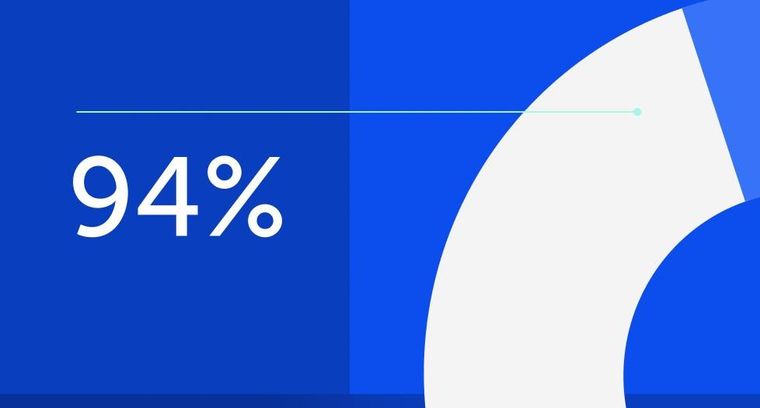
94% of researchers rate our articles as excellent or good
Learn more about the work of our research integrity team to safeguard the quality of each article we publish.
Find out more
REVIEW article
Front. Pediatr., 02 August 2023
Sec. Neonatology
Volume 11 - 2023 | https://doi.org/10.3389/fped.2023.1154611
This article is part of the Research TopicBiomarkers of Gut Blood Flow, Oxygenation, Inflammation and NEC in NeonatesView all 6 articles
The gut is a relatively silent organ in utero but takes on a major role after birth for the absorption and digestion of feed for adequate nutrition and growth. The neonatal circulation undergoes a transition period after birth, and gut perfusion increases rapidly to satisfy the oxygen demand and consumption. If this process is compromised at any stage, preterm and fetal growth restricted infants are at particular risk of gut tissue injury secondary to hypoxia, leading to necrotizing enterocolitis. Feeding can also be a challenge in these high-risk groups due to gut dysmotility. Superior mesenteric artery (SMA) Doppler is a safe, bedside investigation that could rapidly aid clinicians with feeding strategies and in monitoring high-risk infants. This article aims to establish normal patterns of gut blood flow velocity in neonates using SMA Doppler and reviews how it might be used clinically in pathologic states.
Following birth, there is a transition from fetal to newborn circulation, and thereafter, a newborn grows rapidly. Consistent forward flow through the superior mesenteric artery (SMA) to the gut is needed to perfuse the gut in order to support the main gut function, which is the absorption of nutrients for adequate growth. SMA Doppler ultrasound (US) allows us to assess the direction and velocity of gut blood flow; these factors affect a neonate's tolerance to feed. It may also be helpful in predicting and identifying the onset of gut-related diseases, such as necrotizing enterocolitis (NEC), and may guide clinicians on the best timing of blood transfusion (BT) for anemia, as this is currently under debate.
Doppler US has a high frequency and a short wavelength than the audible sound so it can be turned into a beam. A US probe emits a vertical beam and measures the returning echoes to formulate an image; if the echo is received from a moving tissue or cell (e.g., blood flow), then the transmitted and received frequencies are not equal. This is called the Doppler principle.
Blood flowing toward the probe has a higher frequency (red on color Doppler), and blood flowing away from the probe has a lower frequency (blue on color Doppler). Velocity, ultrasound frequency, and angle of insonation all influence the Doppler waveform. The higher the velocity, the higher the Doppler and US frequency; however, tissue penetration is better at lower US frequencies. A balance between the best achievable sensitivity to blood flow and tissue penetration is required. A pulsed wave Doppler (PWD) emits a single wave repeatedly fired at a particular frequency called pulse repetition frequency (PRF), and the echoes received from the moving blood cells will shift in frequency, which determines the blood velocity. PRF is also referred to as a scale on the echo machine. This is important where the blood velocity is not uniform [limited by changes in direction or at the level of valves/shunts such as patent ductus arteriosus (PDA)]. The highest detectable velocity is half the PRF (known as the Nyquist limit). PWD use is limited by aliasing where the blood velocity and beam/flow angle are greater than the Nyquist limit, which causes ambiguity with the results. For example, aliasing may occur if a low scale (low PRF) is used on a vessel with high velocity. This may cause the direction of blood flow to be registered incorrectly (known as color aliasing artifact). To prevent aliasing, the scale should be increased (which increases PRF) and the angle of insonation adjusted.
US is widely used in many aspects of medicine and is useful for screening and prevention (e.g., mammograms), in diagnosis and assessing anatomy, and in assisting procedures (such as vessel catheterization). Doppler US produces flow velocity waveforms that allows physicians to monitor and identify changes in organ perfusion.
The peak systolic velocity (PSV), end diastolic velocity (EDV), and time-averaged mean velocity (TAMV) are used to calculate the pulsatility index (PI) and resistance index (RI) (1). See formula below:
PI and RI are related measures of arterial pulsatility and can identify resistance in the blood vessel. The mean blood flow velocity (BFV) is often used to describe organ perfusion, and Doppler indices add information regarding pattern evolution over time.
Assessing the velocity of blood flow to a tissue alone does not directly describe the oxygenation of that tissue. Several other factors affect the blood's affinity for oxygen and its release to tissues (such as temperature, degree of acidosis, and proportion of fetal hemoglobin), which is well described by the oxyhemoglobin dissociation curve and Bohr effect.
US is a safe and convenient bedside investigation and widely used in neonates in both clinical and research settings. Clinically, it is used regularly for the monitoring of the brain and is a valid bedside test to exclude intraventricular hemorrhage and parenchymal changes. US and Doppler US are also used to assess the neonatal skeleton, heart, abdomen, kidneys, and liver.
