- Department of Pharmaceutics, University of Washington, Seattle, WA, USA
The choroid plexus (CP) is a highly vascularized tissue in the brain ventricles and acts as the blood-cerebrospinal fluid (CSF) barrier (BCSFB). A main function of the CP is to secrete CSF, which is accomplished by active transport of small ions and water from the blood side to the CSF side. The CP also supplies the brain with certain nutrients, hormones, and metal ions, while removing metabolites and xenobiotics from the CSF. Numerous membrane transporters are expressed in the CP in order to facilitate the solute exchange between the blood and the CSF. The solute carrier (SLC) superfamily represents a major class of transporters in the CP that constitutes the molecular mechanisms for CP function. Recently, we systematically and quantitatively examined Slc gene expression in 20 anatomically comprehensive brain areas in the adult mouse brain using high-quality in situ hybridization data generated by the Allen Brain Atlas. Here we focus our analysis on Slc gene expression at the BCSFB using previously obtained data. Of the 252 Slc genes present in the mouse brain, 202 Slc genes were found at detectable levels in the CP. Unsupervised hierarchical cluster analysis showed that the CP Slc gene expression pattern is substantially different from the other 19 analyzed brain regions. The majority of the Slc genes in the CP are expressed at low to moderate levels, whereas 28 Slc genes are present in the CP at the highest levels. These highly expressed Slc genes encode transporters involved in CSF secretion, energy production, and transport of nutrients, hormones, neurotransmitters, sulfate, and metal ions. In this review, the functional characteristics and potential importance of these Slc transporters in the CP are discussed, with particular emphasis on their localization and physiological functions at the BCSFB.
Introduction
The mammalian brain is protected from circulating metabolites, neuroactive substances, drugs, toxins, and blood-borne pathogens by two major barriers: the blood-brain barrier (BBB) and the blood-cerebrospinal fluid (CSF) barrier (BCSFB; Graff and Pollack, 2004; Redzic, 2011). The BBB, formed by brain capillary endothelial cells, is characterized by highly developed tight junctions. The tight junctions of the BBB restrict porous and paracellular pathways of solute diffusion from the blood into the central nervous system (CNS). However, in the choroid plexus (CP), which is a highly vascularized structure located in the four brain ventricles, capillaries are relatively leaky due to a more relaxed tight junction architecture. Hence the barrier function in the CP is provided by the BCSFB, which is formed by a tight monolayer of cuboidal epithelial cells known as choroid plexus epithelial cells (CPEs). The integrity of the BBB and the BCSFB is essential for protecting the brain from the insults of circulating toxins and blood-borne pathogens. Due to their neuroprotective roles, the barrier functions of the BBB and the BCSFB pose a great challenge in delivering many important diagnostic and therapeutic agents to the brain.
This review focuses on the CP, the anatomic locus of the BCSFB. A major function of the CP is to produce and secrete CSF. The tightly sealed cell junctions between the CPEs separate the peripheral blood from the CSF (Spector and Johanson, 1989; Groothuis and Levy, 1997; Segal, 2000; Choudhuri et al., 2003; Zhang et al., 2010). The basolateral membrane of the CP monolayer is in free contact with blood perfusate whereas the apical membrane (also known as the luminal membrane) faces the CSF. The CPEs synthesize the components of the CSF using materials from the circulation and secrete the CSF into the ventricles. Secretion of the CSF is accomplished by active transport of small ions (e.g., Na+, Cl−, K+, and ) and water across the choroidal epithelium. Besides CSF production, the CP may also supply the brain with certain nutrients, hormones, and metal ions while removing metabolites and toxins from the CSF (Miller, 2004; Smith et al., 2004; Redzic et al., 2005). The CP is clinically important as many anatomical and physiological changes occur in this organ following cerebral ischemia and trauma (Ferrand-Drake and Wieloch, 1999; Ferrand-Drake, 2001; Redzic et al., 2005). The CP is also suggested to be a pharmacologically and toxicologically important tissue as it may regulate the distribution of certain drugs and neurotoxic agents between the blood and the CSF (Choudhuri et al., 2003; Smith et al., 2004, 2011; Keep and Smith, 2011).
Owing to their barrier functions, the BBB and the BCSFB express a myriad of distinct membrane transporters to facilitate solute exchange between the CNS and the blood. A major group of membrane transport proteins present at the BBB and the BCSFB belongs to the solute carrier (SLC) superfamily. Currently, more than 370 SLC genes have been identified from the human genome and assigned to 51 gene families (Hediger et al., 2004, and the HUGO Gene Nomenclature Committee SLC Family Series)1. SLC transporters at the BBB and the BCSFB are vital in maintaining the homeostasis of the CNS via selective and regulated transport of nutrients, hormones, electrolytes, metal ions, and metabolic byproducts at the blood-brain interface. These transporters are also of great pharmaceutical and toxicological significance as they are important determinants of drug disposition and targeting into the brain (Smith et al., 2004; Spector and Johanson, 2006, 2010; Varatharajan and Thomas, 2009; Redzic, 2011). A detailed knowledge of the major SLC transporters expressed at the BBB and the BCSFB is important for the understanding of how various transport systems work at the two CNS barriers to maintain normal functions of the brain. Information on the BBB and the BCSFB transporters also provides insights into the range and characteristics of endogenous compounds and xenobiotics that can traverse the CNS. This knowledge will help to guide rational approaches to target drugs into the brain through endogenous transport systems. In this review, we summarize our current knowledge on the expression, localization, and functional characteristics of transporters encoded by major Slc genes found at the BCSFB based upon our recent expression profiling work using high-throughput in situ hybridization (ISH) data from the Allen Brain Atlas (ABA).
Expression Profiling of Slc Genes at the Mouse BCSFB
The Allen Brain Atlas2 is an open-access genome-wide digital atlas of gene expression in the adult mouse brain developed by the Allen Institute for Brain Science. Using standardized, automated high-throughput ISH procedures, anatomically comprehensive expression patterns of individual genes were obtained at cellular resolution in 56-day-old male C57BL/6J mouse brain (Bhattacharjee, 2006; Lein et al., 2007; Sunkin and Hohmann, 2007). The ABA project has made it possible to examine gene expression in the adult mouse brain at an unprecedented scale and level of resolution. In 2006, we initiated a collaboration with the Allen Institute for Brain Science to systematically analyze mouse Slc gene expression using high-quality ISH data generated by the ABA. While the present ABA database has been expanded to ∼20,000 genes, our analysis was primarily based on the dataset released in 2006, which consists of ISH data for over 16,000 genes.
We manually analyzed original ISH photomicrographs for the expression of 307 Slc genes, corresponding to ∼82% of presently known mouse Slc genes, in 20 anatomically comprehensive brain areas including the BCSFB and the BBB (Dahlin et al., 2009). Of the 307 Slc genes analyzed in our study, 252 Slc genes were present in the mouse brain, of which ∼80% (202 Slc genes) were found at detectable levels in the CP (Figure 1A). The majority of the Slc genes in the CP are expressed at low to moderate levels, whereas 28 Slc genes are expressed at the highest levels (Figure 1A; Table 1). Most Slc genes express in the CP are co-expressed in other brain regions and cell types. However, a very small number of Slc genes, such as the sodium-independent sulfate transporter Slc26a11, seemed to be expressed only in the choroidal epithelium (Figure 1B). We also compared the Slc gene expression patterns in the CP with those in the other 19 brain anatomical areas using unsupervised hierarchical cluster analysis. This analysis, which evaluates the distributional and potential functional relatedness of the Slc genes across the brain, generated unique gene expression clusters reflecting the expression relationships of Slc gene expression profiles in various brain regions (Figure 1C). The 20 evaluated brain regions formed several subclusters based on correlated Slc gene expression patterns (Figure 1C). Strikingly, the expression profile of Slc genes in the CP comprises a very distinct cluster outlier, suggesting that there are substantial differences in the types and functions of Slc genes in the CP as compared to the other 19 internal structures of the CNS. In these 19 brain areas, anatomically proximal regions were closely co-clustered (Figure 1C), illustrating that functionally and anatomically related structures share a higher degree of overlapping Slc gene expression profiles.
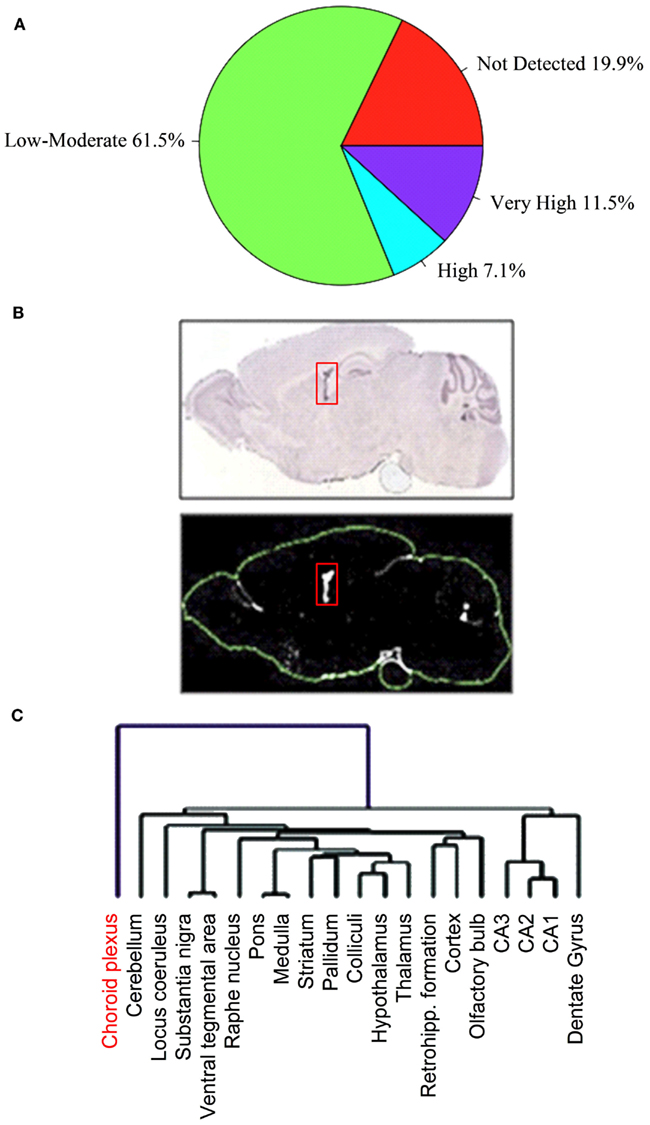
Figure 1. Distribution of expression profiles for Slc genes in the choroid plexus (CP). Of the 307 Slc genes analyzed in our ABA expression profiling study, 252 Slc genes were present in the mouse brain. The pie chart (A) shows percentage of these 252 Slc genes with expression categories based upon their average expression factor values (Ê) in the CP: “not detected” (E = 0); “low to moderate” (0 < E ≤ 9); “high” (9 < E ≤ 13); “very high” (13 < E ≤ 20). See Dahlin et al. (2009) for details regarding the definition and calculation of the E value. Panels in (B) show the restricted localization of Slc26a11 ISH signals in the CP (structure is indicated by the red boxes). The upper panel represents ISH image and the lower panel is the expression mask generated from the ISH image. In (C), a dendrogram of clustered brain regions (choroid plexus in red) is shown. Reproduced with permission from Dahlin et al. (American Society for Pharmacology and Experimental Therapeutics).
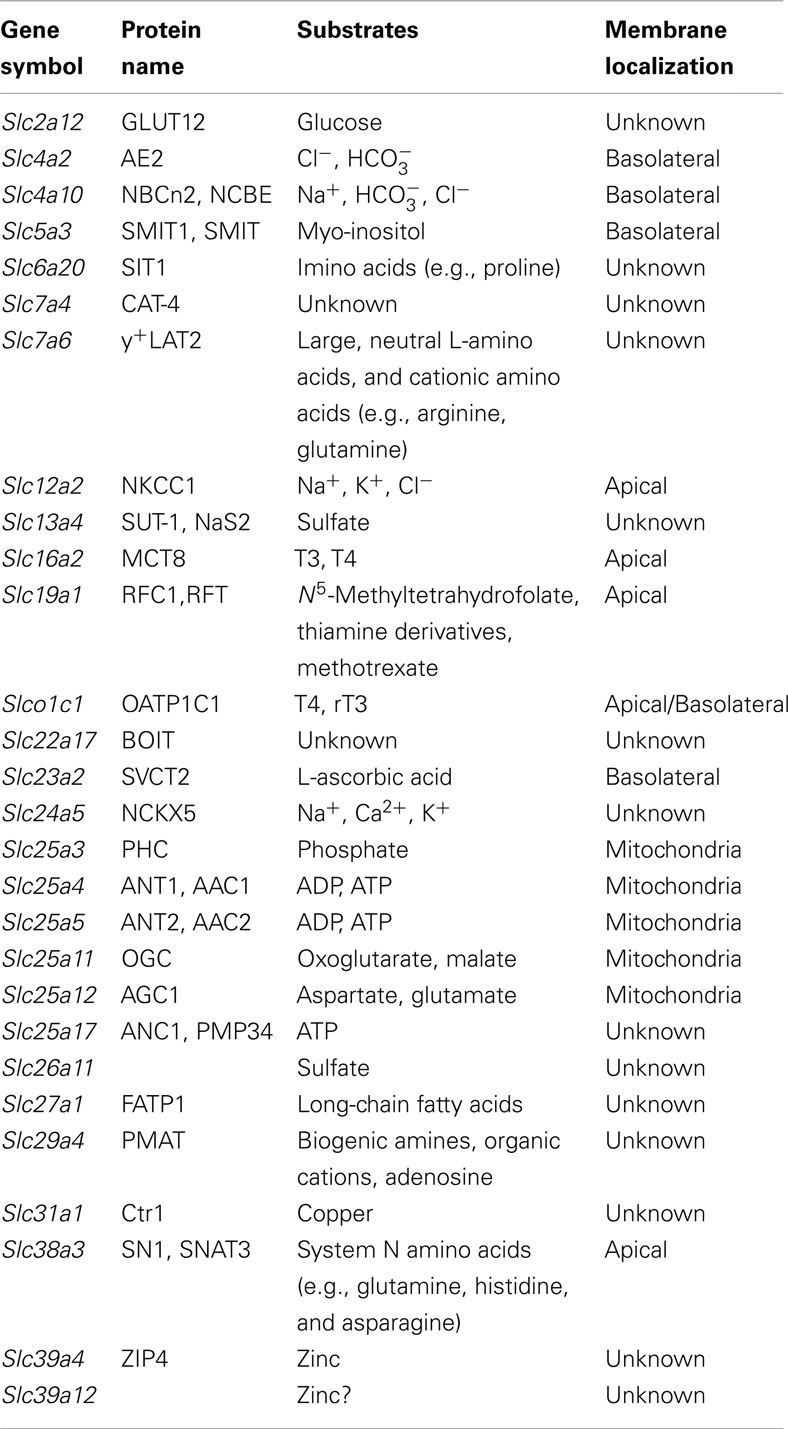
Table 1. Slc genes with highest expression in the mouse choroid plexus (CP; Dahlin et al., 2009).
