- 1Pediatric Unit, Department of Precision and Regenerative Medicine and Ionian Area, University of Bari “Aldo Moro”, Bari, Italy
- 2Giovanni XXIII Pediatric Hospital, Bari, Italy
- 3Department of Interdisciplinary Medicine, University of Bari “Aldo Moro”, Bari, Italy
The purpose of this narrative review was to investigate the key determinants of musculoskeletal health in childhood and adolescence, with particular attention to the role of physical activity. First, we examined the importance of bone modeling and remodeling in maintaining the bone health and the integrity and mechanical characteristic of the skeleton. In addition, we reported the evidence on an appropriate calcium and vitamin D intake, as well as local load variation in achieving proper peak bone mass. Proteomic and transcriptomic studies identified the skeletal muscle “secretoma”, consisting of several myokines involved in endocrine and paracrine functions. Among these, we explored the role of irisin, a myokine involved in the muscle-bone crosstalk, and in the regulation of metabolic pathways. It is known that physical activity during growing positively impacts on skeleton and can protect by bone loss in adulthood. However, there are still concerns about the optimal interval duration and exercise intensity, particularly at the pubertal growth spurt which represents a window of opportunity to increase skeletal strength. We reported data from clinical trials performed in the last 5 years analyzing the impact of the type and timing of physical activity during childhood on skeletal development. Finally, we reported recent data on the significance of physical activity in some rare diseases.
Introduction
The coordinated action of osteoblasts, osteoclasts and osteocytes directs bone modelling and remodeling (1). The former process promotes longitudinal growth of the bones and adaption of the skeleton to mechanical stress, while the latter removes old or damaged bone. Thus, bone remodeling maintains the strength of the skeleton over the course of life. During bone modeling, osteoclasts and osteoblasts work individually, while when bone remodeling occurs, bone resorption and bone formation are coupled into bone remodeling units (1). With ageing, the skeleton undergoes substantial architectural and metabolic modifications, possibly predisposing to osteoporosis and increased risk of fractures. Although osteoporosis is typically associated with ageing, the contributing factors can act already during growth. Hence, the impairment of bone health occurs during the developmental age, when over a third of bone mass is accumulated, reaching a peak around the second decade of life (2). The conjunction of this critical period of bone growing with bone loading and physical activity represents a “window of opportunity” to develop a healthy skeleton (3). Environmental factors, such as diet and exercise, impact 20%–40% of peak bone mass in adulthood. Physical activity is recognized as a means of health promotion and disease prevention throughout the life (4), although there are few specific recommendations in infancy and childhood, which represent periods of life wherein healthy behaviors can have lasting metabolic and behavioral consequences (5, 6). An adequate development of skeletal muscles during these two periods may have long-term consequences on body composition and inclination to engage in physical activity throughout life (7). Moreover, although muscle fiber composition is genetically determined (8), early physical training can play an important role in “fiber reprogramming” (9). Bone mineral content (BMC) and bone mineral density (BMD) increase in response to repetitive and variable loading activities through increased force and strain. Moderate or intense physical activity and several sports inducing greater than normal bone load are critical for the achievement of bone strength (10). Considering this, the implementation of exercise interventions during childhood and adolescence could maximize peak bone mass and consequently slow down the onset of osteoporosis.
In this narrative review, we focused on the key determinants of musculoskeletal health in pediatric age, and we reported the most recent studies on the impact of physical activity on bone strength during childhood and adolescence. Furthermore, we reported the effects of physical activity in some rare diseases in which has been demonstrated an improving of bone health.
The concept of bone health: bone modeling, bone remodeling and peak bone mass
Bone contains an organic component represented by collagenous and non-collagenous proteins and cells, and a mineral part of hydroxyapatite (11).
Bone cells include osteoblasts, the bone forming cells, which originate from mesenchymal stem cells; osteocytes, differentiated from osteoblasts and inserted in the bone matrix; and osteoclasts, the bone reabsorbing cells, differentiated from hematopoietic progenitors. Skeletal growth is the result of the bone expansion of cortical bone, and bone growing through endochondral ossification (12). This process called “bone modeling”, which starts during fetal life and proceeds until epiphyseal fusion, is particularly sensitive to mechanical load, supporting the significance of physical activity during growth (13). The acquisition of bone mass occurs slowly throughout childhood, while it proceeds quickly with the onset of puberty and at the time of growth spurt. Peak bone mass occurs at 12.5 ± 0.90 years in girls and 14.1 ± 0.95 years in boys (13).
The changes of bone sizes and thickness occur more rapidly after epiphyseal fusion and continue until the attainment of peak bone mass during the second decade of life (13). However, the changes of bone shape and bone composition occurring during pubertal development, influence the bone strength (14). Thus, the “bone bank” is built in the first two decades of life, and most of the risk of osteoporosis depends on what occurs in this period. Peak bone mass represents a key determinant of bone health and risk of osteoporosis in adulthood (15). Indeed, it is strictly related to bone strength that in turn is determined by bone mass, bone density, microarchitecture, micro repairs and bone geometry. The attainment of a suitable peak bone mass can prevent fractures both in childhood and adulthood (16–18).
Bone is also a living tissue as old and damaged bone is removed and replaced with newly formed bone. This process of bone renewal and repair is called “bone remodeling”, and it is due to the action of osteoclasts and the osteoblasts (19). In healthy bones, the osteoclast and osteoblast activity are balanced. When this process becomes unbalanced, so that the bone reabsorbing happens faster than bone replacing, bones can become thin and fragile. Bone remodeling is determinant to maintaining the integrity and the mechanical properties of the skeleton (20). This balance is controlled by the RANK/RANKL/osteoprotegerin (OPG) and Wnt/β-catenin pathways which regulate osteoclastogenesis and osteoblastogenesis, respectively (21). Bone remodeling's alterations have been observed in several congenital and acquired pediatric disorders (22). Particularly, in subjects with obesity, the condition of low-grade inflammation activates osteoclasts by up regulating the production of RANKL and other inflammatory cytokines, and inhibiting osteoblastogenesis, thus accelerating bone resorption (23).
Key determinants of bone health in pediatric age
According to the National Osteoporosis Foundation, bone health is the consequence of both genetic and environmental factors (16). Genetic factors impact skeletal development for approximately 60%–80% (16, 24). Numerous loci linked with low bone mass and osteoporosis have been recognized by Genome Wide Association (GWA) studies (25), although few studies have been conducted in children. Environmental factors, such as diet and physical activity are responsible for 20%–40% of the peak bone mass (16).
Calcium and vitamin D intake
Adequate calcium and vitamin D intake, in association with physical activity, maximizes peak bone mass and reduces the risk of osteoporosis and fractures in childhood and adulthood (26). The National Institutes of Health provided the recommendations for calcium intake and calcium content of foods (27). The recommended dietary allowance (RDA) for calcium is 1,300 mg for subjects aged 9–18 years (24). Calcium intakes <600 mg/day, may expose to substantial risks of inadequate mineralization (28). Intakes <400 mg/day, especially when combined with low vitamin D levels, represent a risk of rickets and fractures.
A systematic review evaluated the methods and quality of guidelines on calcium and vitamin D supplementation in healthy children (29). The authors observed significant variations on calcium and vitamin D recommendations across 24 guidelines and consensus among countries around the world. The recommended calcium intake for children ranged from 400 to 1,150 mg/day. Additionally, data on vitamin D supplementation at different ages, vitamin D type, and sunlight exposure were conflicting across studies (29).