The primitive gut (made up of fore, mid, and hind gut) is supplied by three anterior aortic abdominal arteries. The coeliac trunk supplies the foregut (esophagus, stomach, and proximal duodenum as well as the hepatobiliary structures, pancreas, and spleen); the SMA supplies the midgut (the duodenum distal to ampulla of Vater, jejunum, and ileum; ascending colon and proximal two-thirds of the transverse colon); and the inferior mesenteric artery supplies the hindgut (distal third of the transverse colon, descending colon, and rectum) (2).
In utero nutrients pass via the placenta from the mother to the fetus, and the gut vasculature is relatively inactive. Achiron et al. investigated the changes in fetal SMA flow from 14 to 37 weeks gestation in normal fetuses; as expected, the intestinal resistance remained high throughout the pregnancy (as indicated by PI) (3).
Gut oxygen demand changes dramatically after birth. The relatively silent organ in utero requires a rapid switch to immediately absorb and digest nutrients for an animal to grow. Animal and human studies suggest that human gut maturation starts earlier in fetal life but is slower than other mammals postnatally (well beyond the age of weaning) (4, 5). This could be impacted by preterm birth where the gut and lungs are immature. The fetus comes from a relatively hypoxic environment in utero, and a rapid and dramatic rise of arterial oxygenation is seen when breathing is established. If this does not occur (e.g., due to immature preterm lungs/birth asphyxia), then the gut does not function as expected [which explains the increased risk of NEC seen in preterm and fetal growth restriction (FGR) infants] (5). As the SMA irrigates the majority of the gut of interest in neonatology, few studies have interrogated the SMA blood flow velocities delineating the effects of various factors such as growth restriction and prematurity (Table 1).
Gut disease in neonates is a particular challenge as we rely upon radiographic and laboratory findings to assess likelihood of diagnosis. However, many of these findings are not specific to that pathology, rather they appear in multiple gut pathologies. NEC is a potentially devastating inflammatory bowel condition, which affects mainly preterm infants (up to 10%) (6); however, the incidence differs depending on gestation, birth weight, and country at birth (7).
Gordon described that the diagnostic “pitfall” surrounding NEC is the inclusion of “NEC-like” illnesses in NEC data sets (8). For example, clinical signs of spontaneous intestinal perforation (SIP) are akin to the presentation of NEC. Often, diagnosis of SIP is only made surgically, so these infants may be incorrectly included as NEC if surgery is not warranted. Pneumatosis is pathognomonic for NEC, but is only present in 40% of the cases (9). Much work is being done to tighten NEC diagnosis, and clinically the focus lies on reducing the risk of NEC using feeding strategies. Currently, clinicians err on the side of caution if there are any abdominal concerns and stop feeding/start antibiotics, which are not conducive to good weight gain or beneficial to the gut microbiome (10).
Up to 90% of extremely preterm infants require a BT to treat anemia in the neonatal unit. However, evidence for treating anemia in infants is conflicting; both anemia and BT may play a part in the development of gut tissue injury and NEC, possibly due to a period of gut hypoxia followed by a reperfusion injury. In addition, multiple studies suggest a causal relationship between BT and NEC [known as “transfusion-related NEC” (TRNEC)]. This relationship has been identified as a priority area for international researchers, and a recent meta-analysis of liberal and restrictive BT approaches showed no difference in the incidence of gut tissue injury (11).
SMA Doppler US may assist in identifying those infants who have reduced gut blood flow due to anemia. The basal intestinal vascular resistance drops rapidly after birth, and gut blood flow increases significantly during the first week of life (12). Several factors may impair this transition including anemia (13), need for cardiorespiratory support, and use of parenteral nutrition (12). Our group performed SMA Dopplers pre- and post-transfusion in 59 preterm infants and found higher pre-transfusion SMA PSV in babies ≥8 days of age compared with those who were transfused in the first week of life; however, there were no changes in SMA PSV or EDV following BT (14). Several studies have demonstrated an attenuated response to feed immediately after BT, and this may last for 24–48 h (15). Currently, it is also not clear whether to withhold feeds or not during BT and the international WHEAT trial (WithHolding Enteral feeds Around packed red cell Transfusion to prevent necrotizing enterocolitis in preterm neonates) is likely to answer to this uncertainty (16).
Performing a duplex scan of the mesenteric vessels was first suggested by Nicholls in the mid-1980s to aid the diagnosis of chronic intestinal ischemia in adults (17). Since the 1990s, the patterns of flow in SMA Doppler were established and deemed as highly accurate in detecting SMA stenosis/occlusion with a diagnostic accuracy of up to 90% (18–21). By catheterizing the SMA and using dye dilution in adults, Norryd et al. showed a redistribution in blood flow after a meal and a corresponding drop in vascular resistance (22). Celiac artery flow did not demonstrate change in response to feed and did not affect SMA flow in any studies (17, 22, 23).
Cardiac output is distributed approximately equally to the upper and lower parts of the body in a healthy newborn (14), with abdominal organs accounting for the largest proportion of blood flow to the lower part of the body. After birth, there is a dramatic increase in intestinal growth and oxygen demand, and the SMA BFV increases significantly in the first few weeks of life to support this, particularly in preterm infants where the changes are more rapid. Where this rapid rise is not seen, infants are more likely to have intestinal dysmotility and feed intolerance (24, 25).
