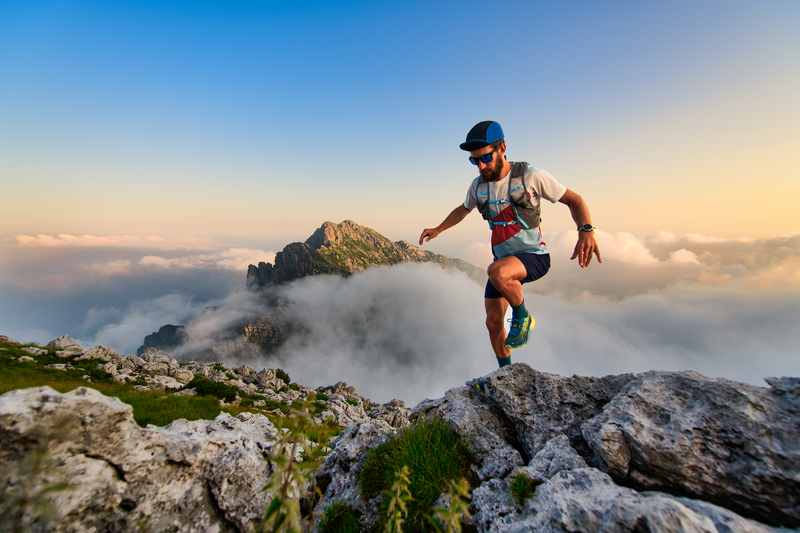
95% of researchers rate our articles as excellent or good
Learn more about the work of our research integrity team to safeguard the quality of each article we publish.
Find out more
REVIEW article
Front. Pediatr. , 10 June 2024
Sec. Pediatric Neurology
Volume 12 - 2024 | https://doi.org/10.3389/fped.2024.1400468
This article is part of the Research Topic Recent Advances in Pediatric Neuroradiology View all 9 articles
Attention deficit hyperactivity disorder (ADHD) is the most common neurodevelopmental disorder in children, characterized by age-inappropriate inattention, hyperactivity, and impulsivity, which can cause extensive damage to children's academic, occupational, and social skills. This review will present current advancements in the field of attention deficit hyperactivity disorder, including genetics, environmental factors, epigenetics, and neuroimaging features. Simultaneously, we will discuss the highlights of promising directions for further study.
Attention deficit hyperactivity disorder (ADHD) is a prevalent neurodevelopmental disorder, characterized by extensive hyperactive, impulsive and/or inattentive behaviors that impair daily functioning. Notably, it is estimated the global prevalence of ADHD as 7.2% (1, 2). And in China specifically, ADHD affected around 6.4% of children (3). Long-lasting clinical symptoms are present in between 50% and 60% of ADHD patients, frequently coexisting with additional disorders, namely anxiety, oppositional defiant disorder, and tic disorders. These conditions can increase the risk of suicide and delinquency and have a major negative influence on families and society (4, 5). The complicated etiology of ADHD is frequently attributed to a confluence of hereditary and environmental variables (6, 7). Research has demonstrated that structural abnormalities in the brain, such as decreased brain volume and cortical surface area in ADHD, can cause disturbances in brain function, which can result in executive dysfunction and a variety of clinical symptoms, including impulsivity, hyperactivity, and inattention (7, 8).
Although the exact cause of ADHD remains unidentified, most researchers contend that it is a result of a combination of hereditary and environmental factors. Besides, ADHD has a comparatively variable clinical presentation, by thoroughly examining its various etiology and pathogenesis, new treatment approaches can be developed and researched more easily.
The development of ADHD is largely influenced by genetic factors, and investigations involving twins and family lines have demonstrated a distinct familial clustering in the disorder's development. Additionally, research conducted by Uchida et al. (9) found that children whose parents had ADHD were more likely than their non-ADHD parents to experience ADHD and other cognitive and psychiatric disorders. Faraone et al. demonstrated that the heritability of ADHD in twins is approximately 74% (10). Through a genome-wide association study (GWAS) of ADHD, Demontis et al. (11) presented a significant research paper in 2019 which achieved substantial progress in identifying genetic risk factors and refining the genetic architecture of the disease. The research revealed 12 independent genome-wide significant loci associated with ADHD by combining a genome-wide association meta-analysis of ADHD risk genes with data from the Danish Integrative Psychiatric Research (iPSYCH) and 11 ADHD cohorts from various nations that were gathered by the Psychiatric Genomics Consortium (PGC), totaling 20,183 ADHD patients and 35,191 controls.
Primarily, the identified risk genes are clustered in the cerebral cortex and are associated with early brain development, principally involving several brain-specific neuronal subtypes and midbrain dopaminergic neurons. The risk gene loci are linked to neurodevelopment, neurotransmitter transmission, and regulation of gene expression. The aforementioned study provides compelling evidence that ADHD is a polygenic disorder, with several risk genes working together to determine the development of the condition and varying degrees of illness risk associated with each gene. In 2023, Demontis et al. identified 27 risk loci, comprising both the common loci from the 2019 analysis and recently found rarer genetic loci. Simultaneously, after examining the expression of ADHD risk genes in different tissues and cell types, the researchers discovered that many of these genes were enriched in excitatory and inhibitory brain neuronal cell types. Additionally, it was demonstrated that genes expressed in dopaminergic mesencephalic neurons and genes related to ADHD were significantly correlated (P = 0.005) based on the results of single-cell RNA sequencing data and cell type-specific analysis (12).
Furthermore, the ongoing advancement of research on disease risk gene loci (13) will gradually lead to the genetic elucidation of the effects of risk genes on the development of particular neuronal subtypes and brain network connections, as well as the further clarification of the connection between attention-deficit, hyperactivity, and impulsive behaviors in children with ADHD and abnormalities in brain development. Based on prior research, an estimated 300 candidate genes have been linked to the onset of ADHD. These genes are primarily found in midbrain interneurons. We will then describe in detail three common interneurons including dopaminergic neurons, noradrenergic neurons, and 5-hydroxytryptamine (5-HT) neurons.
Dopamine receptor D4 (DRD4) is the most studied candidate gene associated with increased risk of ADHD, DRD4 receptor regulates dopamine signaling in the CNS and plays important roles in attention, reward, and motivation. Chang et al. (14) found that children with the DRD4 GG genotype were more likely to experience ADHD than children with the DRD4 GA/AA. Notably, variable Number Tandem Repeats (VNTR) is a repeated section of the DNA sequence that is the main target of variations in the DRD4 gene. The 7 repeat (7R) and the 4 repeat (4R) are two of the most prevalent variation types. Research has identified a correlation between the 7R variation and a higher likelihood of developing ADHD. According to some research, those who have the DRD4 7R variation may be more prone to ADHD in specific groups. However, the results are inconsistent, as the association's intensity and direction changed based on populations and research. Thus, the DRD4 gene's connection to ADHD remains debatable, and other genetic and environmental variables may influence how the gene functions. The relationship between DRD5 gene variations and ADHD has not been researched adequately. Despite this, Tong et al. (15) have suggested that some variations in the DRD5 gene may be associated with an increased chance of developing ADHD. Whereas other research has suggested that the DRD5 gene and ADHD do not appear to be correlated. Therefore, to validate the DRD5 gene's involvement in the pathogenesis of ADHD, additional research is required.
An increased risk of ADHD has also been linked to variations in dopamine transporter genes, such as those in the dopamine transporter 1 (DAT1, also known as SLC6A3) gene. One of the most extensively researched potential genes in the pathophysiology of childhood ADHD is DAT1 (16). Research has indicated that the 10R of the 40 bp VNTR in the 3′ untranslated region (3′UTR) of DAT1 is strongly correlated with clinical symptoms in ADHD children, particularly those with attention deficits and that DAT1 haplotypes comprising the 10R of the 40 bp VNTR of the 3′UTR/the 6R of the 30 bp VNTR of the intron 8 are strongly correlated with cognitive impairments in ADHD. The 10R/10R genotype of DAT1 VNTR is related to ADHD in Korean children (17). Study in Jordan children showed that the 10R allele of DAT1 was associated with ADHD in the children (18). On the other hand, conflicting results were reported in the studies in the Omani children, Han Chinese children, Iranian population and Turkish population which showed no association significant between VNTR polymorphism with ADHD (19–22). Meanwhile, it has been reported that there is no association between VNTR polymorphism of DAT1 genes and ADHD among Indonesian children based on a case-control study (23). In 2020, Kuc et al. also found that the SLC6A3 gene polymorphism was not associated with the presence of ADHD (24).
The frontal orbital-striatal pathway is regulated by 5-hydroxytryptamine (5-HT), and 5-hydroxytryptamine receptors (5-HTR) are mainly located in the brain's prefrontal cortex, amygdala, and hippocampus (25). Deviations from normalcy in these brain regions have a substantial impact on children with ADHD's hyperactivity, impulsivity and attention span. The presence of two crucial 5-HT receptors, specifically 5-hydroxytryptamine 1A and 2A receptors, have been identified as closely related to the behaviors associated with ADHD (26). Additionally, the 5-hydroxytryptamine transporter (SERT), 5-HTR 1B and 5-HTR 2A genes are involved in the pathophysiology of ADHD (27). The 5-hydroxytryptamine transporter gene (SLC6A4/5-HTT) encodes the 5-hydroxytryptamine transporter (SERT) and regulates the effectiveness of 5-HT. This gene promoter region (5-HTTLPR) is associated with ADHD pathogenesis as the long allele (L) and the short allele (S). Children with ADHD exhibit more evident behavioral issues and hyperactivity when they have the “S” allele and the “S/S” genotype, and a greater degree of executive function impairment when they have the “L” allele (28).