In a previous study, the supplementation of 800 mg of calcium and 400 IU of vitamin D daily for 6 months resulted in increases in cortical and trabecular bone (30). In contrast, a meta-analysis demonstrated that rising dairy products in the diet significantly improved bone mineral content only in children with low baseline calcium intake (31). Thus, calcium and vitamin D supplementation would appear to have little influence on bone health.
Physical activity and musculoskeletal health
Muscle and bone interact through both anatomically and mechanically, as well as through paracrine and endocrine signals (32). As regards the mechanical interaction, bones represent a site of attachment for muscles and, in turn, skeletal muscles facilitate locomotion by giving strength to the bone; hence, muscles are the primary source of tension for bones. This is testified by the fact that astronauts experience bone loss and muscle atrophy as they are exposed to an environment that lacks gravity, such as space; as they return to the ground, the speed of recovery that they experience in their muscle mass exceeds that of bone, suggesting muscle contraction is essential for bone recovery. Thus, immobility, aging and other diseases can cause changes in both bone and muscle mass. In addition, skeletal muscles produce factors which regulate bone metabolism. These muscle-secreted factors named “myokines”, include myostatin, interleukin (IL)-6, IL-7, IL-15, IGF-1, FGF-2, irisin, and BAIBA (33).
Physical activity has a positive impact on musculoskeletal health. The skeleton responds to physical stress quickly and bone remodeling starts. Exercise leads to bone adaptation by cellular mechano transduction (34). Quickly, upon exercise, the mechanosensors located through the cells, such as stretch-activated ion channels and integrins, modify their conformation (Figure 1). These conformational changes activate a signaling cascade which determines bone accretion at the site of deformation (34). To obtain an osteogenic response, the bones must undergo such a deformation that exceeds the usual deformation threshold. The threshold varies between individuals and bone sites according to physical activity habits, age, hormone levels, and other metabolic factors (35). Thus, children and adolescents may have a different response to comparable mechanical loads: inactive children may improve bone mass in response to low-impact loads, while active children will need a greater mechanical load (36). The response is regional, allowing specific bone to meet increasing loading requirements. Indeed, a greater bone density has been observed in the dominant arms of baseball, tennis, and squash players with side-to-side variations ranging from 8% to 22% (37). It has also been demonstrated that the magnitude of loading and deviations from normal loading patterns are more important for bone modeling than stimulus duration. Mechanical loading induced by physical activity is necessary to stimulate bone modeling as it provides the stimulus required to develop a robust skeleton (38). Mechanical loads of 3.5 g-force for 3 days/week with 100 loads per session and 7 months of intervention determine an osteogenic effect. Furthermore, the mechanical loading stimulates the Wnt/-catenin signaling pathway, which in turn downregulates osteoclastogenesis and osteoclast activity, thus influencing bone remodeling (39).
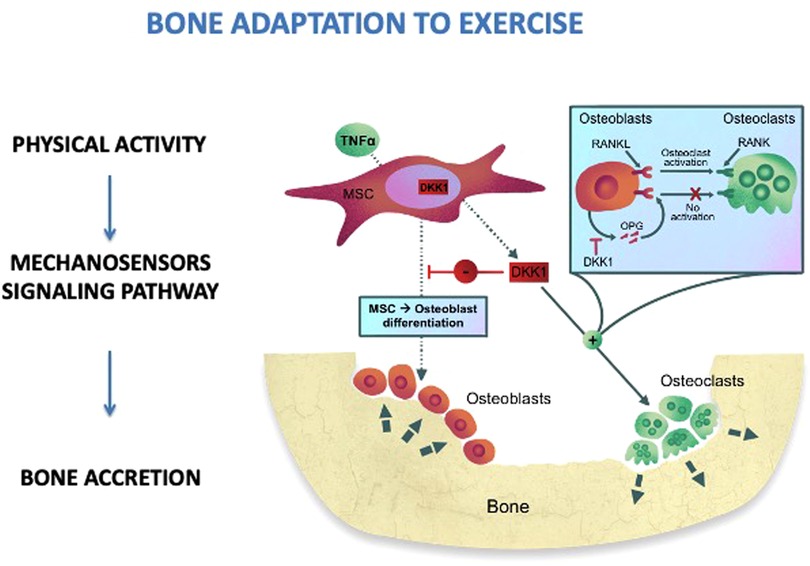
Figure 1. Bone adaptation to exercise. MSC, mesenchimal stem cells; RANKL, receptor activator of nuclear factor kappa-Β ligand; RANK, RANKL/OPG receptor; DKK1, dickkopf-1; OPG, osteoprotegerin; TNFα, tumor necrosis factor alpha.
Regarding the muscle response to exercise, the increased load contributes to muscle size and strength, primarily as a result of muscle cell hypertrophy rather than hyperplasia (40). The increase in muscle mass results in an inhibition of myostatin which hence regulates muscle mass. Nonetheless, it is not yet clear why only the muscles under load increases muscle mass, while the decrease in circulating levels of myostatin resulting from exercise should affect the whole body (41). This suggests that other unknown soluble factors produced by skeletal muscles after exercise may contribute to increases in muscle size and strength. On the other hand, during aging and immobility, the physiological changes caused by skeletal unloading determine the onset of sarcopenia, characterized by a decrease in the size (atrophy) and number (hypoplasia) of muscle fibers. Therapeutic strategies to treat sarcopenia are currently aimed at targeting the myostatin/activin signaling mechanism, on the basis of the principle of “muscle-bone crosstalk”.
Irisin: a myokine involved in the crosstalk between muscle and bone
Irisin is a myokine firstly identified for its role in inducing browning of white adipose tissue and increasing energy expenditure (42). Then, both in human and mouse it has been demonstrated that irisin is also involved in glucose homeostasis by promoting liver glycogen synthesis and inhibiting gluconeogenesis (43, 44). Further role of this myokine in cognitive functions, learning, and memory has been recognized (45). In humans irisin represents a link between physical activity and metabolic homeostasis, as it mediates the beneficial effects of exercise on glucose and lipid metabolism, and it provides to maintain musculoskeletal homeostasis (46, 47). This evidence strengthens the hypothesis that muscle can be considered an endocrine organ. Studies of proteomics and transcriptomics identified the composition of the skeletal muscle “secretome” leading to identification of several myokines (48). Most of the proteins identified in cultures of myotube were predicted as putative secreted proteins, pointing out that skeletal muscle acts as an endocrine organ. Functional analysis suggests their role in skeletal muscle as paracrine regulators of oxidation, hypertrophy, angiogenesis, and extracellular matrix (49). These myokines are also involved in the regulation of body weight, inflammation, insulin sensitivity, and cognitive function. Thus, muscle derived regulatory RNAs could represent a novel frontier for the treatment of chronic diseases. Exercise as downhill running, eccentric exercise, and resistance training determine a systemic marked cytokine response, with a higher degree of muscle involvement (50). However, a strong elevation of several cytokines and chemokines in skeletal muscle has been described after exercise with long duration or high intensity (51), while a less evident or absent response has been observed after moderate intense physical activity (52). The direct involvement of irisin in bone metabolism, by inducing the differentiation of bone marrow stromal cells into mature osteoblasts, has been demonstrated (53). In healthy children, irisin serum levels positively correlate with bone mineral status and negatively with inhibitors of Wnt signaling pathway (54). Furthermore, high irisin levels correlate with a better glycemic control and bone health in children affected with type 1 diabetes (55). Zhang et al., confirmed the positive regulatory effect of irisin on bone during physical exercise, as they found an increased expression of biochemical markers of bone formation and irisin increase in bone tissue after 2 weeks of free wheel-running exercise (56). Exercise-induced irisin, or exogenous administration of irisin, can prevent bone loss, as demonstrated in hind-limb suspended mice (47). However, the true effect of physical activity in promoting secretion of irisin is still debated. Some studies reported a huge effect of exercise in promoting irisin release (45–47), while others did not document any changes in irisin levels after both acute and chronic exercise (57, 58). Probably, this disagreement depends on the type and duration of exercise, and the type of assay for irisin assessment. Conversely, serum irisin levels has been identified as a predictive biomarker for sarcopenia (59, 60). Moreover, irisin can activate the differentiation of osteoblasts under microgravity conditions by promoting the secretion of β-catenin protein and increase OPG levels (61).
