagomir in hypertrophic scarring. Mol. Med. Rep. 15, 1211–1221. doi: 10.3892/mmr.2017.6104
Guo, R., Xu, S., Ma, L., Huang, A., and Gao, C. (2011). The healing of full-thickness burns treated by using plasmid DNA encoding VEGF-165 activated collagen-chitosan dermal equivalents. Biomaterials 32, 1019–1031. doi: 10.1016/j.biomaterials.2010.08.087
Hacker, S., Mittermayr, R., Nickl, S., Haider, T., Lebherz-Eichinger, D., Beer, L., et al. (2016). Paracrine factors from irradiated peripheral blood mononuclear cells improve skin regeneration and angiogenesis in a porcine burn model. Sci. Rep. 6:25168. doi: 10.1038/srep25168
Hamill, R. J. (2013). Amphotericin B formulations: a comparative review of efficacy and toxicity. Drugs 73, 919–934. doi: 10.1007/s40265-013-0069-4
Hammer, D., Rendon, J. L., Sabino, J., Latham, K., Fleming, M. E., and Valerio, I. L. (2017). Restoring full-thickness defects with spray skin in conjunction with dermal regenerate template and split-thickness skin grafting: a pilot study. J. Tissue Eng. Regen. Med. 11, 3523–3529. doi: 10.1002/term.2264
Han, G., Li, F., Singh, T. P., Wolf, P., and Wang, X.-J. (2012). The pro-inflammatory role of TGFbeta1: a paradox. Int. J. Biol. Sci. 8, 228–235. doi: 10.7150/ijbs.8.228
Hayashida, K., and Akita, S. (2017). Surgical treatment algorithms for post-burn contractures. Burns Trauma 5:9. doi: 10.1186/s41038-017-0074-z
Heimbach, D. M., Afromowitz, M. A., Engrav, L. H., Marvin, J. A., and Perry, B. (1984). Burn depth estimation–man or machine. J. Trauma 24, 373–378. doi: 10.1097/00005373-198405000-00001
Hernon, C. A., Dawson, R. A., Freedlander, E., Short, R., Haddow, D. B., Brotherston, M., et al. (2006). Clinical experience using cultured epithelial autografts leads to an alternative methodology for transferring skin cells from the laboratory to the patient. Regen. Med. 1, 809–821. doi: 10.2217/17460751.1.6.809
Heyneman, A., Hoeksema, H., Vandekerckhove, D., Pirayesh, A., and Monstrey, S. (2016). The role of silver sulphadiazine in the conservative treatment of partial thickness burn wounds: a systematic review. Burns 42, 1377–1386. doi: 10.1016/j.burns.2016.03.029
Hirche, C., Citterio, A., Hoeksema, H., Koller, J., Lehner, M., Martinez, J. R., et al. (2017). Eschar removal by bromelain based enzymatic debridement (Nexobrid®) in burns: an European consensus. Burns 43, 1640–1653. doi: 10.1016/j.burns.2017.07.025
Hirche, C., Senghaas, A., Fischer, S., Hollenbeck, S. T., Kremer, T., and Kneser, U. (2016). Novel use of a flowable collagen-glycosaminoglycan matrix (Integra Flowable Wound Matrix) combined with percutaneous cannula scar tissue release in treatment of post-burn malfunction of the hand–A preliminary 6 month follow-up. Burns 42, e1–e7. doi: 10.1016/j.burns.2015.10.013
Horch, R. E., Debus, M., Wagner, G., and Stark, G. B. (2000). Cultured human keratinocytes on type I collagen membranes to reconstitute the epidermis. Tissue Eng. 6, 53–67. doi: 10.1089/107632700320892
Hu, C., Yong, X., Li, C., Lu, M., Liu, D., Chen, L., et al. (2013). CXCL12/CXCR4 axis promotes mesenchymal stem cell mobilization to burn wounds and contributes to wound repair. J. Surg. Res. 183, 427–434. doi: 10.1016/j.jss.2013.01.019
Huang, H. N., Pan, C. Y., Wu, H. Y., and Chen, J. Y. (2017). Antimicrobial peptide Epinecidin-1 promotes complete skin regeneration of methicillin-resistant Staphylococcus aureus-infected burn wounds in a swine model. Oncotarget 8, 21067–21080. doi: 10.18632/oncotarget.15042
Huang, J., Beyer, C., Palumbo-Zerr, K., Zhang, Y., Ramming, A., Distler, A., et al. (2016). Nintedanib inhibits fibroblast activation and ameliorates fibrosis in preclinical models of systemic sclerosis. Ann. Rheum. Dis. 75, 883–890. doi: 10.1136/annrheumdis-2014-207109
Hudson, D. A., and Renshaw, A. (2006). An algorithm for the release of burn contractures of the extremities. Burns 32, 663–668. doi: 10.1016/j.burns.2006.02.009
Hultman, C. S., Edkins, R. E., Lee, C. N., Calvert, C. T., and Cairns, B. A. (2012). Shine on: review of laser- and light-based therapies for the treatment of burn scars. Dermatol. Res. Pract. 2012:243651. doi: 10.1155/2012/243651
Hung, A., Vu, Q., and Mostovoy, L. (2017). A systematic review of U.S. biosimilar approvals: what evidence does the FDA require and how are manufacturers responding? J. Manag. Care Spec. Pharm. 23, 1234–1244. doi: 10.18553/jmcp.2017.23.12.1234
Hurler, J., Berg, O. A., Skar, M., Conradi, A. H., Johnsen, P. J., and Skalko-Basnet, N. (2012). Improved burns therapy: liposomes-in-hydrogel delivery system for mupirocin. J. Pharm. Sci. 101, 3906–3915. doi: 10.1002/jps.23260
Hussain, S., and Ferguson, C. (2006). Best evidence topic report. Silver sulphadiazine cream in burns. Emerg. Med. J. 23, 929–932. doi: 10.1136/emj.2006.043059
Janka-Zires, M., Almeda-Valdes, P., Uribe-Wiechers, A. C., Juarez-Comboni, S. C., Lopez-Gutierrez, J., Escobar-Jimenez, J. J., et al. (2016). Topical administration of pirfenidone increases healing of chronic diabetic foot ulcers: a randomized crossover study. J. Diabetes Res. 2016:7340641. doi: 10.1155/2016/7340641
Jin, H. J., Bae, Y. K., Kim, M., Kwon, S. J., Jeon, H. B., Choi, S. J., et al. (2013). Comparative analysis of human mesenchymal stem cells from bone marrow, adipose tissue, and umbilical cord blood as sources of cell therapy. Int. J. Mol. Sci. 14, 17986–18001. doi: 10.3390/ijms140917986
