- Department of Endocrine, Genetics and Metabolism, Shanghai Children’s Hospital, School of Medicine, Shanghai Jiao Tong University, Shanghai, China
The gut microbiota plays a critical role in human growth and development as well as the regulation of human pathophysiological processes. According to research, the gut microbiota controls the host's growth and development in areas such as nutrition, metabolism, endocrine hormones, and immune modulation. The human gut microbiota has an important role in child and adolescent growth, especially when nutritional conditions are poor. In this review, we focus on recent findings about the gut microbiota's influence on child growth, including the relationship between the gut microbiota and linear growth during pregnancy, infancy, childhood, and adolescence. Furthermore, we also review some mechanisms by which intestinal flora influence the host's linear growth. Although the data supports a link between intestinal flora and linear development in children, our review has limitations that prohibit us from fully verifying the causal relationship between gut flora and linear development in children. Improving the gut microbiota, in conjunction with renutrition techniques, has the potential to ameliorate the growth and development impairments currently associated with chronic illness and malnutrition in children.
Introduction
Human development is divided into four distinct stages: fetal, infant, childhood, and adolescence. Each growth phase is governed by specific endocrine processes and is influenced by genetic, nutritional, and environmental factors (1). Growth retardation is defined as a problem in which individuals have significantly lower growth rates than healthy individuals, which is accompanied by metabolic disorders, systemic inflammation, or intestinal ecological dysregulation (2). After the age of two years, linear growth retardation caused by childhood malnutrition is largely irreversible (3). As a result, prevention or reversal of early interventions for factors contributing to growth retardation provides the best opportunity to improve outcomes (4). Growth retardation is the underlying cause of high morbidity and mortality in children under the age of five (5); it affects approximately 20% of children worldwide (2). Childhood malnutrition, which includes fetal growth restriction, growth retardation, wasting, vitamin A and zinc deficiency, and inadequate breastfeeding, has recently been estimated to cause 3.1 million child deaths per year, accounting for 45% of all child mortality (6). Infants with growth disorders are more likely to die from sepsis, pneumonia, diarrhea, and other infections, as well as from growth retardation (7). Long-term effects of infant malnutrition include short stature and low body weight, immune dysfunction and increased infection risk that persist into adulthood, cognitive impairment, poor academic performance, and decreased productivity in adulthood (8), and being less likely to reach their growth potential in adulthood (3).
Intestinal flora and human development
The gastrointestinal tract is home to trillions of microbes, known as the “gut microbiome”. Bacteria, fungi, archaea, viruses, and protozoa make up the vast majority of the normal human gut microbiota. Firmicutes and Bacteroidetes were the most abundant in the intestinal flora, while Actinomycetes, Verrucomicrobia, and Proteobacteria were less abundant. The gut microbiota has the potential to influence our physiology in both health and disease (9). Because of the relationship between diet, different physiological states, and the microbiota's ability to produce metabolites from dietary consumption, these important features of the gut microbiota drive research into the functional aspects of microbial diversity (10). Dysbiosis, or disruption of the normal balance between gut microbiota and host, has been linked to the development and progression of obesity and other metabolic disorders, such as diabetes, insulin resistance, and early features of metabolic syndrome, in which the microbiota, the immune system, metabolic pathways, and inflammatory and allergic processes may be involved. Early in infancy, the fecal microbiota is dynamic and can be classified into three separate phases: developmental (3–14 months), transitional (15–30 months), and stable (31–46 months) (9). The first microbial occupancy has a significant impact on the overall health of life. Probiotic supplementation early in life reduces the incidence of neuropsychiatric issues in infancy, according to a study that investigates how the gut microbiota may influence nervous system function (11). Within the first 6 months of life, 75 infants were randomly assigned to either Lactobacillus rhamnosus GG or a placebo and were observed for 13 years. At the age of 13, 17.1% of children in the placebo group had attention deficit hyperactivity disorder (ADHD) or Asperger's syndrome, but no children in the probiotic group did (11). Any disruption in the colonization process of the gut microbiome could have long-term consequences for the host's growth, development, and later health. Infants' length, weight, and head and chest circumferences all increase dramatically during this phase of rapid growth. As the gut microbiota matures three years after birth, the prenatal period and the first three years of life are regarded as critical times for the formation of microbial colonization patterns (9). One of the most critical goals of postnatal development is to acquire a gut microbiota capable of benefiting from functions in the environment while also establishing a mucosal immune system capable of tolerating preferred community members and suppressing pathogens. Initially, it was thought that this colonization began during the birth process, but a study describing the identification of bacterial DNA in the placentas of healthy term infants and the discovery of bacteria in the amniotic fluid and meconium of preterm infants confirmed that the fetal gut bacteria groups may have appeared earlier (12).
Many factors influence the development and maturation of the gut microbiota, including placental inflammation, maternal infection during pregnancy, pregnancy course, duration, type of delivery, perinatal conditions, hospital environment and length of stay, feeding methods, antibiotic use, life methods, and geographic factors (13–16). Growth retardation has been linked to a variety of causes, including microbiome dysbiosis, neuroinflammation, endocrine disruptions, starvation, maternal influence, and stress (17). Immature microbiomes interact with risk factors for growth retardation in a two-way fashion, with gut infections, nutrition, birth weight, and other factors both impacting and being influenced by the “growth-restricted” microbiome (2). Overall, improper gut microbiota establishment, particularly in the first two years of life, can affect growth trajectories. Some of the theorized mechanisms of how the gut microbiota influences body weight include increased food energy acquisition, fat deposition promotion, altered exercise activity, satiety effects, and systemic inflammation activation (18).
Gut microbiota and linear growth
The influence of gut flora on prenatal linear growth
Fetal growth and development throughout pregnancy are significantly influenced by the fetal environment and the exchange of the fetal-maternal interface. Problems with fetal growth may result from changes in the intestinal flora of the pregnant mother brought on by the mother's genetic make-up, diet throughout pregnancy, delivery method, etc. The findings of genome-wide association studies indicate that there are 12,111 distinct single-nucleotide polymorphisms that are significantly linked with height and are predicted to explain 40%–50% of the phenotypic variance in human height (19). According to studies, the gut microbiota of preterm neonates' siblings in Actinobacteria, Bacilli, Bacteroidia, Clostridia, Erysipelotrichia, and Negativicutes bacteria share significant similarities, implying genetic or shared maternal and environmental effects on the preterm infant gut microbiota (20). Different genetic backgrounds also have an impact on microbial composition, immunological response, and host metabolism, all of which are critical for growth and development. It was shown that some genes associated with growth retardation in genetically defective mice have a dysbiosis of the gut flora. Nod2-deficient mice, for example, lack apoptotic and antifungal responses, have low bacterial populations in the gut, and are vulnerable to pathogenic infections; Card9 knockout mice show hindgut flora dysbiosis (21).
Maternal stunting is a risk factor for low birth weight and subsequent childhood stunting in low- and middle-income countries, sustaining a vicious intergenerational cycle of starvation. This cycle has a negative impact on the child's survival, growth, and neurodevelopment (4). Maternal gut inflammation is linked to poor fetal growth and poor delivery outcomes (4). According to a study of 19 longitudinal birth cohorts, small-for-gestational-age newborns contribute for 20% of childhood stunting and 30% of childhood wasting globally (22). Maternal height was found to be inversely related to child stunting and overall child mortality in all low-income nations (23). This finding could be attributed to physical limits on fetal growth in smaller mothers, but other factors such as maternal inflammation, gut function, microbiota, and epigenetics could also play a role (4, 24). With an estimated 20% of stunting occurring in utero, intervention during the first few years of life may not be enough to prevent some of the most severe consequences of growth failure (25).
The diet of a mother during pregnancy and lactation influences the quantity of her microbiota, changing the bacterial repertoire that can be passed down to her kids during pregnancy and early life (26). The acquisition, composition, and microbial activity of the early newborn microbiota are influenced by maternal weight growth during pregnancy. Women who gained more weight during pregnancy had more bacterial diversity and richness than pregnant mothers who gained less weight. Infants born to moms more gestational weight were more likely to have a significant Bacteroidetes pattern and were less likely to have a Firmicutes dominant profile (27, 28). Several studies have confirmed the link between changes in maternal gut flora during childbirth and maternal gestational weight gain. Overweight moms' babies exhibited considerably higher amounts of fecal Bacteroides and Staphylococcus throughout the first 6 months. More Bacteroides, Clostridium, and Staphylococcus and fewer Bifidobacterium were related with higher maternal body weight and body mass index (BMI). Concentrations of Akkermansia muciniphila, Staphylococcus, and Clostridium were lower in infants born to normal-weight moms and mothers who reached normal gestational weight (29, 30).
In utero growth retardation is linked to maternal and placental inflammation and infection, as well as significant changes in host hormone levels, revealing a role for the microbiota-brain axis in fetal growth before birth (31). It is commonly acknowledged that vertical mother-to-infant microbiota transfer has a major impact on baby growth trajectories. Maintaining maternal microbial homeostasis is therefore crucial for preventing metabolic disruptions and growth deficits in children (32). Unfavorable factors, such as an unhealthy maternal diet during pregnancy, can have an effect on the mother's endocrine system and the acquisition of the infant's gut microbiota. One study revealed that a high-fat diet during pregnancy reduced bacterial colonization of the infant's gut and increased enterococci enrichment, with the impact lasting around a month after birth (33).
The manner of delivery, which transmits the neonatal gut microbiota and influences microbial composition, heredity, and function, is a fundamental driver of gut categorization in the first year of life. Vaginal birth has been demonstrated to enhance gut maturation and microbial variety, but cesarean surgery has been linked to gut microbiota acquired through maternal skin commensal bacteria. Bacteroides and Bifidobacterium were more abundant in infants born vaginally during the first three months of life, Lactobacillus and Bacteroides during the second three months of life, Bacteroides and Bifidobacterium during the second six months of life, and Bacteroides, Enterobacter, and Streptococcus after the first year of life. While infants born via cesarean section showed greater levels of Clostridium and Lactobacillus during the first three months of life, Enterococcus and Clostridium during the second three months of life, and Lactobacillus and Staphylococcus beyond the first year of life (34–36).