Furthermore, body composition is also a relevant factor for musculoskeletal health, in fact age-related loss of skeletal muscle (sarcopenia) is often associated with obesity (“sarcopenic obesity”). Increased myostatin levels have been shown to result in low levels of muscle tissue mass and at the same time inhibit insulin signaling, muscle mitochondrial biogenesis, lipid oxidation and energy expenditure, therefore this myokine could play a key role in sarcopenic obesity (62).
The impact of exercise interventions on bone strength
Physical exercise and healthy eating habits during growing increases the probabilities of accruing bone, and potentially delays osteoporosis in adulthood. Literature data on the effectiveness of physical activity interventions in childhood and adolescence are heterogeneous.
A meta-analysis of 27 studies found a significant effect of weight bearing activity on BMC and BMD (63). Children involved in school-based exercise programs for 9 months showed higher whole-body, femoral neck and total hip BMC compared with their counterparts not involved in exercising (64). Furthermore, a persistence of the benefits 3 years after ceasing the intervention has been observed. Longitudinal studies reached different conclusions regarding whether the osteogenic effect depends on continuing physical activity into adulthood (65–67). Subject who practiced sports during childhood showed a greater hip BMC in adulthood than their sedentary counterparts (68). A cross-sectional cohort study investigated the longstanding effects of soccer on BMD and fracture risk, demonstrating a higher BMD, and lower risk of fractures in the athletes 30 years after retirement (69).
A recent study demonstrated that the age at which children first start walking might affect their bone strength in later life (70). Ireland et al., examined the association between walking age and bone mineralization by Dual-energy x-ray absorptiometry (DXA) and Quantitative Computed Tomography (pQCT) in subjects aged between the ages of 60 and 64 years (70). Later independent walking age has been associated with lower BMC, suggesting that early mechanical loading on the skeleton might influence bone strength development. A systematic review of the literature evaluated the changes induced on bone mineralization by ball games, dancing, jumping and other physical activities. The results of this study showed that weight-bearing exercise in childhood had a positive effect on bone strength, while exercise performed during prepubertal and peripubertal age caused an increase in bone mineral accrual (71). These results have been confirmed by other studies which observed that, although osteogenesis and bone anabolism are more pronounced during the peripubertal phase, the period immediately preceding puberty represents a “window of opportunity” in which the skeleton is more sensitive to mechanical stress (35).
The impact of physical activity on musculoskeletal health
In this section we reported the most important clinical trial conducted in the last 5 years that investigated weight-bearing physical activities improving musculoskeletal health. Table 1 shows the recent data on the effects of sport interventions on bone mineralization.
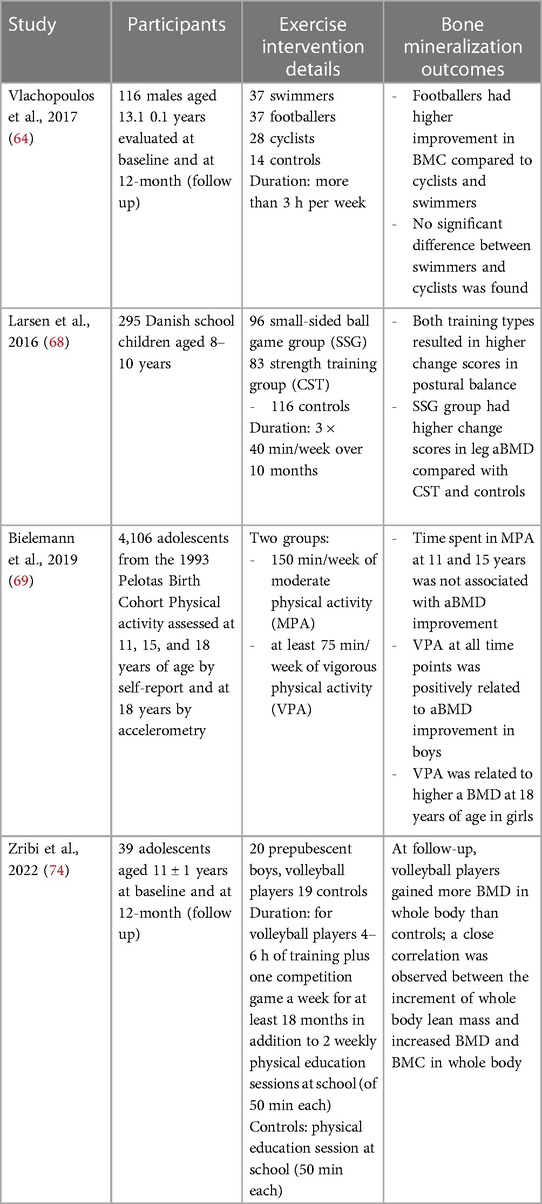
Table 1. Clinical trial investigated weight-bearing physical activities improving musculoskeletal health.
The PRO-BONE study explored the effect of 12-months involvement in osteogenic (football) and non-osteogenic (swimming and cycling) sports in 116 adolescent male athletes aged 13.1 years ±1.0 (72). The authors observed that after 12-months participation, footballers showed significantly higher BMC at most skeletal sites than swimmers and cyclists. A previous study demonstrated that 8 months of sport-specific training improved total body BMD by 2.9% in footballers, but not in swimmers (73). These results confirm previous studies that reported that swimming and cycling have no effect on bone strength, possibly due to the low ground reaction forces produced during exercise (74, 75). Therefore, football improves bone strength compared to non-osteogenic sports which should be combined with weight-bearing activities.
The randomized controlled trial FIT FIRST involving 295 Danish children aged between 8 and 10 years evaluated the effects on bone mineralization and muscular structure after 10 months of high-intensity school training (3 × 40 min/week) consisting of team soccer and other ball games or circuit training with weight-bearing exercises (76). The authors observed that both high-impact school interventions improved musculoskeletal health in children. The observations confirmed that in childhood the skeleton adapts to the physiological changes induced by physical training and suggested that both high-intensity interval program and odd-impact training may improve musculoskeletal health.
