- 1Department of Integrative Neurophysiology, Center for Neurogenomics and Cognitive Research, Neuroscience Amsterdam, Vrije Universiteit Amsterdam, Amsterdam, Netherlands
- 2Center for Neuroscience and Cell Biology, University of Coimbra, Coimbra, Portugal
- 3Portuguese National Institute of Legal Medicine and Forensic Sciences, Coimbra, Portugal
- 4Department of Neurosurgery, Neuroscience Amsterdam, VU University Medical Center Amsterdam, Amsterdam, Netherlands
- 5Faculty of Medicine, University of Coimbra, Coimbra, Portugal
Caffeine is the most widely used psychoactive drug, bolstering attention and normalizing mood and cognition, all functions involving cerebral cortical circuits. Whereas studies in rodents showed that caffeine acts through the antagonism of inhibitory A1 adenosine receptors (A1R), neither the role of A1R nor the impact of caffeine on human cortical neurons is known. We here provide the first characterization of the impact of realistic concentrations of caffeine experienced by moderate coffee drinkers (50 μM) on excitability of pyramidal neurons and excitatory synaptic transmission in the human temporal cortex. Moderate concentrations of caffeine disinhibited several of the inhibitory A1R-mediated effects of adenosine, similar to previous observations in the rodent brain. Thus, caffeine restored the adenosine-induced decrease of both intrinsic membrane excitability and excitatory synaptic transmission in the human pyramidal neurons through antagonism of post-synaptic A1R. Indeed, the A1R-mediated effects of endogenous adenosine were more efficient to inhibit synaptic transmission than neuronal excitability. This was associated with a distinct affinity of caffeine for synaptic versus extra-synaptic human cortical A1R, probably resulting from a different molecular organization of A1R in human cortical synapses. These findings constitute the first neurophysiological description of the impact of caffeine on pyramidal neuron excitability and excitatory synaptic transmission in the human temporal cortex, providing adequate ground for the effects of caffeine on cognition in humans.
Introduction
Coffee is the second most consumed beverage after water and its main constituent, caffeine, is the most widely consumed drug, improving attention and alertness, and normalizing mood and cognition (Fredholm et al., 1999; Smith, 2002). These central effects of caffeine result from the antagonism of adenosine receptors (Fredholm et al., 1999), in particular A1 receptors (A1R) and A2A receptors (A2AR), which are the main adenosine receptors in the brain (Fredholm et al., 2005). Because of its hydrophobic properties, brain concentrations of caffeine are similar to plasma concentrations (McCall et al., 1982; Fredholm et al., 1999), in the range of 20–70 μM upon moderate intake (Thithapandha et al., 1972; Kaplan et al., 1990; Costenla et al., 2010). These concentrations trigger the maximal psychostimulant effects of caffeine (Bruce et al., 1986). Careful consideration of caffeine concentration is important since most effects of caffeine are bell-shaped, being a psychostimulant with neuroprotective actions at moderate doses and a depressant with deleterious effects at higher doses (Rogers and Dernoncourt, 1998; Smith, 2002; Doepker et al., 2016).
Moderate doses of caffeine antagonize adenosine receptors (Daly et al., 1981; El Yacoubi et al., 2000; Karcz-Kubicha et al., 2003; Huang et al., 2005; Yang et al., 2009; Simões et al., 2016), whereas higher caffeine concentrations act on other targets such as the inhibition of phosphodiesterases, the modification of GABAA receptor function or the release of calcium from intracellular calcium stores (Lopez et al., 1989; McPherson et al., 1991; Fredholm et al., 1999; Boswell-Smith et al., 2006). These are likely associated with the toxic effects of caffeine (Yu et al., 2009). Since A1R are inhibitory, reducing excitatory synaptic transmission and neuronal excitability (Dunwiddie and Masino, 2001), whereas A2AR are mostly excitatory, facilitating synaptic plasticity processes (Cunha, 2016), it is assumed that the neurostimulant effect of caffeine mostly results from the partial antagonism of A1R whereas the neuroprotective effects of caffeine may results from limiting excessive A2AR activation (Ferré, 2008; Cunha, 2016). The role of A1R in the cerebral cortex has mostly been studied in rodents, where A1R are mostly located at synapses (Tetzlaff et al., 1987; Rebola et al., 2003), in particular at excitatory rather than at inhibitory synapses (e.g., Rombo et al., 2015). At excitatory synapses, A1R depress synaptic transmission and neuronal excitability through a combined presynaptic action decreasing glutamate release, post-synaptic actions decreasing the activation of glutamate receptors and voltage-sensitive calcium channels (de Mendonça et al., 1995; Klishin et al., 1995) as well as extra-synaptic actions through a decrease of neuronal excitability by controlling potassium rectifier channels (Greene et al., 1985), after-hyperpolarization potentials (Haas and Greene, 1984) and HCN (Li et al., 2011). Both in vivo and in vitro studies in rodents concluded that the inhibitory effect of A1R predominantly results from the presynaptic inhibition of glutamate release (Phillis et al., 1979; Thompson et al., 1992).
Despite the clear effects of caffeine in human subjects on cortical regions resulting in alterations of vigilance, mood, and cognition (Smith, 2002; Doepker et al., 2016), the functional impact of caffeine on neuronal excitability and information flow in the human cerebral cortex is not yet characterized. On a molecular level, moderate doses of caffeine affect A1R binding (Boulenger et al., 1982) and occupancy in human cortical neurons (Elmenhorst et al., 2012; Paul et al., 2014). While studies in rodents revealed an ability of caffeine to partially antagonize some A1R-mediated effects (Phillis et al., 1979; Qi et al., 2016), responses in the human cortical network might differ as A1R have clear inter-species differences, typified by a lower density (Boulenger et al., 1982; Dodd et al., 1986; Fastbom et al., 1987; Svenningsson et al., 1997), a higher affinity for agonists and a lower affinity for antagonists in human versus rodent cerebral cortex (Murphy and Snyder, 1982; Ferkany et al., 1986; Maemoto et al., 1997). Therefore, we here delineate the synaptic and subsynaptic localization of A1R in the human cerebral cortex, and test how A1R affect neuronal excitability and excitatory synaptic transmission. We report how caffeine at realistic concentrations reached in the brain parenchyma and experienced by coffee consumers after 1–2 cups of coffee, affects these neuronal and synaptic A1R actions in human neocortex.
Materials and Methods
Human Samples
All procedures on human brain resection material that had to be removed for the surgical treatment of deeper brain structures were performed with the approval of the Medical Ethical Committee of the VU University Medical Centre, written informed consent by patients involved, and in accordance with Dutch license procedures and the declaration of Helsinki, as previously described (Verhoog et al., 2016). Human brain samples were also collected at autopsies, performed at the Instituto Nacional de Medicina Legal e Ciências Forenses, which approved all procedures according to the rules of the European Consortium of Nervous Tissues: BrainNet Europe II, to protect the identity of individual donors, as previously described (Pliássova et al., 2016).
Membrane Preparation and Binding Assays
Total membranes and synaptic membranes (from a synaptosomal preparation) were obtained by isopicnic and gradient centrifugations of homogenized brain tissue, as previously described (Rebola et al., 2005; Pliássova et al., 2016). To determine the enrichment and basic binding characteristics of A1R in cortical synapses, we compared saturation binding isotherms of the selective A1R antagonist 3H-DPCPX (0.1–10 nM; specific activity of 102.1 Ci/mmol; from DuPont NEN) in total and synaptosomal membranes (72–164 μg) incubated for 2 h incubation at room temperature in a buffer containing 50 mM Tris, 1 mM EDTA, 2 mM EGTA, pH 7.4, with adenosine deaminase (4 U/ml, Roche) before filtration through Whatman GF/C filters (Millipore), as previously described (Rebola et al., 2003; Coelho et al., 2006). To estimate the binding affinity of caffeine, we carried out displacement curves of 3H-DPCPX binding with caffeine (0.1–300 μM; from Sigma), as previously described (Coelho et al., 2006). Results are expressed as specific binding, determined by subtraction of the non-specific binding, which was measured in the presence of 2 μM 8-{4-[(2-aminoethyl)amino]carbonylmethyloxyphenyl}xanthine (XAC, a mixed A1R/A2AR antagonist; from Tocris) and normalized per amount of protein (bicinchoninic acid assay). To derive the binding parameters from saturation curves (KD and Bmax values) the data were fitted by a rectangular hyperbola using the GraphPad Prism software. For displacement binding curves, IC50 values were converted to Ki values by non-linear fitting of the semi-logarithmic curves derived from the competitions curves.
Subsynaptic Fractionation and Western Blot Analysis
To separate the extrasynaptic (non-active) zone, presynaptic active zone and post-synaptic fractions from synaptosomes, we used a fractionation method previously described in detail (Rebola et al., 2005; Canas and Cunha, 2016). The efficiency of separation is based on the segregation of different markers in the several fractions: SNAP-25 in the presynaptic active zone, PSD-95 in the post-synaptic density and synaptophysin outside the active zone (extrasynaptic fraction). Western blot analysis was performed with a rabbit anti-A1R antibody (1:500, Thermo Scientific), as previously described (Rebola et al., 2003).