The norepinephrine transporter (NET, SLC6A2) was discovered to reuptake sympathetically released norepinephrine (NE) into the presynaptic membrane via active transport. Since norepinephrine transporter antagonists can affect the effectiveness of pharmacological treatment in children with ADHD, the norepinephrine transporter gene has emerged as the most extensively researched noradrenergic system gene. According to Shang et al. (29), children with ADHD can have their intrinsic brain activity, attention, and visual memory regulated by the norepinephrine transporter gene. Shirama et al. (30) concluded that NE modulation of serum concentrations and gene interactions alter alertness in ADHD patients, leading to an increased likelihood of dangerous behaviors in ADHD patients. The findings of Hawi et al. (31) revealed a high correlation between the development of ADHD and SLC6A2. Wang et al. (32) found that the α-2A adrenergic receptor (ADRA2A) gene is also a candidate gene, and children with the ADRA2A rs553668 GG/GA genotype were more likely to develop ADHD than those with the ADRA2A rs553668 AA genotype.
Environmental risk factors, which mostly include psychosocial variables, pregnancy, and perinatal risk factors, are directly linked to the development of ADHD.
The development of children with ADHD has been discovered to be influenced by perinatal circumstances, environmental variables, intrauterine factors, and maternal self-factors throughout pregnancy. For example, children are more likely to acquire ADHD if their mothers smoke, drink excessively, or are exposed to air pollutants (polycyclic aromatic hydrocarbons), or field nonionizing nonionizing radiation when they are pregnant (33–36). Acetaminophen exposure during pregnancy has been reported to be highly related to a high incidence of ADHD in children (37). There have also been reports of possible risk factors for ADHD in children, including maternal pre-pregnancy overweight or obesity, severe mental illness in the parents, hypothyroxinemia, depression, and gestational diabetes mellitus (38–43).
Psychosocial factors primarily include parental mental health, marital harmony, parental relationships, and family education methods. However, the impact of the natural physical environment, including the children's living conditions and family's financial status on the onset of symptoms and overall course of ADHD patients should not be discounted. For instance, Nilsen et al. (44) discovered that children who are exposed to cigarette smoke may be more likely to experience symptoms of ADHD. The financial status of a family may also effect a child's likelihood of developing ADHD (45). Low family income has often been linked to an elevated risk of ADHD in children within research (46, 47). Meanwhile, the development of ADHD can also be influenced by parental mental health, marital status, and parental relationship status. A study (48) confirmed that mothers of ADHD children exhibit more pronounced symptoms of anxiety and depression than their fathers. Furthermore, children with ADHD are frequently exposed to inappropriate educational techniques for extended periods, which makes them increasingly likely to exhibit abnormal behavioral patterns.
Scholars have studied the mechanisms by which environmental factors contribute to the development of ADHD in children. It was found that children whose mothers smoked during pregnancy had reduced volumes of cortical gray matter, cerebellum, and corpus callosum, and thinning of frontal, temporal, and parietal regions, along with alterations in the white matter microstructure of several major connective bundles. Importantly these alterations in brain regions have also been associated with deficits in cognitive performance, auditory processing, social development and ADHD. At the same time, studies have shown that adolescents whose mothers smoked during pregnancy showed inefficient recruitment of relevant brain regions (including the temporal lobe, hippocampus and cerebellum) during response inhibition, attention and memory tasks (49). Prenatal alcohol-exposed children have reduced brain volumes and abnormalities in the frontal, parietal, and temporal lobes in terms of volume, gray matter density, shape, and cortical thickness (50), which have been associated with attention deficits and impulsive behavior (51).
Peterson et al. applied magnetic resonance imaging studies have found that prenatal exposure to PAH air pollutants disrupts the development of white matter in the left hemisphere of children, particularly in the frontal, parietal, and temporal lobes, which can lead to ADHD symptoms and externalizing problems since this white matter contributes to attention and impulse control (33). Non-clinical studies have shown evidence of various potential mechanisms for the deleterious effects of acetaminophen on neurodevelopment. Blecharz-Klin et al. (52) found that rats receiving therapeutic doses of acetaminophen significantly modulated neurotransmission in brain structures (prefrontal cortex, hypothalamus, and striatum) associated with behavior and working memory.
The contribution of maternal overweight/obesity to unfavorable brain development is partly influenced by inflammatory phenomena. Thus, inflammation may play a role in the association between maternal overweight/obesity and ADHD in children and adolescents. Obese pregnant women have higher circulating levels of pro-inflammatory cytokines than normal-weight pregnant women, and the normal developmental trajectory of the fetal brain may be interrupted by exposure to infections and high levels of pro-inflammatory cytokines, with long-lasting or persistent consequences for gray matter volume and white matter integrity (53, 54). This leaves the fetus vulnerable to psychiatric complications, and thus ADHD is associated with elevated levels of inflammatory cytokines (55, 56).
Recent data from the existing literature suggests that gestational diabetes mellitus (GDM) promotes altered structural brain morphology in the offspring. Van Dam et al. found reduced neuronal excitability and neuronal plasticity in children whose mothers had GDM, suggesting that GDM may lead to central nervous system dysfunction, which may be further associated with impaired cognitive and motor outcomes (57). In a recent study, researchers used diffusion tension imaging studies to show that infants of mothers with GDM exhibited microstructural white matter abnormalities associated with impaired neurocognitive abilities (58). Lynch et al. found that reduced hippocampal volume in specific subregions of children exposed to GDM in utero is accompanied by specific alterations in hippocampal morphology (59). In another recent study, Ahmed et al. observed reduced cortical thickness in multiple brain structures and poorer overall cognitive performance in diabetes-exposed offspring (60).
In the field of genetics, epigenetics focuses on gene expression and functional regulation rather than alterations to the DNA sequence. The most popular topics of study in epigenetics include DNA methylation, histone modification and non-coding RNA (61). Neurodevelopmental disorders are diseases in which epigenetics plays a prominent role.
DNA methylation is one of the most researched types of modification in the field of epigenetics. By adding methyl groups to the DNA bases, it controls the expression of genes. Research has revealed that the DRD4 gene exhibits evidence of methylation, with varying levels of methylation among individuals diagnosed with ADHD (61). Moreover, variations in the DNA methylation of genes associated with the dopamine signaling pathway may result in modified expression levels of the relevant genes, which could impact dopamine signaling and neurodevelopment, ultimately linking them to the pathogenesis of ADHD (62, 63). In the methylome analysis of salivary DNA from children with ADHD, Wilmot et al. (64) discovered altered DNA methylation of the vasoactive intestinal peptide receptor 2 (VIPR2). Additionally, methylation of cytosine-phosphate-guanine (CpG), a crucial location upstream of DRD4, plays a significant part in dopamine's ability to regulate. Meanwhile, Wilmot also demonstrated that male children with ADHD had higher levels of CpG methylation in their peripheral tissues, indicating that DNA methylation markers in these children's peripheral tissues may be useful for research in the future. While the study by Walton et al. (65) did not find evidence of differential methylation of DRD4 and VIPR2, it discovered that potential symptomatic changes in children with ADHD have been distinguished by DNA methylation at birth at multiple genomic locations.
Additionally, early-life methylation patterns in the peroxisome network may impair the production of docosahexaenoic acid (DHA), which may contribute to the symptoms of ADHD in children and adolescents. Chen et al. (66) studied twin children with ADHD and discovered several different methylated genes between ADHD and non-ADHD siblings. In contrast to the Wilmot result of hypomethylation of the VIPR2 gene, differential methylation of the VIPR2 gene was also discovered, with hypermethylation in three afflicted twins.
Another significant epigenetic mechanism that alters the modification marks on histone proteins to control gene expression is histone modification. Common histone modifications linked to gene activation and expression include acetylation, methylation and phosphorylation. They play an important role in different nuclear processes, such as replication, DNA repair, transcription, and chromatin structure stabilization (67). However, relatively little work has been done on the role of histone modifications in the pathogenesis of ADHD. Xu et al. (62) studied blood samples from Chinese Han children and found increased expression of histone deacetylase 1 (HDAC1) in children with ADHD compared to healthy controls, suggesting that protein acetylation is reduced in the group of children with ADHD. Histone methylation and acetylation abnormalities, can all result in aberrant gene expression, which can then influence neurodevelopment, synaptic function, and dopamine signaling, impacting the pathogenesis of ADHD.
Non-coding RNAs are also implicated in the pathophysiology of ADHD, in addition to DNA methylation and histone modification. The expression and function of genes can be controlled by these non-coding RNA molecules (68). Notably, individuals with ADHD may have different levels of several non-coding RNAs' expression. These non-coding RNAs have the potential to impact neurodevelopment, synaptic function, and dopamine signaling pathways through controlling the expression of particular genes. Srivastav et al. (69) observed that peripheral microRNA concentrations were different in both animal models and children with ADHD. In 2023, Dypås et al. discovered 32 microRNAs that were strongly linked to features related to ADHD, including hyperactivity (29) and inattention (3) (70).
