- Department of Pain Medicine, Division of Anesthesiology and Critical Care, The University of Texas MD Anderson Cancer Center, Houston, TX, USA
Chemotherapy-induced neuropathic pain is difficult to treat and prevent. Tempol decreases cellular superoxide radical levels and oxidative stress. The aims of our study were to investigate the analgesic and preventive effects of tempol on paclitaxel-induced neuropathic pain in rats and to identify the associated mechanisms of action. Neuropathic pain was induced with intraperitoneally injected paclitaxel on four alternate days in male Sprague–Dawley rats. Tempol was administered systemically as a single injection and a continuous infusion before or after the injection of paclitaxel. The mechanical threshold for allodynia, protein levels, and free radical levels were measured using von Frey filaments, Western blotting, and live cell imaging, respectively. After the rats developed neuropathic pain behavior, a single intraperitoneal injection and continuous infusion of tempol ameliorated paclitaxel-induced mechanical allodynia. Systemic infusion of tempol in the early phase of the development of pain behavior prevented the development of paclitaxel-induced pain behavior. Paclitaxel increased the levels of phosphorylated protein kinase C, phosphorylated nuclear factor κB, phosphodiesterase 4D (PDE4D), IL-1β, and monocyte chemoattractant protein-1 in the lumbar dorsal root ganglia; however, tempol decreased these levels. Paclitaxel also increased superoxide levels in a culture of primary dorsal root ganglion cells and tempol decreased these levels. In conclusion, tempol alleviates and prevents chemotherapy-induced neuropathic pain in rats by reducing the levels of inflammatory cytokines and free radicals in dorsal root ganglia.
Introduction
Chemotherapeutic agents, including taxanes (e.g., paclitaxel, docetaxel), vinca alkaloids (e.g., vincristine, vinblastine), platinum agents (e.g., cisplatin, carboplatin, oxaliplatin), and others (e.g., thalidomide, bortezomib, lenalidomide), produce peripheral pain in the distal extremities in a symmetrical glove and stocking distribution (Yazdani and Abdi, 2014). Chemotherapy-induced neuropathic pain is a dose-limiting adverse effect that can take place at any time during the course of the treatment or even after its termination (Windebank and Grisold, 2008). This neuropathy can significantly decrease the overall quality of life in cancer patients (Mols et al., 2014; Rivera and Cianfrocca, 2015).
To date, analgesic drugs such as opioids, non-steroidal anti-inflammatory agents, anticonvulsants, antidepressants, and sodium channel blockers show little or no analgesic effects in paclitaxel-induced neuropathic pain (PINP) models (Xiao et al., 2008). It has also been reported that glutamine, glutathione, N-acetylcysteine, oxcarbazepine, and xaliproden did not prevent chemotherapy-induced neuropathy (Wolf et al., 2008). On the other hand, there have been several preclinical reports indicating cannabinoids might have some effects in reducing chemotherapy-induced peripheral neuropathy (Deng et al., 2012, 2015; Khasabova et al., 2012; Guindon et al., 2013; Vera et al., 2013; Harris et al., 2016), but their clinical efficacy has not been proved yet. Therefore, currently, no effective medications are available to treat or prevent neuropathic pain (Lee and Swain, 2006; Wolf et al., 2008; Hershman et al., 2014) and new alternatives need to be explored.
Several pain models have shown that systemic or spinal administration of free radical scavengers, including phenyl N-tert-butylnitrone and vitamin E, reduce pain behavior (Kim et al., 2004, 2006). In addition, tempol, a membrane-permeable superoxide dismutase mimetic, was reported to attenuate zymosan-induced visceral pain behavior and peroxynitrite-enhanced carrageenan-induced hyperalgesia (Khattab, 2006; Ji et al., 2015). Tempol is a member of nitroxide compounds and reacts with superoxide anion to form hydrogen peroxide. In addition, the sensitivity of tempol was greatest for hydroxyl radical, intermediate for hydrogen peroxide, and least for superoxide radical (Soule et al., 2007; Wilcox and Pearlman, 2008). The toxic doses of tempol are about 172.25–344.5 mg/kg when animals were intraperitoneally injected (Hahn et al., 1998). Tempol reduced oxidative damage in cerebral synaptosomes from gerbils and reduced exacerbated hypoxic brain damage (Howard et al., 1996; MacGregor et al., 2003). Tempol blocked the enhanced N-methyl-D-aspartate-induced neurotoxicity in cultured cortical brain cells (Hewett et al., 1994; Teichner et al., 2003). In addition, several studies have reported that tempol reduced tumor growth and incidence of cancer. Tempol decreased glioma formation in rats and delayed the development of tumors, and prolonged the lifespan of cancer-prone mice (Gariboldi et al., 2003; Erker et al., 2005; Zhang et al., 2008). However, the analgesic and preventive effects of tempol on chemotherapy-induced neuropathic pain have not been studied. The aims of this study were to investigate (1) the analgesic effects of tempol on PINP in rats, (2) the preventive effects of tempol on the development of PINP in rats, and (3) the mechanisms of action of tempol in the dorsal root ganglia (DRGs).
Materials and Methods
Experimental Animals
Male adult Sprague–Dawley rats (200–350 g; Harlan Sprague–Dawley Company, Houston, TX, USA) were used for the experiment. They had free access to food and water and were housed in a room with a normal light-dark cycle (light cycle: 7:00 a.m. to 7:00 p.m.). All animals were habituated for 1 week before the experiments. The experimental protocol was approved by the institutional animal care and use committees of The University of Texas MD Anderson Cancer Center.
Paclitaxel-Induced Neuropathic Pain
Paclitaxel (Sigma, St. Louis, MO, USA) was dissolved in a vehicle solution (4% dimethyl sulfoxide and 4% Tween 80 in sterile saline) and was injected intraperitoneally at a dose of 2 mg/kg on days 0, 2, 4, and 6 (Polomano et al., 2001; Kim et al., 2010). Control rats were injected with the same volume of vehicle without paclitaxel.
Measurement of Mechanical Allodynia
To measure mechanical allodynia, we used a behavior test that has been described previously (Chaplan et al., 1994). Briefly, rats were placed in a plastic chamber on top of a mesh screen, and the mechanical threshold of the left hind paw was determined by the up-down method (Dixon, 1980) using monofilaments (0.45–14.45 g). A filament was applied to the most sensitive parts of the paw’s plantar surface – the center of the paw or the base of the third or fourth toes – for 3–4 s. A sudden withdrawal of the foot during stimulation or immediately after removal of the filament was considered to be a positive response. A 50% mechanical threshold value was calculated. The investigator who conducted the behavioral tests was blinded to the control or treatment status of the rats.
Western Blot Analysis
The rats were anesthetized deeply with 4% isoflurane for induction for 5 min and then 3% for the maintenance. During anesthesia, rats were removed hair, opened chest cavity, and then perfused with cold saline. The L1-6 DRGs were removed, frozen in liquid nitrogen, and then stored in deep freezer (-80°C). They were homogenized in 150 μl of RIPA cell lysis buffer with a protease inhibitor on ice by homogenizer four times for 20 s (interval time: 2 min) and centrifuged at 17,000 × g at 4°C for 10 min. And then supernatants were loaded in 10% sodium dodecyl sulfate-polyacrylamide gels and transferred to polyvinylidene fluoride membranes. Blots were incubated with primary antibody against IL-1β (1:1000; Santa Cruz Biotechnology, Dallas, TX, USA), MCP-1 (1:500; Santa Cruz Biotechnology, Dallas, TX, USA), PDE4D (1:5000; Abcam, Cambridge, MA, USA), phosphorylated nuclear factor κB (p-NF-κB, 1:1000; Cell Signaling Technology, Danvers, MA, USA), phosphorylated protein kinase C (p-PKC, 1:1000; Cell Signaling Technology, Danvers, MA, USA), and GAPDH (1:5000; Santa Cruz Biotechnology, Dallas, TX, USA) overnight at 4°C. The blots were then incubated with anti-rabbit horseradish peroxidase-conjugated secondary antibody (1:5000; GenDepot, Katy, TX, USA) or anti-goat horseradish peroxidase-conjugated secondary antibody (1:5000; GenDepot, Katy, TX, USA). The immunoblots were analyzed with a chemiluminescence detection system and normalized to GAPDH. For equalizing protein loading, GAPDH expression was used as a control.
Behavioral Testing for Sedation
Behavioral testing for sedation was based on five-point scales of posture (0 = normal, 4 = flaccid atonia) and righting reflexes (0 = struggles, 4 = no movement; Devor and Zalkind, 2001; Kim et al., 2004). Sedation was assessed immediately after each pain behavior test.
Administration of Tempol
This study consisted of two parts: (1) Assessment of the therapeutic effects of tempol on PINP, given as a single intraperitoneal injection (50, 100, or 200 mg/kg) or as intraperitoneal infusion (10 mg/day for 7 days), and (2) investigation of the preventive effects of tempol given as intraperitoneal infusion on development of PINP (Figures 1A–C).