Human Brain Slice Preparation and Electrophysiological Recordings
Human brain slices were derived from resected tissue obtained from patients suffering from mild-to severe forms of epilepsy (n = 6, of which 5 were diagnosed with meso-temporal epilepsia, 1 with dysplasia; average age 44.8 ± 10.0). All obtained tissue is derived from the temporal lobe area (n = 6), away from the focal area of the epilepsy. Slices were prepared as described previously (Testa-Silva et al., 2014; Verhoog et al., 2016). Briefly, blocks of resected cortical tissue were transported to the laboratory in carbogen-saturated (95% O2, 5% CO2 at pH 7.4) ice-cold choline-based slicing solution containing (in mM): 110 choline chloride, 11.6 sodium ascorbate, 2.5 KCl, 1.3 NaH2PO4, 7 MgCl2, 0.5 CaCl2, 26 NaHCO3, 10 glucose. Cortical slices (350–400 μm) were prepared in the same ice-cold solution as used for transport, and then transferred to holding chambers with artificial cerebrospinal fluid (aCSF) containing (in mM) 125 NaCl, 3 KCl, 1.25 NaH2PO4, 1 MgSO4, 2 CaCl2, 26 NaHCO3, 10 glucose. Here, they were stored for 30 min at 34°C and subsequently at room temperature for at least 1 h before recording.
Following recovery, recordings from cells in deeper layers of the human temporal cortex were made in oxygenated aCSF (flow rate of 2–3 ml/min, 32°C). Whole-cell patch-clamp recordings were made with borosilicate glass pipettes (3–6 MΩ) filled with an intracellular solution containing (in mM): 111 K-gluconate, 8 KCl, 10 HEPES, 4 Mg-ATP, 10 K2HPO4, 0.4 GTP, 0.2 EGTA. Biocytin (0.5%) was added to all solutions for post hoc cell identification, and osmolarity was adjusted to 290–295 mOsm. Pyramidal neurons were visualized with differential interference contrast microscopy, selected based on their large and pyramidal shape and further identified by their spike profile. During recordings, cells were kept at a holding potential close to -70 mV. Recordings were made using MultiClamp 700 A/B amplifiers (Axon Instruments, Sunnyvale, CA, United States), sampling at 10 kHz and low-pass filtering at 3–4 kHz. Recordings were digitized with an Axon Digidata 1440A and acquired using pClamp software (Axon). Acquired data were stored for off-line analysis.
To characterize the electrophysiological properties of pyramidal neurons, we used a step protocol to calculate the input resistance (Rin) as the slope of the linear fit through the current–voltage relationship in 10 pA steps. The membrane time constant was obtained by fitting a single exponential function to the membrane potential deflection in response to a -50 pA current injection. The sag was calculated as the percentage of the difference between transient and stable membrane potentials to a hyperpolarization amplitude of -10 mV after injecting a negative current. The rheobase was defined as the minimal current amplitude of 1 s duration that resulted in the first action potential (AP). Analysis of spike waveforms was performed on single APs elicited by depolarizing threshold current pulses. The AP half-width was defined as the spike width at its half amplitude. To study synaptic transmission, we evaluated spontaneous events that were detected using MiniAnalysis software. The average amplitude and frequency of excitatory post-synaptic potentials (EPSPs) was determined over a time span of 5 min.
The tested drugs, adenosine (20 μM, Sigma), caffeine (50 μM, Sigma) or DPCPX (100 nM, Tocris), were used in concentrations previously tested in rodent preparations (Cunha et al., 1998; Sebastião et al., 2000; Costenla et al., 2010) and were dissolved in aCSF at the desired concentration and bath applied during the experiments.
Morphological Analysis
After recording, slices were fixed at 4°C for at least 24 h in 100 mM phosphate buffer saline containing 4% paraformaldehyde (pH 7.4), for subsequent neuronal visualization and reconstruction as previously described (Mohan et al., 2015). Slices containing biocytin-filled neuronal pairs were processed using a protocol described previously (Mohan et al., 2015). Slices were incubated in 0.1% Triton X-100 solution containing avidin biotinylated horseradish peroxidase (ABC-Elite; Camon); subsequently, they were reacted using 3,3-diaminobenzidine as a chromogen under visual control until the dendritic and axonal arborization was clearly visible (usually after 2–4 min). Slices were dehydrated and then mounted on slides, embedded in Mowiol and enclosed with a thin coverslip.
Biocytin-labeled neurons were examined under the light microscope. Representative pairs were photographed at low magnification to document the dendritic and axonal arborization. Subsequently, neurons were reconstructed with the aid of Neurolucida software (MicroBright Field).
Statistical Analysis
Data are means ± SEM. Paired Student’s t-test or Wilcoxon signed-rank test (n < 10) was used for statistical comparisons between two groups and one-way ANOVA followed by post hoc Tukey’s test for statistical comparisons among multiple groups. The normality of the distribution of values was determined by the Kolmogorov–Smirnov test. Statistical significance was set at p < 0.05.
Results
Synaptic Localization of A1R in the Human Cerebral Cortex
To define if A1R were enriched in synapses of the human cerebral cortex, as occurs in rodents (Tetzlaff et al., 1987; Rebola et al., 2003), we compared the binding density of the previously validated selective A1R antagonist 3H-DPCPX (Svenningsson et al., 1997) in membranes from synaptosomes (purified synapses) and in total membranes (mostly representing non-synaptic neuronal and astrocytic membranes) from human neocortical tissue. Figure 1A shows that the binding density of A1R was larger in synaptosomal membranes (Bmax = 755 ± 22 fmol/mg protein, n = 5) than in total membranes (Bmax = 421 ± 17 fmol/mg protein, n = 5; p < 0.001 versus synaptosomal membranes; unpaired Student’s t-test).
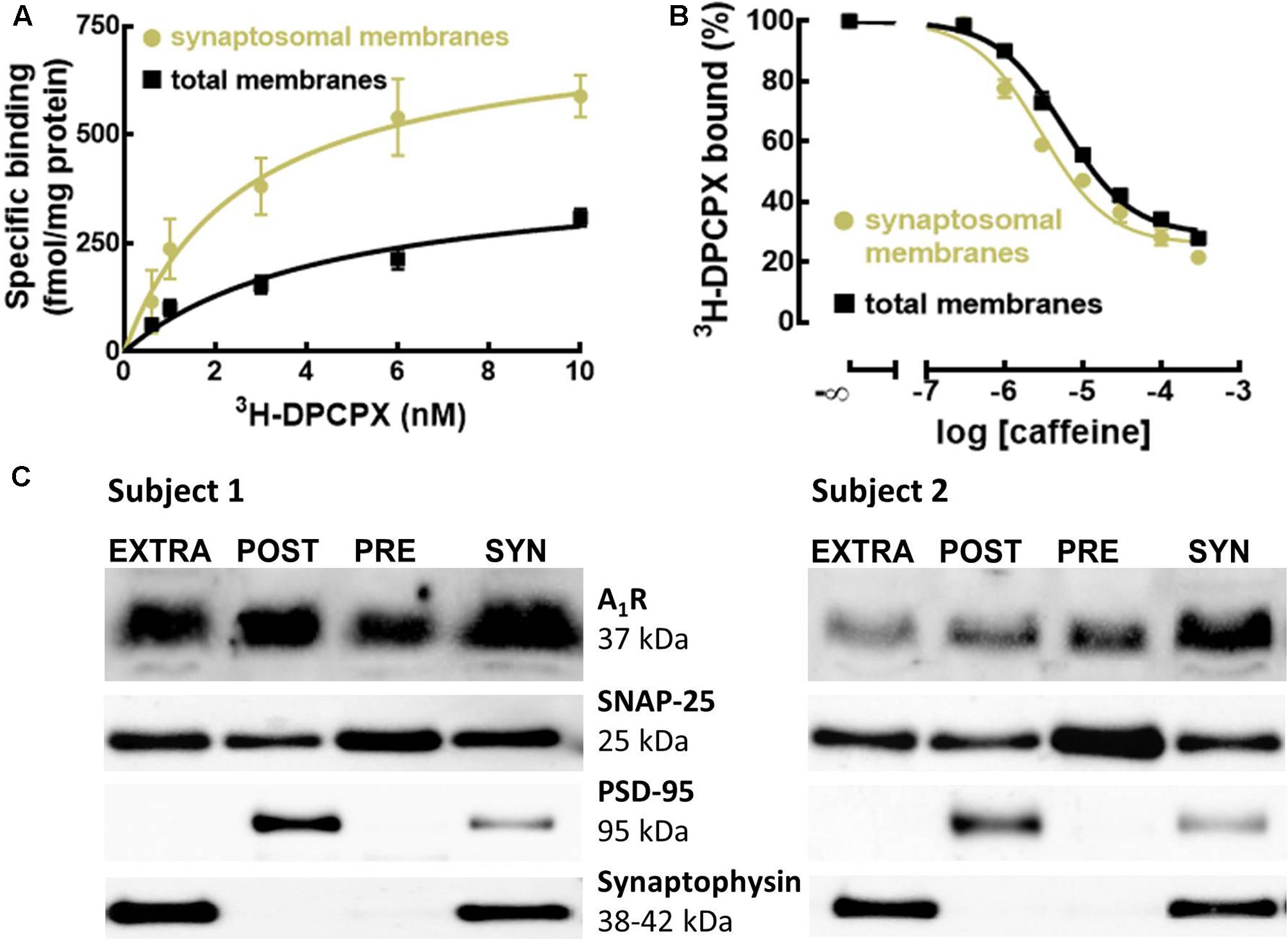
FIGURE 1. A1R are enriched and evenly distributed in human cortical synapses, and have greater affinity for caffeine and selective A1R antagonist compared to extra-synaptic A1R. (A) The selective A1R antagonist 3H-DPCPX binds with greater affinity and to a larger number of A1R in synaptosomal compared to total membranes of the human temporal cortex. Data are mean ± SEM of five subjects. (B) Caffeine has a greater affinity to displace the binding of the selective A1R antagonist 3H-DPCPX (2 nM) from synaptosomal compared to total membranes of the human temporal cortex. Data are mean ± SEM of five subjects. (C) Western blots of subsynaptic fractions prepared from synaptosomes (SYN) showing the subsynaptic distribution of A1R in the human temporal cortex (n = 2). A1R are present in all the subsynaptic fractions, outside (EXTRA) and inside the presynaptic active zone (PRE) and post-synaptic density (POST).