Slc Genes Highly Expressed at the Mouse BCSFB
In our ABA expression profiling study, 202 Slc genes were found at detectable levels in the mouse CP (Dahlin et al., 2009). Since it is beyond the scope of this review to discuss every single Slc gene expressed in the CP, we limit our discussion to the 28 Slc genes found to be most highly expressed in the mouse CP in our ABA expression profile study (Table 1). These Slc genes encode several electrolyte transporters involved in CSF secretion (Figure 2) as well as transporters for the transport of nutrients, thyroid hormones, biogenic amines, sulfate, and metal ions (Figure 3). A number of genes from the Slc25 family of mitochondrial carriers are also highly expressed in the CP, consistent with previous observations that there are mitochondrial enrichment and high Na+/K+-ATPase activity in the CP (Keep et al., 1986; Keep and Jones, 1990; Cornford et al., 1997; Smith et al., 2004). Two Slc genes (Slc7a4 and Slc22a17) appear to be orphan transporters with unknown substrates and are thus not discussed further. Below we will discuss the functional characteristics and potential physiological and pharmacological importance of the transporters encoded by the highly expressed Slc genes in the CP, with particular emphasis on their localization and physiological functions at the BCSFB. It should be noted that considerable work has been done previously to characterize a number of Slc members in the CP, including ion transporters (Slc4 and Slc12 families) involved in CSF production (Brown et al., 2004; Praetorius, 2007; Damkier et al., 2010; Majumdar and Bevensee, 2010), oligopeptide transporter 2 (Pept2, Slc15a2; Smith et al., 2004; Kamal et al., 2008), nucleoside transporters (Slc29 family; Markovic et al., 2008), and organic anion and cation transporters (Slc21 and Slc22 families; Ghersi-Egea and Strazielle, 2002; Alebouyeh et al., 2003; Kusuhara and Sugiyama, 2004; Miller, 2004; Zhang et al., 2010; Hosoya and Tachikawa, 2011). Interested readers can refer to related review articles on these transporter families (Table 2).
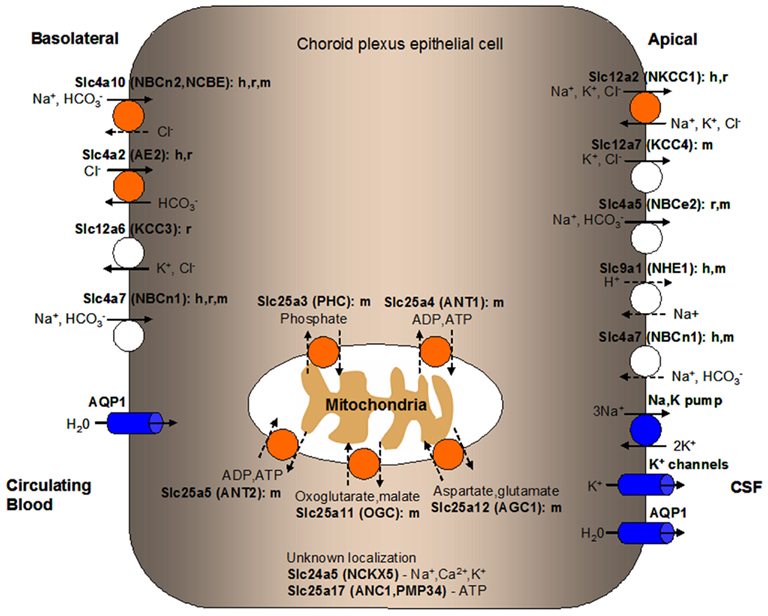
Figure 2. Localization of solute carrier (SLC) family transporters in the mammalian blood-CSF barrier/choroid plexus (CP) important for the production and secretion of CSF. Transporters colored in orange are discussed in details in this manuscript. Transporters known to play a role in ion transport at the BCSFB but are not discussed in this review are colored in white. Ion pumps and channels important in mammalian CP function are colored in blue. The suggested membrane localization of these transporters are based on in vitro, ex vivo, or in vivo studies using cells or tissues from various mammalian species as indicated (h, human; r, rat; m, mouse). Ions or substrates being transported by each transporter or channel are shown. Relatively well-established directionality of transport is shown by solid arrows while dashed arrows indicate potential directionality of transport. Transporters with unknown CP localization are listed along with their main substrates. Predicted localization of mitochondrial carriers is shown along with potential physiological substrates. CSF, cerebrospinal fluid.
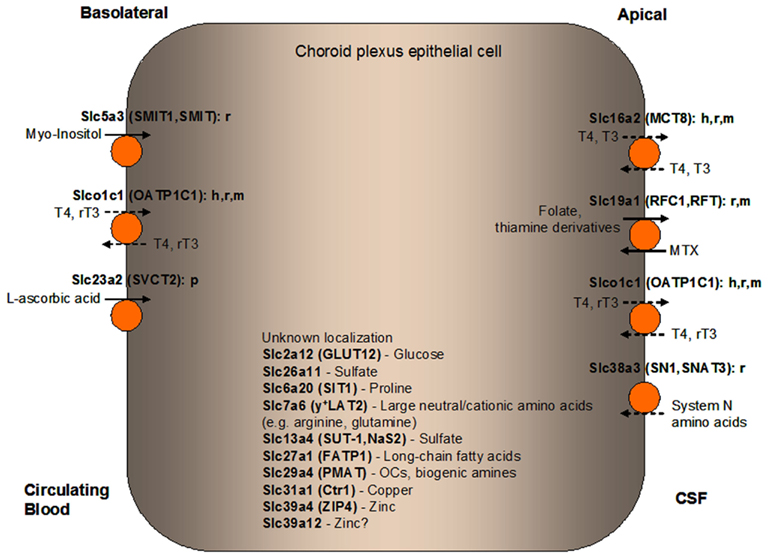
Figure 3. Localization of solute carrier (SLC) family transporters in the mammalian blood-CSF barrier/choroid plexus (CP) suggested to have a role in nourishing the CP and the brain with amino acids, carbohydrates, fatty acids, vitamins, hormones, monoamines, sulfate, and metal ions. Transporters colored in orange are discussed in details in this manuscript. The suggested membrane localization of these transporters are based on in vitro, ex vivo, or in vivo studies using cells or tissues from various mammalian species as indicated (h, human; r, rat; m, mouse; p, porcine). Major classes or representatives of substrates for each transporter are shown (T3, triiodothyronine; rT3, reverse triiodothyronine; T4, thyroxine; MTX, methotrexate; OCs, organic cations). Relatively well-established directionality of transport is shown by solid arrows while dashed arrows indicate potential directionality of transport. Transporters with unknown CP localization are listed along with their main substrates. CSF, cerebrospinal fluid.
Slc Transporters Involved in Ion Transport and CSF Secretion
A major function of the CP is to secrete CSF, which fills the four brain ventricles, the cranial subarachnoid space, the central canal, and surrounding cavities of the spinal cord. The CSF provides mechanical protection and a stable physiological environment for the CNS. The total CSF volume is ∼140–160 mL in humans and ∼40 μL in mice (Brown et al., 2004; Johanson et al., 2008). The CSF secreted by the CP passes from the lateral ventricles to the third and fourth ventricles, and then to the cranial and spinal subarachnoid spaces, and finally drains into the venous sinus blood via the arachnoid villi (Pardridge, 2011). In humans, the normal CSF turnover rate is about 4–5 times per day (Brown et al., 2004; Johanson et al., 2008). The CSF is not an ultrafiltrate of the plasma (de Rougemont et al., 1960; Davson et al., 1987) and is slightly hypertonic compared to the plasma (Damkier et al., 2010). The overall solute composition of the CSF is similar to that of the blood plasma but the CSF has a much lowered concentration of proteins compared to the plasma (Brown et al., 2004; Redzic and Segal, 2004). The driving force for fluid secretion across the CP epithelium is the active, unidirectional flux of ions from the blood side to the CSF side (e.g., Na+, Cl−, and ), which creates an osmotic gradient that is accompanied by the movement of water. On the other hand, there is a net movement of K+ from the CSF to the blood. There is also a positive electrical potential difference established across the CP epithelium (Damkier et al., 2010).
Since maintaining water volume and electrolyte composition in the CSF is crucial for the CNS to function normally, ion and water transport in the CP need to be tightly regulated. The movement of ions across the CP is mediated by transporters and ion channels differentially expressed at the basolateral (blood-facing) and apical (CSF-facing) membranes of the CPEs (Brown et al., 2004; Redzic and Segal, 2004). The net transport direction of most solutes and water is from the blood to the CSF. Various transporters, ion channels, and aquaporins expressed in the CP mediate CSF secretion and regulate electrolyte composition of the CSF (see Figure 2; Murphy and Johanson, 1990; Speake et al., 2003; Brown et al., 2004; Redzic et al., 2005; Praetorius, 2007; Damkier et al., 2010; Majumdar and Bevensee, 2010). Correspondingly, the highly expressed Slc genes in the CP identified in our ABA expression profiling study encode several ion transporters involved in CSF secretion, Slc12a2 (NKCC1), Slc4a2 (AE2), and Slc4a10 [NBCn2, Na+-driven Cl−-bicarbonate exchanger (NCBE)]. In the following sections, we will discuss the molecular and functional characteristics of these transporters based on published data in the literature. For a more comprehensive review of ion transporters and channels involved in ion transport and CSF secretion across the BCSFB, please refer to Brown et al. (2004), Praetorius (2007), Majumdar and Bevensee (2010), and Damkier et al. (2010).
Slc12a2 (NKCC1)
Our ABA expression profiling study identified high expression levels of Slc12a2 (Nkcc1) in the mouse CP (Dahlin et al., 2009), which is consistent with previous reports that demonstrated expression of this transporter in the CP in other species (Plotkin et al., 1997a,b; Kanaka et al., 2001; Johanson et al., 2004). NKCC1 was localized to the apical side of the rat and human CP (Plotkin et al., 1997a; Wu et al., 1998; Johanson et al., 2004; Praetorius and Nielsen, 2006), and was suggested to contribute to the regulation of electrolyte concentration in the CSF by mediating the electrically neutral transport of Na+, K+, and Cl- ions across cell membrane, with a stoichiometry of 1 Na+:1 K+:2 Cl- (Plotkin et al., 1997a; Wu et al., 1998; Haas and Forbush, 2000). The flux of these ions through the choroidal epithelium is modulated by ion gradients and hormonal activities (Keep et al., 1994). Versatile bidirectional transport via NKCC1 confers flexibility for regulating ion movements and concentrations in the CSF (Johanson et al., 2004).
Studies of the mammalian CP suggest that the key event in CSF secretion is the active, ouabain-sensitive transport of Na+ from the epithelial cells to the CSF, which is mediated by the apically positioned Na+/K+-ATPase (Davson and Segal, 1970; Masuzawa et al., 1984). NKCC1 likely contributes to Na+ secretion (supply the CSF with Na+) because the apical application of bumetanide (a NKCC inhibitor) inhibits CSF formation (Bairamian et al., 1991; Javaheri and Wagner, 1993; Keep et al., 1994). NKCC1 also likely enriches the CSF with K+ to feed the apical Na+/K+-ATPase (Bairamian et al., 1991; Keep et al., 1994). Alternatively, NKCC1 may take up ions from the CSF as part of regulatory cell volume increase (Wu et al., 1998). The activity of NKCC1 also modulates the level of intracellular Cl− in the CP epithelial cells, helping to maintain cellular volume against changes in extracellular osmolality and intracellular solute content to prevent excessive swelling or shrinkage (Kahle et al., 2008). There is also a growing body of evidence that diuretics which inhibit NKCC1 elicit neuroprotective effects on neurons (Ringel et al., 2000; O’Donnell et al., 2004; Busse et al., 2005). For example, NKCC1 was transiently up-regulated in the CP after traumatic brain injury and bumetanide protected animals from traumatic brain injury-induced edema and neuronal damage (Lu et al., 2006). In diabetes, there is enhanced expression of the NKCC1 cotransporter in the CP for adjusting CSF dynamics and water distribution (Egleton et al., 2003), suggesting compensatory responses at the BCSFB. NKCC1 has also been implicated in Alzheimer’s disease (AD; Johanson et al., 2004). It was suggested that ventriculomegaly and transient elevations in intracranial pressure in AD and normal pressure hydrocephalus may elicit a compensatory response in the CP to downregulate CSF formation by promoting ion reabsorption via the NKCC1 (Johanson et al., 2004). Up-regulated NKCC1 in AD may also help to counter cell shrinkage in the CP (Gosmanov et al., 2003; Johanson et al., 2004), while a rise in CSF K+ concentration resulting from neuronal damage could be buffered by the NKCC1 (Keep et al., 1994) and Na+ pump in the CP (Johanson et al., 1974). These data implicate the dynamic regulation of NKCC1 under normal and pathophysiological conditions and underscore the importance of NKCC1 in CP and CNS functions.
Slc4a10 (NBCn2, NCBE)
Slc4a10 encodes a Na+ - cotransporter highly expressed in the mouse, rat, and human CP, where it has been localized to the basolateral membrane (Praetorius et al., 2004; Bouzinova et al., 2005; Praetorius and Nielsen, 2006; Damkier et al., 2007; Jacobs et al., 2008; Dahlin et al., 2009). The rodent protein product of Slc4a10 also exports Cl−, and was therefore named NCBE (Wang et al., 2000; Damkier et al., 2010). However, as the human protein product expressed in oocytes does not export Cl− and functions as an electroneutral Na+ - cotransporter, it was suggested to be named as the second electroneutral Na+ - cotransporter (NBCn2; Parker et al., 2008). Slc4a10 is likely to be the main basolateral uptake mechanism for Na+ entry into the CP since the secretion of Na+ (proportional to the production of CSF) is not only sensitive to basolateral pH but also toward the concentration, while it is inhibited by the basolateral application of the anion exchanger inhibitor 4,4′ diisothiocyanostilbene-2,2′-disulfonic acid (DIDS; Saito and Wright, 1983; Deng and Johanson, 1989; Mayer and Sanders-Bush, 1993; Bouzinova et al., 2005; Praetorius and Nielsen, 2006; Damkier et al., 2010). Further, disruption of Slc4a10 in mice leads to an 80% reduction in brain ventricle size, suggesting that the rate of CSF secretion is greatly decreased (Jacobs et al., 2008). Slc4a10 knockout mice also showed a 70% decrease in Na+-dependent uptake capacity in isolated CP cells, indicating that this transporter is the major importer (Jacobs et al., 2008; Damkier et al., 2009). Deletion of Slc4a10 led to altered expression patterns of other CP ion transporters/channels, which presumably promoted epithelial cell survival at the expense of maintaining CSF secretion (Damkier et al., 2009; Damkier and Praetorius, 2012). For example, expression of the aquaporin-1 (AQP1) water channel and the apical Na+/K+-ATPase, which mediates Na+ exit from the CPE cells, was greatly decreased in the Slc4a10 knockout mice (Damkier and Praetorius, 2012). In addition, Slc4a10 deficient mice displayed an increased threshold to experimentally induced seizures, probably due to an abnormal regulation of brain pH in the absence of Slc4a10 (Jacobs et al., 2008). In humans, a mutation in the SLC4A10 promoter region has been implicated in severe complex partial epilepsy and mental retardation (Gurnett et al., 2008).
Slc4a2 (AE2)
Similar to Slc4a10, another member of the bicarbonate cotransporter family, Slc4a2 (Ae2), is also highly expressed in the mouse CP (Dahlin et al., 2009), and it has been localized to the basolateral membrane of the rat and human CP (Lindsey et al., 1990; Alper et al., 1994; Speake et al., 2003; Alper, 2006; Praetorius and Nielsen, 2006). The epithelial exchanger AE2 is a Na+-independent anion exchanger (Lindsey et al., 1990) which is also expressed in many different cell types. The main function of AE2 in the CP is to regulate intracellular pH through efflux which helps to acidify the cytoplasm. However, AE2 may also provide an important route for Cl- influx across the basolateral membrane of the CP. It is speculated that is first accumulated across the basolateral membrane through the action of NaHCO3 loaders, and is then exchanged for Cl− by AE2 (Damkier et al., 2010). AE2 may also serve cell volume regulatory functions or protect cells against alkalization (Praetorius and Nielsen, 2006). The Ae2 knockout mice died at or before weaning and exhibited achlorhydria and osteopetrosis (Gawenis et al., 2004; Alper, 2009).
Slc24a5 (NCKX5)
Another ion transporter that is highly expressed in the mouse CP is the Na+/(Ca2+-K+) exchanger Slc24a5 (Nckx5; Dahlin et al., 2009). NCKX5 is a potassium-dependent cation exchanger that affects pigmentation in zebrafish and humans (Lamason et al., 2005; Ginger et al., 2008). This is confirmed by a recent knockout study of Nckx5 in the mouse that showed a phenotype of ocular albinism, microscopic differences in the melanosomes, and paler than normal exposed skin surfaces (Vogel et al., 2008). At present, there is no other expression or functional study of this transporter in the CP. However, as a cation exchanger involving Na+, Ca2+, and K+, it is possible that NCKX5 in the CP may participate in the transport of these ions at the BCSFB.