Preterm delivery, small-for-gestational age (SGA), or both cause approximately 20% of stunting (22). Preterm infants' initial gut microbiota differs from that of full-term newborns, and its microbiota composition is linked to changes in the composition of the mother's gut microbiota. A cross-sectional research of 55 preterm newborns discovered that preterm neonates had much lower gut microbiota alpha diversity and unique beta diversity clustering than term neonates. The contribution of maternal gut microbiota to first preterm gut colonization was greater after spontaneous delivery than after iatrogenic delivery and was not dependent on delivery mode (37). An Italian pilot study discovered that an increase ɑ-diversity levels, and hence a decrease in Lactobacillus in the vaginal environment, may be connected with an increased risk of spontaneous preterm birth (38). When compared to full-term infants, the microbiota of preterm infants is determined by the date of gestation, with decreased variety and increased abundance of potentially pathogenic bacteria and decreased abundance of beneficial bacteria such as Bifidobacterium (39). SGA infants frequently have difficult pregnancies and deliveries, and prenatal events can alter gut and immune system maturation, as well as impair microbial balance and succession. Furthermore, stressors associated with neonatal life in the hospital, such as frequent antibiotic usage, invasive procedures, and maternal separation, can all lead to dysbiosis (40). A small cohort study discovered that SGA newborns had smaller abundances of Klebsiella and Enterobacter than AGA infants, and the Beta diversity of bacterial community structure began to segregate at postnatal day 30 (41). Transcriptome investigations of the SGA rat model revealed that IGF-2 expression was considerably reduced in CUG (catch-up growth)-SGA rats, which was associated with a decrease in lactic acid bacteria (42). The gut microbiome influences SGA infants' long-term prognosis in addition to regulating intrauterine growth. The incidence of Neisseriaceae, mucosal-hemolytic bacteria known to absorb iron-bound host proteins including hemoglobin, was considerably greater in the placental microbiota of intrauterine growth restriction (IUGR) patients. Furthermore, the rise of anaerobic bacteria like Desulfovibrio represents the development of a hypoxic environment in the IUGR placenta (43). In SGA newborns, certain pathogenic and conditional pathogenic bacteria, such as Shigella, Ralstonia, and Clostridium, increased or became the dominant microbiota. Bacteroides fragilis and Clostridium saccharobutylicum were detected in SGA newborns and may be linked to neurodevelopmental outcomes at 6 months (44).
In summary, the maternal microbiota is directly linked to the health of the baby, and its disruption can result in fetal growth and development abnormalities such as premature birth, SGA newborns, and macrosomia. Genetic variables (20), nutrition before and during pregnancy (30), manner of birth (36), and gestational age at birth (41) all influence baby microbiome colonization. Lactobacillus and Bifidobacterium have been shown to promote fetal growth, but pathogenic bacteria such as Shigella, Ralstonia, and Clostridium have been found to inhibit baby growth (29, 39). Furthermore, particular early-life microbes such as Bacteroides fragilis and Clostridium saccharobutylicum are important for offspring brain development, which can affect baby health and long-term health (44). As a result, sensible treatments to change maternal or offspring microbiome from pregnancy to early childhood have significant implications for offspring health. However, current research on the gut microbiota of mothers and infants is primarily based on 16S RNA gene sequencing results. More research is needed to determine the actual mechanism of the effect on the microbiota and fetal growth, and there is still a long way to go before employing microbiota to interfere in fetal growth. There is still more to be discovered. The investigations on the effect of gut flora on prenatal linear development are summarized in Table 1.
Intestinal flora and early infant growth and development
Colonization of the newborn gut is thought to be vital for healthy growth because it influences gut maturation, metabolic, immunological, and brain development in early life. Microbiota interactions throughout infancy may be an important predictor of the host's long-term metabolic effects (45). Infants' early eating patterns and nutritional status are critical for the early molding of intestinal flora, and the interplay between intestinal flora and nutrition is critical for growth and development during infancy. In southern India, a longitudinal investigation of the gut microbiota of 10 infants with low birthweight and chronic stunting and 10 children with normal birthweight and no indications of stunting was done. From 3 months to 24 months of age, fecal samples were collected and examined every 3 months. The LEfSe algorithm was used to analyze differentially enriched taxa and found that the microbiota of stunted children was enriched in inflammatory bacteria from the Proteobacteria phylum, whereas the microbiota of normally developing children was enriched in probiotics, such as Bifidobacteria longum (12). A 6-year retrospective study of preterm children born at 35 weeks indicated that optimum postnatal nutrition enhanced early catch-up weight growth and improved linear development while having no influence on childhood BMI (46). According to a research of 108 healthy neonates in their first half year of life, breastfed newborns had more Lactobacillus, Bacteroides, and Bifidobacterium and less pathogens in their gut, which correlates to an accelerated rate of growth in infants (45). A significant decrease in the abundance of sialylated human milk oligosaccharides (HMOs) in human milk can result in severe growth failure in infants. Supplementation with sialylated HMOs promotes microbiota-dependent growth in stunted infants, possibly due to increased Bifidobacteriaceae abundance in infant gut (47). The amount of this oligosaccharide in the milk of malnourished mothers was reduced. The researchers discovered increased muscle mass, stronger bones, and significant changes in liver and brain metabolism when they administered oligosaccharides purified from whey to mice transplanted with stool from severely malnourished children. It implies that these findings have far-reaching implications and that controlling intestinal flora can affect children's nutritional status, but more clinical research is needed to back up these animal-based speculations (48). Microbial changes caused by weaning disruption delay intestinal barrier maturation and increase susceptibility to allergic inflammation, which may result in later growth retardation (49). Human breast milk contains a “different type of lactose” than cow's milk, which contains hundreds of oligosaccharides that promote Bifidobacteriales growth, according to research. Breastfeeding promotes the development of sensible and beneficial flora and assists babies in developing normally (50).
Poor infant hygiene and antibiotic-induced intestinal flora disruption are other key causes of linear growth disorder in newborns and early children. In Bangladeshi 2-years-old, small intestinal bacterial overgrowth (SIBO) was linked to poor hygiene, intestinal inflammation, and shorter length for age (51). Although no differences in intestinal permeability fecal markers were found in these SIBO-positive children, they did have elevated fecal calprotectin levels and were more likely to have growth retardation by the age of two (52). Evidence suggests that early-life antibiotic exposure is related to baby development and speed. One putative biological mechanism underpinning the effects of antibiotics on offspring development was structural and functional changes in the gut microbiota. A study from the Shanghai Mother-Child Pair Cohort examined 18 common antibiotics in meconium, including chlortetracycline, penicillin, and chloramphenicol, and used a multivariate linear regression model to examine antibiotic exposure, infant gut flora, and growth and development. Interdependence of indicators penicillin was discovered to have a negative relationship with gut microbiota Pielou and Simpson's index and a favorable relationship with growth velocity at 2–6 months (53). Another research investigating the long-term effects of neonatal and early childhood antibiotic exposure on child growth in an unselected birth cohort of 12,422 full-term infants discovered that males had significantly lower weight and height gains than girls during the first 6 years of life. Neonatal antibiotic exposure was linked to significant changes in the gut microbiome, notably a decrease in the number and diversity of fecal Bifidobacteriales before the age of two. Transplanting fecal microbiota from antibiotic-exposed children onto germ-free male mice resulted in substantial growth failure. Antibiotic exposure during pregnancy has been related to long-term changes in the gut microbiota, which may result in reduced growth in males during the first six years of life, according to this research (54). Another study revealed that early antibiotic exposure was not connected with enhanced growth velocity between delivery and discharge in neonates and infants in intensive care units inpatient antibiotics (55).
Linear growth disorder in infancy is connected with abnormal immunological inflammation and the disruption of gut flora. Prior to growth decrease, children with developmental delay had higher gut bacterial diversity and elevated inflammatory biomarkers, according to a longitudinal study of 78 Peruvian infants aged 5–12 months. Throughout the study, the fecal microbiota composition of stunted children was more diverse than that of healthy controls. Ruminococcus 1 and 2, Clostridium sensu stricto, and Collinsella abundance increased in stunted children but not in controls, but Providencia abundance dropped. The authors suggest that chronic, low levels of microbial translocation across the gastrointestinal mucosa may be the source of immune activation in children with developmental delays. However, because abnormalities in the gut microbiome exist prior to growth retardation, inflammatory chemicals derived by microbes may also contribute to chronic local irritation (56). Another study of 46 duodenal samples, 57 stomach samples, and 404 stool samples from stunted children aged 2–5 years in Africa found that the vast majority of stunted children exhibited gastrointestinal symptoms. In addition, Escherichia coli/Shigella sp. and Campylobacter sp. were shown to be more common in stunted children, although Clostridia, well-known butyrate makers, were reduced in comparison to nonstunted children. Oral bacteria were overrepresented in fecal samples from stunted children (57).
During infancy, there is a “critical window” for gut microbiota development, and disruptions in this process may be critical for children's growth and development (9, 16). Nutritional status (46), feeding techniques (47), hygienic conditions (51), antibiotic use (53), and intestinal inflammation (56) are major factors that induce intestinal flora alteration and impair children's growth and development, according to current clinical research. The majority of research support the identification of gut flora, such as Bifidobacterium, Bacteroides, and Lactobacillus, as being favorably associated with early baby growth. Actinomycetes, particularly Bifidobacteriales, are the most prevalent members of the gut microbiota in well-growing newborns (45, 47). However, research reports on the intestinal flora related with infant development retardation differ widely, owing to overgrowth of intestinal flora, overexpression of pathogenic bacteria, an abundance of Ruminococcus, Clostridium sensu stricto, and Collinsella, among other factors (51, 56). This may be related to the various research objects chosen by the researchers. In short, infancy is a vital stage for the creation of gut flora. Food influences the gut flora. The connection between nutritional status and gut flora is a significant area of interest in baby growth and development research. The clinical investigations on the effect of gut flora on early infant growth and development are summarized in Table 2.
