- 1Division of Pediatric Gastroenterology, Department of Pediatrics, University of North Carolina at Chapel Hill, Chapel Hill, NC, United States
- 2Division of Neonatal-Perinatal Medicine, Department of Pediatrics, University of North Carolina at Chapel Hill, Chapel Hill, NC, United States
Necrotizing enterocolitis (NEC) is an intestinal disease that primarily impacts preterm infants. The pathophysiology of NEC involves a complex interplay of factors that result in a deleterious immune response, injury to the intestinal mucosa, and in its most severe form, irreversible intestinal necrosis. Treatments for NEC remain limited, but one of the most effective preventative strategies for NEC is the provision of breast milk feeds. In this review, we discuss mechanisms by which bioactive nutrients in breast milk impact neonatal intestinal physiology and the development of NEC. We also review experimental models of NEC that have been used to study the role of breast milk components in disease pathophysiology. These models are necessary to accelerate mechanistic research and improve outcomes for neonates with NEC.
Introduction
Necrotizing enterocolitis (NEC) is a severe gastrointestinal disease that impacts 2%–7% of preterm infants (1). Risk factors for NEC include prematurity, low birth weight, delivery via cesarean section, lack of breast milk feeds, microbial dysbiosis, inadequate intestinal perfusion, and exposure to medications such as antibiotics and acid blockers (2). Disease pathogenesis is characterized by unrestrained inflammation, injury to the intestinal epithelium, and bowel ischemia, which can rapidly progress to bowel necrosis, sepsis, and death (3). Treatment options for NEC include the discontinuation of enteral nutrition, gastric decompression, broad-spectrum antibiotics, and surgical removal of necrotic bowel (3). There are no targeted therapies available due to our incomplete understanding of disease pathogenesis; however, it has been well described that breast milk feedings are a protective factor against the development of NEC (4–7). Bioactive components in human milk have been demonstrated to reduce intestinal inflammation, enhance stem cell proliferation, decrease enterocyte apoptosis, and promote the development of a healthy microbiome (5–11).
In this review, we discuss important components of breast milk and their role in intestinal immune homeostasis, barrier function, and the prevention of NEC (Figure 1). Finally, we outline models of NEC that can be utilized for mechanistic studies into the impact of breast milk components on intestinal physiology.
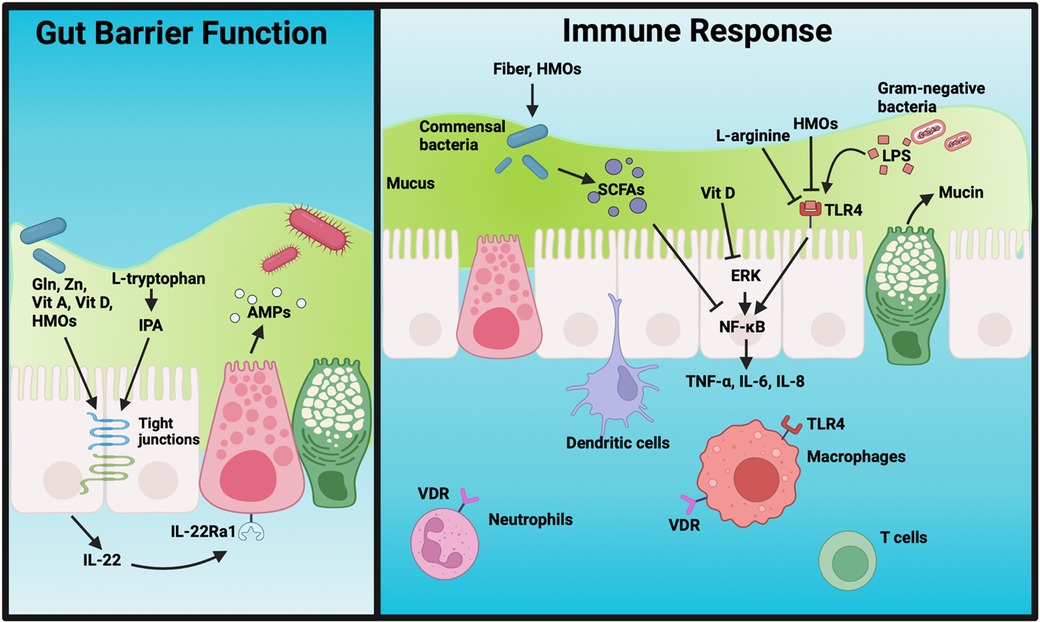
Figure 1. Summary of the impact of nutritional factors on gut barrier integrity and the mucosal immune response. Nutritional components improve the intestinal barrier by enhancing the expression of tight junctions, increasing IL-22 production, promoting mucus secretion, and inducing Paneth cell AMP release. They also have diverse effects on the immune response via modulation of the microbiome, downregulation of inflammatory signaling pathways, and prevention of potentially deleterious immune cell activation. Gln, glutamine; Zn, zinc; Vit A, vitamin A; Vit D, vitamin D; HMOs, human milk oligosaccharides; IPA, 3-indole propionic acid; AMP, antimicrobial peptides; SCFAs, short-chain fatty acids; LPS, lipopolysaccharide; VDR, vitamin D receptor; TLR4, toll-like receptor 4; ERK, extracellular signal-regulated protein kinase; ROS, reactive oxygen species. Figure created with Biorender.com.
Lipids
Breast milk lipids are important in supporting a diverse array of physiologic functions in early life, such as organogenesis, lipid membrane development, and signaling molecule synthesis (12). Long-chain polyunsaturated fatty acids (LC-PUFAs) are a class of bioactive lipids that are predominately acquired during the third trimester of pregnancy (13). This translates into inadequate LC-PUFA stores in preterm neonates and rapid declines in LC-PUFA levels after birth (14). The impact of these deficiencies on intestinal health remains an area of active research. In a study of preterm piglets, enteral provision of a lipid emulsion containing varying ratios of the LC-PUFAs arachidonic acid (ARA, C20:4n-6) and docosahexaenoic acid (DHA, 22:6n-3) found greater villus height in the ileum of piglets that were adequately supplemented with ARA (15). In a rat model of NEC, supplementation of formula with ARA and DHA led to reduced disease severity relative to controls (16). Finally, in vitro studies using human fetal intestinal epithelial cells found that treatment with ARA and/or DHA reduced cytokine production in response to an inflammatory stimulus (17). Additional research is needed in the form of both preclinical models and clinical trials to determine the optimal dose and ratio of LC-PUFA supplementation to support intestinal development and reduce the risk of NEC in preterm infants.
Lactoferrin
Lactoferrin is an abundant component of the whey protein fraction of breast milk that has a diverse array of potentially beneficial functions, including enhancing immunity, controlling inflammation, and promoting intestinal epithelial cell growth (18–21). Host defense properties of lactoferrin arise from iron binding properties as well as direct interactions with microbes and immune cells (22). Clinical trials and a 2020 Cochrane Review have thus far not detected a significant benefit for lactoferrin supplementation in the risk of NEC or mortality for preterm neonates (23–25). Additional studies, such as the Lactoferrin Infant Feeding Trial (LIFT_Canada), are needed to examine the impact of lactoferrin supplementation on the health of preterm neonates (26).
Human milk oligosaccharides (HMOs)
Human milk oligosaccharides (HMOs) are a family of over 150 structurally complex glycans that are abundant in human milk, with concentrations varying based on the stage of lactation (27–30). HMOs are metabolized by intestinal bacteria such as Bifidobacteria and Lactobacilli spp., and thus shape the development of the intestinal microbiome (31). Additionally, HMOs serve a diverse array of potentially beneficial roles in the intestine, including augmenting host defense, modulating immune cell function, and enhancing intestinal barrier integrity (32–34). For example, HMOs act as soluble adhesion receptor decoys, blocking the attachment of viral and bacterial pathogens to intestinal epithelial cells (35, 36). HMOs also possess bacteriostatic and bactericidal properties and can modulate intestinal inflammatory responses (34). In addition, maternal breast milk HMO levels have been associated with an infant's risk of developing NEC (37).
The role of HMOs in attenuating inflammatory immune responses in the gut is well described in preclinical models. In a recent study by Suligoj et al., the effects of HMOs on intestinal barrier function were explored using Caco-2 cell monolayers (38). A combination of 2'-O-fucosyllactose (2’FL), the most abundant oligosaccharide in human milk, and lacto-N-neotetraose (LNnT) was shown to significantly decrease paracellular permeability while increasing tight junction protein (claudin-8) expression (38). In an ex vivo model of human intestinal tissue, galactosyloligosaccharides (GOs) were shown to downregulate TNF-α and interleukin (IL)-1β production (39). In addition, colostrum HMOs, particularly GOs, attenuated Toll-like receptor (TLR) 3 and TLR5 signaling (32). Lastly, the HMO α-3 sialyllactose was shown to downregulate the expression of the inflammatory cytokines IL-8 and IL-12 in Caco-2 cells by inhibiting nuclear factor-κB (NF-κB) signaling and stimulating peroxisome proliferator-activated receptor gamma (PPAR-γ) expression (40).
Similar anti-inflammatory properties of HMOs have been described in animal models of NEC. For example, in a rat model of NEC, the HMO disialyllacto-N-tetraose (DSLNT) increased survival rates from 73.1% to 95% (P < 0.001) and led to a reduction in intestinal pathology (41). A human study found that significantly decreased levels DSLNT in maternal breastmilk were detectable for infants who developed NEC relative to controls (42). In addition, in a mouse model of NEC, administration of 2'FL resulted in a decreased severity of intestinal injury that was associated with improved intestinal perfusion (43). Lastly, the HMOs 2'FL and 6'-sialyllactose (6'-SL) decreased intestinal injury in mouse and piglet models of NEC, which was associated with reductions in TLR4 activation (44). These findings support further investigation into the role of HMO supplementation in the development of a healthy microbiome and prevention of NEC in preterm neonates.
