- 1School of Pharmacy, University of East Anglia, Norwich Research Park, Norwich, United Kingdom
- 2School of Biomedical and Health Sciences, RMIT University, Bundoora, VIC, Australia
- 3Biomedical Research Centre, School of Biological Sciences, University of East Anglia, Norwich, United Kingdom
Adenosine 5′-triphosphate is a well-known extracellular signaling molecule and neurotransmitter known to activate purinergic P2X receptors. Information has been elucidated about the structure and gating of P2X channels following the determination of the crystal structure of P2X4 (zebrafish), however, there is still much to discover regarding the role of this receptor in the central nervous system (CNS). In this review we provide an overview of what is known about P2X4 expression in the CNS and discuss evidence for pathophysiological roles in neuroinflammation and neuropathic pain. Recent advances in the development of pharmacological tools including selective antagonists (5-BDBD, PSB-12062, BX430) and positive modulators (ivermectin, avermectins, divalent cations) of P2X4 will be discussed.
Introduction
Purinergic P2X receptors are involved in mediating a multitude of functions in health and disease via the actions of extracellular adenosine 5′-triphosphate (ATP) (North, 2002; Burnstock and Kennedy, 2011; Khakh and North, 2012). Although ATP has long been recognized as an intracellular energy source, its acceptance as an extracellular signaling molecule has taken considerably longer (Burnstock, 2006). It is now widely recognized that extracellular ATP acts as either sole neurotransmitter or crucial co-neurotransmitter in most nerves in both the peripheral nervous system and central nervous system (CNS) (Burnstock, 2006; Khakh and North, 2012). For example, under physiological conditions, astrocytes and neurons are responsible for releasing small amounts of ATP, which can then act on purinergic receptors – either P2X ion channels or P2Y G protein-coupled receptors - expressed on both neurons and glial cells. In healthy tissues, ATP released from cells is tightly regulated with cell surface ectonucleotidases serving to terminate purinergic signaling (Cardoso et al., 2015) (this is analogous to the activity of cholinesterases at cholinergic synapses). However, when released from injured cells, ATP can initiate inflammation and further amplify and sustain cell-mediated immunity through P2 receptors (Idzko et al., 2014).
P2X receptors are non-selective cation channels that open in response to ATP binding, allowing the rapid flow of ions (K+, Na+, Ca2+) across the membrane. Seven genes encode P2X receptor subunits (P2X1-7) that are expressed in all tissues in humans and mice (North, 2002; Khakh and North, 2006). P2X receptors share a common topology assembling as trimers (Hattori and Gouaux, 2012), with evidence of both homomeric and heteromeric assembly (Saul et al., 2013). Different subtypes are involved in specialized functions depending on their distribution and biophysical properties (Surprenant and North, 2009). These differences provide an opportunity for tissue-specific inhibition of one subtype without affecting the function of others. Such challenges represent a pharmacological opportunity to intervene in physiological processes including modulation of synaptic transmission, taste, pain sensation, and inflammation (Khakh and North, 2006).
Progress on several fronts has revealed the involvement of P2X4 in a variety of pathophysiological processes. For example, P2X4 are implicated in neuropathic pain (Tsuda et al., 2003, 2009a; Coull et al., 2005; Ulmann et al., 2008), inflammatory pain (Ulmann et al., 2010), epilepsy (Ulmann et al., 2013), lung surfactant secretion (Miklavc et al., 2013), alcohol intake and preference (Wyatt et al., 2014; Franklin et al., 2015), morphine-induced hyperalgesia (Ferrini et al., 2013), cardiac function (Yang et al., 2014) and P2X4 deficiency is associated with sociocommunicative impairments (Wyatt et al., 2013) and altered flow-dependent blood vessel remodeling (Yamamoto et al., 2006). However, the study of P2X4 has been seriously hampered by an absence of selective pharmacological tools. While the discovery of selective antagonist molecules against P2X4 would facilitate the further elucidation of their physiological roles, their application might also hold promises to lead us into novel therapeutics for human diseases. Here we aim to present a broad, comprehensive overview of P2X4 receptors, their function in the CNS, and their emerging pharmacology.
P2X4; Ion Channel Structure and Permeability
The P2X4 receptor, now simply termed P2X4 using the latest IUPHAR nomenclature (Di Virgilio et al., 2015), is a ligand-gated ion channel belonging to the P2X receptor family. This family of ion channels are activated by extracellular ATP and function as non-selective cation channels permitting Na+, K+ and Ca2+ ion fluxes (North, 2002). The Ca2+ permeability of P2X4 is the highest among the P2X family (Egan and Khakh, 2004). Measurement of the fractional calcium current (Pf%) through P2X4 using the patch clamp photometry technique (measuring the ATP-induced inward current and the concomitant decrease in the emission of fura-2 fluorescence excited at 380nm) yields values of 11% and 15% for rat P2X4 and human P2X4, respectively (Egan and Khakh, 2004).
P2X subunits are arranged into trimeric structures in the plasma membrane, either in homomeric or heteromeric formations (Saul et al., 2013). This trimeric organization of P2X4 was confirmed in 2009 by solving the crystal structure of a zebrafish P2X4 (zP2X4) in the closed state at 3.1A resolution (Kawate et al., 2009). Three inter-subunit binding sites for ATP were defined in the P2X extracellular domain. In 2012 a crystal structure for the ATP-bound state of zP2X4 was solved revealing the open pore conformation of the channel (Hattori and Gouaux, 2012). This also gave structural insight into the mechanics of channel opening in response to ATP binding; fenestrations open up in close proximity to the plasma membrane providing lateral access pathways for cation entry to the ion channel pore (Samways et al., 2011; Hattori and Gouaux, 2012; Gao et al., 2015). This movement of the extracellular domains upon agonist binding leads to iris-like rotational movements of the transmembrane domains lining the channel pore thus describing the predicted activation mechanism of P2X4 channels (Hattori and Gouaux, 2012). Fast-scanning atomic force microscopy has also been used to reveal the trimeric structure of P2X4 and movement of subunits following ATP binding (Shinozaki et al., 2009).
The P2X4 subtype can heteromerically assemble with other P2X members including P2X6, P2X7 and P2X1 (Le et al., 1998; Nicke et al., 2005; Antonio et al., 2011). These studies have typically used immunoprecipitation of P2X4-containing protein complexes and detection of other P2X using antibodies in combination with altered functional and pharmacological properties as major pieces of experimental evidence. For example, Xenopus oocytes expressing both P2X1 and P2X4 exhibit a slowly desensitizing current similar to homomeric P2X4 but in the presence of P2X1 there is significant activation by αβ-MeATP and inhibition by suramin (Nicke et al., 2005), properties not seen with either P2X4 or P2X1 alone. The reported interaction with P2X7 is much more contentious. Evidence first suggested that P2X4 and P2X7 could form heterotrimeric complexes (Boumechache et al., 2009) but then further investigations revealed that homotrimers of P2X4 and P2X7 may interact with each other (Antonio et al., 2011).
Permeability
Khakh and colleagues first described different permeability states for P2X4, denoting two states (I1 and I2 state) in response to a sustained exposure to ATP (Khakh et al., 1999a). This secondary permeability state (I2) displays increased NMDG+ permeation suggestive of a larger channel pore size and a dynamic change in ion selectivity. This concept has recently been challenged and Vrev changes recorded during NMDG+/Na+ bi-ionic conditions is thought to be due to alterations in intracellular ion depletion and accumulation (Li et al., 2015). This data can be interpreted as lack of evidence for dynamic changes in ion conductance but whether P2X channels are directly permeable to large cations such as NMDG+ still warrants further investigation. Further evidence for a secondary permeability state (or permeation of large organic cations) for P2X4 comes from the use of membrane impermeant fluorescent dyes such as YOPRO-1 iodide and ethidium bromide (Khakh et al., 1999a; Bernier et al., 2012). Whether these dyes permeate directly through the P2X4 channel or an associated conduit is not yet clear. Much evidence exists for a large secondary permeation pathway activated by P2X7 in numerous cell types. Classically this is measured by the use of dye uptake assays (in particular such as ethidium bromide, YOPRO-1 iodide, Lucifer yellow). Controversy surrounds the underlying mechanism of action for P2X7 with some studies suggesting a second protein may carry the dyes, such as pannexin-1 (Pelegrin and Surprenant, 2006) or anoctamin-6 (Ousingsawat et al., 2015) and others suggesting that P2X7 itself can allow passage of large molecules (Browne et al., 2013). The physiological role of this permeability pathway for large cations is still unclear for P2X7 and P2X4 channels, and indeed those other ligand-gated ion channels demonstrating a similar phenomenon (Chung et al., 2008; Banke et al., 2010).
Expression of P2X4 in the CNS
P2X4 was originally cloned from rat brain cDNA in 1996 (Soto et al., 1996a) and was the first P2X receptor detected in CNS neurons and blood vessels. P2X4 was subsequently cloned from a human brain sample (Garcia-Guzman et al., 1997) and was also found to exhibit a broad tissue expression pattern. Amongst the P2X receptor subtypes, P2X4, along with P2X2 and P2X6, has been shown to be the most widespread and abundantly expressed functional ATP-gated purinergic receptor in the CNS, being found in most neurons and glial cells (Buell et al., 1996; Soto et al., 1996a,b; Tsuda et al., 2003; Guo et al., 2005; Amadio et al., 2007; Vazquez-Villoldo et al., 2014). This has been illustrated at both mRNA and protein level by in situ hybridisation, immunohistochemistry, PCR and immunoblotting (Soto et al., 1996b; Lê et al., 1998; Bo et al., 2003).
Neurons
Throughout the CNS, expression of P2X4 is widely observed in neurons. In the original paper cloning P2X4 from rat brain, Soto and colleagues demonstrated high levels of P2X4 mRNA in rat dentate gyrus granule cells, CA1/CA3 pyramidal cells, cerebellar cortex Purkinje cells, and neurons of the pontine nucleus (Soto et al., 1996a). Electron microscopy analysis suggests P2X4 localisation in peri-synaptic regions of post-synaptic terminals and on pre-synaptic terminals (Rubio and Soto, 2001). Immunohistochemistry also shows P2X4 to be expressed in GABAergic interneurons and GABAergic spiny neurons of the rat striatum and substantia nigra (Amadio et al., 2007).
The hypothalamus and anterior pituitary gland abundantly express P2X4 and this receptor may be involved in regulation of hypothalamo-pituitary functions in the CNS, as reviewed in Stojilkovic (2009). Immunohistochemistry studies identified P2X4 on paraventricular nucleus neurons projecting to the rostral ventrolateral medulla and a potential role in regulating sympathetic nerve activity (Cham et al., 2006). Paraventricular neurons, arcuate nucleus GnRH neurons and secretory cells of the anterior pituitary all express P2X4 as illustrated through molecular biology techniques in combination with electrophysiology (Zemkova et al., 2010). Functional P2X4 receptors have also been identified in lactotrophs (He et al., 2003), and in the posterior pituitary system functional P2X4 responses have been recorded from supraoptic neurons (Vavra et al., 2011; Stojilkovic and Zemkova, 2013). P2X4 has also been demonstrated to be expressed in somatosensory cortical neurons (Lalo et al., 2007), nodose ganglion neurons (Tan et al., 2009), trigeminal neurons (Luo et al., 2006), vestibular ganglion neurons (Ito et al., 2010), retinal ganglion and bipolar cells (Wheeler-Schilling et al., 2001) and in spinal cord neurons (Bardoni et al., 1997; Kobayashi et al., 2005).
P2X4 has been implicated in physiological functions in the CNS including modulation of neurotransmission and synaptic strengthening (Rubio and Soto, 2001; Sim et al., 2006; Baxter et al., 2011). In the hippocampus P2X4 expression on pyramidal neurons is thought to contribute to synaptic plasticity and long-term potentiation (LTP). One of the initial studies that elaborated a role for P2X4 in LTP was performed in mice with a global deficiency in the p2rx4 gene (P2X4-/-) (Sim et al., 2006). Extracellular recording of field potentials from the CA1 region of the hippocampus in these P2X4-deficient mice revealed reduced synaptic facilitation and induction of LTP compared to wild-type counterparts. In addition, ivermectin, a positive allosteric modulator of P2X4, increased LTP in wild-type mice but was ineffective in the P2X4-/- mice (Sim et al., 2006). This suggested that P2X4 contributes to strengthened synaptic activity during LTP and it is hypothesized that calcium entry through sub-synaptic P2X4 contributes to synaptic strengthening by NMDA receptor incorporation (Baxter et al., 2011).