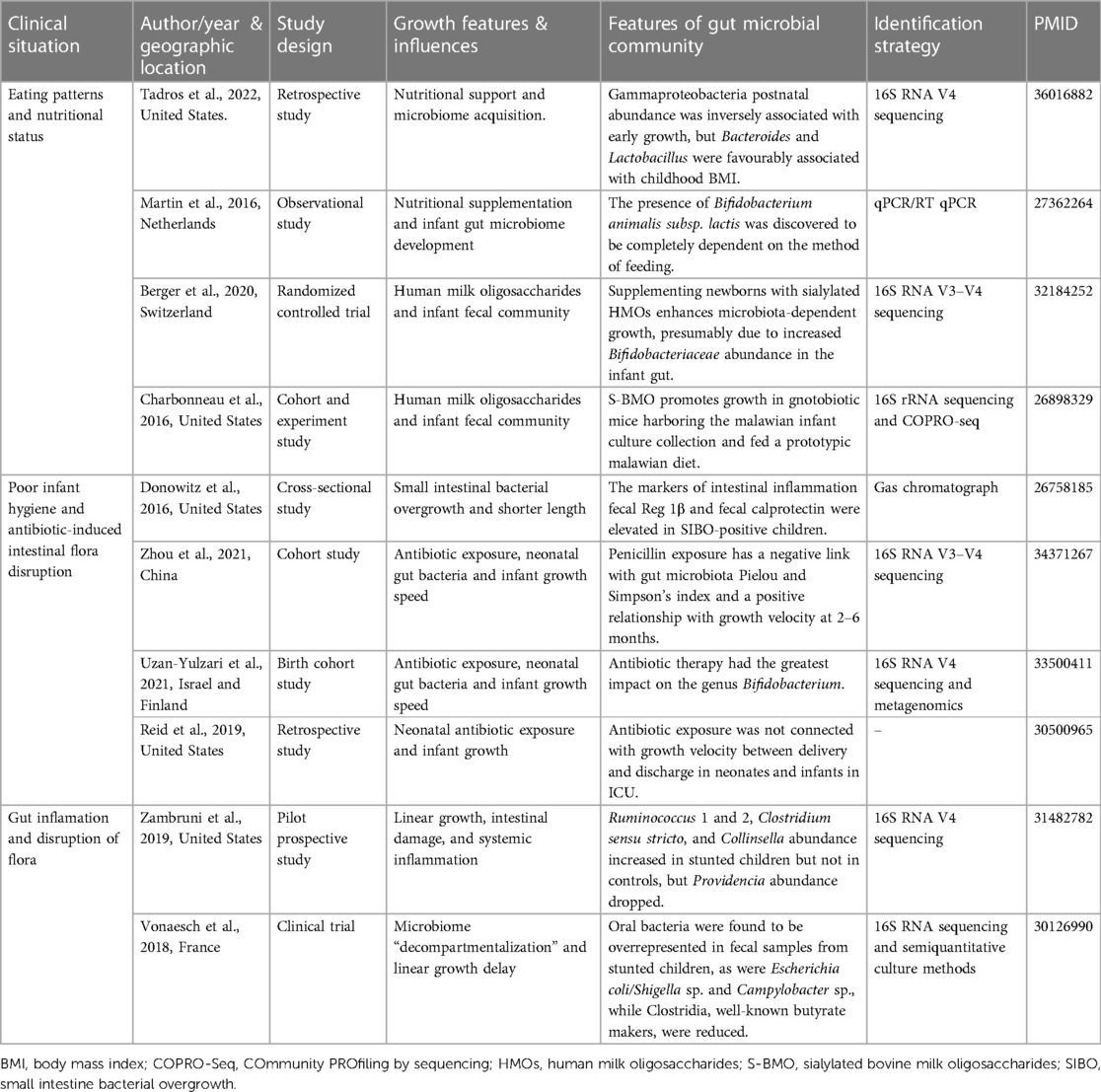
Table 2. Summarizes clinical studies on the effect of gut flora on early infant growth and development.
Adolescent linear growth and intestinal flora
Adolescence is a transitional period between childhood and maturity, a period of physical, neurological, psychological, and social changes, and a key time for growth and development. Some chronic mental illness states in childhood and adolescence, such as cognitive, emotional/social disturbances, sensory functional impairment, communication impairment, and so on, are intimately related to adolescents' growth and development. Although the pathophysiology of how psychiatric diseases such as anorexia nervosa (AN), ADHD, and autism spectrum disorder (ASD) affect growth and development in adolescents is not fully known, dysbiosis of the microbiota has been proposed as a possible explanation (18). AN is a severe psychiatric condition that primarily affects adolescents as a result of the severely detrimental consequences of caloric restriction on linear growth during puberty (58). A study of the composition and diversity of the gut microbiome in adolescents with anorexia before and after nutritional supplementation discovered that patients had greater individual differences in gut bacterial and metagenomic content, with fecal levels of serotonin, gamma-aminobutyric acid, dopamine, butyrate, and acetate decreasing in the samples (59). ADHD is one of the most frequent neurodevelopmental diseases in children. Numerous studies indicate that ADHD is linked to teenage growth and development (60). A Finnish study revealed that adolescents with hyperactive-impulsive ADHD were taller, and elementary school kids with ADHD were shorter and smaller than a control group of children of the same age (61). A case-control research comparing Chinese children with ADHD to healthy controls discovered that those with ADHD had lower levels of Faecalibacterium and Veillonellaceae, whereas Enterococcus and Odoribacter were significantly higher (62). Despite the fact that autistic children's gut microbiota differs significantly from that of normal children, with a reduced proportion of Coprococcus and Bifidobacterium, the children's height does not alter (63). In adolescence, there has been minimal research on height in children with ASD, and some studies have found that the ASD group was significantly larger than the control group in terms of head circumference, weight, and BMI, but there was no difference in height (64).
Nutritional status can influence linear bone growth during adolescence and puberty by regulating growth plate chondrocytes, which are critical components of juvenile growth (65). Excess body weight early in childhood might have an impact on growth patterns. There is evidence that excess adiposity throughout childhood alters growth patterns and pubertal development. Several studies have found that obese children have a higher height velocity and a faster bone age during their prepubertal years (66). Several hormones released by adipose tissue may influence linear growth in the context of obesity, both through the growth hormone insulin-like growth factor-1 (IGF-1) axis and directly through the epiphyseal growth plate. In 11 of the 12 cohort studies that were the subject of a systematic review and meta-analysis, there was a positive connection between total protein intake and BMI. The meta-analysis suggested a favorable relationship between total protein intake and BMI. There may also be evidence showing a connection between a higher intake of animal protein in the diet and a higher BMI. However, there is no clear evidence linking total protein intake with an elevated risk of being overweight or obese. Only suggestive data partially support this association between total protein intake and an elevated risk of being overweight or obese (67). The effect of nutrition on the gut flora during adolescence is linked to linear growth in puberty. In respect to linear growth, a study of 350 girls aged 12–13 years that followed three major dietary patterns: healthy, heavy in sugar and salt, and a Western diet found that a healthy dietary pattern with enough intake of plant protein and white meat was connected with more favorable linear growth (65). Diet and dietary components have a significant impact on the composition of the gut microbiota and are among the most important contributors to bacterial flora changes. Existing research suggests that adopting a plant-based diet benefits the host microbiome, reduces inflammation, improves insulin sensitivity, and promotes optimal energy balance, which can lead to the prevention of chronic low-inflammation-related diseases (68).
In comparison to the high prevalence of malnutrition in newborns and early children, obesity or overweight in adolescents has a greater influence on children's growth and development. Obesity with developmental delay is more common in younger children and adolescents than obesity without developmental delay. A research in Vietnam found that 5% of overweight children were also stunted, whereas a study in Sao Paulo, Brazil found that 6% of children in low-income urban families were overweight and stunted, and that obesity with stunting was more common than obesity without stunting. Similarly, growth retardation and increased obesity were shown to coexist in a study of young children in urban areas of the Cape Peninsula, South Africa, and researchers believe that the community has transitioned from undernutrition to overnutrition without reaching optimal nutritional status (69). A bioinformatically re-analyzed study based on published amplicon sequencing data from the National Center for Biotechnology Information discovered that the impacts of obesity on the gut microbiota may be more severe in infancy than in adulthood and eventually endure throughout life. The study discovered significant changes in gut microbiota between children with and without obesity, while no similar differences were seen in the adult group. Using gut microbiota to predict pediatric obesity is more difficult, according to random forest models, than adult obesity. The data show that the gut microbiota is more vulnerable in childhood than in maturity (70). A cross-sectional study of taxonomic characteristics of the gut microbiota in 46 children and their association with obesity in diet-dependent children discovered that children with an abundance of Holdemania spp. and high protein and complex carbohydrate consumption had a lower z-BMI, waist circumference, and hip circumference. In contrast, they found a link between Coprococcus catus and a low intake of this dietary pattern and hip circumference (71). Decades of observational research have revealed differences in the composition of the gut microbial community between obese and healthy people, and seminal studies in which fecal microbes from obese adults were transplanted into gnotobiotic mice recapitulate weight gain and obesity-related metabolic signatures, demonstrating a direct causal link between disrupted gut microbiota and obesity. Correction of flora issues appears to help prevent or treat obesity-related growth and metabolic disorders (72). However, clinical trials of microbiota-targeting treatments have had conflicting outcomes. A recent systematic review and meta-analysis (73) identified 19 trials comparing probiotics or synbiotics to any strategy other than bariatric surgery or FMT. Individual trials show no significant improvement in body weight, while combined case analyses show no differences in body weight or body mass index between probiotics or synbiotics and controls (73). Obese children mature more quickly than lean children, which increases the risk of poor adult height and early puberty. Despite the fact that obese children have a quicker linear growth rate, body obesity may promote neuroendocrine events that contribute to the start of puberty (74).
Precocious puberty, which can disrupt the gut microbiome, is another concern associated with linear growth in teenagers. According to a Korean study that investigated the composition of the gut microbial community in obese teenagers, the abundance of Bacteroides and Prevotella is strongly associated with BMI, and the composition of Bacteroides is adversely associated with triglycerides and total cholesterol (75). Dong et al. (76). discovered changes in the gut microbiota between individuals with idiopathic central precocious puberty (ICPP) and healthy girls. They discovered that the gastrointestinal genera found in ICPP are comparable to those linked to obesity, including Ruminococcus, Gemmiger, Oscillibacter, and Clostridium XIVb. In terms of microbial species, girls with ICPP had higher amounts of Rumicoccus bromii, Ruminococcus gnavus, and Ruminococcus leptum. The first two were discovered in obese people and were found to boost energy absorption and adipose tissue hyperplasia, whereas Ruminococcus leptum was found to influence human weight changes. These findings underscore the link between obesity, ICPP, and gut microbiome dysbiosis (32, 77). Another study discovered that the gut flora of central precocious puberty patients behaves similarly to that of other neurological illnesses, with an abundance of Alistipes, Klebsiella, and Sutterella. These microbes create neurotransmitter-like metabolites (serotonin and dopamine), which initiate early puberty and activate the hypothalamic-pituitary-gonadal axis (78). Although accurate estimates of height loss due to premature puberty are difficult to obtain, prior studies of untreated patients revealed an average height loss of 10 cm in girls and 20 cm in boys. Girls who receive gonadotropin-releasing hormone analog (GnRHa) treatment before the age of 6 years, on the other hand, can achieve a final height gain of 2–10 cm (79, 80). When compared to Tanner stage-matched controls, girls with true central precocious puberty show an adverse metabolic profile at diagnosis; even GnRHa treatment cannot correct this shortfall (81). The investigations on the effect of gut flora on adolescent linear growth are summarized in Table 3.