Jull, A. B., Cullum, N., Dumville, J. C., Westby, M. J., Deshpande, S., and Walker, N. (2015). Honey as a topical treatment for wounds. Cochrane Database Syst. Rev. CD005083. doi: 10.1002/14651858.CD005083.pub4
Kachgal, S., and Putnam, A. J. (2011). Mesenchymal stem cells from adipose and bone marrow promote angiogenesis via distinct cytokine and protease expression mechanisms. Angiogenesis 14, 47–59. doi: 10.1007/s10456-010-9194-9
Kadouri, D. E., To, K., Shanks, R. M., and Doi, Y. (2013). Predatory bacteria: a potential ally against multidrug-resistant Gram-negative pathogens. PLoS ONE 8:e63397. doi: 10.1371/journal.pone.0063397
Kagan, R. J., Peck, M. D., Ahrenholz, D. H., Hickerson, W. L., Holmes, J. T., Korentager, R., et al. (2013). Surgical management of the burn wound and use of skin substitutes: an expert panel white paper. J. Burn Care Res. 34, e60–e79. doi: 10.1097/BCR.0b013e31827039a6
Kearns, R. D., Conlon, K. M., Valenta, A. L., Lord, G. C., Cairns, C. B., Holmes, J. H., et al. (2014). Disaster planning: the basics of creating a burn mass casualty disaster plan for a burn center. J. Burn Care Res. 35, e1–e13. doi: 10.1097/BCR.0b013e31829afe25
Kesting, M. R., Wolff, K. D., Hohlweg-Majert, B., and Steinstraesser, L. (2008). The role of allogenic amniotic membrane in burn treatment. J. Burn Care Res. 29, 907–916. doi: 10.1097/BCR.0b013e31818b9e40
Kilroy, G. E., Foster, S. J., Wu, X., Ruiz, J., Sherwood, S., Heifetz, A., et al. (2007). Cytokine profile of human adipose-derived stem cells: expression of angiogenic, hematopoietic, and pro-inflammatory factors. J. Cell. Physiol. 212, 702–709. doi: 10.1002/jcp.21068
Kosaraju, R., Rennert, R. C., Maan, Z. N., Duscher, D., Barrera, J., Whittam, A. J., et al. (2016). Adipose-derived stem cell-seeded hydrogels increase endogenous progenitor cell recruitment and neovascularization in wounds. Tissue Eng. Part A 22, 295–305. doi: 10.1089/ten.tea.2015.0277
Krausz, A. E., Adler, B. L., Landriscina, A., Rosen, J. M., Musaev, T., Nosanchuk, J. D., et al. (2015). Biafine topical emulsion accelerates excisional and burn wound healing in mice. Arch. Dermatol. Res. 307, 583–594. doi: 10.1007/s00403-015-1559-x
Krishnan, P., Frew, Q., Green, A., Martin, R., and Dziewulski, P. (2013). Cause of death and correlation with autopsy findings in burns patients. Burns 39, 583–588. doi: 10.1016/j.burns.2012.09.017
Kumar, R. J., Kimble, R. M., Boots, R., and Pegg, S. P. (2004). Treatment of partial-thickness burns: a prospective, randomized trial using Transcyte. ANZ J. Surg. 74, 622–626. doi: 10.1111/j.1445-1433.2004.03106.x
Lal, S., Barrow, R. E., Wolf, S. E., Chinkes, D. L., Hart, D. W., Heggers, J. P., et al. (2000). Biobrane improves wound healing in burned children without increased risk of infection. Shock 14, 314–318. discussion: 318–319. doi: 10.1097/00024382-200014030-00013
Lam, J. K., Chow, M. Y., Zhang, Y., and Leung, S. W. (2015). siRNA versus miRNA as therapeutics for gene silencing. Mol. Ther. Nucleic Acids 4:e252. doi: 10.1038/mtna.2015.23
Lawrence, J. W., Mason, S. T., Schomer, K., and Klein, M. B. (2012). Epidemiology and impact of scarring after burn injury: a systematic review of the literature. J. Burn Care Res. 33, 136–146. doi: 10.1097/BCR.0b013e3182374452
Lazarus, G. S., Cooper, D. M., Knighton, D. R., Margolis, D. J., Pecoraro, R. E., Rodeheaver, G., et al. (1994). Definitions and guidelines for assessment of wounds and evaluation of healing. Arch. Dermatol. 130, 489–493. doi: 10.1001/archderm.1994.01690040093015
Leonard, D. A., Mallard, C., Albritton, A., Torabi, R., Mastroianni, M., Sachs, D. H., et al. (2017). Skin grafts from genetically modified alpha-1,3-galactosyltransferase knockout miniature swine: a functional equivalent to allografts. Burns 43, 1717–1724. doi: 10.1016/j.burns.2017.04.026
Leto Barone, A. A., Mastroianni, M., Farkash, E. A., Mallard, C., Albritton, A., Torabi, R., et al. (2015). Genetically modified porcine split-thickness skin grafts as an alternative to allograft for provision of temporary wound coverage: preliminary characterization. Burns 41, 565–574. doi: 10.1016/j.burns.2014.09.003
Levin, G., and Sukhareva, E. (2016). The influence of thermal trauma on pro- and anticoagulant activity of erythrocyte-derived microvesicles. Burns 42, 1528–1533. doi: 10.1016/j.burns.2016.04.013
Li, P., Liu, P., Xiong, R. P., Chen, X. Y., Zhao, Y., Lu, W. P., et al. (2011). Ski, a modulator of wound healing and scar formation in the rat skin and rabbit ear. J. Pathol. 223, 659–671. doi: 10.1002/path.2831
Li, X., Liu, L., Yang, J., Yu, Y., Chai, J., Wang, L., et al. (2016). Exosome derived from human umbilical cord mesenchymal stem cell mediates MiR-181c attenuating burn-induced excessive inflammation. EBioMedicine 8, 72–82. doi: 10.1016/j.ebiom.2016.04.030
Liechty, K. W., Adzick, N. S., and Crombleholme, T. M. (2000a). Diminished interleukin 6 (IL-6) production during scarless human fetal wound repair. Cytokine 12, 671–676. doi: 10.1006/cyto.1999.0598