Comorbidity refers to the presence of more than one disease diagnosis in a patient, and ADHD is frequently comorbid with other disorders, both psychiatric disorders and non-mental diseases, with up to 70%–80% of individuals with ADHD experiencing concomitant psychiatric disorders throughout their lives (71), including oppositional defiant disorder (ODD), conduct disorder (CD), major depressive disorder (MDD), bipolar disorder (BD), anxiety disorders (AD), and substance use disorders (SUD). In addition, ADHD is highly comorbid with other neurodevelopmental disorders, such as autism spectrum disorders (ASD), learning disabilities (LD), and tic disorders (TD). According to recent research, non-mental disorders are very common in individuals with ADHD and greatly lower quality of life. Research on non-mental diseases that co-occur with ADHD has demonstrated a strong correlation with obesity, diabetes mellitus, sleep disorders, epilepsy, and allergic diseases (72–74). Comorbidities frequently cause children with ADHD to have significantly impaired social functioning and complicate their clinical presentation, diagnosis, and course of treatment. For this reason, it is critical to understand why comorbidity between ADHD and other diseases is so common.
Studies have revealed a biological explanation for the co-occurrence of ODD in children with ADHD. This includes a shared genetic basis involving the genes encoding androgen receptors and adrenal hormones. Additionally, it has been proposed that there is a strong correlation between serum 5-hydroxytryptamine concentrations and aggressive behavior in individuals. Furthermore, Noordermeer et al. demonstrated that children with comorbid ODD and ADHD displayed genetic variations in working memory, facial expression detection, and temporal processing, indicating neurocognitive impairment (75). According to an etiological study of children with co-occurring CD and ADHD, there may be hereditary and environmental factors that contribute to both conditions (76). The persistent weight of linked emotional and cognitive features in people with ADHD, as well as the association between psychosocial and functional stressors in daily life, may be the cause of ADHD and mood disorders and the prevalence of comorbid anxiety and SUD (77–79). Moreover, rather than being the result of exposure to these exposures, mental comorbidity may also be a direct expression of common genetic variables between ADHD, comorbid conditions, and related emotional, cognitive, and behavioral features. Fraporti et al. (80) reported that the interaction between the ADORA2A gene and the DRD2 gene affects anxiety disorders in children with ADHD. Demontis et al. discovered an association between anxiety disorders and the DRD2 gene in children with ADHD. A GWAS meta-analysis of ADHD revealed that obesity, insomnia, ASD, schizophrenia, MDD, and cannabis use disorders have significant genetic correlations with ADHD, and there is genetic overlap between them (74). Therefore, a major contributing factor to the explanation of the correlation between co-occurring features and comorbid psychiatric disorders and ADHD is genetics.
One of the most widely studied co-occurring nonmental diseases in ADHD patients is epilepsy. The prevalence of epilepsy ranges from 0.5% to 0.9% in children worldwide (81), and it is often comorbid with other psychiatric disorders, such as ASD, ADHD, and AD, with ADHD being the most common. Some researchers have also suggested that the relationship between epilepsy and ADHD is bidirectional (82) and that ADHD may contribute to an elevated risk of seizures, while chronic recurrent abnormal discharges in children with epilepsy may exacerbate symptoms of ADHD, such as poor attentional control and impulsive and hyperactive behavior. The pathogenesis of epilepsy co-occurring with ADHD is often caused by a combination of factors and may include related mechanisms such as structural brain abnormalities, genetic factors, and neurobiochemical mechanisms.
In terms of brain structure, Karalok et al. (83) reported that both children with benign childhood epilepsy with centrotemporal spikes (BECTs) and children with BECTs with comorbid ADHD showed cortical structure abnormalities (cortical area thinning), but comorbid ADHD in children with epilepsy was more strongly associated with cortical area thinning. In terms of genetic factors, the genotypic association between ADHD and epilepsy is extremely high, and this correlation can be explained by the influence of family aggregation, mainly on the maternal side, as well as by individual-specific environmental factors (84). A number of studies have suggested that epilepsy comorbid with ADHD may be due to the presence of common genetic abnormalities between the two, mainly in the IQSec2 gene, the SLC6A1 gene, the SLC9A9 gene, the Dlg4 gene, and the Vamp2 gene (85–87). In terms of neurobiochemistry, abnormalities in dopamine receptor function or dopamine overproduction in the central nervous system are currently considered to be the main mechanisms for the co-occurrence of epilepsy and ADHD.
Based on some studies, cortical maturation in ADHD patients involves multiple regions and cortical dimensions. Primarily, this is manifested in ADHD patients as delayed developmental trajectories. Previously, Shaw et al. (88) also demonstrated that cortical maturation in ADHD patients is significantly delayed. Likewise, Qian et al. (89) found developmentally relevant delays in inhibitory and transfer functions in children with ADHD. Children with ADHD are more affected by subcortical abnormalities; the more delayed subcortical structure development, the more prominent the hyperactivity and impulsivity symptoms in the child, and the slower the cortical thinning of the prefrontal and cingulate regions (90, 91). Besides, a report published in 2021 identified that children with ADHD had smaller cerebral cortex total surface area, cortical thickness and subcortical areas (7). Simultaneously, the developmental trajectory of gray matter volume and cortical thickness is correlated with changes in symptoms of ADHD. The more severe the symptoms, the slower the brain matures (65, 92). Therefore, the characterization of the corresponding brain regions of ADHD patients in magnetic resonance imaging will be explored in terms of the following imaging techniques.
Functional magnetic resonance imaging (fMRI) primarily uses magnetic fields to measure the blood oxygen level-dependent (BOLD) response in the cerebral cortex and subcortical regions. By gathering data from participants while they are at rest and in various task-design scenarios, fMRI can indirectly reflect how the brain functions.
The resting-state fMRI describes the low-frequency fluctuations that arise spontaneously during an MRI scan while the patient's body is at rest and not thinking. The resting-state network describes the multiple brain regions where the fMRI signals are correlated with each other in the resting state. The default mode network (DMN), a significant resting-state network, is made up of brain areas that, in healthy individuals, exhibit increased activity during waking rest and deactivation with increasing attentional demands (93, 94). However, this negative correlation between the default mode network and attention will be diminished or nonexistent in ADHD patients, which could account for the reduced sustained attention brought on by default mode network-mediated attention deficiencies (95–98).
Moreover, Sun et al. (99) demonstrated that children with ADHD had more fragmented resting-state network connection patterns and delayed functional network development. Research employing resting-state fMRI has demonstrated dysfunctional connectivity in the brain regions of the dorsal anterior cingulate cortex and posterior cingulate cortex and aberrant developmental patterns in the interaction between the dorsal anterior cingulate cortex and DMN in patients with ADHD (99). Furthermore, TIAN et al. (100) discovered that children with ADHD had significantly improved functional connectivity in the dorsal anterior cingulate cortex as well as bilateral thalamus, bilateral cerebellum, and bilateral insula. Additionally, children diagnosed with ADHD exhibited reduced functional connectivity in the areas of the thalamus and basal ganglia (96).
fMRI combines many task paradigms to represent the various cognitive processes occurring in the brain. Studies on ADHD patients have revealed impairments in cognitive abilities such as working memory, sustained attention, and inhibitory function (101). Research has demonstrated (102) that when engaged in working memory tasks, individuals with ADHD exhibit decreased activity in bilateral frontal lobes, frontal-to-parietal areas and the insulae. Likewise, in comparison to normal controls, working memory-related brain regions consistently and repetitively exhibit underactivation (103, 104). Children with ADHD have also been observed to show under-activation of brain regions associated with inhibitory control in inhibitory function tasks (103, 104).
Furthermore, Christakou et al. (105) used magnetic resonance imaging to demonstrate that patients with ADHD exhibited significantly higher activation in precuneus regions but significantly lower activation in the left dorsolateral prefrontal cortex, superior parietal gyrus, and striatal-thalamic regions in an alertness task involving sustained attention. Additionally, children with ADHD mostly exhibit decreased activation of the right dorsolateral frontal-basal ganglia-thalamic-parietal network when doing tasks that target attentional processes (103, 106). Reduced frontal-striatal loop activation has also been seen in children with ADHD during sustained attention activities (107, 108).
Functional near-infrared spectroscopy (fNIRS) utilizes near-infrared light to track variations in oxygen and deoxyhemoglobin concentrations over time. Notably, it has been used extensively in studies because it is less sensitive to motion artifacts than fMRI, safe, inexpensive, and requires less body immobilization. fNIRS is a useful tool for measuring brain activity during resting and task states. Blood flow and volume rise when brain areas are activated in response to stimuli, and this can be measured by determining the concentration of local hemoglobin (HbO), deoxyhemoglobin (HbR), or total hemoglobin (HbT). Thus, fNIRS is a valuable assessment instrument for neurodevelopmental research, particularly in analyzing the effects of interventions on children with ADHD (109, 110).