Mitochondrial Carriers Involved in Energy Production
An interesting finding of our ABA expression profiling study is that a number of mitochondrial carrier family members are highly expressed in the mouse CP (Dahlin et al., 2009). These include Slc25a3 (phosphate carrier/Phc), Slc25a4 (adenine nucleotide translocase-1/Ant1/Aac1), Slc25a5 (adenine nucleotide translocase-2/Ant2/Aac2), Slc25a11 (oxoglutarate carrier/Ogc), Slc25a12 (aspartate/glutamate carrier 1/Agc1), and Slc25a17 (Adenine nucleotide carrier from peroxisomes/Anc1/Pmp34). These mitochondrial carrier family members are important for various mitochondrial functions such as phosphorylation of ADP to ATP, uptake of metabolites, linking the energy metabolism compartmentalized between the mitochondrial matrix and the cytosol, and transportation of intermediates important to the Krebs cycle for cellular energy generation (Palmieri, 2004). In contrast to other Slc25 members, Slc25a17 was suggested to be a non-mitochondrial carrier and may be required for the activation of branched-chain and very-long-chain fatty acids that are oxidized in peroxisomes. Details on the functions and physiological/pathological implications of these carriers have been reviewed by Palmieri (2004). Mitochondrial enrichment has been shown in the CP, which is probably due to increased energy demands to fuel the Na+/K+-ATPase and maintain ion gradient for CSF production (Keep et al., 1986; Keep and Jones, 1990; Cornford et al., 1997; Smith et al., 2004). To date, no studies have been done on any of these proteins with regard to their specific expression, localization and functions in the CP.
Amino and Imino Acid Transporters
Slc6a20 (SIT1)
Slc6a20 (SIT1) is a Na+-dependent imino acid (proline) transporter and its high expression in the mouse CP was shown by our ABA expression profiling study while another study revealed strong labeling of Sit1 mRNA in the rat CP (Takanaga et al., 2005; Dahlin et al., 2009). There is currently no information as to whether the SIT1 transporter is localized to the apical or basolateral membrane in the CP. SIT1 may serve a primary role in the Na+-dependent transport of L-proline in the CPEs. As proline is required for collagen synthesis (McAnulty and Laurent, 1987), SIT1-mediated proline transport may support collagen synthesis in the CP, which has a higher collagen content than elsewhere in the brain (Takanaga et al., 2005).
Slc38a3 (SN1, SNAT3)
A variety of cells can use glutamine for ATP generation (Spolarics et al., 1991). Thus, glutamine transport might be present in the CP and the capillary endothelial cells to meet the energy requirements of these tissues. In support of this hypothesis, the CP that lines the cerebral ventricles expresses high levels of Slc38a3 (Sn1; Boulland et al., 2002). SN1 is a System N amino acid transporter so named because its naturally occurring substrates (glutamine, histidine, and asparagine) have nitrogen-containing side groups, and are amino acids that have important roles in the brain (Kilberg et al., 1980; Xiang et al., 2003). Sn1 is present in the rat CP as determined by ISH and ICC studies (Chaudhry et al., 1999; Boulland et al., 2002). Our ABA expression profiling study also showed high expression of Sn1 in the mouse CP (Dahlin et al., 2009). Another member of the Slc38a family, Slc38a5 (Sn2), which shares similar amino acid substrates with Sn1, is also expressed in the CP (supplemental Figure 2 from Dahlin et al., 2009). RT-PCR analysis indicated the presence of Sn1 and Sn2 in freshly isolated rat CP (Xiang et al., 2003). The activities of both SN1 and SN2 are pH and Na+-dependent (Chaudhry et al., 1999; Nakanishi et al., 2001). Transport studies in isolated CP and primary cultured CP epithelial cells demonstrated predominant pH-dependent apical uptake of glutamine and histidine, suggesting the presence of Sn1 and Sn2 at the apical membrane in the CP (Xiang et al., 1998, 2003). It is possible that the presence of both transporters enables differential regulation of the clearance of glutamine from the CSF. There is currently no definitive conclusion to the relative roles of Sn1 and Sn2 in CP glutamine transport as there are no specific inhibitors or knockout mice for these transporters.
Slc7a6 (y+LAT2)
Slc7a6 (y+LAT2) is a member of the heteromeric amino acid transporter family and our ABA expression profiling study found high expression of y+Lat2 in the mouse CP (Dahlin et al., 2009). Currently no other expression or functional studies have been done regarding this transporter in the CP. In the rodent brain, y+Lat2 is expressed in both neurons and astrocytes (Broer et al., 2000; Heckel et al., 2003; Bae et al., 2005) and it was suggested to function as a bidirectional arginine/glutamine exchanger (Broer et al., 2000; Dye et al., 2004). y+Lat2 may play a role in neurons by taking up glutamine as a precursor for glutamate synthesis as well as regulate arginine homeostasis in astrocytes/neurons which is related to nitric oxide synthesis and oxidative/nitrosative stress under hyperammonemia conditions (Broer et al., 2000; Wagner et al., 2001; Zielinska et al., 2012). It is known that y+Lat2 needs to be associated with another membrane glycoprotein Slc3a2 (4F2hc) for insertion into the plasma membrane (Torrents et al., 1998). Given that the expression of 4F2hc is also detected in the mouse CP (supplemental Figure 3 from Dahlin et al., 2009), it will be interesting to see if 4F2hc/y+LAT2 may play a role in the mammalian BCSFB in the regulation of neutral/cationic amino acids homeostasis in the brain. More studies are needed to identify the localization and physiological substrates of 4F2hc/y+LAT2 in the mammalian CP.
Carbohydrate Transporters
Slc2a12 (GLUT12)
Glucose is the primary metabolic fuel for the mammalian brain and a continuous supply is required to maintain normal CNS function. The BBB plays a predominate role in glucose supply to the brain via facilitative glucose transporters such as Slc2a1 (GLUT-1; Devraj et al., 2011). Indeed, in our ABA expression profiling study, several facilitative glucose transporters (e.g., Slc2a1 and Slc2a10) are highly expressed at the BBB (Dahlin et al., 2009). Interestingly, we identified Slc2a12 (Glut12) as highly expressed in the mouse CP (Dahlin et al., 2009). Among other facilitative glucose transporter family members examined in the ABA expression profiling study, only Slc2a3 (Glut3) showed moderate levels of expression in the mouse CP (supplemental Figure 4 from Dahlin et al., 2009). GLUT3 is mainly considered as a neuron-specific glucose transporter in rat and human brain tissues (Maher et al., 1992; Shepherd et al., 1992; Haber et al., 1993; Nagamatsu et al., 1993; McCall et al., 1994). Currently, there is little information available regarding the specific GLUT12 expression or function in the CP. GLUT12 transported glucose when it was expressed in Xenopus laevis oocytes (Rogers et al., 2003) but the affinity of GLUT12 for this substrate is not known (Augustin, 2010). Both endogenous and over-expressed GLUT12 proteins localize to intracellular compartments (Golgi network) and the plasma membrane in various cell lines (Rogers et al., 2002; Chandler et al., 2003; Flessner and Moley, 2009). Whether GLUT12 is expressed at the apical or basolateral membrane in the CP, and its role in CP glucose transport and/or consumption, remain to be studied.
Slc5a3 (SMIT1, SMIT)
Myo-Inositol (MI) is a carbohydrate that plays a role in many important aspects of cellular regulation including membrane structure, signal transduction, and osmoregulation (Prpic et al., 1982; Garcia-Perez and Burg, 1991; Handler and Kwon, 1996; Guo et al., 1997). MI is a precursor for a family of signal transduction molecules, phosphatidylinositol, and its derivatives that regulate many cellular functions. MI and its various biochemical derivatives are widely distributed in mammalian tissues and cells (Holub, 1986), wherein levels of MI are much higher than in the plasma and interstitial fluids (Dawson and Freinkel, 1961; Berry et al., 1995). Two Na+/MI cotransporters, SMIT1 (SLC5A3), and SMIT2 (SLC5A11), are important for regulating cellular and tissue inositol levels by coupling inositol transport with the physiological transmembrane Na+ ion concentration gradient (Kwon et al., 1992; Guo et al., 1997; Coady et al., 2002). The brain inositol levels are 100-fold greater than those found in the periphery (Palmano et al., 1977; Michaelis et al., 1993). Active brain transport of inositol and its stereoisomers is thought to be mediated by inositol transporters at the BBB and the BCSFB. Strong Slc5a3 mRNA hybridization signals were seen in the rat CP (Inoue et al., 1996; Guo et al., 1997; Yamashita et al., 1998), and our ABA expression profiling study identified high expression of Slc5a3 in the mouse CP (Dahlin et al., 2009). Smit1 is located at the basal side of the rat CP and is part of the mechanism that uptakes inositol from the basal surface into the CP both in vitro and in vivo (Spector and Lorenzo, 1975; Inoue et al., 1996; Hakvoort et al., 1998; Angelow et al., 2004). As a Na+ and MI cotransporter, SMIT1 was also suggested to play a role in ionic balance in CP epithelial cells by mediating Na+ influx (Angelow et al., 2003). At present it is not known how inositol is transported across the apical side of the CP into the CSF.
Studies with Smit1 knockout mice showed neonatal lethality (Berry et al., 2003; Chau et al., 2005) which could be prevented by prenatal maternal MI supplement (Chau et al., 2005). Brain levels of MI were depleted in Smit1 knockout mice (Berry et al., 2003). In adult Smit1 deficient mice rescued by MI supplement, Smit1 is essential for the development and function of the peripheral nerves (Chau et al., 2005). Defects related to CP and CSF functions in Smit1 knockout mice have yet to be elucidated. Clinically, a significant increase in the level of MI was observed in the CSF in Down syndrome (trisomy 21) patients compared with controls (Shetty et al., 1995). It was hypothesized that disruption of MI homeostasis in Down syndrome may affect the developing brain and thus contribute to the pathogenesis of mental retardation, the most consistent and debilitating feature of the syndrome. The mapping of the human SMIT1 gene onto the long arm of chromosome 21 (Berry et al., 1995) supports the hypothesis that altered MI homeostasis may result from increased transport to the CSF (Guo et al., 1997).
Fatty Acid Transporters
Slc27a1 (FATP1)
Slc27a1 (Fatp1) is a long-chain fatty acid transporter identified as highly expressed in the mouse CP in our ABA expression profiling study (Dahlin et al., 2009). At present, no known function of this transporter has been described in the CP. In adipocytes, some FATP1 translocate to the plasma membrane in response to insulin, whereas the majority of FATP1 remain within intracellular structures and primarily reside in the mitochondria (Stahl et al., 2002; Lobo et al., 2007). In one study, FATP1 was suggested as a long-chain fatty acid transporter in human brain microvessel endothelial cells (HBMEC), and reduction in oleate and linoleic acid transport across HBMEC monolayers was detected following loss of FATP1 expression (Mitchell et al., 2011). The physiological role of FATP1 remains to be determined.
Vitamin Transporters
Slc19a1 (RFC1, RFT)
Slc19a1, encoding the reduced folate carrier 1 (Rfc1), is highly expressed in the mouse CP (Dahlin et al., 2009) and is localized to the apical membrane of the mouse and rat CP (Wang et al., 2001; Halwachs et al., 2011; Hinken et al., 2011). RFC1 mediates cellular uptake of folate (vitamin B9) and its derivatives, and prefers reduced folates over non-reduced folates (Goldman, 1971; Zhao et al., 2001b; Hinken et al., 2011). RFC1 is a major route for the transport of folates in mammalian cells, and inactivation of Rfc1 in mice resulted in early embryonic lethality (Zhao et al., 2001a,b). While RFC1 is highly expressed at the apical membrane of the CP, the folate receptor is localized to the basolateral membrane of the CP (Weitman et al., 1992; Spector and Johanson, 2006). RFC1 mediates secretion of reduced folates into the CSF, resulting in four times the plasma folate concentration (Spector, 2009; Spector and Johanson, 2010). RFC1 also plays a critical role in cerebral folate homeostasis. Clinically, slowly progressive neurologic dysfunctions have been described in adults and children with an isolated deficiency of folate in the CSF (Botez et al., 1982; Wevers et al., 1994; Ramaekers et al., 2002, 2003; Blau et al., 2003). In addition to folate and its derivatives, RFC1 also transports methotrexate (MTX), an important antifolate drug, and has been implicated in the first step of the elimination of MTX from the CSF into the blood (Spector and Johanson, 2010; Halwachs et al., 2011). This could be important as neurotoxicity is a common complication in patients who receive systemic or intrathecal MTX therapy, resulting in neurologic disorders such as seizures (Hinken et al., 2011). The phosphate derivatives of thiamine (vitamin B1), including thiamine monophosphate (TMP) and thiamine pyrophosphate (TPP), are also known substrates of RFC1 (Rindi and Laforenza, 2000; Ganapathy et al., 2004). TPP is the major thiamine intracellular metabolite and plays a critical role in oxidative phosphorylation and in the pentose phosphate pathway (Zhao et al., 2002). In the CP, RFC1 was suggested as an important regulator of TMP levels (Tallaksen et al., 1997; Wang et al., 2001; Spector and Johanson, 2007).
Slc23a2 (SVCT2)
Within the CNS, L-ascorbic acid (vitamin C) plays a pivotal role as a cofactor in a number of different enzymatic activities related to neurotransmitter processing and as a neuroprotective antioxidant (Angelow et al., 2003; Salmaso et al., 2009). L-ascorbic acid is also necessary for proper myelination of neurons (Eldridge et al., 1987) and has neuromodulatory effects on synaptic transmission (Grunewald, 1993; Rebec and Pierce, 1994). L-ascorbic acid is not synthesized in the brain and therefore needs to be transported from the blood (Spector and Johanson, 2006). The concentration of L-ascorbic acid in some neurons can reach up to 200-fold higher than that measured in the systemic circulation (Rice, 2000) and specific transport mechanisms are responsible for transporting ascorbic acid from the blood (where its concentration is 30–60 μM) into the CSF (where the concentration is maintained at 200–300 μM; Tallaksen et al., 1992; Reiber et al., 1993; Harrison et al., 2010). The CP is a major site of vitamin transport in the brain (Spector and Johanson, 2006). Evidence that L-ascorbic acid reaches the CSF through the route of the CP followed by slow penetration into the brain has been demonstrated by intravenous and intraventricular injection of [14C] L-ascorbic acid in animal model experiments (Hammarstrom, 1966; Spector, 1981). Other studies have shown that L-ascorbic acid is taken up by a mechanism that involves the sodium dependent-vitamin C transporter SVCT2 (Tsukaguchi et al., 1999; Angelow et al., 2003).
Our ABA expression profiling study showed that the mouse CP has high expression of the Na+-dependent ascorbic acid transporter Slc23a2 (Svct2; Dahlin et al., 2009). Other studies have also confirmed the expression of Svct2 in the mouse as well as the rat CP (Tsukaguchi et al., 1999; Garcia Mde et al., 2005; Salmaso et al., 2009). Another study in primary cultured epithelial cells of the porcine CP suggested that SVCT2 is present at the basolateral membrane, where it transports L-ascorbic acid from the blood to the CP (Angelow et al., 2003). Meanwhile, Svct2 KO mice fetuses were shown to have significantly lower L-ascorbic acid levels in various tissues and they died perinatally (Harrison et al., 2010). The essential role of Svct2 in mouse development makes it difficult to study the in vivo function of Svct2 in the CP using animal knockout models.