Dietary amino acids
Dietary amino acids (AA) are a primary energy source for intestinal epithelial cells (45). AA in human milk are predominantly protein-bound, with approximately 5%–10% present in free form (46). Free AA are more readily absorbed into the intestinal circulation than their protein-bound counterparts and contribute significantly to the initial rise in AA serum levels in infants following a feed (47). These free AA support intestinal health and may contribute to preventing NEC in preterm infants (45, 48–51). We will discuss amino acids that have been studied in relationship to NEC.
Glutamine
Glutamine (Gln) is the most abundant essential free AA in human milk, particularly in the first three months of lactation (52), and a deficiency in circulating Gln is associated with an increased risk of NEC in neonates (53). The beneficial effects of Gln include promoting intestinal epithelial growth, improving barrier function, reducing oxidative stress, and downregulating inflammation.
Gln promotes intestinal growth by providing energy for intestinal epithelial cell proliferation as well as regulating signaling pathways, including the mammalian target of rapamycin (mTOR), mitogen-activated protein kinase (MAPK), and extracellular signal-regulated protein kinase (ERK) pathways (54). Additionally, Gln enhances the effects of growth factors such as epidermal growth factor (EGF), transforming growth factor alpha (TGFα), and insulin-like growth factor-1 (IGF-1) (54).
Gln is critical in preventing epithelial cell atrophy in catabolic states and improves barrier function by regulating the expression of tight junction proteins, including claudin-1, occludin, and zonula occludens (ZO-1) (55, 56). In a randomized clinical trial, improved intestinal barrier integrity was observed for preterm neonates receiving enteral Gln (57).
Gln exerts anti-oxidative properties by acting as a substrate for glutathione (GSH) biosynthesis (58). GSH is a tripeptide composed of Gln, glycine, and cysteine that scavenges potentially damaging reactive oxidants and free radicals (58). In a study involving breastfed newborn rats, enteral Gln supplementation reduced markers of oxidative stress in intestinal tissue (59). In another study examining intestinal epithelial cells (IECs) in the setting of oxidative and non-oxidative stress, Gln exerted anti-apoptotic properties by decreasing the level of cleaved caspase-3 and increasing the expression of heat shock proteins (53).
Gln has also been shown to downregulate inflammation. In an in vitro study using healthy human intestinal tissue, Gln supplementation downregulated the production of the inflammatory cytokine interleukin-1 beta and upregulated the level of the anti-inflammatory cytokines IL-4 and IL-10 (60). In a rat model of NEC, Gln supplementation was associated with decreased mucosal injury, reduced inflammation, and downregulated expression of the innate immune receptors Toll-like receptor-2 and TLR4 in ileal and colonic tissue (61). Although these studies indicate that Gln may have a beneficial role in intestinal health, a 2016 Cochrane review found that glutamine supplementation was unlikely to significantly improve outcomes for preterm neonates (62).
L-arginine
L-arginine is a semi-essential amino acid exclusively synthesized by intestinal epithelial cells (63). It is a substrate for nitric oxide (NO) production via the arginine-nitric oxide synthase (NOS) pathway, which plays a vital role in regulating intestinal blood flow and maintaining intestinal integrity (64–67).
The role of L-arginine in NEC has been examined in animal models. In a neonatal piglet model of NEC, reduced arginine levels were detected for preterm piglets prior to NEC onset (68). In addition, supplementation of L-arginine attenuated intestinal injury in another study using this model (69). Mechanistically, this was attributed to enhanced NOS activity and NO production in the intestine (69). In a murine model of NEC, endothelial cell TLR4 activation was associated with increased tissue damage and reduced endothelial NOS (eNOS) activity (70). NEC severity was also found to be increased in eNOS-deficient mice (70). In addition, enteral L-arginine supplementation attenuated hypoxia-reoxygenation-induced bowel injury in a murine model of NEC (71).
In neonates, low levels of circulating L-arginine have been associated with an increased risk of NEC (72). Data from animal studies and RCTs support a potential role for L-arginine supplementation in NEC prevention (59, 63, 68, 69, 71–73). However, a 2017 Cochrane review determined that L-arginine supplementation was associated with a significant reduction in the risk of Bell's Stage 1 but not Stage 2 or 3 NEC (74). A large high-quality study is needed before the routine arginine supplementation for preterm neonates can be implemented.
L-Tryptophan
L-tryptophan is an essential amino acid found in human milk (75). It is metabolized by tryptophanase expressed by the gut microbiota leading to the production of tryptamine and indole derivatives such as 3-indole propionic acid (IPA) (76). IPA and other tryptophan metabolites have important roles in gut immunity and intestinal barrier integrity.
IPA regulates intestinal barrier function and inflammation by activating the xenobiotic sensor pregnane-X receptor (PXR) (77). PXR activation upregulates the expression of tight junction proteins and downregulates the expression of the inflammatory cytokine tumor necrosis factor-alpha (TNF-α) (78). In epithelial cell-specific PXR-deficient mouse models, enhanced TLR4 signaling results in significant inflammation and loss of intestinal barrier integrity (79).
Indole derivatives also activate the aryl hydrocarbon receptor (AhR) (80, 81). Decreased AhR expression has been associated with the development of NEC, with reduced levels detected in the intestine of neonates, mice, and piglets with NEC (82). Recent evidence from a murine model of NEC found that administration of the AhR proligand indole-3-carbinol (I3C) resulted in reduced severity of NEC (81). Mechanistically, this was associated with downregulated expression of inflammatory cytokines and increased expression of the polyfunctional cytokine IL-22, which has been shown to be an effective therapeutic against NEC (27, 81, 82). Further investigation is needed to determine the protective mechanisms induced by tryptophan metabolites in both animal models and human studies.
Vitamins
Vitamin D
Vitamin D is important in immunoregulation and enhancement of intestinal barrier function. Vitamin D exerts diverse immunomodulatory effects by binding to vitamin D receptors (VDR) expressed on immune cells (83, 84). For example, vitamin D inhibits Th17 differentiation and decreases IL-17 production (85). VDR activation also inhibits IL-17 expression in the intestine and reduces IEC apoptosis by blocking NF-κB activation (86). Moreover, activation of VDR signaling reduces tissue damage by promoting T-cell differentiation into Th2 cells rather than inflammatory Th1 cells (87). T-cell phenotype is important in the pathogenesis of NEC, with a role for increased Th17 cells and IL-17-related inflammatory signaling in disease development (88, 89).
Vitamin D deficiency is prevalent in preterm infants, particularly in those below 32 weeks of gestation, and decreased levels of vitamin D have been associated with NEC (90). The role of Vitamin D in supporting intestinal health has been supported by findings in animal models. In a rat model of NEC, vitamin D downregulated TLR4 expression and attenuated apoptosis of intestinal epithelial cells (91). Moreover, vitamin D protected against intestinal barrier disruption and the loss of tight junction proteins by increasing occludin expression (91). In another study, supplementation of vitamin D to lipopolysaccharide (LPS)-treated cells improved cell viability, increased proliferation and growth, and decreased expression of IL-6, IL-1β, and TNF-α (92). Although the protective role of vitamin D is documented using human cell lines and mouse models, there is limited data available on the impact of vitamin D supplementation in NEC prevention.
Vitamin A
Vitamin A is present in human milk, but concentrations are significantly lower in milk from mothers of preterm infants (93). Vitamin A levels also vary by lactational stage with higher levels found in colostrum relative to mature milk (94). In addition, serum levels of vitamin A in patients with NEC are decreased relative to healthy controls (95). It is possible that Vitamin A is involved in improving intestinal health in preterm neonates, as it has been previously implicated in regulating intestinal immunity and in maintaining intestinal barrier function (96).
Studies in mice found that the intestinal mucosa of vitamin A deficient mice contains a reduced number of immune cells, including macrophages, B- and T-cells (97, 98). Vitamin A deficiency in rats is associated with an increased abundance of Escherichia coli, decreased mucin-2 (MUC2) and defensin-6, and upregulation of TLR2 and TLR5 expression in the intestine (99). In a study using a mouse model of NEC, vitamin A supplementation reduced TNF-α and IL-6 mRNA levels relative to controls (100). Vitamin A supplementation also increased the expression levels of claudin-1, occludin, and ZO-1, indicating vitamin A's role in improving intestinal barrier function (95). In another study using murine epithelial cells cultured with retinoic acid (RA), the expression of several tight junction proteins, including occludin, claudin-6, and ZO-1 were induced (101). Finally, decreased permeability and increased transepithelial electrical resistance were noted in another study using intestinal epithelial monolayers grown with all-trans RA (102). These findings support the role of vitamin A in supporting intestinal homeostasis.
Trace elements
Trace elements are micronutrients present in variable concentrations in human milk (103). Essential trace elements such as zinc (Zn), selenium (Se), and calcium (Ca) improve intestinal barrier integrity, modulate the immune response, and interact with the gut microbiota (104–106).
Zinc
Zinc (Zn) is involved in essential metabolic functions such as immunoregulation, reduction of oxidative stress, and development of the intestinal tract (107, 108). Zn is primarily acquired in the third trimester of pregnancy leading to low stores in preterm infants (100). Zn content in human milk is dependent on the stage of lactation, while absorption is correlated with the maturity of the infant's gut and bioavailability (109–112).