Interestingly, 3H-DPCPX displayed a higher affinity to bind A1R in synaptosomal membranes (KD = 2.68 nM, 95% confidence interval: 2.12–3.24 nM, n = 5) than in total membranes (KD = 4.58 nM, 95% confidence interval: 3.69–5.46 nM, n = 5; p = 0.0079 versus synaptosomal membranes; unpaired Student’s t-test). These different binding properties of synaptic versus extra-synaptic A1R in the human cerebral cortex were confirmed by the different apparent affinity of caffeine for these two populations of A1R. In fact, competition curves of 3H-DPCPX binding by caffeine (a non-selective adenosine receptor antagonist; Daly et al., 1981) showed that caffeine displaced 3H-DPCPX binding with greater efficiency in synaptosomal membranes (Ki = 11.53 μM, 95% confidence interval: 7.89–15.17 μM, n = 5) than in total membranes (Ki = 33.02 μM, 95% confidence interval: 25.18–40.86 μM, n = 5; p = 0.0079 versus synaptosomal membranes; unpaired Student’s t-test) (Figure 1B). This is suggestive of a possible difference in human cerebral cortical synaptic and extra-synaptic membranes of the homo- or heterodimerization of A1R, which has been shown to affect the binding of caffeine and A1R antagonists (Ciruela et al., 2006; Gracia et al., 2013).
We further detailed the localization of A1R within human cerebral cortical synapses. Using different subsynaptic fractions prepared from synaptosomes of the human temporal cortex, we concluded that A1R are present in all the subsynaptic fractions on somatodendritic (post-synaptic) and axon terminal (presynaptic) compartments, inside and outside the presynaptic active zone and post-synaptic density (Figure 1C and Supplementary Figure S1). Such a widespread distribution predicts a complex role for the A1R in the control of synaptic communication in the human cerebral cortex.
Adenosine Effects on Intrinsic Membrane Properties of Pyramidal Neurons Are Mediated by A1R
Adenosine, at non-toxic concentrations (i.e., in low μM range), is known to affect intrinsic membrane properties of layers 2–3 and layer 5 pyramidal neurons in the rodent cerebral cortex through the activation of A1R (Phillis et al., 1979; van Aerde et al., 2015). Since A1R activation also controls excitability in the human brain (Klaft et al., 2016) by still undefined mechanisms, we first characterized the effect of exogenous adenosine application on membrane properties of pyramidal neurons in the human temporal cortex (Figure 2A). On average, adenosine (20–100 μM) did not decrease the resting membrane potential (RMP; control: -67.2 ± 2.3 mV; adenosine: -67.2 ± 3.4 mV; difference: 0.01 ± 3.7 mV, n = 12, paired t-test: p = 0.99), while it did lower the input resistance (Rinput; control: 45.9 ± 16.7 MΩ; adenosine: 40.4 ± 14.5 MΩ; difference: 5.5 ± 6.5 MΩ, n = 12, paired t-test: p = 0.01; Figures 2C,F) and increased the current injection needed before firing of the first AP, expressed as the rheobase value (Rheobase; control: 251.7 ± 130.5 pA; adenosine: 344.5 ± 149.9 pA; difference: 92.8 ± 45.0 pA, n = 12, paired t-test: p < 0.0001; Figures 2B,E). Altogether these changes lead to a decreased neuronal excitability and a need for a larger input to fire an AP. Furthermore, adenosine decreased the voltage sag (Sag; control: 2.1 ± 1.2 mV; adenosine: 1.6 ± 1.1 mV; difference: 0.45 ± 0.44 mV, n = 12, paired t-test: p = 0.003; Figures 2D,G), reflecting an adenosine-induced partial block of HCN channels, as described in rodents (Li et al., 2011). These effects of adenosine were not seen at 5 μM and were concentration-dependent in the range of 20–100 μM.
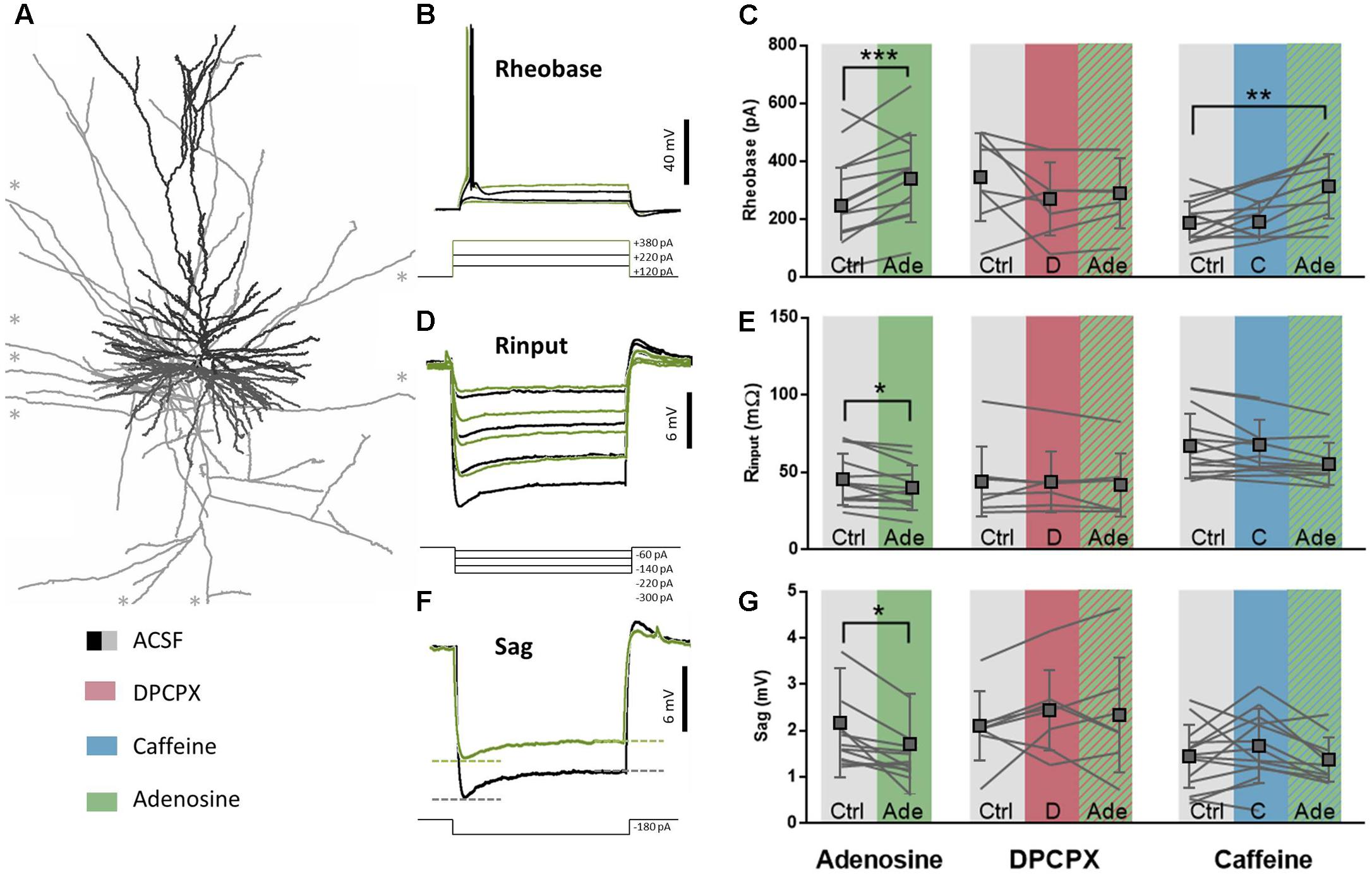
FIGURE 2. A1R-mediated effects on intrinsic membrane properties are moderately affected by caffeine. (A) Biocytin reconstruction of deeper layer pyramidal neuron from slice of human temporal cortex showing dendritic (black) and axonal (gray) branches. ∗Axonal branches stretch longer than could be depicted here. (B,E) Rheobase value of pyramidal neurons increased after adenosine application (20–100 μM, green, E, left panel). This effect was blocked by pre-incubation of DPCPX (100 nM, red, E, middle panel), but still occurred when caffeine was pre-incubated (50 μM, blue, E, right panel). Paired t-test comparing adenosine (20–100 μM) to ACSF condition: ∗∗p < 0.01; ∗∗∗p < 0.001. Boxes depict average ± SD. (C,F) Input resistance of pyramidal neurons decreased after adenosine application (20–100 μM, F, left panel). Both DPCPX (100 nM, red, F, middle panel) and caffeine (50 μM, F, blue, right panel) inhibited adenosine-induced effects (striped green). Paired t-test comparing adenosine (20–100 μM) to ACSF condition: ∗p < 0.05. Boxes depict average ± SD. (D,G) The sag of hyperpolarization-activated cationic depolarizing current (Ih) decreased after adenosine application (20–100 μM, G, left panel). Both DPCPX (100 nM, red, G, middle panel) and caffeine (50 μM, blue, G, right panel) inhibited adenosine-induced effects (striped green). Paired t-test comparing adenosine (20–100 μM) to ACSF condition: ∗p < 0.05. Boxes depict average ± SD.