Liechty, K. W., Kim, H. B., Adzick, N. S., and Crombleholme, T. M. (2000b). Fetal wound repair results in scar formation in interleukin-10-deficient mice in a syngeneic murine model of scarless fetal wound repair. J. Pediatr. Surg. 35, 866–872. discussion: 872–863. doi: 10.1053/jpsu.2000.6868
Lindberg, T., Andersson, O., Palm, M., and Fagerstrom, C. (2015). A systematic review and meta-analysis of dressings used for wound healing: the efficiency of honey compared to silver on burns. Contemp. Nurse 51, 121–134. doi: 10.1080/10376178.2016.1171727
Lozito, T. P., and Tuan, R. S. (2011). Mesenchymal stem cells inhibit both endogenous and exogenous MMPs via secreted TIMPs. J. Cell. Physiol. 226, 385–396. doi: 10.1002/jcp.22344
Ma, Z., Han, J., Chang, B., Gao, L., Lu, Z., Lu, F., et al. (2017). Membrane-active amphipathic peptide WRL3 with in vitro antibiofilm capability and in vivo efficacy in treating methicillin-resistant staphylococcus aureus burn wound infections. ACS Infect. Dis. 3, 820–832. doi: 10.1021/acsinfecdis.7b00100
Macías-Barragán, J., Sandoval-Rodríguez, A., Navarro-Partida, J., and Armendáriz-Borunda, J. (2010). The multifaceted role of pirfenidone and its novel targets. Fibrogenesis Tissue Repair. 3:16. doi: 10.1186/1755-1536-3-16
Madden, J. W., and Peacock, E. E. Jr. (1968). Studies on the biology of collagen during wound healing. I. Rate of collagen synthesis and deposition in cutaneous wounds of the rat. Surgery 64, 288–294.
Mahlapuu, M., Håkansson, J., Ringstad, L., and Bjorn, C. (2016). Antimicrobial peptides: an emerging category of therapeutic agents. Front. Cell. Infect. Microbiol. 6:194. doi: 10.3389/fcimb.2016.00194
Malik, K. I., Malik, M. A., and Aslam, A. (2010). Honey compared with silver sulphadiazine in the treatment of superficial partial-thickness burns. Int. Wound J. 7, 413–417. doi: 10.1111/j.1742-481X.2010.00717.x
Maranda, E. L., Rodriguez-Menocal, L., and Badiavas, E. V. (2017). Role of mesenchymal stem cells in dermal repair in burns and diabetic wounds. Curr. Stem Cell Res. Ther. 12, 61–70. doi: 10.2174/1574888X11666160714115926
Marck, R. E., Gardien, K. L., Stekelenburg, C. M., Vehmeijer, M., Baas, D., Tuinebreijer, W. E., et al. (2016). The application of platelet-rich plasma in the treatment of deep dermal burns: A randomized, double-blind, intra-patient controlled study. Wound Repair Regen. 24, 712–720. doi: 10.1111/wrr.12443
Marks, J. G., and Miller, J. J. (2013). Lookingbill and Marks' Principles of Dermatology, 1 online resource (x, 298 p.). Available online at: http://www.clinicalkey.com/dura/browse/bookChapter/3-s2.0-C20100660068
Marx, D. E., and Barillo, D. J. (2014). Silver in medicine: the basic science. Burns 40(Suppl. 1), S9–S18. doi: 10.1016/j.burns.2014.09.010
Mashiko, T., Minabe, T., Yamakawa, T., Araki, J., Sano, H., and Yoshimura, K. (2016). Platelet-derived factor concentrates with hyaluronic acid scaffolds for treatment of deep burn wounds. Plast. Reconstr. Surg. Glob. Open 4:e1089. doi: 10.1097/GOX.0000000000001089
Mathews, A. L., Cheng, M. H., Muller, J. M., Lin, M. C., Chang, K. W., and Chung, K. C. (2017). Cost analysis of 48 burn patients in a mass casualty explosion treated at chang gung memorial hospital. Injury 48, 80–86. doi: 10.1016/j.injury.2016.08.007
Matsumura, H., Gondo, M., Imai, R., Shibata, D., and Watanabe, K. (2013). Chronological histological findings of cultured epidermal autograft over bilayer artificial dermis. Burns 39, 705–713. doi: 10.1016/j.burns.2012.10.004
McHeik, J. N., Barrault, C., Levard, G., Morel, F., Bernard, F. X., and Lecron, J. C. (2014). Epidermal healing in burns: autologous keratinocyte transplantation as a standard procedure: update and perspective. Plast Reconstr. Surg. Glob. Open 2:e218. doi: 10.1097/GOX.0000000000000176
Mitchell, R. T., Funk, D., Spiwak, R., and Logsetty, S. (2011). Phenylephrine tumescence in split-thickness skin graft donor sites in surgery for burn injury- a concentration finding study. J. Burn Care Res. 32, 129–134. doi: 10.1097/BCR.0b013e318204b39b
Mittermayr, R., Branski, L., Moritz, M., Jeschke, M. G., Herndon, D. N., Traber, D., et al. (2016). Fibrin biomatrix-conjugated platelet-derived growth factor AB accelerates wound healing in severe thermal injury. J. Tissue Eng. Regen. Med. 10, E275–E285. doi: 10.1002/term.1749
Mohammadi, A. A., Seyed Jafari, S. M., Kiasat, M., Tavakkolian, A. R., Imani, M. T., Ayaz, M., et al. (2013). Effect of fresh human amniotic membrane dressing on graft take in patients with chronic burn wounds compared with conventional methods. Burns 39, 349–353. doi: 10.1016/j.burns.2012.07.010
Monsaingeon, A., and Molimard, R. (1976). Wound healing. Comparison of healing rates of burn wounds and of excisional wounds. Eur. Surg. Res. 8, 337–343. doi: 10.1159/000127878
Monstrey, S., Hoeksema, H., Verbelen, J., Pirayesh, A., and Blondeel, P. (2008). Assessment of burn depth and burn wound healing potential. Burns 34, 761–769. doi: 10.1016/j.burns.2008.01.009
Moura, L. I. F., Dias, A. M. A., Carvalho, E., and de Sousa, H. C. (2013). Recent advances on the development of wound dressings for diabetic foot ulcer treatment—a review. Acta Biomater. 9, 7093–7114. doi: 10.1016/j.actbio.2013.03.033
Mowry, K. C., Bonvallet, P. P., and Bellis, S. L. (2017). Enhanced skin regeneration using a novel amniotic-derived tissue graft. Wounds 29, 277–285.