Various studies have measured the SMA blood flow in clinically stable neonates (Table 2). Stritzke et al. studied 21 healthy neonates born >36 weeks gestation to characterize brain, gut, and kidney BFV over time during the first 24 h. SMA PI and RI was elevated at birth and dropped significantly over time; it had the highest blood flow and Vmax and the largest variation compared with the other major arteries. The reported Vmax of 70.6 cm/s in SMA at 24 h corresponds with other reported normal values between 38 cm/s and 90 cm/s, and the wide variation is also similar to previous findings (probably related to feeds) (26, 27). These findings support the concept of an independent autoregulation of blood flow in different organs, and the greatest change in SMA occurred in the first 2 h after birth (27, 28). The low resistance, high blood flow pattern is similar to the studies using near-infrared spectroscopy (NIRS) to assess gut oxygenation (29).
Table 2. Studies using SMA Doppler to assess gut blood flow in clinically stable neonates: validation of SMA Doppler in neonates.
Several factors impact intestinal blood flow, both intrinsic (including the cardiovascular status of the baby, mural and humoral control of vasculature) and extrinsic (volume and type of feed and certain drugs). It is important to optimize the factors we can control and understand better how they affect the rate of rise in SMA BFV especially in preterm infants (24). Research suggests that gestational age (GA), weight, FGR, feed, presence of PDA, and cardiovascular abnormalities all influence SMA BFV (30); and neonatal Dopplers correlate with disease severity and may differentiate those with anemia (27, 31, 32). The effects of these clinical factors will be individually discussed in more detail.
Compromising blood flow to the intestines causes hypoxia and ischemia. The cause of NEC is multifactorial, but perfusion and oxygenation of the gut certainly plays a part. Researchers have studied the effect of gut pathologies on the SMA blood flow (Table 3). Murdoch et al. postulated that SMA Doppler performed on day 1 may identify infants at risk of NEC and dysmotility. They found that preterm infants with abnormal antenatal Dopplers who later developed NEC were more likely to have a raised PI on day 1 of life, suggesting that abnormal splanchnic circulation in fetal life or vasoconstriction in neonatal life increases the risk of NEC and this could be identified using SMA Doppler (33). However, this was not supported by a more recent study who found SMA Doppler on day 1 of life in preterm FGR infants to be a poor predictor of NEC (34).
Table 3. Studies using SMA Doppler to assess gut blood flow in times of pathology: anemia, blood transfusion, sepsis, and NEC.
The normal pattern of gut blood flow is more difficult to establish than of the other organs as it is a motile organ and variation in intestinal wall tension affects vascular resistance inversely. The GI tract produces hormones that dilate intestinal vessels in response to enteric feed, which also contributes to increased blood flow (14).
Doppler assessment requires trained personnel and is prone to operator-dependent bias. The internal diameter of the vessel affects results and needs to be accurately measured (26). Neonatal blood vessels are <3 mm in diameter, bordering on the limit of resolution due to their size; fortunately, this is now less of a challenge with the availability of the more sophisticated color Doppler equipment (26). The angle of insonation affects results; Coombs et al. described an error of 10% in velocity for a 5% angle error when studying SMA flow, while Bel et al. found that an insonation angle of >15° caused underestimation in velocity (30).
Doppler provides a snapshot in time and does not allow continuous evaluation and does not provide a direct estimate of regional tissue perfusion (35). Other studies have reported difficulties with intestinal gas obscuring the view, infant restlessness, and variations in the course of proximal SMA that led to an unfavorable insonation angle (30).
NEC is least likely to occur in the portion of gut supplied by the coeliac artery. There is no proven correlation between the coeliac artery and SMA Doppler results in infants with NEC (17, 25, 26, 33). Some studies suggest a “qualitatively similar but quantitatively smaller” response to feed compared with SMA Doppler (26) but a significant reduction in the flow volume of the celiac artery has been demonstrated following blood transfusion (36). However, coeliac trunk Doppler to assess gut blood flow in relation to gut tissue injury is unlikely to be clinically useful in neonates.
Bel et al. studied normal values for SMA BFV in 128 preterm and term infants and identified a positive correlation between rising BFV (all parameters) with GA and body weight (30); this was similar to Murdoch et al. (33). Resistance (as evidenced by PI) was higher in lower gestations suggesting that degree of prematurity is associated with higher mesenteric flow resistance (33).
Our group studied infants in three postnatal age groups in the context of blood transfusion and found a significant positive correlation between pre-transfusion PSV and postnatal age; this difference was particularly apparent when comparing infants in the first week of life vs. >28 days. This was attributed to the circulatory adaption after birth and possibly the presence of PDA. However, higher PSV with postnatal age remained significant when corrected for GA, birthweight (BW), and the presence of PDA (37).
Fetal brain sparing or cardiovascular adaptation that occurs during FGR is to the detriment of the “non-essential” fetal organs such as the gut and is particularly evident where abnormal umbilical Doppler results are present (38, 39). Infants predisposed to gut hypoxia develops feed intolerance and therefore are at increased risk of developing NEC (38, 40–43). These infants are also more likely to require BT for anemia (44).
Several studies have demonstrated a positive correlation between gut BFV and body weight (30, 33, 45). FGR infants appear to have different waveforms compared with their appropriately grown for gestational age (AGA) counterparts (30). Infants with FGR have high resistance patterns of flow in the first few days of life particularly if they later develop NEC (34), suggesting that in utero resistance/vasoconstriction persists after birth (33).