Zn plays an important role in maintaining intestinal barrier integrity. In a study using Caco-2 cells, induced Zn deficiency led to increased intestinal epithelial permeability and decreased expression of tight junction proteins (113). Similarly, Zn depletion led to the downregulation of occludin and claudin-3 in another study using intestinal Caco-2 cells and ex vivo mouse colons (104). Zn has also been shown to directly enhance the production of intestinal epithelial cells in crypts and promote IEC differentiation, particularly in disease states with increased mucosal turnover (110, 114, 115). Lastly, Zn deficiency decreases mucin synthesis through disturbances in the goblet cell homeostasis (116). Taken together, these data suggest the importance of Zn in maintaining intestinal barrier function.
Several studies highlight Zn's regulation of intestinal immune function. In an in vitro study using chicken intestinal tissue, Zn supplementation (Zn-Gly) increased the expression of secretory immunoglobulin A (IgA), promoted a Th1 and Th2 balance, and reduced the expression of inflammatory cytokines such as TNF-α and IL-1β (117). Zn is also critical for the normal function and morphology of Paneth cells in animal models (118). Similarly, decreased Paneth cell function occurs in human intestinal tissue in response to low levels of Zn (119).
In addition to its immunomodulatory effects, Zn directly affects the composition of the gut microbiota (120). Zn deficiency reduces gut microbial diversity by indirectly promoting the growth of bacteria adapted to low Zn environments, such as Proteobacteria spp. (120). Several studies have associated Gammaproteobacteria, a class of Proteobacteria, with an increased risk for NEC (121–123). Conversely, Zn excess may also lead to gut dysbiosis. Excess levels of Zn in mice colonized with Clostridium difficile were found to exacerbate inflammation and intestinal disease by increasing toxin activity (124). Understanding the interplay between Zn deficiency and the intestinal microbiome could provide new insights into NEC pathophysiology.
Interaction between nutrients and the gut microbiota in NEC
One of the central roles of human breast milk feeds in neonatal health is shaping the development of the neonatal microbiome. Breast milk contains its own microbiome, and these bacteria directly colonize the neonatal intestine (125, 126). In addition, breast milk components directly influence the composition of the gut microbiome. For example, HMOs can facilitate Bifidobacteria and Lactobacilli spp. growth (31), and breast milk IgA supports the growth of Bifidobacteria spp. (127).
There is a complex interplay between the intestinal microbiome and the developing intestine. For example, commensal bacteria, including Bifidobacterium spp. and Clostridium leptum as well as Faecealbacterium prausnitzii, Eubacterium rectale, and Roseburia spp. produce short-chain fatty acids (SCFAs) (128–130). SCFAs such as butyrate, acetate, and propionate regulate inflammation (131–133). Specifically, butyrate inhibits LPS-induced inflammatory cytokines such as IL-1β, TNF-α, and IL-6 (134). Butyrate also enhances regulatory T-cell development and production of the anti-inflammatory cytokine IL-10 (135). In addition to producing SCFAs, these commensal bacteria occupy a niche in the intestine that prevents the overgrowth of potentially pathogenic bacteria. In preterm neonates, the growth of these harmful bacteria can have devastating consequences, and intestinal microbial dysbiosis has been repeatedly associated with the development of NEC (121–123, 136).
Numerous studies have investigated if increasing the abundance of commensal bacteria in the neonatal intestine with probiotics impacts the incidence of NEC. Although data point to a potential benefit of probiotics (137), this remains an area of controversy within the field of neonatology (138). There is a lack of consistency among probiotics used in clinical trials and the lack of regulation of available commercial products. Further research is needed before probiotics become a standard of care in preventing NEC.
Milk composition by stage of lactation
Human milk composition by stage of lactation has been previously reviewed in detail (139–141). Colostrum is the first stage of milk production and consists of a high concentration of potentially beneficial and immunomodulatory components, including secretory IgA, lactoferrin, growth factors, cytokines, and HMOs (139, 141, 142). Although colostrum contains a high concentration of factors that are protective against NEC such as IgA (143), EGF (5) and HMOs (43), studies investigating provision of an extended course of exclusive colostrum feeding on the risk of NEC are limited by the volume of maternal colostrum available. Over the course of lactation, milk content shifts to a composition that promotes infant growth and development with higher concentrations of lactose and fat in mature milk relative to colostrum, although the composition is influenced by a variety of maternal factors (141).
Donor milk
Donor milk is an alternative source of human milk feeds when maternal milk is not available in adequate quantities. The composition of donor milk is significantly impacted by pasteurization and storage (144–147), and it is generally derived from a pool of high-producing donors, which can also lead to significant differences in milk composition from maternal milk. Meta-analyses point to a reduced risk of NEC for donor milk feeds, although it remains to be determined if there is a significant impact on death or neurodevelopmental impairment (148). The Milk trial is a recently completed randomized control trial that will address these questions by investigating the impact of donor milk vs. formula on neurodevelopmental outcomes 22–26 months.
Breast milk fortification and risk of NEC
The caloric density of human milk feeds is commonly increased with the addition of fortifiers to enhance the growth of preterm neonates. Comparison of human milk-based and bovine milk-based fortifiers has not demonstrated a significant difference in either mortality or morbidity, including in NEC rates, between these types of fortification (149, 150). This remains an area of active research.
Models for studying the roles of nutrients in NEC
Due to the limited availability of human neonatal intestinal samples, mechanistic studies into the pathogenesis of NEC rely upon animal studies and in vitro models. NEC-like intestinal inflammation is induced in neonatal rats, mice, rabbits, and piglets through brief periods of hypoxia, feeding formula, LPS, and bacteria isolated from the microbiota of infants with NEC (151, 152). These models have been used to investigate the roles of prebiotics, probiotics, maternal milk constituents (milk proteins, HMOs), vitamins, fatty acid supplementation, and amino acids in the pathophysiology of NEC (81, 82, 91, 95, 153–155).
Numerous in vitro models and cell lines have been used in studies investigating the mechanisms involved in NEC (156–159). The human colorectal adenocarcinoma cell line, Caco-2, is often used to study intestinal disease; however, these cells are unable to differentiate into goblet cells leading to a lack of mucus secretion. The human colon adenocarcinoma cell line, HT-29, is also used to study NEC and will differentiate and produce mucus-secreting goblet cells in specific cell culture conditions (160). The benefit of using cell lines for mechanistic studies include abundance, reproducibility, and ease of culture. However, the cellular complexity of the intestine is hard to emulate in these static monoculture cell models. In addition, the relevance of findings in these adult tumor cell lines to neonatal disease is questionable. To overcome these difficulties, an ex vivo three-dimensional (3D) human organoid culture was developed to bridge the gap between traditional cell culture and studying primary human samples.
Gastrointestinal organoids are multicellular, 3D structures developed from primary intestinal stem cells (ISCs) or from inducible pluripotent stem cells (iPSCs) (161, 162). Intestinal organoids (also called enteroids) contain multiple intestinal epithelial cell types, which retain their critical structural and functional properties of the intestinal epithelium, such as barrier integrity, mucus and antimicrobial peptide (AMP) secretion, and differentiation capabilities. Therefore, enteroids allow for the study of numerous biologic properties, including barrier function, inflammation, cellular proliferation, therapeutic responses, nutrient effects, and epithelial-microbial interactions (163, 164). Limitations of using enteroids include their polarity and difficulties in co-culturing with immune and endothelial cells (165, 166). These challenges led to the development of novel Gut-on-a-Chip or Intestine-on-a-Chip platforms (167, 168).
The Gut-on-a-Chip platform is a technical advance on enteroid models due to the ability to co-culture multiple cell types, provide a constant flow of media, access the apical side of the epithelium, and mimic intestinal peristalsis via stretch (167). We recently developed a NEC-on-a-Chip model using enteroids cultured from intestinal tissue obtained from neonates undergoing intestinal surgery (168). These enteroids were cultured on a microfluidic device in the presence of an endothelial cell line and the intestinal microbiome of an infant that died from NEC (168). In these culture conditions, we detected cellular and gene expression changes similar to what is observed upon studying samples from neonates with NEC (168). This study highlights the scientific relevance of Gut-on-a-Chip models for mechanistic investigations related to the pathogenesis of NEC.
Conclusions and future directions
The intestine of the preterm neonate faces the difficult task of meeting their nutritional requirements while still undergoing postnatal development and being inundated with microbes and the challenges posed by critical illness. Optimizing the provision of the beneficial components of breast milk is central to supporting neonates through this difficult stage. Disrupted intestinal homeostasis and dysregulated inflammation can lead to NEC. Breast milk provides protection against this dangerous disease, and further research into how modulation of enteral nutrition can prevent NEC and improve outcomes for neonates with NEC remains a priority.
Author contributions
All authors contributed to the article and approved the submitted version.
Funding
MG is supported by National Institutes of Health (NIH) grants R01DK124614, R01DK118568, and R01HD105301, the Chan Zuckerberg Initiative Grant number 2022–316749, and the University of North Carolina at Chapel Hill Department of Pediatrics. LCF is supported by a Thrasher Research Fund Early Career Award (LCF) and a UNC Children's Development Early Career Investigator Grant (LCF) through the generous support of donors to the University of North Carolina at Chapel Hill.
Conflict of interest
The authors declare that the research was conducted in the absence of any commercial or financial relationships that could be construed as a potential conflict of interest.
Publisher's note
All claims expressed in this article are solely those of the authors and do not necessarily represent those of their affiliated organizations, or those of the publisher, the editors and the reviewers. Any product that may be evaluated in this article, or claim that may be made by its manufacturer, is not guaranteed or endorsed by the publisher.