Researchers have demonstrated that resting-state fNIRS is repeatable in terms of functional connectivity and network topological characteristics (111–114). According to research conducted in 2020 by Wang et al. (115), children with ADHD had significantly lower functional connectivity and global efficiency of brain networks during the resting state when using fNIRS. Concurrently, each network node in the brain experienced corresponding changes in efficiency. The results may indicate deficiencies in reaction inhibition and information overload in vision and attention in children with ADHD, according to the dual pathway hypothesis. It was further established that functional connection networks and symptoms of ADHD are related. Besides, a negative correlation was identified between the reduced efficiency of the right somatomotor network nodes and the symptoms of hyperactivity and impulsivity. In 2021, based on a multiscale entropy study, Hu et al. found (116) that children with ADHD had lower brain signal variability in several functional brain networks (such as the default mode, frontoparietal network, attentional network, and visual network) than did healthy children, aligning with Wang's et al. (115) findings.
When examining the potential connection between alterations in brain activation and executive function in ADHD patients, fNIRS is a highly effective tool (117, 118). Meanwhile, a task-based investigation of dynamic functional connectivity in children with ADHD was carried out by Sutoko et al. (119). The frontal-cingulate-striatal-thalamic and frontal-parietal-cerebellar networks, which control working memory, attention, and inhibitory function, exhibit complicated multisystem deficits in ADHD patients.
In inhibitory control tasks, adolescents with ADHD have inferior connectivity within the inhibitory network (120) Sutoko et al. (121) discovered that children with ADHD tend to show a decreased likelihood of an advantageous connectivity state and an increased likelihood of other connectivity states in an inhibitory control task. In a 2023 study, Hou et al. (122) demonstrated that activation areas inhibiting cognitive interference ability were concentrated in the bilateral prefrontal cortex, whereas activation areas inhibiting control skills were broadly distributed in the bilateral prefrontal cortex, parietal and frontal regions, the left temporal and superior temporal cortex, the right inferior frontal gyrus, and the middle frontal gyrus. According to Inoue et al. (123), children with ADHD showed decreased prefrontal activation during a go/no-go task. Children with ADHD showed lower levels of brain activity in the left prefrontal cortex during go/no-go task, according to a 2017 study (124). Furthermore, Wu et al. (125) discovered that during a go/no-go task, children with ADHD had decreased levels of oxyhemoglobin concentration in the prefrontal brain. Prior research utilizing fMRI has also revealed that when children with ADHD do an inhibitory task, there is a significant bilateral decrease in cerebral blood flow in the prefrontal cortex region (126, 127).
In working memory tasks, the activation areas of verbal working memory in healthy individuals were primarily located in the right prefrontal cortex (PFC), particularly in the right ventral lateral prefrontal cortex (VLPFC) and dorsal lateral prefrontal cortex (DLPFC), and the activation areas of visuospatial working memory were found in the right prefrontal cortex, the frontal pole, and the left superior frontal cortex. However, the activation areas of patients with ADHD were less significant than those of healthy individuals, and the corresponding brain regions of working memory were either non-activated or weakly activated (122). The well-known working memory paradigm known as the n-back task has been used in functional neuroimaging studies of ADHD (128). Using fNIRS during the n-back task, Gu et al. (129) discovered that prefrontal complexity was lower in ADHD patients than in healthy controls. Two recent fNIRS investigations have demonstrated that when working on working memory tasks, the left DLPFC is more active in patients with ADHD (130, 131).
Future research on ADHD will focus on the following areas. Firstly, to more precisely clinically phenotype ADHD, it might be possible to synthesize genetic GWAS big data analysis with findings from ADHD cognitive science, brain connectivity mapping, and neuroimaging; in the future, and it might be possible to phenotype the ADHD phenotype in terms of functional impairment dimensions. Secondly, objective markers from genetics, epigenetics, and environmental factors that are strongly linked to the development of ADHD will be identified and utilized to facilitate early screening and support early diagnosis. Thirdly, neuroimaging studies of ADHD will gradually transition from MRI or near-infrared spectroscopic studies of ADHD to comprehensive studies integrating ADHD risk genes, specific cell types, developmental age-related brain networks, and neural network connectivity related to specific brain functions (cognition, emotion regulation, etc.). Additionally, they will map ADHD brain functions related to the age of onset of ADHD and its phenotypes, providing a powerful adjunct to the diagnosis of ADHD, as well as to typing, intervention and evaluation of therapeutic efficacy.
Furthermore, given the research on the mechanism of ADHD psychology and cognitive deficits, a digital, multi-scenario cognitive training system suitable for ADHD children of different ages will be developed, an accurate ADHD assessment system based on cognitive, behavioral, and emotional analyses as well as a digital cognitive and psychological intervention system will be established, and an intervention on ADHD cognitive deficits will be carried out with ADHD data-based prescriptions.
Besides, we will integrate ADHD neurological examination, cognitive and behavioral assessment, and brain network connection image analysis (resting state and task state) to assess ADHD children in all aspects, formulate individualized intervention plans, carry out related neuromodulation including repetitive transcranial magnetic stimulation, electroencephalographic biofeedback, magnetic resonance biofeedback, and transcranial direct current (DC)/alternating current (AC) stimulation, and observe the changes in the neuromodulation technology on the neural network connection and neural function, and establish reasonable and feasible neuromodulation therapeutic guidelines.
Finally, the pharmacological personalized treatment approach is a new direction for future ADHD treatment, aiming to identify biomarkers that can predict treatment response and guide personalized intervention. Based on genomics, neuroimaging and other neurological techniques to reveal the underlying biological mechanisms of ADHD, new interventional drugs can be developed to combat ADHD, which will help to target the treatment of different phenotypes of ADHD.
In summary, ADHD is a critically important neurodevelopmental condition in children. Through genetic GWAS analysis, epigenetic and neuroimaging studies in children with ADHD, researchers have discovered genome-wide significant loci associated with the development of ADHD, as well as thinning of gray matter thickness throughout the entire cerebral cortex, abnormalities in brain structure and function, and delayed development of neural networks in children with ADHD. This review offers potential uses in the investigation of the brain underpinnings of development, aging, and neurological illnesses.
FS: Writing – original draft. HZ: Writing – review & editing.
The authors declare that no financial support was received for the research, authorship, and/or publication of this article.
The authors declare that the research was conducted in the absence of any commercial or financial relationships that could be construed as a potential conflict of interest.
All claims expressed in this article are solely those of the authors and do not necessarily represent those of their affiliated organizations, or those of the publisher, the editors and the reviewers. Any product that may be evaluated in this article, or claim that may be made by its manufacturer, is not guaranteed or endorsed by the publisher.
1. Sayal K, Prasad V, Daley D, Ford T, Coghill D. ADHD in children and young people: prevalence, care pathways, and service provision. Lancet Psychiatry. (2018) 5(2):175–86. doi: 10.1016/S2215-0366(17)30167-0