Thyroid Hormone Transporters
Slco1c1 (OATP1C1)
Slco1c1 (Oatp1c1) is highly expressed in the mouse CP (Dahlin et al., 2009) and the expression of its encoded protein has been detected by Western blot analysis in the rat CP (Sugiyama et al., 2003). Oatp1c1 is highly expressed at the basolateral (blood) side of the mouse CP as shown by immunohistochemical (IHC) study (Tohyama et al., 2004) and it may play a role in the thyroid hormone thyroxine (T4) uptake from the blood into the CSF (Tohyama et al., 2004). However, in a number of other studies, OATP1C1 protein expression was observed at both basal and apical cell surfaces in mouse, rat as well as human CPs, albeit with a potential bias toward basal localization (Tohyama et al., 2004; Roberts et al., 2008). Oatp1c1 protein expression was also observed at both basal and apical cell surfaces in fetal rat CP (Grijota-Martinez et al., 2011). Rat Oatp1c1 specifically accepts T4 and its inactive metabolite reverse triiodothyronine (rT3) as substrates, and the transport activity of organic anions by the human ortholog OATP1C1 (or OATP-F) is relatively low (Pizzagalli et al., 2002). T4 and rT3 show the highest transport activity and affinity for rat Oatp1c1 among the known substrates (Sugiyama et al., 2003; Tohyama et al., 2004; Chu et al., 2008). Rodent Oatp1c1 shares an 84% sequence homology with its human counterpart (Sugiyama et al., 2003), and human OATP1C1 was also shown to have high transport activity and affinity for T4 and rT3 (Pizzagalli et al., 2002; van der Deure et al., 2008). T4 and its metabolite rT3 affect diverse biological processes in vertebrates, and are important for the function of various organs through their influences on cellular metabolism (Zhang et al., 2010). To exert their effects in the CNS, free thyroid hormones in the circulating blood have to cross the BBB and the BCSFB (Tohyama et al., 2004). Free T4 can cross the CP from the blood to the brain (Southwell et al., 1993) and one-fifth of the T4 in the brain is thought to originate from the CSF that has passed through the CP (Chanoine et al., 1992). OATP1C1 may act as an uptake system in the CP to supply T4 to ependymal cells and circumventricular organs (Dratman et al., 1991). There is also evidence that OATP1C1 participates in the efflux of T4 and metabolites from the brain and the CP to the blood (Kassem et al., 2007; Zhang et al., 2010).
In addition to Oatp1c1, other organic anion transporter family members that are expressed in the rat CP include Oatp1a5 (Oatp3) and Oatp1a4 (Oatp2; Choudhuri et al., 2003; Ohtsuki et al., 2003). Oatp1a5 is localized to the apical membrane of the rat (Kusuhara et al., 2003; Ohtsuki et al., 2003) and mouse CP (Ohtsuki et al., 2004), while Oatp1a4 is localized to the basolateral side of the rat CP (Gao et al., 1999). Both of these transporters may contribute to thyroid hormone transport at the BCSFB.
Slc16a2 (MCT8)
Besides Oatp1c1, Slc16a2 (Mct8) from the monocarboxylate transporter family is also highly expressed in the mouse CP (Heuer et al., 2005; Dahlin et al., 2009). While OATP1C1 localizes primarily to the basolateral surface in human and rodent CP epithelial cells (as mentioned above), MCT8 expression is mainly concentrated on the apical (CSF-facing) epithelial cell surface in human, mouse, and rat CP (Heuer et al., 2005; Trajkovic et al., 2007; Roberts et al., 2008; Grijota-Martinez et al., 2011). MCT8 has been identified as an active and specific thyroid hormone transporter and it transports T4 and T3, as opposed to OATP1C1 which does not recognize T3 as a substrate (Suzuki and Abe, 2008; Grijota-Martinez et al., 2011; Lang et al., 2011). The transport of T3 by MCT8 is Na+-independent, although T4 transport is decreased in the absence of Na+ (Friesema et al., 2003). MCT8 is the only transporter for neuronal T3 uptake that has been identified to date. Studies with Mct8-null mice suggest Mct8 as a key player in T3 transport into the brain while other transporters (such as Oatp1c1) may play a more important role in T4 transport into the brain (Tohyama et al., 2004; Dumitrescu et al., 2006; Trajkovic et al., 2007).
The above studies suggest that MCT8 and OATP1C1 may function together to transport T4 from the blood into the CSF, and to efflux thyroid hormones or their inactive metabolites such as rT3 and T2 from the CSF into the blood (Roberts et al., 2008). It should be noted that MCT8 and OATP1C1 are also expressed at the BBB (Sugiyama et al., 2003; Heuer et al., 2005; Dahlin et al., 2009). Previous studies in rats have indicated that only a fraction of T4 in the brain passes through the BCSFB (Dratman et al., 1991), and therefore it was suggested that the main entry of thyroid hormones into the brain occurs via the BBB (Heuer et al., 2005). Further studies will be needed to clarify the respective roles of the BBB and the BCSFB in regulating the concentrations of thyroid hormones and their metabolites in the mammalian brain.
Plasma Membrane Monoamine Transporter
Slc29a4 (PMAT)
Plasma membrane monoamine transporter (PMAT) is a relatively new SLC transporter first cloned and characterized in our laboratory (Engel et al., 2004). PMAT mediates electrogenic, Na+, and Cl− independent, low affinity and high capacity transport of monoamine neurotransmitters (Engel et al., 2004; Itagaki et al., 2012). While PMAT transports many endogenous amines (e.g., 5-HT, dopamine, norepinephrine, histamine), it strongly prefers 5-HT and dopamine over other amines (Engel et al., 2004; Duan and Wang, 2010). PMAT also transports a variety of structurally diverse xenobiotic organic cations such as the neurotoxin 1-methyl-4-phenylpyridinium (MPP+) and therapeutic drugs (e.g., metformin; Engel et al., 2004; Engel and Wang, 2005; Zhou et al., 2007) and shares a large substrate overlap with the polyspecific organic cation transporters (OCTs) in the SLC22 family (Engel and Wang, 2005). In humans, PMAT is most strongly expressed in the brain, but transcripts were also found in other organs such as the kidney, heart, and small intestine (Engel et al., 2004; Zhou et al., 2007; Xia et al., 2009).
In our ABA expression profiling study, Pmat was found to be particularly enriched in the mouse CP (Dahlin et al., 2009). This confirms our earlier study in which mouse Pmat was observed as highly expressed within the ventricular epithelial cells of the CP by RT-PCR, non-radioactive ISH, and IHC methods (Dahlin et al., 2007). Pmat mRNA is also highly expressed in the rat CP as shown by ISH study (Vialou et al., 2007). The membrane localization of PMAT in the CP has not been determined. Functional expression in MDCK cells showed apical localization of PMAT in polarized epithelial cells (Xia et al., 2007, 2009), suggesting that the protein may localize to the apical membrane in the CP. Recently, Okura et al. (2011) examined the in vivo function of Pmat in the rat CP. Intracerebroventricular (i.c.v.) administration studies were performed to investigate the transport of [3H]MPP+ across the BCSFB by measuring the elimination rate of [3H]MPP+ from the CSF after administration. It was found that [3H]MPP+ administered i.c.v. was eliminated from the CSF, and the elimination was partially inhibited by 5-HT and dopamine, both of which are known substrates of PMAT (Okura et al., 2011). The marked Pmat mRNA expression in the CP epithelium and the transport properties of MPP+ across the BCSFB suggest that Pmat is one of the key transporters mediating MPP+ clearance from the CSF. We recently demonstrated that two β-carbolines compounds, harmalan, and norharmanium, are transportable substrates of PMAT using cell toxicity assays (Ho et al., 2011). β-carbolines are considered as natural or environmental analogs of MPP+ and have been implicated as environmental risk factors for Parkinson’s disease (Nagatsu, 1997; Storch et al., 2004). Thus, by mediating neurotoxin clearance from the CSF, PMAT in the CP may play a protective role against cationic neurotoxins.
Sulfate Transporters
Slc26a11
Our ABA expression profiling study identified a sodium-independent sulfate transporter (Slc26a11) highly expressed in the mouse CP, and also revealed that while many Slc genes expressed in the CP are co-expressed in other brain regions, Slc26a11 is selectively expressed only in the choroidal epithelia (Figure 1B; Dahlin et al., 2009). Currently, there is no other expression or functional study of Slc26a11 in the CP. Slc26a11 is reportedly expressed at high levels in the placenta, kidney, and high endothelial venules (Vincourt et al., 2003). Functional expression studies in culture cells revealed that SLC26A11 is targeted to the cell membrane and exhibits Na+-independent sulfate transport activity, and is sensitive to the anion exchanger inhibitor DIDS (Vincourt et al., 2003). It was found that in high endothelial venules endothelial cells (HEVEC), SLC26A11 modulates sulfate incorporation into these cells and could play a major role in sulfation of cell adhesion molecules (Vincourt et al., 2003). Sulfation is important in xenobiotic activation/suppression and in posttranslational modification of secreted or membrane-bound proteins and could modify protein–protein interactions involved in leukocyte adhesion, homeostasis, and receptor-mediated signaling (Nowell and Falany, 2006; Sasaki, 2011). If SLC26A11 regulates sulfate availability in the CSF, it may potentially play a role in sulfation of drugs in the brain and/or in sulfation-related posttranslational modification of proteins that are implicated in numerous physiological and pathological processes (Nowell and Falany, 2006). Additional in vitro and in vivo studies are warranted to elucidate the function of SLC26A11 in the mammalian CP.
Slc13a4 (SUT-1, NaS2)
Slc13a4 (Sut-1), another transporter that recognizes sulfate as substrate, was also highly expressed in the mouse CP (Matsumoto et al., 2003; Dahlin et al., 2009). However, there is no functional data on this transporter in the CP at present. Unlike SLC26A11, SUT-1 is Na+-dependent and is resistant to DIDS (Girard et al., 1999). SUT-1 is speculated to modulate sulfate incorporation into HEVEC and was also suggested to play a role in sulfate incorporation in the placenta (Girard et al., 1999). It has been suggested that the co-expression in HEVEC of the two functional classes of sulfate transporters, SUT-1 and SLC26A11, may allow efficient sulfate incorporation under various physiological conditions because they have very different modes of regulation (Markovich et al., 1993; Bissig et al., 1994). SUT-1 and SLC26A11 may potentially work coordinately to mediate sulfate flux at the BCSFB and/or provide different modes of sulfate uptake and utilization in CP cells. Future studies are required to study the specific roles of SUT-1 and SLC26A11 in sulfate uptake and transport in the CP.
Metal Transporters
Copper transporters
All living organisms require copper for growth and development (Harris, 1991; Bull and Cox, 1994). Equipped with a high redox potential, copper serves as a cofactor for proteins involved in a variety of neurological functions and biological reactions such as free radical eradication and connective tissue formation. Thus, copper homeostasis is critical for cell development and survival but excessive levels are not desirable as copper in excess of cellular needs mediates free radical production and the direct oxidation of lipids, proteins, and DNA (Harris, 1991). Cellular uptake, export, and utilization of copper are regulated to control copper homeostasis (Harris, 1991; Vulpe and Packman, 1995; Gybina and Prohaska, 2006). In complex organisms such as mammals, the balance between copper necessity and toxicity is achieved at both cellular level as well as tissue and organismal levels (Harris, 1991; Bull and Cox, 1994).
Slc31a1 (Ctr1). Slc31a1 (Ctr1) was suggested to be the primary avenue for copper uptake in mammalian cells (Kuo et al., 2001). Ctr1 is highly expressed in the mouse CP during development and in adult mice (Kuo et al., 2001, 2006; Dahlin et al., 2009). Copper deficiency enhanced Ctr1 expression in the mouse and rat CP (Prohaska et al., 2003; Gybina and Prohaska, 2006; Kuo et al., 2006), suggesting an attempt to sequester limiting copper from the CSF (Gybina and Prohaska, 2006). The generation of Ctr1 knockout mice supports the importance of Ctr1 in brain function (Kuo et al., 2001; Lee et al., 2001). Early embryonic lethality was observed in homozygous mutant embryos and a deficiency in copper uptake was observed in the brains of heterozygous animals (Kuo et al., 2001). Ctr1 homozygous embryos could be recovered at E8.5 but were severely developmentally retarded and morphologically abnormal (Kuo et al., 2001). Copper was reduced to approximately half in the brains of heterozygous adult mice compared with control littermates, implicating Ctr1 is essential for copper entry into the brain (Kuo et al., 2001). The activity of the copper-dependent enzymes cytochrome c oxidase (CCO) and Cu, Zn-superoxide dismutase (Sod1) were also affected in the heterozygous mice (Lee et al., 2001). This reconfirmed older dietary data suggesting the importance of copper for normal mammalian development.
Zinc transporters
Zinc is required as a catalytic cofactor for many enzymes and it is important for protein domain structure stabilization (Devirgiliis et al., 2007). It is an essential component for the catalytic and structural activities of a number of secretory, membrane-bound, and endosome/lysosome-resident enzymes and thus plays important roles in a number of secretory processes (Coleman, 1992; Vallee and Falchuk, 1993). Zinc also acts as a signaling molecule and has been implicated in synaptic transmission (Takeda, 2000; Frederickson et al., 2005; Sensi et al., 2009). In the CNS, zinc plays a role in myelination by stabilizing interactions between lipids and myelin basic protein (Earl et al., 1988; Nuzzo et al., 2002). Mild zinc deficiency impairs cognitive development in rats and it might also have similar effect in humans (Massaro et al., 1982; Keller et al., 2000; Bhatnagar and Taneja, 2001). On the other hand, zinc can mediate brain toxicity as shown in experimental brain trauma and ischemic models in rats (Suh et al., 2000a; Lee et al., 2002). The involvement of zinc in AD was also suggested as elevated levels of this metal have been measured in plaques in AD, and in vitro zinc is able to promote β-amyloid aggregation (Lee et al., 1999; Suh et al., 2000b; Bush, 2002).
Because zinc is both essential and potentially toxic to the brain, mechanisms are needed to regulate its homeostasis. This is accomplished predominantly by members of two families of zinc transporters, the Slc30 family which mediates zinc efflux, and the Slc39 family which mediates zinc influx (Belloni-Olivi et al., 2009). Among the zinc transporters from the Slc30 and Slc39 families, Slc39a4 (Zip4) was found to be expressed at a high level in the mouse CP by our ABA expression profiling study (supplemental Figure 1 from Dahlin et al., 2009). Slc39a4 has also been detected in the rat CP along with Slc39a1 (Zip1; Belloni-Olivi et al., 2009). In our ABA expression profiling study, Slc39a1 is expressed at a low level in the mouse CP (supplemental Figure 4 from Dahlin et al., 2009).
Slc39a4 (ZIP4). ZIP4 appears to transport a narrow range of metals (Dufner-Beattie et al., 2003a). Currently, there is no data on the function of ZIP4 in the CP. ZIP4 mRNA is most abundantly expressed in tissues involved in zinc homeostasis, such as the intestine, visceral endoderm, pancreatic islets, and yolk sac (Dufner-Beattie et al., 2004; Belloni-Olivi et al., 2009). Several members of the Slc39 family respond to changes in extracellular zinc at the protein level, but Zip4 is the only member whose level of mRNA and protein increases in mouse tissues such as intestines and embryonic visceral yolk sac (Dufner-Beattie et al., 2003b). Because ZIP4 levels are regulated by dietary zinc, it was suggested that the brain has the potential to adapt to changes in zinc status (Belloni-Olivi et al., 2009). Embryonic lethality was observed in homozygote Zip4 mice while severe neuro-developmental problems were seen in heterozygous Zip4 knockout mice (Dufner-Beattie et al., 2007; Andrews, 2008). Heterozygous Zip4 knockout mice were also hydrocephalic (Dufner-Beattie et al., 2007; Andrews, 2008), which may be due to abnormal accumulation of CSF in the ventricles. These studies suggest that Zip4 might be involved in zinc homeostasis in the brain and may play an important role in the mammalian CP.
Slc39a12. In addition to Zip4, our ABA expression profiling study identified high levels of expression of a putative zinc transporter, Slc39a12, in the mouse CP (Dahlin et al., 2009). Slc39a12 is assigned to the zinc transporter family Slc39 but whether zinc is a physiological substrate of Slc39a12 is not known at present. There is currently no known function of this transporter in the CP or other tissues, although an earlier study suggested it might be a susceptibility gene in schizophrenia (Bly, 2006). Clearly, more studies are needed to elucidate the substrate, localization, and functional role of Slc39a12 in the CP.