References
1. Alsaied A, Islam N, Thalib L. Global incidence of necrotizing enterocolitis: a systematic review and meta-analysis. BMC Pediatr. (2020) 20(1):344. doi: 10.1186/s12887-020-02231-5
2. Singh DK, Miller CM, Orgel KA, Dave M, Mackay S, Good M. Necrotizing enterocolitis: bench to bedside approaches and advancing our understanding of disease pathogenesis. Front Pediatr. (2022) 10:1107404. doi: 10.3389/fped.2022.1107404
3. Nino DF, Sodhi CP, Hackam DJ. Necrotizing enterocolitis: new insights into pathogenesis and mechanisms. Nat Rev Gastroenterol Hepatol. (2016) 13(10):590–600. doi: 10.1038/nrgastro.2016.119
4. Nolan LS, Rimer JM, Good M. The role of human milk oligosaccharides and probiotics on the neonatal microbiome and risk of necrotizing enterocolitis: a narrative review. Nutrients. (2020) 12(10):3052. doi: 10.3390/nu12103052
5. Good M, Sodhi CP, Egan CE, Afrazi A, Jia H, Yamaguchi Y, et al. Breast milk protects against the development of necrotizing enterocolitis through inhibition of toll-like receptor 4 in the intestinal epithelium via activation of the epidermal growth factor receptor. Mucosal Immunol. (2015) 8(5):1166–79. doi: 10.1038/mi.2015.30
6. Chowning R, Radmacher P, Lewis S, Serke L, Pettit N, Adamkin DH. A retrospective analysis of the effect of human milk on prevention of necrotizing enterocolitis and postnatal growth. J Perinatol. (2016) 36(3):221–4. doi: 10.1038/jp.2015.179
7. Hair AB, Peluso AM, Hawthorne KM, Perez J, Smith DP, Khan JY, et al. Beyond necrotizing enterocolitis prevention: improving outcomes with an exclusive human milk-based diet. Breastfeed Med. (2016) 11(2):70–4. doi: 10.1089/bfm.2015.0134
8. Nolan LS, Parks OB, Good M. A review of the immunomodulating components of maternal breast milk and protection against necrotizing enterocolitis. Nutrients. (2019) 12(1):14. doi: 10.3390/nu12010014
9. Zivkovic AM, German JB, Lebrilla CB, Mills DA. Human milk glycobiome and its impact on the infant gastrointestinal microbiota. Proc Natl Acad Sci U S A. (2011) 108(Suppl 1):4653–8. doi: 10.1073/pnas.1000083107
10. Laursen MF, Pekmez CT, Larsson MW, Lind MV, Yonemitsu C, Larnkjaer A, et al. Maternal milk microbiota and oligosaccharides contribute to the infant gut microbiota assembly. ISME Commun. (2021) 1(1):21. doi: 10.1038/s43705-021-00021-3
11. Walsh C, Lane JA, van Sinderen D, Hickey RM. Human milk oligosaccharides: shaping the infant gut microbiota and supporting health. J Funct Foods. (2020) 72:104074. doi: 10.1016/j.jff.2020.104074
12. Ganeshalingam M, Enstad S, Sen S, Cheema S, Esposito F, Thomas R. Role of lipidomics in assessing the functional lipid composition in breast milk. Front Nutr. (2022) 9:899401. doi: 10.3389/fnut.2022.899401
13. Haggarty P. Fatty acid supply to the human fetus. Annu Rev Nutr. (2010) 30:237–55. doi: 10.1146/annurev.nutr.012809.104742
14. Martin CR, Dasilva DA, Cluette-Brown JE, Dimonda C, Hamill A, Bhutta AQ, et al. Decreased postnatal docosahexaenoic and arachidonic acid blood levels in premature infants are associated with neonatal morbidities. J Pediatr. (2011) 159(5):743–9. e1−2. doi: 10.1016/j.jpeds.2011.04.039
15. Akinsulire O, Perides G, Anez-Bustillos L, Cluette-Brown J, Nedder A, Pollack E, et al. Early enteral administration of a Complex lipid emulsion supplement prevents postnatal deficits in docosahexaenoic and arachidonic acids and increases tissue accretion of lipophilic nutrients in preterm piglets. JPEN J Parenter Enteral Nutr. (2020) 44(1):69–79. doi: 10.1002/jpen.1697
16. Caplan MS, Russell T, Xiao Y, Amer M, Kaup S, Jilling T. Effect of polyunsaturated fatty acid (PUFA) supplementation on intestinal inflammation and necrotizing enterocolitis (NEC) in a neonatal rat model. Pediatr Res. (2001) 49(5):647–52. doi: 10.1203/00006450-200105000-00007
17. Wijendran V, Brenna JT, Wang DH, Zhu W, Meng D, Ganguli K, et al. Long-chain polyunsaturated fatty acids attenuate the IL-1beta-induced proinflammatory response in human fetal intestinal epithelial cells. Pediatr Res. (2015) 78(6):626–33. doi: 10.1038/pr.2015.154
18. Sherman MP, Bennett SH, Hwang FF, Yu C. Neonatal small bowel epithelia: enhancing anti-bacterial defense with lactoferrin and Lactobacillus GG. Biometals. (2004) 17(3):285–9. doi: 10.1023/B:BIOM.0000027706.51112.62
19. Wisgrill L, Wessely I, Spittler A, Forster-Waldl E, Berger A, Sadeghi K. Human lactoferrin attenuates the proinflammatory response of neonatal monocyte-derived macrophages. Clin Exp Immunol. (2018) 192(3):315–24. doi: 10.1111/cei.13108
20. Comstock SS, Reznikov EA, Contractor N, Donovan SM. Dietary bovine lactoferrin alters mucosal and systemic immune cell responses in neonatal piglets. J Nutr. (2014) 144(4):525–32. doi: 10.3945/jn.113.190264
21. Liu J, Zhu H, Li B, Robinson SC, Lee C, O'Connell JS, et al. Lactoferrin reduces necrotizing enterocolitis severity by upregulating intestinal epithelial proliferation. Eur J Pediatr Surg. (2020) 30(1):90–5. doi: 10.1055/s-0039-1693728
22. Telang S. Lactoferrin: a critical player in neonatal host defense. Nutrients. (2018) 10(9):1228. doi: 10.3390/nu10091228
23. Pammi M, Suresh G. Enteral lactoferrin supplementation for prevention of sepsis and necrotizing enterocolitis in preterm infants. Cochrane Database Syst Rev. (2020) 3(3):CD007137. doi: 10.1002/14651858.CD007137
24. group Eti. Enteral lactoferrin supplementation for very preterm infants: a randomised placebo-controlled trial. Lancet. (2019) 393(10170):423–33. doi: 10.1016/S0140-6736(18)32221-9
25. Ochoa TJ, Zegarra J, Bellomo S, Carcamo CP, Cam L, Castaneda A, et al. Randomized controlled trial of bovine lactoferrin for prevention of sepsis and neurodevelopment impairment in infants weighing less than 2000 grams. J Pediatr. (2020) 219:118–25.e5. doi: 10.1016/j.jpeds.2019.12.038
26. Asztalos EV, Barrington K, Lodha A, Tarnow-Mordi W, Martin A. Lactoferrin infant feeding trial_Canada (LIFT_Canada): protocol for a randomized trial of adding lactoferrin to feeds of very-low-birth-weight preterm infants. BMC Pediatr. (2020) 20(1):40. doi: 10.1186/s12887-020-1938-0
27. Mihi B, Gong Q, Nolan LS, Gale SE, Goree M, Hu E, et al. Interleukin-22 signaling attenuates necrotizing enterocolitis by promoting epithelial cell regeneration. Cell Rep Med. (2021) 2(6):100320. doi: 10.1016/j.xcrm.2021.100320
28. Thum C, Wall CR, Weiss GA, Wang W, Szeto IM-Y, Day L. Changes in HMO concentrations throughout lactation: influencing factors, health effects and opportunities. Nutrients. (2021) 13(7):2272. doi: 10.3390/nu13072272
29. Chichlowski M, German JB, Lebrilla CB, Mills DA. The influence of milk oligosaccharides on microbiota of infants: opportunities for formulas. Annu Rev Food Sci Technol. (2011) 2:331–51. doi: 10.1146/annurev-food-022510-133743
30. Kuntz S, Rudloff S, Kunz C. Oligosaccharides from human milk influence growth-related characteristics of intestinally transformed and non-transformed intestinal cells. Br J Nutr. (2008) 99(3):462–71. doi: 10.1017/S0007114507824068
31. Thomson P, Medina DA, Garrido D. Human milk oligosaccharides and infant gut bifidobacteria: molecular strategies for their utilization. Food Microbiol. (2018) 75:37–46. doi: 10.1016/j.fm.2017.09.001
32. He Y, Liu S, Leone S, Newburg DS. Human colostrum oligosaccharides modulate major immunologic pathways of immature human intestine. Mucosal Immunol. (2014) 7(6):1326–39. doi: 10.1038/mi.2014.20
33. Moore RE, Xu LL, Townsend SD. Prospecting human milk oligosaccharides as a defense against viral infections. ACS Infect Dis. (2021) 7(2):254–63. doi: 10.1021/acsinfecdis.0c00807
34. Craft KM, Townsend SD. Mother knows best: deciphering the antibacterial properties of human milk oligosaccharides. Acc Chem Res. (2019) 52(3):760–8. doi: 10.1021/acs.accounts.8b00630
35. Laucirica DR, Triantis V, Schoemaker R, Estes MK, Ramani S. Milk oligosaccharides inhibit human rotavirus infectivity in MA104 cells. J Nutr. (2017) 147(9):1709–14. doi: 10.3945/jn.116.246090