2. Wolraich ML, Hagan JF Jr, Allan C, Chan E, Davison D, Earls M, et al. Clinical practice guideline for the diagnosis, evaluation, and treatment of attention-deficit/hyperactivity disorder in children and adolescents. Pediatrics. (2019) 144(4). doi: 10.1542/peds.2019-2528
3. Li F, Cui Y, Li Y, Guo L, Ke X, Liu J, et al. Prevalence of mental disorders in school children and adolescents in China: diagnostic data from detailed clinical assessments of 17,524 individuals. J Child Psychol Psychiatry. (2022) 63(1):34–46. doi: 10.1111/jcpp.13445
4. Prieto AT. Attention-deficit/hyperactivity disorder and substance abuse. Scientific evidence. Medicina. (2020) 80(Suppl. 2):76–9.32150719
5. Shi X, Ji Y, Cai S, Wu Y, Zhang L, Shen L, et al. Comorbidities and functional impairments in children with attention deficit hyperactivity disorder in China: a hospital-based retrospective cross-sectional study. BMJ Open. (2021) 11(3):e042196. doi: 10.1136/bmjopen-2020-042196
6. Cortese S. The neurobiology and genetics of attention-deficit/hyperactivity disorder (ADHD): what every clinician should know. Eur J Paediatr Neurol. (2012) 16(5):422–33. doi: 10.1016/j.ejpn.2012.01.009
7. Faraone SV, Banaschewski T, Coghill D, Zheng Y, Biederman J, Bellgrove MA, et al. The world federation of ADHD international consensus statement: 208 evidence-based conclusions about the disorder. Neurosci Biobehav Rev. (2021) 128:789–818. doi: 10.1016/j.neubiorev.2021.01.022
8. Barkley RA. Behavioral inhibition, sustained attention, and executive functions: constructing a unifying theory of ADHD. Psychol Bull. (1997) 121(1):65–94. doi: 10.1037/0033-2909.121.1.65
9. Uchida M, DiSalvo M, Walsh D, Biederman J. The heritability of ADHD in children of ADHD parents: a post-hoc analysis of longitudinal data. J Atten Disord. (2023) 27(3):250–7. doi: 10.1177/10870547221136251
10. Faraone SV, Larsson H. Genetics of attention deficit hyperactivity disorder. Mol Psychiatry. (2019) 24(4):562–75. doi: 10.1038/s41380-018-0070-0
11. Demontis D, Walters RK, Martin J, Mattheisen M, Als TD, Agerbo E, et al. Discovery of the first genome-wide significant risk loci for attention deficit/hyperactivity disorder. Nat Genet. (2019) 51(1):63–75. doi: 10.1038/s41588-018-0269-7
12. Demontis D, Walters GB, Athanasiadis G, Walters R, Therrien K, Nielsen TT, et al. Genome-wide analyses of ADHD identify 27 risk loci, refine the genetic architecture and implicate several cognitive domains. Nat Genet. (2023) 55(2):198–208. doi: 10.1038/s41588-022-01285-8
13. Warikoo N, Faraone SV. Background, clinical features and treatment of attention deficit hyperactivity disorder in children. Expert Opin Pharmacother. (2013) 14(14):1885–906. doi: 10.1517/14656566.2013.818977
14. Chang CH, Yu CJ, Du JC, Chiou HC, Chen HC, Yang W, et al. The interactions among organophosphate pesticide exposure, oxidative stress, and genetic polymorphisms of dopamine receptor D4 increase the risk of attention deficit/hyperactivity disorder in children. Environ Res. (2018) 160:339–46. doi: 10.1016/j.envres.2017.10.011
15. Tong JH, Cummins TD, Johnson BP, McKinley LA, Pickering HE, Fanning P, et al. An association between a dopamine transporter gene (SLC6A3) haplotype and ADHD symptom measures in nonclinical adults. Am J Med Genet B Neuropsychiatr Genet. (2015) 168b(2):89–96. doi: 10.1002/ajmg.b.32283
16. Pineau G, Villemonteix T, Slama H, Kavec M, Balériaux D, Metens T, et al. Dopamine transporter genotype modulates brain activity during a working memory task in children with ADHD. Res Dev Disabil. (2019) 92:103430. doi: 10.1016/j.ridd.2019.103430
17. Hong JH, Hwang IW, Lim MH, Kwon HJ, Jin HJ. Genetic associations between ADHD and dopaminergic genes (DAT1 and DRD4) VNTRs in Korean children. Genes Genomics. (2018) 40(12):1309–17. doi: 10.1007/s13258-018-0726-9
18. Gharaibeh MY, Batayneh S, Khabour OF, Daoud A. Association between polymorphisms of the DBH and DAT1 genes and attention deficit hyperactivity disorder in children from Jordan. Exp Ther Med. (2010) 1(4):701–5. doi: 10.3892/etm_00000108
19. Guney E, Işeri E, Ergun SG, Percin EF, Ergun MA, Yalcin O, et al. The correlation of attention deficit hyperactivity disorder with DRD4 gene polymorphism in Turkey. Int J Hum Genet. (2013) 13:145–52. doi: 10.1080/09723757.2013.11886210
20. Qian Q, Wang Y, Zhou R, Yang L, Faraone SV. Family-based and case-control association studies of DRD4 and DAT1 polymorphisms in Chinese attention deficit hyperactivity disorder patients suggest long repeats contribute to genetic risk for the disorder. Am J Med Genet B Neuropsychiatr Genet. (2004) 128b(1):84–9. doi: 10.1002/ajmg.b.30079
21. Banoei MM, Majidizadeh T, Shirazi E, Moghimi N, Ghadiri M, Najmabadi H, et al. No association between the DAT1 10-repeat allele and ADHD in the Iranian population. Am J Med Genet B Neuropsychiatr Genet. (2008) 147b(1):110–1. doi: 10.1002/ajmg.b.30578
22. Simsek M, Al-Sharbati M, Al-Adawi S, Ganguly SS, Lawatia K. Association of the risk allele of dopamine transporter gene (DAT1*10) in Omani male children with attention-deficit hyperactivity disorder. Clin Biochem. (2005) 38(8):739–42. doi: 10.1016/j.clinbiochem.2005.04.016
23. Thursina C, Nurputra DK, Harahap ISK, Harahap NIF, Sa’adah N, Wibowo S, et al. Determining the association between polymorphisms of the DAT1 and DRD4 genes with attention deficit hyperactivity disorder in children from Java Island. Neurol Int. (2020) 12(1):8292. doi: 10.4081/ni.2020.8292
24. Kuc K, Bielecki M, Racicka-Pawlukiewicz E, Czerwinski MB, Cybulska-Klosowicz A. The SLC6A3 gene polymorphism is related to the development of attentional functions but not to ADHD. Sci Rep. (2020) 10(1):6176. doi: 10.1038/s41598-020-63296-x
25. Cifariello A, Pompili A, Gasbarri A. 5-HT(7) receptors in the modulation of cognitive processes. Behav Brain Res. (2008) 195(1):171–9. doi: 10.1016/j.bbr.2007.12.012
26. Albert PR, Le François B, Vahid-Ansari F. Genetic, epigenetic and posttranscriptional mechanisms for treatment of major depression: the 5-HT1A receptor gene as a paradigm. J Psychiatry Neurosci. (2019) 44(3):164–76. doi: 10.1503/jpn.180209
27. Kautzky A, Vanicek T, Philippe C, Kranz GS, Wadsak W, Mitterhauser M, et al. Machine learning classification of ADHD and HC by multimodal serotonergic data. Transl Psychiatry. (2020) 10(1):104. doi: 10.1038/s41398-020-0781-2
28. Chatterjee M, Saha S, Sinha S, Mukhopadhyay K. A three-pronged analysis confirms the association of the serotoninergic system with attention deficit hyperactivity disorder. World J Pediatr. (2022) 18(12):825–34. doi: 10.1007/s12519-022-00614-5
29. Shang CY, Lin HY, Gau SS. The norepinephrine transporter gene modulates intrinsic brain activity, visual memory, and visual attention in children with attention-deficit/hyperactivity disorder. Mol Psychiatry. (2021) 26(8):4026–35. doi: 10.1038/s41380-019-0545-7
30. Shirama A, Takeda T, Ohta H, Iwanami A, Toda S, Kato N. Atypical alert state control in adult patients with ADHD: a pupillometry study. PLoS ONE. (2020) 15(12):e0244662. doi: 10.1371/journal.pone.0244662
31. Hawi Z, Matthews N, Barry E, Kirley A, Wagner J, Wallace RH, et al. A high density linkage disequilibrium mapping in 14 noradrenergic genes: evidence of association between SLC6A2, ADRA1B and ADHD. Psychopharmacology. (2013) 225(4):895–902. doi: 10.1007/s00213-012-2875-x
32. Wang Y, Wang T, Du Y, Hu D, Zhang Y, Li H, et al. Polygenic risk of genes involved in the catecholamine and serotonin pathways for ADHD in children. Neurosci Lett. (2021) 760:136086. doi: 10.1016/j.neulet.2021.136086
33. Peterson BS, Rauh VA, Bansal R, Hao X, Toth Z, Nati G, et al. Effects of prenatal exposure to air pollutants (polycyclic aromatic hydrocarbons) on the development of brain white matter, cognition, and behavior in later childhood. JAMA psychiatry. (2015) 72(6):531–40. doi: 10.1001/jamapsychiatry.2015.57
34. He Y, Chen J, Zhu LH, Hua LL, Ke FF. Maternal smoking during pregnancy and ADHD: results from a systematic review and meta-analysis of prospective cohort studies. J Atten Disord. (2020) 24(12):1637–47. doi: 10.1177/1087054717696766
35. Wetherill L, Foroud T, Goodlett C. Meta-analyses of externalizing disorders: genetics or prenatal alcohol exposure? Alcohol Clin Exp Res. (2018) 42(1):162–72. doi: 10.1111/acer.13535
36. Li DK, Chen H, Ferber JR, Hirst AK, Odouli R. Association between maternal exposure to magnetic field nonionizing radiation during pregnancy and risk of attention-deficit/hyperactivity disorder in offspring in a longitudinal birth cohort. JAMA Netw Open. (2020) 3(3):e201417. doi: 10.1001/jamanetworkopen.2020.1417