Studies have also investigated cross-talk between P2X4 and other ion channels in neurons, in particular GABA(A) receptors (Jo et al., 2011) and nicotinic acetylcholine receptors (Khakh et al., 2000). In hypothalamic neurons increased expression of P2X4 is associated with a reduction in GABAergic currents (Jo et al., 2011). There is some evidence for a physical coupling between P2X4 and GABA(A) receptors and this may play a role in regulating synaptic signaling (Jo et al., 2011). A similar cross-talk has been demonstrated for P2X2 receptors and GABA(A) receptors and this cross-talk appears to be a general mechanism for the regulation of GABAergic signaling, as reviewed in Shrivastava et al. (2011). Therefore, P2X4 may be involved in regulating excitatory vs. inhibitory neurotransmission in neurons, acting as a neuromodulator. The contribution of P2X4 in this process will likely become clearer in the future with the development of selective pharmacological tools and more knockout mouse studies.
Glial Cells
In the CNS P2X4 plays a role in modulating synaptic transmission and communication between neurons and neighboring glial cells. Glia are the most abundant cell type accounting for >70% of total cells in the CNS and can be classified into three main types; astrocytes, oligodendrocytes and microglia.
The role of P2X4 in microglial cells has received much attention in the last decade. Microglial cells, known as the resident macrophages in the CNS, originate from the yolk sac and are related to myeloid immune cells (Kettenmann et al., 2011; Saijo and Glass, 2011). Immunohistochemistry analysis has revealed abundant P2X4 reactivity on microglia within the brain and spinal cord (Tsuda et al., 2003; Ulmann et al., 2008). Despite the fact that P2X4 is abundantly expressed in microglial cells, the majority of labeled P2X4 appears to be predominantly localized to intracellular lysosomal compartments (Qureshi et al., 2007; Toyomitsu et al., 2012). P2X4 has been suggested to play a role in regulating the activation and migration of microglial cells at sites of injury (Guo et al., 2005; Schwab et al., 2005). Following peripheral nerve injury, the expression of P2X4 is up-regulated in activated spinal cord microglia at the transcriptional, translational and post-translational levels (Ulmann et al., 2008). Several factors that have been associated with regulating P2X4 expression include the chemokine CCL21 (Biber et al., 2011), fibronectin (Nasu-Tada et al., 2006; Tsuda et al., 2008), and the cytokine interferon gamma (IFN-γ) (Tsuda et al., 2009b), while activation of the chemokine receptor CCR2 is a key factor involved in post-translational regulation of P2X4 (Toyomitsu et al., 2012). Fibronectin and IFN-γ can regulate P2X4 expression through the IRF5 transcription factor in microglia (Masuda et al., 2014).
P2X4 activation in spinal microglia leads to the release of brain-derived neurotrophic factor (BDNF), which communicates between microglia and spinal interneurons resulting in pain hypersensitivity via disinhibition of GABAergic input (Tsuda et al., 2003; Coull et al., 2005; Ulmann et al., 2008; Trang et al., 2009). Allodynia induced through peripheral nerve injury was reversed by pharmacological blockade of P2X4 receptors in the spinal cord (Tsuda et al., 2003) and P2X4-/- mouse models have reduced inflammatory and neuropathic pain (Ulmann et al., 2008). These findings highlight the importance of P2X4 in microglial cells in the development of neuropathic pain, as reviewed by Inoue and Tsuda (2012) and Tsuda et al. (2013) and summarized in Figure 1. Furthermore, activated microglia play a critical role in the pathogenesis of other diseases such as spinal cord injury, stroke and neurodegenerative disease (i.e., Alzheimer’s and Parkinson’s disease), therefore P2X4 may also contribute to pathogenesis of these conditions.
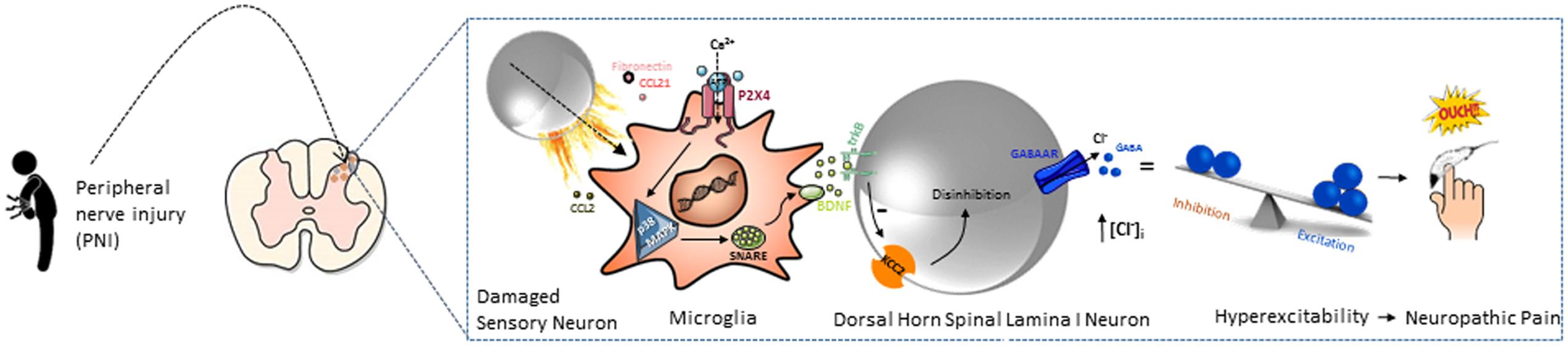
FIGURE 1. Postulated role of P2X4 signaling in chronic pain. Peripheral nerve injury (PNI) activates microglia in the dorsal horn of the spinal cord. This causes the upregulation of the P2X4 expression which is modulated by fibronectin and chemokine ligand 21 (CCL21). CCL2 signaling promotes P2X4 trafficking to cell surface of microglia. Influx of Ca2+ through ATP-stimulated P2X4 activates p38-MAPK and drives the synthesis and SNARE-dependent release of brain-derived neurotrophic factor (BDNF). After BDNF is released, it acts on its cognate receptor, trkB which consequently downregulates potassium-chloride cotransporter KCC2 expression in dorsal horn spinal lamina 1 neurons. This results in increase of intracellular [CI-] and leads to the reduction in anion gradient in dorsal horn which in turn induces depolarization of these neurons. The altered chloride gradient causes GABA to switch its effects from inhibition to excitation. The resultant hyperexcitability in the dorsal horn could underlie the increased sensitivity that is a feature of neuropathic pain.
Astrocytes are star-shaped glial cells providing a wide variety of functional support for neurons. P2X1-5 and P2X7 receptors have been identified at the transcriptional level in situ and in vitro in primary cultured rat cortical astrocytes, hippocampal astrocytes and astrocytes in the nucleus accumbens (Franke et al., 2001; Fumagalli et al., 2003; Dixon et al., 2004) and protein expression was further confirmed (Kukley et al., 2001). In rat hippocampus, immunostaining revealed expression of P2X4 particularly in S100β positive astrocytes in the CA1, CA3 and dentate gyrus regions (Kukley et al., 2001). However, in acutely isolated mouse cortical astrocytes no mRNA expression for P2X4 was detected using qRT-PCR. This correlated with electrophysiology studies showing insensitivity of ATP-induced currents to the positive modulator ivermectin (Jabs et al., 2007). At a functional level, P2X4 responses are not ubiquitously found since P2X4-mediated currents were not detected in acutely isolated cortical astrocytes (Lalo et al., 2008) or hippocampal slices (Jabs et al., 2007). Currently, therefore, there is limited evidence for P2X4 in astrocytes.
Oligodendrocytes are glial cells primarily responsible for myelination of neurons in the CNS and expression of several types of P2 receptor including P2X4 has been demonstrated in oligodendrocytes and in oligodendrocyte precursor cells (OPCs) (Agresti et al., 2005). Aside from neurons and neuroglial cells there is a vast network of blood vessels in the CNS. There is a long standing interest in the role of P2X4 in peripheral endothelial cells and the regulation of blood vessel tone (Yamamoto et al., 2000, 2006). P2X4 is abundantly expressed in peripheral vessel endothelial cells but it is unclear if this is also true for brain microvascular endothelial cells which play an important role in constitution of the blood brain barrier (BBB) and deterioration of this barrier is implicated in several neurological disorders. To date expression of P2X4 has been demonstrated in the hCMEC/D3 human microvascular endothelial cell line and the mouse microvascular cell line bend.3 (Bintig et al., 2012; Ozaki et al., 2016). Similar to the periphery, brain endothelial P2X4 may be involved in regulating shear stress responses in the cerebral vasculature and stimulation of protective factors such as osteopontin to enable ischaemic tolerance (Ozaki et al., 2016).
Subcellular Localisation of P2X4
The cellular localisation of most P2X receptors is thought to be predominantly at the plasma membrane. However, P2X4 expression at the cell surface is limited with the majority of receptors occupying an intracellular residency in resting cells (Robinson and Murrell-Lagnado, 2013). P2X4 contains an additional C-terminal trafficking motif (YxxxGφ) which facilitates rapid internalization of cell surface P2X4 via AP2 and clathrin-dependent endocytosis (Royle et al., 2005). The subcellular distribution of P2X4 in many cell types shows a punctate, clustered staining pattern with overlapping markers of intracellular vesicles including recycling endosomes and lysosomes (Bobanovic et al., 2002; Qureshi et al., 2007; Stokes and Surprenant, 2009). Although regulated trafficking is not unique to P2X4 among the P2X receptor family, the ability of P2X4 to rapidly recycle between the plasma membrane and intracellular compartments is a robust feature studied by electrophysiological and biochemical methods. There is strong evidence that P2X4 receptors localize to the plasma membrane, endosomes, lysosomes, and vacuoles. Insights about dynamic P2X4 trafficking were revealed with use of a pH-sensitivecou fluorescent protein, pHluorin. Khakh and colleagues have recently used P2X4-pHluorin for in vitro trafficking studies to quantify and track P2X4 distribution within subcellular compartments (Xu et al., 2014). Similarly, other mechanistic studies also showed that P2X4 upregulation occurs both via altered trafficking and via increased P2X4 gene expression (Toulme et al., 2006, 2010; Raouf et al., 2007; Toulme and Khakh, 2012). Recently it was suggested that P2X4 functions as an ATP-gated channel in lysosomes (Huang et al., 2014) and regulates endolysosomal membrane fusion via Ca2+-dependent activation of calmodulin (Cao et al., 2015).
P2X4 Downstream Signaling Pathways
Following ATP activation of P2X4 channels in the plasma membrane, the rapid influx of cations (Na+ and Ca2+) will cause membrane depolarisation. In addition the significant influx of Ca2+, as has been reported for P2X4, will likely trigger important downstream signaling pathways. For example, Ca2+ is important in neurons in the regulation of neurotransmitter release (Neher and Sakaba, 2008) and in various microglial functions (Farber and Kettenmann, 2006).
Recently, several lines of evidence suggest that P2X4 stimulated microglia signal to spinal lamina I dorsal horn neurons causing nociceptive output and that the critical microglia-neuron signaling molecule is BDNF (Coull et al., 2005). Notably, accumulation of BDNF in spinal dorsal horn microglia following nerve injury in global P2X4-/- mice showed impaired ATP-evoked BDNF release which further demonstrated that P2X4 plays an important role in controlling BDNF release from microglia (Ulmann et al., 2008). The mechanistic gap between the activation of P2X4 and release of BDNF from microglia was bridged with a study from Salter and colleagues (Trang et al., 2009). Influx of Ca2+ through P2X4 in cultured microglia is a critical intracellular step linking stimulation of these receptors to the activation of p38 MAPK. This implicates p38 MAPK activation and BDNF release as a key step in microglia-neuron communication leading to nerve injury induced pain hypersensitivity. However, there may be other cell types expressing P2X4 involved in pain pathways. Peripheral macrophages also express P2X4 triggering Ca2+ influx and p38 MAPK phosphorylation (Ulmann et al., 2010). This signaling could further activate cytosolic PLA2 liberating arachidonic acid (AA) and release of prostaglandin E2 (PGE2). Consequently, the release of PGE2 leads to hypersensitivity of peripheral nociceptive pathways which is a hallmark of inflammatory pain (Basbaum et al., 2009).