Bieleman et al., examined the relations between physical activity and areal BMD (aBMD) according to intensity of exercise in several sports: outdoor soccer, indoor football, athletics, basketball, volleyball, tennis, handball, dance, gymnastics, martial arts, swimming, trapper, and playing bat (77). Vigorous physical activity improved aBMD more than moderate physical activity, especially in boys. In particular, the involvement in vigorous activities from the middle to the end of adolescence seems to be related to higher aBMD. Other studies described differences induced by physical activities on aBMD between boys and girls (78–80). The better impact of vigorous-intensity physical activity on bone density in boys than in girls may be explained by the increased sensitivity to mechanical loading in boys during adolescence and exposure to testosterone which increases bone and muscle mass (81).
Zribi et al. evaluated longitudinally the consequences of 1-year of volleyball on BMD and BMC, assessed by DXA (82). Volleyball is a team sport which includes different movements such as accelerations and decelerations, rapid changes of direction and repetitive jumps. All actions generate high stresses on the upper and lower limbs from the reaction forces produced by the jumps, which measure three to six times the body weight.
This study demonstrated that playing volleyball 4–6 h per week for approximately 1 year resulted in higher BMD and BMC at all skeletal sites analyzed in prepubertal boys compared with non-physically active controls. In volleyball the mechanical forces acting on the bones derive both by the high reaction forces produced by the impact with the ground in the jump, and by the muscular contractions which pull their bone attachment.
Physical activity in rare diseases
Physical activity has a key role in the care of patients affected with rare diseases, as it exerts both physic and psychological effects, pointing to increase quality of life. In addition, there is evidence that physical activity can improve bone health in some skeletal and extra-skeletal rare diseases.
Rheumatic and musculoskeletal diseases include a group of systemic diseases such as osteoarthritis, rheumatoid arthritis, systemic lupus erythematosus, axial spondyloarthritis, psoriatic arthritis, systemic sclerosis, characterized by pain, disability, and low quality of life. Patients with rheumatic and musculoskeletal disorders experience loss of mobility, loss of autonomy, and higher mortality rates. Consequently, these disorders have a high impact on the social and health system.
The recent recommendations of the European League Against Rheumatism (EULAR) taskforce suggested that exercise interventions improve pain and functions, although the size of the effect varied by type of diseases and type of intervention (83). As weight gain has been associated with worse outcomes for most of these conditions, maintaining an adequate body weight is also recommended.
Physical activity is essential for children with hemophilia, a rare X-linked bleeding disorder caused by a missing or defective clotting factor, to maintain joint movement, reduce joint bleeding, develop muscle mass and strength, and prevent secondary chronic disease and osteopenia/osteoporosis (84–86). In the past, since there was no treatment for hemophilia, the affected children were forbidden to exercise because the risk of bleeding. Current therapies not only treat acute bleeding, but also prevent it. It is sufficient to pre-administer the deficient clotting factor and the risk of bleeding is temporarily reduced. The availability of effective and safe drugs drives patients and caregivers to insistently ask doctors to start physical activity as for their peers (87).
Although for a hemophilic child under pharmacological treatment there are no contraindications for practicing sports, the sport promotion for hemophilic children is yet an obstacle course (88).
Different guidelines indicated hemophilic subjects' sports participation according with type and severity (89). In addition, the selection of activities should consider individual preferences, abilities, and physical conditions. However, high-impact sports such as rugby, boxing, soccer and basketball, or sports with a higher risk of injury are often discouraged despite good prophylaxis. The National Hemophilia Foundation (NHF) has suggested guidelines for athletic participation of patients with bleeding disorders (90). For hemophilic patients, a minimum of 60 min of exercise per day, with adequate supervision, is recommended after receiving prophylaxis.
To overcome the problem and allow physical activity for every child with hemophilia, the Italian study group has drawn up some recommendations which invite gradual training before accessing intense physical activity. Gradual training allows the individual subject to understand which and what physical activity practice and favors the possibility of daily moderate physical activity to limit the chronic evolution of arthropathy (91).
Prader-Willi syndrome (PWS) is the most frequent form of genetic obesity. It is characterized by severe hypotonia and feeding problems in early infancy, followed by excessive eating and gradual development of severe obesity (92). In adulthood, PWS patients develop cardio-respiratory diseases, psychiatric disorders as well as various comorbidities such as muscle weakness and scoliosis. Encouraging physical activity is an essential objective of the management of PWS both in children and adults. In the last years, several studies have considered physical activity in PWS patients (93–95). Although most studies have described a low exercise in patients with PWS, it is not clear whether the decreased physical activity in these patients is related to obesity per se, or to the physical and intellectual disabilities related to this syndrome. A recent systematic review which considered controlled trials, single-group interventions, observational, and qualitative studies reported that only 5%–8% of PWS children (93, 96, 97) and 15%–25% of adults (94, 98) met the WHO physical activity and sedentary behavior guidelines (99). According with WHO guidelines, 60 min/day of moderate-to-vigorous physical activity is suggested for children, and 150–300 min/week (i.e., at least 30 min/day) for adults (98). Replacing sedentary lifestyle with physical activity has beneficial effects for lifelong health preservation, particularly for individuals with low physical activity levels such as PWS patients (100).
Recently, a protocol for a randomized trial on increasing resistance training in young people with PWS has been started (101). The purpose of this study is to establish whether progressive resistance training is effective in improving muscle strength in PWS subjects, understand participants' experiences and identify factors influencing implementation, and determine long-term efficacy in terms of healthcare expenditure.
Conclusion and future directions
The skeleton is a structure made up of living tissue that grows, repairs, and renews itself. It plays a supportive role for muscles and a protecting role for internal organs. Furthermore, the skeleton influences energy metabolism through a continuous interaction with cytokines derived from both adipose and muscle tissue, and with insulin. There is substantial evidence that skeletal muscle secretes factors which act as mediators of endocrine signaling and are also implicated in the favorable effects of exercise. Skeletal muscle “secretoma” during exercise has not yet been described, but research has developing modified myokines with the aim of supporting the treatment of chronic diseases.
Adequate nutrition and physical activity can influence musculoskeletal health; however how exercise during pediatric age affects bone health remains to be investigated. Previous studies have demonstrated a cumulative effect of vigorous physical activity during adolescence on BMD, suggesting that any time in adolescence is a window of opportunity to increase bone mass. The mechanical forces acting on the loaded bones are generated both by the high reaction forces produced by the impact with the ground in sports such as high jump, basketball, and volleyball, and by the muscle contractions that pull their attachment on the skeleton. This agrees with previous studies that have shown that lean mass development is the best predictor of bone mass accumulation. Osteogenic sports, such as football, result in a higher BMC than non-osteogenic sports, such as swimming and cycling, in which physical activities should be combined with weight-bearing movements to optimize bone growth.
Studies of larger cohorts of children and adolescents addressing issues such as the complex interaction between bone, gut, white and brown adipose tissue, nutrition, and physical activity will be needed in the future to provide new insights into this fascinating field of metabolic endocrinology.
Author contributions
MF wrote the manuscript. FU, GL and MC performed medline research and selected the literature. PG critically revised the manuscript. All authors contributed to the article and approved the submitted version.
Conflict of interest
The authors declare that the research was conducted in the absence of any commercial or financial relationships that could be construed as a potential conflict of interest.