Conclusion
In this review, we have discussed the localization and physiological significance of a number of Slc transporters in the mammalian CP based on data obtained from our recent Slc gene expression profiling study in the adult mouse brain (Dahlin et al., 2009). Our prior analysis revealed distinct expression patterns of CP Slc transcripts as compared to other brain structures and identified Slc genes with the highest expression levels in the CP (Dahlin et al., 2009). A review of these highly expressed Slc genes suggests critical roles of Slc transporters in CSF secretion, energy production, and transport of nutrients, hormones, neurotransmitters, sulfate, and metal ions at the BCSFB. We hope our study will provide a useful resource for investigators to explore the roles of Slc genes in physiological and pathological processes and stimulate further research interest in this area. It should be noted that at the time of our ABA expression profiling analysis, ISH data was only available for ∼82% of the known mouse Slc genes, therefore a significant number of Slc genes was not examined in our study. In addition, given the large number of Slc genes expressed in the CP, it is beyond the scope of this review to discuss each individual gene involved. While we chose to focus on the 28 Slc genes most highly expressed in the CP based on our ABA expression profiling study, this by no means suggests that Slc genes expressed at lower levels are not as important. In fact, many Slc genes previously shown to be important for CP function (e.g., those shown in Table 2) are not among the 28 most highly expressed Slc genes in the mouse CP we have identified. An example is the electrogenic sodium bicarbonate cotransporter Slc4a5 (NBCe2), which was not included in our ABA expression profiling study but is essential for normal CP function based on studies with genetic knockout mice (Kao et al., 2011). In addition, our ABA expression profiling study was based on ISH analysis of mRNA expression levels, which may not faithfully reflect protein expression and did not account for the influences of posttranslational regulation. For instance, Slc transporters with slower turnover rates could have higher protein expression/activities than would be expected from their mRNA levels.
While a considerable amount of studies have been performed to elucidate the molecular mechanisms of ion transport and CSF production in the CP (Brown et al., 2004; Praetorius, 2007; Damkier et al., 2010; Majumdar and Bevensee, 2010), our knowledge regarding the specific function of various Slc transporters, such as those involved in energy balance, metabolism, signaling, nutrient, and xenobiotic transport at the BCSFB, is still rather limited. Our previous expression profiling study identified a large number of Slc genes expressed in the mouse CP (Dahlin et al., 2009). Detailed characterization of the encoded transporters, including the elucidation of their substrate specificities, transport mechanisms, and membrane localization (apical vs. basolateral) in the CP represent the very first steps in understanding their physiological functions at the BCSFB. In addition, ex vivo transport assays and generation of animal models such as CP-specific gene knockout mice by the Cre-recombinase/loxP system (Crouthamel et al., 2012) along with the use of specific inhibitors or antisense knockdown methods could be used to study the in vivo functions of these transporters. These studies will provide unique insights into the specific roles Slc transporters play at the BCSFB and will reveal the significance of these transporters in sustaining the normal functions of the CP and the CNS.
It should be pointed out that although the focus of this review is on SLC/Slc transporters expressed in the CP, members of both the ATP-binding cassette (ABC) and SLC transporter superfamilies may act in concert to mediate solute transport at the BCSFB. Therefore further knowledge of both of these transporter superfamilies will be required for a comprehensive understanding of the transport mechanisms at the BCSFB. In addition, although the BBB and the BCSFB are two distinct barrier systems, both of them play a role in the supply of important ingredients for brain function and homeostasis as well as protect the brain from circulating xenobiotics and blood-borne pathogens. It is interesting that data from our previous study showed a few Slc genes, including the thyroid hormone transporters Slc16a2 and Slco1c1, the sulfate transporter Slc13a4, the reduced folate transporter Slc19a1, the L-ascorbic acid transporter Slc23a2, the proline transporter Slc6a20, and the system N amino acid transporter Slc38a3, are all co-expressed at high levels in both the brain microvessels and the CP (Dahlin et al., 2009). Transporters encoded by these Slc genes may play coordinated roles at the two blood-CNS interfaces to regulate brain homeostasis of nutrients and hormones and protect the brain from xenobiotics and toxins. Additional expression and functional studies are needed to further characterize ABC and SLC proteins in the mammalian BBB and BCSFB. Knowledge from these studies will help us understand how the brain interacts with the rest of the body through the two well-known but still under-studied brain barriers.
Conflict of Interest Statement
The authors declare that the research was conducted in the absence of any commercial or financial relationships that could be construed as a potential conflict of interest.
Acknowledgments
This work was supported by the National Institutes of Health (Grants R01-GM066233, T32-GM007750).
Footnotes
References
Alebouyeh, M., Takeda, M., Onozato, M. L., Tojo, A., Noshiro, R., Hasannejad, H., Inatomi, J., Narikawa, S., Huang, X. L., Khamdang, S., Anzai, N., and Endou, H. (2003). Expression of human organic anion transporters in the choroid plexus and their interactions with neurotransmitter metabolites. J. Pharmacol. Sci. 93, 430–436.
Alper, S. L. (2009). Molecular physiology and genetics of Na+-independent SLC4 anion exchangers. J. Exp. Biol. 212, 1672–1683.
Alper, S. L., Stuart-Tilley, A., Simmons, C. F., Brown, D., and Drenckhahn, D. (1994). The fodrin-ankyrin cytoskeleton of choroid plexus preferentially colocalizes with apical Na+K(+)-ATPase rather than with basolateral anion exchanger AE2. J. Clin. Invest. 93, 1430–1438.
Andrews, G. K. (2008). Regulation and function of Zip4, the acrodermatitis enteropathica gene. Biochem. Soc. Trans. 36, 1242–1246.
Angelow, S., Haselbach, M., and Galla, H. J. (2003). Functional characterisation of the active ascorbic acid transport into cerebrospinal fluid using primary cultured choroid plexus cells. Brain Res. 988, 105–113.
Angelow, S., Zeni, P., and Galla, H. J. (2004). Usefulness and limitation of primary cultured porcine choroid plexus epithelial cells as an in vitro model to study drug transport at the blood-CSF barrier. Adv. Drug Deliv. Rev. 56, 1859–1873.
Augustin, R. (2010). The protein family of glucose transport facilitators: It’s not only about glucose after all. IUBMB Life 62, 315–333.
Bae, S. Y., Xu, Q., Hutchinson, D., and Colton, C. A. (2005). Y+ and y+ L arginine transporters in neuronal cells expressing tyrosine hydroxylase. Biochim. Biophys. Acta 1745, 65–73.
Bairamian, D., Johanson, C. E., Parmelee, J. T., and Epstein, M. H. (1991). Potassium cotransport with sodium and chloride in the choroid plexus. J. Neurochem. 56, 1623–1629.
Belloni-Olivi, L., Marshall, C., Laal, B., Andrews, G. K., and Bressler, J. (2009). Localization of zip1 and zip4 mRNA in the adult rat brain. J. Neurosci. Res. 87, 3221–3230.
Berry, G. T., Mallee, J. J., Kwon, H. M., Rim, J. S., Mulla, W. R., Muenke, M., and Spinner, N. B. (1995). The human osmoregulatory Na+/myo-inositol cotransporter gene (SLC5A3): molecular cloning and localization to chromosome 21. Genomics 25, 507–513.
Berry, G. T., Wu, S., Buccafusca, R., Ren, J., Gonzales, L. W., Ballard, P. L., Golden, J. A., Stevens, M. J., and Greer, J. J. (2003). Loss of murine Na+/myo-inositol cotransporter leads to brain myo-inositol depletion and central apnea. J. Biol. Chem. 278, 18297–18302.
Bhatnagar, S., and Taneja, S. (2001). Zinc and cognitive development. Br. J. Nutr. 85(Suppl. 2), S139–S145.
Bhattacharjee, Y. (2006). Neuroscience. “Google of the brain”: atlas maps brain’s genetic activity. Science 313, 1879.
Bissig, M., Hagenbuch, B., Stieger, B., Koller, T., and Meier, P. J. (1994). Functional expression cloning of the canalicular sulfate transport system of rat hepatocytes. J. Biol. Chem. 269, 3017–3021.
Blau, N., Bonafe, L., Krageloh-Mann, I., Thony, B., Kierat, L., Hausler, M., and Ramaekers, V. (2003). Cerebrospinal fluid pterins and folates in Aicardi-Goutieres syndrome: a new phenotype. Neurology 61, 642–647.
Botez, M. I., Young, S. N., Bachevalier, J., and Gauthier, S. (1982). Effect of folic acid and vitamin B12 deficiencies on 5-hydroxyindoleacetic acid in human cerebrospinal fluid. Ann. Neurol. 12, 479–484.
Boulland, J. L., Osen, K. K., Levy, L. M., Danbolt, N. C., Edwards, R. H., Storm-Mathisen, J., and Chaudhry, F. A. (2002). Cell-specific expression of the glutamine transporter SN1 suggests differences in dependence on the glutamine cycle. Eur. J. Neurosci. 15, 1615–1631.
Bouzinova, E. V., Praetorius, J., Virkki, L. V., Nielsen, S., Boron, W. F., and Aalkjaer, C. (2005). Na+-dependent HCO3- uptake into the rat choroid plexus epithelium is partially DIDS sensitive. Am. J. Physiol. Cell Physiol. 289, C1448–C1456.
Broer, A., Wagner, C. A., Lang, F., and Broer, S. (2000). The heterodimeric amino acid transporter 4F2hc/y+ LAT2 mediates arginine efflux in exchange with glutamine. Biochem. J. 349(Pt 3), 787–795.
Brown, P. D., Davies, S. L., Speake, T., and Millar, I. D. (2004). Molecular mechanisms of cerebrospinal fluid production. Neuroscience 129, 957–970.
Bull, P. C., and Cox, D. W. (1994). Wilson disease and Menkes disease: new handles on heavy-metal transport. Trends Genet. 10, 246–252.
Bush, A. I. (2002). Metal complexing agents as therapies for Alzheimer’s disease. Neurobiol. Aging 23, 1031–1038.
Busse, S., Breder, J., Dinkel, K., Reymann, K. G., and Schroder, U. H. (2005). Inhibitors of cation-chloride-cotransporters affect hypoxic/hypoglycemic injury in hippocampal slices. Brain Res. 1046, 116–121.
Chandler, J. D., Williams, E. D., Slavin, J. L., Best, J. D., and Rogers, S. (2003). Expression and localization of GLUT1 and GLUT12 in prostate carcinoma. Cancer 97, 2035–2042.
Chanoine, J. P., Alex, S., Fang, S. L., Stone, S., Leonard, J. L., Korhle, J., and Braverman, L. E. (1992). Role of transthyretin in the transport of thyroxine from the blood to the choroid plexus, the cerebrospinal fluid, and the brain. Endocrinology 130, 933–938.
Chau, J. F., Lee, M. K., Law, J. W., Chung, S. K., and Chung, S. S. (2005). Sodium/myo-inositol cotransporter-1 is essential for the development and function of the peripheral nerves. FASEB J. 19, 1887–1889.
Chaudhry, F. A., Reimer, R. J., Krizaj, D., Barber, D., Storm-Mathisen, J., Copenhagen, D. R., and Edwards, R. H. (1999). Molecular analysis of system N suggests novel physiological roles in nitrogen metabolism and synaptic transmission. Cell 99, 769–780.
Choudhuri, S., Cherrington, N. J., Li, N., and Klaassen, C. D. (2003). Constitutive expression of various xenobiotic and endobiotic transporter mRNAs in the choroid plexus of rats. Drug Metab. Dispos. 31, 1337–1345.
Chu, C., Li, J. Y., Boado, R. J., and Pardridge, W. M. (2008). Blood-brain barrier genomics and cloning of a novel organic anion transporter. J. Cereb. Blood Flow Metab. 28, 291–301.
Coady, M. J., Wallendorff, B., Gagnon, D. G., and Lapointe, J. Y. (2002). Identification of a novel Na+/myo-inositol cotransporter. J. Biol. Chem. 277, 35219–35224.
Coleman, J. E. (1992). Zinc proteins: enzymes, storage proteins, transcription factors, and replication proteins. Annu. Rev. Biochem. 61, 897–946.
Cornford, E. M., Varesi, J. B., Hyman, S., Damian, R. T., and Raleigh, M. J. (1997). Mitochondrial content of choroid plexus epithelium. Exp. Brain Res. 116, 399–405.
Crouthamel, M. H., Kelly, E. J., and Ho, R. J. (2012). Development and characterization of transgenic mouse models for conditional gene knockout in the blood-brain and blood-CSF barriers. Transgenic Res. 21, 113–130.
Dahlin, A., Royall, J., Hohmann, J. G., and Wang, J. (2009). Expression profiling of the solute carrier gene family in the mouse brain. J. Pharmacol. Exp. Ther. 329, 558–570.
Dahlin, A., Xia, L., Kong, W., Hevner, R., and Wang, J. (2007). Expression and immunolocalization of the plasma membrane monoamine transporter in the brain. Neuroscience 146, 1193–1211.
Damkier, H. H., Brown, P. D., and Praetorius, J. (2010). Epithelial pathways in choroid plexus electrolyte transport. Physiology (Bethesda) 25, 239–249.
Damkier, H. H., Nielsen, S., and Praetorius, J. (2007). Molecular expression of SLC4-derived Na+-dependent anion transporters in selected human tissues. Am. J. Physiol. Regul. Integr. Comp. Physiol. 293, R2136–R2146.
Damkier, H. H., and Praetorius, J. (2012). Genetic ablation of Slc4a10 alters the expression pattern of transporters involved in solute movement in the mouse choroid plexus. Am. J. Physiol. Cell Physiol. 302, C1452–C1459.
Damkier, H. H., Prasad, V., Hubner, C. A., and Praetorius, J. (2009). Nhe1 is a luminal Na+/H+ exchanger in mouse choroid plexus and is targeted to the basolateral membrane in Ncbe/Nbcn2-null mice. Am. J. Physiol. Cell Physiol. 296, C1291–C1300.
Davson, H., and Segal, M. B. (1970). The effects of some inhibitors and accelerators of sodium transport on the turnover of 22Na in the cerebrospinal fluid and the brain. J. Physiol. (Lond.) 209, 131–153.
Davson, H., Welch, K., and Segal, M. B. (1987). The Physiology and Pathophysiology of the Cerebrospinal Fluid. Edinburgh: Churchill Livingston.
Dawson, R. M., and Freinkel, N. (1961). The distribution of free mesoinositol in mammalian tissues, including some observations on the lactating rat. Biochem. J. 78, 606–610.
de Rougemont, J., Ames, A. III, Nesbett, F. B., and Hofmann, H. F. (1960). Fluid formed by choroid plexus; a technique for its collection and a comparison of its electrolyte composition with serum and cisternal fluids. J. Neurophysiol. 23, 485–495.
Deng, Q. S., and Johanson, C. E. (1989). Stilbenes inhibit exchange of chloride between blood, choroid plexus and cerebrospinal fluid. Brain Res. 501, 183–187.
Devirgiliis, C., Zalewski, P. D., Perozzi, G., and Murgia, C. (2007). Zinc fluxes and zinc transporter genes in chronic diseases. Mutat. Res. 622, 84–93.
Devraj, K., Klinger, M. E., Myers, R. L., Mokashi, A., Hawkins, R. A., and Simpson, I. A. (2011). GLUT-1 glucose transporters in the blood-brain barrier: differential phosphorylation. J. Neurosci. Res. 89, 1913–1925.
Dratman, M. B., Crutchfield, F. L., and Schoenhoff, M. B. (1991). Transport of iodothyronines from bloodstream to brain: contributions by blood:brain and choroid plexus:cerebrospinal fluid barriers. Brain Res. 554, 229–236.
Duan, H., and Wang, J. (2010). Selective transport of monoamine neurotransmitters by human plasma membrane monoamine transporter and organic cation transporter 3. J. Pharmacol. Exp. Ther. 335, 743–753.