The effects of adenosine on membrane intrinsic properties were due to A1R activation, since the selective A1R antagonist DPCPX (100 nM) blocked the effects of adenosine on the rheobase (DPCPX: 275.0 ± 125.5 pA; DPCPX+adenosine: 293.6 ± 120.4 pA; difference: 16.4 ± 25.0 pA, n = 7, paired t-test: p = 0.13; Figures 2B,E), input resistance (DPCPX: 42.2 ± 20.5 MΩ; DPCPX+adenosine: 33.0 ± 12.9 MΩ; difference: 2.2 ± 5.5 MΩ, n = 7, paired t-test: p = 0.33; Figures 2C,F), and sag (DPCPX: 2.3 ± 1.2 mV; DPCPX+adenosine: 2.5 ± 0.6 mV; difference: 0.1 ± 0.6 mV, n = 7, paired t-test: p = 0.72; Figures 2D,G). By itself, DPCPX (100 nM) did not significantly alter these membrane properties: rheobase (control: 350.0 ± 151.2 pA; DPCPX: 275.0 ± 125.5 pA; difference 75.0 ± 133.8 pA, n = 8, paired t-test: p = 0.16; Figures 2B,E), Input resistance (control: 44.4 ± 22.7 MΩ; DPCPX: 42.2 ± 20.5 MΩ; difference: 0.2 ± 5.8 MΩ, n = 8, paired t-test: p = 0.92; Figures 2C,F) or sag (control: 2.1 ± 0.7 mV; DPCPX: 2.3 ± 1.2 mV; difference: 0.3 ± 0.6 mV, n = 8, paired t-test: p = 0.17; Figures 2D,G). This indicates the lack of a constitutive effect of endogenous adenosine on the A1R controlling membrane intrinsic properties of pyramidal neurons in human cortical slices.
Caffeine Does Not Modify Intrinsic Membrane Properties of Pyramidal Neurons
As with DPCPX, there were no effects of caffeine (50 μM) by itself on intrinsic membrane properties of pyramidal neurons in human temporal-cortical slices. The rheobase (control: 192.9 ± 73.4 pA; caffeine: 196.4 ± 69.8 pA; difference 18.2 ± 72.9 pA, n = 11, paired t-test: p = 0.42; Figures 2B,E), input resistance (control: 67.5 ± 21.0 MΩ; caffeine: 68.2 ± 16.3 MΩ; difference: 4.1 ± 10.3 MΩ, n = 11, paired t-test: p = 0.21; Figures 2C,F) and sag were unaltered (control: 1.4 ± 0.7 mV; caffeine: 1.6 ± 0.8 mV; difference: 0.3 ± 0.6 mV, n = 11, paired t-test: p = 0.12; Figures 2D,G). Since the selective A1R antagonist DPCPX had no effect by itself and thereby ruled out constitutive activation of A1R in human cortical slices, the lack of a direct effect of caffeine on intrinsic membrane properties suggests that A2AR are also not constitutively altering intrinsic membrane properties in human pyramidal neurons in vitro (reviewed in Cunha, 2016).
We next tested if concentrations of caffeine reached in the brain parenchyma by moderate coffee drinkers (50 μM) could effectively antagonize the A1R-mediated control of the intrinsic membrane properties of pyramidal neurons. The effect of exogenously applied adenosine on the rheobase was still observed in the presence of caffeine (50 μM). Thus, when adenosine (20 μM) was added in the presence of caffeine (50 μM), the rheobase increased significantly (control: 192.9 ± 73.4 pA; caffeine+adenosine: 318.2 ± 110.8 pA; difference: 116.4 ± 108.7 pA, n = 11, paired t-test: p = 0.005; Figures 2B,E). In contrast, caffeine partially blocked the effects of adenosine on the input resistance (control: 67.5 ± 21.0 MΩ; caffeine+adenosine: 55.8 ± 13.6 MΩ; difference: 4.1 ± 10.3 MΩ, n = 11, paired t-test: p = 0.21; Figures 2C,F) and voltage sag (control: 1.4 ± 0.7 mV; caffeine+adenosine: 1.3 ± 0.5 mV; difference: 0.3 ± 0.7 mV, n = 11, paired t-test: p = 0.12; Figures 2D,G). This indicates that caffeine concentrations experienced by coffee drinkers can interfere with effects of adenosine on the intrinsic membrane properties of pyramidal neurons in human neocortex.
Adenosine Controls Synaptic Transmission through A1R
The impact of caffeine on cerebral cortical-mediated behavioral responses may also result from its ability to control glutamatergic synaptic transmission, as observed in rodents (Phillis et al., 1979; Thompson et al., 1992; Costenla et al., 2010; Qi et al., 2016). To evaluate the effect of caffeine on glutamatergic synaptic transmission in the human neocortex, we made whole-cell recordings of large deeper-layer pyramidal neurons of the human temporal cortex and recorded spontaneous excitatory transmission. Adenosine (20 μM) significantly decreased both the amplitude of excitatory events (control: 41.7 ± 10.6 pA; adenosine: 38.1 ± 6.6 pA; difference: 3.9 ± 4.9 pA, n = 10, paired t-test: p = 0.03, Figures 3D,E) as well as the frequency of excitatory events (control: 1.8 ± 1.6 Hz; adenosine: 0.6 ± 0.5 Hz; difference: 1.2 ± 1.4 Hz; n = 10, paired t-test: p = 0.02; Figure 3A). Both effects were reversible upon washout of adenosine (Figure 3E; EPSC amplitude: wash-out: 43.6 ± 12.2 pA; difference vs. adenosine: 5.8 ± 6.6 pA; n = 10, paired t-test: p = 0.02; EPSC frequency: wash-out: 2.8 ± 2.2 Hz; difference vs. adenosine: 2.1 ± 1.8 Hz; n = 9, paired t-test: p = 0.009). The effects of adenosine were mediated by A1R, since the selective A1R antagonist DPCPX (100 nM) blunted the effect of exogenously added adenosine (20 μM) on frequency (control: 2.0 ± 1.8 Hz; DPCPX+adenosine: 1.9 ± 1.2 Hz; difference: 0.1 ± 0.9 Hz; n = 6, paired t-test: p = 0.77; Figure 3B) and amplitude of excitatory post-synaptic events (control: 41.8 ± 12.9 pA; DPCPX+adenosine: 51.7 ± 30.0 pA; difference: 9.7 ± 17.2 pA, n = 7, paired t-test: p = 0.18; Figure 3F). DPCPX (100 nM) by itself increased the frequency (control: 2.0 ± 1.8 Hz; DPCPX: 3.0 ± 2.0 Hz; difference: 1.1 ± 0.6 Hz; n = 6, paired t-test: p = 0.01; Figure 3B) but not the amplitude of excitatory synaptic events (control: 41.8 ± 12.9 pA; DPCPX: 49.8 ± 24.9 pA; difference: 8.0 ± 13.0 pA, n = 8, paired t-test: p = 0.13; Figure 3F), which may suggest a constitutive activity of presynaptic A1R inhibiting synaptic release of glutamate.