P2X4 in CNS Disorders
As knowledge regarding the expression and the characteristics of the individual P2X channels has emerged and expanded since they were first cloned in the late 1990s, questions have arisen over the importance of P2X channels in normal physiological functioning and their functioning in pathological conditions. Information regarding the specific role of P2X4 has been slower to accumulate largely due to the lack of selective antagonists for this P2X subtype (see P2X4 pharmacology section below). The first evidence for a pathological role for P2X4 was in pain processing. A landmark study by Tsuda and colleagues used antisense P2X4 oligonucleotides and described a major role for P2X4 in chronic pain and mechanical allodynia (Tsuda et al., 2003). The development of transgenic mice lacking P2X4 expression has since confirmed the role of this ion channel in modulation of pain signaling (Ulmann et al., 2008, 2010; Tsuda et al., 2009a).
Chronic Pain
Chronic pain is described as persistent pain arising from a modification of pain processing pathways in the spinal cord and can be labeled as allodynia, hypersensitivity or neuropathic pain (Basbaum et al., 2009; Cohen and Mao, 2014). There are several review articles describing purinergic receptors in the context of pain and it is important to acknowledge here that more than one purinergic receptor has been implicated in pain – currently P2X2/3, P2X7 and P2Y1, P2Y2, P2Y12 are thought to be involved in the modulation of pain processing (Jarvis, 2010; Tsuda, 2016).
Tsuda and colleagues demonstrated that P2X4 was upregulated in spinal microglia after nerve injury (ligation of the fifth lumbar spinal nerve) and mediated tactile allodynia (defined as a hypersensitivity to non-painful stimuli) (Tsuda et al., 2003). Blocking P2X4 (with TNP-ATP) or knocking down P2X4 with antisense oligonucleotides reversed the allodynia phenotype and thus suggested that P2X4 may be a potential therapeutic target for this type of neuropathic pain. In 2005 it was demonstrated that communication between microglia and neurons was essential for the development of allodynia and that the critical factor involved was BDNF (Coull et al., 2005). Stimulation of spinal microglia with ATP induced BDNF secretion which through activation of TrkB receptors on spinal output neurons, alters Eanion causing disinhibition of GABAergic inputs from inhibitory spinal neurons. This leads to hyperexcitability of output neurons in the pain pathway. BDNF was already known to be a pro-nociceptive factor able to sensitize neurons (Kerr et al., 1999) but this study was the first to connect ATP-P2X4-BDNF to the neuronal shift in Eanion. Ulmann et al. (2008) then provided direct evidence for P2X4 in mediating BDNF secretion from spinal microglia using a P2X4-deficient mouse generated by the Rassendren lab. They further demonstrated that P2X4-/- mice did not develop mechanical hyperalgesia following peripheral nerve injury. This lack of mechanical hyperalgesia was confirmed using a second global P2X4 deficient mouse generated by the Ando lab. Behavioral tests demonstrated no difference in acute thermal, mechanical and chemical induced pain sensing in these P2X4-/- mice but there was significant suppression of chronic inflammatory pain (induced by CFA injection) and allodynia due to nerve injury (Tsuda et al., 2009a).
More recently, P2X4 has been implicated in tolerance to morphine (Horvath et al., 2010) and morphine-induced hyperalgesia (Ferrini et al., 2013). Morphine enhanced microglial migration in vitro in a μ-opioid receptor dependent manner, and antisense P2X4 oligonucleotides reduced the development of morphine tolerance in rats (Horvath and DeLeo, 2009; Horvath et al., 2010). Furthermore, Ferrini et al. (2013) demonstrated morphine hyperalgesia required μ-opioid receptor-mediated upregulation of P2X4 in spinal microglia. Blocking the BDNF-TrkB pathway reversed the hyperalgesia. Therefore the microglial ATP-P2X4-BDNF axis seems to be a central pathway involved in the setting of pain thresholds. However, recent evidence has revealed that this mechanism may be restricted to males since female rodents did not display upregulation of P2X4 on microglia (Sorge et al., 2015; Mapplebeck et al., 2016).
Alcohol-Related Disorders
In the search to identify therapeutic targets for Alcohol Use Disorders (AUD), several studies have demonstrated that P2X4 plays a role in alcohol-induced behavior (Yardley et al., 2012; Wyatt et al., 2014). P2X4 are expressed in key brain regions implicated in the reinforcing properties of alcohol and other drugs (Kimpel et al., 2007). This supports a role for P2X4 in alcohol addiction probably via modulation of P2X4 in the mesolimbic dopamine system, the brain reward system (Khoja et al., 2016). In vitro, P2X4 are inhibited by ethanol concentrations as low as 5mM and ethanol is thought to block the P2X4 channel in the open state but does not affect channel deactivation (Davies et al., 2005; Ostrovskaya et al., 2011). Crucial residues that mediates ethanol effects on P2X4 gating are Trp46, His241, Asp331 and Met336 (Xiong et al., 2005; Popova et al., 2010, 2013).
In P2X4-/- mice heightened ethanol intake behavior is observed over wild-type counterparts suggesting that the P2X4 gene may be linked with alcohol intake and/or preference (Asatryan et al., 2011; Franklin et al., 2014). It has been shown that alcohol-preferring rats have lower expression of the P2X4 gene than alcohol-non-preferring rats, suggesting a correlation between P2X4 expression and alcohol intake (Kimpel et al., 2007; Franklin et al., 2015). Ivermectin, a broad-spectrum antiparasitic used worldwide in humans and animals, can antagonize the inhibitory action of ethanol on P2X4 and is suggested to interfere with ethanol binding to the channel (Asatryan et al., 2010). Other avermectins have been demonstrated to act in a similar fashion (Asatryan et al., 2014; Huynh et al., 2017). Studies also identify a role for P2X4 in regulating dopaminergic neurotransmission within the mesolimbic system. In dopaminergic neurons of the mesolimbic system, P2X4 has been shown to play a role in mediating alcohol-drinking behavior (Franklin et al., 2015). P2X4 receptor activity has also been linked to other behaviors of associated with dopaminergic neurotransmission, including motor control and sensorimotor gating (Khoja et al., 2016). Overall, these findings suggest that alcohol intake may be modulated by ethanol acting on P2X4 and that pharmacological potentiation of P2X4 by ivermectin or avermectin analogs may reduce alcohol consumption and preference.
Neuroinflammatory Disorders
Neuroinflammation describes inflammatory changes in the brain and spinal cord associated with activation of the immune system (DiSabato et al., 2016). This is thought to be mediated by mediators such as chemokines, cytokines and second messengers (such as nitric oxide and prostaglandins). Resident immune cells such as microglia are involved, as are endothelial cells and astrocytes. Microglia regulate cytokines and inflammatory processes in the brain, typically elevated pro-inflammatory cytokines (IL-6, TNF-α, and IL-1β) and chemokines (CCL2, CCL5, CXCL1) define a state of neuroinflammation, as reviewed in DiSabato et al. (2016).
Neurodegenerative diseases such as Alzheimer’s disease and Parkinson’s disease are associated with neuroinflammation (Ransohoff, 2016) and microglia appear to be central (Saijo and Glass, 2011; Joers et al., 2016; Wes et al., 2016). P2X4 and the related purinergic channel P2X7 (Bhattacharya and Biber, 2016) are involved in the regulation of microglial pathways therefore may also play roles in exacerbating inflammation in the CNS. P2X4-/- mice (Rassendren mouse model) have an attenuated inflammatory response associated with spinal cord injury with reduced NLRP1 inflammasome-related signaling pathways (de Rivero Vaccari et al., 2012). P2X4 deficiency also reduced the infiltration of peripheral immune cells (neutrophils and macrophages) to the spinal cord (de Rivero Vaccari et al., 2012).
P2X4 was up-regulated in microglial cells in an animal model of multiple sclerosis (autoimmune EAE in rats) (Vazquez-Villoldo et al., 2014). Using LPS as a model of neuroinflammation the P2X4 antagonists TNP-ATP and 5-BDBD were shown to reduce microglial activation in vivo and reduce the microglial loss in spinal cord. Following i.c.v. injection of LPS, the dentate gyrus region of the hippocampus showed classical hallmarks of microglial activation which were blocked by TNP-ATP (Vazquez-Villoldo et al., 2014).
Alcohol abuse is thought to enhance neuroinflammation (Potula et al., 2006) and the P2X4 receptor on microglial cells has been implicated (Gofman et al., 2014, 2016). In addition neuroinflammatory responses are also associated with ischaemic events in the brain and studies demonstrate upregulation of P2X4 in microglia following hypoxia and ischaemia (Wixey et al., 2009; Li et al., 2011). Recently, using a mouse model of middle cerebral artery occlusion (MCAO) endothelial P2X4 was required for the neuroprotective effect of ischaemic preconditioning (transient ischaemia followed by reperfusion) (Ozaki et al., 2016). P2X4 is known to be activated by shear stress generated by conditions of flow in peripheral endothelial cells (Yamamoto et al., 2000, 2006). Mice with a conditional knockout of vascular endothelial P2X4 showed severe deficits after MCAO due to an attenuation in release of the neuroprotective factor osteopontin (Ozaki et al., 2016).
P2X4 Pharmacology
There has been significant progress in understanding P2X receptor pharmacology in recent years. Despite such advances, there remains a paucity of potent and selective pharmacological tools for some receptors. Agonists that selectively activate distinct members of this family are elusive, and with a few exceptions, progress has been slow when identifying selective inhibitors, some of which are shown in Figure 2. Here we describe what is currently known regarding pharmacological modulators of P2X4.
Agonists
The most potent agonist of homomeric P2X4 is ATP. Dose-responses for ATP at rat and human P2X4 gave EC50 values of 6.9 ± 0.8 and 7.4 ± 0.5 μM, respectively (Soto et al., 1996a; Garcia-Guzman et al., 1997). In the original article characterizing rat P2X4 expressed in Xenopus oocytes the electrophysiological profile of agonists was ATP > 2-methylthio-ATP > CTP > α, β-methylene-ATP > dATP and no response was elicited by ADP, AMP, GTP, adenosine or the β,γ-methylene-ATP analog (Soto et al., 1996a). For human P2X4 expressed in Xenopus oocytes the same order of efficacy was demonstrated (Garcia-Guzman et al., 1997). BzATP is also known to act as a partial agonist at rat and human P2X4 (Bowler et al., 2003; Stokes et al., 2011).
Positive Modulators
One of the most distinguishing features of P2X4 is potentiation by ivermectin – a macrocyclic lactone derived from the selective hydrogenation of avermectin B1, a natural product synthesized by Streptomyces avermitilis. Ivermectin is a mixture of 22,23-dihydroavermectin B1a and B1b in an approximate 80:20 ratio (Laing et al., 2017). Use of ivermectin as a successful anti-helminthic drug is due to its positive allosteric modulation of glutamate–gated chloride channels in nematode worms. Ivermectin also potentiates responses at vertebrate GABA(A) and nicotinic α7 receptors (Krůšek and Zemková, 1994; Krause et al., 1998). Of all purinergic receptors, P2X4 is the most sensitive to ivermectin (Khakh et al., 1999b) although a recent report claims some potentiation of human P2X7 responses by ivermectin (Nörenberg et al., 2012). Extracellular, but not intracellular, application of ivermectin (≤10 μM) potentiates P2X4 currents and delays channel deactivation (Khakh et al., 1999b; Priel and Silberberg, 2004). These observations are likely the result of ivermectin binding to separate sites on P2X4, a high affinity ivermectin binding site (pEC50 6.6) which increases maximal current activated by saturating concentrations of ATP, and a low affinity site (pEC50 5.7) which slows channel deactivation (Priel and Silberberg, 2004). Ivermectin appears to interact with the transmembrane domains of P2X4 and lateral fenestrations (Jelinkova et al., 2006; Silberberg et al., 2007; Hattori and Gouaux, 2012; Samways et al., 2012; Rokic et al., 2013) including a binding pocket which overlaps with an inhibitory ethanol binding site (Asatryan et al., 2010).
Avermectin analogs in addition to ivermectin also have positive modulator activity at P2X4. These include avermectin, emamectin, doramectin (Silberberg et al., 2007) and more recently abamectin, selamectin, and moxidectin (Asatryan et al., 2014; Huynh et al., 2017). Of these avermectin, abamectin and moxidectin have similar effects to ivermectin in preventing the action of ethanol on P2X4, but have less effect on GABA(A) receptors (Asatryan et al., 2014; Huynh et al., 2017).