36. Ruiz-Palacios GM, Cervantes LE, Ramos P, Chavez-Munguia B, Newburg DS. Campylobacter jejuni binds intestinal H(O) antigen (fuc alpha 1, 2Gal beta 1, 4GlcNAc), and fucosyloligosaccharides of human milk inhibit its binding and infection. J Biol Chem. (2003) 278(16):14112–20. doi: 10.1074/jbc.M207744200
37. Wejryd E, Martí M, Marchini G, Werme A, Jonsson B, Landberg E, et al. Low diversity of human milk oligosaccharides is associated with necrotising enterocolitis in extremely low birth weight infants. Nutrients. (2018) 10(10):1556. doi: 10.3390/nu10101556
38. Šuligoj T, Vigsnæs LK, Abbeele PVD, Apostolou A, Karalis K, Savva GM, et al. Effects of human milk oligosaccharides on the adult gut Microbiota and barrier function. Nutrients. (2020) 12(9):2808. doi: 10.3390/nu12092808
39. Newburg DS, Ko JS, Leone S, Nanthakumar NN. Human milk oligosaccharides and synthetic galactosyloligosaccharides contain 3'-, 4-, and 6'-galactosyllactose and attenuate inflammation in human T84, NCM-460, and H4 cells and intestinal tissue ex vivo. J Nutr. (2016) 146(2):358–67. doi: 10.3945/jn.115.220749
40. Zenhom M, Hyder A, de Vrese M, Heller KJ, Roeder T, Schrezenmeir J. Prebiotic oligosaccharides reduce proinflammatory cytokines in intestinal caco-2 cells via activation of PPARgamma and peptidoglycan recognition protein 3. J Nutr. (2011) 141(5):971–7. doi: 10.3945/jn.110.136176
41. Jantscher-Krenn E, Zherebtsov M, Nissan C, Goth K, Guner YS, Naidu N, et al. The human milk oligosaccharide disialyllacto-N-tetraose prevents necrotising enterocolitis in neonatal rats. Gut. (2012) 61(10):1417–25. doi: 10.1136/gutjnl-2011-301404
42. Masi AC, Embleton ND, Lamb CA, Young G, Granger CL, Najera J, et al. Human milk oligosaccharide DSLNT and gut microbiome in preterm infants predicts necrotising enterocolitis. Gut. (2021) 70(12):2273–82. doi: 10.1136/gutjnl-2020-322771
43. Good M, Sodhi CP, Yamaguchi Y, Jia H, Lu P, Fulton WB, et al. The human milk oligosaccharide 2'-fucosyllactose attenuates the severity of experimental necrotising enterocolitis by enhancing mesenteric perfusion in the neonatal intestine. Br J Nutr. (2016) 116(7):1175–87. doi: 10.1017/S0007114516002944
44. Sodhi CP, Wipf P, Yamaguchi Y, Fulton WB, Kovler M, Nino DF, et al. The human milk oligosaccharides 2'-fucosyllactose and 6'-sialyllactose protect against the development of necrotizing enterocolitis by inhibiting toll-like receptor 4 signaling. Pediatr Res. (2021) 89(1):91–101. doi: 10.1038/s41390-020-0852-3
45. Ma N, Ma X. Dietary amino acids and the gut-microbiome-immune axis: physiological metabolism and therapeutic prospects. Compr Rev Food Sci Food Saf. (2019) 18(1):221–42. doi: 10.1111/1541-4337.12401
46. Carratù B, Concetta B, Francesco S, Elisabetta S. Nitrogenous components of human milk: non-protein nitrogen, true protein and free amino acids. Food Chem. (2003) 81(3):357–62. doi: 10.1016/S0308-8146(02)00430-2
47. Koopman R, Crombach N, Gijsen AP, Walrand S, Fauquant J, Kies AK, et al. Ingestion of a protein hydrolysate is accompanied by an accelerated in vivo digestion and absorption rate when compared with its intact protein. Am J Clin Nutr. (2009) 90(1):106–15. doi: 10.3945/ajcn.2009.27474
48. Beaumont M, Blachier F. Amino acids in intestinal physiology and health. Adv Exp Med Biol. (2020) 1265:1–20. doi: 10.1007/978-3-030-45328-2_1
49. Liu Y, Hou Y, Wang G, Zheng X, Hao H. Gut microbial metabolites of aromatic amino acids as signals in host-microbe interplay. Trends Endocrinol Metab. (2020) 31(11):818–34. doi: 10.1016/j.tem.2020.02.012
50. Leitao-Goncalves R, Carvalho-Santos Z, Francisco AP, Fioreze GT, Anjos M, Baltazar C, et al. Commensal bacteria and essential amino acids control food choice behavior and reproduction. PLoS Biol. (2017) 15(4):e2000862. doi: 10.1371/journal.pbio.2000862
51. Kedia-Mehta N, Finlay DK. Competition for nutrients and its role in controlling immune responses. Nat Commun. (2019) 10(1):2123. doi: 10.1038/s41467-019-10015-4
52. Agostoni C, Carratu B, Boniglia C, Lammardo AM, Riva E, Sanzini E. Free glutamine and glutamic acid increase in human milk through a three-month lactation period. J Pediatr Gastroenterol Nutr. (2000) 31(5):508–12. doi: 10.1097/00005176-200011000-00011
53. Kallweit AR, Baird CH, Stutzman DK, Wischmeyer PE. Glutamine prevents apoptosis in intestinal epithelial cells and induces differential protective pathways in heat and oxidant injury models. JPEN J Parenter Enteral Nutr. (2012) 36(5):551–5. doi: 10.1177/0148607112445579
54. Marc Rhoads J, Wu G. Glutamine, arginine, and leucine signaling in the intestine. Amino Acids. (2009) 37(1):111–22. doi: 10.1007/s00726-008-0225-4
55. Li N, Neu J. Glutamine deprivation alters intestinal tight junctions via a PI3-K/akt mediated pathway in caco-2 cells. J Nutr. (2009) 139(4):710–4. doi: 10.3945/jn.108.101485
56. Li N, Lewis P, Samuelson D, Liboni K, Neu J. Glutamine regulates caco-2 cell tight junction proteins. Am J Physiol Gastrointest Liver Physiol. (2004) 287(3):G726–33. doi: 10.1152/ajpgi.00012.2004
57. Sevastiadou S, Malamitsi-Puchner A, Costalos C, Skouroliakou M, Briana DD, Antsaklis A, et al. The impact of oral glutamine supplementation on the intestinal permeability and incidence of necrotizing enterocolitis/septicemia in premature neonates. J Matern Fetal Neonatal Med. (2011) 24(10):1294–300. doi: 10.3109/14767058.2011.564240
58. Kang YP, Mockabee-Macias A, Jiang C, Falzone A, Prieto-Farigua N, Stone E, et al. Non-canonical glutamate-cysteine ligase activity protects against ferroptosis. Cell Metab. (2021) 33(1):174–89. e7. doi: 10.1016/j.cmet.2020.12.007
59. Kul M, Vurucu S, Demirkaya E, Tunc T, Aydinoz S, Meral C, et al. Enteral glutamine and/or arginine supplementation have favorable effects on oxidative stress parameters in neonatal rat intestine. J Pediatr Gastroenterol Nutr. (2009) 49(1):85–9. doi: 10.1097/MPG.0b013e318198cd36
60. Coeffier M, Marion R, Ducrotte P, Dechelotte P. Modulating effect of glutamine on IL-1beta-induced cytokine production by human gut. Clin Nutr. (2003) 22(4):407–13. doi: 10.1016/S0261-5614(03)00040-2
61. Zhou W, Li W, Zheng XH, Rong X, Huang LG. Glutamine downregulates TLR-2 and TLR-4 expression and protects intestinal tract in preterm neonatal rats with necrotizing enterocolitis. J Pediatr Surg. (2014) 49(7):1057–63. doi: 10.1016/j.jpedsurg.2014.02.078
62. Moe-Byrne T, Brown JV, McGuire W. Glutamine supplementation to prevent morbidity and mortality in preterm infants. Cochrane Database Syst Rev. (2016) 4(4):CD001457. doi: 10.1002/14651858.CD001457.pub6
63. Puiman PJ, Stoll B, van Goudoever JB, Burrin DG. Enteral arginine does not increase superior mesenteric arterial blood flow but induces mucosal growth in neonatal pigs. J Nutr. (2011) 141(1):63–70. doi: 10.3945/jn.110.131888
64. Alican I, Kubes P. A critical role for nitric oxide in intestinal barrier function and dysfunction. Am J Physiol. (1996) 270(2 Pt 1):G225–37. doi: 10.1152/ajpgi.1996.270.2.G225
65. Stark ME, Szurszewski JH. Role of nitric oxide in gastrointestinal and hepatic function and disease. Gastroenterology. (1992) 103(6):1928–49. doi: 10.1016/0016-5085(92)91454-C
66. Luo CC, Chen HM, Chiu CH, Lin JN, Chen JC. Effect of N(G)-nitro-L-arginine methyl ester on intestinal permeability following intestinal ischemia-reperfusion injury in a rat model. Biol Neonate. (2001) 80(1):60–3. doi: 10.1159/000047121
67. Kubes P. Nitric oxide modulates epithelial permeability in the feline small intestine. Am J Physiol. (1992) 262(6 Pt 1):G1138–42. doi: 10.1152/ajpgi.1992.262.6.G1138
68. Robinson JL, Smith VA, Stoll B, Agarwal U, Premkumar MH, Lau P, et al. Prematurity reduces citrulline-arginine-nitric oxide production and precedes the onset of necrotizing enterocolitis in piglets. Am J Physiol Gastrointest Liver Physiol. (2018) 315(4):G638–G49. doi: 10.1152/ajpgi.00198.2018
69. Di Lorenzo M, Bass J, Krantis A. Use of L-arginine in the treatment of experimental necrotizing enterocolitis. J Pediatr Surg. (1995) 30(2):235–40. discussion 40–1. doi: 10.1016/0022-3468(95)90567-7