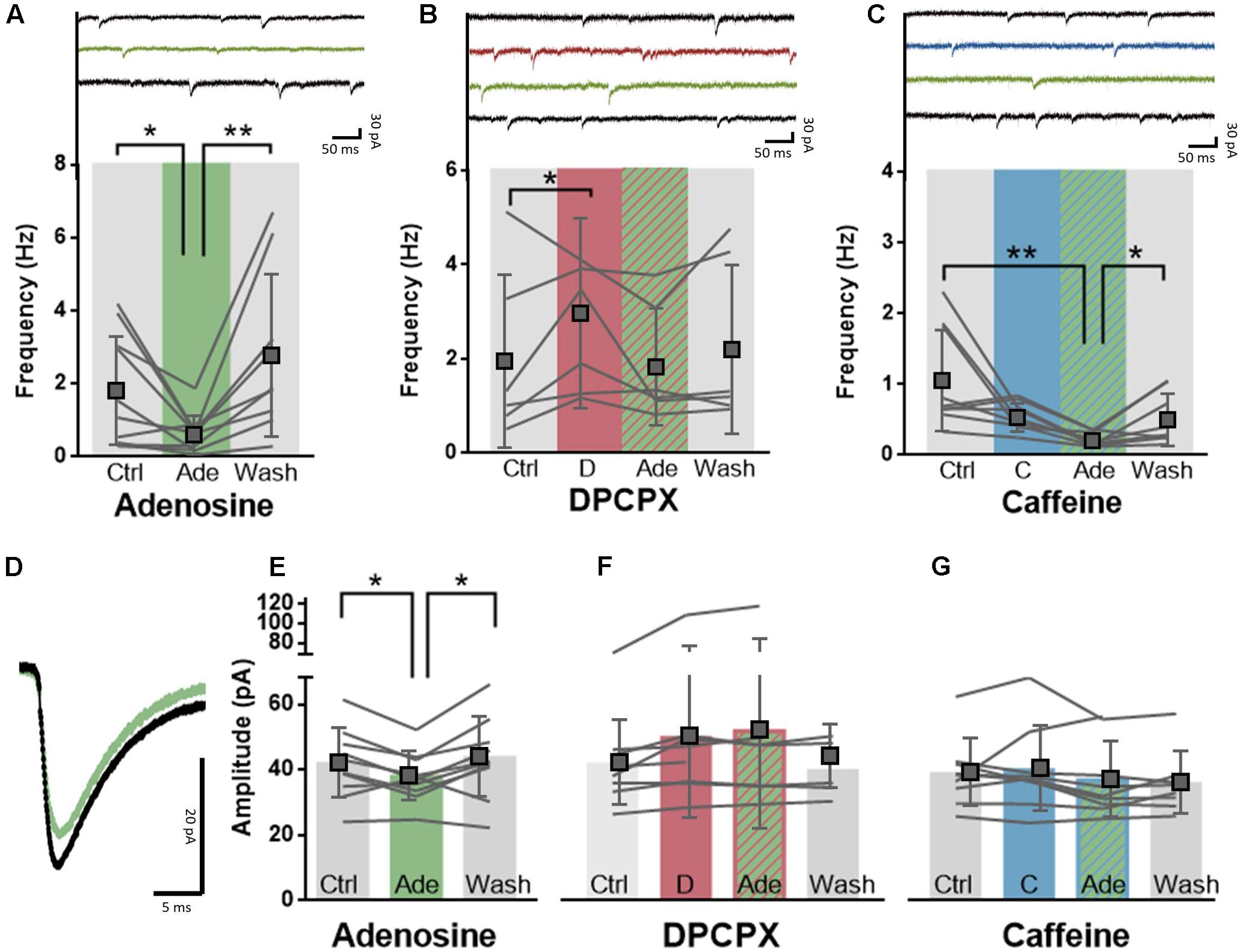
FIGURE 3. A1R-mediated effects of adenosine on synaptic transmission are post-synaptically blocked by caffeine. (A) Adenosine (20 μM, green) significantly decreased the frequency of excitatory events onto pyramidal neurons in a reversible manner. Paired t-test comparing adenosine (20 μM) to ACSF condition: ∗p < 0.05; ∗∗p < 0.01. Boxes depict average ± SD. (B) Direct application of DPCPX (100 nM, red) significantly increased the frequency of excitatory events and blocked adenosine-induced inhibition (green). Paired t-test comparing DPCPX (100 nM) to ACSF condition: ∗p < 0.05. Boxes depict average ± SD. (C) Caffeine pre-incubation (50 μM, blue) did not affect the potency of adenosine (20 μM, green) to decrease the frequency of excitatory events onto pyramidal neurons. Wash-out of adenosine and caffeine restored the frequency of events. Paired t-test comparing adenosine (20 μM) to ACSF condition: ∗p < 0.05; ∗∗p < 0.01. Boxes depict average ± SD. (D) Example trace of the average effect of adenosine application onto the amplitude of excitatory events onto a pyramidal neuron. (E–G) Adenosine (20 μM, green) significantly decreased the amplitude of excitatory events onto pyramidal neurons in a reversible manner (E). Pre-incubation with either DPCPX (100 nM, red, F) or caffeine (50 μM, blue, G) completely abolished this effect. Paired t-test comparing adenosine (20 μM) to ACSF control/wash-out condition: ∗p < 0.05.
We next investigated the effect of caffeine concentrations experienced by most coffee drinkers (50 μM) on synaptic transmission in the presence of adenosine. Caffeine (50 μM) blocked the effect of adenosine (20 μM) on the amplitude of spontaneous excitatory events which indicates a post-synaptic effect of caffeine (control: 38.9 ± 10.3 pA; caffeine+adenosine: 36.7 ± 11.5 pA; difference: 2.1 ± 8.9 pA, n = 9, paired t-test: p = 0.49; Figure 3G), but did not prevent the effect of adenosine on the frequency of spontaneous events (control: 1.1 ± 0.7 Hz; caffeine+adenosine: 0.2 ± 0.1 Hz; difference: 0.9 ± 0.7 Hz; n = 9, paired t-test: p = 0.007; Figure 3C). Similar to the adenosine-only group, effects of adenosine in the case of pre-incubated caffeine could be washed out (wash-out: 0.5 ± 0.4 pA; difference vs. adenosine: 0.3 ± 0.3 pA; n = 8, paired t-test: p = 0.04; Figure 3C). In contrast to DPCPX, caffeine (50 μM) by itself had no effect on spontaneous glutamatergic synaptic transmission. It did not modify the amplitude (control: 38.9 ± 10.3 pA; caffeine: 40.1 ± 12.9 pA; difference: 1.1 ± 0.6 pA, n = 9, paired t-test: p = 0.59; Figure 3G) or frequency of spontaneous excitatory post-synaptic events (control: 1.1 ± 0.7 Hz; caffeine: 0.6 ± 0.2 Hz; difference: 0.5 ± 0.7 Hz; n = 9, paired t-test: p = 0.06; Figure 3C). This may suggest that, in contrast to DPCPX, moderate concentrations of caffeine are not sufficient to interfere with constitutive activity of presynaptic A1R. Together, our findings show that caffeine concentrations experienced by coffee drinkers interfere with adenosine signaling when there is an additional adenosine load, allowing to restore excitatory synaptic transmission and pyramidal neuron excitability in the human neocortex.
Discussion
In this study, we provide the first characterization of the impact of caffeine concentrations experienced by moderate coffee drinkers (50 μM) on neuronal excitability in pyramidal neurons and excitatory synapses of the human temporal cortex. Caffeine had a larger affinity for synaptic A1R compared to extra-synaptic A1R in the human temporal cortex, which contrasts with the affinity profile of caffeine in the rodent cortex; this difference probably results from a different molecular organization of A1R within human cortical synapses compared to rodent cortical synapses. Caffeine interfered with several of the inhibitory A1R-mediated effects of adenosine in the human cortex, similar to previous observations in the rodent brain. Caffeine blunted the effects of adenosine on intrinsic membrane properties and prevented the amplitude reduction of excitatory synaptic transmission through antagonistic actions at post-synaptic A1R.
A distinct interaction of caffeine with A1R in the human cerebral cortex compared with rodent cortex is in agreement with several previous studies describing differences of binding properties of brain A1R between humans and different animal models (Boulenger et al., 1982; Murphy and Snyder, 1982; Dodd et al., 1986; Ferkany et al., 1986; Fastbom et al., 1987; Maemoto et al., 1997; Svenningsson et al., 1997). Our results confirmed previous evidence that the binding density of A1R in the temporal cortex is lower in humans compared to mice or rats (Boulenger et al., 1982; Dodd et al., 1986; Fastbom et al., 1987; Svenningsson et al., 1997). We also confirmed previous evidence that human A1R display a different affinity for xanthine-based selective antagonists, which tend to have lower affinity in humans compared to rodents (Murphy and Snyder, 1982; Ferkany et al., 1986; Maemoto et al., 1997). Despite these different pharmacological properties, human A1R seem to exert an overall inhibitory effect in the cerebral cortex that is similar to that observed in rodents: in fact, the exogenous administration of adenosine decreased the excitability of pyramidal neurons in the human temporal cortex in a manner similar to the inhibition previously reported to occur through A1R activation in the cerebral cortex of rodents (Phillis et al., 1979; Li et al., 2011). In rodents (Thompson et al., 1992), tonic adenosine inhibition is dominated by a A1R-mediated inhibition of excitatory transmission. In human neocortex, both intrinsic membrane properties of pyramidal neurons as well as excitatory synaptic transmission received by these neurons, were inhibited by somatodendritic post-synaptic A1R. Whether one of these mechanisms dominates effects of adenosine on cortical overall excitability in human neocortex cannot be concluded based on our present results. We did find a high density of A1R in human cortical synapses, both at somatodendritic (post-synaptic) and axon terminals (presynaptic) compartments, as well as a greater affinity for DPCPX of synaptic A1R in comparison with extra-synaptic A1R, which may point to a stronger control of synaptic transmission by A1R. Since one of the few factors that has been documented to regulate the affinity of A1R for antagonists is their relative homomerization (Gracia et al., 2013) or heteromerization (Ciruela et al., 2006), the present findings are suggestive of a different molecular arrangement of A1R in synapses of the human cerebral cortex.
To characterize the impact of caffeine on human cortical neurons, we used a concentration of caffeine within the range of concentrations reached by caffeine in the brain parenchyma upon moderate consumption of caffeine (20–70 μM) (Thithapandha et al., 1972; Kaplan et al., 1990; Costenla et al., 2010; Duarte et al., 2012; Silva et al., 2013), which are similar to plasma concentrations of caffeine that cause maximal psycho-activating responses in humans (Bruce et al., 1986). Many studies have described neuronal effects of caffeine using high millimolar or submillimolar concentrations of caffeine (Lee et al., 1987; Martin and Buno, 2003; Margineanu and Klitgaard, 2004; Vyleta and Smith, 2008; Grigoryan et al., 2012), which represent toxic effects of caffeine found in extreme cases of caffeinism (Gilliland and Andress, 1981). Using concentrations of caffeine realistic for normal human consumption, we found that caffeine only targets inhibitory A1R, since caffeine mimics the effects of DPCPX. This is in agreement with PET imaging studies that documented a decreased occupancy of cortical A1R in subjects consuming caffeine (Elmenhorst et al., 2012; Paul et al., 2014). Importantly, we now report that caffeine displays a greater affinity for synaptic A1R compared to extra-synaptic A1R. This may suggest that synaptic A1R are more strongly affected by caffeine and that interference with inhibitory effects of adenosine on transmission at excitatory synapses is a major effect in the human cerebral cortex. In contrast to effects in rodent neocortex, we find that effects of caffeine in human cortical neurons interfered both with adenosine effects on neuronal excitability and excitatory synaptic transmission through post-synaptic A1R, while in rodents, caffeine mostly controls synaptic transmission through presynaptic A1R and affect the release of excitatory neurotransmitters (Phillis et al., 1979; Greene et al., 1985). Nevertheless, also in rodent neocortex, caffeine can exert a post-synaptic effect (Greene et al., 1985; Simons et al., 2011) in accordance with the ability of post-synaptic A1R to induce robust responses in rodent cortical neurons (van Aerde et al., 2015; Qi et al., 2016). Differences in effects of caffeine in human and rodent neocortex may result from the previously discussed different molecular organization of A1R or to a different subsynaptic distribution of A1R in rodent and human cortical synapses; thus, A1R seem to be more abundant presynaptically in rodent synapses (Tetzlaff et al., 1987; Rebola et al., 2003), whereas we find here in human neocortex an even distribution of A1R in all subsynaptic fractions.