Publisher's note
All claims expressed in this article are solely those of the authors and do not necessarily represent those of their affiliated organizations, or those of the publisher, the editors and the reviewers. Any product that may be evaluated in this article, or claim that may be made by its manufacturer, is not guaranteed or endorsed by the publisher.
References
1. Sims NA, Martin TJ. Coupling the activities of bone formation and resorption: a multitude of signals within the basic multicellular unit. Bonekey Rep. (2014) 3:481. doi: 10.1038/bonekey.2013.215
2. Baxter-Jones AD, Faulkner RA, Forwood MR, Mirwald RL, Bailey DA. Bone mineral accrual from 8 to 30 years of age: an estimation of peak bone mass. J Bone Miner Res. (2011) 26(8):1729–39. doi: 10.1002/jbmr.412
3. Forwood MR. Growing a healthy skeleton: the importance of mechanical loading. In: Rosen CJ, Bouillon R, Compston JE, Rosen V, editors. Primer on the metabolic diseases and disorders of mineral metabolism. Hoboken, NJ: John Wiley & Sons, Inc. (2013). p. 149–55.
4. Haskell WL, Blair SN, Hill JO. Physical activity: health outcomes and importance for public health policy. Prev Med. (2009) 49(4):280–2. doi: 10.1016/j.ypmed.2009.05.002
5. World Health Organization. Guidelines on physical activity, sedentary behaviour and sleep for children under 5 years of age. World Health Organization (2019). ISBN: 9789241550536.
6. Centers for Disease Control and Prevention. Physical activity guidelines for school-aged children and adolescents. Washington, DC, United States: US Department of Health and Human Services (2019).
7. van Deutekom AW, Chinapaw MJ, Vrijkotte TG, Gemke RJ. Study protocol: the relation of birth weight and infant growth trajectories with physical fitness, physical activity and sedentary behavior at 8-9 years of age - the ABCD study. BMC Pediatr. (2013) 13(1):102. doi: 10.1186/1471-2431-13-102
8. Simoneau J, Lortie G, Boulay M, Marcotte M, Thibault M, Bouchard C. Inheritance of human skeletal muscle and anaerobic capacity adaptation to high-intensity intermittent training*. Int J Sports Med. (1986) 07(03):167–71. doi: 10.1055/s-2008-1025756
9. Esbjörnsson M, Hellsten-Westing Y, Balsom PD, Sjödin B, Jansson E. Muscle fibre type changes with sprint training: effect of training pattern. Acta Physiol Scand. (1993) 149(2):245–6. doi: 10.1111/j.1748-1716.1993.tb09618.x
10. Sardinha LB, Baptista F, Ekelund U. Objectively measured physical activity and bone strength in 9-year-old boys and girls. Pediatrics. (2008) 122(3):e728–36. doi: 10.1542/peds.2007-2573
11. Boskey AL. Bone composition: relationship to bone fragility and antiosteoporotic drug effects. Bonekey Rep. (2013) 2:447. doi: 10.1038/bonekey.2013.181
12. Faienza MF, Chiarito M, Brunetti G, D'Amato G. Growth plate gene involment and isolated short stature. Endocrine. (2021) 71(1):28–34. doi: 10.1007/s12020-020-02362-w
13. Heaney RP, Abrams S, Dawson-Hughes B, Looker A, Marcus R, Matkovic V, et al. Peak bone mass. Osteoporos Int. (2000) 11(12):985–1009. doi: 10.1007/s001980070020
14. Kirmani S, Christen D, van Lenthe GH, Fischer PR, Bouxsein ML, McCready LK, et al. Bone structure at the distal radius during adolescent growth. J Bone Miner Res. (2009) 24(6):1033–42. doi: 10.1359/jbmr.081255
15. Xue S, Kemal O, Lu M, Lix LM, Leslie WD, Yang S. Age at attainment of peak bone mineral density and its associated factors: the national health and nutrition examination survey 2005-2014. Bone. (2020) 131:115163. doi: 10.1016/j.bone.2019.115163
16. Weaver CM, Gordon CM, Janz KF, Kalkwarf HJ, Lappe JM, Lewis R, et al. The national osteoporosis foundation’s position statement on peak bone mass development and lifestyle factors: a systematic review and implementation recommendations. Osteoporos Int. (2016) 27(4):1281–386. doi: 10.1007/s00198-015-3440-3
17. Kalkwarf HJ, Laor T, Bean JA. Fracture risk in children with a forearm injury is associated with volumetric bone density and cortical area (by peripheral QCT) and areal bone density (by DXA). Osteoporos Int. (2011) 22(2):607–16. doi: 10.1007/s00198-010-1333-z
18. Holloway KL, Brennan SL, Kotowicz MA, Bucki-Smith G, Timney EN, Dobbins AG, et al. Prior fracture as a risk factor for future fracture in an Australian cohort. Osteoporos Int. (2015) 26(2):629–35. doi: 10.1007/s00198-014-2897-9
19. Boyle WJ, Simonet WS, Lacey DL. Osteoclast differentiation and activation. Nature. (2003) 423(6937):337–42. doi: 10.1038/nature01658
20. Maggioli C, Stagi S. Bone modeling, remodeling, and skeletal health in children and adolescents: mineral accrual, assessment and treatment. Ann Pediatr Endocrinol Metab. (2017) 22(1):1–5. doi: 10.6065/apem.2017.22.1.1
21. Baron R, Kneissel M. WNT signaling in bone homeostasis and disease: from human mutations to treatments. Nat Med. (2013) 19(2):179–92. doi: 10.1038/nm.3074
22. Brunetti G, D'Amato G, Chiarito M, Tullo A, Colaianni G, Colucci S, et al. An update on the role of RANKL-RANK/osteoprotegerin and WNT-ß-catenin signaling pathways in pediatric diseases. World J Pediatr. (2019) 15(1):4–11. doi: 10.1007/s12519-018-0198-7
23. Faienza MF, D'Amato G, Chiarito M, Colaianni G, Colucci S, Grano M, et al. Mechanisms involved in childhood obesity-related bone fragility. Front Endocrinol. (2019) 10:269. doi: 10.3389/fendo.2019.00269
24. Golden NH, Abrams SA, Committee on Nutrition. Optimizing bone health in children and adolescents. Pediatrics. (2014) 134(4):e1229–43. doi: 10.1542/peds.2014-2173
25. Zheng HF, Tobias JH, Duncan E, Evans DM, Eriksson J, Paternoster L, et al. WNT16 influences bone mineral density, cortical bone thickness, bone strength, and osteoporotic fracture risk. PLoS Genet. (2012) 8(7):e1002745. doi: 10.1371/journal.pgen.1002745
26. Greer FR, Krebs NF, American Academy of Pediatrics Committee on Nutrition. Optimizing bone health and calcium intakes of infants, children, and adolescents. Pediatrics. (2006) 117(2):578–85. doi: 10.1542/peds.2005-2822
27. National Institutes of Health, Office of Dietary Supplements. Calcium: fact sheet for health professionals (2020). Available at: http://ods.od.nih.gov/factsheets/calcium