In many forms of anemia, the hematocrit is low causing reduced viscosity and increased velocity, and our group have demonstrated higher SMA PSV in anemic preterm infants during the first week of life (46). Anemia may not impair post-prandial increase in mesenteric blood flow but causes a greater than normal increase in velocity in response to feed (47). Blood viscosity increases following BT (up to 20%), and as expected, several studies have demonstrated a concurrent significant reduction in the mean PSV (15, 46, 47). However, it is not clear how long these changes persist for after BT (15). The effects of anemia and blood transfusion on SMA Doppler are summarized in Table 3.
In the septic state, acute hypotensive shock may occur, and vascular bed arterioles constrict in response to pathologic decrease in systemic vascular resistance. Redistribution occurs that reduces splanchnic flow, predisposing infants to gut tissue hypoxia and injury as early as day 1 of life (48). Animal and human models suggest a rise in RI, and a drop in EDV is seen in culture-positive sepsis (49).
The risk of NEC increases with raised PI and falls with high EDV, and infants with risk factors for NEC tend to have higher resistance patterns as early as day 1 of life (48). Studies using SMA Doppler during NEC are outlined in Table 3. However, in active NEC/sepsis, this resistance reduces due to dilatation of the vascular bed and inflammation (26, 33).
Feeding is often difficult in preterm and FGR infants, and clinicians approach cautiously (43). It is the introduction of colostrum after birth that increases gut blood supply and encourages development of vascular bed. Human gut maturation starts early in fetal life, but is slower than other mammals and does not fully mature until beyond the time of weaning onto solid foods (5). Both animal and human models demonstrate a rapid rise in SMA BFV and a drop in vascular resistance on Doppler in the first week of life as feeding is established (5, 45, 50). This is clinically significant as a more rapid rise in SMA BFV reduces gut dysmotility, and a high resistance, reversed or turbulent flow pattern across SMA, is associated with a delay in establishing feeds (5, 50).
In normal infants, enteral feed stimulates intestinal motility and release of circulating vasoactive substance (e.g., CCK P2, secretin, and gastrin), which increases intestinal blood flow. It is accepted that PSV is higher in fed babies, and PSV rises further following a feed. Preterm infants are able to regulate SMA BFV in response to milk feeds, and increases in velocity have been shown to be positively correlated with feeding tolerance (26, 27, 52). Response may also be affected by the feed volume, frequency, and type (11, 25, 50). Havranek et al. demonstrated that the total volume of enteral feed (regardless of human or formula milk) was significantly related to a daily increase in TAMV (12). Lane et al. showed persistent hyperemic state in infants fed by hourly bolus compared with every 3 h bolus feeds with significantly higher pre-prandial PSV (70 vs. 53 cm/s). Those receiving breast milk had a longer but smaller change in amplitude compared with those receiving preterm formula (51), suggesting hourly bolus feeds cause persistent hyperemic state, and the composition of feed and feed interval affects gut perfusion (26, 51). Coombs et al. made similar conclusions with regard to bolus feeds that may persist for up to 2 h (26). Hypoxia/ischemia of intestine may alter the interaction between intestinal motility and the release of vasoactive substances in response to feed since infants who showed poor vasomotor response were symptomatic of gut dysmotility, and almost 50% of these infants went on to develop sepsis (25). This is outlined in more detail in Table 4.
Table 4. Studies using SMA Doppler to assess gut blood flow in the presence of confounding variables: effect of neonatal feeding and PDA.
The presence of a PDA is inversely related to gestational age, and while exact numbers differ, PDA is present in at least 50% of infants born at <28 weeks (52) but may be as high as 90% in <24 week infants and 80% in 25–28 weeks (53). A significant proportion of these is hemodynamically significant PDA (hsPDA) and requires treatment for closure (53, 54). An hsPDA is when a left to right shunt leading to pulmonary overcirculation and a left heart volume overload are seen. Clinically, infants might present with ventilator dependence due to pulmonary edema and ineffective gas exchange. An hsPDA and the resultant ductal steal lead to retrograde diastolic flow in the abdominal aorta, and a low antegrade or retrograde diastolic flow in systemic arteries leading to systemic hypoperfusion (55). A low PSV or reversed end diastolic flow (EDF) with raised PI has been reported in anterior cerebral artery and renal and mesenteric arteries, and the degree of resistance is proportional to the size of the shunt (56). The size of the shunt is proportional to the degree of morbidity (increased risk of intraventricular hemorrhage, NEC, acute kidney injury, and death).
The association between PDA and the risk of NEC is complex. Gut hypoxia has been demonstrated to increase the risk of NEC, and the presence of both PDA and the drugs that encourage PDA closure may cause gut hypoperfusion. Prophylactic surgical ligation may reduce the risk of NEC (57), but more recent RCTs revealed no difference in NEC rates in infants receiving prophylactic pre-symptomatic medical treatment (58). As such, prophylactic treatment of PDA is no longer used, and clinicians approach treatment more cautiously (59, 60).
McCurnin and Clyman found that the presence of a PDA limits the usual increase in post-prandial SMA flow in preterm baboons (61), and similar effects were seen in neonatal studies (55, 62, 63) (Table 4). Our group showed significantly higher PSV in preterm infants with a PDA if they were receiving at least half of the total fluids enterally compared with those who received <50%, but EDV was similar in both feed groups (37), indicating that interrupting the SMA blood flow in response to feed may impede normal digestion (62).
Infants with left ventricular outflow obstruction of any cause (e.g., critical aortic stenosis, coarctation, or interruption of aorta) may be at risk of abnormal BFV of the SMA (reduced PSV and mean velocity and increased resistance) (28). While the ductus arteriosus is open, some blood flow is maintained to the lower extremities. However, when the duct closes, BFV in the distal vessel falls and resistance rises dramatically, causing profound hypoxia to all distal organs including the gut. This can cause a devastating, potentially fatal NEC due to a rapid and profound reduction in blood supply and oxygen delivery and causes gut ischemia.