Key bacterial taxa associated with linear growth at different stages of childhood
Evidence suggests that certain aspects of the gut microbiota are linked to certain stages of growth and development in children (18). Figure 1 depicts the characteristics of intestinal flora at various phases of children's growth and development, as well as the impact of intestinal flora on linear growth in children. The oral cavity (31, 82), vagina (36), and intestines (37) of the mother are major sources of neonatal intestinal flora. Hematogenous transfer of maternal oral bacteria during pregnancy may be an important factor in placental colonization (31, 83). Evidence suggests that patients who gave birth prematurely had a distinct gut microbiome dysbiosis compared to those who gave birth at term. Porphyromonas, Streptococcus, Fusobacterium, and Veillonell a were enriched in the preterm group, whereas Coprococcus and Gemmiger were significantly depleted. The majority of the enriched bacteria were oral bacteria that had been annotated (82). The mother's gut and the maternal vagina are the principal sources of microbiota for vaginally delivered newborns (84). The microbiome of newborns born via cesarean section is mostly derived from the mother's skin and the hospital environment (85). The most important factors influencing prenatal growth and development are prenatal weight gain (28–30), premature birth (31, 38, 39), and being small for gestational age (41, 44). According to research, these elements are linked to the creation and features of newborn gut flora. As illustrated in Figure 1, Lactobacillus and Bifidobacterium are advantageous to newborn growth throughout the perinatal period, however pathogenic bacteria such as Shigella, Ralstonia, and Clostridium may be detrimental. During infancy, nutritional status, feeding procedures, hygienic conditions, antibiotic usage, and intestinal inflammation are important factors that affect intestinal flora and impede children's growth and development. As illustrated in Figure 1, gut flora such as Bifidobacterium, Bacteroides, and Lactobacillus are favorably associated with infant growth. Pathogenic bacterial overexpression, as well as an abundance of Ruminococcus, Clostridium sensu stricto, and Collinsella, are all unfavorable factors. Infants' gut flora has stabilized between 31 and 46 months, hence children's growth rate tends to be constant and relatively slow from infancy until pre-adolescence (9). Adolescence is characterized by a succession of physical, neurological, psychological, and social changes, as well as the second growth spurt in life. Microbiota abnormalities associated with children's growth and development during this period are mostly associated with some particular diseases such as AN (59), ADHD (62), ASD (63), precocious puberty (76, 78), and obesity (71). Alistipes, Clostridiales, Christensenellaceae, and Ruminococcaceae abundance was linked to AN (59). ADHD was linked to an abundance of Bacteroides caccae, Odoribacter splanchnicus, Paraprevotella xylaniphila, and Veillonella parvula (62). Bacteroides, Parabacteroides, Clostridium, Faecalibacterium, and Phascolarctobacterium are more abundant in the gut of ASD children (63).
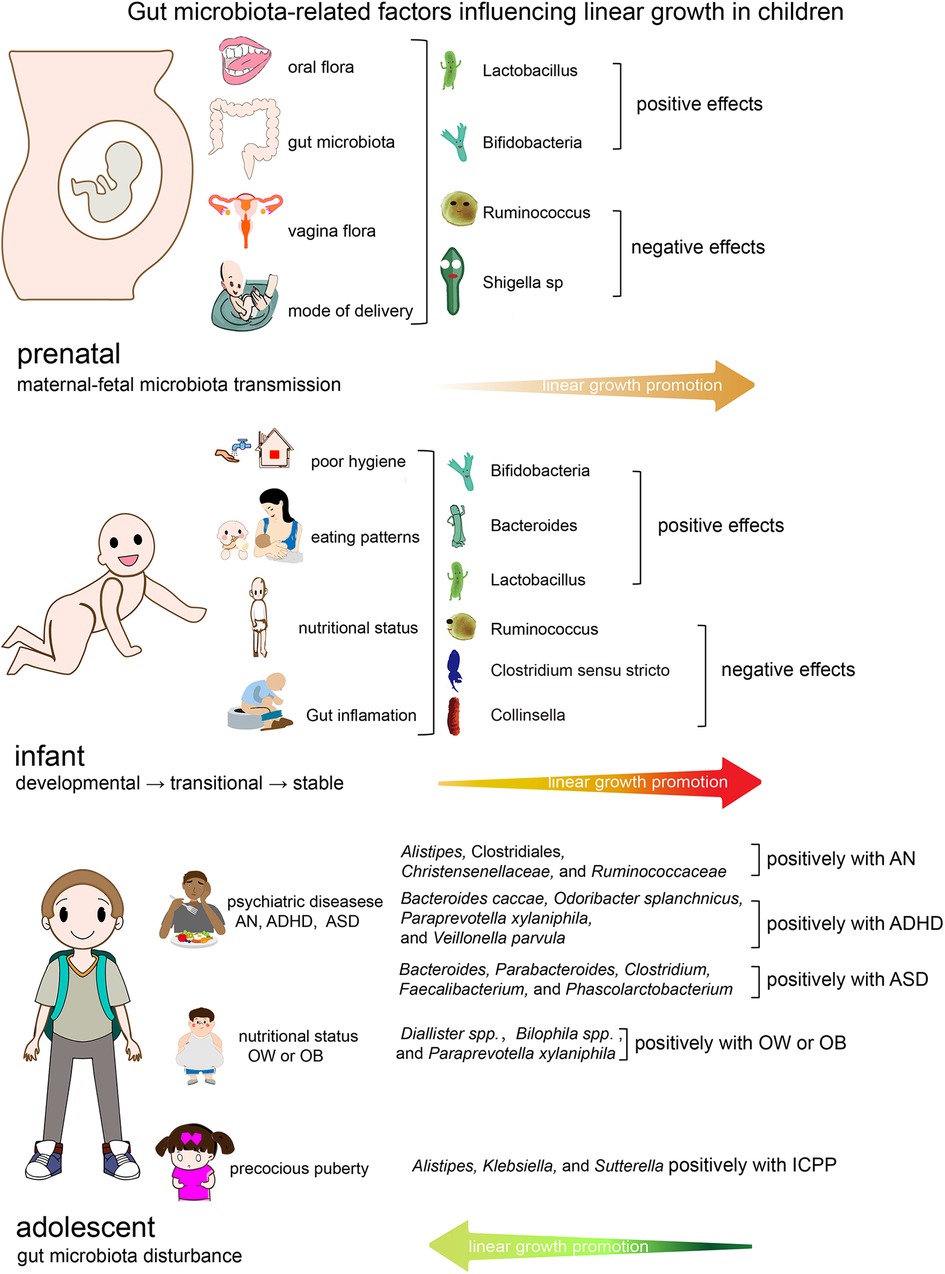
Figure 1. Gut microbiota-related factors influencing linear growth in children. OW/OB, overweight/obese; ADHD, attention-deficit/hyperactivity disorder; AN, anorexia nervosa; ASD, Autism spectrum disorder.
Possible mechanism of gut microbiota affecting linear growth
The anterior pituitary gland secretes growth hormone, which regulates insulin-like growth factor-1 (IGF-1) synthesis. One of the most fascinating theories that could explain the association between gut microbiota composition and linear and bulky growth in children is the involvement of gut microorganisms in boosting growth hormone. The gut microbiota can influence linear growth by influencing growth axis activity and changing hormone release. It could be one of the key methods by which the gut flora regulates linear development by modulating the action of the GH (growth hormone)/IGF-1 axis. Children who have chronic nutritional deficiencies develop growth hormone resistance and become stunted. The liver and peripheral tissues, including muscle, create IGF-1 which promotes growth throughout the body and organs. IGF-1 is a key mediator of bone growth that works in the endocrine, paracrine, and autocrine systems (86). In one mice investigation, researchers demonstrated that Lactobacillus plantarum strains in the gut microbiota maintain growth hormone action via signaling pathways in the liver, overcoming malnutrition-induced GH resistance. According to this research, certain beneficial bacteria can be employed to treat stunted growth and development caused by malnutrition (87). A study of Drosophila revealed that a common bacterium, Acetobacter pomorum, can modulate insulin/insulin-like growth factor signaling and hence influence Drosophila developmental speed, body size, and energy metabolism (88). On the one hand, excess short-chain fatty acids (SCFAs) produced by a specific benign bacteria provide an additional source of energy, resulting in an imbalance in energy regulation that promotes growth. SCFAs, on the other hand, have a role in glucose-stimulated insulin secretion from cells through interactions with the free fatty acid receptors FFA2 and FFA3, as well as the release of hunger-regulating peptide hormones. This seemingly contradictory finding demonstrates that, in addition to the SCFA levels produced by some gut microbiota members, some G-protein-coupled receptors (GPCRs) in the host can directly identify specific bacterial components, thereby controlling other metabolic processes that may contribute to body growth (10). In obese or overweight patients, a 12-week intervention with a low-carbohydrate diet (LCD) resulted in a considerable increase in the relative abundance of butyrate-producing bacteria, including Parabacteroides and Oscillospira. Furthermore, in the LCD group, participants with higher relative abundance of Bacteroides at baseline responded better to the LCD intervention and had greater weight loss outcomes. Some Oscillospira species may produce considerable levels of SCFAs, which aid in weight management as well as glucose and lipid homeostasis. Another notion is that Oscillospira can disrupt host glycans, allowing hosts to expend metabolic energy to restore destroyed glycoproteins. In this study, the increased abundance of Parabacteroides and Oscillospira could be a gut microbiota response to dietary intervention, assisting in LCD weight loss (89). Children who grow up in polluted surroundings are more likely to get intestinal infections and malnutrition, and they are more likely to develop overweight/obesity and accompanying comorbidities later in life. When exposed to energy-rich meals, children who had height deficits in childhood were more likely to be overweight or obese as adults (90). To summarize, numerous studies have demonstrated that gut flora is closely associated to obesity, and that changing gut flora may be an essential factor in weight control. A randomized clinical trial of adolescent obesity patients discovered that fecal microbiota transplantation (FMT) had no effect on weight loss but did ameliorate metabolic abnormalities (91). The most important link between and host IGF-1 levels appears to be SCFAs metabolized by gut bacteria from indigestible fiber-rich diets, but other mechanisms by which gut microbes influence bone growth may exist (92).
Another potentially growth-promoting mechanism for gut bacteria is the tryptophan (TRP)-kynurenine (KYN) -niacin pathway. In this process, indoleamine 2,3-dioxygenase (IDO) converts dietary tryptophan to kynurenine, which is then converted to niacin. Niacin is an important precursor of nicotinamide adenine dinucleotide (NAD+). Children with a greater kynurenine to tryptophan ratio (KT) had lower linear growth, according to studies, and experimental animal models have also indicated that a tryptophan deficit is associated with decreased growth velocity (93, 94). Undernutrition was found to affect various metabolic pathways, including choline and tryptophan metabolism, while also increasing the proteolytic activity of the gut flora. Additionally, metabolic adaptation to lower energy expenditure was observed in malnourished children, as demonstrated by increased N-methylnicotinamide and decreased -aminoisobutyric acid excretion. Undernourished children with stronger metabolic adaptability demonstrated quick catch-up growth many months early (95). The KYN: TRP/ KT ratios were shown to be strongly linked with children's growth in a study of stunted children in Bangladesh. This change in the TRP pathway could be due to environmental stresses such as chronic inflammation, environmental enteric dysfunction, inadequate protein consumption, or alterations in the gut microbiota, all of which can have a substantial impact on growth (96).