There is also evidence for positive allosteric modulation of P2X4 by cibacron blue, an isomer of reactive blue-2, in functional studies. Potentiation of P2X4 responses were attributable to an increase in the apparent affinity of ATP for the rat P2X4 (Miller et al., 1998). This potentiation of P2X4 responses is smaller than that of ivermectin and was not demonstrated in cells expressing human P2X4 (Garcia-Guzman et al., 1997).
Broad Spectrum Purinergic Receptor Antagonists
P2X4 receptors are generally less sensitive to the broad P2X antagonists, such as suramin and pyridoxalphosphate-6-azophenyl-2′,4′-disulfonic acid (PPADS). Suramin shows very weak activity at mouse, rat and human P2X4 orthologs with maximal inhibition of P2X4 currents ranging between 11 and 35% with pIC50 > 4 for all orthologs (Jones et al., 2000). In contrast, PPADS fully inhibits human and mouse P2X4 (pIC50 ∼ 5 for both), whilst the rat receptor is relatively insensitive to PPADS (Jones et al., 2000). It has been hypothesized that PPADS acts, in part, by forming a Schiff base with a lysine residue in P2X1 and P2X2. However, this residue in the P2X4 is replaced by a glutamate at the analogous position (Glu249) and when it is replaced by a lysine, the resultant P2X4 mutant is sensitive to inhibition by PPADS. Notably, the human P2X4 has only one lysine (Lys127), which is absent in the rat P2X4, conferring sensitivity to PPADS, and mutation of the residue to a lysine in the rat P2X4 (Asn127Lys) did not produce a PPADS-sensitive channel (Buell et al., 1996). Critically, the increased sensitivity of the human P2X4 to PPADS inhibition might not be only due to the different ability of PPADS to form Schiff base via lysine residues. In addition, several studies have confirmed that 2′,3′-O-(2,4,6-trinitrophenyl) ATP (TNP-ATP), which is a potent antagonist at P2X1, P2X3 and P2X2/3 receptors, acts as a weakly effective antagonist (IC50 15.2 μM) at P2X4 receptors (Virginio et al., 1998). TNP-ATP is a competitive antagonist at P2X4 and can displace [35S]ATPγS binding to human P2X4 (Hernandez-Olmos et al., 2012; Abdelrahman et al., 2016). The potency of TNP-ATP at P2X4 estimated by Ca2+ influx assays in 1321N1 astrocytoma cells was 1.46–4.22 μM dependent on species (Abdelrahman et al., 2016).
Divalent Cations and pH
With regard to ions, P2X4 is amongst the most sensitive P2 receptor to potentiation by zinc ions (Zn2+). Low concentrations (1–5 μM) of extracellular Zn2+ increases the gating efficiency of human or rat P2X4 without affecting the maximal response, however, high (>100 μM) extracellular Zn2+ inhibits P2X4 currents in a voltage-dependent fashion (Garcia-Guzman et al., 1997; Wildman et al., 1999; Acuna-Castillo et al., 2000). Similarly, the potentiating effects of Zn2+ are mimicked by Cd2+, however, Cu2+ modifies P2X4 activity in the opposite direction causing inhibition (Acuna-Castillo et al., 2000; Coddou et al., 2005). High concentrations of extracellular Cu2+ (300 μM) inhibit rat P2X4 in a non-competitive and voltage-independent fashion and this effect can be seen with other metal cations such as Hg2+ (Coddou et al., 2005). His140 plays a crucial role in the inhibitory action of Cu2+ but this was not required for the action of Zn2+ at P2X4 (Coddou et al., 2003). Moreover, Asp138 was further identified as important for Cu2+ binding at P2X4 and Cys132 was critical for Zn2+ potentiation at P2X4 (Coddou et al., 2007). These residues were validated by an NMR resolved structure of rat P2X4 extracellular domain as key residues that coordinate Cu2+ binding (Igawa et al., 2015).
The activity of P2X4 is not only regulated by metals and ions, but is also modulated by extracellular H+, such that pH < 6 completely inhibits channel activity and responses are potentiated above physiological pH (Wildman et al., 1999; Clarke et al., 2000). One key residue, His286, has been identified in conveying pH-sensitivity to P2X4 (Clarke et al., 2000). Inhibition by low pH may be an important physiological regulator of P2X4 receptor since P2X4 displays a predominant lysosomal distribution (Qureshi et al., 2007) where it appears to function as a lysosomal ion channel in addition to its plasma membrane role (Huang et al., 2014). In lysosomes the luminal pH is typically acidic with a resting pH 4.6 maintained by vacuolar H+-ATPases. Under these conditions P2X4 was not active but following alkalinisation of lysosomes with NH4Cl, ATP-induced responses could be recorded (Huang et al., 2014).
Antidepressants
Recent studies have documented that some antidepressants may inhibit P2X4 receptors, however, this evidence remains controversial in view of contrasting findings. P2X4 is inhibited by several clinically used antidepressants including paroxetine, fluoxetine, desipramine, fluvoxamine, nortriptyline, and clomipramine (Nagata et al., 2009). Of these paroxetine is the most potent, non-competitively inhibiting human P2X4 heterologously expressed in 1321N1 cells at a pIC50 of 5.7 (Nagata et al., 2009; Abdelrahman et al., 2016). The studies suggest that such compounds may mediate analgesic effects in neuropathic pain models via the inhibition of P2X4 (Nagata et al., 2009; Zarei et al., 2014; Yamashita et al., 2016). However, paroxetine is much more potent as a serotonin reuptake inhibitor than an inhibitor of P2X4. There is also evidence that antidepressants may affect lysosomal trafficking of P2X4 in cerebellar microglial cells (Toulme et al., 2010). Furthermore, the hypothesis that the widely used tricyclic antidepressant amitriptyline may exert analgesic effects through P2X4 inhibition has also been tested (Sim and North, 2010). Amitriptyline applied acutely at 10 μM or by pre-incubation for several hours, had no effect on human P2X4 channel activity though modest inhibitory effects of 10 μM amitriptyline were observed at mouse and rat P2X4 (Sim and North, 2010). It is therefore highly unlikely that amitriptyline mediates analgesic effects via P2X4 inhibition in human subjects.
Statins and Cholesterol Depleting Agents
Statins have been described as the most effective class of drugs to reduce serum cholesterol levels (Oesterle et al., 2017). The HMG-CoA reductase inhibitor fluvastatin inhibited the activity of heterologously expressed human P2X4 and native P2X4 in human monocytes (Li and Fountain, 2012). The activity of fluvastatin is likely to be due to depletion of cellular cholesterol as its inhibitory action was mimicked by methyl-β-cyclodextrin and filipin III (Li and Fountain, 2012). Some further studies have shown association of P2X4 within lipid rafts (Allsopp et al., 2010).
Recent Advances in P2X4 Pharmacological Agents
Research surrounding P2X4 has been greatly hindered due to the lack of selective receptor antagonists. For a long time, researchers had to rely on non-selective P2X receptor antagonists such as TNP-ATP, BBG and paroxetine to study P2X4. However, these agents possess low affinity for P2X4 and are significantly more potent at other targets. Recently growing evidence that highlights the importance of P2X4 in health and disease has triggered interest in the development of selective receptor antagonists (Table 1). The discovery of selective P2X4 antagonists will, therefore, present a new avenue for scientific research to selectively target P2X4 therapeutically.
5-BDBD
The benzodiazepine derivative 5-BDBD (5-(3-bromophenyl)-1,3-dihydro-2H-benzofuro[3,2-e]-1,4-diazepin-2-one) was one of the first selective P2X4 antagonists to be described in the literature (Balazs et al., 2013). The original experimental details are documented in a patent (Fischer et al., 2005), however, 5-BDBD is now commercially available. This compound has been reported as a moderately potent and selective P2X4 antagonist with an IC50 value of 1.2 μM in HEK-293 cells expressing human P2X4 (Balazs et al., 2013). The application of two different concentrations of 5-BDBD resulted in a rightward shift in ATP dose-response curve indicating that 5-BDBD acts as a competitive antagonist to P2X4 (Balazs et al., 2013). However, radioligand binding assays illustrated that 5-BDBD was unable to displace [35S]ATPγS binding to P2X4 indicating that rather than acting as a true competitive antagonist, it has an allosteric mechanism at P2X4 (Abdelrahman et al., 2016).
N-Substituted Phenoxazine Derivatives
More recently, compounds derived from N-substituted phenoxazine were identified as potent and selective allosteric P2X4 antagonists (Hernandez-Olmos et al., 2012). One of the derivatives, N-(Benzyloxycarbonyl) phenoxazine (PSB-12054), is an extremely potent antagonist of the human P2X4 (IC50 value of 0.189 μM), but is less potent toward rat P2X4 (IC50 value of 2.1 μM) and mouse P2X4 (IC50 value of 1.77 μM). It has been reported to possess high selectivity for human P2X4 with over 50-fold against P2X2, P2X3 and P2X7 and over 30-fold against P2X1 (Hernandez-Olmos et al., 2012). The second less potent, but more water-soluble, of the two derivatives is N-(p-methylphenylsulfonyl)phenoxazine (PSB-12062). PSB-12062 was shown to have similar potency in all three species: human P2X4 (IC50 value of 1.38 μM), rat P2X4 (IC50 value of 0.928 μM) and mouse P2X4 (IC50 value of 1.76 μM) (Hernandez-Olmos et al., 2012). PSB-12062 has been shown to be allosteric in nature with a 35-fold selectivity toward P2X4 versus P2X1, P2X2, P2X3, and P2X7. However, PSB-12062 was unable to completely block ATP-induced P2X4-mediated calcium influx even when used at high concentrations (>30 μM) (Hernandez-Olmos et al., 2012). A third compound also derived from N-substituted phenoxazine, PSB-12253, was used in a study on HUVECs to investigate P2X4 responses. The effects of this selective antagonist were confirmed by siRNA in HUVECs (Sathanoori et al., 2015).
Carbamazepine Derivatives
Several carbamazepine derivatives were investigated as potential P2X4 antagonists, with N,N-diisopropyl-5H-dibenz[b,f]azepine-5-carboxamide being found to be the most potent toward human P2X4 (IC50 of 3.44 μM) although less potent at mouse and rat P2X4 (Tian et al., 2014). Despite its potency toward human P2X4, it appeared to lack selectivity against two other P2X subtype (P2X1 and P2X3) but was selective versus P2X2 and P2X7. Further optimization will be required to improve the selectivity of this compound.
BX430
One of the most recently discovered P2X4 antagonists is the phenylurea BX430 (1-(2,6-dibromo-4-isopropyl-phenyl)-3-(3-pyriudyl)urea which has been shown through a patch-clamp study to have sub-micromolar potency (IC50 value of 0.54 μM) and a 10 – 100 fold selectivity toward P2X4 versus other P2X subtypes (Ase et al., 2015). Acting through a non-competitive allosteric route, BX430 has been shown to be able to potently antagonize zebrafish P2X4 but has no effect on rat and mouse P2X4 orthologs (Ase et al., 2015). BX430 was seen to completely abolish ivermectin facilitated pore formation and, according to the authors, BX430 is the only known compound to inhibit P2X4 mediated pore formation (Ase et al., 2015).
NP-1815-PX
Screening a chemical library identified the compound NP-1815-PX (5-[3-(5-thioxo-4H-[1,2,4]oxadiazol-3-yl)phenyl]-1H-naphtho[1,2-b][1,4]diazepine-2,4(3H,5H)-dione) as a novel human P2X4 antagonist (IC50 value of 0.26 μM) (Matsumura et al., 2016). The inhibitory effect of NP-1815-PX was also shown on rat and mouse P2X4 and it showed selectivity against other P2X receptors (Matsumura et al., 2016). Intrathecal administration of NP-1815-PX alleviated nerve damage-associated mechanical allodynia in a chronic pain model and in a herpetic pain model without altering acute pain sensing (Matsumura et al., 2016).
Future Perspectives
The discovery of new pharmacological tools, some of which are now available commercially, will facilitate our understanding of P2X4 cellular function in health and disease. In addition, such tools may provide chemical scaffolds for the development of new drugs that may be beneficial in the treatment of neuropathic pain. P2X4-selective antagonists with high potency, no species-restricted effect, water-solubility and, most importantly, an anti-allodynia effect in rodent chronic pain models remain to be identified.