The use of NIRS in monitoring gut oxygenation in preterm infants is still being understood. While SMA Doppler reflects blood flow, NIRS measures oxygen delivery (and subsequent oxygen extraction). These two aspects of oxygen delivery to an organ are inherently different while still being related. Nevertheless, their association is an important one to understand. Gillam-Krakauer et al. found that the change in splanchnic regional oxygenation (SrSO2) measured using NIRS correlated well with the changes in SMA BFV (using Doppler US) before and after feeds, suggesting that SrSO2 reflects intestinal blood flow and can be used to assess intestinal perfusion (64). Our group performed concurrent SMA Doppler and gut NIRS in investigating the effects of anemia and the changes in response to BT and found that BT improved intestinal oxygenation but did not alter mesenteric BFV, suggesting these two measures may be more clinically useful when used together rather than independently (37).
Lactic acidosis is a non-specific marker of tissue ischemia and is easy to identify on bedside blood test. It would be useful for future work to include SMA Doppler measurements during lactic acidosis, as well as its relationship with known biological markers of gut injury such as intestinal fatty acid binding protein (iFABP), the latter of which is currently being investigated by our group in both FGR and AGA preterm infants.
SMA Doppler is a safe and reliable bedside test to assess gut blood flow for unwell and premature neonates. Doppler can assist in identifying adequate transition from fetal to newborn circulation and may be useful in high-risk infants (e.g., FGR). High resistance patterns of flow persist after birth for approximately 24 h in these infants; perhaps SMA Doppler could identify when this resistance is dropping and guide clinicians when to safely start feeding.
The risk of NEC increases with raised PI and falls with high EDV, and infants with high-risk factors for NEC have high resistance flow patterns on day 1 of life. While an early use of SMA Doppler may identify infants who are at high risk of developing NEC, there is a lack of evidence that the use of routine SMA Doppler would reduce the incidence of NEC. Clinically, SMA Doppler may be a useful tool to assess intestinal perfusion if used alongside gut NIRS.
CM and SB contributed to the study design, screened the search results, reviewed all included studies, drafted the initial manuscript, and reviewed and revised the manuscript. JB contributed to the study design and reviewed and revised the manuscript. NA conceptualized this systematic review and reviewed and revised the manuscript. All authors contributed to the article and approved the submitted version.
The authors declare that the research was conducted in the absence of any commercial or financial relationships that could be construed as a potential conflict of interest.
All claims expressed in this article are solely those of the authors and do not necessarily represent those of their affiliated organizations, or those of the publisher, the editors and the reviewers. Any product that may be evaluated in this article, or claim that may be made by its manufacturer, is not guaranteed or endorsed by the publisher.
FGR, fetal growth restriction; NEC, necrotizing enterocolitis; BFV, blood flow velocity; SMA, superior mesenteric artery; US, ultrasound; PRF, pulse repetition frequency; PWD, pulsed wave Doppler; PDA, patent ductus arteriosus; EDF, end diastolic flow; PSV, peak systolic velocity; EDV, end diastolic velocity; PI, pulsatility index; RI, resistance index; AEDF, absent end diastolic flow; REDF, reversed end diastolic flow; BT, blood transfusion; TRNEC, transfusion-related NEC; hsPDA, hemodynamically significant PDA; NIRS, near-infrared spectroscopy; CA, celiac artery; TAMV, time-averaged mean velocity; Vmean, mean velocity; SGA, small for gestational age; AGA, appropriately grown for gestational age; GA, gestational age; CGA, corrected gestational age; DCC, delayed cord clamping; PN, parenteral nutrition; NG, nasogastric; CHD, congenital heart disease; IVH, intraventricular hemorrhage; SIP, spontaneous intestinal perforation; TTTS, twin to twin transfusion syndrome; A-rSO2, abdominal regional saturation.
1. van Elburg RM, van den Berg A, Bunkers CM, van Lingen RA, Smink EW, van Eyck J. Minimal enteral feeding, fetal blood flow pulsatility, and postnatal intestinal permeability in preterm infants with intrauterine growth retardation. Arch Dis Child Fetal Neonatal Ed. (2004) 89(4):F293–6. doi: 10.1136/adc.2003.027367
2. Zimmerman P, Huseynova K, Pillai L. Chapter 86—anatomy and physiology of the mesenteric circulation. In: Yeo CJ, editor. Shackelford's surgery of the alimentary tract, 2 volume set (eighth edition). Philadelphia, PA: Elsevier (2019). p. 1014–26.
3. Achiron R, Orvieto R, Lipitz S, Yagel S, Rotstein Z. Superior mesenteric artery blood flow velocimetry: cross-sectional Doppler sonographic study in normal fetuses. J Ultrasound Med. (1998) 17(12):769–73. doi: 10.7863/jum.1998.17.12.769
4. Edelstone DI, Holzman IR. Fetal and neonatal intestinal circulations. In: Shepherd AP, Granger DN, editors. Physiology of the intestinal circulation. New York: Raven Press (1984). p. 181–90.
5. Sangild P. Transitions in the life of the gut at birth. In: The digestive physiology of pigs. Proceedings of the 8th symposium; 2000 June 20—22; Uppsala, Sweden. England, UK: CAB publishing (2001). p. 3–17.