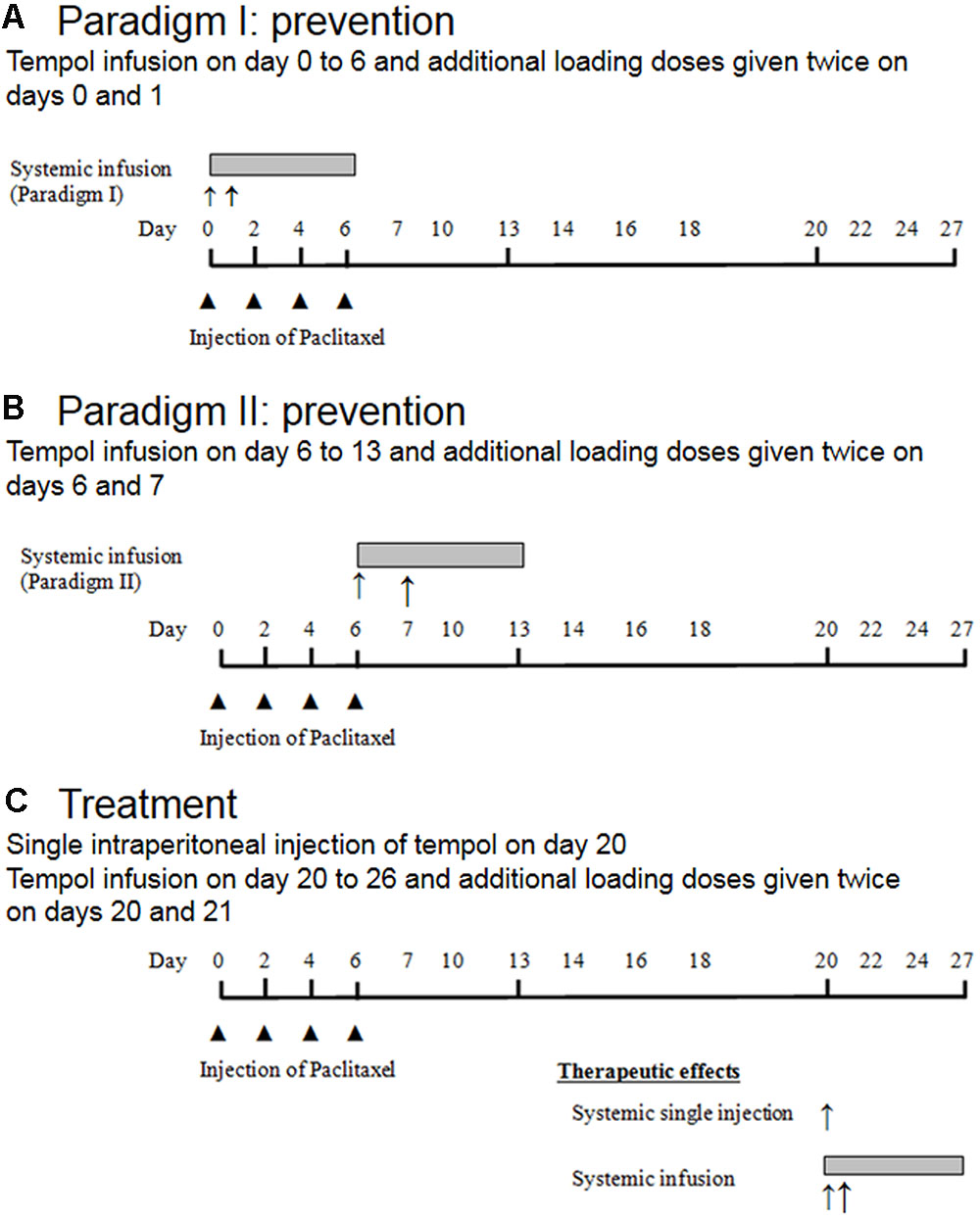
FIGURE 1. Schematic overview of the experimental design. Note, represented single intraperitoneal injection of paclitaxel, ↑ represented single intraperitoneal injection of tempol, and
represented intraperitoneal infusion of tempol.
Assessment of the Therapeutic Effects of Tempol
For administration of tempol as a single intraperitoneal injection, on day 20 after the first paclitaxel injection, 24 rats were divided into four groups. After the rats developed neuropathic pain behavior, they were randomly assigned to one of three treatment groups or a control group. Rats in the treatment groups (six rats per group) received 50, 100, or 200 mg/kg tempol in 5 mL/kg saline, and rats in the control group received a single 5-mL/kg injection of saline (Figure 1C).
For administration of tempol as a systemic intraperitoneal infusion (Figure 1C), on the 20th day after the first paclitaxel injection, the rats that developed neuropathic pain behavior were randomly assigned to either a treatment group (tempol, six rats) or a control group (vehicle, five rats) before the insertion of a mini-osmotic pump (Alzet model 2001; Alzet, Cupertino, CA, USA). The rats in the treatment group received an infusion of tempol at a rate of 1 μL/h for 7 days (10 mg/day). Additionally, loading doses of tempol (200 mg/kg) were administered on days 20 and 21 after the first paclitaxel injection. The rats in the control group received equivalent volumes of saline via the pump and as loading doses (5 mL/kg).
Assessment of the Preventive Effects of Tempol
Tempol (10 mg/day) was infused intraperitoneally (Alzet model 2001 mini-osmotic pump) by one of the following methods of administration. In paradigm I, a bolus of tempol was injected intraperitoneally at a dose of 200 mg/kg on days 0 and 1 and continuously infused intraperitoneally for 7 days (days 0 through 6; Figure 1A). In paradigm II, the same dose of tempol was given as a bolus on days 6 and 7, and continuous infusion was administered on days 6 through 13 (Figure 1B). Mechanical allodynia was measured on days 0, 2, 4, 6, 7, 9, 10, 11, 12, 13, 14, 16, 17, 18, 19, 20, 24, 26, 30, 34, 37, and 40. We measured the animal’s body weight regularly after the aforementioned behavioral tests. There was normal increase in their body weight. Furthermore, while observing animals, we did not notice any stress.
Intraperitoneal Implantation of the Mini-Osmotic Pump
The mini-osmotic pump was implanted intraperitoneally according to the manufacturer’s protocol. Briefly, a midline skin and peritoneal wall incision was made in the lower abdomen of rats that had received isoflurane anesthesia in oxygen. The pump was filled with tempol or saline and inserted into the peritoneal cavity. The musculoperitoneal layer was sutured with silk sutures, and the skin incision was closed with wound clips. Anesthesia was discontinued, and the animals were allowed to recover from anesthesia.
DRG Cell Culture
Dorsal root ganglia cells were cultured as previously described with slight modifications (Shetty et al., 2015). Briefly, under general anesthesia with isoflurane, the lumbar DRGs from L1 to L6 were removed in sterile conditions. The DRGs were then dissociated with 1.25 mg/mL collagenase (Sigma) twice for 1 h at 37°C and mechanical dissociation. Cells were plated on a 35-mm dish (Costar, Corning, NY, USA) coated with poly-L-lysine and laminin in Dulbecco’s Modified Eagle’s Medium (DMEM) with 10% fetal bovine serum (FBS), 1% penicillin/streptomycin, and 4 mM glutamine for 5 days in CO2 incubator (5% CO2, 37°C). DRG cells were plated in a chambered coverglass with cover No. 1.5 borosilicate sterile (four well, Thermo Fisher Scientific, Waltham, MA, USA) in a volume of 1 mL of DMEM with 5% FBS for live cell microscopy studies. The cells were stained with trypan blue, and viability was measured by a Luna automated cell counter (Logos Biosystems, Annandale, VA, USA).
Live Cell Imaging for Mitochondrial Superoxide Level
Dorsal root ganglia cells were plated in a chambered coverglass with cover No. 1.5 borosilicate sterile (four well, Thermo Fisher Scientific) in a volume of 1 mL of DMEM with 5% FBS. After 24 h incubation, cells were stained with 5 μM MitoSOX (Molecular Probes, Eugene, OR, USA) for 10 min, washed with DMEM twice, and then treated for 2 h with 1 μM paclitaxel in vehicle (0.025% dimethyl sulfoxide in DMEM). MitoSOX is a novel fluorogenic dye and produces red fluorescence by superoxide from only mitochondria in live cells. In addition, MitoSOX can permeate live cells where it selectively targets mitochondria. Red fluorescent intensity in living cells were measured using a Zeiss LSM510 Meta laser scanning confocal system (Carl Zeiss, Inc, Thornwood, NY, USA) in a box incubator (5% CO2, 37°C, Reinach, Switzerland). Confocal scanning setting was used at the lowest laser intensity to capture pixels in the range of 0–255. The images were quantified using Volocity imaging software (Improvision, Perkin Elmer, Waltham, MA, USA). The fluorescent threshold was set at 50–255. Data represent the sum of pixels in an image by the area that produced red fluorescence.
Statistical Analyses
Data were summarized as means with standard errors of the means for the behavioral testing, Western blotting, and live cell imaging. The data were analyzed using the GraphPad Prism 6 and two-way repeated-measures analyses of variance with one repeated factor (time), followed by the Tukey post hoc test for behavioral testing and the Mann–Whitney U test for Western blotting and cell imaging. In all cases, P < 0.05 was considered statistically significant.