28. Abrams SA, Griffin IJ, Hicks PD, Gunn SK. Pubertal girls only partially adapt to low dietary calcium intakes. J Bone Miner Res. (2004) 19(5):759–63. doi: 10.1359/JBMR.040122
29. He L, Zhou P, Zhou X, Tian S, Han J, Zhai S. Evaluation of the clinical practice guidelines and consensuses on calcium and vitamin D supplementation in healthy children using the appraisal of guidelines for research and evaluation II instrument and reporting items for practice guidelines in healthcare statement. Front Nutr. (2022) 9:984423. doi: 10.3389/fnut.2022.984423
30. Greene DA, Naughton GA. Calcium and vitamin-D supplementation on bone structural properties in peripubertal female identical twins: a randomised controlled trial. Osteoporos Int. (2011) 22(2):489–98. doi: 10.1007/s00198-010-1317-z
31. Huncharek M, Muscat J, Kupelnick B. Impact of dairy products and dietary calcium on bone-mineral content in children: results of a meta-analysis. Bone (2008) 43(2):312–21. doi: 10.1016/j.bone.2008.02.022
32. Herrmann M, Engelke K, Ebert R, Müller-Deubert S, Rudert M, Ziouti F, et al. Interactions between muscle and bone-where physics meets biology. Biomolecules. (2020) 10(3):432. doi: 10.3390/biom10030432
33. Li G, Zhang L, Wang D, AIQudsy L, Jiang JX, Xu H, et al. Muscle-bone crosstalk and potential therapies for sarco-osteoporosis. J Cell Biochem. (2019) 120(9):14262–73. doi: 10.1002/jcb.28946
34. Goodman CA, Hornberger TA, Robling AG. Bone and skeletal muscle: key players in mechanotransduction and potential overlapping mechanisms. Bone. (2015) 80:24–36. doi: 10.1016/j.bone.2015.04.014
35. Faienza MF, Lassandro G, Chiarito M, Valente F, Ciaccia L, Giordano P. How physical activity across the lifespan can reduce the impact of bone ageing: a literature review. Int J Environ Res Public Health. (2020) 17(6):1862. doi: 10.3390/ijerph17061862
36. Turner CH, Robling AG. Designing exercise regimens to increase bone strength. Exerc Sport Sci Rev. (2003) 31(1):45–50. doi: 10.1097/00003677-200301000-00009
37. Kannus P, Haapasalo H, Sankelo M, Sievänen H, Pasanen M, Heinonen A, et al. Effect of starting age of physical activity on bone mass in the dominant arm of tennis and squash players. Ann. Intern. Med. (1995) 123:27–31. doi: 10.7326/0003-4819-123-1-199507010-00003
38. Robling AG, Turner CH. Mechanical signaling for bone modeling and remodeling. Crit Rev Eukaryot Gene Expr. (2009) 19(4):319–38. doi: 10.1615/critreveukargeneexpr.v19.i4.50
39. Niu Q, Li F, Zhang L, Xu X, Liu Y, Gao J, et al. Role of the wnt/β-catenin signaling pathway in the response of chondrocytes to mechanical loading. Int J Mol Med. (2016) 37(3):755–62. doi: 10.3892/ijmm.2016.2463
40. Goodman CA, Frey JW, Mabrey DM, Jacobs BL, Lincoln HC, You JS, et al. The role of skeletal muscle mTOR in the regulation of mechanical load-induced growth. J Physiol. (2011) 589(Pt 22):5485–501. doi: 10.1113/jphysiol.2011.218255
41. Wong TS, Booth FW. Skeletal muscle enlargement with weight-lifting exercise by rats. J Appl Physiol. (1988) 65(2):950–4. doi: 10.1152/jappl.1988.65.2.950
42. Boström P, Wu J, Jedrychowski MP, Korde A, Ye L, Lo JC, et al. A PGC1-α-dependent myokine that drives brown-fat-like development of white fat and thermogenesis. Nature. (2012) 481(7382):463–8. doi: 10.1038/nature10777
43. Polyzos SA, Kountouras J, Anastasilakis AD, Geladari EV, Mantzoros CS. Irisin in patients with nonalcoholic fatty liver disease. Metab Clin Exp. (2014) 63(2):207–17. doi: 10.1016/j.metabol.2013.09.013
44. Canivet CM, Bonnafous S, Rousseau D, Leclere PS, Lacas-Gervais S, Patouraux S, et al. Hepatic FNDC5 is a potential local protective factor against non-alcoholic fatty liver. Biochim Biophys Acta Mol Basis Dis. (2020) 1866(5):165705. doi: 10.1016/j.bbadis.2020.165705
45. Wrann CD, White JP, Salogiannnis J, Laznik-Bogoslavski D, Wu J, Ma D, et al. Exercise induces hippocampal BDNF through a PGC-1α/FNDC5 pathway. Cell Metab. (2013) 18(5):649–59. doi: 10.1016/j.cmet.2013.09.008
46. Reza MM, Subramaniyam N, Sim CM, Ge X, Sathiakumar D, McFarlane C, et al. Irisin is a pro-myogenic factor that induces skeletal muscle hypertrophy and rescues denervation-induced atrophy. Nat Commun. (2017) 8(1):1104. doi: 10.1038/s41467-017-01131-0
47. Colaianni G, Mongelli T, Cuscito C, Pignataro P, Lippo L, Spiro G, et al. Irisin prevents and restores bone loss and muscle atrophy in hind-limb suspended mice. Sci Rep. (2017) 7(1):2811. doi: 10.1038/s41598-017-02557-8
48. Weigert C, Lehmann R, Hartwig S, Lehr S. The secretome of the working human skeletal muscle–a promising opportunity to combat the metabolic disaster? Proteomics Clin Appl. (2014) 8(1-2):5–18. doi: 10.1002/prca.201300094
49. Hoffmann C, Weigert C. Skeletal muscle as an endocrine organ: the role of myokines in exercise adaptations. Cold Spring Harb Perspect Med. (2017) 7(11):a029793. doi: 10.1101/cshperspect.a029793
50. Paulsen G, Mikkelsen UR, Raastad T, Peake JM. Leucocytes, cytokines and satellite cells: what role do they play in muscle damage and regeneration following eccentric exercise? Exerc Immunol Rev. (2012) 18:42–97.22876722
51. Neubauer O, Sabapathy S, Ashton KJ, Desbrow B, Peake JM, Lazarus R, et al. Time course-dependent changes in the transcriptome of human skeletal muscle during recovery from endurance exercise: from inflammation to adaptive remodeling. J Appl Physiol. (1985) 116(3):274–87. doi: 10.1152/japplphysiol.00909.2013
52. Catoire M, Mensink M, Kalkhoven E, Schrauwen P, Kersten S. Identification of human exercise-induced myokines using secretome analysis. Physiol Genomics. (2014) 46(7):256–67. doi: 10.1152/physiolgenomics.00174.2013
53. Colaianni G, Cuscito C, Mongelli T, Oranger A, Mori G, Brunetti G, et al. Irisin enhances osteoblast differentiation in vitro. Int J Endocrinol. (2014) 2014:902186. doi: 10.1155/2014/902186
54. Colaianni G, Faienza MF, Sanesi L, Brunetti G, Pignataro P, Lippo L, et al. Irisin serum levels are positively correlated with bone mineral status in a population of healthy children. Pediatr Res. (2019) 85(4):484–88. doi: 10.1038/s41390-019-0278-y
55. Faienza MF, Brunetti G, Sanesi L, Colaianni G, Celi M, Piacente L, et al. High irisin levels are associated with better glycemic control and bone health in children with type 1 diabetes. Diabetes Res Clin Pract. (2018) 141:10–7. doi: 10.1016/j.diabres.2018.03.046