6. Costeloe K, E.P.S. Group. EPICure: facts and figures: why preterm labour should be treated. BJOG. (2006) 113(Suppl 3):10–2. doi: 10.1111/j.1471-0528.2006.01118.x
7. Battersby C, Santhalingam T, Costeloe K, Modi N. Incidence of neonatal necrotising enterocolitis in high-income countries: a systematic review. Arch Dis Child Fetal Neonatal Ed. (2018) 103(2):F182–9. doi: 10.1136/archdischild-2017-313880
8. Gordon P, Christensen R, Weitkamp JH, Maheshwari A. Mapping the new world of necrotizing enterocolitis (NEC): review and opinion. EJ Neonatol Res. (2012) 2(4):145–72.23730536
9. Yee WH, Soraisham AS, Shah VS, Aziz KY, Woojin Lee SK. Incidence and timing of presentation of necrotizing enterocolitis in preterm infants. Pediatrics. (2012) 129(2):e298–304. doi: 10.1542/peds.2011-2022
10. Christensen RD, Gordon PV, Besner GE. Can we cut the incidence of necrotizing enterocolitis in half—today? Fetal Pediatr Pathol. (2010) 29(4):185–98. doi: 10.3109/15513815.2010.483874
11. Kirpalani H, Whyte RK, Andersen C, Asztalos EV, Heddle N, Blajchman MA, et al. The premature infants in need of transfusion (PINT) study: a randomized, controlled trial of a restrictive (LOW) versus liberal (HIGH) transfusion threshold for extremely low birth weight infants. J Pediatr. (2006) 149(3):301–7.e3. doi: 10.1016/j.jpeds.2006.05.011
12. Havranek T, Thompson Z, Carver J. Factors that influence mesenteric artery blood flow velocity in newborn preterm infants. J Perinatol. (2006) 26(8):493–7. doi: 10.1038/sj.jp.7211551
13. La Gamma EF, Blau J. Transfusion-related acute gut injury: feeding, flora, flow, and barrier defense. Semin Perinatol. (2012) 36(4):294–305. doi: 10.1053/j.semperi.2012.04.011
14. Banerjee J, Leung TS, Aladangady NA. Blood transfusion in preterm infants improves intestinal tissue oxygenation without alteration in blood flow. Vox Sang. (2016) 111(4):399–408. doi: 10.1111/vox.12436
15. Pitzele A, Rahimi M, Armbrecht E, Havranek T. Packed red blood cell transfusion (PRBC) attenuates intestinal blood flow responses to feedings in pre-term neonates with normalization at 24 h. J Matern Fetal Neonatal Med. (2015) 28(15):1770–3. doi: 10.3109/14767058.2014.971746
16. Gale C. neoEPOCH (2019). Available at: http://neoepoch.com/wheat-trial. (Accessed April 01, 2023)
17. Nicholls SC, Kohler T, Martin RL, Strandness DE. Use of hemodynamic parameters in the diagnosis of mesenteric insufficiency. J Vasc Surg. (1986) 3(3):507–10. doi: 10.1016/0741-5214(86)90117-5
18. Moneta GL, Lee RW, Yeager RA, Taylor LM Jr, Porter JM. Mesenteric duplex scanning: a blinded prospective study. J Vasc Surg. (1993) 17(1):79–86. doi: 10.1016/0741-5214(93)90011-A
19. Lim HK, Lee WJ, Kim SH, Lee SJ, Choi SH, Park HS, et al. Splanchnic arterial stenosis or occlusion: diagnosis at Doppler US. Radiology. (1999) 211(2):405–10. doi: 10.1148/radiology.211.2.r99ma27405
20. Zwolak RM, Fillinger MF, Walsh DB, LaBombard FE, Musson A, Darling CE, et al. Mesenteric and celiac duplex scanning: a validation study. J Vasc Surg. (1998) 27(6):1078–88. doi: 10.1016/S0741-5214(98)60010-0
21. AbuRahma AF, Stone PA, Srivastava M, Dean LS, Keiffer T, Hass SM, et al. Mesenteric/celiac duplex ultrasound interpretation criteria revisited. J Vasc Surg. (2012) 55(2):428–36.e6. doi: 10.1016/j.jvs.2011.08.052
22. Norryd C, Denker H, Lunderquist A, Olin T, Tylén U. Superior mesenteric blood flow during digestion in man. Acta Chir Scand. (1975) 141(3):197–202. PMID: 1166743.1166743
23. Mitchell EL, Moneta GL. Mesenteric duplex scanning. Perspect Vasc Surg Endovasc Ther. (2006) 18(2):175–83. doi: 10.1177/1531003506291885
24. Bozzetti V, Tagliabue PE. Enteral feeding of intrauterine growth restriction preterm infants: theoretical risks and practical implications. Pediatr Med Chir. (2017) 39(2):71–4. doi: 10.4081/pmc.2017.160
25. Fang S, Kempley ST, Gamsu HR. Prediction of early tolerance to enteral feeding in preterm infants by measurement of superior mesenteric artery blood flow velocity. Arch Dis Child Fetal Neonatal Ed. (2001) 85(1):F42. doi: 10.1136/fn.85.1.F42
26. Coombs RC, Morgan M, Durbin GM, Booth IW, McNeish AS. Doppler assessment of human neonatal gut blood flow velocities: postnatal adaptation and response to feeds. J Pediatr Gastroenterol Nutr. (1992) 15(1):6–12. doi: 10.1097/00005176-199207000-00002
27. Stritzke A, Murthy P, Kaur S, Kuret V, Liang Z, Howell S. Arterial flow patterns in healthy transitioning near-term neonates. BMJ Paediatr Open. (2019) 3(1):e000333. doi: 10.1136/bmjpo-2018-000333
28. Martinussen M, Brubakk A, Linker D, Vik T, Yao A. Mesenteric blood flow velocity and its relation to circulatory adaptation during the first week of life in healthy term infants. Pediatr Res. (1994) 36(3):334–9. doi: 10.1203/00006450-199409000-00011