Author Contributions
LS initiated, designed, co-wrote, and edited the article. JL co-wrote the article and prepared the table. LB co-wrote and prepared the Figures. KD co-wrote the article. SF co-wrote and edited the article.
Funding
Both LS and SF are funded by BBSRC.
Conflict of Interest Statement
The authors declare that the research was conducted in the absence of any commercial or financial relationships that could be construed as a potential conflict of interest.
References
Abdelrahman, A., Namasivayam, V., Hinz, S., Schiedel, A. C., Köse, M., Burton, M., et al. (2016). Characterization of P2X4 receptor agonists and antagonists by calcium influx and radioligand binding studies. Biochem. Pharmacol. 125, 41–54. doi: 10.1016/j.bcp.2016.11.016
Acuna-Castillo, C., Morales, B., and Huidobro-Toro, J. P. (2000). Zinc and copper modulate differentially the P2X4 receptor. J. Neurochem. 74, 1529–1537. doi: 10.1046/j.1471-4159.2000.0741529.x
Agresti, C., Meomartini, M. E., Amadio, S., Ambrosini, E., Volonté, C., Aloisi, F., et al. (2005). ATP regulates oligodendrocyte progenitor migration, proliferation, and differentiation: involvement of metabotropic P2 receptors. Brain Res. Rev. 48, 157–165. doi: 10.1016/j.brainresrev.2004.12.005
Allsopp, R. C., Lalo, U., and Evans, R. J. (2010). Lipid raft association and cholesterol sensitivity of p2x1-4 receptors for atp: chimeras and point mutants identify intracellular amino-terminal residues involved in lipid regulation of p2x1 receptors. J. Biol. Chem. 285, 32770–32777. doi: 10.1074/jbc.M110.148940
Amadio, S., Montilli, C., Picconi, B., Calabresi, P., and Volonte, C. (2007). Mapping P2X and P2Y receptor proteins in striatum and substantia nigra: an immunohistological study. Purinergic Signal. 3, 389–398. doi: 10.1007/s11302-007-9069-8
Antonio, L. S., Stewart, A. P., Xu, X. J., Varanda, W. A., Murrell-Lagnado, R. D., and Edwardson, J. M. (2011). P2X4 receptors interact with both P2X2 and P2X7 receptors in the form of homotrimers. Br. J. Pharmacol. 163, 1069–1077. doi: 10.1111/j.1476-5381.2011.01303.x
Asatryan, L., Nam, H. W., Lee, M. R., Thakkar, M. M., Saeed Dar, M., Davies, D. L., et al. (2011). Implication of the purinergic system in alcohol use disorders. Alcohol. Clin. Exp. Res. 35, 584–594. doi: 10.1111/j.1530-0277.2010.01379.x
Asatryan, L., Popova, M., Perkins, D., Trudell, J. R., Alkana, R. L., and Davies, D. L. (2010). Ivermectin antagonizes ethanol inhibition in purinergic P2X4 receptors. J. Pharmacol. Exp. Ther. 334, 720–728. doi: 10.1124/jpet.110.167908
Asatryan, L., Yardley, M. M., Khoja, S., Trudell, J. R., Hyunh, N., Louie, S. G., et al. (2014). Avermectins differentially affect ethanol intake and receptor function: implications for developing new therapeutics for alcohol use disorders. Int. J. Neuropsychopharmacol. 17, 907–916. doi: 10.1017/S1461145713001703
Ase, A. R., Honson, N. S., Zaghdane, H., Pfeifer, T. A., and Seguela, P. (2015). Identification and characterization of a selective allosteric antagonist of human P2X4 receptor channels. Mol. Pharmacol. 87, 606–616. doi: 10.1124/mol.114.096222
Balazs, B., Danko, T., Kovacs, G., Koles, L., Hediger, M. A., and Zsembery, A. (2013). Investigation of the inhibitory effects of the benzodiazepine derivative, 5-BDBD on P2X4 purinergic receptors by two complementary methods. Cell Physiol. Biochem. 32, 11–24. doi: 10.1159/000350119
Banke, T. G., Chaplan, S. R., and Wickenden, A. D. (2010). Dynamic changes in the TRPA1 selectivity filter lead to progressive but reversible pore dilation. Am. J. Physiol. Cell Physiol. 298, C1457–C1468. doi: 10.1152/ajpcell.00489.2009
Bardoni, R., Goldstein, P. A., Lee, C. J., Gu, J. G., and Macdermott, A. B. (1997). ATP P2X Receptors Mediate fast synaptic transmission in the dorsal horn of the rat spinal cord. J. Neurosci. 17, 5297–5304.
Basbaum, A. I., Bautista, D. M., Scherrer, G., and Julius, D. (2009). Cellular and molecular mechanisms of pain. Cell 139, 267–284. doi: 10.1016/j.cell.2009.09.028
Baxter, A. W., Choi, S. J., Sim, J. A., and North, R. A. (2011). Role of P2X4 receptors in synaptic strengthening in mouse CA1 hippocampal neurons. Eur. J. Neurosci. 34, 213–220. doi: 10.1111/j.1460-9568.2011.07763.x
Bernier, L. P., Ase, A. R., Boue-Grabot, E., and Seguela, P. (2012). P2X4 receptor channels form large noncytolytic pores in resting and activated microglia. Glia 60, 728–737. doi: 10.1002/glia.22301
Bhattacharya, A., and Biber, K. (2016). The microglial ATP-gated ion channel P2X7 as a CNS drug target. Glia 64, 1772–1787. doi: 10.1002/glia.23001
Biber, K., Tsuda, M., Tozaki-Saitoh, H., Tsukamoto, K., Toyomitsu, E., Masuda, T., et al. (2011). Neuronal CCL21 up-regulates microglia P2X4 expression and initiates neuropathic pain development. EMBO J. 30, 1864–1873. doi: 10.1038/emboj.2011.89
Bintig, W., Begandt, D., Schlingmann, B., Gerhard, L., Pangalos, M., Dreyer, L., et al. (2012). Purine receptors and Ca(2+) signalling in the human blood-brain barrier endothelial cell line hCMEC/D3. Purinergic Sig. 8, 71–80. doi: 10.1007/s11302-011-9262-7
Bo, X., Kim, M., Nori, S. L., Schoepfer, R., Burnstock, G., and North, R. A. (2003). Tissue distribution of P2X4 receptors studied with an ectodomain antibody. Cell Tissue Res. 313, 159–165. doi: 10.1007/s00441-003-0758-5
Bobanovic, L. K., Royle, S. J., and Murrell-Lagnado, R. D. (2002). P2X receptor trafficking in neurons is subunit specific. J. Neurosci. 22, 4814–4824.
Boumechache, M., Masin, M., Edwardson, J. M., Gorecki, D. C., and Murrell-Lagnado, R. (2009). Analysis of assembly and trafficking of native P2X4 and P2X7 receptor complexes in rodent immune cells. J. Biol. Chem. 284, 13446–13454. doi: 10.1074/jbc.M901255200
Bowler, J. W., Jayne Bailey, R., Alan North, R., and Surprenant, A. (2003). P2X4, P2Y1 and P2Y2 receptors on rat alveolar macrophages. Br. J. Pharmacol. 140, 567–575. doi: 10.1038/sj.bjp.0705459
Browne, L. E., Compan, V., Bragg, L., and North, R. A. (2013). P2X7 receptor channels allow direct permeation of nanometer-sized dyes. J. Neurosci. 33, 3557–3566. doi: 10.1523/JNEUROSCI.2235-12.2013
Buell, G., Lewis, C., Collo, G., North, R. A., and Surprenant, A. (1996). An antagonist-insensitive P2X receptor expressed in epithelia and brain. EMBO J. 15, 55–62.
Burnstock, G. (2006). Historical review: ATP as a neurotransmitter. Trends Pharmacol. Sci. 27, 166–176. doi: 10.1016/j.tips.2006.01.005
Burnstock, G., and Kennedy, C. (2011). “P2X Receptors in Health and Disease,” in Advances in Pharmacology, Chap. 11, eds A. J. Kenneth and L. Joel (Cambridge, MA: Academic Press), 333–372. doi: 10.1016/b978-0-12-385526-8.00011-4
Cao, Q., Zhong, X. Z., Zou, Y., Murrell-Lagnado, R., Zhu, M. X., and Dong, X. P. (2015). Calcium release through P2X4 activates calmodulin to promote endolysosomal membrane fusion. J. Cell Biol. 209, 879–894. doi: 10.1083/jcb.201409071
Cardoso, A. M., Schetinger, M. R., Correia-De-Sa, P., and Sevigny, J. (2015). Impact of ectonucleotidases in autonomic nervous functions. Auton. Neurosci. 191, 25–38. doi: 10.1016/j.autneu.2015.04.014
Cham, J. L., Owens, N. C., Barden, J. A., Lawrence, A. J., and Badoer, E. (2006). P2X purinoceptor subtypes on paraventricular nucleus neurones projecting to the rostral ventrolateral medulla in the rat. Exp. Physiol. 91, 403–411. doi: 10.1113/expphysiol.2005.032409
Chung, M. K., Guler, A. D., and Caterina, M. J. (2008). TRPV1 shows dynamic ionic selectivity during agonist stimulation. Nat. Neurosci. 11, 555–564. doi: 10.1038/nn.2102
Clarke, C. E., Benham, C. D., Bridges, A., George, A. R., and Meadows, H. J. (2000). Mutation of histidine 286 of the human P2X4 purinoceptor removes extracellular pH sensitivity. J. Physiol. 523(Pt 3), 697–703. doi: 10.1111/j.1469-7793.2000.00697.x
Coddou, C., Acuna-Castillo, C., Bull, P., and Huidobro-Toro, J. P. (2007). Dissecting the facilitator and inhibitor allosteric metal sites of the P2X4 receptor channel: critical roles of CYS132 for zinc potentiation and ASP138 for copper inhibition. J. Biol. Chem. 282, 36879–36886. doi: 10.1074/jbc.M706925200
Coddou, C., Lorca, R. A., Acuna-Castillo, C., Grauso, M., Rassendren, F., and Huidobro-Toro, J. P. (2005). Heavy metals modulate the activity of the purinergic P2X4 receptor. Toxicol. Appl. Pharmacol. 202, 121–131. doi: 10.1016/j.taap.2004.06.015
Coddou, C., Morales, B., Gonzalez, J., Grauso, M., Gordillo, F., Bull, P., et al. (2003). Histidine 140 plays a key role in the inhibitory modulation of the P2X4 nucleotide receptor by copper but not zinc. J. Biol. Chem. 278, 36777–36785. doi: 10.1074/jbc.M305177200
Cohen, S. P., and Mao, J. (2014). Neuropathic pain: mechanisms and their clinical implications. BMJ 348:f7656. doi: 10.1136/bmj.f7656
Coull, J. A. M., Beggs, S., Boudreau, D., Boivin, D., Tsuda, M., Inoue, K., et al. (2005). BDNF from microglia causes the shift in neuronal anion gradient underlying neuropathic pain. Nature 438, 1017–1021. doi: 10.1038/nature04223
Davies, D. L., Kochegarov, A. A., Kuo, S. T., Kulkarni, A. A., Woodward, J. J., King, B. F., et al. (2005). Ethanol differentially affects ATP-gated P2X3 and P2X4 receptor subtypes expressed in Xenopus oocytes. Neuropharmacology 49, 243–253. doi: 10.1016/j.neuropharm.2005.03.015
de Rivero Vaccari, J. P., Bastien, D., Yurcisin, G., Pineau, I., Dietrich, W. D., De Koninck, Y., et al. (2012). P2X4 receptors influence inflammasome activation after spinal cord injury. J. Neurosci. 32, 3058–3066. doi: 10.1523/JNEUROSCI.4930-11.2012
Di Virgilio, F., Evans, R. J., Jarvis, M. F., Kennedy, C., Khakh, B. S., Pellegatti, P., et al. (2015). P2X receptors; P2X4. IUPHAR/BPS Guide to Pharmacology. Available at: http://www.guidetopharmacology.org/GRAC/FamilyDisplayForward?familyId=77.