Dufner-Beattie, J., Kuo, Y. M., Gitschier, J., and Andrews, G. K. (2004). The adaptive response to dietary zinc in mice involves the differential cellular localization and zinc regulation of the zinc transporters ZIP4 and ZIP5. J. Biol. Chem. 279, 49082–49090.
Dufner-Beattie, J., Langmade, S. J., Wang, F., Eide, D., and Andrews, G. K. (2003a). Structure, function, and regulation of a subfamily of mouse zinc transporter genes. J. Biol. Chem. 278, 50142–50150.
Dufner-Beattie, J., Wang, F., Kuo, Y. M., Gitschier, J., Eide, D., and Andrews, G. K. (2003b). The acrodermatitis enteropathica gene ZIP4 encodes a tissue-specific, zinc-regulated zinc transporter in mice. J. Biol. Chem. 278, 33474–33481.
Dufner-Beattie, J., Weaver, B. P., Geiser, J., Bilgen, M., Larson, M., Xu, W., and Andrews, G. K. (2007). The mouse acrodermatitis enteropathica gene Slc39a4 (Zip4) is essential for early development and heterozygosity causes hypersensitivity to zinc deficiency. Hum. Mol. Genet. 16, 1391–1399.
Dumitrescu, A. M., Liao, X. H., Weiss, R. E., Millen, K., and Refetoff, S. (2006). Tissue-specific thyroid hormone deprivation and excess in monocarboxylate transporter (MCT) 8-deficient mice. Endocrinology 147, 4036–4043.
Dye, J. F., Vause, S., Johnston, T., Clark, P., Firth, J. A., D’Souza, S. W., Sibley, C. P., and Glazier, J. D. (2004). Characterization of cationic amino acid transporters and expression of endothelial nitric oxide synthase in human placental microvascular endothelial cells. FASEB J. 18, 125–127.
Earl, C., Chantry, A., Mohammad, N., and Glynn, P. (1988). Zinc ions stabilise the association of basic protein with brain myelin membranes. J. Neurochem. 51, 718–724.
Egleton, R. D., Campos, C. C., Huber, J. D., Brown, R. C., and Davis, T. P. (2003). Differential effects of diabetes on rat choroid plexus ion transporter expression. Diabetes 52, 1496–1501.
Eldridge, C. F., Bunge, M. B., Bunge, R. P., and Wood, P. M. (1987). Differentiation of axon-related Schwann cells in vitro. I. Ascorbic acid regulates basal lamina assembly and myelin formation. J. Cell Biol. 105, 1023–1034.
Engel, K., and Wang, J. (2005). Interaction of organic cations with a newly identified plasma membrane monoamine transporter. Mol. Pharmacol. 68, 1397–1407.
Engel, K., Zhou, M., and Wang, J. (2004). Identification and characterization of a novel monoamine transporter in the human brain. J. Biol. Chem. 279, 50042–50049.
Ferrand-Drake, M. (2001). Cell death in the choroid plexus following transient forebrain global ischemia in the rat. Microsc. Res. Tech. 52, 130–136.
Ferrand-Drake, M., and Wieloch, T. (1999). The time-course of DNA fragmentation in the choroid plexus and the CA1 region following transient global ischemia in the rat brain. The effect of intra-ischemic hypothermia. Neuroscience 93, 537–549.
Flessner, L. B., and Moley, K. H. (2009). Similar [DE]XXXL[LI] motifs differentially target GLUT8 and GLUT12 in Chinese hamster ovary cells. Traffic 10, 324–333.
Frederickson, C. J., Koh, J. Y., and Bush, A. I. (2005). The neurobiology of zinc in health and disease. Nat. Rev. Neurosci. 6, 449–462.
Friesema, E. C., Ganguly, S., Abdalla, A., Manning Fox, J. E., Halestrap, A. P., and Visser, T. J. (2003). Identification of monocarboxylate transporter 8 as a specific thyroid hormone transporter. J. Biol. Chem. 278, 40128–40135.
Ganapathy, V., Smith, S. B., and Prasad, P. D. (2004). SLC19: the folate/thiamine transporter family. Pflugers Arch. 447, 641–646.
Gao, B., Stieger, B., Noe, B., Fritschy, J. M., and Meier, P. J. (1999). Localization of the organic anion transporting polypeptide 2 (Oatp2) in capillary endothelium and choroid plexus epithelium of rat brain. J. Histochem. Cytochem. 47, 1255–1264.
Garcia Mde, L., Salazar, K., Millan, C., Rodriguez, F., Montecinos, H., Caprile, T., Silva, C., Cortes, C., Reinicke, K., Vera, J. C., Aguayo, L. G., Olate, J., Molina, B., and Nualart, F. (2005). Sodium vitamin C cotransporter SVCT2 is expressed in hypothalamic glial cells. Glia 50, 32–47.
Garcia-Perez, A., and Burg, M. B. (1991). Renal medullary organic osmolytes. Physiol. Rev. 71, 1081–1115.
Gawenis, L. R., Ledoussal, C., Judd, L. M., Prasad, V., Alper, S. L., Stuart-Tilley, A., Woo, A. L., Grisham, C., Sanford, L. P., Doetschman, T., Miller, M. L., and Shull, G. E. (2004). Mice with a targeted disruption of the AE2 Cl-/HCO3-exchanger are achlorhydric. J. Biol. Chem. 279, 30531–30539.
Ghersi-Egea, J. F., and Strazielle, N. (2002). Choroid plexus transporters for drugs and other xenobiotics. J. Drug Target 10, 353–357.
Ginger, R. S., Askew, S. E., Ogborne, R. M., Wilson, S., Ferdinando, D., Dadd, T., Smith, A. M., Kazi, S., Szerencsei, R. T., Winkfein, R. J., Schnetkamp, P. P., and Green, M. R. (2008). SLC24A5 encodes a trans-Golgi network protein with potassium-dependent sodium-calcium exchange activity that regulates human epidermal melanogenesis. J. Biol. Chem. 283, 5486–5495.
Girard, J. P., Baekkevold, E. S., Feliu, J., Brandtzaeg, P., and Amalric, F. (1999). Molecular cloning and functional analysis of SUT-1, a sulfate transporter from human high endothelial venules. Proc. Natl. Acad. Sci. U.S.A. 96, 12772–12777.
Goldman, I. D. (1971). The characteristics of the membrane transport of amethopterin and the naturally occurring folates. Ann. N. Y. Acad. Sci. 186, 400–422.
Gosmanov, A. R., Schneider, E. G., and Thomason, D. B. (2003). NKCC activity restores muscle water during hyperosmotic challenge independent of insulin, ERK, and p38 MAPK. Am. J. Physiol. Regul. Integr. Comp. Physiol. 284, R655–R665.
Graff, C. L., and Pollack, G. M. (2004). Drug transport at the blood-brain barrier and the choroid plexus. Curr. Drug Metab. 5, 95–108.
Grijota-Martinez, C., Diez, D., Morreale de Escobar, G., Bernal, J., and Morte, B. (2011). Lack of action of exogenously administered T3 on the fetal rat brain despite expression of the monocarboxylate transporter 8. Endocrinology 152, 1713–1721.
Groothuis, D. R., and Levy, R. M. (1997). The entry of antiviral and antiretroviral drugs into the central nervous system. J. Neurovirol. 3, 387–400.
Guo, W., Shimada, S., Tajiri, H., Yamauchi, A., Yamashita, T., Okada, S., and Tohyama, M. (1997). Developmental regulation of Na+/myo-inositol cotransporter gene expression. Brain Res. Mol. Brain Res. 51, 91–96.
Gurnett, C. A., Veile, R., Zempel, J., Blackburn, L., Lovett, M., and Bowcock, A. (2008). Disruption of sodium bicarbonate transporter SLC4A10 in a patient with complex partial epilepsy and mental retardation. Arch. Neurol. 65, 550–553.
Gybina, A. A., and Prohaska, J. R. (2006). Variable response of selected cuproproteins in rat choroid plexus and cerebellum following perinatal copper deficiency. Genes Nutr. 1, 51–59.
Haas, M., and Forbush, B. III. (2000). The Na-K-Cl cotransporter of secretory epithelia. Annu. Rev. Physiol. 62, 515–534.
Haber, R. S., Weinstein, S. P., O’Boyle, E., and Morgello, S. (1993). Tissue distribution of the human GLUT3 glucose transporter. Endocrinology 132, 2538–2543.
Hakvoort, A., Haselbach, M., and Galla, H. J. (1998). Active transport properties of porcine choroid plexus cells in culture. Brain Res. 795, 247–256.
Halwachs, S., Lakoma, C., Schafer, I., Seibel, P., and Honscha, W. (2011). The antiepileptic drugs phenobarbital and carbamazepine reduce transport of methotrexate in rat choroid plexus by down-regulation of the reduced folate carrier. Mol. Pharmacol. 80, 621–629.
Hammarstrom, L. (1966). Autoradiographic studies on the distribution of 14C-labelled ascorbic acid and dehydroascorbic acid. Acta Physiol. Scand. 70(Suppl. 289), 1–83.
Handler, J. S., and Kwon, H. M. (1996). Regulation of the myo-inositol and betaine cotransporters by tonicity. Kidney Int. 49, 1682–1683.
Harrison, F. E., Dawes, S. M., Meredith, M. E., Babaev, V. R., Li, L., and May, J. M. (2010). Low vitamin C and increased oxidative stress and cell death in mice that lack the sodium-dependent vitamin C transporter SVCT2. Free Radic. Biol. Med. 49, 821–829.
Heckel, T., Broer, A., Wiesinger, H., Lang, F., and Broer, S. (2003). Asymmetry of glutamine transporters in cultured neural cells. Neurochem. Int. 43, 289–298.
Hediger, M. A., Romero, M. F., Peng, J. B., Rolfs, A., Takanaga, H., and Bruford, E. A. (2004). The ABCs of solute carriers: physiological, pathological and therapeutic implications of human membrane transport proteinsIntroduction. Pflugers Arch. 447, 465–468.
Heuer, H., Maier, M. K., Iden, S., Mittag, J., Friesema, E. C., Visser, T. J., and Bauer, K. (2005). The monocarboxylate transporter 8 linked to human psychomotor retardation is highly expressed in thyroid hormone-sensitive neuron populations. Endocrinology 146, 1701–1706.
Hinken, M., Halwachs, S., Kneuer, C., and Honscha, W. (2011). Subcellular localization and distribution of the reduced folate carrier in normal rat tissues. Eur. J. Histochem. 55, e3.
Ho, H. T., Pan, Y., Cui, Z., Duan, H., Swaan, P. W., and Wang, J. (2011). Molecular analysis and structure-activity relationship modeling of the substrate/inhibitor interaction site of plasma membrane monoamine transporter. J. Pharmacol. Exp. Ther. 339, 376–385.
Holub, B. J. (1986). Metabolism and function of myo-inositol and inositol phospholipids. Annu. Rev. Nutr. 6, 563–597.
Hosoya, K., and Tachikawa, M. (2011). Roles of organic anion/cation transporters at the blood-brain and blood-cerebrospinal fluid barriers involving uremic toxins. Clin. Exp. Nephrol. 15, 478–485.
Inoue, K., Shimada, S., Minami, Y., Morimura, H., Miyai, A., Yamauchi, A., and Tohyama, M. (1996). Cellular localization of Na+/MYO-inositol co-transporter mRNA in the rat brain. Neuroreport 7, 1195–1198.
Itagaki, S., Ganapathy, V., Ho, H. T., Zhou, M., Babu, E., and Wang, J. (2012). Electrophysiological characterization of the polyspecific organic cation transporter plasma membrane monoamine transporter. Drug Metab. Dispos. 40, 1138–1143.
Jacobs, S., Ruusuvuori, E., Sipila, S. T., Haapanen, A., Damkier, H. H., Kurth, I., Hentschke, M., Schweizer, M., Rudhard, Y., Laatikainen, L. M., Tyynela, J., Praetorius, J., Voipio, J., and Hubner, C. A. (2008). Mice with targeted Slc4a10 gene disruption have small brain ventricles and show reduced neuronal excitability. Proc. Natl. Acad. Sci. U.S.A. 105, 311–316.
Javaheri, S., and Wagner, K. R. (1993). Bumetanide decreases canine cerebrospinal fluid production. In vivo evidence for NaCl cotransport in the central nervous system. J. Clin. Invest. 92, 2257–2261.
Johanson, C., McMillan, P., Tavares, R., Spangenberger, A., Duncan, J., Silverberg, G., and Stopa, E. (2004). Homeostatic capabilities of the choroid plexus epithelium in Alzheimer’s disease. Cerebrospinal Fluid Res. 1, 3.
Johanson, C. E., Duncan, J. A. III, Klinge, P. M., Brinker, T., Stopa, E. G., and Silverberg, G. D. (2008). Multiplicity of cerebrospinal fluid functions: new challenges in health and disease. Cerebrospinal Fluid Res. 5, 10.
Johanson, C. E., Reed, D. J., and Woodbury, D. M. (1974). Active transport of sodium and potassium by the choroid plexus of the rat. J. Physiol. (Lond.) 241, 359–372.
Kahle, K. T., Staley, K. J., Nahed, B. V., Gamba, G., Hebert, S. C., Lifton, R. P., and Mount, D. B. (2008). Roles of the cation-chloride cotransporters in neurological disease. Nat. Clin. Pract. Neurol. 4, 490–503.
Kamal, M. A., Keep, R. F., and Smith, D. E. (2008). Role and relevance of PEPT2 in drug disposition, dynamics, and toxicity. Drug Metab. Pharmacokinet. 23, 236–242.
Kanaka, C., Ohno, K., Okabe, A., Kuriyama, K., Itoh, T., Fukuda, A., and Sato, K. (2001). The differential expression patterns of messenger RNAs encoding K-Cl cotransporters (KCC1,2) and Na-K-2Cl cotransporter (NKCC1) in the rat nervous system. Neuroscience 104, 933–946.
Kao, L., Kurtz, L. M., Shao, X., Papadopoulos, M. C., Liu, L., Bok, D., Nusinowitz, S., Chen, B., Stella, S. L., Andre, M., Weinreb, J., Luong, S. S., Piri, N., Kwong, J. M., Newman, D., and Kurtz, I. (2011). Severe neurologic impairment in mice with targeted disruption of the electrogenic sodium bicarbonate cotransporter NBCe2 (Slc4a5 gene). J. Biol. Chem. 286, 32563–32574.
Kassem, N. A., Deane, R., Segal, M. B., Chen, R., and Preston, J. E. (2007). Thyroxine (T4) transfer from CSF to choroid plexus and ventricular brain regions in rabbit: contributory role of P-glycoprotein and organic anion transporting polypeptides. Brain Res. 1181, 44–50.
Keep, R. F., and Jones, H. C. (1990). A morphometric study on the development of the lateral ventricle choroid plexus, choroid plexus capillaries and ventricular ependyma in the rat. Brain Res. Dev. Brain Res. 56, 47–53.
Keep, R. F., Jones, H. C., and Cawkwell, R. D. (1986). A morphometric analysis of the development of the fourth ventricle choroid plexus in the rat. Brain Res. 392, 77–85.
Keep, R. F., and Smith, D. E. (2011). Choroid plexus transport: gene deletion studies. Fluids Barriers CNS 8, 26.
Keep, R. F., Xiang, J., and Betz, A. L. (1994). Potassium cotransport at the rat choroid plexus. Am. J. Physiol. 267, C1616–C1622.
Keller, K. A., Chu, Y., Grider, A., and Coffield, J. A. (2000). Supplementation with L-histidine during dietary zinc repletion improves short-term memory in zinc-restricted young adult male rats. J. Nutr. 130, 1633–1640.
Kilberg, M. S., Handlogten, M. E., and Christensen, H. N. (1980). Characteristics of an amino acid transport system in rat liver for glutamine, asparagine, histidine, and closely related analogs. J. Biol. Chem. 255, 4011–4019.