Muangman, P., Pundee, C., Opasanon, S., and Muangman, S. (2010). A prospective, randomized trial of silver containing hydrofiber dressing versus 1% silver sulfadiazine for the treatment of partial thickness burns. Int. Wound J. 7, 271–276. doi: 10.1111/j.1742-481X.2010.00690.x
Munteanu, A., Florescu, I. P., and Nitescu, C. (2016). A modern method of treatment: The role of silver dressings in promoting healing and preventing pathological scarring in patients with burn wounds. J. Med. Life 9, 306–315.
Myers, S. R., Grady, J., Soranzo, C., Sanders, R., Green, C., Leigh, I. M., et al. (1997). A hyaluronic acid membrane delivery system for cultured keratinocytes: clinical “take” rates in the porcine kerato-dermal model. J. Burn Care Rehabil. 18, 214–222. doi: 10.1097/00004630-199705000-00007
Najar, M., Raicevic, G., Boufker, H. I., Fayyad Kazan, H., De Bruyn, C., Meuleman, N., et al. (2010). Mesenchymal stromal cells use PGE2 to modulate activation and proliferation of lymphocyte subsets: combined comparison of adipose tissue, Wharton's Jelly and bone marrow sources. Cell. Immunol. 264, 171–179. doi: 10.1016/j.cellimm.2010.06.006
Nasir, N. A., Halim, A. S., Singh, K. K., Dorai, A. A., and Haneef, M. N. (2010). Antibacterial properties of tualang honey and its effect in burn wound management: a comparative study. BMC Complement. Altern. Med. 10:31. doi: 10.1186/1472-6882-10-31
National Institute of Health. (2018). Tissue Engineering and Regenerative Medicine. Available online at: https://www.nibib.nih.gov/science-education/science-topics/tissue-engineering-and-regenerative-medicine#1166
Newman, D. J., and Cragg, G. M. (2012). Natural products as sources of new drugs over the 30 years from 1981 to 2010. J. Nat. Prod. 75, 311–335. doi: 10.1021/np200906s
Ng, W. L., Wang, S., Yeong, W. Y., and Naing, M. W. (2016). Skin bioprinting: impending reality or fantasy? Trends Biotechnol. 34, 689–699. doi: 10.1016/j.tibtech.2016.04.006
Nidadavolu, P., Amor, W., Tran, P. L., Dertien, J., Colmer-Hamood, J. A., and Hamood, A. N. (2012). Garlic ointment inhibits biofilm formation by bacterial pathogens from burn wounds. J. Med. Microbiol. 61(Pt 5), 662–671. doi: 10.1099/jmm.0.038638-0
Noordenbos, J., Doré, C., and Hansbrough, J. F. (1999). Safety and efficacy of TransCyte for the treatment of partial-thickness burns. J. Burn Care Rehabil. 20, 275–281. doi: 10.1097/00004630-199907000-00002
Norbury, W., Herndon, D. N., Tanksley, J., Jeschke, M. G., and Finnerty, C. C. (2016). Infection in burns. Surg. Infect. 17, 250–255. doi: 10.1089/sur.2013.134
Norman, G., Christie, J., Liu, Z., Westby, M. J., Jefferies, J. M., Hudson, T., et al. (2017). Antiseptics for burns. Cochrane Database Syst. Rev. 7:Cd011821. doi: 10.1002/14651858.CD011821.pub2
Nunes, P. S., Rabelo, A. S., Souza, J. C., Santana, B. V., da Silva, T. M., Serafini, M. R., et al. (2016). Gelatin-based membrane containing usnic acid-loaded liposome improves dermal burn healing in a porcine model. Int. J. Pharm. 513, 473–482. doi: 10.1016/j.ijpharm.2016.09.040
O'Brien, L., and Jones, D. J. (2013). Silicone gel sheeting for preventing and treating hypertrophic and keloid scars. Cochrane Database Syst Rev. CD003826. doi: 10.1002/14651858.CD003826.pub3
O'Dea, K. P., Porter, J. R., Tirlapur, N., Katbeh, U., Singh, S., Handy, J. M., et al. (2016). Circulating microvesicles are elevated acutely following major burns injury and associated with clinical severity. PLoS ONE 11:e0167801. doi: 10.1371/journal.pone.0167801
Orgill, D. P. (2009). Excision and skin grafting of thermal burns. N. Engl. J. Med. 360, 893–901. doi: 10.1056/NEJMct0804451
Palmieri, T. L., and Greenhalgh, D. G. (2002). Topical treatment of pediatric patients with burns: a practical guide. Am. J. Clin. Dermatol. 3, 529–534. doi: 10.2165/00128071-200203080-00003