56. Zhang J, Valverde P, Zhu X, Murray D, Wu Y, Yu L, et al. Exercise-induced irisin in bone and systemic irisin administration reveal new regulatory mechanisms of bone metabolism. Bone Res. (2017) 5:16056. doi: 10.1038/boneres.2016.56
57. Li H, Qin S, Liang Q, Xi Y, Bo W, Cai M, et al. Exercise training enhances myocardial mitophagy and improves cardiac function via irisin/FNDC5-PINK1/Parkin pathway in MI mice. Biomedicines. (2021) 9(6):701. doi: 10.3390/biomedicines9060701
58. Jedrychowski MP, Wrann CD, Paulo JA, Gerber KK, Szpyt J, Robinson MM, et al. Detection and quantitation of circulating human irisin by tandem mass spectrometry. Cell Metab. (2015) 22(4):734–40. doi: 10.1016/j.cmet.2015.08.001
59. Chang JS, Kim TH, Nguyen TT, Park KS, Kim N, Kong ID. Circulating irisin levels as a predictive biomarker for sarcopenia: a cross-sectional community-based study. Geriatr Gerontol Int. (2017) 17(11):2266–73. doi: 10.1111/ggi.13030
60. Park HS, Kim HC, Zhang D, Yeom H, Lim SK. The novel myokine irisin: clinical implications and potential role as a biomarker for sarcopenia in postmenopausal women. Endocrine. (2019) 64(2):341–8. doi: 10.1007/s12020-018-1814-y
61. Colucci S, Colaianni G, Brunetti G, Ferranti F, Mascetti G, Mori G, et al. Irisin prevents microgravity-induced impairment of osteoblast differentiation in vitro during the space flight CRS-14 mission. FASEB J. (2020) 34(8):10096–106. doi: 10.1096/fj.202000216R
62. Consitt LA, Clark BC. The vicious cycle of myostatin signaling in sarcopenic obesity: myostatin role in skeletal muscle growth, insulin signaling and implications for clinical trials. J Frailty Aging. (2018) 7(1):21–7. doi: 10.14283/jfa.2017.33
63. Behringer M, Gruetzner S, McCourt M, Mester J. Effects of weight-bearing activities on bone mineral content and density in children and adolescents: a meta-analysis. J Bone Miner Res. (2014) 29(2):467–78. doi: 10.1002/jbmr.2036
64. Meyer U, Ernst D, Zahner L, Schindler C, Puder JJ, Kraenzlin M, et al. 3-Year follow-up results of bone mineral content and density after a school-based physical activity randomized intervention trial. Bone. (2013) 55(1):16–22. doi: 10.1016/j.bone.2013.03.005
65. Foley S, Quinn S, Dwyer T, Venn A, Jones G. Measures of childhood fitness and body mass index are associated with bone mass in adulthood: a 20-year prospective study. J Bone Miner Res. (2008) 23(7):994–1001. doi: 10.1359/jbmr.080223
66. Tervo T, Nordström P, Neovius M, Nordström A. Constant adaptation of bone to current physical activity level in men: a 12-year longitudinal study. J Clin Endocrinol Metab. (2008) 93(12):4873–9. doi: 10.1210/jc.2008-1313
67. Duckham RL, Baxter-Jones AD, Johnston JD, Vatanparast H, Cooper D, Kontulainen S. Does physical activity in adolescence have site-specific and sex-specific benefits on young adult bone size, content, and estimated strength? J Bone Miner Res. (2014) 29(2):479–86. doi: 10.1002/jbmr.2055
68. Santos L, Elliott-Sale KJ, Sale C. Exercise and bone health across the lifespan. Biogerontology. (2017) 18(6):931–46. doi: 10.1007/s10522-017-9732-6
69. Tveit M, Rosengren BE, Nilsson JÅ, Karlsson MK. Exercise in youth: high bone mass, large bone size, and low fracture risk in old age. Scand J Med Sci Sports. (2015) 25(4):453–61. doi: 10.1111/sms.12305
70. Ireland A, Muthuri S, Rittweger J, Adams JE, Ward KA, Kuh D, et al. Later age at onset of independent walking is associated with lower bone strength at fracture-prone sites in older men. J Bone Miner Res. (2017) 32(6):1209–17. doi: 10.1002/jbmr.3099
71. MacKelvie KJ, Khan KM, McKay HA. Is there a critical period for bone response to weight-bearing exercise in children and adolescents? A systematic review. Br J Sports Med. (2002) 36(4):250–7. doi: 10.1136/bjsm.36.4.250
72. Vlachopoulos D, Barker AR, Ubago-Guisado E, Ortega FB, Krustrup P, Metcalf B, et al. The effect of 12-month participation in osteogenic and non-osteogenic sports on bone development in adolescent male athletes. The PRO-BONE study. J Sci Med Sport. (2018) 21(4):404–9. doi: 10.1016/j.jsams.2017.08.018
73. Ferry B, Lespessailles E, Rochcongar P, Duclos M, Courteix D. Bone health during late adolescence: effects of an 8-month training program on bone geometry in female athletes. Joint Bone Spine. (2013) 80(1):57–63. doi: 10.1016/j.jbspin.2012.01.006
74. Olmedillas H, González-Agüero A, Moreno LA, Casajús JA, Vicente-Rodríguez G. Bone related health status in adolescent cyclists. PLoS One. (2011) 6(9):e24841. doi: 10.1371/journal.pone.0024841
75. Gómez-Bruton A, González-Agüero A, Gómez-Cabello A, Matute-Llorente A, Casajús JA, Vicente-Rodríguez G. The effects of swimming training on bone tissue in adolescence. Scand J Med Sci Sports. (2015) 25(6):e589–602. doi: 10.1111/sms.12378
76. Larsen MN, Nielsen CM, Helge EW, Madsen M, Manniche V, Hansen L, et al. Positive effects on bone mineralisation and muscular fitness after 10 months of intense school-based physical training for children aged 8-10 years: the FIT FIRST randomised controlled trial. Br J Sports Med. (2018) 52(4):254–60. doi: 10.1136/bjsports-2016-096219
77. Bielemann RM, Ramires VV, Wehrmeister FC, Gonçalves H, Assunção MCF, Ekelund U, et al. Is vigorous-intensity physical activity required for improving bone mass in adolescence? Findings from a Brazilian birth cohort. Osteoporos Int. (2019) 30(6):1307–15. doi: 10.1007/s00198-019-04862-6
78. Janz KF, Letuchy EM, Eichenberger Gilmore JM, Burns TL, Torner JC, Willing MC, et al. Early physical activity provides sustained bone health benefits later in childhood. Med Sci Sports Exerc. (2010) 42(6):1072–8. doi: 10.1249/MSS.0b013e3181c619b2
79. Kriemler S, Zahner L, Puder JJ, Braun-Fahrländer C, Schindler C, Farpour-Lambert NJ, et al. Weight-bearing bones are more sensitive to physical exercise in boys than in girls during pre- and early puberty: a cross-sectional study. Osteoporos Int. (2008) 19(12):1749–58. doi: 10.1007/s00198-008-0611-5
80. Macdonald H, Kontulainen S, Petit M, Janssen P, McKay H. Bone strength and its determinants in pre- and early pubertal boys and girls. Bone. (2006) 39(3):598–608. doi: 10.1016/j.bone.2006.02.057