DiSabato, D. J., Quan, N., and Godbout, J. P. (2016). Neuroinflammation: the devil is in the details. J. Neurochem. 139, 136–153. doi: 10.1111/jnc.13607
Dixon, S. J., Yu, R., Panupinthu, N., and Wilson, J. X. (2004). Activation of P2 nucleotide receptors stimulates acid efflux from astrocytes. Glia 47, 367–376. doi: 10.1002/glia.20048
Egan, T. M., and Khakh, B. S. (2004). Contribution of calcium ions to P2X channel responses. J. Neurosci. 24, 3413–3423. doi: 10.1523/JNEUROSCI.5429-03.2004
Farber, K., and Kettenmann, H. (2006). Functional role of calcium signals for microglial function. Glia 54, 656–665. doi: 10.1002/glia.20412
Ferrini, F., Trang, T., Mattioli, T.-A. M., Laffray, S., Del’guidice, T., Lorenzo, L.-E., et al. (2013). Morphine hyperalgesia gated through microglia-mediated disruption of neuronal Cl- homeostasis. Nat. Neurosci 16, 183–192. doi: 10.1038/nn.3295
Fischer, R., Kalthof, B., Gruetzmann, R., Woltering, E., Stelte-Ludwig, B., and Wuttke, M. (2005). Benzofuro-1,4-diazepin-2-one Derivatives. US CA 2519987 A1.
Franke, H., Grosche, J., Schädlich, H., Krügel, U., Allgaier, C., and Illes, P. (2001). P2X receptor expression on astrocytes in the nucleus accumbens of rats. Neuroscience 108, 421–429. doi: 10.1016/S0306-4522(01)00416-X
Franklin, K. M., Asatryan, L., Jakowec, M. W., Trudell, J. R., Bell, R. L., and Davies, D. L. (2014). P2X4 receptors (P2X4Rs) represent a novel target for the development of drugs to prevent and/or treat alcohol use disorders. Front. Neurosci. 8:176. doi: 10.3389/fnins.2014.00176
Franklin, K. M., Hauser, S. R., Lasek, A. W., Bell, R. L., and Mcbride, W. J. (2015). Involvement of purinergic P2X4 receptors in alcohol intake of high-alcohol-drinking (HAD) rats. Alcohol. Clin. Exp. Res. 39, 2022–2031. doi: 10.1111/acer.12836
Fumagalli, M., Brambilla, R., D’ambrosi, N., Volonté, C., Matteoli, M., Verderio, C., et al. (2003). Nucleotide-mediated calcium signaling in rat cortical astrocytes: Role of P2X and P2Y receptors. Glia 43, 218–230. doi: 10.1002/glia.10248
Gao, C., Yu, Q., Xu, H., Zhang, L., Liu, J., Jie, Y., et al. (2015). Roles of the lateral fenestration residues of the P2X(4) receptor that contribute to the channel function and the deactivation effect of ivermectin. Purinergic Signal. 11, 229–238. doi: 10.1007/s11302-015-9448-5
Garcia-Guzman, M., Soto, F., Gomez-Hernandez, J. M., Lund, P. E., and Stuhmer, W. (1997). Characterization of recombinant human P2X4 receptor reveals pharmacological differences to the rat homologue. Mol. Pharmacol. 51, 109–118.
Gofman, L., Cenna, J. M., and Potula, R. (2014). P2X4 receptor regulates alcohol-induced responses in microglia. J. Neuroimmune Pharmacol. 9, 668–678. doi: 10.1007/s11481-014-9559-8
Gofman, L., Fernandes, N. C., and Potula, R. (2016). Relative role of Akt, ERK and CREB in alcohol-induced microglia P2X4R Receptor Expression. Alcohol Alcohol. 51, 647–654. doi: 10.1093/alcalc/agw009
Guo, L. H., Trautmann, K., and Schluesener, H. J. (2005). Expression of P2X4 receptor by lesional activated microglia during formalin-induced inflammatory pain. J. Neuroimmunol. 163, 120–127. doi: 10.1016/j.jneuroim.2005.03.007
Hattori, M., and Gouaux, E. (2012). Molecular mechanism of ATP binding and ion channel activation in P2X receptors. Nature 485, 207–212. doi: 10.1038/nature11010
He, M.-L., Gonzalez-Iglesias, A. E., and Stojilkovic, S. S. (2003). Role of Nucleotide P2 receptors in calcium signaling and prolactin release in pituitary lactotrophs. J. Biol. Chem. 278, 46270–46277. doi: 10.1074/jbc.M309005200
Hernandez-Olmos, V., Abdelrahman, A., El-Tayeb, A., Freudendahl, D., Weinhausen, S., and Muller, C. E. (2012). N-substituted phenoxazine and acridone derivatives: structure-activity relationships of potent P2X4 receptor antagonists. J. Med. Chem. 55, 9576–9588. doi: 10.1021/jm300845v
Horvath, R. J., and DeLeo, J. A. (2009). Morphine enhances microglial migration through modulation of P2X4 receptor signaling. J. Neurosci. 29, 998–1005. doi: 10.1523/JNEUROSCI.4595-08.2009
Horvath, R. J., Romero-Sandoval, E. A., and De Leo, J. A. (2010). Inhibition of microglial P2X4 receptors attenuates morphine tolerance, Iba1, GFAP and mu opioid receptor protein expression while enhancing perivascular microglial ED2. Pain 150, 401–413. doi: 10.1016/j.pain.2010.02.042
Huang, P., Zou, Y., Zhong, X. Z., Cao, Q., Zhao, K., Zhu, M. X., et al. (2014). P2X4 forms functional ATP-activated cation channels on lysosomal membranes regulated by luminal pH. J. Biol. Chem. 289, 17658–17667. doi: 10.1074/jbc.M114.552158
Huynh, N., Arabian, N., Naito, A., Louie, S., Jakowec, M. W., Asatryan, L., et al. (2017). Preclinical development of moxidectin as a novel therapeutic for alcohol use disorder. Neuropharmacology 113, 60–70. doi: 10.1016/j.neuropharm.2016.09.016
Idzko, M., Ferrari, D., and Eltzschig, H. K. (2014). Nucleotide signalling during inflammation. Nature 509, 310–317. doi: 10.1038/nature13085
Igawa, T., Abe, Y., Tsuda, M., Inoue, K., and Ueda, T. (2015). Solution structure of the rat P2X4 receptor head domain involved in inhibitory metal binding. FEBS Lett. 589, 680–686. doi: 10.1016/j.febslet.2015.01.034
Inoue, K., and Tsuda, M. (2012). P2X4 receptors of microglia in neuropathic pain. CNS Neurol. Disord. Drug Targets 11, 699–704. doi: 10.2174/187152712803581065
Ito, K., Chihara, Y., Iwasaki, S., Komuta, Y., Sugasawa, M., and Sahara, Y. (2010). Functional ligand-gated purinergic receptors (P2X) in rat vestibular ganglion neurons. Hear. Res. 267, 89–95. doi: 10.1016/j.heares.2010.03.081
Jabs, R., Matthias, K., Grote, A., Grauer, M., Seifert, G., and Steinhauser, C. (2007). Lack of P2X receptor mediated currents in astrocytes and GluR type glial cells of the hippocampal CA1 region. Glia 55, 1648–1655. doi: 10.1002/glia.20580
Jarvis, M. F. (2010). The neural-glial purinergic receptor ensemble in chronic pain states. Trends Neurosci. 33, 48–57. doi: 10.1016/j.tins.2009.10.003
Jelinkova, I., Yan, Z., Liang, Z., Moonat, S., Teisinger, J., Stojilkovic, S. S., et al. (2006). Identification of P2X4 receptor-specific residues contributing to the ivermectin effects on channel deactivation. Biochem. Biophys. Res. Commun. 349, 619–625. doi: 10.1016/j.bbrc.2006.08.084
Jo, Y. H., Donier, E., Martinez, A., Garret, M., Toulme, E., and Boue-Grabot, E. (2011). Cross-talk between P2X4 and gamma-aminobutyric acid, type A receptors determines synaptic efficacy at a central synapse. J. Biol. Chem. 286, 19993–20004. doi: 10.1074/jbc.M111.231324
Joers, V., Tansey, M. G., Mulas, G., and Carta, A. R. (2016). Microglial phenotypes in Parkinson’s disease and animal models of the disease. Prog. Neurobiol. [Epub ahead of print].
Jones, C. A., Chessell, I. P., Simon, J., Barnard, E. A., Miller, K. J., Michel, A. D., et al. (2000). Functional characterization of the P2X4 receptor orthologues. Br. J. Pharmacol. 129, 388–394. doi: 10.1038/sj.bjp.0703059
Kawate, T., Michel, J. C., Birdsong, W. T., and Gouaux, E. (2009). Crystal structure of the ATP-gated P2X4 ion channel in the closed state. Nature 460, 592–598. doi: 10.1038/nature08198
Kerr, B. J., Bradbury, E. J., Bennett, D. L., Trivedi, P. M., Dassan, P., French, J., et al. (1999). Brain-derived neurotrophic factor modulates nociceptive sensory inputs and NMDA-evoked responses in the rat spinal cord. J. Neurosci. 19, 5138–5148.
Kettenmann, H., Hanisch, U. K., Noda, M., and Verkhratsky, A. (2011). Physiology of microglia. Physiol. Rev. 91, 461–553. doi: 10.1152/physrev.00011.2010
Khakh, B. S., Bao, X. R., Labarca, C., and Lester, H. A. (1999a). Neuronal P2X transmitter-gated cation channels change their ion selectivity in seconds. Nat. Neurosci. 2, 322–330.
Khakh, B. S., Proctor, W. R., Dunwiddie, T. V., Labarca, C., and Lester, H. A. (1999b). Allosteric control of gating and kinetics at P2X4 receptor channels. J. Neurosci. 19, 7289–7299.
Khakh, B. S., and North, R. A. (2006). P2X receptors as cell-surface ATP sensors in health and disease. Nature 442, 527–532. doi: 10.1038/nature04886
Khakh, B. S., and North, R. A. (2012). Neuromodulation by extracellular ATP and P2X receptors in the CNS. Neuron 76, 51–69. doi: 10.1016/j.neuron.2012.09.024
Khakh, B. S., Zhou, X., Sydes, J., Galligan, J. J., and Lester, H. A. (2000). State-dependent cross-inhibition between transmitter-gated cation channels. Nature 406, 405–410. doi: 10.1038/35019066
Khoja, S., Shah, V., Garcia, D., Asatryan, L., Jakowec, M. W., and Davies, D. L. (2016). Role of purinergic P2X4 receptors in regulating striatal dopamine homeostasis and dependent behaviors. J. Neurochem. 139, 134–148. doi: 10.1111/jnc.13734
Kimpel, M. W., Strother, W. N., Mcclintick, J. N., Carr, L. G., Liang, T., Edenberg, H. J., et al. (2007). Functional gene expression differences between inbred alcohol-preferring and –non-preferring rats in five brain regions. Alcohol 41, 95–132. doi: 10.1016/j.alcohol.2007.03.003
Kobayashi, K., Fukuoka, T., Yamanaka, H., Dai, Y., Obata, K., Tokunaga, A., et al. (2005). Differential expression patterns of mRNAs for P2X receptor subunits in neurochemically characterized dorsal root ganglion neurons in the rat. J. Comp. Neurol. 481, 377–390. doi: 10.1002/cne.20393
Krause, R. M., Buisson, B., Bertrand, S., Corringer, P.-J., Galzi, J.-L., Changeux, J.-P., et al. (1998). Ivermectin: a positive allosteric effector of the α7 neuronal nicotinic acetylcholine receptor. Mol. Pharmacol. 53, 283.
Krůšek, J., and Zemková, H. (1994). Effect of ivermectin on γ-aminobutyric acid-induced chloride currents in mouse hippocampal embryonic neurones. Eur. J. Pharmacol. 259, 121–128. doi: 10.1016/0014-2999(94)90500-2
Kukley, M., Barden, J. A., Steinhäuser, C., and Jabs, R. (2001). Distribution of P2X receptors on astrocytes in juvenile rat hippocampus. Glia 36, 11–21. doi: 10.1002/glia.1091
Laing, R., Gillan, V., and Devaney, E. (2017). Ivermectin – Old Drug, New Tricks? Trends Parasitol. [Epub ahead of print].