Pappas, P. G., Kauffman, C. A., Andes, D., Benjamin, D. K. Jr., Calandra, T. F., Edwards, J. E. Jr., et al. (2009). Clinical practice guidelines for the management of candidiasis: 2009 update by the Infectious Diseases Society of America. Clin. Infect. Dis. 48, 503–535. doi: 10.1086/596757
Peral, M. C., Martinez, M. A., and Valdez, J. C. (2009). Bacteriotherapy with Lactobacillus plantarum in burns. Int. Wound J. 6, 73–81. doi: 10.1111/j.1742-481X.2008.00577.x
Phillips, P. L., Yang, Q., Davis, S., Sampson, E. M., Azeke, J. I., Hamad, A., et al. (2015). Antimicrobial dressing efficacy against mature Pseudomonas aeruginosa biofilm on porcine skin explants. Int. Wound J. 12, 469–483. doi: 10.1111/iwj.12142
Phinney, D. G., and Pittenger, M. F. (2017). Concise review: MSC-derived exosomes for cell-free therapy. Stem Cells 35, 851–858. doi: 10.1002/stem.2575
Picard, F., Hersant, B., Bosc, R., and Meningaud, J. P. (2015). The growing evidence for the use of platelet-rich plasma on diabetic chronic wounds: a review and a proposal for a new standard care. Wound Repair Regen. 23, 638–643. doi: 10.1111/wrr.12317
Porter, C., Tompkins, R. G., Finnerty, C. C., Sidossis, L. S., Suman, O. E., and Herndon, D. N. (2016). The metabolic stress response to burn trauma: current understanding and therapies. Lancet 388, 1417–1426. doi: 10.1016/S0140-6736(16)31469-6
Prockop, D. J., and Oh, J. Y. (2012). Mesenchymal stem/stromal cells (MSCs): role as guardians of inflammation. Mol. Ther. 20, 14–20. doi: 10.1038/mt.2011.211
Profyris, C., Tziotzios, C., and Do Vale, I. (2012). Cutaneous scarring: pathophysiology, molecular mechanisms, and scar reduction therapeutics Part I. The molecular basis of scar formation. J Am Acad Dermatol. 66, 1–10; quiz 11–12. doi: 10.1016/j.jaad.2011.05.055
Rahmanian-Schwarz, A., Beiderwieden, A., Willkomm, L. M., Amr, A., Schaller, H. E., and Lotter, O. (2011). A clinical evaluation of Biobrane® and Suprathel® in acute burns and reconstructive surgery. Burns 37, 1343–1348. doi: 10.1016/j.burns.2011.07.010
Rapp, S. J., Pan, B. S., Schwentker, A. R., and Van Aalst, J. (2017). Effects of autologous fat and ASCs on swine hypertrophic burn scars: a multimodal quantitative analysis. Plast. Reconstr. Surg. Glob. Open 5:e1547. doi: 10.1097/GOX.0000000000001547
Rea, S., Giles, N. L., Webb, S., Adcroft, K. F., Evill, L. M., Strickland, D. H., et al. (2009). Bone marrow-derived cells in the healing burn wound–more than just inflammation. Burns 35, 356–364. doi: 10.1016/j.burns.2008.07.011
Reilly, D. A., Hickey, S., Glat, P., Lineaweaver, W. C., and Goverman, J. (2017). Clinical experience: using dehydrated human amnion/chorion membrane allografts for acute and reconstructive burn care. Ann. Plast. Surg. 78(2 Suppl. 1), S19–S26. doi: 10.1097/SAP.0000000000000981
Rojas, S., Pérez Del Caz, M. D., Esteban Vico, J. R., Villaverde, E., Llinas, A., Martinez, J. R., et al. (2017). EHTIC study: evaluation of a new hemostatic agent based on tissue factor in skin grafting procedures. Burns 43, 780–788. doi: 10.1016/j.burns.2017.01.007
Rose, L. F., and Chan, R. K. (2016). The burn wound microenvironment. Adv. Wound Care 5, 106–118. doi: 10.1089/wound.2014.0536
Rosenberg, L., Krieger, Y., Bogdanov-Berezovski, A., Silberstein, E., Shoham, Y., and Singer, A. J. (2014). A novel rapid and selective enzymatic debridement agent for burn wound management: a multi-center RCT. Burns 40, 466–474. doi: 10.1016/j.burns.2013.08.013
Rosenberg, L., Shoham, Y., Krieger, Y., Rubin, G., Sander, F., Koller, J., et al. (2015). Minimally invasive burn care: a review of seven clinical studies of rapid and selective debridement using a bromelain-based debriding enzyme (Nexobrid®). Ann. Burns Fire Disasters 28, 264–274.