81. Ho-Pham LT, Nguyen UD, Nguyen TV. Association between lean mass, fat mass, and bone mineral density: a meta-analysis. J Clin Endocrinol Metab. (2014) 99(1):30–8. doi: 10.1210/jc.2014-v99i12-30A
82. Zribi A, Chaari H, Masmoudi L, Dardouri W, Khanfir MA, Bouajina E, et al. Volleyball practice increases bone mass in prepubescent boys during growth: a 1-year longitudinal study. PLoS One. (2022) 17(4):e0266257. doi: 10.1371/journal.pone.0266257
83. Gwinnutt JM, Wieczorek M, Cavalli G, Balanescu A, Bischoff-Ferrari HA, Boonen A, et al. Effects of physical exercise and body weight on disease-specific outcomes of people with rheumatic and musculoskeletal diseases (RMDs): systematic reviews and meta-analyses informing the 2021 EULAR recommendations for lifestyle improvements in people with RMDs. RMD Open. (2022) 8(1):e002168. doi: 10.1136/rmdopen-2021-002168
84. Groen WG, Takken T, van der Net J, Helders PJ, Fischer K. Habitual physical activity in Dutch children and adolescents with haemophilia. Haemophilia. (2011) 17(5):e906–12. doi: 10.1111/j.1365-2516.2011.02555.x
85. Wang M, Álvarez-Román MT, Chowdary P, Quon DV, Schafer K. Physical activity in individuals with haemophilia and experience with recombinant factor VIII fc fusion protein and recombinant factor IX fc fusion protein for the treatment of active patients: a literature review and case reports. Blood Coagul Fibrinolysis. (2016) 27(7):737–44. doi: 10.1097/MBC.0000000000000565
86. Giordano P, Brunetti G, Lassandro G, Notarangelo LD, Luciani M, Mura RM, et al. High serum sclerostin levels in children with haemophilia A. Br J Haematol. (2016) 172(2):293–5. doi: 10.1111/bjh.13481
87. Giordano P, Lassandro G, Valente M, Molinari AC, Ieranò P, Coppola A. Current management of the hemophilic child: a demanding interlocutor. Quality of life and adequate cost-efficacy analysis. Pediatr Hematol Oncol. (2014) 31(8):687–702. doi: 10.3109/08880018.2014.930768
88. Lassandro G, Pastore C, Amoruso A, Accettura D, Giordano P. Sport and hemophilia in Italy: an obstacle course. Curr Sports Med Rep. (2018) 17(7):230–31. doi: 10.1249/JSR.0000000000000499
89. Hoefnagels JW, Versloot O, Schrijvers LH, van der Net J, Leebeek FWG, Gouw SC, et al. Sports participation is not associated with adherence to prophylaxis in Dutch patients with haemophilia. Haemophilia. (2021) 27(3):e402–5. doi: 10.1111/hae.14244
90. Howell C, Scott K, Patel DR. Sports participation recommendations for patients with bleeding disorders. Transl Pediatr. (2017) 6(3):174–80. doi: 10.21037/tp.2017.04.07
91. Biasoli C, Baldacci E, Coppola A, De Cristofaro R, Di Minno MND, Lassandro G, et al. Promoting physical activity in people with haemophilia: the MEMO (movement for persons with haEMOphilia) expert consensus project. Blood Transfus. (2022) 20(1):66–77. doi: 10.2450/2021.0138-21
92. Cassidy SB, Schwartz S, Miller JL, Driscoll DJ. Prader-Willi syndrome. Genet Med. (2012) 14(1):10–26. doi: 10.1038/gim.0b013e31822bead0
93. McAlister KL, Fisher KL, Dumont-Driscoll MC, Rubin DA. The relationship between metabolic syndrome, cytokines and physical activity in obese youth with and without Prader-Willi syndrome. J Pediatr Endocrinol Metab. (2018) 31(8):837–45. doi: 10.1515/jpem-2017-053
94. Bellicha A, Coupaye M, Hocquaux L, Speter F, Oppert JM, Poitou C. Increasing physical activity in adult women with Prader-Willi syndrome: a transferability study. J Appl Res Intellect Disabil. (2020) 33(2):258–67. doi: 10.1111/jar.12669
95. Woods SG, Knehans A, Arnold S, Dionne C, Hoffman L, Turner P, et al. The associations between diet and physical activity with body composition and walking a timed distance in adults with Prader-Willi syndrome. Food Nutr Res. (2018) 62. doi: 10.29219/fnr.v62.1343
96. Duran AT, Wilson KS, Castner DM, Tucker JM, Rubin DA. Association between physical activity and bone in children with Prader-Willi syndrome. J Pediatr Endocrinol Metab. (2016) 29(7):819–26. doi: 10.1515/jpem-2015-0233
97. Castner DM, Tucker JM, Wilson KS, Rubin DA. Patterns of habitual physical activity in youth with and without Prader-Willi syndrome. Res Dev Disabil. (2014) 35(11):3081–8. doi: 10.1016/j.ridd.2014.07.035
98. Nordstrøm M, Hansen BH, Paus B, Kolset SO. Accelerometer-determined physical activity and walking capacity in persons with Down syndrome, Williams syndrome and Prader-Willi syndrome. Res Dev Disabil. (2013) 12:4395–403. doi: 10.1016/j.ridd.2013.09.021
99. WHO. WHO guidelines on physical activity and sedentary behavior. Geneva, Switzerland: WHO (2020).
100. Ekelund U, Tarp J, Fagerland MW, Johannessen JS, Hansen BH, Jefferis BJ, et al. Joint associations of accelero-meter measured physical activity and sedentary time with all-cause mortality: a harmonised meta-analysis in more than 44 000 middle-aged and older individuals. Br J Sports Med. (2020) 54(24):1499–506. doi: 10.1136/bjsports-2020-103270
Keywords: musculoskeletal health, children, adolescents, irisin, physical activity, sports, rare diseases
Citation: Faienza MF, Urbano F, Chiarito M, Lassandro G and Giordano P (2023) Musculoskeletal health in children and adolescents. Front. Pediatr. 11:1226524. doi: 10.3389/fped.2023.1226524
Received: 21 May 2023; Accepted: 24 November 2023;
Published: 15 December 2023.
Edited by:
Rashmi Ranjan Das, All India Institute of Medical Sciences Bhubaneswar, IndiaReviewed by:
Nirmal Kumar Mohakud, KIIT University, IndiaJoseph John, All India Institute of Medical Sciences Bhubaneswar, India
Eero Haapala, University of Jyvaskyla, Finland
Vedran Hadzic, University of Ljubljana, Slovenia
© 2023 Faienza, Urbano, Chiarito, Lassandro and Giordano. This is an open-access article distributed under the terms of the Creative Commons Attribution License (CC BY). The use, distribution or reproduction in other forums is permitted, provided the original author(s) and the copyright owner(s) are credited and that the original publication in this journal is cited, in accordance with accepted academic practice. No use, distribution or reproduction is permitted which does not comply with these terms.
*Correspondence: Maria Felicia Faienza bWFyaWFmZWxpY2lhLmZhaWVuemFAdW5pYmEuaXQ=