Kuo, Y. M., Gybina, A. A., Pyatskowit, J. W., Gitschier, J., and Prohaska, J. R. (2006). Copper transport protein (Ctr1) levels in mice are tissue specific and dependent on copper status. J. Nutr. 136, 21–26.
Kuo, Y. M., Zhou, B., Cosco, D., and Gitschier, J. (2001). The copper transporter CTR1 provides an essential function in mammalian embryonic development. Proc. Natl. Acad. Sci. U.S.A. 98, 6836–6841.
Kusuhara, H., He, Z., Nagata, Y., Nozaki, Y., Ito, T., Masuda, H., Meier, P. J., Abe, T., and Sugiyama, Y. (2003). Expression and functional involvement of organic anion transporting polypeptide subtype 3 (Slc21a7) in rat choroid plexus. Pharm. Res. 20, 720–727.
Kusuhara, H., and Sugiyama, Y. (2004). Efflux transport systems for organic anions and cations at the blood-CSF barrier. Adv. Drug Deliv. Rev. 56, 1741–1763.
Kwon, H. M., Yamauchi, A., Uchida, S., Preston, A. S., Garcia-Perez, A., Burg, M. B., and Handler, J. S. (1992). Cloning of the cDNa for a Na+/myo-inositol cotransporter, a hypertonicity stress protein. J. Biol. Chem. 267, 6297–6301.
Lamason, R. L., Mohideen, M. A., Mest, J. R., Wong, A. C., Norton, H. L., Aros, M. C., Jurynec, M. J., Mao, X., Humphreville, V. R., Humbert, J. E., Sinha, S., Moore, J. L., Jagadeeswaran, P., Zhao, W., Ning, G., Makalowska, I., McKeigue, P. M., O’Donnell, D., Kittles, R., Parra, E. J., Mangini, N. J., Grunwald, D. J., Shriver, M. D., Canfield, V. A., and Cheng, K. C. (2005). SLC24A5, a putative cation exchanger, affects pigmentation in zebrafish and humans. Science 310, 1782–1786.
Lang, M. F., Salinin, S., Ridder, D. A., Kleesiek, J., Hroudova, J., Berger, S., Schutz, G., and Schwaninger, M. (2011). A transgenic approach to identify thyroxine transporter-expressing structures in brain development. J. Neuroendocrinol. 23, 1194–1203.
Lee, J., Prohaska, J. R., and Thiele, D. J. (2001). Essential role for mammalian copper transporter Ctr1 in copper homeostasis and embryonic development. Proc. Natl. Acad. Sci. U.S.A. 98, 6842–6847.
Lee, J. M., Zipfel, G. J., Park, K. H., He, Y. Y., Hsu, C. Y., and Choi, D. W. (2002). Zinc translocation accelerates infarction after mild transient focal ischemia. Neuroscience 115, 871–878.
Lee, J. Y., Mook-Jung, I., and Koh, J. Y. (1999). Histochemically reactive zinc in plaques of the Swedish mutant beta-amyloid precursor protein transgenic mice. J. Neurosci. 19, RC10.
Lein, E. S., Hawrylycz, M. J., Ao, N., Ayres, M., Bensinger, A., Bernard, A., Boe, A. F., Boguski, M. S., Brockway, K. S., Byrnes, E. J., Chen, L., Chen, T. M., Chin, M. C., Chong, J., Crook, B. E., Czaplinska, A., Dang, C. N., Datta, S., Dee, N. R., Desaki, A. L., Desta, T., Diep, E., Dolbeare, T. A., Donelan, M. J., Dong, H. W., Dougherty, J. G., Duncan, B. J., Ebbert, A. J., Eichele, G., Estin, L. K., Faber, C., Facer, B. A., Fields, R., Fischer, S. R., Fliss, T. P., Frensley, C., Gates, S. N., Glattfelder, K. J., Halverson, K. R., Hart, M. R., Hohmann, J. G., Howell, M. P., Jeung, D. P., Johnson, R. A., Karr, P. T., Kawal, R., Kidney, J. M., Knapik, R. H., Kuan, C. L., Lake, J. H., Laramee, A. R., Larsen, K. D., Lau, C., Lemon, T. A., Liang, A. J., Liu, Y., Luong, L. T., Michaels, J., Morgan, J. J., Morgan, R. J., Mortrud, M. T., Mosqueda, N. F., Ng, L. L., Ng, R., Orta, G. J., Overly, C. C., Pak, T. H., Parry, S. E., Pathak, S. D., Pearson, O. C., Puchalski, R. B., Riley, Z. L., Rockett, H. R., Rowland, S. A., Royall, J. J., Ruiz, M. J., Sarno, N. R., Schaffnit, K., Shapovalova, N. V., Sivisay, T., Slaughterbeck, C. R., Smith, S. C., Smith, K. A., Smith, B. I., Sodt, A. J., Stewart, N. N., Stumpf, K. R., Sunkin, S. M., Sutram, M., Tam, A., Teemer, C. D., Thaller, C., Thompson, C. L., Varnam, L. R., Visel, A., Whitlock, R. M., Wohnoutka, P. E., Wolkey, C. K., Wong, V. Y., Wood, M., Yaylaoglu, M. B., Young, R. C., Youngstrom, B. L., Yuan, X. F., Zhang, B., Zwingman, T. A., and Jones, A. R. (2007). Genome-wide atlas of gene expression in the adult mouse brain. Nature 445, 168–176.
Lindsey, A. E., Schneider, K., Simmons, D. M., Baron, R., Lee, B. S., and Kopito, R. R. (1990). Functional expression and subcellular localization of an anion exchanger cloned from choroid plexus. Proc. Natl. Acad. Sci. U.S.A. 87, 5278–5282.
Lobo, S., Wiczer, B. M., Smith, A. J., Hall, A. M., and Bernlohr, D. A. (2007). Fatty acid metabolism in adipocytes: functional analysis of fatty acid transport proteins 1 and 4. J. Lipid Res. 48, 609–620.
Lu, K. T., Wu, C. Y., Cheng, N. C., Wo, Y. Y., Yang, J. T., Yen, H. H., and Yang, Y. L. (2006). Inhibition of the Na+-K+-2Cl−-cotransporter in choroid plexus attenuates traumatic brain injury-induced brain edema and neuronal damage. Eur. J. Pharmacol. 548, 99–105.
Maher, F., Vannucci, S., Takeda, J., and Simpson, I. A. (1992). Expression of mouse-GLUT3 and human-GLUT3 glucose transporter proteins in brain. Biochem. Biophys. Res. Commun. 182, 703–711.
Majumdar, D., and Bevensee, M. O. (2010). Na-coupled bicarbonate transporters of the solute carrier 4 family in the nervous system: function, localization, and relevance to neurologic function. Neuroscience 171, 951–972.
Markovic, I., Segal, M., Djuricic, B., and Redzic, Z. (2008). Kinetics of nucleoside uptake by the basolateral side of the sheep choroid plexus epithelium perfused in situ. Exp. Physiol. 93, 325–333.
Markovich, D., Forgo, J., Stange, G., Biber, J., and Murer, H. (1993). Expression cloning of rat renal Na+/SO4(2-) cotransport. Proc. Natl. Acad. Sci. U.S.A. 90, 8073–8077.
Massaro, T. F., Mohs, M., and Fosmire, G. (1982). Effects of moderate zinc deficiency on cognitive performance in young adult rats. Physiol. Behav. 29, 117–121.
Masuzawa, T., Ohta, T., Kawamura, M., Nakahara, N., and Sato, F. (1984). Immunohistochemical localization of Na+, K+-ATPase in the choroid plexus. Brain Res. 302, 357–362.
Matsumoto, N., Kitayama, H., Kitada, M., Kimura, K., Noda, M., and Ide, C. (2003). Isolation of a set of genes expressed in the choroid plexus of the mouse using suppression subtractive hybridization. Neuroscience 117, 405–415.
Mayer, S. E., and Sanders-Bush, E. (1993). Sodium-dependent antiporters in choroid plexus epithelial cultures from rabbit. J. Neurochem. 60, 1308–1316.
McAnulty, R. J., and Laurent, G. J. (1987). Collagen synthesis and degradation in vivo. Evidence for rapid rates of collagen turnover with extensive degradation of newly synthesized collagen in tissues of the adult rat. Coll. Relat. Res. 7, 93–104.
McCall, A. L., Van Bueren, A. M., Moholt-Siebert, M., Cherry, N. J., and Woodward, W. R. (1994). Immunohistochemical localization of the neuron-specific glucose transporter (GLUT3) to neuropil in adult rat brain. Brain Res. 659, 292–297.
Michaelis, T., Helms, G., Merboldt, K. D., Hanicke, W., Bruhn, H., and Frahm, J. (1993). Identification of Scyllo-inositol in proton NMR spectra of human brain in vivo. NMR. Biomed. 6, 105–109.
Miller, D. S. (2004). Confocal imaging of xenobiotic transport across the choroid plexus. Adv. Drug Deliv. Rev. 56, 1811–1824.
Mitchell, R. W., On, N. H., Del Bigio, M. R., Miller, D. W., and Hatch, G. M. (2011). Fatty acid transport protein expression in human brain and potential role in fatty acid transport across human brain microvessel endothelial cells. J. Neurochem. 117, 735–746.
Murphy, V. A., and Johanson, C. E. (1990). Na(+)-H+ exchange in choroid plexus and CSF in acute metabolic acidosis or alkalosis. Am. J. Physiol. 258, F1528–F1537.
Nagamatsu, S., Sawa, H., Kamada, K., Nakamichi, Y., Yoshimoto, K., and Hoshino, T. (1993). Neuron-specific glucose transporter (NSGT): CNS distribution of GLUT3 rat glucose transporter (RGT3) in rat central neurons. FEBS Lett. 334, 289–295.
Nagatsu, T. (1997). Isoquinoline neurotoxins in the brain and Parkinson’s disease. Neurosci. Res. 29, 99–111.
Nakanishi, T., Sugawara, M., Huang, W., Martindale, R. G., Leibach, F. H., Ganapathy, M. E., Prasad, P. D., and Ganapathy, V. (2001). Structure, function, and tissue expression pattern of human SN2, a subtype of the amino acid transport system N. Biochem. Biophys. Res. Commun. 281, 1343–1348.
Nowell, S., and Falany, C. N. (2006). Pharmacogenetics of human cytosolic sulfotransferases. Oncogene 25, 1673–1678.
Nuzzo, S., Meneghini, C., Mobilioo, S., Haas, H., Riccio, P., Fasano, A., Cavatorta, P., and Morante, S. (2002). An x-ray absorption spectroscopy study of the zinc environment in Langmuir-Blodgett phospholipid multilayers. Biophys. J. 83, 3507–3512.
O’Donnell, M. E., Tran, L., Lam, T. I., Liu, X. B., and Anderson, S. E. (2004). Bumetanide inhibition of the blood-brain barrier Na-K-Cl cotransporter reduces edema formation in the rat middle cerebral artery occlusion model of stroke. J. Cereb. Blood Flow Metab. 24, 1046–1056.
Ohtsuki, S., Takizawa, T., Takanaga, H., Hori, S., Hosoya, K., and Terasaki, T. (2004). Localization of organic anion transporting polypeptide 3 (oatp3) in mouse brain parenchymal and capillary endothelial cells. J. Neurochem. 90, 743–749.
Ohtsuki, S., Takizawa, T., Takanaga, H., Terasaki, N., Kitazawa, T., Sasaki, M., Abe, T., Hosoya, K., and Terasaki, T. (2003). In vitro study of the functional expression of organic anion transporting polypeptide 3 at rat choroid plexus epithelial cells and its involvement in the cerebrospinal fluid-to-blood transport of estrone-3-sulfate. Mol. Pharmacol. 63, 532–537.
Okura, T., Kato, S., Takano, Y., Sato, T., Yamashita, A., Morimoto, R., Ohtsuki, S., Terasaki, T., and Deguchi, Y. (2011). Functional characterization of rat plasma membrane monoamine transporter in the blood-brain and blood-cerebrospinal fluid barriers. J. Pharm. Sci. 100, 3924–3938.
Palmano, K. P., Whiting, P. H., and Hawthorne, J. N. (1977). Free and lipid myo-inositol in tissues from rats with acute and less severe streptozotocin-induced diabetes. Biochem. J. 167, 229–235.
Palmieri, F. (2004). The mitochondrial transporter family (SLC25): physiological and pathological implications. Pflugers Arch. 447, 689–709.
Pardridge, W. M. (2011). Drug transport in brain via the cerebrospinal fluid. Fluids Barriers CNS 8, 7.
Parker, M. D., Musa-Aziz, R., Rojas, J. D., Choi, I., Daly, C. M., and Boron, W. F. (2008). Characterization of human SLC4A10 as an electroneutral Na/HCO3 cotransporter (NBCn2) with Cl− self-exchange activity. J. Biol. Chem. 283, 12777–12788.
Pizzagalli, F., Hagenbuch, B., Stieger, B., Klenk, U., Folkers, G., and Meier, P. J. (2002). Identification of a novel human organic anion transporting polypeptide as a high affinity thyroxine transporter. Mol. Endocrinol. 16, 2283–2296.
Plotkin, M. D., Kaplan, M. R., Peterson, L. N., Gullans, S. R., Hebert, S. C., and Delpire, E. (1997a). Expression of the Na(+)-K(+)-2Cl− cotransporter BSC2 in the nervous system. Am. J. Physiol. 272, C173–C183.
Plotkin, M. D., Snyder, E. Y., Hebert, S. C., and Delpire, E. (1997b). Expression of the Na-K-2Cl cotransporter is developmentally regulated in postnatal rat brains: a possible mechanism underlying GABA’s excitatory role in immature brain. J. Neurobiol. 33, 781–795.
Praetorius, J., Nejsum, L. N., and Nielsen, S. (2004). A SCL4A10 gene product maps selectively to the basolateral plasma membrane of choroid plexus epithelial cells. Am. J. Physiol. Cell Physiol. 286, C601–C610.
Praetorius, J., and Nielsen, S. (2006). Distribution of sodium transporters and aquaporin-1 in the human choroid plexus. Am. J. Physiol. Cell Physiol. 291, C59–C67.
Prohaska, J. R., Broderius, M., and Brokate, B. (2003). Metallochaperone for Cu,Zn-superoxide dismutase (CCS) protein but not mRNA is higher in organs from copper-deficient mice and rats. Arch. Biochem. Biophys. 417, 227–234.
Prpic, V., Blackmore, P. F., and Exton, J. H. (1982). myo-Inositol uptake and metabolism in isolated rat liver cells. J. Biol. Chem. 257, 11315–11322.
Ramaekers, V. T., Hansen, S. I., Holm, J., Opladen, T., Senderek, J., Hausler, M., Heimann, G., Fowler, B., Maiwald, R., and Blau, N. (2003). Reduced folate transport to the CNS in female Rett patients. Neurology 61, 506–515.
Ramaekers, V. T., Hausler, M., Opladen, T., Heimann, G., and Blau, N. (2002). Psychomotor retardation, spastic paraplegia, cerebellar ataxia and dyskinesia associated with low 5-methyltetrahydrofolate in cerebrospinal fluid: a novel neurometabolic condition responding to folinic acid substitution. Neuropediatrics 33, 301–308.
Rebec, G. V., and Pierce, R. C. (1994). A vitamin as neuromodulator: ascorbate release into the extracellular fluid of the brain regulates dopaminergic and glutamatergic transmission. Prog. Neurobiol. 43, 537–565.
Redzic, Z. (2011). Molecular biology of the blood-brain and the blood-cerebrospinal fluid barriers: similarities and differences. Fluids Barriers CNS 8, 3.
Redzic, Z. B., Preston, J. E., Duncan, J. A., Chodobski, A., and Szmydynger-Chodobska, J. (2005). The choroid plexus-cerebrospinal fluid system: from development to aging. Curr. Top. Dev. Biol. 71, 1–52.
Redzic, Z. B., and Segal, M. B. (2004). The structure of the choroid plexus and the physiology of the choroid plexus epithelium. Adv. Drug Deliv. Rev. 56, 1695–1716.