Results
Tempol Did Not Produce Sedation
All rats treated with single (50, 100, 200 mg/kg) or infusion (10 mg/day) of tempol or vehicle had a score of 0 on both the posture and righting reflex scales, indicating that tempol did not produce sedation. Reviewer recommended to use positive control such as diazepam to validate the sedation experiment. At this time, we did not use positive control because we had reported several publications using this method (Devor and Zalkind, 2001; Kim et al., 2004, 2006, 2010, 2015). In addition, all rats treated with tempol without paclitaxel showed normal behavior including no sedation, no pain behavior, and normal increase in body weight.
Tempol Increased the Mechanical Threshold of PINP
Single intraperitoneal injection of tempol at doses of 50, 100, and 200 mg/kg on day 20 increased mechanical threshold in a dose-dependent manner in rats (Figure 2A). Moreover, the 200-mg dose significantly increased mechanical threshold from 0.9 to 9.7 g at 0.5 h after injection compared with the saline group, but the threshold returned to baseline at 2 h. We selected 50, 100, and 200 mg/kg based on our preliminary data.
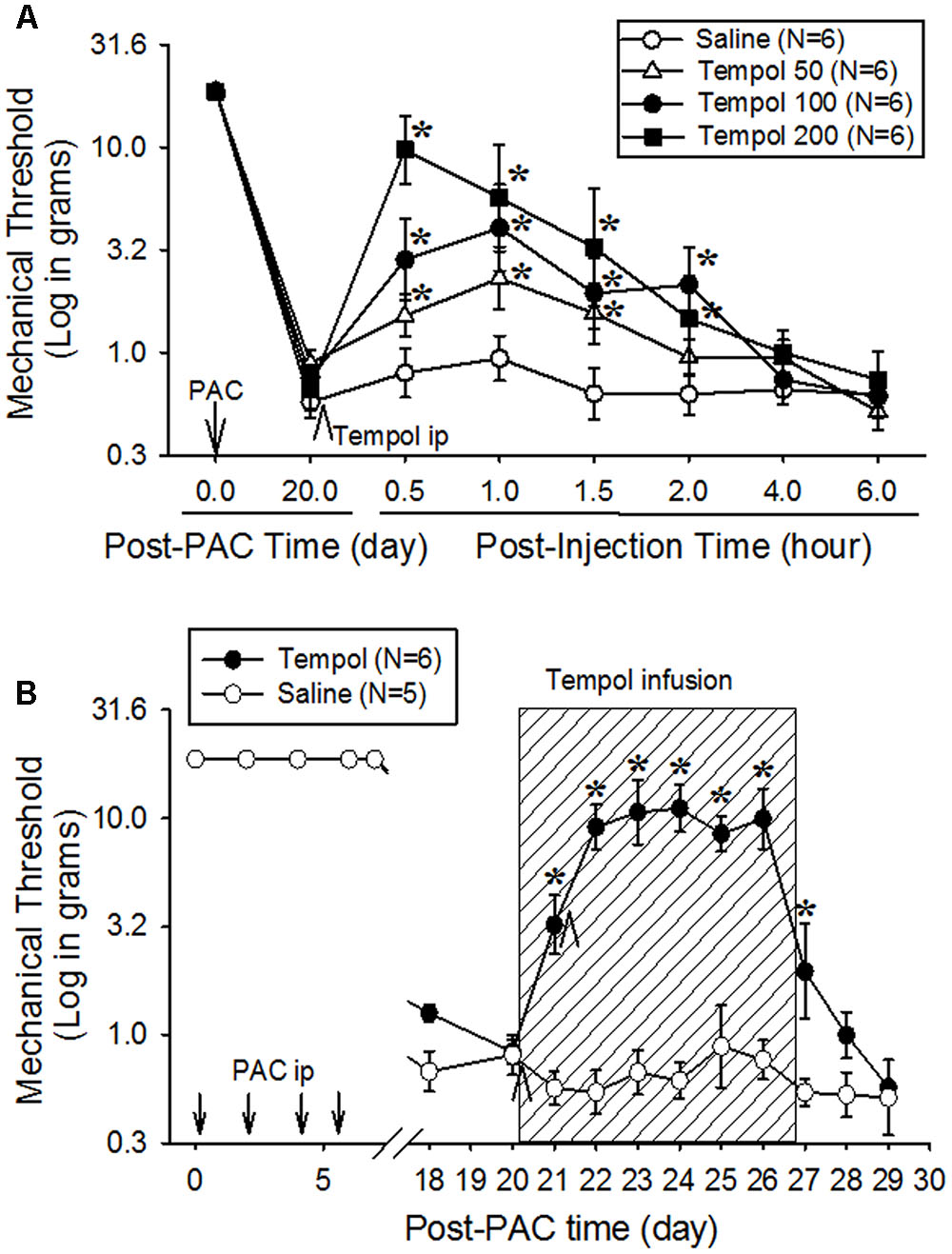
FIGURE 2. Analgesic effects of a single systemic injection (A) or systemic infusion (B) of tempol on paclitaxel-induced neuropathic pain (PINP) in rats. Paclitaxel (PAC, 2 mg/kg) was injected intraperitoneally on four alternate days (days 0, 2, 4, and 6), and the mechanical threshold was measured. (A) On day 20 after the first paclitaxel injection, 24 rats were divided into four groups, which received an intraperitoneal injection of saline or 50, 100, or 200 mg/kg of tempol (5 mL/kg). Note that the mechanical threshold returned to more than 10 g of mechanical threshold (no pain condition in rats) at 0.5 h after the injection of 200 mg/kg of tempol. (B) On day 20 after the first paclitaxel injection, 11 rats were divided into two groups. In the tempol group (N = 6), the rats received an intraperitoneal infusion of tempol (10 mg/day) for 7 days (hatched box) in combination with intraperitoneal injections of tempol (200 mg/kg) on days 20 and 21 (arrowheads). In the saline group (N = 5), the rats received saline (vehicle) instead of tempol. The systemic infusion of tempol significantly increased the mechanical threshold on day 21, and the threshold remained significantly higher than the threshold in the control group for 8 days. The data are expressed as means with standard errors of the means. The asterisks indicate values that are significantly different (P < 0.05) from the corresponding values for the saline group as determined by a two-way repeated-measures analysis of variance with one repeated factor (time) followed by the Tukey post hoc test.
To investigate the extended analgesic effects of tempol, 200 mg/kg was intraperitoneally injected on days 20 and 21, and 10 mg/day was intraperitoneally infused for 7 days (Figure 2B). The control group received intraperitoneal injections and infusion of saline. Mechanical threshold was measured each morning. Tempol significantly increased the mechanical threshold over that of the saline group on day 21, and the threshold remained significantly higher on day 27. These data indicate that infusion of tempol produced a prolonged analgesic effect without sedation.
Tempol Prevented the Development of PINP
To examine its preventive effects, tempol (10 mg/day) was infused intraperitoneally for 7 days beginning 1 h before the first paclitaxel injection (day 0) in paradigm I or beginning on day 6 in paradigm II. The early treatment (starting on day 0) did not affect the development of pain behavior (Figure 3A). In contrast, the treatment beginning on day 6 completely prevented further development of pain behavior for 40 days in paradigm II (Figure 3B). These data indicate that tempol prevented the development of PINP when given during the development phase of mechanical hyperalgesia.