Furthermore, Gut microbiota may prompt immune cells to release certain cytokines, influencing the host's linear growth (56, 97, 98), and greater intestinal permeability produced by environmental intestinal dysfunction is hypothesized to cause higher bacterial product translocation, culminating in systemic inflammation and immunological activation (99). Chronic inflammatory illnesses, such as inflammatory bowel disease and juvenile idiopathic arthritis, are a major cause of stunted growth and development in children (100, 101). Systemic inflammation and immune activation may play a role in the development of linear growth disorders via cytokine-induced anorexia, nutrient utilization regulation, and/or interference with the growth hormone axis and bone metabolism (99). In a Zimbabwe birth cohort study, Prendergast et al. discovered that developmental delay was related to raised systemic inflammatory markers (C-reactive protein and 1-acid glycoprotein) and lower IGF-1 levels. The presence of intestinal injury in infants with developmental delays was demonstrated by higher plasma intestinal fatty acid-binding protein levels (102). Saari et al. discovered that children with Helicobacter pylori infection had low serum acylated ghrelin and growth retardation in a one-year longitudinal cohort study. Helicobacter pylori eradication successfully restores ghrelin levels and increases growth in children (103). Saari et al. found the same link between antibiotics and height in a large cohort of Finnish infants (104), and a meta-analysis of 10 randomized controlled trials found that antibiotic use increased height by 0.04 cm/month (105). However, in Niger, a large-scale study of azithromycin use and growth and development in children aged 6–60 months noticed no link between antibiotic use and improved human growth (106). Kosek et al. observed that kids with higher levels of fecal biomarkers of intestinal inflammation and intestinal barrier disruption were more likely to have linear growth retardation during the first 18 months of life in an international multicenter prospective study (107). Current studies (17, 104–107) on antibiotics and children's growth vary significantly, and there are many reasons for some of the differences, but the influence of environmental factors, which include the nutritional status of the children included in the studies, hygiene, feeding practices, drinking water, chronic inflammatory diseases, endocrine disorders, and so on, must all be considered.
In summary, the determinants of stunting operate at multiple causal levels, ranging from the most distal socioeconomic and political variables to the most proximal, such as food quantity and quality, as well as their biotransformation by the gut microbiota, host infection, immune dysfunction, and systemic physiology (108). Figure 2 shows a schematic representation of the gut microbiota's contribution to children's linear growth. The potential mechanisms include: (1) alterations in food absorption (10, 47) (i.e., increased levels of health-promoting microbial metabolites such SCFAs and HMOs); and (2) endocrine hormones (86) (GH/IGF-1 axis). (3) Changes in immune regulation (56, 97) (including cytokines and gut inflammation); (4) Control of the gut-brain-bone axis (94, 96) (including neurotransmitters, appetite control, and Kyn/Trp).
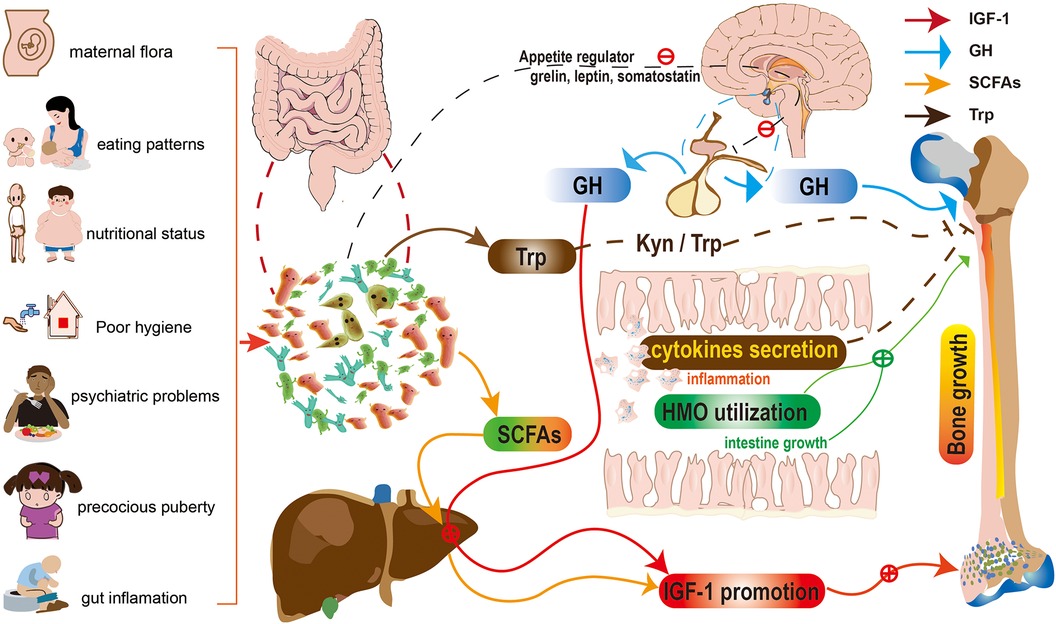
Figure 2. Schematic representation for the role of gut microbiota in children's linear growth. The potential mechanisms include: (1) alterations in food absorption (i.e., increased levels of health-promoting microbial metabolites such SCFAs and HMOs); and (2) endocrine hormones (GH/IGF-1 axis). (3) Changes in immune regulation (including cytokines and gut inflammation); (4) Control of the gut-brain-bone axis (including neurotransmitters, appetite control, and Kyn/Trp). HMOs, human milk oligosaccharides; SCFAs, Short-chain fatty acids; Kyn/Trp, kynurenine to tryptophan ratio; GH, growth hormone; IGF-1 insulin-like growth factor 1.
Conclusion and future perspectives
Microbiome research is still in its early stages and has mostly concentrated on bacterial taxa, but the microbiome also contains the virome and fungiome. The convergence of the microbiome and metabolic window omics is an area with potential for optimization, particularly during important developmental phases. Potential modifications include taking probiotic supplements, reducing antibiotic exposure, and using the microbiome as a biomarker for precision treatment. These pathways need to be investigated further, and while there are many extensive multi-omics studies in the adult literature, pediatric data is limited. To examine the makeup and impact of changes in the gut microbiome, clinical trials, particularly in large cohorts of healthy children and children with various disorders, are required. Moreover, geography and food culture have an impact on the gut flora in children. Depending on the research subjects chosen and the research methodologies employed, various countries and regions may reach different findings. Table 4 illustrates the clinical studies on intestinal microbiota and child growth in this review based on the cities/countries where the research was conducted, as well as the research methodology used, characteristics of the included populations, and main conclusions. Currently, the countries and regions undertaking study on this topic are primarily centered in Europe (28–30, 37, 38, 45, 47, 54, 57), the United States (33, 43, 46, 48, 51, 56), and China (39, 44, 53, 62, 76), with Africans, Bangladeshis, Europeans, and Chinese being the primary research subjects recruited. The primary areas of study are premature birth, intrauterine growth retardation, environmental sanitation, malnutrition, and so on. Chinese researchers are more interested in precocious puberty. According to the existing research literature, the association between gut microbiota and adolescent linear growth has received insufficient attention, and clinical research publications are limited. Furthermore, a better understanding of the relationships between the diverse microbiota and their components (bacteria, viruses, and fungi) is required (12). However, the mechanisms by which factors like maternal diet during pregnancy influence the microbiome of the offspring remain unknown. The vast majority of studies have been conducted in rodent models, with only a few mechanistic research studies conducted in humans. As a result, more validation is required before proceeding to clinical studies. Furthermore, there can be significant inter-individual variation in microbiota composition and drug responsiveness, meaning that conventional microbiota treatment may not be appropriate for every stunted patient. In phenotypically healthy populations, the use of multi-omics approaches such as metagenomics, metatranscriptomics, metaproteomics, and metabolomics aids in the discovery of microbial signals of growth retardation (2). Our present understanding of the gut microbiome is based on phylogenetic makeup (16S rRNA sequencing) or functional capacity (shotgun sequencing and identification of genes involved in metabolic pathways). Neither method fully captures trends in molecular crosstalk (caused by metabolites, antigens, signaling molecules, immunomodulators, and hormones) between the host and bacteria, resulting in systemic impacts on metabolism and the immune system. By including transcriptomics and metabolomics in the study of the dystrophic microbiota, researchers will gain a better understanding of how imbalances emerge. The majority of existing epidemiologic studies of malnutrition and gut microbiota are based on associations or correlations, making it impossible to determine the temporal order of these connections. To some extent, these problems can be addressed through longitudinal birth cohort studies of children or animal research. However, new and inventive ways are required to overcome these difficulties (12). With increased awareness and understanding comes the prospect of novel pharmaceutical targets and avenues for treating these diseases and promoting human health at all stages and ages (109).
Limitations
The purpose of this study is to review the establishment and alterations of the gut microbiota in children at various phases of development, as well as the impact on children's linear growth. We mainly talked about the association between growth and development disorders at various stages and changes in gut flora, but we also briefly discussed the connection between gut flora and nutrition, neurophysiology, endocrine, and immune inflammation in children at various growth and development stages. They have complicated interactions with each other. This review mostly includes cohort studies (26, 28, 30, 39, 41, 44, 45, 48, 53, 54, 56, 59), cross-sectional studies (31, 37, 46, 51, 71, 76, 78), case-control studies (29, 47, 55, 57, 62), and some animal studies focusing on particular mechanisms (48, 87, 88). Although the data supports a link between intestinal flora and linear development in children, our review has limitations that prohibit us from fully verifying the causal relationship between gut flora and linear development in children. First, there is a bidirectional association between changes in the gut microbiota and the risk/resilience of children to linear growth disorders, making it difficult to separate between cause and effect. The cohort and cross-sectional studies included in this review were limited to account for the complexities of gut microbiome affects on linear growth in humans due to sample size and study scope limitations. Second, to determine the causal link between the gut microbiota and linear growth, long-term clinical controlled trials with large sample sizes are required. However, due to the limits of currently available 16S RNA sequencing or metagenomics-based research methodologies, determining which species or groups of gut bacteria play a key role in addressing growth problems, even in animal studies, is difficult. As a result, the clinical research included in this review primarily used commercial probiotic or prebiotic supplements as treatment options, and the findings of these studies do not yet indicate which strains are relevant for resolving growth deficiency in which situations. Furthermore, the goal of this literature review is to provide readers with up-to-date and thorough research advances on the association between gut microbiota and linear growth in children. Although relevant studies are categorised and described, it is a general literature review with limits in how to evaluate a set of studies and make appropriate recommendations, which is inferior to the evaluation effect of a systematic review. In addition, we included research material on systematic reviews (63), meta-analyses (73), and biological data mining investigations (70) in this review. However, because of the scarcity of existing study data and a small number of research projects engaged, these studies have limited interpretation of the association between gut microbiota and linear development in children. In short, due to the limitations of current research, our review can only attempt to educate readers of the most recent findings in key subject areas. When the relevant research literature is substantial, a systematic review study on this topic can be done in the future to compensate for the deficiencies of this review.