Reiber, H., Ruff, M., and Uhr, M. (1993). Ascorbate concentration in human cerebrospinal fluid (CSF) and serum. Intrathecal accumulation and CSF flow rate. Clin. Chim. Acta 217, 163–173.
Rice, M. E. (2000). Ascorbate regulation and its neuroprotective role in the brain. Trends Neurosci. 23, 209–216.
Rindi, G., and Laforenza, U. (2000). Thiamine intestinal transport and related issues: recent aspects. Proc. Soc. Exp. Biol. Med. 224, 246–255.
Ringel, F., Chang, R. C., Staub, F., Baethmann, A., and Plesnila, N. (2000). Contribution of anion transporters to the acidosis-induced swelling and intracellular acidification of glial cells. J. Neurochem. 75, 125–132.
Roberts, L. M., Woodford, K., Zhou, M., Black, D. S., Haggerty, J. E., Tate, E. H., Grindstaff, K. K., Mengesha, W., Raman, C., and Zerangue, N. (2008). Expression of the thyroid hormone transporters monocarboxylate transporter-8 (SLC16A2) and organic ion transporter-14 (SLCO1C1) at the blood-brain barrier. Endocrinology 149, 6251–6261.
Rogers, S., Chandler, J. D., Clarke, A. L., Petrou, S., and Best, J. D. (2003). Glucose transporter GLUT12-functional characterization in Xenopus laevis oocytes. Biochem. Biophys. Res. Commun. 308, 422–426.
Rogers, S., Macheda, M. L., Docherty, S. E., Carty, M. D., Henderson, M. A., Soeller, W. C., Gibbs, E. M., James, D. E., and Best, J. D. (2002). Identification of a novel glucose transporter-like protein-GLUT-12. Am. J. Physiol. Endocrinol. Metab. 282, E733–E738.
Saito, Y., and Wright, E. M. (1983). Bicarbonate transport across the frog choroid plexus and its control by cyclic nucleotides. J. Physiol. (Lond.) 336, 635–648.
Salmaso, S., Pappalardo, J. S., Sawant, R. R., Musacchio, T., Rockwell, K., Caliceti, P., and Torchilin, V. P. (2009). Targeting glioma cells in vitro with ascorbate-conjugated pharmaceutical nanocarriers. Bioconjug. Chem. 20, 2348–2355.
Sasaki, N. (2011). Current status and future prospects for research on tyrosine sulfation. Curr. Pharm. Biotechnol. PMID: 22039814. [Epub ahead of print].
Segal, M. B. (2000). The choroid plexuses and the barriers between the blood and the cerebrospinal fluid. Cell. Mol. Neurobiol. 20, 183–196.
Sensi, S. L., Paoletti, P., Bush, A. I., and Sekler, I. (2009). Zinc in the physiology and pathology of the CNS. Nat. Rev. Neurosci. 10, 780–791.
Shepherd, P. R., Gould, G. W., Colville, C. A., McCoid, S. C., Gibbs, E. M., and Kahn, B. B. (1992). Distribution of GLUT3 glucose transporter protein in human tissues. Biochem. Biophys. Res. Commun. 188, 149–154.
Shetty, H. U., Schapiro, M. B., Holloway, H. W., and Rapoport, S. I. (1995). Polyol profiles in Down syndrome. myo-Inositol, specifically, is elevated in the cerebrospinal fluid. J. Clin. Invest. 95, 542–546.
Smith, D. E., Hu, Y., Shen, H., Nagaraja, T. N., Fenstermacher, J. D., and Keep, R. F. (2011). Distribution of glycylsarcosine and cefadroxil among cerebrospinal fluid, choroid plexus, and brain parenchyma after intracerebroventricular injection is markedly different between wild-type and Pept2 null mice. J. Cereb. Blood Flow Metab. 31, 250–261.
Smith, D. E., Johanson, C. E., and Keep, R. F. (2004). Peptide and peptide analog transport systems at the blood-CSF barrier. Adv. Drug Deliv. Rev. 56, 1765–1791.
Southwell, B. R., Duan, W., Alcorn, D., Brack, C., Richardson, S. J., Kohrle, J., and Schreiber, G. (1993). Thyroxine transport to the brain: role of protein synthesis by the choroid plexus. Endocrinology 133, 2116–2126.
Speake, T., Freeman, L. J., and Brown, P. D. (2003). Expression of aquaporin 1 and aquaporin 4 water channels in rat choroid plexus. Biochim. Biophys. Acta 1609, 80–86.
Spector, R. (1981). Penetration of ascorbic acid from cerebrospinal fluid into brain. Exp. Neurol. 72, 645–653.
Spector, R. (2009). Nutrient transport systems in brain: 40 years of progress. J. Neurochem. 111, 315–320.
Spector, R., and Johanson, C. (2006). Micronutrient and urate transport in choroid plexus and kidney: implications for drug therapy. Pharm. Res. 23, 2515–2524.
Spector, R., and Johanson, C. E. (2007). Vitamin transport and homeostasis in mammalian brain: focus on vitamins B and E. J. Neurochem. 103, 425–438.
Spector, R., and Johanson, C. E. (2010). Vectorial ligand transport through mammalian choroid plexus. Pharm. Res. 27, 2054–2062.
Spector, R., and Lorenzo, A. V. (1975). Myo-inositol transport in the central nervous system. Am. J. Physiol. 228, 1510–1518.
Spolarics, Z., Lang, C. H., Bagby, G. J., and Spitzer, J. J. (1991). Glutamine and fatty acid oxidation are the main sources of energy for Kupffer and endothelial cells. Am. J. Physiol. 261, G185–G190.
Stahl, A., Evans, J. G., Pattel, S., Hirsch, D., and Lodish, H. F. (2002). Insulin causes fatty acid transport protein translocation and enhanced fatty acid uptake in adipocytes. Dev. Cell 2, 477–488.
Storch, A., Hwang, Y. I., Gearhart, D. A., Beach, J. W., Neafsey, E. J., Collins, M. A., and Schwarz, J. (2004). Dopamine transporter-mediated cytotoxicity of beta-carbolinium derivatives related to Parkinson’s disease: relationship to transporter-dependent uptake. J. Neurochem. 89, 685–694.
Sugiyama, D., Kusuhara, H., Taniguchi, H., Ishikawa, S., Nozaki, Y., Aburatani, H., and Sugiyama, Y. (2003). Functional characterization of rat brain-specific organic anion transporter (Oatp14) at the blood-brain barrier: high affinity transporter for thyroxine. J. Biol. Chem. 278, 43489–43495.
Suh, S. W., Chen, J. W., Motamedi, M., Bell, B., Listiak, K., Pons, N. F., Danscher, G., and Frederickson, C. J. (2000a). Evidence that synaptically-released zinc contributes to neuronal injury after traumatic brain injury. Brain Res. 852, 268–273.
Suh, S. W., Jensen, K. B., Jensen, M. S., Silva, D. S., Kesslak, P. J., Danscher, G., and Frederickson, C. J. (2000b). Histochemically-reactive zinc in amyloid plaques, angiopathy, and degenerating neurons of Alzheimer’s diseased brains. Brain Res. 852, 274–278.
Sunkin, S. M., and Hohmann, J. G. (2007). Insights from spatially mapped gene expression in the mouse brain. Hum. Mol. Genet. 16, R209–R219.
Takanaga, H., Mackenzie, B., Suzuki, Y., and Hediger, M. A. (2005). Identification of mammalian proline transporter SIT1 (SLC6A20) with characteristics of classical system imino. J. Biol. Chem. 280, 8974–8984.
Takeda, A. (2000). Movement of zinc and its functional significance in the brain. Brain Res. Brain Res. Rev. 34, 137–148.
Tallaksen, C. M., Bohmer, T., and Bell, H. (1992). Concentrations of the water-soluble vitamins thiamin, ascorbic acid, and folic acid in serum and cerebrospinal fluid of healthy individuals. Am. J. Clin. Nutr. 56, 559–564.
Tallaksen, C. M., Bohmer, T., Karlsen, J., and Bell, H. (1997). Determination of thiamin and its phosphate esters in human blood, plasma, and urine. Meth. Enzymol. 279, 67–74.
Tohyama, K., Kusuhara, H., and Sugiyama, Y. (2004). Involvement of multispecific organic anion transporter, Oatp14 (Slc21a14), in the transport of thyroxine across the blood-brain barrier. Endocrinology 145, 4384–4391.
Torrents, D., Estevez, R., Pineda, M., Fernandez, E., Lloberas, J., Shi, Y. B., Zorzano, A., and Palacin, M. (1998). Identification and characterization of a membrane protein (y+L amino acid transporter-1) that associates with 4F2hc to encode the amino acid transport activity y+L. A candidate gene for lysinuric protein intolerance. J. Biol. Chem. 273, 32437–32445.
Trajkovic, M., Visser, T. J., Mittag, J., Horn, S., Lukas, J., Darras, V. M., Raivich, G., Bauer, K., and Heuer, H. (2007). Abnormal thyroid hormone metabolism in mice lacking the monocarboxylate transporter 8. J. Clin. Invest. 117, 627–635.
Tsukaguchi, H., Tokui, T., Mackenzie, B., Berger, U. V., Chen, X. Z., Wang, Y., Brubaker, R. F., and Hediger, M. A. (1999). A family of mammalian Na+-dependent L-ascorbic acid transporters. Nature 399, 70–75.
Vallee, B. L., and Falchuk, K. H. (1993). The biochemical basis of zinc physiology. Physiol. Rev. 73, 79–118.
van der Deure, W. M., Hansen, P. S., Peeters, R. P., Kyvik, K. O., Friesema, E. C., Hegedus, L., and Visser, T. J. (2008). Thyroid hormone transport and metabolism by organic anion transporter 1C1 and consequences of genetic variation. Endocrinology 149, 5307–5314.
Varatharajan, L., and Thomas, S. A. (2009). The transport of anti-HIV drugs across blood-CNS interfaces: summary of current knowledge and recommendations for further research. Antiviral Res. 82, A99–A109.
Vialou, V., Balasse, L., Dumas, S., Giros, B., and Gautron, S. (2007). Neurochemical characterization of pathways expressing plasma membrane monoamine transporter in the rat brain. Neuroscience 144, 616–622.
Vincourt, J. B., Jullien, D., Amalric, F., and Girard, J. P. (2003). Molecular and functional characterization of SLC26A11, a sodium-independent sulfate transporter from high endothelial venules. FASEB J. 17, 890–892.
Vogel, P., Read, R. W., Vance, R. B., Platt, K. A., Troughton, K., and Rice, D. S. (2008). Ocular albinism and hypopigmentation defects in Slc24a5−/− mice. Vet. Pathol. 45, 264–279.
Wagner, C. A., Lang, F., and Broer, S. (2001). Function and structure of heterodimeric amino acid transporters. Am. J. Physiol. Cell Physiol. 281, C1077–C1093.
Wang, C. Z., Yano, H., Nagashima, K., and Seino, S. (2000). The Na+-driven Cl-/HCO3-exchanger. Cloning, tissue distribution, and functional characterization. J. Biol. Chem. 275, 35486–35490.
Wang, Y., Zhao, R., Russell, R. G., and Goldman, I. D. (2001). Localization of the murine reduced folate carrier as assessed by immunohistochemical analysis. Biochim. Biophys. Acta 1513, 49–54.
Weitman, S. D., Weinberg, A. G., Coney, L. R., Zurawski, V. R., Jennings, D. S., and Kamen, B. A. (1992). Cellular localization of the folate receptor: potential role in drug toxicity and folate homeostasis. Cancer Res. 52, 6708–6711.
Wevers, R. A., Hansen, S. I., van Hellenberg Hubar, J. L., Holm, J., Hoier-Madsen, M., and Jongen, P. J. (1994). Folate deficiency in cerebrospinal fluid associated with a defect in folate binding protein in the central nervous system. J. Neurol. Neurosurg. Psychiatr. 57, 223–226.
Wu, Q., Delpire, E., Hebert, S. C., and Strange, K. (1998). Functional demonstration of Na+-K+-2Cl-cotransporter activity in isolated, polarized choroid plexus cells. Am. J. Physiol. 275, C1565–C1572.
Xia, L., Engel, K., Zhou, M., and Wang, J. (2007). Membrane localization and pH-dependent transport of a newly cloned organic cation transporter (PMAT) in kidney cells. Am. J. Physiol. Renal Physiol. 292, F682–F690.
Xia, L., Zhou, M., Kalhorn, T. F., Ho, H. T., and Wang, J. (2009). Podocyte-specific expression of organic cation transporter PMAT: implication in puromycin aminonucleoside nephrotoxicity. Am. J. Physiol. Renal Physiol. 296, F1307–F1313.
Xiang, J., Ennis, S. R., Abdelkarim, G. E., Fujisawa, M., Kawai, N., and Keep, R. F. (2003). Glutamine transport at the blood-brain and blood-cerebrospinal fluid barriers. Neurochem. Int. 43, 279–288.
Xiang, J., Fowkes, R. L., and Keep, R. F. (1998). Choroid plexus histidine transport. Brain Res. 783, 37–43.
Yamashita, T., Tamatani, M., Taniguchi, M., Takagi, T., Yoshimine, T., and Tohyama, M. (1998). Regulation of Na+/myo-inositol cotransporter gene expression in hyperglycemic rat hippocampus. Brain Res. Mol. Brain Res. 57, 167–172.
Zhang, H., Song, Y. N., Liu, W. G., Guo, X. L., and Yu, L. G. (2010). Regulation and role of organic anion-transporting polypeptides (OATPs) in drug delivery at the choroid plexus. J. Clin. Neurosci. 17, 679–684.
Zhao, R., Gao, F., and Goldman, I. D. (2002). Reduced folate carrier transports thiamine monophosphate: an alternative route for thiamine delivery into mammalian cells. Am. J. Physiol. Cell Physiol. 282, C1512–C1517.
Zhao, R., Gao, F., Wang, Y., Diaz, G. A., Gelb, B. D., and Goldman, I. D. (2001a). Impact of the reduced folate carrier on the accumulation of active thiamin metabolites in murine leukemia cells. J. Biol. Chem. 276, 1114–1118.
Zhao, R., Russell, R. G., Wang, Y., Liu, L., Gao, F., Kneitz, B., Edelmann, W., and Goldman, I. D. (2001b). Rescue of embryonic lethality in reduced folate carrier-deficient mice by maternal folic acid supplementation reveals early neonatal failure of hematopoietic organs. J. Biol. Chem. 276, 10224–10228.
Zhou, M., Xia, L., and Wang, J. (2007). Metformin transport by a newly cloned proton-stimulated organic cation transporter (plasma membrane monoamine transporter) expressed in human intestine. Drug Metab. Dispos. 35, 1956–1962.
Keywords: choroid plexus, blood-cerebrospinal fluid barrier, BCSFB, solute carriers, CSF, transporters, Slc gene, Allen Brain Atlas
Citation: Ho HTB, Dahlin A and Wang J (2012) Expression profiling of solute carrier gene families at the blood-CSF barrier. Front. Pharmacol. 3:154. doi: 10.3389/fphar.2012.00154
Received: 16 May 2012; Accepted: 01 August 2012;
Published online: 24 August 2012.
Edited by:
Joana A. Palha, University of Minho, PortugalReviewed by:
Ken-ichi Hosoya, University of Toyama, JapanJason B. Wu, Cedars-Sinai Medical Center, USA
Helle Hasager Damkier, Aarhus University, Denmark
Copyright: © 2012 Ho, Dahlin and Wang. This is an open-access article distributed under the terms of the Creative Commons Attribution License, which permits use, distribution and reproduction in other forums, provided the original authors and source are credited and subject to any copyright notices concerning any third-party graphics etc.
*Correspondence: Joanne Wang, Department of Pharmaceutics, University of Washington, Box 357610, 1959 NE Pacific Street, Seattle, WA 98195, USA. e-mail:am93YW5nQHUud2FzaGluZ3Rvbi5lZHU=
†Present address: Amber Dahlin, Channing Division of Network Medicine, Brigham and Women’s Hospital and Harvard Medical School, Boston, MA, USA.