For our experiments, we used neocortical tissue derived from epileptic patients that underwent surgery for treatment of deeper brain structures. Although the resected tissue was from regions outside the focal area of epilepsy or tumor, and was not part of the disease, our observations could be affected by disease and medication history. Earlier, adenosine A1R density was shown to be up- or downregulated in surrounding neocortical areas of patients suffering from temporal lobe epilepsy (Angelatou et al., 1993; Glass et al., 1996) and adenosine A1R agonists are explored as therapeutic targets to treat epilepsy (Boison, 2016). Therefore, the possibility that epileptic conditions affect the adenosine neuromodulation system in the cerebral cortex (Rebola et al., 2005) should be considered as a factor influencing the presently reported effects of caffeine. However, the impact of caffeine in epileptic conditions is still largely unclear (Dworetzky et al., 2010; Samsonsen et al., 2013) and seems to be more evident in the developing than in the mature brain (e.g., Tchekalarova et al., 2006), with reports of proconvulsant (e.g., Chu, 1981) and anticonvulsant effects of caffeine (e.g., Rigoulot et al., 2003). It is also important to keep in mind that the present study focused on the human temporal cortex and it remains to be confirmed if the effects of caffeine are similar in other cortical regions, although both the density of A1R and the occupancy of A1R by caffeine seem to be similar in different cortical areas (Svenningsson et al., 1997; Diukova et al., 2012; Elmenhorst et al., 2012; Park et al., 2014).
The present characterization of the effects of a physiological concentration of caffeine (50 μM) on the human temporal cortex provides an important insight in the subtle effects of cortical exposure to human caffeine consumption. By acting mainly post-synaptically, caffeine is able to counteract adenosine-induced inhibition of the received excitatory signal. This provides a mechanism to explain the enhancement by caffeine on human cognition (Borota et al., 2014), which is not readily observed in adult rodents (e.g., Dall’Igna et al., 2007; Duarte et al., 2012; Espinosa et al., 2013; Kaster et al., 2015; reviewed in Cunha and Agostinho, 2010), where caffeine intake mostly normalizes rather than bolsters learning and memory performance. Thus, these findings provide the first neurophysiological description of the impact of caffeine on excitatory synaptic transmission in the human temporal cortex, providing adequate ground for the effects of caffeine in normal consumption amounts on cognition in humans.
Author Contributions
AK, HM, RC, and SF designed the research; AK, AX, BdS, and PC performed the experiments; RC performed the research; JCB and SI provided the resources; AK, HM, and RC wrote the manuscript. All authors commented on the manuscript.
Funding
Supported by Maratona da Saúde, Santa Casa da Misericórdia, GAI-FMUC and Banco Santander-Totta, NARSAD, Erasmus Mundus Joint Doctorate grant (ENC-Network) and ERDF, through Centro 2020 (project CENTRO-01-0145-FEDER-000008:BrainHealth 2020), and through FCT (projects POCI-01-0145-FEDER-007440 and PTDC/NEU-NMC/4154/2016) to RC. HM received funding for this work from the Netherlands Organization for Scientific Research (NWO; VICI grant), ERC StG “BrainSignals,” EU H2020 Framework Programme (agreement no. 604102 “Human Brain Project”) and the EU 7th framework program (EU MSCA-ITN CognitionNet FP7-PEOPLE-2013-ITN 607508).
Conflict of Interest Statement
RC is a scientific consultant for the Institute for Scientific Information on Coffee.
The other authors declare that the research was conducted in the absence of any commercial or financial relationships that could be construed as a potential conflict of interest.
Acknowledgments
We thank Anton Pieneman, Eline Mertens, and Christiaan de Kock for histology and Neurolucida reconstructions. We thank Hans Lodder for technical assistance.
Supplementary Material
The Supplementary Material for this article can be found online at: https://www.frontiersin.org/articles/10.3389/fphar.2017.00899/full#supplementary-material
References
Angelatou, F., Pagonopoulou, O., Maraziotis, T., Olivier, A., Villemeure, J. G., Avoli, M., et al. (1993). Upregulation of A1 adenosine receptors in human temporal lobe epilepsy: a quantitative autoradiographic study. Neurosci. Lett. 163, 11–14. doi: 10.1016/0304-3940(93)90217-9
Boison, D. (2016). Adenosinergic signaling in epilepsy. Neuropharmacology 104, 131–139. doi: 10.1016/j.neuropharm.2015.08.046
Borota, D., Murray, E., Keceli, G., Chang, A., Watabe, J. M., Ly, M., et al. (2014). Post-study caffeine administration enhances memory consolidation in humans. Nat. Neurosci. 17, 201–203. doi: 10.1038/nn.3623
Boswell-Smith, V., Spina, D., and Page, C. P. (2006). Phosphodiesterase inhibitors. Br. J. Pharmacol. 147(Suppl. 1), S252–S257. doi: 10.1038/sj.bjp.0706495
Boulenger, J. P., Patel, J., and Marangos, P. J. (1982). Effects of caffeine and theophylline on adenosine and benzodiazepine receptors in human brain. Neurosci. Lett. 30, 161–166. doi: 10.1016/0304-3940(82)90290-7
Bruce, M., Scott, N., Lader, M., and Marks, V. (1986). The psychopharmacological and electrophysiological effects of single doses of caffeine in healthy human subjects. Br. J. Clin. Pharmacol. 22, 81–87. doi: 10.1111/j.1365-2125.1986.tb02883.x
Canas, P. M., and Cunha, R. A. (2016). “Subsynaptic membrane fractionation,” in Receptor and Ion Channel Detection in the Brain, Vol. 110, eds R. Luján and F. Ciruela (New York, NY: Humana Press), 31–37. doi: 10.1007/978-1-4939-3064-7_3
Chu, N. S. (1981). Caffeine- and aminophylline-induced seizures. Epilepsia 22, 85–94. doi: 10.1111/j.1528-1157.1981.tb04335.x
Ciruela, F., Casadó, V., Rodrigues, R. J., Luján, R., Burgueño, J., Canals, M., et al. (2006). Presynaptic control of striatal glutamatergic neurotransmission by adenosine A1-A2A receptor heteromers. J. Neurosci. 26, 2080–2087. doi: 10.1523/JNEUROSCI.3574-05.2006
Coelho, J. E., Rebola, N., Fragata, I., Ribeiro, J. A., de Mendonça, A., and Cunha, R. A. (2006). Hypoxia-induced desensitization and internalization of adenosine A1 receptors in the rat hippocampus. Neuroscience 138, 1195–1203. doi: 10.1016/j.neuroscience.2005.12.012
Costenla, A. R., Cunha, R. A., and de Mendonça, A. (2010). Caffeine, adenosine receptors, and synaptic plasticity. J. Alzheimers Dis. 20(Suppl. 1), S25–S34. doi: 10.3233/JAD-2010-091384
Cunha, R. A. (2016). How does adenosine control neuronal dysfunction and neurodegeneration? J. Neurochem. 139, 1019–1055. doi: 10.1111/jnc.13724
Cunha, R. A., and Agostinho, P. M. (2010). Chronic caffeine consumption prevents memory disturbance in different animal models of memory decline. J. Alzheimers Dis. 20(Suppl. 1), S95–S116. doi: 10.3233/JAD-2010-1408
Cunha, R. A., Sebastião, A. M., and Ribeiro, J. A. (1998). Inhibition by ATP of hippocampal synaptic transmission requires localized extracellular catabolism by ecto-nucleotidases into adenosine and channeling to adenosine A1 receptors. J. Neurosci. 18, 1987–1995.