Author contributions
PH and XS did the literature review and drafted the text. SG gathered the literature, wrote, and revised the manuscript. All authors contributed to the article and approved the submitted version.
Funding
This work was financially supported by the Program of Science and Technology Commission of Shanghai Municipality (No. 20Y11905300).
Acknowledgments
The authors would like to thank Qixuan Guo (Shanghai Yichuan Senior High School) for her assistance in drawing the figures.
Conflict of interest
The authors declare that the research was conducted in the absence of any commercial or financial relationships that could be construed as a potential conflict of interest.
Publisher's note
All claims expressed in this article are solely those of the authors and do not necessarily represent those of their affiliated organizations, or those of the publisher, the editors and the reviewers. Any product that may be evaluated in this article, or claim that may be made by its manufacturer, is not guaranteed or endorsed by the publisher.
References
1. Schei K, Simpson MR, Avershina E, Rudi K, Oien T, Juliusson PB, et al. Early gut fungal and bacterial microbiota and childhood growth. Front Pediatr. (2020) 8:572538. doi: 10.3389/fped.2020.572538
2. Qi M, Tan B, Wang J, Liao S, Deng Y, Ji P, et al. The microbiota-gut-brain axis: a novel nutritional therapeutic target for growth retardation. Crit Rev Food Sci. (2022) 62:4867–92. doi: 10.1080/10408398.2021.1879004
3. de Onis M, Branca F. Childhood stunting: a global perspective. Matern Child Nutr. (2016) 12(Suppl 1):12–26. doi: 10.1111/mcn.12231
4. Cowardin CA, Syed S, Iqbal N, Jamil Z, Sadiq K, Iqbal J, et al. Environmental enteric dysfunction: gut and microbiota adaptation in pregnancy and infancy. Nat Rev Gastroenterol Hepatol. (2023) 20(4):223–37. doi: 10.1038/s41575-022-00714-7
5. Muller O, Krawinkel M. Malnutrition and health in developing countries. Can Med Assoc J. (2005) 173:279–86. doi: 10.1503/cmaj.050342
6. Black RE, Victora CG, Walker SP, Bhutta ZA, Christian P, de Onis M, et al. Maternal and child undernutrition and overweight in low-income and middle-income countries. Lancet. (2013) 382:427–51. doi: 10.1016/S0140-6736(13)60937-X
7. McDonald CM, Olofin I, Flaxman S, Fawzi WW, Spiegelman D, Caulfield LE, et al. The effect of multiple anthropometric deficits on child mortality: meta-analysis of individual data in 10 prospective studies from developing countries. Am J Clin Nutr. (2013) 97:896–901. doi: 10.3945/ajcn.112.047639
8. Gwela A, Mupere E, Berkley JA, Lancioni C. Undernutrition, host immunity and vulnerability to infection among young children. Pediatr Infect Dis J. (2019) 38:e175–7. doi: 10.1097/INF.0000000000002363
9. Stewart CJ, Ajami NJ, O'Brien JL, Hutchinson DS, Smith DP, Wong MC, et al. Temporal development of the gut microbiome in early childhood from the TEDDY study. Nature. (2018) 562:583–8. doi: 10.1038/s41586-018-0617-x
10. Murugesan S, Nirmalkar K, Hoyo-Vadillo C, Garcia-Espitia M, Ramirez-Sanchez D, Garcia-Mena J. Gut microbiome production of short-chain fatty acids and obesity in children. Eur J Clin Microbiol. (2018) 37:621–5. doi: 10.1007/s10096-017-3143-0
11. Partty A, Kalliomaki M, Wacklin P, Salminen S, Isolauri E. A possible link between early probiotic intervention and the risk of neuropsychiatric disorders later in childhood: a randomized trial. Pediatr Res. (2015) 77:823–8. doi: 10.1038/pr.2015.51
12. Kane AV, Dinh DM, Ward HD. Childhood malnutrition and the intestinal microbiome. Pediatr Res. (2015) 77:256–62. doi: 10.1038/pr.2014.179
13. Dominguez-Bello MG, Costello EK, Contreras M, Magris M, Hidalgo G, Fierer N, et al. Delivery mode shapes the acquisition and structure of the initial microbiota across multiple body habitats in newborns. Proc Natl Acad Sci U S A. (2010) 107:11971–5. doi: 10.1073/pnas.1002601107
14. Brooks B, Olm MR, Firek BA, Baker R, Geller-McGrath D, Reimer SR, et al. The developing premature infant gut microbiome is a major factor shaping the microbiome of neonatal intensive care unit rooms. Microbiome. (2018) 6:112. doi: 10.1186/s40168-018-0493-5
15. Pabst O, Cerovic V, Hornef M. Secretory IgA in the coordination of establishment and maintenance of the microbiota. Trends Immunol. (2016) 37:287–96. doi: 10.1016/j.it.2016.03.002
16. Korpela K, de Vos WM. Early life colonization of the human gut: microbes matter everywhere. Curr Opin Microbiol. (2018) 44:70–8. doi: 10.1016/j.mib.2018.06.003
17. Wells JC, Sawaya AL, Wibaek R, Mwangome M, Poullas MS, Yajnik CS, et al. The double burden of malnutrition: aetiological pathways and consequences for health. Lancet. (2020) 395:75–88. doi: 10.1016/S0140-6736(19)32472-9
18. Ronan V, Yeasin R, Claud EC. Childhood development and the microbiome-the intestinal microbiota in maintenance of health and development of disease during childhood development. Gastroenterology. (2021) 160:495–506. doi: 10.1053/j.gastro.2020.08.065
19. Yengo L, Vedantam S, Marouli E, Sidorenko J, Bartell E, Sakaue S, et al. A saturated map of common genetic variants associated with human height. Nature. (2022) 610:704–12. doi: 10.1038/s41586-022-05275-y
20. Lim SJ, Aguilar-Lopez M, Wetzel C, Dutra S, Bray V, Groer MW, et al. The effects of genetic relatedness on the preterm infant gut microbiota. Microorganisms. (2021) 9(2):278. doi: 10.3390/microorganisms9020278
21. Rehman A, Sina C, Gavrilova O, Hasler R, Ott S, Baines JF, et al. Nod2 is essential for temporal development of intestinal microbial communities. Gut. (2011) 60:1354–62. doi: 10.1136/gut.2010.216259
22. Christian P, Lee SE, Donahue AM, Adair LS, Arifeen SE, Ashorn P, et al. Risk of childhood undernutrition related to small-for-gestational age and preterm birth in low- and middle-income countries. Int J Epidemiol. (2013) 42:1340–55. doi: 10.1093/ije/dyt109
23. Ozaltin E, Hill K, Subramanian SV. Association of maternal stature with offspring mortality, underweight, and stunting in low- to middle-income countries. JAMA. (2010) 303:1507–16. doi: 10.1001/jama.2010.450
24. Addo OY, Stein AD, Fall CH, Gigante DP, Guntupalli AM, Horta BL, et al. Maternal height and child growth patterns. J Pediatr. (2013) 163:549–54. doi: 10.1016/j.jpeds.2013.02.002
25. Prendergast AJ, Humphrey JH. The stunting syndrome in developing countries. Paediatr Int Child Health. (2014) 34:250–65. doi: 10.1179/2046905514Y.0000000158
26. Chu DM, Meyer KM, Prince AL, Aagaard KM. Impact of maternal nutrition in pregnancy and lactation on offspring gut microbial composition and function. Gut Microbes. (2016) 7:459–70. doi: 10.1080/19490976.2016.1241357
27. de Cuevillas B, Milagro FI, Tur JA, Gil-Campos M, de Miguel-Etayo P, Martinez JA, et al. Fecal microbiota relationships with childhood obesity: a scoping comprehensive review. Obes Rev. (2022) 23(Suppl 1):e13394. doi: 10.1111/obr.13394
28. Aatsinki AK, Uusitupa HM, Munukka E, Pesonen H, Rintala A, Pietila S, et al. Gut microbiota composition in mid-pregnancy is associated with gestational weight gain but not prepregnancy body mass Index. J Womens Health. (2018) 27:1293–301. doi: 10.1089/jwh.2017.6488
29. Collado MC, Isolauri E, Laitinen K, Salminen S. Effect of mother’s weight on infant’s microbiota acquisition, composition, and activity during early infancy: a prospective follow-up study initiated in early pregnancy. Am J Clin Nutr. (2010) 92:1023–30. doi: 10.3945/ajcn.2010.29877
30. Stanislawski MA, Dabelea D, Wagner BD, Sontag MK, Lozupone CA, Eggesbo M. Pre-pregnancy weight, gestational weight gain, and the gut microbiota of mothers and their infants. Microbiome. (2017) 5:113. doi: 10.1186/s40168-017-0332-0
31. Prince AL, Ma J, Kannan PS, Alvarez M, Gisslen T, Harris RA, et al. The placental membrane microbiome is altered among subjects with spontaneous preterm birth with and without chorioamnionitis. Am J Obstet Gynecol. (2016) 214:627.e1–e16. doi: 10.1016/j.ajog.2016.01.193
32. Neuman H, Debelius JW, Knight R, Koren O. Microbial endocrinology: the interplay between the microbiota and the endocrine system. FEMS Microbiol Rev. (2015) 39:509–21. doi: 10.1093/femsre/fuu010
33. Chu DM, Antony KM, Ma J, Prince AL, Showalter L, Moller M, et al. The early infant gut microbiome varies in association with a maternal high-fat diet. Genome Med. (2016) 8:77. doi: 10.1186/s13073-016-0330-z
34. Dedrick S, Sundaresh B, Huang Q, Brady C, Yoo T, Cronin C, et al. The role of gut microbiota and environmental factors in type 1 diabetes pathogenesis. Front Endocrinol. (2020) 11:78. doi: 10.3389/fendo.2020.00078
35. Lundgren SN, Madan JC, Emond JA, Morrison HG, Christensen BC, Karagas MR, et al. Maternal diet during pregnancy is related with the infant stool microbiome in a delivery mode-dependent manner. Microbiome. (2018) 6:109. doi: 10.1186/s40168-018-0490-8