29. Mintzer J, Parvez B, Chelala M, Alpan G, Lagamma E. Quiescent variability of cerebral, renal, and splanchnic regional tissue oxygenation in very low birth weight neonates. J Neonatal-Perinat Med. (2014) 7(3):199–206. doi: 10.3233/NPM-14814035
30. Van Bel F, Van Zwieten P, Guit G, Schipper P, et al. Superior mesenteric artery blood flow velocity and estimated volume flow: duplex Doppler US study of preterm and term neonates. Radiology. (1990) 174(1):165–9. doi: 10.1148/radiology.174.1.2403678
31. Cheung YF, Lam PK, Yeung CY. Early postnatal cerebral Doppler changes in relation to birth weight. Early Hum Dev. (1994) 37(1):57–66. doi: 10.1016/0378-3782(94)90147-3
32. Weissman A, Olanovski I, Weinder Z, Blazer S. Doppler middle cerebral artery peak systolic velocity for diagnosis of neonatal anemia. J Ultrasound Med. (2012) 31(9):1381–5. doi: 10.7863/jum.2012.31.9.1381
33. Murdoch EM, Sinha AK, Shanmugalingam ST, Smith GC, Kempley ST. Doppler flow velocimetry in the superior mesenteric artery on the first day of life in preterm infants and the risk of neonatal necrotizing enterocolitis. Pediatrics. (2006) 118(5):1999–2003. doi: 10.1542/peds.2006-0272
34. Louis D, Mukhopachyay K, Sodhi K, Jain V, Kumar P. Superior mesenteric artery Doppler is poor at predicting feed intolerance and NEC in preterm small for gestational age neonates. J Matern Fetal Neonatal Med. (2013) 26(18):1855–9. doi: 10.3109/14767058.2013.799649
35. Bailey SM, Mally PN. Review of splanchnic oximetry in clinical medicine. J Biomed Opt. (2016) 21(9):091306. doi: 10.1117/1.JBO.21.9.091306
36. Nelle M, Höcker C, Zilow EP, Linderkamp O. Effects of red cell transfusion on cardiac output and blood flow velocities in cerebral and gastrointestinal arteries in premature infants. Arch Dis Child Fetal Neonatal Ed. (1994) 71(1):F45. doi: 10.1136/fn.71.1.F45
37. Banerjee J, Leung TS, Aladangady NA. Blood transfusion in preterm infants improves intestinal tissue oxygenation without alteration in blood flow. Vox Sang. (2016) 111(4):399–408. doi: 10.1111/vox.12436
38. Bozzetti V, Tagliabue PE, Visser GH, van Bel F, Gazzolo D. Feeding issues in IUGR preterm infants. Early Hum Dev. (2013) 89(Suppl 2):S21–3. doi: 10.1016/j.earlhumdev.2013.07.006
39. Flood K, Unterscheider J, Daly S, Geary M, Kennelly M, McAuliffe F. The role of brain sparing in the prediction of adverse outcomes in intrauterine growth restriction: results of the multicenter PORTO study. Am J Obstet Gynecol. (2014) 211(3):288.e1–e5. doi: 10.1016/j.ajog.2014.05.008
40. Bansal S, Deka D, Dhadwal V, Mahendru R. Doppler changes as the earliest parameter in fetal surveillance to detect fetal compromise in intrauterine growth-restricted fetuses. Srp Arh Celok Lek. (2016) 144(1–2):69–73. doi: 10.2298/SARH1602069B
41. Baschat AA, Cosmi E, Bilardo CM, Wolf H, Berg C, Rigano S. Predictors of neonatal outcome in early-onset placental dysfunction. Obstet Gynecol. (2007) 109(2 PART 1):253–61. doi: 10.1097/01.AOG.0000253215.79121.75
42. Sharma D, Farahbakhsh N, Shastri S, Sharma P. Intrauterine growth restriction—part 2. J Matern Fetal Neonatal Med. (2016) 29(24):4037–48. doi: 10.3109/14767058.2016.1154525
43. Kempley S, Gupta N, Linsell L, Dorling J, McCormick K, Mannix P. Feeding infants below 29 weeks’ gestation with abnormal antenatal Doppler: analysis from a randomised trial. Arch Dis Child Fetal Neonatal Ed. (2014) 99(1):6–11. doi: 10.1136/archdischild-2013-304393
44. Patel R, Knezevic A, Shenvi N, Hinkes M, Keene S, Roback J. Association of red blood cell transfusion, anemia, and necrotizing enterocolitis in very low-birth-weight infants. JAMA. (2016) 315(9):889–97. doi: 10.1001/jama.2016.1204
45. Maruyama K, Koizumi T, Tomomasa T, Morikawa A. Intestinal blood-flow velocity in uncomplicated preterm infants during the early neonatal period. Pediatr Radiol. (1999) 29(6):472–7. doi: 10.1007/s002470050621
46. Banerjee J, Leung TS, Aladangady N. Effect of blood transfusion on intestinal blood flow and oxygenation in extremely preterm infants during first week of life. Transfusion. (2016) 56(4):808–15. doi: 10.1111/trf.13434
47. Krimmel GA, Baker R, Yanowitz TD. Blood transfusion alters the superior mesenteric artery blood flow velocity response to feeding in premature infants. Am J Perinatol. (2009) 26(02):99–105. doi: 10.1055/s-0028-1090595
48. Kempley ST, Murdoch E. Splanchnic haemodynamic disturbances in perinatal sepsis. Arch Dis Child Fetal Neonatal Ed. (2000) 83(2):F139–42. doi: 10.1136/fn.83.2.F139