Dall’Igna, O. P., Fett, P., Gomes, M. W., Souza, D. O., Cunha, R. A., and Lara, D. R. (2007). Caffeine and adenosine A2a receptor antagonists prevent beta-amyloid (25-35)-induced cognitive deficits in mice. Exp. Neurol. 203, 241–245. doi: 10.1016/j.expneurol.2006.08.008
Daly, J. W., Bruns, R. F., and Snyder, S. H. (1981). Adenosine receptors in the central nervous system: relationship to the central actions of methylxanthines. Life Sci. 28, 2083–2097. doi: 10.1016/0024-3205(81)90614-7
de Mendonça, A., Sebastião, A. M., and Ribeiro, J. A. (1995). Inhibition of NMDA receptor-mediated currents in isolated rat hippocampal neurones by adenosine A1 receptor activation. Neuroreport 6, 1097–1100. doi: 10.1097/00001756-199505300-00006
Diukova, A., Ware, J., Smith, J. E., Evans, C. J., Murphy, K., Rogers, P. J., et al. (2012). Separating neural and vascular effects of caffeine using simultaneous EEG-FMRI: differential effects of caffeine on cognitive and sensorimotor brain responses. Neuroimage 62, 239–249. doi: 10.1016/j.neuroimage.2012.04.041
Dodd, P. R., Watson, W. E., and Johnston, G. A. (1986). Adenosine receptors in post-mortem human cerebral cortex and the effect of carbamazepine. Clin. Exp. Pharmacol. Physiol. 13, 711–722. doi: 10.1111/j.1440-1681.1986.tb02412.x
Doepker, C., Lieberman, H. R., Smith, A. P., Peck, J. D., El-Sohemy, A., and Welsh, B. T. (2016). Caffeine: friend or foe? Annu. Rev. Food Sci. Technol. 7, 117–137. doi: 10.1146/annurev-food-041715-033243
Duarte, J. M., Agostinho, P. M., Carvalho, R. A., and Cunha, R. A. (2012). Caffeine consumption prevents diabetes-induced memory impairment and synaptotoxicity in the hippocampus of NONcZNO10/LTJ mice. PLOS ONE 7:e21899. doi: 10.1371/journal.pone.0021899
Dunwiddie, T. V., and Masino, S. A. (2001). The role and regulation of adenosine in the central nervous system. Annu. Rev. Neurosci. 24, 31–55. doi: 10.1146/annurev.neuro.24.1.31
Dworetzky, B. A., Bromfield, E. B., Townsend, M. K., and Kang, J. H. (2010). A prospective study of smoking, caffeine, and alcohol as risk factors for seizures or epilepsy in young adult women: data from the Nurses’ Health Study II. Epilepsia 51, 198–205. doi: 10.1111/j.1528-1167.2009.02268.x
El Yacoubi, M., Ledent, C., Menard, J. F., Parmentier, M., Costentin, J., and Vaugeois, J. M. (2000). The stimulant effects of caffeine on locomotor behaviour in mice are mediated through its blockade of adenosine A2A receptors. Br. J. Pharmacol. 129, 1465–1473. doi: 10.1038/sj.bjp.0703170
Elmenhorst, D., Meyer, P. T., Matusch, A., Winz, O. H., and Bauer, A. (2012). Caffeine occupancy of human cerebral A1 adenosine receptors: in vivo quantification with 18F-CPFPX and PET. J. Nucl. Med. 53, 1723–1729. doi: 10.2967/jnumed.112.105114
Espinosa, J., Rocha, A., Nunes, F., Costa, M. S., Schein, V., Kazlauckas, V., et al. (2013). Caffeine consumption prevents memory impairment, neuronal damage, and adenosine A2A receptors upregulation in the hippocampus of a rat model of sporadic dementia. J. Alzheimers Dis. 34, 509–518. doi: 10.3233/JAD-111982
Fastbom, J., Pazos, A., Probst, A., and Palacios, J. M. (1987). Adenosine A1 receptors in the human brain: a quantitative autoradiographic study. Neuroscience 22, 827–839. doi: 10.1016/0306-4522(87)92962-9
Ferkany, J. W., Valentine, H. L., Stone, G. A., and Williams, M. (1986). Adenosine A1 receptors in mammalian brain: species differences in their interactions with agonists and antagonists. Drug Dev. Res. 9, 85–93. doi: 10.1002/ddr.430090202
Ferré, S. (2008). An update on the mechanisms of the psychostimulant effects of caffeine. J. Neurochem. 105, 1067–1079. doi: 10.1111/j.1471-4159.2007.05196.x
Fredholm, B. B., Bättig, K., Holmén, J., Nehlig, A., and Zvartau, E. E. (1999). Actions of caffeine in the brain with special reference to factors that contribute to its widespread use. Pharmacol. Rev. 51, 83–133.
Fredholm, B. B., Chen, J. F., Cunha, R. A., Svenningsson, P., and Vaugeois, J. M. (2005). Adenosine and brain function. Int. Rev. Neurobiol. 63, 191–270. doi: 10.1016/S0074-7742(05)63007-3
Gilliland, K., and Andress, D. (1981). Ad lib caffeine consumption, symptoms of caffeinism, and academic performance. Am. J. Psychiatry 138, 512–514. doi: 10.1176/ajp.138.4.512
Glass, M., Faull, R. L., Bullock, J. Y., Jansen, K., Mee, E. W., Walker, E. B., et al. (1996). Loss of A1 adenosine receptors in human temporal lobe epilepsy. Brain Res. 710, 56–68. doi: 10.1016/0006-8993(95)01313-X
Gracia, E., Moreno, E., Cortés, A., Lluís, C., Mallol, J., McCormick, P. J., et al. (2013). Homodimerization of adenosine A1 receptors in brain cortex explains the biphasic effects of caffeine. Neuropharmacology 71, 56–69. doi: 10.1016/j.neuropharm.2013.03.005
Greene, R. W., Haas, H. L., and Hermann, A. (1985). Effects of caffeine on hippocampal pyramidal cells in vitro. Br. J. Pharmacol. 85, 163–169. doi: 10.1111/j.1476-5381.1985.tb08843.x
Grigoryan, G., Korkotian, E., and Segal, M. (2012). Selective facilitation of LTP in the ventral hippocampus by calcium stores. Hippocampus 22, 1635–1644. doi: 10.1002/hipo.22000
Haas, H. L., and Greene, R. W. (1984). Adenosine enhances afterhyperpolarization and accommodation in hippocampal pyramidal cells. Pflugers Arch. 402, 244–247. doi: 10.1007/BF00585506
Huang, Z. L., Qu, W. M., Eguchi, N., Chen, J. F., Schwarzschild, M. A., Fredholm, B. B., et al. (2005). Adenosine A2A, but not A1, receptors mediate the arousal effect of caffeine. Nat. Neurosci. 8, 858–859. doi: 10.1038/nn1491
Kaplan, G. B., Tai, N. T., Greenblatt, D. J., and Shader, R. I. (1990). Caffeine-induced behavioural stimulation is dose- and concentration-dependent. Br. J. Pharmacol. 100, 435–440. doi: 10.1111/j.1476-5381.1990.tb15824.x
Karcz-Kubicha, M., Antoniou, K., Terasmaa, A., Quarta, D., Solinas, M., Justinova, Z., et al. (2003). Involvement of adenosine A1 and A2A receptors in the motor effects of caffeine after its acute and chronic administration. Neuropsychopharmacology 28, 1281–1291. doi: 10.1038/sj.npp.1300167
Kaster, M. P., Machado, N. J., Silva, H. B., Nunes, A., Ardais, A. P., Santana, M., et al. (2015). Caffeine acts through neuronal adenosine A2A receptors to prevent mood and memory dysfunction triggered by chronic stress. Proc. Natl. Acad. Sci. U.S.A. 112, 7833–7838. doi: 10.1073/pnas.1423088112
Klaft, Z. J., Hollnagel, J. O., Salar, S., Calişkan, G., Schulz, S. B., Schneider, U. C., et al. (2016). Adenosine A1 receptor-mediated suppression of carbamazepine-resistant seizure-like events in human neocortical slices. Epilepsia 57, 746–756. doi: 10.1111/epi.13360
Klishin, A., Tsintsadze, T., Lozovaya, N., and Krishtal, O. (1995). Latent N-methyl-D-aspartate receptors in the recurrent excitatory pathway between hippocampal CA1 pyramidal neurons: Ca2+-dependent activation by blocking A1 adenosine receptors. Proc. Natl. Acad. Sci. U.S.A. 92, 12431–12435. doi: 10.1073/pnas.92.26.12431
Lee, W. L., Anwyl, R., and Rowan, M. (1987). Caffeine inhibits post-tetanic potentiation but does not alter long-term potentiation in the rat hippocampal slice. Brain Res. 426, 250–256. doi: 10.1016/0006-8993(87)90879-1
Li, Y., Fan, S., Yan, J., Li, B., Chen, F., Xia, J., et al. (2011). Adenosine modulates the excitability of layer II stellate neurons in entorhinal cortex through A1 receptors. Hippocampus 21, 265–280. doi: 10.1002/hipo.20745
Lopez, F., Miller, L. G., Greenblatt, D. J., Kaplan, G. B., and Shader, R. I. (1989). Interaction of caffeine with the GABAA receptor complex: alterations in receptor function but not ligand binding. Eur. J. Pharmacol. 172, 453–459. doi: 10.1016/0922-4106(89)90028-X
Maemoto, T., Finlayson, K., Olverman, H. J., Akahane, A., Horton, R. W., and Butcher, S. P. (1997). Species differences in brain adenosine A1 receptor pharmacology revealed by use of xanthine and pyrazolopyridine based antagonists. Br. J. Pharmacol. 122, 1202–1208. doi: 10.1038/sj.bjp.0701465
Margineanu, D. G., and Klitgaard, H. (2004). Caffeine-induced epileptiform field potentials in rat hippocampal slices: a pharmacological characterization. Neuropharmacology 47, 926–934. doi: 10.1016/j.neuropharm.2004.06.014
Martin, E. D., and Buno, W. (2003). Caffeine-mediated presynaptic long-term potentiation in hippocampal CA1 pyramidal neurons. J. Neurophysiol. 89, 3029–3038. doi: 10.1152/jn.00601.2002
McCall, A. L., Millington, W. R., and Wurtman, R. J. (1982). Blood-brain barrier transport of caffeine: dose-related restriction of adenine transport. Life Sci. 31, 2709–2715. doi: 10.1016/0024-3205(82)90715-9
McPherson, P. S., Kim, Y. K., Valdivia, H., Knudson, C. M., Takekura, H., Franzini-Armstrong, C., et al. (1991). The brain ryanodine receptor: a caffeine-sensitive calcium release channel. Neuron 7, 17–25. doi: 10.1016/0896-6273(91)90070-G
Mohan, H., Verhoog, M. B., Doreswamy, K. K., Eyal, G., Aardse, R., Lodder, B. N., et al. (2015). Dendritic and axonal architecture of individual pyramidal neurons across layers of adult human neocortex. Cereb. Cortex 25, 4839–4853. doi: 10.1093/cercor/bhv188
Murphy, K. M., and Snyder, S. H. (1982). Heterogeneity of adenosine A1 receptor binding in brain tissue. Mol. Pharmacol. 22, 250–257.