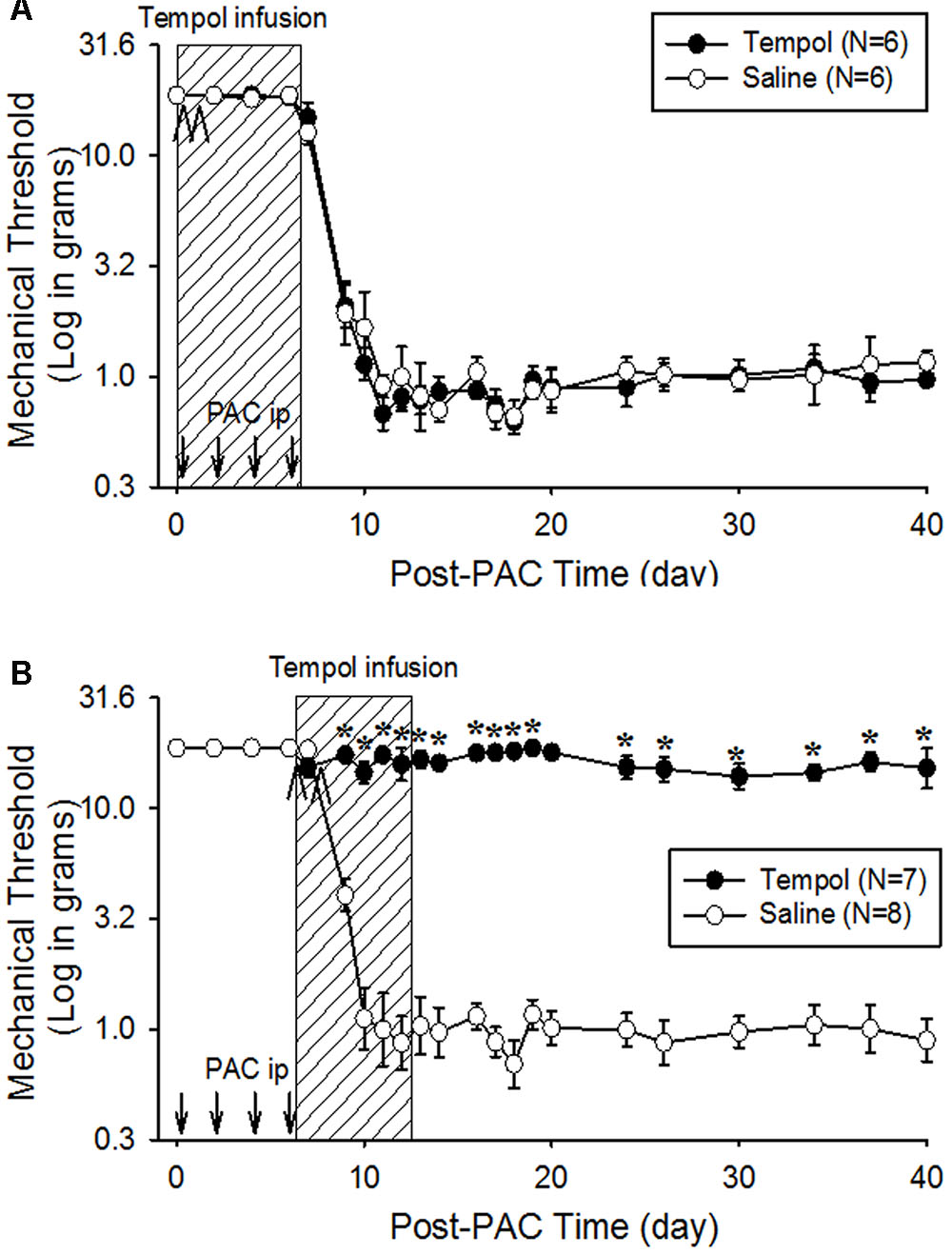
FIGURE 3. Preventive effects of tempol on PINP in rats. Paclitaxel (PAC, 2 mg/kg) was injected intraperitoneally on four alternate days (days 0, 2, 4, and 6), and the mechanical threshold was measured. (A) On the day of the first paclitaxel injection, 12 rats were divided into two groups. The rats in the tempol group (N = 6), received an intraperitoneal infusion of tempol (10 mg/day) for 7 days (hatched box) and an intraperitoneal injection of tempol (200 mg/kg) on days 0 and 1 (arrowheads) of the infusion period. The rats in the saline group (N = 6) received equivalent amounts of saline. The systemic infusion of tempol did not affect the development of PINP. (B) On the day of the last paclitaxel injection, another 15 rats were divided into two groups. The rats in the tempol group (N = 7) received an intraperitoneal infusion of tempol (10 mg/day) for 7 days (hatched box) and intraperitoneal injections of tempol (200 mg/kg) on days 6 and 7 (arrowheads). The rats in the saline group (N = 8) received equivalent volume of saline. The systemic infusion of tempol significantly prevented the development of PINP. The data represent means with standard errors of the means. The asterisks indicate values that are significantly different (P < 0.05) from the corresponding values for the saline group as determined by a two-way repeated-measures analysis of variance with one repeated factor (time) followed by the Tukey post hoc test.
Paclitaxel Increased the Levels of p-PKC, p-NF-κB, PDE4D, IL-1β, and MCP-1 in DRGs, and Tempol Subsequently Decreased the Levels
To examine the levels of signaling molecules, paclitaxel (2 mg/kg on days 0, 2, 4, and 6) or vehicle (4% dimethyl sulfoxide and 4% Tween 80 in saline) was intraperitoneally injected, and L1-6 DRGs were collected on day 20 after the first injection of paclitaxel or vehicle for Western blot analysis. Paclitaxel significantly increased the levels of p-PKC (1.9 times), p-NF-κB (2.1 times), PDE4D (1.7 times), IL-1β (1.9 times), and MCP-1 (2.2 times) in the DRGs compared to those in the vehicle control group (Figures 4A–F). Subsequently, tempol was infused for 7 days (days 14–20), and lumbar DRGs were removed on day 20 for Western blot analysis. Tempol decreased the levels of p-PKC, p-NF-κB, PDE4D, IL-1β, and MCP-1 in the DRGs of paclitaxel-treated rats to the levels in the DRGs of vehicle-injected rats (Figures 4A–F).
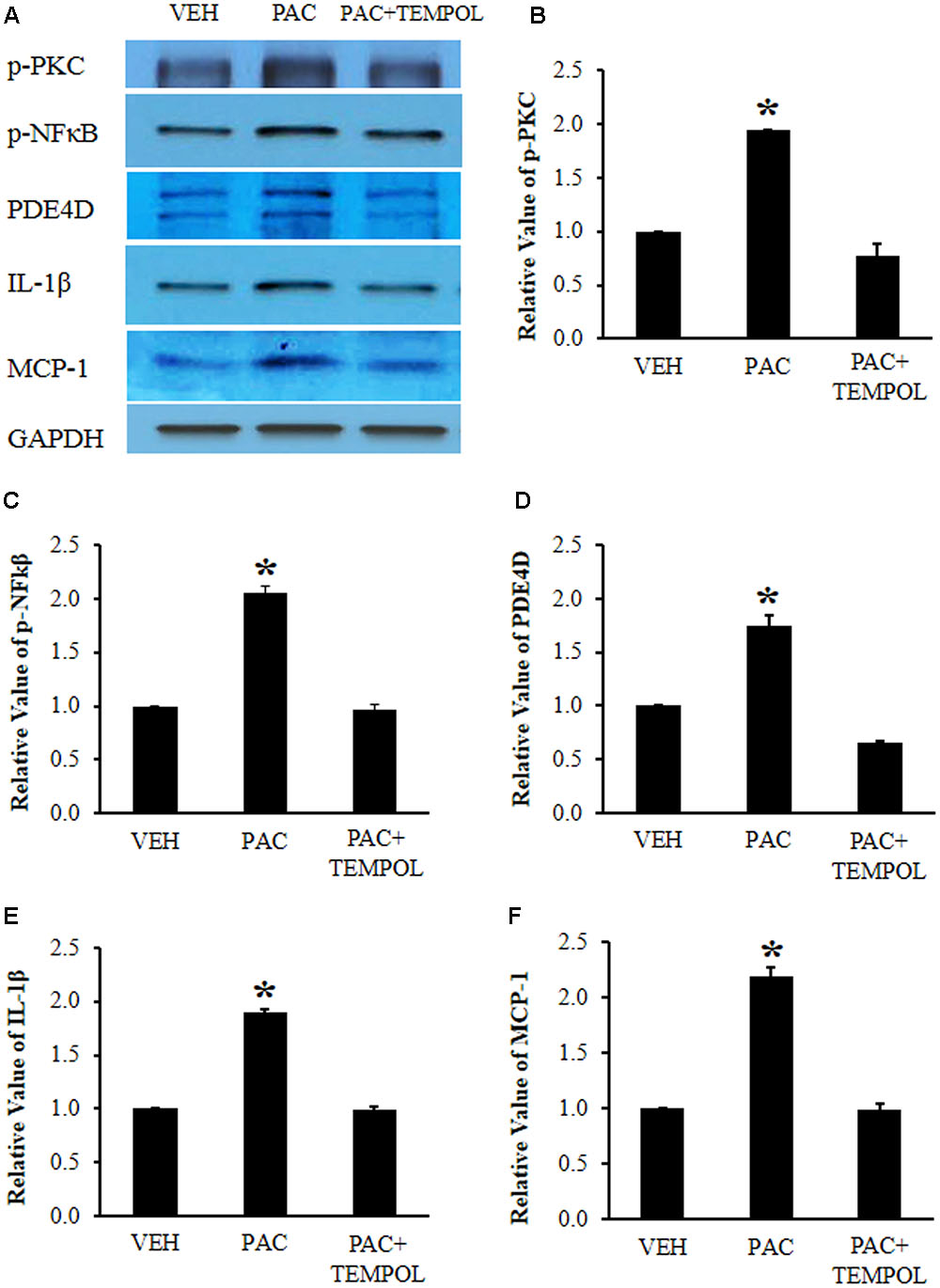
FIGURE 4. Paclitaxel increased the levels of phosphorylated protein kinase C (p-PKC), phosphorylated nuclear factor κB (p-NF-κB), PDE4D, IL-1β, and monocyte chemoattractant protein-1 (MCP-1) in rat dorsal root ganglia (DRGs). (A) Western blot showing the expression of p-PKC, p-NF-κB, PDE4D, IL-1β, and MCP-1 in DRGs after an injection of vehicle (VEH) or paclitaxel (PAC) on day 20. Tempol was infused for 7 days (days 14–20). (B–F) Quantification of p-PKC, p-NF-κB, PDE4D, IL-1β, and MCP-1 in DRGs. Note that paclitaxel increased the levels of p-PKC, p-NF-κB, PDE4D, IL-1β, and MCP-1 in rat DRGs, and tempol subsequently decreased these protein levels. The data are expressed as means ± standard deviations for three rats. The asterisks indicate values that are significantly different (P < 0.05) from the values for the vehicle group as determined by the Mann–Whitney U test.