36. Shaterian N, Abdi F, Ghavidel N, Alidost F. Role of cesarean section in the development of neonatal gut microbiota: a systematic review. Open Med (Wars). (2021) 16:624–39. doi: 10.1515/med-2021-0270
37. Hiltunen H, Collado MC, Ollila H, Kolari T, Tolkko S, Isolauri E, et al. Spontaneous preterm delivery is reflected in both early neonatal and maternal gut microbiota. Pediatr Res. (2022) 91:1804–11. doi: 10.1038/s41390-021-01663-8
38. Tirone C, Paladini A, De Maio F, Tersigni C, D'Ippolito S, Di Simone N, et al. The relationship between maternal and neonatal microbiota in spontaneous preterm birth: a pilot study. Front Pediatr. (2022) 10:909962. doi: 10.3389/fped.2022.909962
39. Jia Q, Yu X, Chang Y, You Y, Chen Z, Wang Y, et al. Dynamic changes of the gut microbiota in preterm infants with different gestational age. Front Microbiol. (2022) 13:923273. doi: 10.3389/fmicb.2022.923273
40. Groer MW, Gregory KE, Louis-Jacques A, Thibeau S, Walker WA. The very low birth weight infant microbiome and childhood health. Birth Defects Res C Embryo Today. (2015) 105:252–64. doi: 10.1002/bdrc.21115
41. Chang HY, Chiang CJ, Chang JH, Hsu CH, Lin CY, Ko MH, et al. Characteristics of gut microbiota in small for gestational age infants with very low birth weight. Nutrients. (2022) 14(23):5158. doi: 10.3390/nu14235158
42. An J, Wang J, Guo L, Xiao Y, Lu W, Li L, et al. The impact of gut microbiome on metabolic disorders during catch-up growth in small-for-gestational-age. Front Endocrinol. (2021) 12:630526. doi: 10.3389/fendo.2021.630526
43. Hu J, Benny P, Wang M, Ma Y, Lambertini L, Peter I, et al. Intrauterine growth restriction is associated with unique features of the reproductive microbiome. Reprod Sci. (2021) 28:828–37. doi: 10.1007/s43032-020-00374-5
44. Chen X, Yan Z, Liu L, Zhang R, Zhang X, Peng C, et al. Characteristics of gut microbiota of term small gestational age infants within 1 week and their relationship with neurodevelopment at 6 months. Front Microbiol. (2022) 13:912968. doi: 10.3389/fmicb.2022.912968
45. Martin R, Makino H, Cetinyurek YA, Ben-Amor K, Roelofs M, Ishikawa E, et al. Early-life events, including mode of delivery and type of feeding, siblings and gender, shape the developing gut microbiota. PLoS One. (2016) 11:e0158498. doi: 10.1371/journal.pone.0158498
46. Tadros JS, Llerena A, Sarkar A, Johnson R, Miller EM, Gray HL, et al. Postnatal growth and gut microbiota development influenced early childhood growth in preterm infants. Front Pediatr. (2022) 10:850629. doi: 10.3389/fped.2022.850629
47. Berger B, Porta N, Foata F, Grathwohl D, Delley M, Moine D, et al. Linking human milk oligosaccharides, infant fecal community types, and later risk to require antibiotics. mBio. (2020) 11(2):e03196–19.. doi: 10.1128/mBio.03196-19
48. Charbonneau MR, O'Donnell D, Blanton LV, Totten SM, Davis JC, Barratt MJ, et al. Sialylated milk oligosaccharides promote microbiota-dependent growth in models of infant undernutrition. Cell. (2016) 164:859–71. doi: 10.1016/j.cell.2016.01.024
49. Al NZ, Dulauroy S, Marques R, Cousu C, Al BS, Dejardin F, et al. A weaning reaction to microbiota is required for resistance to immunopathologies in the adult. Immunity. (2019) 50:1276–88.e5. doi: 10.1016/j.immuni.2019.02.014
50. Gura T. Nature’s first functional food. Science. (2014) 345:747–9. doi: 10.1126/science.345.6198.747
51. Donowitz JR, Haque R, Kirkpatrick BD, Alam M, Lu M, Kabir M, et al. Small intestine bacterial overgrowth and environmental enteropathy in Bangladeshi children. mBio. (2016) 7:e02102–15. doi: 10.1128/mBio.02102-15
52. McGrath CJ, Arndt MB, Walson JL. Biomarkers to stratify risk groups among children with malnutrition in resource-limited settings and to monitor response to intervention. Horm Res Paediatr. (2017) 88:111–7. doi: 10.1159/000471875
53. Zhou Y, Ma W, Zeng Y, Yan C, Zhao Y, Wang P, et al. Intrauterine antibiotic exposure affected neonatal gut bacteria and infant growth speed. Environ Pollut. (2021) 289:117901. doi: 10.1016/j.envpol.2021.117901
54. Uzan-Yulzari A, Turta O, Belogolovski A, Ziv O, Kunz C, Perschbacher S, et al. Neonatal antibiotic exposure impairs child growth during the first six years of life by perturbing intestinal microbial colonization. Nat Commun. (2021) 12:443. doi: 10.1038/s41467-020-20495-4
55. Reid BM, Eisenberg R, Forman K, LaTuga MS. Relationship between early antibiotic exposure and short-term growth velocity in premature neonates. Am J Perinatol. (2019) 36:1014–22. doi: 10.1055/s-0038-1675833
56. Zambruni M, Ochoa TJ, Somasunderam A, Cabada MM, Morales ML, Mitreva M, et al. Stunting is preceded by intestinal mucosal damage and microbiome changes and is associated with systemic inflammation in a cohort of Peruvian infants. Am J Trop Med Hyg. (2019) 101:1009–17. doi: 10.4269/ajtmh.18-0975
57. Vonaesch P, Morien E, Andrianonimiadana L, Sanke H, Mbecko JR, Huus KE, et al. Stunted childhood growth is associated with decompartmentalization of the gastrointestinal tract and overgrowth of oropharyngeal taxa. Proc Natl Acad Sci U S A. (2018) 115:E8489–98. doi: 10.1073/pnas.1806573115
58. Modan-Moses D, Yaroslavsky A, Pinhas-Hamiel O, Levy-Shraga Y, Kochavi B, Iron-Segev S, et al. Prospective longitudinal assessment of linear growth and adult height in female adolescents with anorexia Nervosa. J Clin Endocrinol Metab. (2021) 106:e1–10. doi: 10.1210/clinem/dgaa510
59. Prochazkova P, Roubalova R, Dvorak J, Kreisinger J, Hill M, Tlaskalova-Hogenova H, et al. The intestinal microbiota and metabolites in patients with anorexia nervosa. Gut Microbes. (2021) 13:1–25. doi: 10.1080/19490976.2021.1902771
60. Ptacek R, Kuzelova H, Stefano GB, Raboch J, Kream RM, Goetz M. ADHD and growth: questions still unanswered. Neuroendocrinol Lett. (2014) 35:1–6.24625909
61. Rojo-Marticella M, Arija V, Morales-Hidalgo P, Esteban-Figuerola P, Voltas-Moreso N, Canals-Sans J. Anthropometric status of preschoolers and elementary school children with ADHD: preliminary results from the EPINED study. Pediatr Res. (2023) 94(4):1570–8. doi: 10.1038/s41390-023-02671-6
62. Wan L, Ge WR, Zhang S, Sun YL, Wang B, Yang G. Case-control study of the effects of gut microbiota composition on neurotransmitter metabolic pathways in children with attention deficit hyperactivity disorder. Front Neurosci. (2020) 14:127. doi: 10.3389/fnins.2020.00127
63. Iglesias-Vazquez L, Van Ginkel RG, Arija V, Canals J. Composition of gut microbiota in children with autism spectrum disorder: a systematic review and meta-analysis. Nutrients. (2020) 12(3):792. doi: 10.3390/nu12030792
64. Green C, Dissanayake C, Loesch D. A review of physical growth in children and adolescents with autism pectrum disorder. Dev Rev. (2015) 36:156–78. doi: 10.1016/j.dr.2015.02.001
65. Samadi M, Moradi S, Azadbakht L, Rezaei M, Hojati N. Adherence to healthy diet is related to better linear growth with open growth plate in adolescent girls. Nutr Res. (2020) 76:29–36. doi: 10.1016/j.nutres.2020.02.002
66. Marcovecchio ML, Chiarelli F. Obesity and growth during childhood and puberty. World Rev Nutr Diet. (2013) 106:135–41. doi: 10.1159/000342545
67. Arnesen EK, Thorisdottir B, Lamberg-Allardt C, Barebring L, Nwaru B, Dierkes J, et al. Protein intake in children and growth and risk of overweight or obesity: a systematic review and meta-analysis. Food Nutr Res. (2022) 66:10.29219/fnr.v66.8242. doi: 10.29219/fnr.v66.8242
68. Beam A, Clinger E, Hao L. Effect of diet and dietary components on the composition of the gut microbiota. Nutrients. (2021) 13(8):2795. doi: 10.3390/nu13082795
69. Lobstein T, Jackson-Leach R, Moodie ML, Hall KD, Gortmaker SL, Swinburn BA, et al. Child and adolescent obesity: part of a bigger picture. Lancet. (2015) 385:2510–20. doi: 10.1016/S0140-6736(14)61746-3
70. Yu Z, Yu XF, Zhao X, Su Z, Ren PG. Greater alteration of gut microbiota occurs in childhood obesity than in adulthood obesity. Front Pediatr. (2023) 11:1087401. doi: 10.3389/fped.2023.1087401
71. Orbe-Orihuela YC, Godoy-Lozano EE, Lagunas-Martinez A, Castaneda-Marquez AC, Murga-Garrido S, Diaz-Benitez CE, et al. Association of gut microbiota with dietary-dependent childhood obesity. Arch Med Res. (2022) 53:407–15. doi: 10.1016/j.arcmed.2022.03.007
72. Edwards PT, Kashyap PC, Preidis GA. Microbiota on biotics: probiotics, prebiotics, and synbiotics to optimize growth and metabolism. Am J Physiol Gastrointest Liver Physiol. (2020) 319:G382–90. doi: 10.1152/ajpgi.00028.2020
73. Suzumura EA, Bersch-Ferreira AC, Torreglosa CR, Da SJ, Coqueiro AY, Kuntz M, et al. Effects of oral supplementation with probiotics or synbiotics in overweight and obese adults: a systematic review and meta-analyses of randomized trials. Nutr Rev. (2019) 77:430–50. doi: 10.1093/nutrit/nuz001