Lalo, U., Pankratov, Y., Wichert, S. P., Rossner, M. J., North, R. A., Kirchhoff, F., et al. (2008). P2X1 and P2X5 subunits form the functional P2X receptor in mouse cortical astrocytes. J. Neurosci. 28, 5473–5480. doi: 10.1523/JNEUROSCI.1149-08.2008
Lalo, U., Verkhratsky, A., and Pankratov, Y. (2007). Ivermectin potentiates ATP-induced ion currents in cortical neurones: evidence for functional expression of P2X4 receptors? Neurosci. Lett. 421, 158–162. doi: 10.1016/j.neulet.2007.03.078
Le, K. T., Babinski, K., and Seguela, P. (1998). Central P2X4 and P2X6 channel subunits coassemble into a novel heteromeric ATP receptor. J. Neurosci. 18, 7152–7159.
Lê, K. T., Villeneuve, P., Ramjaun, A. R., Mcpherson, P. S., Beaudet, A., and Séguéla, P. (1998). Sensory presynaptic and widespread somatodendritic immunolocalization of central ionotropic P2X ATP receptors. Neuroscience 83, 177–190. doi: 10.1016/S0306-4522(97)00365-5
Li, F., Wang, L., Li, J. W., Gong, M., He, L., Feng, R., et al. (2011). Hypoxia induced amoeboid microglial cell activation in postnatal rat brain is mediated by ATP receptor P2X4. BMC Neurosci. 12:111. doi: 10.1186/1471-2202-12-111
Li, J., and Fountain, S. J. (2012). Fluvastatin suppresses native and recombinant human P2X4 receptor function. Purinergic Signal. 8, 311–316. doi: 10.1007/s11302-011-9289-9
Li, M., Toombes, G. E. S., Silberberg, S. D., and Swartz, K. J. (2015). Physical basis of apparent pore dilation of ATP-activated P2X receptor channels. Nat. Neurosci. 18, 1577–1583. doi: 10.1038/nn.4120
Luo, J., Yin, G.-F., Gu, Y.-Z., Liu, Y., Dai, J.-P., Li, C., et al. (2006). Characterization of three types of ATP-activated current in relation to P2X subunits in rat trigeminal ganglion neurons. Brain Res. 1115, 9–15. doi: 10.1016/j.brainres.2006.07.084
Mapplebeck, J. C., Beggs, S., and Salter, M. W. (2016). Sex differences in pain: a tale of two immune cells. Pain 157(Suppl. 1), S2–S6. doi: 10.1097/j.pain.0000000000000389
Masuda, T., Iwamoto, S., Yoshinaga, R., Tozaki-Saitoh, H., Nishiyama, A., Mak, T. W., et al. (2014). Transcription factor IRF5 drives P2X4R+-reactive microglia gating neuropathic pain. Nat. Commun. 5:3771. doi: 10.1038/ncomms4771
Matsumura, Y., Yamashita, T., Sasaki, A., Nakata, E., Kohno, K., Masuda, T., et al. (2016). A novel P2X4 receptor-selective antagonist produces anti-allodynic effect in a mouse model of herpetic pain. Sci. Rep. 6:32461. doi: 10.1038/srep32461
Miklavc, P., Thompson, K. E., and Frick, M. (2013). A new role for P2X4 receptors as modulators of lung surfactant secretion. Front. Cell. Neurosci 7:171. doi: 10.3389/fncel.2013.00171
Miller, K. J., Michel, A. D., Chessell, I. P., and Humphrey, P. P. (1998). Cibacron blue allosterically modulates the rat P2X4 receptor. Neuropharmacology 37, 1579–1586. doi: 10.1016/S0028-3908(98)00153-1
Nagata, K., Imai, T., Yamashita, T., Tsuda, M., Tozaki-Saitoh, H., and Inoue, K. (2009). Antidepressants inhibit P2X4 receptor function: a possible involvement in neuropathic pain relief. Mol. Pain 5:20. doi: 10.1186/1744-8069-5-20
Nasu-Tada, K., Koizumi, S., Tsuda, M., Kunifusa, E., and Inoue, K. (2006). Possible involvement of increase in spinal fibronectin following peripheral nerve injury in upregulation of microglial P2X4, a key molecule for mechanical allodynia. Glia 53, 769–775. doi: 10.1002/glia.20339
Neher, E., and Sakaba, T. (2008). Multiple roles of calcium ions in the regulation of neurotransmitter release. Neuron 59, 861–872. doi: 10.1016/j.neuron.2008.08.019
Nicke, A., Kerschensteiner, D., and Soto, F. (2005). Biochemical and functional evidence for heteromeric assembly of P2X1 and P2X4 subunits. J. Neurochem. 92, 925–933. doi: 10.1111/j.1471-4159.2004.02939.x
Nörenberg, W., Sobottka, H., Hempel, C., Plötz, T., Fischer, W., Schmalzing, G., et al. (2012). Positive allosteric modulation by ivermectin of human but not murine P2X7 receptors. Br. J. Pharmacol. 167, 48–66. doi: 10.1111/j.1476-5381.2012.01987.x
North, R. A. (2002). Molecular physiology of P2X receptors. Physiol. Rev. 82, 1013–1067. doi: 10.1152/physrev.00015.2002
Oesterle, A., Laufs, U., and Liao, J. K. (2017). Pleiotropic effects of statins on the cardiovascular system. Circ. Res. 120, 229. doi: 10.1161/CIRCRESAHA.116.308537
Ostrovskaya, O., Asatryan, L., Wyatt, L., Popova, M., Li, K., Peoples, R. W., et al. (2011). Ethanol is a fast channel inhibitor of P2X4 receptors. J. Pharmacol. Exp. Ther. 337, 171–179. doi: 10.1124/jpet.110.176990
Ousingsawat, J., Wanitchakool, P., Kmit, A., Romao, A. M., Jantarajit, W., Schreiber, R., et al. (2015). Anoctamin 6 mediates effects essential for innate immunity downstream of P2X7 receptors in macrophages. Nat. Commun. 6:6245. doi: 10.1038/ncomms7245
Ozaki, T., Muramatsu, R., Sasai, M., Yamamoto, M., Kubota, Y., Fujinaka, T., et al. (2016). The P2X4 receptor is required for neuroprotection via ischemic preconditioning. Sci. Rep. 6, 25893. doi: 10.1038/srep25893
Pelegrin, P., and Surprenant, A. (2006). Pannexin-1 mediates large pore formation and interleukin-1β release by the ATP-gated P2X<sub>7</sub> receptor. EMBO J. 25, 5071. doi: 10.1038/sj.emboj.7601378
Popova, M., Asatryan, L., Ostrovskaya, O., Wyatt, L. R., Li, K., Alkana, R. L., et al. (2010). A point mutation in the ectodomain-transmembrane 2 interface eliminates the inhibitory effects of ethanol in P2X4 receptors. J. Neurochem. 112, 307–317. doi: 10.1111/j.1471-4159.2009.06460.x
Popova, M., Trudell, J., Li, K., Alkana, R., Davies, D., and Asatryan, L. (2013). Tryptophan 46 is a site for ethanol and ivermectin action in P2X4 receptors. Purinergic Signal 9, 621–632. doi: 10.1007/s11302-013-9373-4
Potula, R., Haorah, J., Knipe, B., Leibhart, J., Chrastil, J., Heilman, D., et al. (2006). Alcohol abuse enhances neuroinflammation and impairs immune responses in an animal model of human immunodeficiency virus-1 encephalitis. Am. J. Pathol. 168, 1335–1344. doi: 10.2353/ajpath.2006.051181
Priel, A., and Silberberg, S. D. (2004). Mechanism of ivermectin facilitation of human P2X4 receptor channels. J. Gen. Physiol. 123, 281–293. doi: 10.1085/jgp.200308986
Qureshi, O. S., Paramasivam, A., Yu, J. C., and Murrell-Lagnado, R. D. (2007). Regulation of P2X4 receptors by lysosomal targeting, glycan protection and exocytosis. J. Cell Sci. 120, 3838–3849. doi: 10.1242/jcs.010348
Ransohoff, R. M. (2016). How neuroinflammation contributes to neurodegeneration. Science 353, 777–783. doi: 10.1126/science.aag2590
Raouf, R., Chabot-Dore, A. J., Ase, A. R., Blais, D., and Seguela, P. (2007). Differential regulation of microglial P2X4 and P2X7 ATP receptors following LPS-induced activation. Neuropharmacology 53, 496–504. doi: 10.1016/j.neuropharm.2007.06.010
Robinson, L. E., and Murrell-Lagnado, R. D. (2013). The trafficking and targeting of P2X receptors. Front. Cell. Neurosci. 7:233. doi: 10.3389/fncel.2013.00233
Rokic, M. B., Stojilkovic, S. S., Vavra, V., Kuzyk, P., Tvrdonova, V., and Zemkova, H. (2013). Multiple roles of the extracellular vestibule amino acid residues in the function of the rat P2X4 receptor. PLoS ONE 8:e59411. doi: 10.1371/journal.pone.0059411
Royle, S. J., Qureshi, O. S., Bobanovic, L. K., Evans, P. R., Owen, D. J., and Murrell-Lagnado, R. D. (2005). Non-canonical YXXGPhi endocytic motifs: recognition by AP2 and preferential utilization in P2X4 receptors. J. Cell Sci. 118, 3073–3080. doi: 10.1242/jcs.02451
Rubio, M. E., and Soto, F. (2001). Distinct localization of P2X receptors at excitatory postsynaptic specializations. J. Neurosci. 21, 641–653.
Saijo, K., and Glass, C. K. (2011). Microglial cell origin and phenotypes in health and disease. Nat. Rev. Immunol. 11, 775–787. doi: 10.1038/nri3086
Samways, D. S., Khakh, B. S., and Egan, T. M. (2012). Allosteric modulation of Ca2+ flux in ligand-gated cation channel (P2X4) by actions on lateral portals. J. Biol. Chem. 287, 7594–7602. doi: 10.1074/jbc.M111.322461
Samways, D. S. K., Khakh, B. S., Dutertre, S., and Egan, T. M. (2011). Preferential use of unobstructed lateral portals as the access route to the pore of human ATP-gated ion channels (P2X receptors). Proc. Natl. Acad. Sci. U.S.A. 108, 13800–13805. doi: 10.1073/pnas.1017550108
Sathanoori, R., Sward, K., Olde, B., and Erlinge, D. (2015). The ATP Receptors P2X7 and P2X4 modulate high glucose and palmitate-induced inflammatory responses in endothelial cells. PLoS ONE 10:e0125111. doi: 10.1371/journal.pone.0125111
Saul, A., Hausmann, R., Kless, A., and Nicke, A. (2013). Heteromeric assembly of P2X subunits. Front. Cell. Neurosci. 7:250. doi: 10.3389/fncel.2013.00250
Schwab, J. M., Guo, L., and Schluesener, H. J. (2005). Spinal cord injury induces early and persistent lesional P2X4 receptor expression. J. Neuroimmunol. 163, 185–189. doi: 10.1016/j.jneuroim.2005.02.016
Shinozaki, Y., Sumitomo, K., Tsuda, M., Koizumi, S., Inoue, K., and Torimitsu, K. (2009). Direct Observation of ATP-induced conformational changes in single P2X4 receptors. PLoS Biol. 7:e1000103. doi: 10.1371/journal.pbio.1000103
Shrivastava, A. N., Triller, A., and Sieghart, W. (2011). GABA(A) receptors: post-synaptic co-localization and cross-talk with other receptors. Front. Cell. Neurosci. 5:7. doi: 10.3389/fncel.2011.00007
Silberberg, S. D., Li, M., and Swartz, K. J. (2007). Ivermectin interaction with transmembrane helices reveals widespread rearrangements during opening of P2X receptor channels. Neuron 54, 263–274. doi: 10.1016/j.neuron.2007.03.020
Sim, J. A., Chaumont, S., Jo, J., Ulmann, L., Young, M. T., Cho, K., et al. (2006). Altered hippocampal synaptic potentiation in P2X4 knock-out mice. J. Neurosci. 26, 9006–9009. doi: 10.1523/JNEUROSCI.2370-06.2006
Sim, J. A., and North, R. A. (2010). Amitriptyline does not block the action of ATP at human P2X4 receptor. Br. J. Pharmacol. 160, 88–92. doi: 10.1111/j.1476-5381.2010.00683.x
Sorge, R. E., Mapplebeck, J. C., Rosen, S., Beggs, S., Taves, S., Alexander, J. K., et al. (2015). Different immune cells mediate mechanical pain hypersensitivity in male and female mice. Nat. Neurosci. 18, 1081–1083. doi: 10.1038/nn.4053
Soto, F., Garcia-Guzman, M., Gomez-Hernandez, J. M., Hollmann, M., Karschin, C., and Stuhmer, W. (1996a). P2X4: an ATP-activated ionotropic receptor cloned from rat brain. Proc. Natl. Acad. Sci. U.S.A. 93, 3684–3688.