Paclitaxel Increased Mitochondrial Superoxide Levels in DRG Cell Culture, and Tempol Subsequently Decreased the Levels
To examine the effects of paclitaxel and tempol on mitochondrial superoxide levels, the L1-6 DRGs were removed from a normal adult rat and DRG cells were cultured with the MitoSOX dye in a CO2 incubator. The MitoSOX reagent produces red fluorescence by mitochondrial superoxide but not by other free radicals. Paclitaxel increased the MitoSOX-detected red fluorescence intensity (Figures 5A–E). The 1 μM of paclitaxel significantly increased the fluorescence intensity at 2 (126%) and 4 h (132%) compared with before the treatment with paclitaxel (Figures 5A,D,E). These data indicate that paclitaxel increased the mitochondrial superoxide levels in the primary DRG cell culture. The DRG cells were alive at levels of 85–95% when treated with 1 μM of paclitaxel, but treatment with 5 μM of paclitaxel decreased cell viability to 62%. Therefore, we chose 1 μM of paclitaxel and 2 h for incubation time.
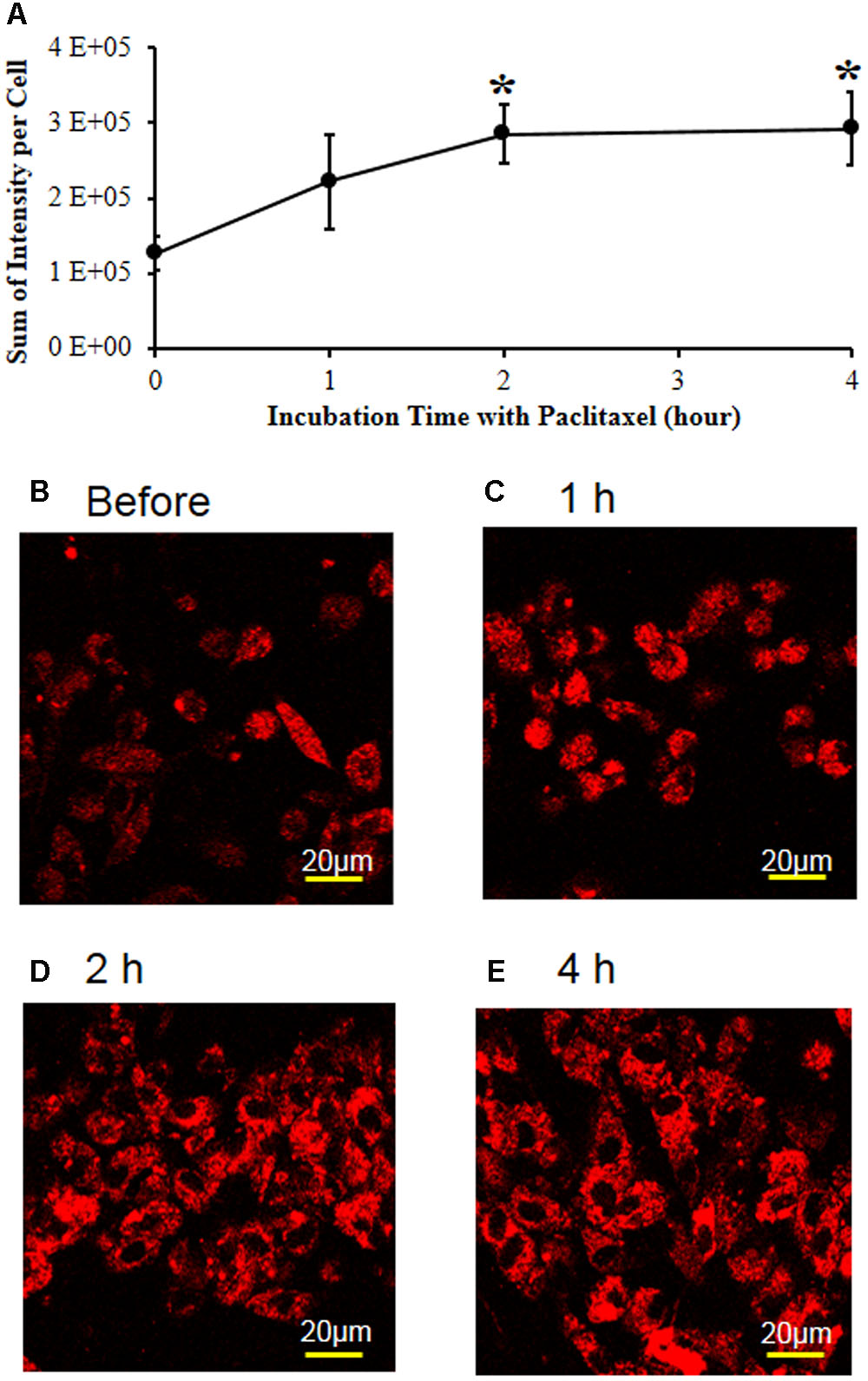
FIGURE 5. Paclitaxel increased mitochondrial superoxide levels in the primary DRG cell culture. (A) The L1-6 DRGs were isolated from an adult male Sprague–Dawley rat. The isolated DRG cells were plated in a chambered coverglass in DMEM media for live cell imaging. Note that paclitaxel (1 μM) significantly increased the MitoSOX-detected red fluorescence intensity in the primary DRG cell cultures. The data are expressed as means ± standard deviations for three independent experiments. The asterisks indicate values that are significantly different (P < 0.05) from the values before treatment with paclitaxel as determined by the unpaired t-test. (B–E) The MitoSOX-detected red fluorescence intensity was measured in live cell imaging before (B) and 1 (C), 2 (D), and 4 h (E) after treatment with paclitaxel (1 μM). Scale bars: 20 μm.
Figure 6 shows that tempol decreased the MitoSOX-detected red fluorescence intensity at concentrations of 1 and 10 mM at 2 h after paclitaxel treatment. These data show that tempol decreased the mitochondrial superoxide levels in the primary DRG cell culture.
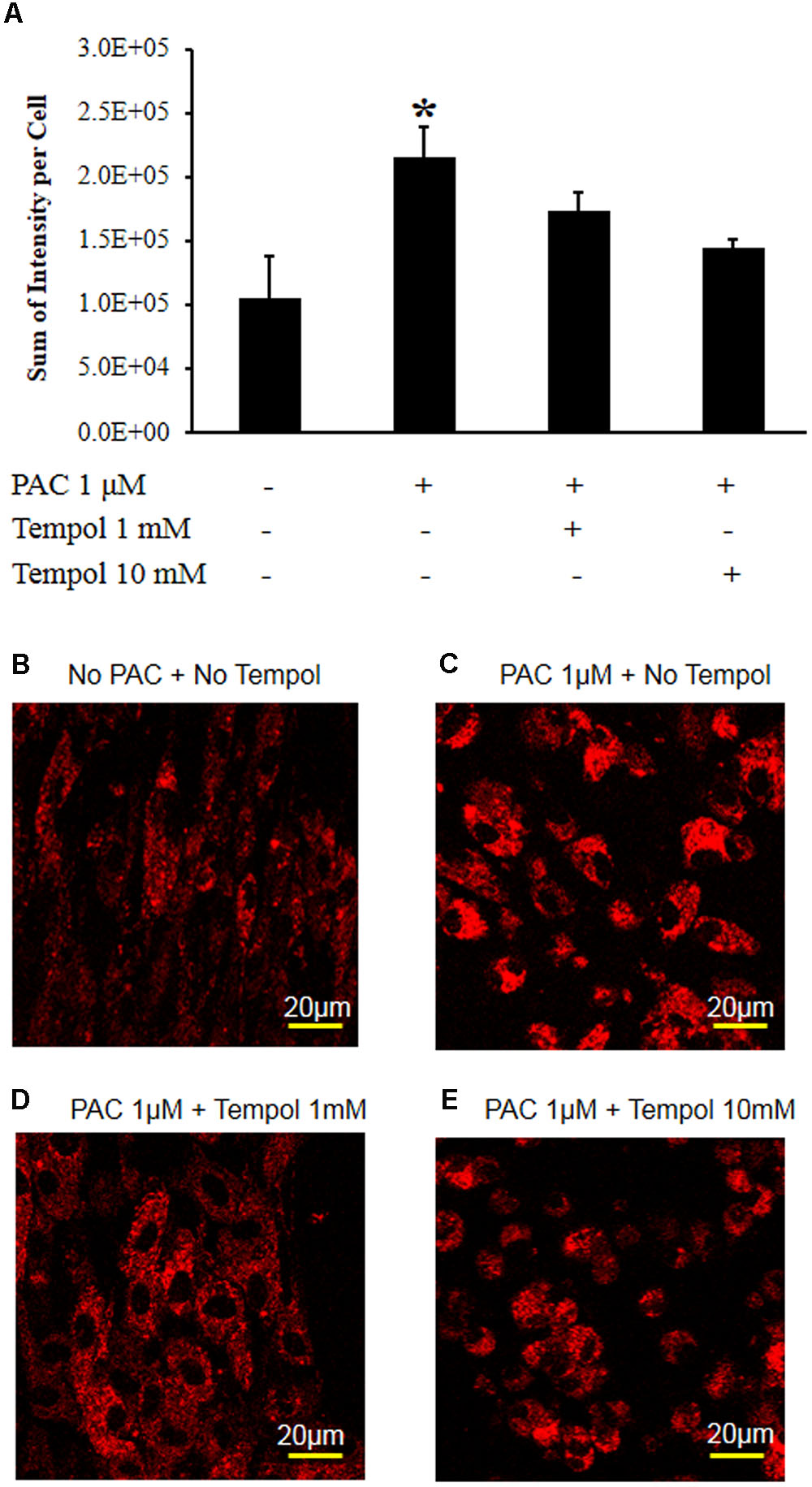
FIGURE 6. Tempol decreased paclitaxel-induced mitochondrial superoxide levels in the primary DRG cell culture. (A) The DRG cells were cultured in a chambered cover glass in DMEM media for live cell imaging. Paclitaxel (1 μM) significantly increased the MitoSOX-detected red fluorescence intensity in the primary DRG cell cultures at 2 h. Tempol (1 and 10 mM) decreased paclitaxel-induced red fluorescence intensity. The data are expressed as means ± standard deviations for three independent experiments. The asterisks indicate values that are significantly different (P < 0.05) from the values before treatment with paclitaxel as determined by the unpaired t-test. (B) The MitoSOX-detected red fluorescence without paclitaxel at 2 h. (C) The MitoSOX-detected red fluorescence at 2 h after treatment with paclitaxel (1 μM). (D) The MitoSOX-detected red fluorescence of DRG cells at 2 h after treatment with paclitaxel (1 μM) and tempol (1 mM). (E) The MitoSOX-detected red fluorescence of DRG cells at 2 h after treatment with paclitaxel (1 μM) and tempol (10 mM). Scale bars: 20 μm.