Rosique, R. G., Rosique, M. J., and Farina Junior, J. A. (2015). Curbing inflammation in skin wound healing: a review. Int. J. Inflam. 2015:316235. doi: 10.1155/2015/316235
Roy, D. C., Tomblyn, S., Isaac, K. M., Kowalczewski, C. J., Burmeister, D. M., Burnett, L. R., et al. (2016). Ciprofloxacin-loaded keratin hydrogels reduce infection and support healing in a porcine partial-thickness thermal burn. Wound Repair Regen. 24, 657–668. doi: 10.1111/wrr.12449
Saeidinia, A., Keihanian, F., Lashkari, A. P., Lahiji, H. G., Mobayyen, M., Heidarzade, A., et al. (2017). Partial-thickness burn wounds healing by topical treatment: a randomized controlled comparison between silver sulfadiazine and centiderm. Medicine (Baltimore) 96:e6168. doi: 10.1097/MD.0000000000006168
Saffle, J. R. (2009). Closure of the excised burn wound: temporary skin substitutes. Clin. Plast. Surg. 36, 627–641. doi: 10.1016/j.cps.2009.05.005
Sanchez, D. A., Schairer, D., Tuckman-Vernon, C., Chouake, J., Kutner, A., Makdisi, J., et al. (2014). Amphotericin B releasing nanoparticle topical treatment of Candida spp. in the setting of a burn wound. Nanomedicine 10, 269–277. doi: 10.1016/j.nano.2013.06.002
Sanchez, J. L., Bastida, J. L., Martinez, M. M., Moreno, J. M., and Chamorro, J. J. (2008). Socio-economic cost and health-related quality of life of burn victims in Spain. Burns 34, 975–981. doi: 10.1016/j.burns.2007.12.011
Sander, E. A., Lynch, K. A., and Boyce, S. T. (2014). Development of the mechanical properties of engineered skin substitutes after grafting to full-thickness wounds. J. Biomech. Eng. 136:051008. doi: 10.1115/1.4026290
Sarabahi, S., Tiwari, V. K., Arora, S., Capoor, M. R., and Pandey, A. (2012). Changing pattern of fungal infection in burn patients. Burns 38, 520–528. doi: 10.1016/j.burns.2011.09.013
Sarrazy, V., Billet, F., Micallef, L., Coulomb, B., and Desmouliere, A. (2011). Mechanisms of pathological scarring: role of myofibroblasts and current developments. Wound Repair Regen. 19(Suppl. 1), S10–S15. doi: 10.1111/j.1524-475X.2011.00708.x
Scholz, C., and Wagner, E. (2012). Therapeutic plasmid DNA versus siRNA delivery: common and different tasks for synthetic carriers. J. Control. Release 161, 554–565. doi: 10.1016/j.jconrel.2011.11.014
Schultz, G. S., White, M., Mitchell, R., Brown, G., Lynch, J., Twardzik, D. R., et al. (1987). Epithelial wound healing enhanced by transforming growth factor-alpha and vaccinia growth factor. Science 235, 350–352. doi: 10.1126/science.3492044
Schwacha, M. G., Chung, C.-S., Ayala, A., Bland, K. I., and Chaudry, I. H. (2002). Cyclooxygenase 2-mediated suppression of macrophage interleukin-12 production after thermal injury. Am. J. Physiol. Cell Physiol. 282, C263–C270. doi: 10.1152/ajpcell.00357.2001
Sevgi, M., Toklu, A., Vecchio, D., and Hamblin, M. R. (2013). Topical antimicrobials for burn infections - an update. Recent Pat. Antiinfect. Drug Discov. 8, 161–197. doi: 10.2174/1574891X08666131112143447
Shander, A., Kaplan, L. J., Harris, M. T., Gross, I., Nagarsheth, N. P., Nemeth, J., et al. (2014). Topical hemostatic therapy in surgery: bridging the knowledge and practice gap. J. Am. Coll. Surg. 219, 570–579.e574. doi: 10.1016/j.jamcollsurg.2014.03.061
Sharma, S., Bajaj, D., and Sharma, P. (2016). Fungal infection in thermal burns: a prospective study in a tertiary care centre. J. Clin. Diagn. Res. 10, Pc05–Pc07. doi: 10.7860/JCDR/2016/20336.8445
Shen, Y. I., Song, H. G., Papa, A., Burke, J., Volk, S. W., and Gerecht, S. (2015). Acellular hydrogels for regenerative burn wound healing: translation from a porcine model. J. Invest. Dermatol. 135, 2519–2529. doi: 10.1038/jid.2015.182
Shoup, M., He, L.-K., Liu, H., Shankar, R., and Gamelli, R. (1998). Cyclooxygenase-2 inhibitor NS-398 improves survival and restores leukocyte counts in burn infection. J. Trauma Acute Care Surg. 45, 215–221. doi: 10.1097/00005373-199808000-00003
Singer, A. J., Choi, Y., Rashel, M., Toussaint, J., and McClain, S. A. (2017). The effects of topical nitric oxide on healing of partial thickness porcine burns. Burns. 44, 423–428. doi: 10.1016/j.burns.2017.07.017
Singer, A. J., and Clark, R. A. (1999). Cutaneous wound healing. N. Engl. J. Med. 341, 738–746. doi: 10.1056/NEJM199909023411006
Singer, A. J., Huang, S. S., Huang, J. S., McClain, S. A., Romanov, A., Rooney, J., et al. (2009). A novel TGF-beta antagonist speeds reepithelialization and reduces scarring of partial thickness porcine burns. J Burn Care Res. 30, 329–334. doi: 10.1097/BCR.0b013e31819a6369
Singh, R., and Chacharkar, M. P. (2011). Dried gamma-irradiated amniotic membrane as dressing in burn wound care. J. Tissue Viability 20, 49–54. doi: 10.1016/j.jtv.2010.06.001
Smith, P. D., Polo, M., Soler, P. M., McClintock, J. S., Maggi, S. P., Kim, Y. J., et al. (2000). Efficacy of growth factors in the accelerated closure of interstices in explanted meshed human skin grafts. J Burn Care Rehabil. 21(1 Pt 1), 5–9. doi: 10.1097/00004630-200021010-00003
So, K., McGrouther, D. A., Bush, J. A., Durani, P., Taylor, L., Skotny, G., et al. (2011). Avotermin for scar improvement following scar revision surgery: a randomized, double-blind, within-patient, placebo-controlled, phase II clinical trial. Plast. Reconstr. Surg. 128, 163–172. doi: 10.1097/PRS.0b013e318217429b
Sood, R., Roggy, D., Zieger, M., Balledux, J., Chaudhari, S., Koumanis, D. J., et al. (2010). Cultured epithelial autografts for coverage of large burn wounds in eighty-eight patients: the Indiana University experience. J. Burn Care Res. 31, 559–568. doi: 10.1097/BCR.0b013e3181e4ca29
Steffens, D., Leonardi, D., Soster, P. R., Lersch, M., Rosa, A., Crestani, T., et al. (2014). Development of a new nanofiber scaffold for use with stem cells in a third degree burn animal model. Burns 40, 1650–1660. doi: 10.1016/j.burns.2014.03.008
Steinstraesser, L., Trust, G., Rittig, A., Hirsch, T., Kesting, M. R., Steinau, H. U., et al. (2011). Colistin-loaded silk membranes against wound infection with Pseudomonas aeruginosa. Plast. Reconstr. Surg. 127, 1838–1846. doi: 10.1097/PRS.0b013e31820cf29a
Stekelenburg, C. M., Simons, J. M., Tuinebreijer, W. E., and van Zuijlen, P. P. (2016). Analyzing contraction of full thickness skin grafts in time: choosing the donor site does matter. Burns 42, 1471–1476. doi: 10.1016/j.burns.2016.02.001
Sterling, J. P., and Heimbach, D. M. (2011). Hemostasis in burn surgery–a review. Burns 37, 559–565. doi: 10.1016/j.burns.2010.06.010
Still, J., Glat, P., Silverstein, P., Griswold, J., and Mozingo, D. (2003). The use of a collagen sponge/living cell composite material to treat donor sites in burn patients. Burns 29, 837–841. doi: 10.1016/S0305-4179(03)00164-5
Storm-Versloot, M. N., Vos, C. G., Ubbink, D. T., and Vermeulen, H. (2010). Topical silver for preventing wound infection. Cochrane Database Syst Rev. CD006478. doi: 10.1002/14651858.CD006478.pub2
Struck, M. F., and Gille, J. (2013). Fungal infections in burns: a comprehensive review. Ann. Burns Fire Disasters 26, 147–153.