70. Yazji I, Sodhi CP, Lee EK, Good M, Egan CE, Afrazi A, et al. Endothelial TLR4 activation impairs intestinal microcirculatory perfusion in necrotizing enterocolitis via eNOS-NO-nitrite signaling. Proc Natl Acad Sci U S A. (2013) 110(23):9451–6. doi: 10.1073/pnas.1219997110
71. Akisu M, Ozmen D, Baka M, Habif S, Yalaz M, Arslanoglu S, et al. Protective effect of dietary supplementation with L-arginine and L-carnitine on hypoxia/reoxygenation-induced necrotizing enterocolitis in young mice. Biol Neonate. (2002) 81(4):260–5. doi: 10.1159/000056757
72. Richir MC, Siroen MP, van Elburg RM, Fetter WP, Quik F, Nijveldt RJ, et al. Low plasma concentrations of arginine and asymmetric dimethylarginine in premature infants with necrotizing enterocolitis. Br J Nutr. (2007) 97(5):906–11. doi: 10.1017/S0007114507669268
73. Mitchell K, Lyttle A, Amin H, Shaireen H, Robertson HL, Lodha AK. Arginine supplementation in prevention of necrotizing enterocolitis in the premature infant: an updated systematic review. BMC Pediatr. (2014) 14:226. doi: 10.1186/1471-2431-14-226
74. Shah PS, Shah VS, Kelly LE. Arginine supplementation for prevention of necrotising enterocolitis in preterm infants. Cochrane Database Syst Rev. (2017) 4(4):CD004339. doi: 10.1002/14651858.CD004339.pub4
75. O'Rourke L, Clarke G, Nolan A, Watkins C, Dinan TG, Stanton C, et al. Tryptophan metabolic profile in term and preterm breast milk: implications for health. J Nutr Sci. (2018) 7:e13. doi: 10.1017/jns.2017.69
76. Meng D, Sommella E, Salviati E, Campiglia P, Ganguli K, Djebali K, et al. Indole-3-lactic acid, a metabolite of tryptophan, secreted by Bifidobacterium longum subspecies infantis is anti-inflammatory in the immature intestine. Pediatr Res. (2020) 88(2):209–17. doi: 10.1038/s41390-019-0740-x
77. Illes P, Krasulova K, Vyhlidalova B, Poulikova K, Marcalikova A, Pecinkova P, et al. Indole microbial intestinal metabolites expand the repertoire of ligands and agonists of the human pregnane X receptor. Toxicol Lett. (2020) 334:87–93. doi: 10.1016/j.toxlet.2020.09.015
78. Li J, Zhang L, Wu T, Li Y, Zhou X, Ruan Z. Indole-3-propionic acid improved the intestinal barrier by enhancing epithelial barrier and mucus barrier. J Agric Food Chem. (2021) 69(5):1487–95. doi: 10.1021/acs.jafc.0c05205
79. Venkatesh M, Mukherjee S, Wang H, Li H, Sun K, Benechet AP, et al. Symbiotic bacterial metabolites regulate gastrointestinal barrier function via the xenobiotic sensor PXR and toll-like receptor 4. Immunity. (2014) 41(2):296–310. doi: 10.1016/j.immuni.2014.06.014
80. Zelante T, Iannitti RG, Cunha C, De Luca A, Giovannini G, Pieraccini G, et al. Tryptophan catabolites from microbiota engage aryl hydrocarbon receptor and balance mucosal reactivity via interleukin-22. Immunity. (2013) 39(2):372–85. doi: 10.1016/j.immuni.2013.08.003
81. Nolan LS, Mihi B, Agrawal P, Gong Q, Rimer JM, Bidani SS, et al. Indole-3-Carbinol-Dependent aryl hydrocarbon receptor signaling attenuates the inflammatory response in experimental necrotizing enterocolitis. Immunohorizons. (2021) 5(4):193–209. doi: 10.4049/immunohorizons.2100018
82. Lu P, Yamaguchi Y, Fulton WB, Wang S, Zhou Q, Jia H, et al. Maternal aryl hydrocarbon receptor activation protects newborns against necrotizing enterocolitis. Nat Commun. (2021) 12(1):1042. doi: 10.1038/s41467-021-21356-4
83. Barragan M, Good M, Kolls JK. Regulation of dendritic cell function by vitamin D. Nutrients. (2015) 7(9):8127–51. doi: 10.3390/nu7095383
84. Chun RF, Liu PT, Modlin RL, Adams JS, Hewison M. Impact of vitamin D on immune function: lessons learned from genome-wide analysis. Front Physiol. (2014) 5:151. doi: 10.3389/fphys.2014.00151
85. Chang SH, Chung Y, Dong C. Vitamin D suppresses Th17 cytokine production by inducing C/EBP homologous protein (CHOP) expression. J Biol Chem. (2010) 285(50):38751–5. doi: 10.1074/jbc.C110.185777
86. Colin EM, Asmawidjaja PS, van Hamburg JP, Mus AM, van Driel M, Hazes JM, et al. 1,25-dihydroxyvitamin D3 modulates Th17 polarization and interleukin-22 expression by memory T cells from patients with early rheumatoid arthritis. Arthritis Rheum. (2010) 62(1):132–42. doi: 10.1002/art.25043
87. Lemire JM, Archer DC, Beck L, Spiegelberg HL. Immunosuppressive actions of 1,25-dihydroxyvitamin D3: preferential inhibition of Th1 functions. J Nutr. (1995) 125(6 Suppl):1704S–8S.7782931
88. Egan CE, Sodhi CP, Good M, Lin J, Jia H, Yamaguchi Y, et al. Toll-like receptor 4-mediated lymphocyte influx induces neonatal necrotizing enterocolitis. J Clin Invest. (2016) 126(2):495–508. doi: 10.1172/JCI83356
89. Tremblay E, Ferretti E, Babakissa C, Burghardt KM, Levy E, Beaulieu JF. IL-17-related signature genes linked to human necrotizing enterocolitis. BMC Res Notes. (2021) 14(1):82. doi: 10.1186/s13104-021-05489-9
90. Zhu T, Liu TJ, Ge X, Kong J, Zhang LJ, Zhao Q. High prevalence of maternal vitamin D deficiency in preterm births in northeast China, Shenyang. Int J Clin Exp Pathol. (2015) 8(2):1459–65. PMID: 25973031.25973031
91. Shi Y, Liu T, Zhao X, Yao L, Hou A, Fu J, et al. Vitamin D ameliorates neonatal necrotizing enterocolitis via suppressing TLR4 in a murine model. Pediatr Res. (2018) 83(5):1024–30. doi: 10.1038/pr.2017.329
92. Lyu C, Jiang S, Kong M, Chen X, Zhang L. Vitamin D protects against necrotising enterocolitis in newborn mice by activating the ERK signalling pathway. Mol Med Rep. (2020) 22(3):2107–14. doi: 10.3892/mmr.2020.11286
93. Redeuil K, Leveques A, Oberson JM, Benet S, Tissot E, Longet K, et al. Vitamins and carotenoids in human milk delivering preterm and term infants: implications for preterm nutrient requirements and human milk fortification strategies. Clin Nutr. (2021) 40(1):222–8. doi: 10.1016/j.clnu.2020.05.012
94. Dror DK, Allen LH. Retinol-to-Fat ratio and retinol concentration in human milk show similar time trends and associations with maternal factors at the population level: a systematic review and meta-analysis. Adv Nutr. (2018) 9(suppl_1):332S–46S. doi: 10.1093/advances/nmy021
95. Xiao S, Li Q, Hu K, He Y, Ai Q, Hu L, et al. Vitamin A and retinoic acid exhibit protective effects on necrotizing enterocolitis by regulating intestinal Flora and enhancing the intestinal epithelial barrier. Arch Med Res. (2018) 49(1):1–9. doi: 10.1016/j.arcmed.2018.04.003
96. de Medeiros PHQS, Pinto DV, de Almeida JZ, Rêgo JMC, Rodrigues FAP, Lima AÂM, et al. Modulation of intestinal immune and barrier functions by vitamin A: implications for current understanding of malnutrition and enteric infections in children. Nutrients. (2018) 10(9):1128. doi: 10.3390/nu10091128
97. Kim CH. Roles of retinoic acid in induction of immunity and immune tolerance. Endocr Metab Immune Disord Drug Targets. (2008) 8(4):289–94. doi: 10.2174/187153008786848312
98. McDaniel KL, Restori KH, Dodds JW, Kennett MJ, Ross AC, Cantorna MT. Vitamin A-deficient hosts become nonsymptomatic reservoirs of Escherichia coli-like enteric infections. Infect Immun. (2015) 83(7):2984–91. doi: 10.1128/IAI.00201-15
99. Amit-Romach E, Uni Z, Cheled S, Berkovich Z, Reifen R. Bacterial population and innate immunity-related genes in rat gastrointestinal tract are altered by vitamin A-deficient diet. J Nutr Biochem. (2009) 20(1):70–7. doi: 10.1016/j.jnutbio.2008.01.002
100. Giles E, Doyle L. Zinc in extremely low-birthweight or very preterm infants. Neoreviews. (2007) 8(4):e165–e72. doi: 10.1542/neo.8-4-e165
101. Kubota H, Chiba H, Takakuwa Y, Osanai M, Tobioka H, Kohama G, et al. Retinoid X receptor alpha and retinoic acid receptor gamma mediate expression of genes encoding tight-junction proteins and barrier function in F9 cells during visceral endodermal differentiation. Exp Cell Res. (2001) 263(1):163–72. doi: 10.1006/excr.2000.5113
102. Yamada S, Kanda Y. Retinoic acid promotes barrier functions in human iPSC-derived intestinal epithelial monolayers. J Pharmacol Sci. (2019) 140(4):337–44. doi: 10.1016/j.jphs.2019.06.012