37. Gou X, Wang Y, Tang Y, Qu Y, Tang J, Shi J, et al. Association of maternal prenatal acetaminophen use with the risk of attention deficit/hyperactivity disorder in offspring: a meta-analysis. Aust N Z J Psychiatry. (2019) 53(3):195–206. doi: 10.1177/0004867418823276
38. Rodriguez A. Maternal pre-pregnancy obesity and risk for inattention and negative emotionality in children. J Child Psychol Psychiatry. (2010) 51(2):134–43. doi: 10.1111/j.1469-7610.2009.02133.x
39. Fuemmeler BF, Zucker N, Sheng Y, Sanchez CE, Maguire R, Murphy SK, et al. Pre-pregnancy weight and symptoms of attention deficit hyperactivity disorder and executive functioning behaviors in preschool children. Int J Environ Res Public Health. (2019) 16(4):669. doi: 10.3390/ijerph16040667
40. McCoy BM, Rickert ME, Class QA, Larsson H, Lichtenstein P, D'Onofrio BM. Mediators of the association between parental severe mental illness and offspring neurodevelopmental problems. Ann Epidemiol. (2014) 24(9):629–34.e1. doi: 10.1016/j.annepidem.2014.05.010
41. Modesto T, Tiemeier H, Peeters RP, Jaddoe VW, Hofman A, Verhulst FC, et al. Maternal mild thyroid hormone insufficiency in early pregnancy and attention-deficit/hyperactivity disorder symptoms in children. JAMA Pediatr. (2015) 169(9):838–45. doi: 10.1001/jamapediatrics.2015.0498
42. Wolford E, Lahti M, Tuovinen S, Lahti J, Lipsanen J, Savolainen K, et al. Maternal depressive symptoms during and after pregnancy are associated with attention-deficit/hyperactivity disorder symptoms in their 3- to 6-year-old children. PLoS ONE. (2017) 12(12):e0190248. doi: 10.1371/journal.pone.0190248
43. Nomura Y, Marks DJ, Grossman B, Yoon M, Loudon H, Stone J, et al. Exposure to gestational diabetes mellitus and low socioeconomic status: effects on neurocognitive development and risk of attention-deficit/hyperactivity disorder in offspring. Arch Pediatr Adolesc Med. (2012) 166(4):337–43. doi: 10.1001/archpediatrics.2011.784
44. Nilsen FM, Tulve NS. A systematic review and meta-analysis examining the interrelationships between chemical and non-chemical stressors and inherent characteristics in children with ADHD. Environ Res. (2020) 180:108884. doi: 10.1016/j.envres.2019.108884
45. Ren Y, Fang X, Fang H, Pang G, Cai J, Wang S, et al. Predicting the adult clinical and academic outcomes in boys with ADHD: a 7- to 10-year follow-up study in China. Front Pediatr. (2021) 9:634633. doi: 10.3389/fped.2021.634633
46. Claussen AH, Holbrook JR, Hutchins HJ, Robinson LR, Bloomfield J, Meng L, et al. All in the family? A systematic review and meta-analysis of parenting and family environment as risk factors for attention-deficit/hyperactivity disorder (ADHD) in children. Prev Sci. (2022):1–23. doi: 10.1007/s11121-022-01358-4
47. Duh-Leong C, Fuller A, Brown NM. Associations between family and community protective factors and attention-deficit/hyperactivity disorder outcomes among US children. J Dev Behav Pediatr. (2020) 41(1):1–8. doi: 10.1097/DBP.0000000000000720
48. Durukan İ, Kara K, Almbaideen M, Karaman D, Gül H. Alexithymia, depression and anxiety in parents of children with neurodevelopmental disorder: comparative study of autistic disorder, pervasive developmental disorder not otherwise specified and attention deficit-hyperactivity disorder. Pediatr Int. (2018) 60(3):247–53. doi: 10.1111/ped.13510
49. Bublitz MH, Stroud LR. Maternal smoking during pregnancy and offspring brain structure and function: review and agenda for future research. Nicotine Tob Res. (2012) 14(4):388–97. doi: 10.1093/ntr/ntr191
50. Lebel C, Roussotte F, Sowell ER. Imaging the impact of prenatal alcohol exposure on the structure of the developing human brain. Neuropsychol Rev. (2011) 21(2):102–18. doi: 10.1007/s11065-011-9163-0
51. Paolozza A, Rasmussen C, Pei J, Hanlon-Dearman A, Nikkel SM, Andrew G, et al. Deficits in response inhibition correlate with oculomotor control in children with fetal alcohol spectrum disorder and prenatal alcohol exposure. Behav Brain Res. (2014) 259:97–105. doi: 10.1016/j.bbr.2013.10.040
52. Blecharz-Klin K, Piechal A, Pyrzanowska J, Joniec-Maciejak I, Kiliszek P, Widy-Tyszkiewicz E. Paracetamol–the outcome on neurotransmission and spatial learning in rats. Behav Brain Res. (2013) 253:157–64. doi: 10.1016/j.bbr.2013.07.008
53. Short SJ, Lubach GR, Karasin AI, Olsen CW, Styner M, Knickmeyer RC, et al. Maternal influenza infection during pregnancy impacts postnatal brain development in the rhesus monkey. Biol Psychiatry. (2010) 67(10):965–73. doi: 10.1016/j.biopsych.2009.11.026
54. Willette AA, Lubach GR, Knickmeyer RC, Short SJ, Styner M, Gilmore JH, et al. Brain enlargement and increased behavioral and cytokine reactivity in infant monkeys following acute prenatal endotoxemia. Behav Brain Res. (2011) 219(1):108–15. doi: 10.1016/j.bbr.2010.12.023
55. Sullivan EL, Nousen EK, Chamlou KA. Maternal high fat diet consumption during the perinatal period programs offspring behavior. Physiol Behav. (2014) 123:236–42. doi: 10.1016/j.physbeh.2012.07.014
56. Kang SS, Kurti A, Fair DA, Fryer JD. Dietary intervention rescues maternal obesity induced behavior deficits and neuroinflammation in offspring. J Neuroinflammation. (2014) 11:156. doi: 10.1186/s12974-014-0156-9
57. Van Dam JM, Garrett AJ, Schneider LA, Hodyl NA, Goldsworthy MR, Coat S, et al. Reduced cortical excitability, neuroplasticity, and salivary cortisol in 11–13-year-old children born to women with gestational diabetes Mellitus. EBioMedicine. (2018) 31:143–9. doi: 10.1016/j.ebiom.2018.04.011
58. Xuan DS, Zhao X, Liu YC, Xing QN, Shang HL, Zhu PY, et al. Brain development in infants of mothers with gestational diabetes mellitus: a diffusion tensor imaging study. J Comput Assist Tomogr. (2020) 44(6):947–52. doi: 10.1097/RCT.0000000000001110
59. Lynch KM, Alves JM, Chow T, Clark KA, Luo S, Toga AW, et al. Selective morphological and volumetric alterations in the hippocampus of children exposed in utero to gestational diabetes mellitus. Hum Brain Mapp. (2021) 42(8):2583–92. doi: 10.1002/hbm.25390
60. Ahmed S, Cano M, Sánchez M, Hu N, Ibañez G. Effect of exposure to maternal diabetes during pregnancy on offspring’s brain cortical thickness and neurocognitive functioning. Child Neuropsychol. (2023) 29(4):588–606. doi: 10.1080/09297049.2022.2103105
61. Wang KC, Chang HY. Epigenomics: technologies and applications. Circ Res. (2018) 122(9):1191–9. doi: 10.1161/CIRCRESAHA.118.310998
62. Xu Y, Chen XT, Luo M, Tang Y, Zhang G, Wu D, et al. Multiple epigenetic factors predict the attention deficit/hyperactivity disorder among the Chinese Han children. J Psychiatr Res. (2015) 64:40–50. doi: 10.1016/j.jpsychires.2015.03.006
63. van Mil NH, Steegers-Theunissen RP, Bouwland-Both MI, Verbiest MM, Rijlaarsdam J, Hofman A, et al. DNA methylation profiles at birth and child ADHD symptoms. J Psychiatr Res. (2014) 49:51–9. doi: 10.1016/j.jpsychires.2013.10.017
64. Wilmot B, Fry R, Smeester L, Musser ED, Mill J, Nigg JT. Methylomic analysis of salivary DNA in childhood ADHD identifies altered DNA methylation in VIPR2. J Child Psychol Psychiatry. (2016) 57(2):152–60. doi: 10.1111/jcpp.12457
65. Walton E, Pingault JB, Cecil CA, Gaunt TR, Relton CL, Mill J, et al. Epigenetic profiling of ADHD symptoms trajectories: a prospective, methylome-wide study. Mol Psychiatry. (2017) 22(2):250–6. doi: 10.1038/mp.2016.85
66. Chen YC, Sudre G, Sharp W, Donovan F, Chandrasekharappa SC, Hansen N, et al. Neuroanatomic, epigenetic and genetic differences in monozygotic twins discordant for attention deficit hyperactivity disorder. Mol Psychiatry. (2018) 23(3):683–90. doi: 10.1038/mp.2017.45
67. Bannister AJ, Kouzarides T. Regulation of chromatin by histone modifications. Cell Res. (2011) 21(3):381–95. doi: 10.1038/cr.2011.22
68. Bartel DP. MicroRNAs: target recognition and regulatory functions. Cell. (2009) 136(2):215–33. doi: 10.1016/j.cell.2009.01.002
69. Srivastav S, Walitza S, Grünblatt E. Emerging role of miRNA in attention deficit hyperactivity disorder: a systematic review. Atten Defic Hyperact Disord. (2018) 10(1):49–63. doi: 10.1007/s12402-017-0232-y
70. Dypås LB, Duale N, Olsen AK, Bustamante M, Maitre L, Escaramis G, et al. Blood miRNA levels associated with ADHD traits in children across six European birth cohorts. BMC psychiatry. (2023) 23(1):696. doi: 10.1186/s12888-023-05199-5