Soto, F., Garcia-Guzman, M., Karschin, C., and Stühmer, W. (1996b). Cloning and tissue distribution of a novel P2X receptor from rat brain. Biochem. Biophys. Res. Commun. 223, 456–460.
Stojilkovic, S. S. (2009). Purinergic regulation of hypothalamopituitary functions. Trends Endocrinol. Metab. 20, 460–468. doi: 10.1016/j.tem.2009.05.005
Stojilkovic, S. S., and Zemkova, H. (2013). P2X receptor channels in endocrine glands, Wiley interdisciplinary reviews. Membr. Trans. Signal. 2, 173–180. doi: 10.1002/wmts.89
Stokes, L., Scurrah, K., Ellis, J. A., Cromer, B. A., Skarratt, K. K., Gu, B. J., et al. (2011). A loss-of-function polymorphism in the human P2X4 receptor is associated with increased pulse pressure. Hypertension 58, 1086–1092. doi: 10.1161/HYPERTENSIONAHA.111.176180
Stokes, L., and Surprenant, A. (2009). Dynamic regulation of the P2X4 receptor in alveolar macrophages by phagocytosis and classical activation. Eur. J. Immunol. 39, 986–995. doi: 10.1002/eji.200838818
Surprenant, A., and North, R. A. (2009). Signaling at purinergic P2X receptors. Annu. Rev. Physiol. 71, 333–359. doi: 10.1146/annurev.physiol.70.113006.100630
Tan, Y., Zhao, B., Zeng, Q.-C., Shi, C.-M., Zhao, F.-B., and Li, Z.-W. (2009). Characteristics of ATP-activated current in nodose ganglion neurons of rats. Neurosci. Lett. 459, 25–29. doi: 10.1016/j.neulet.2009.04.054
Tian, M., Abdelrahman, A., Weinhausen, S., Hinz, S., Weyer, S., Dosa, S., et al. (2014). Carbamazepine derivatives with P2X4 receptor-blocking activity. Bioorg. Med. Chem. 22, 1077–1088. doi: 10.1016/j.bmc.2013.12.035
Toulme, E., Garcia, A., Samways, D., Egan, T. M., Carson, M. J., and Khakh, B. S. (2010). P2X4 receptors in activated C8-B4 cells of cerebellar microglial origin. J. Gen. Physiol. 135, 333–353. doi: 10.1085/jgp.200910336
Toulme, E., and Khakh, B. S. (2012). Imaging P2X4 receptor lateral mobility in microglia: regulation by calcium and p38 MAPK. J. Biol. Chem. 287, 14734–14748. doi: 10.1074/jbc.M111.329334
Toulme, E., Soto, F., Garret, M., and Boue-Grabot, E. (2006). Functional properties of internalization-deficient P2X4 receptors reveal a novel mechanism of ligand-gated channel facilitation by ivermectin. Mol. Pharmacol. 69, 576–587. doi: 10.1124/mol.105.018812
Toyomitsu, E., Tsuda, M., Yamashita, T., Tozaki-Saitoh, H., Tanaka, Y., and Inoue, K. (2012). CCL2 promotes P2X4 receptor trafficking to the cell surface of microglia. Purinergic Signal. 8, 301–310. doi: 10.1007/s11302-011-9288-x
Trang, T., Beggs, S., Wan, X., and Salter, M. W. (2009). P2X4-receptor-mediated synthesis and release of brain-derived neurotrophic factor in microglia is dependent on calcium and p38-mitogen-activated protein kinase activation. J. Neurosci. 29, 3518–3528. doi: 10.1523/JNEUROSCI.5714-08.2009
Tsuda, M. (2016). P2 receptors, microglial cytokines and chemokines, and neuropathic pain. J. Neurosci. Res. 95, 1319–1329. doi: 10.1002/jnr.23816
Tsuda, M., Kuboyama, K., Inoue, T., Nagata, K., Tozaki-Saitoh, H., and Inoue, K. (2009a). Behavioral phenotypes of mice lacking purinergic P2X4 receptors in acute and chronic pain assays. Mol. Pain 5, 28. doi: 10.1186/1744-8069-5-28
Tsuda, M., Masuda, T., Kitano, J., Shimoyama, H., Tozaki-Saitoh, H., and Inoue, K. (2009b). IFN-γ receptor signaling mediates spinal microglia activation driving neuropathic pain. Proc. Natl. Acad. Sci. U.S.A. 106, 8032–8037. doi: 10.1073/pnas.0810420106
Tsuda, M., Masuda, T., Tozaki-Saitoh, H., and Inoue, K. (2013). P2X4 receptors and neuropathic pain. Front. Cell. Neurosci. 7:191. doi: 10.3389/fncel.2013.00191
Tsuda, M., Shigemoto-Mogami, Y., Koizumi, S., Mizokoshi, A., Kohsaka, S., Salter, M. W., et al. (2003). P2X4 receptors induced in spinal microglia gate tactile allodynia after nerve injury. Nature 424, 778–783. doi: 10.1038/nature01786
Tsuda, M., Toyomitsu, E., Komatsu, T., Masuda, T., Kunifusa, E., Nasu-Tada, K., et al. (2008). Fibronectin/integrin system is involved in P2X4 receptor upregulation in the spinal cord and neuropathic pain after nerve injury. Glia 56, 579–585. doi: 10.1002/glia.20641
Ulmann, L., Hatcher, J. P., Hughes, J. P., Chaumont, S., Green, P. J., Conquet, F., et al. (2008). Up-regulation of P2X4 receptors in spinal microglia after peripheral nerve injury mediates BDNF release and neuropathic pain. J. Neurosci. 28, 11263–11268. doi: 10.1523/JNEUROSCI.2308-08.2008
Ulmann, L., Hirbec, H., and Rassendren, F. (2010). P2X4 receptors mediate PGE2 release by tissue-resident macrophages and initiate inflammatory pain. EMBO J. 29, 2290–2300. doi: 10.1038/emboj.2010.126
Ulmann, L., Levavasseur, F., Avignone, E., Peyroutou, R., Hirbec, H., Audinat, E., et al. (2013). Involvement of P2X4 receptors in hippocampal microglial activation after status epilepticus. Glia 61, 1306–1319. doi: 10.1002/glia.22516
Vavra, V., Bhattacharya, A., and Zemkova, H. (2011). Facilitation of glutamate and GABA release by P2X receptor activation in supraoptic neurons from freshly isolated rat brain slices. Neuroscience 188, 1–12. doi: 10.1016/j.neuroscience.2011.04.067
Vazquez-Villoldo, N., Domercq, M., Martin, A., Llop, J., Gomez-Vallejo, V., and Matute, C. (2014). P2X4 receptors control the fate and survival of activated microglia. Glia 62, 171–184. doi: 10.1002/glia.22596
Virginio, C., Robertson, G., Surprenant, A., and North, R. A. (1998). Trinitrophenyl-substituted nucleotides are potent antagonists selective for P2X1, P2X3 & heteromeric P2X2/3 receptors. Mol. Pharmacol. 53, 969–973.
Wes, P. D., Sayed, F. A., Bard, F., and Gan, L. (2016). Targeting microglia for the treatment of Alzheimer’s Disease. Glia 64, 1710–1732. doi: 10.1002/glia.22988
Wheeler-Schilling, T. H., Marquordt, K., Kohler, K., Guenther, E., and Jabs, R. (2001). Identification of purinergic receptors in retinal ganglion cells. Mol. Brain Res. 92, 177–180. doi: 10.1016/S0169-328X(01)00160-7
Wildman, S. S., King, B. F., and Burnstock, G. (1999). Modulation of ATP-responses at recombinant rP2X(4) receptors by extracellular pH and zinc. Br. J. Pharmacol. 126, 762–768. doi: 10.1038/sj.bjp.0702325
Wixey, J. A., Reinebrant, H. E., Carty, M. L., and Buller, K. M. (2009). Delayed P2X4R expression after hypoxia–ischemia is associated with microglia in the immature rat brain. J. Neuroimmunol. 212, 35–43. doi: 10.1016/j.jneuroim.2009.04.016
Wyatt, L. R., Finn, D. A., Khoja, S., Yardley, M. M., Asatryan, L., Alkana, R. L., et al. (2014). Contribution of P2X4 receptors to ethanol intake in male C57BL/6 mice. Neurochem. Res. 39, 1127–1139. doi: 10.1007/s11064-014-1271-9
Wyatt, L. R., Godar, S. C., Khoja, S., Jakowec, M. W., Alkana, R. L., Bortolato, M., et al. (2013). Sociocommunicative and sensorimotor impairments in male P2X4-deficient mice. Neuropsychopharmacology 38, 1993–2002. doi: 10.1038/npp.2013.98
Xiong, K., Hu, X. Q., Stewart, R. R., Weight, F. F., and Li, C. (2005). The mechanism by which ethanol inhibits rat P2X4 receptors is altered by mutation of histidine 241. Br. J. Pharmacol. 145, 576–586. doi: 10.1038/sj.bjp.0706192
Xu, J., Chai, H., Ehinger, K., Egan, T. M., Srinivasan, R., Frick, M., et al. (2014). Imaging P2X4 receptor subcellular distribution, trafficking, and regulation using P2X4-pHluorin. J. Gen. Physiol. 144, 81–104. doi: 10.1085/jgp.201411169
Yamamoto, K., Korenaga, R., Kamiya, A., and Ando, J. (2000). Fluid shear stress activates Ca(2+) influx into human endothelial cells via P2X4 purinoceptors. Circ. Res. 87, 385–391. doi: 10.1161/01.RES.87.5.385
Yamamoto, K., Sokabe, T., Matsumoto, T., Yoshimura, K., Shibata, M., Ohura, N., et al. (2006). Impaired flow-dependent control of vascular tone and remodeling in P2X4-deficient mice. Nat. Med. 12, 133–137. doi: 10.1038/nm1338
Yamashita, T., Yamamoto, S., Zhang, J., Kometani, M., Tomiyama, D., Kohno, K., et al. (2016). Duloxetine inhibits microglial P2X4 receptor function and alleviates neuropathic pain after peripheral nerve injury. PLoS ONE 11:e0165189. doi: 10.1371/journal.pone.0165189
Yang, T., Shen, J. B., Yang, R., Redden, J., Dodge-Kafka, K., Grady, J., et al. (2014). Novel protective role of endogenous cardiac myocyte P2X4 receptors in heart failure. Circ. Heart Fail. 7, 510–518. doi: 10.1161/CIRCHEARTFAILURE.113.001023
Yardley, M. M., Wyatt, L., Khoja, S., Asatryan, L., Ramaker, M. J., Finn, D. A., et al. (2012). Ivermectin reduces alcohol intake and preference in mice. Neuropharmacology 63, 190–201. doi: 10.1016/j.neuropharm.2012.03.014
Zarei, M., Sabetkasaei, M., and Moini Zanjani, T. (2014). Paroxetine attenuates the development and existing pain in a rat model of neurophatic pain. Ir. Biomed. J. 18, 94–100.
Keywords: ATP, P2X4, microglia, pain, antagonist, ivermectin
Citation: Stokes L, Layhadi JA, Bibic L, Dhuna K and Fountain SJ (2017) P2X4 Receptor Function in the Nervous System and Current Breakthroughs in Pharmacology. Front. Pharmacol. 8:291. doi: 10.3389/fphar.2017.00291
Received: 03 February 2017; Accepted: 05 May 2017;
Published: 23 May 2017.
Edited by:
Richard Lowell Bell, Indiana University School of Medicine, United StatesReviewed by:
Thomas Grutter, University of Strasbourg – CNRS, FranceDaryl L. Davies, University of Southern California, United States
Copyright © 2017 Stokes, Layhadi, Bibic, Dhuna and Fountain. This is an open-access article distributed under the terms of the Creative Commons Attribution License (CC BY). The use, distribution or reproduction in other forums is permitted, provided the original author(s) or licensor are credited and that the original publication in this journal is cited, in accordance with accepted academic practice. No use, distribution or reproduction is permitted which does not comply with these terms.
*Correspondence: Leanne Stokes, bC5zdG9rZXNAdWVhLmFjLnVr Samuel J. Fountain, cy5qLmZvdW50YWluQHVlYS5hYy51aw==