103. Klein LD, Breakey AA, Scelza B, Valeggia C, Jasienska G, Hinde K. Concentrations of trace elements in human milk: comparisons among women in Argentina, Namibia, Poland, and the United States. PLoS One. (2017) 12(8):e0183367. doi: 10.1371/journal.pone.0183367
104. Miyoshi Y, Tanabe S, Suzuki T. Cellular zinc is required for intestinal epithelial barrier maintenance via the regulation of claudin-3 and occludin expression. Am J Physiol Gastrointest Liver Physiol. (2016) 311(1):G105–16. doi: 10.1152/ajpgi.00405.2015
105. Liu G, Cao W, Jia G, Zhao H, Chen X, Wang J. Calcium-sensing receptor in nutrient sensing: an insight into the modulation of intestinal homoeostasis. Br J Nutr. (2018) 120(8):881–90. doi: 10.1017/S0007114518002088
106. Zhai Q, Cen S, Peng L, Tian F, Zhao J, Zhang H, et al. Effects of dietary selenium supplementation on intestinal barrier and immune responses associated with its modulation of gut Microbiota. Env Sci and Technol Letters. (2018) 5(12):724–30. doi: 10.1021/acs.estlett.8b00563
107. Zlotkin SH, Atkinson S, Lockitch G. Trace elements in nutrition for premature infants. Clin Perinatol. (1995) 22(1):223–40. doi: 10.1016/S0095-5108(18)30310-5
108. Buccigrossi V, Giannattasio A, Armellino C, Lo Vecchio A, Caiazzo MA, Guarino A. The functional effects of nutrients on enterocyte proliferation and intestinal ion transport in early infancy. Early Hum Dev. (2010) 86(Suppl 1):55–7. doi: 10.1016/j.earlhumdev.2010.01.008
109. Bosscher D, Van Caillie-Bertrand M, Robberecht H, Van Dyck K, Van Cauwenbergh R, Deelstra H. In vitro availability of calcium, iron, and zinc from first-age infant formulae and human milk. J Pediatr Gastroenterol Nutr. (2001) 32(1):54–8. doi: 10.1097/00005176-200101000-00016
110. Duff M, Ettarh R. Crypt cell production rate in the small intestine of the zinc-supplemented mouse. Cells Tissues Organs. (2002) 172(1):21–8. doi: 10.1159/000064383
111. Sazawal S, Black RE, Menon VP, Dinghra P, Caulfield LE, Dhingra U, et al. Zinc supplementation in infants born small for gestational age reduces mortality: a prospective, randomized, controlled trial. Pediatrics. (2001) 108(6):1280–6. doi: 10.1542/peds.108.6.1280
112. Mariani E, Mangialasche F, Feliziani FT, Cecchetti R, Malavolta M, Bastiani P, et al. Effects of zinc supplementation on antioxidant enzyme activities in healthy old subjects. Exp Gerontol. (2008) 43(5):445–51. doi: 10.1016/j.exger.2007.10.012
113. Zhong W, McClain CJ, Cave M, Kang YJ, Zhou Z. The role of zinc deficiency in alcohol-induced intestinal barrier dysfunction. Am J Physiol Gastrointest Liver Physiol. (2010) 298(5):G625–33. doi: 10.1152/ajpgi.00350.2009
114. Ohashi W, Kimura S, Iwanaga T, Furusawa Y, Irie T, Izumi H, et al. Zinc transporter SLC39A7/ZIP7 promotes intestinal epithelial self-renewal by resolving ER stress. PLoS Genet. (2016) 12(10):e1006349. doi: 10.1371/journal.pgen.1006349
115. Camilleri M. What is the leaky gut? Clinical considerations in humans. Curr Opin Clin Nutr Metab Care. (2021) 24(5):473–82. doi: 10.1097/MCO.0000000000000778
116. Maares M, Keil C, Straubing S, Robbe-Masselot C, Haase H. Zinc deficiency disturbs mucin expression, O-glycosylation and secretion by intestinal goblet cells. Int J Mol Sci. (2020) 21(17):6149. doi: 10.3390/ijms21176149
117. Jarosz L, Marek A, Gradzki Z, Kwiecien M, Zylinska B, Kaczmarek B. Effect of feed supplementation with zinc glycine chelate and zinc sulfate on cytokine and immunoglobulin gene expression profiles in chicken intestinal tissue. Poult Sci. (2017) 96(12):4224–35. doi: 10.3382/ps/pex253
118. Podany AB, Wright J, Lamendella R, Soybel DI, Kelleher SL. ZnT2-Mediated zinc import into paneth cell granules is necessary for coordinated secretion and paneth cell function in mice. Cell Mol Gastroenterol Hepatol. (2016) 2(3):369–83. doi: 10.1016/j.jcmgh.2015.12.006
119. Kelly P, Feakins R, Domizio P, Murphy J, Bevins C, Wilson J, et al. Paneth cell granule depletion in the human small intestine under infective and nutritional stress. Clin Exp Immunol. (2004) 135(2):303–9. doi: 10.1111/j.1365-2249.2004.02374.x
120. Reed S, Neuman H, Moscovich S, Glahn RP, Koren O, Tako E. Chronic zinc deficiency alters chick gut Microbiota composition and function. Nutrients. (2015) 7(12):9768–84. doi: 10.3390/nu7125497
121. Mshvildadze M, Neu J, Shuster J, Theriaque D, Li N, Mai V. Intestinal microbial ecology in premature infants assessed with non-culture-based techniques. J Pediatr. (2010) 156(1):20–5. doi: 10.1016/j.jpeds.2009.06.063
122. Mai V, Young CM, Ukhanova M, Wang X, Sun Y, Casella G, et al. Fecal microbiota in premature infants prior to necrotizing enterocolitis. PLoS One. (2011) 6(6):e20647. doi: 10.1371/journal.pone.0020647
123. Wang Y, Hoenig JD, Malin KJ, Qamar S, Petrof EO, Sun J, et al. 16S rRNA gene-based analysis of fecal microbiota from preterm infants with and without necrotizing enterocolitis. ISME J. (2009) 3(8):944–54. doi: 10.1038/ismej.2009.37
124. Zackular JP, Moore JL, Jordan AT, Juttukonda LJ, Noto MJ, Nicholson MR, et al. Dietary zinc alters the microbiota and decreases resistance to Clostridium difficile infection. Nat Med. (2016) 22(11):1330–4. doi: 10.1038/nm.4174
125. Jost T, Lacroix C, Braegger CP, Rochat F, Chassard C. Vertical mother-neonate transfer of maternal gut bacteria via breastfeeding. Environ Microbiol. (2014) 16(9):2891–904. doi: 10.1111/1462-2920.12238
126. Asnicar F, Manara S, Zolfo M, Truong DT, Scholz M, Armanini F, et al. Studying vertical microbiome transmission from mothers to infants by strain-level metagenomic profiling. mSystems. (2017) 2(1):e00164-16. doi: 10.1128/mSystems.00164-16
127. Janzon A, Goodrich JK, Koren O, TEDDY Study Group;, Waters JL, Ley RE. Interactions between the gut microbiome and mucosal immunoglobulins A, M, and G in the developing infant gut. mSystems. (2019) 4(6):e00612-19. doi: 10.1128/mSystems.00612-19
128. Jardon KM, Canfora EE, Goossens GH, Blaak EE. Dietary macronutrients and the gut microbiome: a precision nutrition approach to improve cardiometabolic health. Gut. (2022) 71(6):1214–26. doi: 10.1136/gutjnl-2020-323715
129. Nogal A, Valdes AM, Menni C. The role of short-chain fatty acids in the interplay between gut microbiota and diet in cardio-metabolic health. Gut Microbes. (2021) 13(1):1–24. doi: 10.1080/19490976.2021.1897212
130. Cronin P, Joyce SA, O'Toole PW, O'Connor EM. Dietary fibre modulates the gut Microbiota. Nutrients. (2021) 13(5):1655. doi: 10.3390/nu13051655
131. Huang S, Gao Y, Wang Z, Yang X, Wang J, Zheng N. Anti-inflammatory actions of acetate, propionate, and butyrate in fetal mouse jejunum cultures ex vivo and immature small intestinal cells in vitro. Food Sci Nutr. (2022) 10(2):564–76. doi: 10.1002/fsn3.2682
132. Chang PV, Hao L, Offermanns S, Medzhitov R. The microbial metabolite butyrate regulates intestinal macrophage function via histone deacetylase inhibition. Proc Natl Acad Sci U S A. (2014) 111(6):2247–52. doi: 10.1073/pnas.1322269111
133. Zheng N, Gao Y, Zhu W, Meng D, Walker WA. Short chain fatty acids produced by colonizing intestinal commensal bacterial interaction with expressed breast milk are anti-inflammatory in human immature enterocytes. PLoS One. (2020) 15(2):e0229283. doi: 10.1371/journal.pone.0229283
134. Gill PA, Inniss S, Kumagai T, Rahman FZ, Smith AM. The role of diet and gut Microbiota in regulating gastrointestinal and inflammatory disease. Front Immunol. (2022) 13:866059. doi: 10.3389/fimmu.2022.866059
135. Yamamoto EA, Jorgensen TN. Relationships between vitamin D, gut microbiome, and systemic autoimmunity. Front Immunol. (2019) 10:3141. doi: 10.3389/fimmu.2019.03141
136. Warner BB, Tarr PI. Necrotizing enterocolitis and preterm infant gut bacteria. Semin Fetal Neonatal Med. (2016) 21(6):394–9. doi: 10.1016/j.siny.2016.06.001
137. Sharif S, Meader N, Oddie SJ, Rojas-Reyes MX, McGuire W. Probiotics to prevent necrotising enterocolitis in very preterm or very low birth weight infants. Cochrane Database Syst Rev. (2020) 10(10):CD005496. doi: 10.1002/14651858.CD005496.pub5
138. Poindexter B, Committee on Fetus and Newborn. Use of probiotics in preterm infants. Pediatrics. (2021) 147(6):e2021051485. doi: 10.1542/peds.2021-051485