Park, C. A., Kang, C. K., Son, Y. D., Choi, E. J., Kim, S. H., Oh, S. T., et al. (2014). The effects of caffeine ingestion on cortical areas: functional imaging study. Magn. Reson. Imaging 32, 366–371. doi: 10.1016/j.mri.2013.12.018
Paul, S., Khanapur, S., Sijbesma, J. W., Ishiwata, K., Elsinga, P. H., Meerlo, P., et al. (2014). Use of 11C-MPDX and PET to study adenosine A1 receptor occupancy by nonradioactive agonists and antagonists. J. Nucl. Med. 55, 315–320. doi: 10.2967/jnumed.113.130294
Phillis, J. W., Edstrom, J. P., Kostopoulos, G. K., and Kirkpatrick, J. R. (1979). Effects of adenosine and adenine nucleotides on synaptic transmission in the cerebral cortex. Can. J. Physiol. Pharmacol. 57, 1289–1312. doi: 10.1139/y79-194
Pliássova, A., Canas, P. M., Xavier, A. C., da Silva, B. S., Cunha, R. A., and Agostinho, P. (2016). Age-related changes in the synaptic density of amyloid-β protein precursor and secretases in the human cerebral cortex. J. Alzheimers Dis. 52, 1209–1214. doi: 10.3233/JAD-160213
Qi, G., van Aerde, K., Abel, T., and Feldmeyer, D. (2016). Adenosine differentially modulates synaptic transmission of excitatory and inhibitory microcircuits in layer 4 of rat barrel cortex. Cereb. Cortex 27, 4411–4422. doi: 10.1093/cercor/bhw243
Rebola, N., Pinheiro, P. C., Oliveira, C. R., Malva, J. O., and Cunha, R. A. (2003). Subcellular localization of adenosine A1 receptors in nerve terminals and synapses of the rat hippocampus. Brain Res. 987, 49–58. doi: 10.1016/S0006-8993(03)03247-5
Rebola, N., Porciúncula, L. O., Lopes, L. V., Oliveira, C. R., Soares-da-Silva, P., and Cunha, R. A. (2005). Long-term effect of convulsive behavior on the density of adenosine A1 and A2A receptors in the rat cerebral cortex. Epilepsia 46(Suppl. 5), 159–165. doi: 10.1111/j.1528-1167.2005.01026.x
Rigoulot, M. A., Leroy, C., Koning, E., Ferrandon, A., and Nehlig, A. (2003). Prolonged low-dose caffeine exposure protects against hippocampal damage but not against the occurrence of epilepsy in the lithium-pilocarpine model in the rat. Epilepsia 44, 529–535. doi: 10.1046/j.1528-1157.2003.50502.x
Rogers, P. J., and Dernoncourt, C. (1998). Regular caffeine consumption: a balance of adverse and beneficial effects for mood and psychomotor performance. Pharmacol. Biochem. Behav. 59, 1039–1045. doi: 10.1016/S0091-3057(97)00515-7
Rombo, D. M., Newton, K., Nissen, W., Badurek, S., Horn, J. M., Minichiello, L., et al. (2015). Synaptic mechanisms of adenosine A2A receptor-mediated hyperexcitability in the hippocampus. Hippocampus 25, 566–580. doi: 10.1002/hipo.22392
Samsonsen, C., Bråthen, G., Reimers, A., Helde, G., and Brodtkorb, E. (2013). Is dietary caffeine involved in seizure precipitation? Epilepsy Behav. 28, 147–150. doi: 10.1016/j.yebeh.2013.05.003
Sebastião, A. M., Cunha, R. A., de Mendonça, A., and Ribeiro, J. A. (2000). Modification of adenosine modulation of synaptic transmission in the hippocampus of aged rats. Br. J. Pharmacol. 131, 1629–1634. doi: 10.1038/sj.bjp.0703736
Silva, C. G., Métin, C., Fazeli, W., Machado, N. J., Darmopil, S., Launay, P. S., et al. (2013). Adenosine receptor antagonists including caffeine alter fetal brain development in mice. Sci. Transl. Med. 5:197ra104. doi: 10.1126/scitranslmed.3006258
Simões, A. P., Machado, N. J., Gonçalves, N., Kaster, M. P., Simões, A. T., Nunes, A., et al. (2016). Adenosine A2A receptors in the amygdala control synaptic plasticity and contextual fear memory. Neuropsychopharmacology 41, 2862–2871. doi: 10.1038/npp.2016.98
Simons, S. B., Caruana, D. A., Zhao, M., and Dudek, S. M. (2011). Caffeine-induced synaptic potentiation in hippocampal CA2 neurons. Nat. Neurosci. 15, 23–25. doi: 10.1038/nn.2962
Smith, A. (2002). Effects of caffeine on human behavior. Food Chem. Toxicol. 40, 1243–1255. doi: 10.1016/S0278-6915(02)00096-0
Svenningsson, P., Hall, H., Sedvall, G., and Fredholm, B. B. (1997). Distribution of adenosine receptors in the postmortem human brain: an extended autoradiographic study. Synapse 27, 322–335. doi: 10.1002/(SICI)1098-2396(199712)27:4<322::AID-SYN6>3.0.CO;2-E
Tchekalarova, J., Kubová, H., and Mares, P. (2006). Biphasic effect of chronic postnatal caffeine treatment on cortical epileptic afterdischarges during ontogeny in rats. Brain Res. 1082, 43–49. doi: 10.1016/j.brainres.2006.01.067
Testa-Silva, G., Verhoog, M. B., Linaro, D., de Kock, C. P., Baayen, J. C., Meredith, R. M., et al. (2014). High bandwidth synaptic communication and frequency tracking in human neocortex. PLOS Biol. 12:e1002007. doi: 10.1371/journal.pbio.1002007
Tetzlaff, W., Schubert, P., and Kreutzberg, G. W. (1987). Synaptic and extrasynaptic localization of adenosine binding sites in the rat hippocampus. Neuroscience 21, 869–875. doi: 10.1016/0306-4522(87)90043-1
Thithapandha, A., Maling, H. M., and Gillette, J. R. (1972). Effects of caffeine and theophylline on activity of rats in relation to brain xanthine concentrations. Proc. Soc. Exp. Biol. Med. 139, 582–586. doi: 10.3181/00379727-139-36191
Thompson, S. M., Haas, H. L., and Gähwiler, B. H. (1992). Comparison of the actions of adenosine at pre- and postsynaptic receptors in the rat hippocampus in vitro. J. Physiol. 451, 347–363. doi: 10.1113/jphysiol.1992.sp019168
van Aerde, K. I., Qi, G., and Feldmeyer, D. (2015). Cell type-specific effects of adenosine on cortical neurons. Cereb. Cortex 25, 772–787. doi: 10.1093/cercor/bht274
Verhoog, M. B., Obermayer, J., Kortleven, C. A., Wilbers, R., Wester, J., Baayen, J. C., et al. (2016). Layer-specific cholinergic control of human and mouse cortical synaptic plasticity. Nat. Commun. 7:12826. doi: 10.1038/ncomms12826
Vyleta, N. P., and Smith, S. M. (2008). Fast inhibition of glutamate-activated currents by caffeine. PLOS ONE 3:e3155. doi: 10.1371/journal.pone.0003155
Yang, J. N., Chen, J. F., and Fredholm, B. B. (2009). Physiological roles of A1 and A2A adenosine receptors in regulating heart rate, body temperature, and locomotion as revealed using knockout mice and caffeine. Am. J. Physiol. 296, H1141–H1149. doi: 10.1152/ajpheart.00754.2008
Yu, L., Coelho, J. E., Zhang, X., Fu, Y., Tillman, A., Karaoz, U., et al. (2009). Uncovering multiple molecular targets for caffeine using a drug target validation strategy combining A2A receptor knockout mice with microarray profiling. Physiol. Genomics 37, 199–210. doi: 10.1152/physiolgenomics.90353.2008
Keywords: caffeine, human neocortex, synapses, adenosine, A1R, pyramidal neuron
Citation: Kerkhofs A, Xavier AC, da Silva BS, Canas PM, Idema S, Baayen JC, Ferreira SG, Cunha RA and Mansvelder HD (2018) Caffeine Controls Glutamatergic Synaptic Transmission and Pyramidal Neuron Excitability in Human Neocortex. Front. Pharmacol. 8:899. doi: 10.3389/fphar.2017.00899
Received: 03 September 2017; Accepted: 27 November 2017;
Published: 04 January 2018.
Edited by:
Francisco Ciruela, University of Barcelona, SpainReviewed by:
Carl Richard Lupica, National Institute on Drug Abuse (NIH), United StatesDavid Blum, Institut National de la Santé et de la Recherche Médicale, France
Copyright © 2018 Kerkhofs, Xavier, da Silva, Canas, Idema, Baayen, Ferreira, Cunha and Mansvelder. This is an open-access article distributed under the terms of the Creative Commons Attribution License (CC BY). The use, distribution or reproduction in other forums is permitted, provided the original author(s) or licensor are credited and that the original publication in this journal is cited, in accordance with accepted academic practice. No use, distribution or reproduction is permitted which does not comply with these terms.
*Correspondence: Huibert D. Mansvelder, h.d.mansvelder@vu.nl