74. Shalitin S, Gat-Yablonski G. Associations of obesity with linear growth and puberty. Horm Res Paediatr. (2022) 95:120–36. doi: 10.1159/000516171
75. Hu HJ, Park SG, Jang HB, Choi MK, Park KH, Kang JH, et al. Obesity alters the microbial community profile in Korean adolescents. PLoS One. (2015) 10:e0134333. doi: 10.1371/journal.pone.0134333
76. Dong G, Zhang J, Yang Z, Feng X, Li J, Li D, et al. The association of gut microbiota with idiopathic central precocious puberty in girls. Front Endocrinol. (2019) 10:941. doi: 10.3389/fendo.2019.00941
77. Calcaterra V, Rossi V, Massini G, Regalbuto C, Hruby C, Panelli S, et al. Precocious puberty and microbiota: the role of the sex hormone-gut microbiome axis. Front Endocrinol. (2022) 13:1000919. doi: 10.3389/fendo.2022.1000919
78. Li Y, Shen L, Huang C, Li X, Chen J, Li SC, et al. Altered nitric oxide induced by gut microbiota reveals the connection between central precocious puberty and obesity. Clin Transl Med. (2021) 11:e299. doi: 10.1002/ctm2.299
79. Carel JC, Lahlou N, Roger M, Chaussain JL. Precocious puberty and statural growth. Hum Reprod Update. (2004) 10:135–47. doi: 10.1093/humupd/dmh012
80. Cheuiche AV, Da SL, de Paula L, Lucena I, Silveiro SP. Diagnosis and management of precocious sexual maturation: an updated review. Eur J Pediatr. (2021) 180:3073–87. doi: 10.1007/s00431-021-04022-1
81. Sorensen K, Mouritsen A, Mogensen SS, Aksglaede L, Juul A. Insulin sensitivity and lipid profiles in girls with central precocious puberty before and during gonadal suppression. J Clin Endocrinol Metab. (2010) 95:3736–44. doi: 10.1210/jc.2010-0731
82. Yin C, Chen J, Wu X, Liu Y, He Q, Cao Y, et al. Preterm birth is correlated with increased oral originated microbiome in the gut. Front Cell Infect Microbiol. (2021) 11:579766. doi: 10.3389/fcimb.2021.579766
83. Fardini Y, Chung P, Dumm R, Joshi N, Han YW. Transmission of diverse oral bacteria to murine placenta: evidence for the oral microbiome as a potential source of intrauterine infection. Infect Immun. (2010) 78:1789–96. doi: 10.1128/IAI.01395-09
84. Chu DM, Ma J, Prince AL, Antony KM, Seferovic MD, Aagaard KM. Maturation of the infant microbiome community structure and function across multiple body sites and in relation to mode of delivery. Nat Med. (2017) 23:314–26. doi: 10.1038/nm.4272
85. Xiao L, Zhao F. Microbial transmission, colonisation and succession: from pregnancy to infancy. Gut. (2023) 72:772–86. doi: 10.1136/gutjnl-2022-328970
86. Yan J, Charles JF. Gut microbiota and IGF-1. Calcif Tissue Int. (2018) 102:406–14. doi: 10.1007/s00223-018-0395-3
87. Schwarzer M, Makki K, Storelli G, Machuca-Gayet I, Srutkova D, Hermanova P, et al. Lactobacillus plantarum strain maintains growth of infant mice during chronic undernutrition. Science. (2016) 351:854–7. doi: 10.1126/science.aad8588
88. Shin SC, Kim SH, You H, Kim B, Kim AC, Lee KA, et al. Drosophila microbiome modulates host developmental and metabolic homeostasis via insulin signaling. Science. (2011) 334:670–4. doi: 10.1126/science.1212782
89. Zhang S, Wu P, Tian Y, Liu B, Huang L, Liu Z, et al. Gut microbiota serves a predictable outcome of short-term low-carbohydrate diet (LCD) intervention for patients with obesity. Microbiol Spectr. (2021) 9:e0022321. doi: 10.1128/Spectrum.00223-21
90. Morais MB, Silva G. Environmental enteric dysfunction and growth. J Pediatr (Rio J). (2019) 95(Suppl 1):85–94. doi: 10.1016/j.jped.2018.11.004
91. Leong K, Jayasinghe TN, Wilson BC, Derraik J, Albert BB, Chiavaroli V, et al. Effects of fecal microbiome transfer in adolescents with obesity: the gut bugs randomized controlled trial. JAMA Netw Open. (2020) 3:e2030415. doi: 10.1001/jamanetworkopen.2020.30415
92. Yan J, Herzog JW, Tsang K, Brennan CA, Bower MA, Garrett WS, et al. Gut microbiota induce IGF-1 and promote bone formation and growth. Proc Natl Acad Sci U S A. (2016) 113:E7554–63. doi: 10.1073/pnas.1607235113
93. Le Floc'H N, Otten W, Merlot E. Tryptophan metabolism, from nutrition to potential therapeutic applications. Amino Acids. (2011) 41:1195–205. doi: 10.1007/s00726-010-0752-7
94. Kosek MN, Mduma E, Kosek PS, Lee GO, Svensen E, Pan W, et al. Plasma tryptophan and the kynurenine-tryptophan ratio are associated with the acquisition of statural growth deficits and oral vaccine underperformance in populations with environmental enteropathy. Am J Trop Med Hyg. (2016) 95:928–37. doi: 10.4269/ajtmh.16-0037
95. Mayneris-Perxachs J, Lima AA, Guerrant RL, Leite AM, Moura AF, Lima NL, et al. Urinary N-methylnicotinamide and beta-aminoisobutyric acid predict catch-up growth in undernourished Brazilian children. Sci Rep. (2016) 6:19780. doi: 10.1038/srep19780
96. Gazi MA, Das S, Siddique MA, Alam MA, Fahim SM, Hasan MM, et al. Plasma kynurenine to tryptophan ratio is negatively associated with linear growth of children living in a slum of Bangladesh: results from a community-based intervention study. Am J Trop Med Hyg. (2020) 104:766–73. doi: 10.4269/ajtmh.20-0049
97. Wiggins JB, Trotman R, Perks PH, Swanson JR. Enteral nutrition: the intricacies of human milk from the immune system to the microbiome. Clin Perinatol. (2022) 49:427–45. doi: 10.1016/j.clp.2022.02.009
98. De Benedetti F, Alonzi T, Moretta A, Lazzaro D, Costa P, Poli V, et al. Interleukin 6 causes growth impairment in transgenic mice through a decrease in insulin-like growth factor-I. A model for stunted growth in children with chronic inflammation. J Clin Invest. (1997) 99:643–50. doi: 10.1172/JCI119207
99. Tigas S, Tsatsoulis A. Endocrine and metabolic manifestations in inflammatory bowel disease. Ann Gastroenterol. (2012) 25:37–44.24714153
100. D'Angelo DM, Di Donato G, Breda L, Chiarelli F. Growth and puberty in children with juvenile idiopathic arthritis. Pediatr Rheumatol Online J. (2021) 19:28. doi: 10.1186/s12969-021-00521-5
101. Ferreira P, Cavalcanti AS, Silva G. Linear growth and bone metabolism in pediatric patients with inflammatory bowel disease. J Pediatr (Rio J). (2019) 95(Suppl 1):59–65. doi: 10.1016/j.jped.2018.11.002
102. Prendergast AJ, Rukobo S, Chasekwa B, Mutasa K, Ntozini R, Mbuya MN, et al. Stunting is characterized by chronic inflammation in Zimbabwean infants. PLoS One. (2014) 9:e86928. doi: 10.1371/journal.pone.0086928
103. Yang YJ, Sheu BS, Yang HB, Lu CC, Chuang CC. Eradication of Helicobacter pylori increases childhood growth and serum acylated ghrelin levels. World J Gastroenterol. (2012) 18:2674–81. doi: 10.3748/wjg.v18.i21.2674
104. Saari A, Virta LJ, Sankilampi U, Dunkel L, Saxen H. Antibiotic exposure in infancy and risk of being overweight in the first 24 months of life. Pediatrics. (2015) 135:617–26. doi: 10.1542/peds.2014-3407
105. Gough EK, Moodie EE, Prendergast AJ, Johnson SM, Humphrey JH, Stoltzfus RJ, et al. The impact of antibiotics on growth in children in low and middle income countries: systematic review and meta-analysis of randomised controlled trials. BMJ. (2014) 348:g2267. doi: 10.1136/bmj.g2267
106. Amza A, Kadri B, Nassirou B, Stoller NE, Yu SN, Zhou Z, et al. A cluster-randomized controlled trial evaluating the effects of mass azithromycin treatment on growth and nutrition in Niger. Am J Trop Med Hyg. (2013) 88:138–43. doi: 10.4269/ajtmh.2012.12-0284
107. Kosek M, Haque R, Lima A, Babji S, Shrestha S, Qureshi S, et al. Fecal markers of intestinal inflammation and permeability associated with the subsequent acquisition of linear growth deficits in infants. Am J Trop Med Hyg. (2013) 88:390–6. doi: 10.4269/ajtmh.2012.12-0549
108. Ahmed T, Auble D, Berkley JA, Black R, Ahern PP, Hossain M, et al. An evolving perspective about the origins of childhood undernutrition and nutritional interventions that includes the gut microbiome. Ann N Y Acad Sci. (2014) 1332:22–38. doi: 10.1111/nyas.12487
Keywords: intestinal flora, linear growth, IGF-1 (insulin-like growth factor-1), microbiota, SCFAs (short-chain fatty acids)
Citation: He P, Shen X and Guo S (2023) Intestinal flora and linear growth in children. Front. Pediatr. 11:1252035. doi: 10.3389/fped.2023.1252035
Received: 3 July 2023; Accepted: 2 November 2023;
Published: 16 November 2023.
Edited by:
Sijung Yun, Predictiv Care, Inc., United StatesReviewed by:
Luis Rodrigo Macias Kauffer, Universität zu Lübeck, GermanyModupe Coker, The State University of New Jersey, United States
© 2023 He, Shen and Guo. This is an open-access article distributed under the terms of the Creative Commons Attribution License (CC BY). The use, distribution or reproduction in other forums is permitted, provided the original author(s) and the copyright owner(s) are credited and that the original publication in this journal is cited, in accordance with accepted academic practice. No use, distribution or reproduction is permitted which does not comply with these terms.
*Correspondence: Sheng Guo Z3Vvc2hlbmdAc2hjaGlsZHJlbi5jb20uY24=