Stuermer, E. K., Lipenksy, A., Thamm, O., Neugebauer, E., Schaefer, N., Fuchs, P., et al. (2015). The role of SDF-1 in homing of human adipose-derived stem cells. Wound Repair Regen. 23, 82–89. doi: 10.1111/wrr.12248
Szpaderska, A. M., and DiPietro, L. A. (2005). Inflammation in surgical wound healing: friend or foe? Surgery 137, 571–573. doi: 10.1016/j.surg.2005.01.006
Tan, H., Wasiak, J., Paul, E., and Cleland, H. (2015). Effective use of Biobrane as a temporary wound dressing prior to definitive split-skin graft in the treatment of severe burn: a retrospective analysis. Burns 41, 969–976. doi: 10.1016/j.burns.2014.07.015
Taneja, N., Chari, P., Singh, M., Singh, G., Biswal, M., and Sharma, M. (2013). Evolution of bacterial flora in burn wounds: key role of environmental disinfection in control of infection. Int. J. Burns Trauma 3, 102–107.
Tenenhaus, M. (2017). The use of dehydrated human amnion/chorion membranes in the treatment of burns and complex wounds: current and future applications. Ann Plast Surg. 78(2 Suppl. 1), S11–S13. doi: 10.1097/SAP.0000000000000983
Tierney, E. P., Kouba, D. J., and Hanke, C. W. (2009). Review of fractional photothermolysis: treatment indications and efficacy. Dermatol Surg. 35, 1445–1461. doi: 10.1111/j.1524-4725.2009.01258.x
Tiwari, V. K. (2012). Burn wound: how it differs from other wounds? Indian J. Plast. Surg. 45, 364–373. doi: 10.4103/0970-0358.101319
Tschöp, J., Martignoni, A., Reid, M. D., Adediran, S. G., Gardner, J., Noel, G. J., et al. (2009). Differential immunological phenotypes are exhibited following scald and flame burns. Shock 31:157. doi: 10.1097/SHK.0b013e31817fbf4d
Tziotzios, C., Profyris, C., and Sterling, J. (2012). Cutaneous scarring: Pathophysiology, molecular mechanisms, and scar reduction therapeutics Part II. Strategies to reduce scar formation after dermatologic procedures. J. Am. Acad. Dermatol. 66, 13–24; quiz 25–16. doi: 10.1016/j.jaad.2011.08.035
van der Veer, W. M., Bloemen, M. C., Ulrich, M. M., Molema, G., van Zuijlen, P. P., Middelkoop, E., et al. (2009). Potential cellular and molecular causes of hypertrophic scar formation. Burns 35, 15–29. doi: 10.1016/j.burns.2008.06.020
Venter, N. G., Marques, R. G., Santos, J. S., and Monte-Alto-Costa, A. (2016). Use of platelet-rich plasma in deep second- and third-degree burns. Burns 42, 807–814. doi: 10.1016/j.burns.2016.01.002
Vinish, M., Cui, W., Stafford, E., Bae, L., Hawkins, H., Cox, R., et al. (2016). Dendritic cells modulate burn wound healing by enhancing early proliferation. Wound Repair Regen. 24, 6–13. doi: 10.1111/wrr.12388
Voigt, C. D., Williamson, S., Kagan, R. J., and Branski, L. K. (2018). “Chapter 14 - The skin bank,” in Total Burn Care, 5th Edn., ed D. Herndon (Galveston, TX; New York, NY: Elsevier), 158–166.