Discussion
This study investigated the therapeutic and preventive effect of tempol in rats with PINP. Tempol produced analgesia by inhibiting mitochondrial superoxide level, p-PKC, p-NF-κB, PDE4D, IL-1β, and MCP-1 in DRGs. In addition, tempol prevented the development of PINP when given during the development phase of mechanical hyperalgesia. Therefore, the results suggest that tempol has potential for the treatment and prevention of chemotherapy-induced neuropathic pain.
It is well reported that oxidative stress plays an important role in neurodegenerative diseases (Wagner et al., 1998). Free radicals (superoxide, hydroxyl radicals, hydrogen peroxide, and peroxynitrite) are derivatives of molecular oxygen and nitrogen (Lander, 1997) and are produced by both mitochondrial oxidative metabolisms and several enzymes such as phospholipase A2, cytochrome P450, monoamine oxidase, and tyrosine hydroxylase. In addition, free radicals are removed by antioxidant systems including superoxide dismutase, catalase, glutathione, and glutathione peroxidase. Pathological condition is involved in oxidative stress by the increased production of free radicals and/or decreased antioxidants level (Floyd, 1999; Floyd and Hensley, 2002).
Fidanboylu et al. (2011) reported that single intraperitoneal injection of 100 mg/kg of tempol had no analgesic effect at 1, 3, and 24 h after injection, but 250 mg/kg had analgesic effect on established paclitaxel-induced hypersensitivity at 1 h after injection. In addition, daily systemic injection of 100 mg/kg of tempol for 13 days did not affect the development of paclitaxel-induced hypersensitivity (Fidanboylu et al., 2011). We think daily systemic injection of 100 mg/kg tempol is too low a dose for scavenging free radicals because of the lack of analgesic effect. In our study, the 100 and 200 mg/kg doses of tempol significantly increased the mechanical threshold for only 2 h. So the prolonged effect of tempol was induced by intraperitoneal injection at 200 mg/kg as an initial dose on 2 days and an intraperitoneal infusion at a rate of 10 mg/day as a maintaining dose because of the short analgesic effect of single systemic injection. This infusion dose produced analgesic effect without sedation.
We used a PINP model in rats because paclitaxel is a first-line drug for breast cancer and damages the peripheral nervous system, including nerve endings, peripheral nerves, and DRG (Dougherty et al., 2004). Paclitaxel cannot penetrate the blood-brain barrier and thus does not accumulate located in the central nervous system (Cavaletti et al., 2000). However, this drug does accumulate in DRGs (Cavaletti et al., 2000). The drug may cause damage to the sciatic nerve, nerve endings, and DRGs during the development of pain behavior (Peters et al., 2007b). Paclitaxel induces several events in the DRGs including (1) accumulation of immune cells such as macrophage and polymorphonuclear cells; (2) an increase in calcium channel subunits in the DRG (Matsumoto et al., 2006; Peters et al., 2007a; Xiao et al., 2007); (3) an increase in the expression of phospholipase A2, chemokine ligand 21b, complement components 1 and 3, and matrix metalloproteinase 3 (Nishida et al., 2008); and (4) an increase of inflammatory cytokines such as TNF-α, IL-1, and IL-6 (Ledeboer et al., 2007). These events may be responsible for changes in pain behavior.
In our study, paclitaxel increased the mitochondrial superoxide anion level in DRG cells, and tempol subsequently decreased the level. Superoxide anion is produced by the electron transport system in the mitochondria and is decreased by superoxide dismutase (Liu et al., 1996). Mitochondrial superoxide is generated as a by-product of electron transport chain in mitochondria. MitoSOX is a cationic derivative of dihydroethidium and is reactive with superoxide to produce 2-hydroxyethidium that induces a fluorescence (Zielonka et al., 2008). In our study, paclitaxel increased the superoxide anion level in the mitochondria and then induced oxidative stress in DRGs, which may explain the pain behavior. Superoxide anion forms hydrogen peroxide in the presence of tempol, which is similar to native superoxide dismutase (Reddan et al., 1993). Further, the nitroxide structure of tempol can facilitate metabolism of a wide range of free radicals and reactive nitrogen species. Indeed, tempol can protect cells from superoxide anion, and hydrogen peroxide (Reddan et al., 1992). Therefore, tempol improved pain behavior through scavenging free radicals in the DRGs.
Furthermore, paclitaxel increased the levels of p-PKC, p-NF-κB, PDE4D, IL-1β, and MCP-1 in the DRGs (Figures 4A–F). PDE4D is a subgroup of PDE4 that degrades the phosphodiesterase bond of cAMP and terminates the action of cAMP (Houslay and Adams, 2003). The activation of PDE4 produces inflammatory cytokines (IL-1β) and chemokines (MCP-1) in the immune cells (Boswell-Smith et al., 2006; Kobayashi et al., 2007; Kubo et al., 2012). Recently, rolipram, a selective PDE4 inhibitor, was shown to decrease pain behavior in rat PINP models (Kim et al., 2015). Also, paclitaxel has lipopolysaccharide-like action and then accumulates immune cells in the DRGs (Manthey et al., 1992) and increases intracellular calcium levels, which promote the change from PKC to p-PKC (Peters et al., 2007b; Xiao et al., 2007). The increase in activated PKC in the DRGs was observed after treatment with paclitaxel in a mouse model of PINP (He and Wang, 2015). In addition, p-NF-κB, an activated form of NF-κB, is translocated into the nucleus and then produced various proteins including inflammatory cytokines and chemokines such as TNF-α, IL-1β, and MCP-1, thereby inducing pain behaviors (Deshpande et al., 1997). In addition, paclitaxel was reported to increase the level of TNF-α in the DRG in rats (Kim et al., 2016). In this study, tempol reduced pain behaviors by decreasing the levels of p-PKC, p-NF-κB, PDE4D, IL-1β, and MCP-1 in the DRGs (but not those of TNF-α: data not shown).
Most importantly, tempol completely prevented the development of pain behaviors when it was administered on days 6–13 after the first injection of paclitaxel but not when it was administered on days 0–6. We found that tempol decreased oxidative stress, p-PKC, p-NF-κB, PDE4D, IL-1β, and MCP-1 in the DRGs. Therefore, induction of inflammatory cytokines/chemokines and oxidative stress may have been involved in the development of pain behaviors during days 6–13 after the first injection of paclitaxel. We plan to further investigate the temporal significance in studying the pathological mechanisms of the development of chemotherapy-induced neuropathic pain.
Conclusion
Our study showed that systemic administration of tempol ameliorated and prevented neuropathic pain behavior in a rat model of PINP by inhibiting free radicals, p-PKC, p-NF-κB, PDE4D, IL-1β, and MCP-1 in the DRGs without inducing sedation. We conclude that oxidative stress and inflammatory processes are involved in both development and maintenance of chemotherapy-induced neuropathic pain.
Author Contributions
HK contributed to conception, the design, data acquisition, analysis, interpretation, writing, and revising the manuscript. S-HH contributed to the design, data acquisition, analysis, and interpretation. SA contributed to conception, the design, data acquisition, analysis, interpretation, writing, and revising the manuscript.
Funding
This work was supported by grants from the Peggy and Avinash Ahuja Foundation; and the Helen Buchanan and Stanley Joseph Seeger Endowment at The University of Texas MD Anderson Cancer Center.
Conflict of Interest Statement
The authors declare that the research was conducted in the absence of any commercial or financial relationships that could be construed as a potential conflict of interest.
The reviewer DP and handling Editor declared their shared affiliation, and the handling Editor states that the process nevertheless met the standards of a fair and objective review.
Acknowledgment
The authors thank Bryan Tutt (Department of Scientific Publications, The University of Texas MD Anderson Cancer Center) for editorial assistance.
References
Boswell-Smith, V., Spina, D., and Page, C. P. (2006). Phosphodiesterase inhibitors. Br. J. Pharmacol. 147(Suppl. 1), S252–S257. doi: 10.1038/sj.bjp.0706495
Cavaletti, G., Cavalletti, E., Oggioni, N., Sottani, C., Minoia, C., D’incalci, M., et al. (2000). Distribution of paclitaxel within the nervous system of the rat after repeated intravenous administration. Neurotoxicology 21, 389–393.