71. Kessler RC, Adler LA, Berglund P, Green JG, McLaughlin KA, Fayyad J, et al. The effects of temporally secondary co-morbid mental disorders on the associations of DSM-IV ADHD with adverse outcomes in the US national comorbidity survey replication adolescent supplement (NCS-A). Psychol Med. (2014) 44(8):1779–92. doi: 10.1017/S0033291713002419
72. Muskens JB, Velders FP, Staal WG. Medical comorbidities in children and adolescents with autism spectrum disorders and attention deficit hyperactivity disorders: a systematic review. Eur Child Adolesc Psychiatry. (2017) 26(9):1093–103. doi: 10.1007/s00787-017-1020-0
73. Merikangas KR, Calkins ME, Burstein M, He JP, Chiavacci R, Lateef T, et al. Comorbidity of physical and mental disorders in the neurodevelopmental genomics cohort study. Pediatrics. (2015) 135(4):e927–38. doi: 10.1542/peds.2014-1444
74. Demontis D, Walters GB, Athanasiadis G, Walters R, Therrien K, Nielsen TT, et al. Author correction: genome-wide analyses of ADHD identify 27 risk loci, refine the genetic architecture and implicate several cognitive domains. Nat Genet. (2023) 55(4):730. doi: 10.1038/s41588-023-01350-w
75. Noordermeer SDS, Luman M, Buitelaar JK, Hartman CA, Hoekstra PJ, Franke B, et al. Neurocognitive deficits in attention-deficit/hyperactivity disorder with and without comorbid oppositional defiant disorder. J Atten Disord. (2020) 24(9):1317–29. doi: 10.1177/1087054715606216
76. Tistarelli N, Fagnani C, Troianiello M, Stazi MA, Adriani W. The nature and nurture of ADHD and its comorbidities: a narrative review on twin studies. Neurosci Biobehav Rev. (2020) 109:63–77. doi: 10.1016/j.neubiorev.2019.12.017
77. Hartman CA, Rommelse N, van der Klugt CL, Wanders RBK, Timmerman ME. Stress exposure and the course of ADHD from childhood to young adulthood: comorbid severe emotion dysregulation or mood and anxiety problems. J Clin Med. (2019) 8(11):1824. doi: 10.3390/jcm8111824
78. Rychik N, Fassett-Carman A, Snyder HR. Dependent stress mediates the relation between ADHD symptoms and depression. J Atten Disord. (2021) 25(12):1676–86. doi: 10.1177/1087054720925900
79. Zendarski N, Guo S, Sciberras E, Efron D, Quach J, Winter L, et al. Examining the educational gap for children with ADHD and subthreshold ADHD. J Atten Disord. (2022) 26(2):282–95. doi: 10.1177/1087054720972790
80. Fraporti TT, Contini V, Tovo-Rodrigues L, Recamonde-Mendoza M, Rovaris DL, Rohde LA, et al. Synergistic effects between ADORA2A and DRD2 genes on anxiety disorders in children with ADHD. Prog Neuro-Psychopharmacol Biol Psychiatry. (2019) 93:214–20. doi: 10.1016/j.pnpbp.2019.03.021
81. Park KJ, Kim MJ, Yum MS, Ko TS, Kim HW. Clinical and neuropsychological characteristics of children with epilepsy and attention-deficit/hyperactivity disorder. Seizure. (2021) 91:325–31. doi: 10.1016/j.seizure.2021.06.022
82. Wiggs KK, Chang Z, Quinn PD, Hur K, Gibbons R, Dunn D, et al. Attention-deficit/hyperactivity disorder medication and seizures. Neurology. (2018) 90(13):e1104–10. doi: 10.1212/WNL.0000000000005213
83. Karalok ZS, Öztürk Z, Gunes A. Cortical thinning in benign epilepsy with centrotemporal spikes (BECTS) with or without attention-deficit/hyperactivity (ADHD). J Clin Neurosci. (2019) 68:123–7. doi: 10.1016/j.jocn.2019.07.014
84. Brikell I, Ghirardi L, D'Onofrio BM, Dunn DW, Almqvist C, Dalsgaard S, et al. Familial liability to epilepsy and attention-deficit/hyperactivity disorder: a nationwide cohort study. Biol Psychiatry. (2018) 83(2):173–80. doi: 10.1016/j.biopsych.2017.08.006
85. Moey C, Hinze SJ, Brueton L, Morton J, McMullan DJ, Kamien B, et al. Xp11.2 microduplications including IQSEC2, TSPYL2 and KDM5C genes in patients with neurodevelopmental disorders. Eur J Hum Genet. (2016) 24(3):373–80. doi: 10.1038/ejhg.2015.123
86. Poliquin S, Hughes I, Shen W, Mermer F, Wang J, Mack T, et al. Genetic mosaicism, intrafamilial phenotypic heterogeneity, and molecular defects of a novel missense SLC6A1 mutation associated with epilepsy and ADHD. Exp Neurol. (2021) 342:113723. doi: 10.1016/j.expneurol.2021.113723
87. Xi XJ, Tang JH, Zhang BB, Xiao X, Hu XY, Wan Y, et al. Dlg4 and Vamp2 are involved in comorbid epilepsy and attention-deficit hyperactivity disorder: a microarray data study. Epilepsy & Behav. (2020) 110:107192. doi: 10.1016/j.yebeh.2020.107192
88. Shaw P, Eckstrand K, Sharp W, Blumenthal J, Lerch JP, Greenstein D, et al. Attention-deficit/hyperactivity disorder is characterized by a delay in cortical maturation. Proc Natl Acad Sci USA. (2007) 104(49):19649–54. doi: 10.1073/pnas.0707741104
89. Qian Y, Shuai L, Chan RC, Qian QJ, Wang Y. The developmental trajectories of executive function of children and adolescents with attention deficit hyperactivity disorder. Res Dev Disabil. (2013) 34(5):1434–45. doi: 10.1016/j.ridd.2013.01.033
90. Castellanos FX, Tannock R. Neuroscience of attention-deficit/hyperactivity disorder: the search for endophenotypes. Nat Rev Neurosci. (2002) 3(8):617–28. doi: 10.1038/nrn896
91. Ambrosino S, de Zeeuw P, Wierenga LM, van Dijk S, Durston S. What can cortical development in attention-deficit/hyperactivity disorder teach us about the early developmental mechanisms involved? Cereb Cortex. (2017) 27(9):4624–34. doi: 10.1093/cercor/bhx182
92. Shaw P, Sudre G. Adolescent attention-deficit/hyperactivity disorder: understanding teenage symptom trajectories. Biol Psychiatry. (2021) 89(2):152–61. doi: 10.1016/j.biopsych.2020.06.004
93. Buckner RL, Andrews-Hanna JR, Schacter DL. The brain’s default network: anatomy, function, and relevance to disease. Ann N Y Acad Sci. (2008) 1124:1–38. doi: 10.1196/annals.1440.011
94. Raichle ME, Snyder AZ. A default mode of brain function: a brief history of an evolving idea. NeuroImage. (2007) 37(4):1083–90; discussion 97–9. doi: 10.1016/j.neuroimage.2007.02.041
95. Posner J, Park C, Wang Z. Connecting the dots: a review of resting connectivity MRI studies in attention-deficit/hyperactivity disorder. Neuropsychol Rev. (2014) 24(1):3–15. doi: 10.1007/s11065-014-9251-z
96. Cao X, Cao Q, Long X, Sun L, Sui M, Zhu C, et al. Abnormal resting-state functional connectivity patterns of the putamen in medication-naïve children with attention deficit hyperactivity disorder. Brain Res. (2009) 1303:195–206. doi: 10.1016/j.brainres.2009.08.029
97. Castellanos FX, Margulies DS, Kelly C, Uddin LQ, Ghaffari M, Kirsch A, et al. Cingulate-precuneus interactions: a new locus of dysfunction in adult attention-deficit/hyperactivity disorder. Biol Psychiatry. (2008) 63(3):332–7. doi: 10.1016/j.biopsych.2007.06.025
98. Bos DJ, Oranje B, Achterberg M, Vlaskamp C, Ambrosino S, de Reus MA, et al. Structural and functional connectivity in children and adolescents with and without attention deficit/hyperactivity disorder. J Child Psychol Psychiatry. (2017) 58(7):810–8. doi: 10.1111/jcpp.12712
99. Sun L, Cao Q, Long X, Sui M, Cao X, Zhu C, et al. Abnormal functional connectivity between the anterior cingulate and the default mode network in drug-naïve boys with attention deficit hyperactivity disorder. Psychiatry Res. (2012) 201(2):120–7. doi: 10.1016/j.pscychresns.2011.07.001
100. Tian L, Jiang T, Wang Y, Zang Y, He Y, Liang M, et al. Altered resting-state functional connectivity patterns of anterior cingulate cortex in adolescents with attention deficit hyperactivity disorder. Neurosci Lett. (2006) 400(1–2):39–43. doi: 10.1016/j.neulet.2006.02.022
101. Lee S, Hill TR, Johnson B, Testa R, Priya V, Spencer-Smith M, et al. Can neurocognitive outcomes assist measurement-based care for children with attention-deficit/hyperactivity disorder? A systematic review and meta-analyses of the relationships among the changes in neurocognitive functions and clinical outcomes of attention-deficit/hyperactivity disorder in pharmacological and cognitive training interventions. J Child Adolesc Psychopharmacol. (2022) 32(5):250–77. doi: 10.1089/cap.2022.0028
102. Wu ZM, Bralten J, An L, Cao QJ, Cao XH, Sun L, et al. Verbal working memory-related functional connectivity alterations in boys with attention-deficit/hyperactivity disorder and the effects of methylphenidate. J Psychopharmacol. (2017) 31(8):1061–9. doi: 10.1177/0269881117715607
103. Hart H, Radua J, Nakao T, Mataix-Cols D, Rubia K. Meta-analysis of functional magnetic resonance imaging studies of inhibition and attention in attention-deficit/hyperactivity disorder: exploring task-specific, stimulant medication, and age effects. JAMA psychiatry. (2013) 70(2):185–98. doi: 10.1001/jamapsychiatry.2013.277