Waibel, J. S., Wulkan, A. J., and Shumaker, P. R. (2013). Treatment of hypertrophic scars using laser and laser assisted corticosteroid delivery. Lasers Surg. Med. 45, 135–140. doi: 10.1002/lsm.22120
Wang, X. Q., Chang, H. E., Francis, R., Olszowy, H., Liu, P. Y., Kempf, M., et al. (2009a). Silver deposits in cutaneous burn scar tissue is a common phenomenon following application of a silver dressing. J. Cutan. Pathol. 36, 788–792. doi: 10.1111/j.1600-0560.2008.01141.x
Wang, X. Q., Kempf, M., Mott, J., Chang, H. E., Francis, R., Liu, P. Y., et al. (2009b). Silver absorption on burns after the application of Acticoat: data from pediatric patients and a porcine burn model. J. Burn Care Res. 30, 341–348. doi: 10.1097/BCR.0b013e318198a64c
Wang, Y., Mithieux, S. M., Kong, Y., Wang, X. Q., Chong, C., Fathi, A., et al. (2015). Tropoelastin incorporation into a dermal regeneration template promotes wound angiogenesis. Adv. Healthc. Mater. 4, 577–584. doi: 10.1002/adhm.201400571
Wang, Y. W., Liou, N. H., Cherng, J. H., Chang, S. J., Ma, K. H., Fu, E., et al. (2014). siRNA-targeting transforming growth factor-beta type I receptor reduces wound scarring and extracellular matrix deposition of scar tissue. J. Invest. Dermatol. 134, 2016–2025. doi: 10.1038/jid.2014.84
Wasiak, J., Cleland, H., Campbell, F., and Spinks, A. (2013). Dressings for superficial and partial thickness burns. Cochrane Database Syst Rev. CD002106. doi: 10.1002/14651858.CD002106.pub4
Wasterlain, A. S., Braun, H. J., and Dragoo, J. L. (2012). Contents and formulations of platelet-rich plasma. Oper. Tech. Orthop. 22, 33–42. doi: 10.1053/j.oto.2011.11.001
Waymack, P., Duff, R. G., and Sabolinski, M. (2000). The effect of a tissue engineered bilayered living skin analog, over meshed split-thickness autografts on the healing of excised burn wounds. The Apligraf Burn Study Group. Burns 26, 609–619. doi: 10.1016/S0305-4179(00)00017-6
Werner, S., and Grose, R. (2003). regulation of wound healing by growth factors and cytokines. Physiol. Rev. 83, 835–870. doi: 10.1152/physrev.2003.83.3.835
Wood, F. M., Kolybaba, M. L., and Allen, P. (2006). The use of cultured epithelial autograft in the treatment of major burn wounds: eleven years of clinical experience. Burns 32, 538–544. doi: 10.1016/j.burns.2006.02.025
Wright, K. A., Nadire, K. B., Busto, P., Tubo, R., McPherson, J. M., and Wentworth, B. M. (1998). Alternative delivery of keratinocytes using a polyurethane membrane and the implications for its use in the treatment of full-thickness burn injury. Burns 24, 7–17. doi: 10.1016/S0305-4179(97)00075-2
Wurzer, P., Keil, H., Branski, L. K., Parvizi, D., Clayton, R. P., Finnerty, C. C., et al. (2016). The use of skin substitutes and burn care-a survey. J. Surg. Res. 201, 293–298. doi: 10.1016/j.jss.2015.10.048
Xie, H., Lucchesi, L., Zheng, B., Ladich, E., Pineda, T., Merten, R., et al. (2017). Treatment of burn and surgical wounds with recombinant human tropoelastin produces new elastin fibers in scars. J. Burn Care Res. 38, e859–e867. doi: 10.1097/BCR.0000000000000507
Yan, H., Chen, J., and Peng, X. (2012). Recombinant human granulocyte-macrophage colony-stimulating factor hydrogel promotes healing of deep partial thickness burn wounds. Burns 38, 877–881. doi: 10.1016/j.burns.2012.02.001
Yañez, R., Oviedo, A., Aldea, M., Bueren, J. A., and Lamana, M. L. (2010). Prostaglandin E2 plays a key role in the immunosuppressive properties of adipose and bone marrow tissue-derived mesenchymal stromal cells. Exp. Cell Res. 316, 3109–3123. doi: 10.1016/j.yexcr.2010.08.008
Yang, J. Y., Tsai, Y. C., and Noordhoff, M. S. (1989). Clinical comparison of commercially available Biobrane preparations. Burns 15, 197–203. doi: 10.1016/0305-4179(89)90183-6
Yim, H., Yang, H. T., Cho, Y. S., Seo, C. H., Lee, B. C., Ko, J. H., et al. (2011). Clinical study of cultured epithelial autografts in liquid suspension in severe burn patients. Burns 37, 1067–1071. doi: 10.1016/j.burns.2011.03.018
Zarei, F., Negahdari, B., and Eatemadi, A. (2018). Diabetic ulcer regeneration: stem cells, biomaterials, growth factors. Artif. Cells Nanomed. Biotechnol. 46, 26–32. doi: 10.1080/21691401.2017.1304407
Zhang, A., Wang, M. H., Dong, Z., and Yang, T. (2006). Prostaglandin E2 is a potent inhibitor of epithelial-to-mesenchymal transition: interaction with hepatocyte growth factor. Am. J. Physiol. Renal Physiol. 291, F1323–F1331. doi: 10.1152/ajprenal.00480.2005
Zhang, Y., Wang, T., He, J., and Dong, J. (2016). Growth factor therapy in patients with partial-thickness burns: a systematic review and meta-analysis. Int. Wound J. 13, 354–366. doi: 10.1111/iwj.12313
Zidan, S. M., Eleowa, S. A., Nasef, M. A., Abd-Almoktader, M. A., Elbatawy, A. M., Borhamy, A. G., et al. (2015). Maximizing the safety of glycerol preserved human amniotic membrane as a biological dressing. Burns 41, 1498–1503. doi: 10.1016/j.burns.2015.03.009
Zuccaro, J., Ziolkowski, N., and Fish, J. (2017). A systematic review of the effectiveness of laser therapy for hypertrophic burn scars. Clin. Plast. Surg. 44, 767–779. doi: 10.1016/j.cps.2017.05.008
Keywords: wound healing, burns, tissue engineering, biomaterials, drug delivery, growth factors, stem cells, regenerative medicine
Citation: Stone R II, Natesan S, Kowalczewski CJ, Mangum LH, Clay NE, Clohessy RM, Carlsson AH, Tassin DH, Chan RK, Rizzo JA and Christy RJ (2018) Advancements in Regenerative Strategies Through the Continuum of Burn Care. Front. Pharmacol. 9:672. doi: 10.3389/fphar.2018.00672
Received: 15 March 2018; Accepted: 05 June 2018;
Published: 09 July 2018.
Edited by:
Chris A Bashur, Florida Institute of Technology, United StatesReviewed by:
Heather Powell, The Ohio State University, United StatesAshley Carson Brown, North Carolina State University, United States
Copyright © 2018 Stone, Natesan, Kowalczewski, Mangum, Clay, Clohessy, Carlsson, Tassin, Chan, Rizzo and Christy. This is an open-access article distributed under the terms of the Creative Commons Attribution License (CC BY). The use, distribution or reproduction in other forums is permitted, provided the original author(s) and the copyright owner(s) are credited and that the original publication in this journal is cited, in accordance with accepted academic practice. No use, distribution or reproduction is permitted which does not comply with these terms.
*Correspondence: Robert J. Christy, cm9iZXJ0LmouY2hyaXN0eTEyLmNpdkBtYWlsLm1pbA==