139. Ballard O, Morrow AL. Human milk composition: nutrients and bioactive factors. Pediatr Clin North Am. (2013) 60(1):49–74. doi: 10.1016/j.pcl.2012.10.002
140. Mosca F, Gianni ML. Human milk: composition and health benefits. Pediatr Med Chir. (2017) 39(2):155. doi: 10.4081/pmc.2017.155
141. Andreas NJ, Kampmann B, Mehring Le-Doare K. Human breast milk: a review on its composition and bioactivity. Early Hum Dev. (2015) 91(11):629–35. doi: 10.1016/j.earlhumdev.2015.08.013
142. Castellote C, Casillas R, Ramirez-Santana C, Perez-Cano FJ, Castell M, Moretones MG, et al. Premature delivery influences the immunological composition of colostrum and transitional and mature human milk. J Nutr. (2011) 141(6):1181–7. doi: 10.3945/jn.110.133652
143. Gopalakrishna KP, Macadangdang BR, Rogers MB, Tometich JT, Firek BA, Baker R, et al. Maternal IgA protects against the development of necrotizing enterocolitis in preterm infants. Nat Med. (2019) 25(7):1110–5. doi: 10.1038/s41591-019-0480-9
144. Adhisivam B, Vishnu Bhat B, Rao K, Kingsley SM, Plakkal N, Palanivel C. Effect of holder pasteurization on macronutrients and immunoglobulin profile of pooled donor human milk. J Matern Fetal Neonatal Med. (2019) 32(18):3016–9. doi: 10.1080/14767058.2018.1455089
145. Peila C, Moro GE, Bertino E, Cavallarin L, Giribaldi M, Giuliani F, et al. The effect of holder pasteurization on nutrients and biologically-active components in donor human milk: a review. Nutrients. (2016) 8(8):477. doi: 10.3390/nu8080477
146. Akinbi H, Meinzen-Derr J, Auer C, Ma Y, Pullum D, Kusano R, et al. Alterations in the host defense properties of human milk following prolonged storage or pasteurization. J Pediatr Gastroenterol Nutr. (2010) 51(3):347–52. doi: 10.1097/MPG.0b013e3181e07f0a
147. Colaizy TT. Effects of milk banking procedures on nutritional and bioactive components of donor human milk. Semin Perinatol. (2021) 45(2):151382. doi: 10.1016/j.semperi.2020.151382
148. Quigley M, Embleton ND, McGuire W. Formula versus donor breast milk for feeding preterm or low birth weight infants. Cochrane Database Syst Rev. (2019) 7(7):CD002971. doi: 10.1002/14651858.CD002971.pub5
149. O'Connor DL, Kiss A, Tomlinson C, Bando N, Bayliss A, Campbell DM, et al. Nutrient enrichment of human milk with human and bovine milk-based fortifiers for infants born weighing <1250 g: a randomized clinical trial. Am J Clin Nutr. (2018) 108(1):108–16. doi: 10.1093/ajcn/nqy067
150. Premkumar MH, Pammi M, Suresh G. Human milk-derived fortifier versus bovine milk-derived fortifier for prevention of mortality and morbidity in preterm neonates. Cochrane Database Syst Rev. (2019) 2019(11):CD013145. doi: 10.1002/14651858.CD013145.pub2
151. Nolan LS, Gong Q, Hofmeister HN, Good M. A protocol for the induction of experimental necrotizing enterocolitis in neonatal mice. STAR Protoc. (2021) 2(4):100951. doi: 10.1016/j.xpro.2021.100951
152. Roy SK, Meng Q, Sadowitz BD, Kollisch-Singule M, Yepuri N, Satalin J, et al. Enteral administration of bacteria fermented formula in newborn piglets: a high fidelity model for necrotizing enterocolitis (NEC). PLoS One. (2018) 13(7):e0201172. doi: 10.1371/journal.pone.0201172
153. Sodhi CP, Fulton WB, Good M, Vurma M, Das T, Lai CS, et al. Fat composition in infant formula contributes to the severity of necrotising enterocolitis. Br J Nutr. (2018) 120(6):665–80. doi: 10.1017/S0007114518001836
154. Yu Y, Shiou SR, Guo Y, Lu L, Westerhoff M, Sun J, et al. Erythropoietin protects epithelial cells from excessive autophagy and apoptosis in experimental neonatal necrotizing enterocolitis. PLoS One. (2013) 8(7):e69620. doi: 10.1371/journal.pone.0069620
155. Shen RL, Thymann T, Ostergaard MV, Stoy AC, Krych L, Nielsen DS, et al. Early gradual feeding with bovine colostrum improves gut function and NEC resistance relative to infant formula in preterm pigs. Am J Physiol Gastrointest Liver Physiol. (2015) 309(5):G310–23. doi: 10.1152/ajpgi.00163.2015
156. Artursson P, Karlsson J. Correlation between oral drug absorption in humans and apparent drug permeability coefficients in human intestinal epithelial (caco-2) cells. Biochem Biophys Res Commun. (1991) 175(3):880–5. doi: 10.1016/0006-291X(91)91647-U
157. Vizoso Pinto MG, Rodriguez Gomez M, Seifert S, Watzl B, Holzapfel WH, Franz CM. Lactobacilli stimulate the innate immune response and modulate the TLR expression of HT29 intestinal epithelial cells in vitro. Int J Food Microbiol. (2009) 133(1-2):86–93. doi: 10.1016/j.ijfoodmicro.2009.05.013
158. Zhang D, Wen J, Zhou J, Cai W, Qian L. Milk fat globule membrane ameliorates necrotizing enterocolitis in neonatal rats and suppresses lipopolysaccharide-induced inflammatory response in IEC-6 enterocytes. JPEN J Parenter Enteral Nutr. (2019) 43(7):863–73. doi: 10.1002/jpen.1496
159. Cencic A, Langerholc T. Functional cell models of the gut and their applications in food microbiology–a review. Int J Food Microbiol. (2010) 141(Suppl 1):S4–14. doi: 10.1016/j.ijfoodmicro.2010.03.026
160. Barnett AM, Roy NC, Cookson AL, McNabb WC. Metabolism of caprine milk carbohydrates by probiotic bacteria and caco-2:HT29-MTX epithelial co-cultures and their impact on intestinal barrier integrity. Nutrients. (2018) 10(7):949. doi: 10.3390/nu10070949
161. Sato T, Clevers H. Growing self-organizing mini-guts from a single intestinal stem cell: mechanism and applications. Science. (2013) 340(6137):1190–4. doi: 10.1126/science.1234852
162. Sato T, Vries RG, Snippert HJ, van de Wetering M, Barker N, Stange DE, et al. Single Lgr5 stem cells build crypt-villus structures in vitro without a mesenchymal niche. Nature. (2009) 459(7244):262–5. doi: 10.1038/nature07935
163. Li VSW. Modelling intestinal inflammation and infection using “mini-gut” organoids. Nat Rev Gastroenterol Hepatol. (2021) 18(2):89–90. doi: 10.1038/s41575-020-00391-4
164. Ree IM, Smits-Wintjens VE, Rijntjes-Jacobs EG, Pelsma IC, Steggerda SJ, Walther FJ, et al. Necrotizing enterocolitis in small-for-gestational-age neonates: a matched case-control study. Neonatology. (2014) 105(1):74–8. doi: 10.1159/000356033
165. Taelman J, Diaz M, Guiu J. Human intestinal organoids: promise and challenge. Front Cell Dev Biol. (2022) 10:854740. doi: 10.3389/fcell.2022.854740
166. Burge K, Wilson A, Chaaban H. In vitro apical-out enteroid model of necrotizing enterocolitis. J Vis Exp. (2022) (184):10.3791/64003. doi: 10.3791/64003
167. Kasendra M, Tovaglieri A, Sontheimer-Phelps A, Jalili-Firoozinezhad S, Bein A, Chalkiadaki A, et al. Development of a primary human small intestine-on-a-chip using biopsy-derived organoids. Sci Rep. (2018) 8(1):2871. doi: 10.1038/s41598-018-21201-7
Keywords: breast milk, neonates, prematurity, necrotizing enterocolitis (NEC), intestine, nutrients
Citation: Sami AS, Frazer LC, Miller CM, Singh DK, Clodfelter LG, Orgel KA and Good M (2023) The role of human milk nutrients in preventing necrotizing enterocolitis. Front. Pediatr. 11:1188050. doi: 10.3389/fped.2023.1188050
Received: 16 March 2023; Accepted: 22 May 2023;
Published: 2 June 2023.
Edited by:
Janine Khan, Ann & Robert H. Lurie Children's Hospital of Chicago, United StatesReviewed by:
Yuying Liu, University of Texas Health Science Center at Houston, United StatesRoberto Murgas Torrazza, Secretaría Nacional de Ciencia, Tecnología e Innovación, Panama
© 2023 Sami, Frazer, Miller, Singh, Clodfelter, Orgel and Good. This is an open-access article distributed under the terms of the Creative Commons Attribution License (CC BY). The use, distribution or reproduction in other forums is permitted, provided the original author(s) and the copyright owner(s) are credited and that the original publication in this journal is cited, in accordance with accepted academic practice. No use, distribution or reproduction is permitted which does not comply with these terms.
*Correspondence: Misty Good bWlzdHlnb29kQHVuYy5lZHU=
†These authors have contributed equally to this work