Chaplan, S. R., Bach, F. W., Pogrel, J. W., Chung, J. M., and Yaksh, T. L. (1994). Quantitative assessment of tactile allodynia in the rat paw. J. Neurosci. Methods 53, 55–63. doi: 10.1016/0165-0270(94)90144-9
Deng, L., Guindon, J., Cornett, B. L., Makriyannis, A., Mackie, K., and Hohmann, A. G. (2015). Chronic cannabinoid receptor 2 activation reverses paclitaxel neuropathy without tolerance or cannabinoid receptor 1-dependent withdrawal. Biol. Psychiatry 77, 475–487. doi: 10.1016/j.biopsych.2014.04.009
Deng, L., Guindon, J., Vemuri, V. K., Thakur, G. A., White, F. A., Makriyannis, A., et al. (2012). The maintenance of cisplatin- and paclitaxel-induced mechanical and cold allodynia is suppressed by cannabinoid CB(2) receptor activation and independent of CXCR4 signaling in models of chemotherapy-induced peripheral neuropathy. Mol. Pain 8:71. doi: 10.1186/1744-8069-8-71
Deshpande, R., Khalili, H., Pergolizzi, R. G., Michael, S. D., and Chang, M. D. (1997). Estradiol down-regulates LPS-induced cytokine production and NFkB activation in murine macrophages. Am. J. Reprod. Immunol. 38, 46–54. doi: 10.1111/j.1600-0897.1997.tb00275.x
Devor, M., and Zalkind, V. (2001). Reversible analgesia, atonia, and loss of consciousness on bilateral intracerebral microinjection of pentobarbital. Pain 94, 101–112. doi: 10.1016/S0304-3959(01)00345-1
Dixon, W. J. (1980). Efficient analysis of experimental observations. Annu. Rev. Pharmacol. Toxicol. 20, 441–462. doi: 10.1146/annurev.pa.20.040180.002301
Dougherty, P. M., Cata, J. P., Cordella, J. V., Burton, A., and Weng, H. R. (2004). Taxol-induced sensory disturbance is characterized by preferential impairment of myelinated fiber function in cancer patients. Pain 109, 132–142. doi: 10.1016/j.pain.2004.01.021
Erker, L., Schubert, R., Yakushiji, H., Barlow, C., Larson, D., Mitchell, J. B., et al. (2005). Cancer chemoprevention by the antioxidant tempol acts partially via the p53 tumor suppressor. Hum. Mol. Genet. 14, 1699–1708. doi: 10.1093/hmg/ddi181
Fidanboylu, M., Griffiths, L. A., and Flatters, S. J. (2011). Global inhibition of reactive oxygen species (ROS) inhibits paclitaxel-induced painful peripheral neuropathy. PLoS ONE 6:e25212. doi: 10.1371/journal.pone.0025212
Floyd, R. A. (1999). Antioxidants, oxidative stress, and degenerative neurological disorders. Proc. Soc. Exp. Biol. Med. 222, 236–245. doi: 10.1046/j.1525-1373.1999.d01-140.x
Floyd, R. A., and Hensley, K. (2002). Oxidative stress in brain aging. Implications for therapeutics of neurodegenerative diseases. Neurobiol. Aging 23, 795–807.
Gariboldi, M. B., Ravizza, R., Petterino, C., Castagnaro, M., Finocchiaro, G., and Monti, E. (2003). Study of in vitro and in vivo effects of the piperidine nitroxide Tempol–a potential new therapeutic agent for gliomas. Eur. J. Cancer 39, 829–837. doi: 10.1016/S0959-8049(02)00742-6
Guindon, J., Lai, Y., Takacs, S. M., Bradshaw, H. B., and Hohmann, A. G. (2013). Alterations in endocannabinoid tone following chemotherapy-induced peripheral neuropathy: effects of endocannabinoid deactivation inhibitors targeting fatty-acid amide hydrolase and monoacylglycerol lipase in comparison to reference analgesics following cisplatin treatment. Pharmacol. Res. 67, 94–109. doi: 10.1016/j.phrs.2012.10.013
Hahn, S. M., Deluca, A. M., Coffin, D., Krishna, C. M., and Mitchell, J. B. (1998). In vivo radioprotection and effects on blood pressure of the stable free radical nitroxides. Int. J. Radiat. Oncol. Biol. Phys. 42, 839–842. doi: 10.1016/S0360-3016(98)00317-4
Harris, H. M., Sufka, K. J., Gul, W., and Elsohly, M. A. (2016). Effects of delta-9-tetrahydrocannabinol and cannabidiol on cisplatin-induced neuropathy in mice. Planta Med. 82, 1169–1172. doi: 10.1055/s-0042-106303
He, Y., and Wang, Z. J. (2015). Nociceptor beta II, delta, and epsilon isoforms of PKC differentially mediate paclitaxel-induced spontaneous and evoked pain. J. Neurosci. 35, 4614–4625. doi: 10.1523/JNEUROSCI.1580-14.2015
Hershman, D. L., Lacchetti, C., Dworkin, R. H., Lavoie Smith, E. M., Bleeker, J., Cavaletti, G., et al. (2014). Prevention and management of chemotherapy-induced peripheral neuropathy in survivors of adult cancers: american society of clinical oncology clinical practice guideline. J. Clin. Oncol. 32, 1941–1967. doi: 10.1200/JCO.2013.54.0914
Hewett, S. J., Csernansky, C. A., and Choi, D. W. (1994). Selective potentiation of NMDA-induced neuronal injury following induction of astrocytic iNOS. Neuron 13, 487–494. doi: 10.1016/0896-6273(94)90362-X
Houslay, M. D., and Adams, D. R. (2003). PDE4 cAMP phosphodiesterases: modular enzymes that orchestrate signalling cross-talk, desensitization and compartmentalization. Biochem. J. 370, 1–18. doi: 10.1042/bj20021698
Howard, B. J., Yatin, S., Hensley, K., Allen, K. L., Kelly, J. P., Carney, J., et al. (1996). Prevention of hyperoxia-induced alterations in synaptosomal membrane-associated proteins by N-tert-butyl-alpha-phenylnitrone and 4-hydroxy-2,2,6,6-tetramethylpiperidin-1-oxyl (Tempol). J. Neurochem. 67, 2045–2050. doi: 10.1046/j.1471-4159.1996.67052045.x
Ji, G., Li, Z., and Neugebauer, V. (2015). Reactive oxygen species mediate visceral pain-related amygdala plasticity and behaviors. Pain 156, 825–836. doi: 10.1097/j.pain.0000000000000120
Khasabova, I. A., Khasabov, S., Paz, J., Harding-Rose, C., Simone, D. A., and Seybold, V. S. (2012). Cannabinoid type-1 receptor reduces pain and neurotoxicity produced by chemotherapy. J. Neurosci. 32, 7091–7101. doi: 10.1523/JNEUROSCI.0403-12.2012
Khattab, M. M. (2006). TEMPOL, a membrane-permeable radical scavenger, attenuates peroxynitrite- and superoxide anion-enhanced carrageenan-induced paw edema and hyperalgesia: a key role for superoxide anion. Eur. J. Pharmacol. 548, 167–173. doi: 10.1016/j.ejphar.2006.08.007
Kim, H. K., Hwang, S. H., Lee, S. O., Kim, S. H., and Abdi, S. (2016). Pentoxifylline ameliorates mechanical hyperalgesia in a rat model of chemotherapy-induced neuropathic pain. Pain Physician 19, E589–E600.
Kim, H. K., Kim, J. H., Gao, X., Zhou, J. L., Lee, I., Chung, K., et al. (2006). Analgesic effect of vitamin E is mediated by reducing central sensitization in neuropathic pain. Pain 122, 53–62. doi: 10.1016/j.pain.2006.01.013
Kim, H. K., Kwon, J. Y., Yoo, C., and Abdi, S. (2015). The analgesic effect of rolipram, a phosphodiesterase 4 inhibitor, on chemotherapy-induced neuropathic pain in rats. Anesth. Analg. 121, 822–828. doi: 10.1213/ANE.0000000000000853
Kim, H. K., Park, S. K., Zhou, J. L., Taglialatela, G., Chung, K., Coggeshall, R. E., et al. (2004). Reactive oxygen species (ROS) play an important role in a rat model of neuropathic pain. Pain 111, 116–124. doi: 10.1016/j.pain.2004.06.008
Kim, H. K., Zhang, Y. P., Gwak, Y. S., and Abdi, S. (2010). Phenyl N-tert-butylnitrone, a free radical scavenger, reduces mechanical allodynia in chemotherapy-induced neuropathic pain in rats. Anesthesiology 112, 432–439. doi: 10.1097/ALN.0b013e3181ca31bd
Kobayashi, K., Suda, T., Manabe, H., and Miki, I. (2007). Administration of PDE4 inhibitors suppressed the pannus-like inflammation by inhibition of cytokine production by macrophages and synovial fibroblast proliferation. Mediators Inflamm. 2007:58901. doi: 10.1155/2007/58901
Kubo, S., Kobayashi, M., Iwata, M., Miyata, K., Takahashi, K., and Shimizu, Y. (2012). Anti-neutrophilic inflammatory activity of ASP3258, a novel phosphodiesterase type 4 inhibitor. Int. Immunopharmacol. 12, 59–63. doi: 10.1016/j.intimp.2011.10.011
Lander, H. M. (1997). An essential role for free radicals and derived species in signal transduction. FASEB J. 11, 118–124.