104. Samea F, Soluki S, Nejati V, Zarei M, Cortese S, Eickhoff SB, et al. Brain alterations in children/adolescents with ADHD revisited: a neuroimaging meta-analysis of 96 structural and functional studies. Neurosci Biobehav Rev. (2019) 100:1–8. doi: 10.1016/j.neubiorev.2019.02.011
105. Christakou A, Murphy CM, Chantiluke K, Cubillo AI, Smith AB, Giampietro V, et al. Disorder-specific functional abnormalities during sustained attention in youth with attention deficit hyperactivity disorder (ADHD) and with autism. Mol Psychiatry. (2013) 18(2):236–44. doi: 10.1038/mp.2011.185
106. Dickstein SG, Bannon K, Castellanos FX, Milham MP. The neural correlates of attention deficit hyperactivity disorder: an ALE meta-analysis. J Child Psychol Psychiatry. (2006) 47(10):1051–62. doi: 10.1111/j.1469-7610.2006.01671.x
107. Lukito S, Norman L, Carlisi C, Radua J, Hart H, Simonoff E, et al. Comparative meta-analyses of brain structural and functional abnormalities during cognitive control in attention-deficit/hyperactivity disorder and autism spectrum disorder. Psychol Med. (2020) 50(6):894–919. doi: 10.1017/S0033291720000574
108. Norman LJ, Carlisi C, Lukito S, Hart H, Mataix-Cols D, Radua J, et al. Structural and functional brain abnormalities in attention-deficit/hyperactivity disorder and obsessive-compulsive disorder: a comparative meta-analysis. JAMA psychiatry. (2016) 73(8):815–25. doi: 10.1001/jamapsychiatry.2016.0700
109. Chen WL, Wagner J, Heugel N, Sugar J, Lee YW, Conant L, et al. Functional near-infrared spectroscopy and its clinical application in the field of neuroscience: advances and future directions. Front Neurosci. (2020) 14:724. doi: 10.3389/fnins.2020.00724
110. Grazioli S, Mauri M, Crippa A, Maggioni E, Molteni M, Brambilla P, et al. Light up ADHD: II. Neuropharmacological effects measured by near infrared spectroscopy: is there a biomarker? J Affect Disord. (2019) 244:100–6. doi: 10.1016/j.jad.2018.10.100
111. Niu H, Wang J, Zhao T, Shu N, He Y. Revealing topological organization of human brain functional networks with resting-state functional near infrared spectroscopy. PLoS ONE. (2012) 7(9):e45771. doi: 10.1371/journal.pone.0045771
112. Niu H, Li Z, Liao X, Wang J, Zhao T, Shu N, et al. Test-retest reliability of graph metrics in functional brain networks: a resting-state fNIRS study. PLoS ONE. (2013) 8(9):e72425. doi: 10.1371/journal.pone.0072425
113. Wang M, Yuan Z, Niu H. Reliability evaluation on weighted graph metrics of fNIRS brain networks. Quant Imaging Med Surg. (2019) 9(5):832–41. doi: 10.21037/qims.2019.05.08
114. Niu H, Khadka S, Tian F, Lin ZJ, Lu C, Zhu C, et al. Resting-state functional connectivity assessed with two diffuse optical tomographic systems. J Biomed Opt. (2011) 16(4):046006. doi: 10.1117/1.3561687
115. Wang M, Hu Z, Liu L, Li H, Qian Q, Niu H. Disrupted functional brain connectivity networks in children with attention-deficit/hyperactivity disorder: evidence from resting-state functional near-infrared spectroscopy. Neurophotonics. (2020) 7(1):015012. doi: 10.1117/1.NPh.7.1.015012
116. Hu Z, Liu L, Wang M, Jia G, Li H, Si F, et al. Disrupted signal variability of spontaneous neural activity in children with attention-deficit/hyperactivity disorder. Biomed Opt Express. (2021) 12(5):3037–49. doi: 10.1364/BOE.418921
117. Cui X, Bray S, Bryant DM, Glover GH, Reiss AL. A quantitative comparison of NIRS and fMRI across multiple cognitive tasks. NeuroImage. (2011) 54(4):2808–21. doi: 10.1016/j.neuroimage.2010.10.069
118. Boas DA, Elwell CE, Ferrari M, Taga G. Twenty years of functional near-infrared spectroscopy: introduction for the special issue. NeuroImage. (2014) 85(Pt 1):1–5. doi: 10.1016/j.neuroimage.2013.11.033
119. Sutoko S, Monden Y, Tokuda T, Ikeda T, Nagashima M, Funane T, et al. Exploring attentive task-based connectivity for screening attention deficit/hyperactivity disorder children: a functional near-infrared spectroscopy study. Neurophotonics. (2019) 6(4):045013. doi: 10.1117/1.NPh.6.4.045013
120. van Rooij D, Hartman CA, Mennes M, Oosterlaan J, Franke B, Rommelse N, et al. Altered neural connectivity during response inhibition in adolescents with attention-deficit/hyperactivity disorder and their unaffected siblings. NeuroImage Clin. (2015) 7:325–35. doi: 10.1016/j.nicl.2015.01.004
121. Sutoko S, Monden Y, Tokuda T, Ikeda T, Nagashima M, Funane T, et al. Atypical dynamic-connectivity recruitment in attention-deficit/hyperactivity disorder children: an insight into task-based dynamic connectivity through an fNIRS study. Front Hum Neurosci. (2020) 14:3. doi: 10.3389/fnhum.2020.00003
122. Hou L, Yang J, Xu L, Peng J, Joyce Law CY, Chen T. Activation of brain regions associated with working memory and inhibitory control in patients with attention-deficit/hyperactivity disorder in functional near-infrared spectroscopy: a systematic review. Curr Med Imaging. (2023) 19(8):865–73. doi: 10.2174/1573405618666220822101019
123. Inoue Y, Sakihara K, Gunji A, Ozawa H, Kimiya S, Shinoda H, et al. Reduced prefrontal hemodynamic response in children with ADHD during the go/NoGo task: a NIRS study. Neuroreport. (2012) 23(2):55–60. doi: 10.1097/WNR.0b013e32834e664c
124. Miao S, Han J, Gu Y, Wang X, Song W, Li D, et al. Reduced prefrontal cortex activation in children with attention-deficit/hyperactivity disorder during go/no-go task: a functional near-infrared spectroscopy study. Front Neurosci. (2017) 11:367. doi: 10.3389/fnins.2017.00367
125. Wu T, Liu X, Cheng F, Wang S, Li C, Zhou D, et al. Dorsolateral prefrontal cortex dysfunction caused by a go/no-go task in children with attention-deficit hyperactivity disorder: a functional near-infrared spectroscopy study. Front Neurosci. (2023) 17:1145485. doi: 10.3389/fnins.2023.1145485
126. Passarotti AM, Sweeney JA, Pavuluri MN. Neural correlates of response inhibition in pediatric bipolar disorder and attention deficit hyperactivity disorder. Psychiatry Res. (2010) 181(1):36–43. doi: 10.1016/j.pscychresns.2009.07.002
127. Rubia K, Cubillo A, Smith AB, Woolley J, Heyman I, Brammer MJ. Disorder-specific dysfunction in right inferior prefrontal cortex during two inhibition tasks in boys with attention-deficit hyperactivity disorder compared to boys with obsessive-compulsive disorder. Hum Brain Mapp. (2010) 31(2):287–99. doi: 10.1002/hbm.20864
128. Gu Y, Miao S, Han J, Liang Z, Ouyang G, Yang J, et al. Identifying ADHD children using hemodynamic responses during a working memory task measured by functional near-infrared spectroscopy. J Neural Eng. (2018) 15(3):035005. doi: 10.1088/1741-2552/aa9ee9
129. Gu Y, Miao S, Han J, Zeng K, Ouyang G, Yang J, et al. Complexity analysis of fNIRS signals in ADHD children during working memory task. Sci Rep. (2017) 7(1):829. doi: 10.1038/s41598-017-00965-4
130. Jang S, Choi J, Oh J, Yeom J, Hong N, Lee N, et al. Use of virtual reality working memory task and functional near-infrared spectroscopy to assess brain hemodynamic responses to methylphenidate in ADHD children. Front Psychiatry. (2020) 11:564618. doi: 10.3389/fpsyt.2020.564618
Keywords: attention deficit hyperactivity disorder, genetics, environmental risk factors, epigenetics, neuroimaging features, further research directions
Citation: Shen F and Zhou H (2024) Advances in the etiology and neuroimaging of children with attention deficit hyperactivity disorder. Front. Pediatr. 12:1400468. doi: 10.3389/fped.2024.1400468
Received: 13 March 2024; Accepted: 20 May 2024;
Published: 10 June 2024.
Edited by:
Sahar Ahmad, University of North Carolina at Chapel Hill, United StatesReviewed by:
Francesca Felicia Operto, University of Salerno, Italy© 2024 Shen and Zhou. This is an open-access article distributed under the terms of the Creative Commons Attribution License (CC BY). The use, distribution or reproduction in other forums is permitted, provided the original author(s) and the copyright owner(s) are credited and that the original publication in this journal is cited, in accordance with accepted academic practice. No use, distribution or reproduction is permitted which does not comply with these terms.
*Correspondence: Hui Zhou, MTEwMzU3MDQ4OUBxcS5jb20=
Disclaimer: All claims expressed in this article are solely those of the authors and do not necessarily represent those of their affiliated organizations, or those of the publisher, the editors and the reviewers. Any product that may be evaluated in this article or claim that may be made by its manufacturer is not guaranteed or endorsed by the publisher.
Research integrity at Frontiers
Learn more about the work of our research integrity team to safeguard the quality of each article we publish.