Ledeboer, A., Jekich, B. M., Sloane, E. M., Mahoney, J. H., Langer, S. J., Milligan, E. D., et al. (2007). Intrathecal interleukin-10 gene therapy attenuates paclitaxel-induced mechanical allodynia and proinflammatory cytokine expression in dorsal root ganglia in rats. Brain Behav. Immun. 21, 686–698. doi: 10.1016/j.bbi.2006.10.012
Lee, J. J., and Swain, S. M. (2006). Peripheral neuropathy induced by microtubule-stabilizing agents. J. Clin. Oncol. 24, 1633–1642. doi: 10.1200/JCO.2005.04.0543
Liu, S., Jiao, X., Wang, X., and Zhang, L. (1996). Interaction of electron leak and proton leak in respiratory chain of mitochondria–proton leak induced by superoxide from an electron leak pathway of univalent reduction of oxygen. Sci. China C Life Sci. 39, 168–178.
MacGregor, D. G., Avshalumov, M. V., and Rice, M. E. (2003). Brain edema induced by in vitro ischemia: causal factors and neuroprotection. J. Neurochem. 85, 1402–1411. doi: 10.1046/j.1471-4159.2003.01772.x
Manthey, C. L., Brandes, M. E., Perera, P. Y., and Vogel, S. N. (1992). Taxol increases steady-state levels of lipopolysaccharide-inducible genes and protein-tyrosine phosphorylation in murine macrophages. J. Immunol. 149, 2459–2465.
Matsumoto, M., Inoue, M., Hald, A., Xie, W., and Ueda, H. (2006). Inhibition of paclitaxel-induced A-fiber hypersensitization by gabapentin. J. Pharmacol. Exp. Ther. 318, 735–740. doi: 10.1124/jpet.106.103614
Mols, F., Beijers, T., Vreugdenhil, G., and Van De Poll-Franse, L. (2014). Chemotherapy-induced peripheral neuropathy and its association with quality of life: a systematic review. Support Care Cancer 22, 2261–2269. doi: 10.1007/s00520-014-2255-7
Nishida, K., Kuchiiwa, S., Oiso, S., Futagawa, T., Masuda, S., Takeda, Y., et al. (2008). Up-regulation of matrix metalloproteinase-3 in the dorsal root ganglion of rats with paclitaxel-induced neuropathy. Cancer Sci. 99, 1618–1625. doi: 10.1111/j.1349-7006.2008.00877.x
Peters, C. M., Jimenez-Andrade, J. M., Jonas, B. M., Sevcik, M. A., Koewler, N. J., Ghilardi, J. R., et al. (2007a). Intravenous paclitaxel administration in the rat induces a peripheral sensory neuropathy characterized by macrophage infiltration and injury to sensory neurons and their supporting cells. Exp. Neurol. 203, 42–54. doi: 10.1016/j.expneurol.2006.07.022
Peters, C. M., Jimenez-Andrade, J. M., Kuskowski, M. A., Ghilardi, J. R., and Mantyh, P. W. (2007b). An evolving cellular pathology occurs in dorsal root ganglia, peripheral nerve and spinal cord following intravenous administration of paclitaxel in the rat. Brain Res. 1168, 46–59. doi: 10.1016/j.brainres.2007.06.066
Polomano, R. C., Mannes, A. J., Clark, U. S., and Bennett, G. J. (2001). A painful peripheral neuropathy in the rat produced by the chemotherapeutic drug, paclitaxel. Pain 94, 293–304. doi: 10.1016/S0304-3959(01)00363-3
Reddan, J., Sevilla, M., Giblin, F., Padgaonkar, V., Dziedzic, D., and Leverenz, V. (1992). Tempol and deferoxamine protect cultured rabbit lens epithelial cells from H2O2 insult: insight into the mechanism of H2O2-induced injury. Lens Eye Toxic. Res. 9, 385–393.
Reddan, J. R., Sevilla, M. D., Giblin, F. J., Padgaonkar, V., Dziedzic, D. C., Leverenz, V., et al. (1993). The superoxide dismutase mimic TEMPOL protects cultured rabbit lens epithelial cells from hydrogen peroxide insult. Exp. Eye Res. 56, 543–554. doi: 10.1006/exer.1993.1068
Rivera, E., and Cianfrocca, M. (2015). Overview of neuropathy associated with taxanes for the treatment of metastatic breast cancer. Cancer Chemother. Pharmacol. 75, 659–670. doi: 10.1007/s00280-014-2607-5
Shetty, R., Deshpande, K., Ghosh, A., and Sethu, S. (2015). Management of ocular neuropathic pain with vitamin B12 supplements: a case report. Cornea 34, 1324–1325. doi: 10.1097/ICO.0000000000000572
Soule, B. P., Hyodo, F., Matsumoto, K., Simone, N. L., Cook, J. A., Krishna, M. C., et al. (2007). Therapeutic and clinical applications of nitroxide compounds. Antioxid. Redox Signal. 9, 1731–1743. doi: 10.1089/ars.2007.1722
Teichner, A., Ovadia, H., Lavie, G., and Leker, R. R. (2003). Combination of dexanabinol and tempol in focal cerebral ischemia: is there a ceiling effect? Exp. Neurol. 182, 353–360. doi: 10.1016/S0014-4886(03)00083-9
Vera, G., Cabezos, P. A., Martin, M. I., and Abalo, R. (2013). Characterization of cannabinoid-induced relief of neuropathic pain in a rat model of cisplatin-induced neuropathy. Pharmacol. Biochem. Behav. 105, 205–212. doi: 10.1016/j.pbb.2013.02.008
Wagner, R., Heckman, H. M., and Myers, R. R. (1998). Wallerian degeneration and hyperalgesia after peripheral nerve injury are glutathione-dependent. Pain 77, 173–179. doi: 10.1016/S0304-3959(98)00091-8
Wilcox, C. S., and Pearlman, A. (2008). Chemistry and antihypertensive effects of tempol and other nitroxides. Pharmacol. Rev. 60, 418–469. doi: 10.1124/pr.108.000240
Windebank, A. J., and Grisold, W. (2008). Chemotherapy-induced neuropathy. J. Peripher. Nerv. Syst. 13, 27–46. doi: 10.1111/j.1529-8027.2008.00156.x
Wolf, S., Barton, D., Kottschade, L., Grothey, A., and Loprinzi, C. (2008). Chemotherapy-induced peripheral neuropathy: prevention and treatment strategies. Eur. J. Cancer 44, 1507–1515. doi: 10.1016/j.ejca.2008.04.018
Xiao, W., Boroujerdi, A., Bennett, G. J., and Luo, Z. D. (2007). Chemotherapy-evoked painful peripheral neuropathy: analgesic effects of gabapentin and effects on expression of the alpha-2-delta type-1 calcium channel subunit. Neuroscience 144, 714–720. doi: 10.1016/j.neuroscience.2006.09.044
Xiao, W., Naso, L., and Bennett, G. J. (2008). Experimental studies of potential analgesics for the treatment of chemotherapy-evoked painful peripheral neuropathies. Pain Med. 9, 505–517. doi: 10.1111/j.1526-4637.2007.00301.x
Yazdani, S., and Abdi, S. (2014). Brief review: pain management for cancer survivors: challenges and opportunities. Can. J. Anaesth. 61, 745–753. doi: 10.1007/s12630-014-0170-5
Zhang, Q. S., Eaton, L., Snyder, E. R., Houghtaling, S., Mitchell, J. B., Finegold, M., et al. (2008). Tempol protects against oxidative damage and delays epithelial tumor onset in Fanconi anemia mice. Cancer Res. 68, 1601–1608. doi: 10.1158/0008-5472.CAN-07-5186
Keywords: chemotherapy, neuropathic pain, free radical, inflammatory cytokines, paclitaxel, tempol
Citation: Kim HK, Hwang S-H and Abdi S (2017) Tempol Ameliorates and Prevents Mechanical Hyperalgesia in a Rat Model of Chemotherapy-Induced Neuropathic Pain. Front. Pharmacol. 7:532. doi: 10.3389/fphar.2016.00532
Received: 13 September 2016; Accepted: 22 December 2016;
Published: 16 January 2017.
Edited by:
Raquel Abalo, Universidad Rey Juan Carlos, SpainReviewed by:
David Balayssac, Institut National de la Santé et de la Recherche Médicale (INSERM), FranceDavid Pascual, Universidad Rey Juan Carlos, Spain
Copyright © 2017 Kim, Hwang and Abdi. This is an open-access article distributed under the terms of the Creative Commons Attribution License (CC BY). The use, distribution or reproduction in other forums is permitted, provided the original author(s) or licensor are credited and that the original publication in this journal is cited, in accordance with accepted academic practice. No use, distribution or reproduction is permitted which does not comply with these terms.
*Correspondence: Hee Kee Kim, aGtpbTlAbWRhbmRlcnNvbi5vcmc= Salahadin Abdi, c2FiZGlAbWRhbmRlcnNvbi5vcmc=