49. Kim HY, Kim IO, Kim WS, Kang GH. Bowel sonography in sepsis with pathological correlation: an experimental study. Pediatr Radiol. (2011) 41(2):237–43. doi: 10.1007/s00247-010-1806-4
50. Robel-Tillig E, Knüpfer M, Pulzer F, Vogtmann C. Blood flow parameters of the superior mesenteric artery as an early predictor of intestinal dysmotility in preterm infants. Pediatr Radiol. (2004) 34(12):958–62. doi: 10.1007/s00247-004-1285-6
51. Lane AJP, Coombs RC, Evans DH, Levin RJ. Effect of feed interval and feed type on splanchnic haemodynamics. Arch Dis Child Fetal Neonatal Ed. (1998) 79(1):F49–53. doi: 10.1136/fn.79.1.F49
52. Sung SI, Chang YS, Kim J, Choi JH, Ahn SY, Park WS. Natural evolution of ductus arteriosus with noninterventional conservative management in extremely preterm infants born at 23-28 weeks of gestation. PLoS One. (2019) 14(2):e0212256. doi: 10.1371/journal.pone.0212256
53. Weinberg JG, Evans FJ, Burns KM, Pearson GD, Kaltman JR. Surgical ligation of patent ductus arteriosus in premature infants: trends and practice variation. Cardiol Young. (2016) 26(6):1107–14. doi: 10.1017/S1047951115001869
54. Clyman RI. Ibuprofen and patent ductus arteriosus. Mass Medical Soc. (2000) 343:728–30. doi: 10.1056/NEJM200009073431009
55. Hamrick SEG, Sallmon H, Rose AR, Porras D, Shelton EL, Reese J. Patent ductus arteriosus of the preterm infant. Pediatrics. (2020) 146(5):1–15. doi: 10.1542/peds.2020-1209
56. Hsu K, Nguyen J, Dekon S, Ramanathan R, Noori S. Effects of patent ductus arteriosus on organ blood flow in infants born very preterm: a prospective study with serial echocardiography. J Pediatr. (2020) 216:95–100.e2. doi: 10.1016/j.jpeds.2019.08.057
57. Cassady G, Crouse D, Kirklin J, Strange M, Joiner CH, Godoy G. A randomized, controlled trial of very early prophylactic ligation of the ductus arteriosus in babies who weighed 1000 g or less at birth. N Engl J Med. (1989) 320(23):1511–6. doi: 10.1056/NEJM198906083202302
58. Ohlsson A, Shah PS. Paracetamol (acetaminophen) for patent ductus arteriosus in preterm or low birth weight infants. Cochrane Database Syst Rev. (2018) 4(4):CD010061. doi: 10.1002/14651858.CD010061.pub3
59. Parkerson S, Philip R, Talati A, Sathanandam S. Management of patent ductus arteriosus in premature infants in 2020. Front Pediatr. (2021) 8:590578. doi: 10.3389/fped.2020.590578
60. Gillam-Krakauer M, Hagadorn JI, Reese J. Pharmacological closure of the patent ductus arteriosus: when treatment still makes sense. J Perinatol. (2019) 39(11):1439–41. doi: 10.1038/s41372-019-0518-3
61. McCurnin D, Clyman RI. Effects of a patent ductus arteriosus on postprandial mesenteric perfusion in premature baboons. Pediatrics. (2008) 122(6):e1262–7. doi: 10.1542/peds.2008-2045
62. Yanowitz T, Reese J, Gillam-Krakauer M, Cochran C, Jegatheesan P, Lau J. Superior mesenteric artery blood flow velocities following medical treatment of a patent ductus arteriosus. J Pediatr. (2014) 164(3):661–3. doi: 10.1016/j.jpeds.2013.11.002
63. Coombs RC, Morgan M, Durbin GM, Booth IW, McNeish AS. Gut blood flow velocities in the newborn: effects of patent ductus arteriosus and parenteral indomethacin. Arch Dis Child. (1990) 65(10 Spec No):1067. doi: 10.1136/adc.65.10_Spec_No.1067
Keywords: gut blood flow, gut perfusion, NEC, preterm infants, doppler ultrasound, superior mesenteric artery doppler
Citation: Murphy C, Baskind S, Aladangady N and Banerjee J (2023) Measuring gut perfusion and blood flow in neonates using ultrasound Doppler of the superior mesenteric artery: a narrative review. Front. Pediatr. 11:1154611. doi: 10.3389/fped.2023.1154611
Received: 30 January 2023; Accepted: 2 May 2023;
Published: 2 August 2023.
Edited by:
Elisabeth M.W. Kooi, University Medical Center Groningen, NetherlandsReviewed by:
Robert Roghair, The University of Iowa, United States© 2023 Murphy, Baskind, Aladangady and Banerjee. This is an open-access article distributed under the terms of the Creative Commons Attribution License (CC BY). The use, distribution or reproduction in other forums is permitted, provided the original author(s) and the copyright owner(s) are credited and that the original publication in this journal is cited, in accordance with accepted academic practice. No use, distribution or reproduction is permitted which does not comply with these terms.
*Correspondence: N. Aladangady bi5hbGFkYW5nYWR5QG5ocy5uZXQ=
Disclaimer: All claims expressed in this article are solely those of the authors and do not necessarily represent those of their affiliated organizations, or those of the publisher, the editors and the reviewers. Any product that may be evaluated in this article or claim that may be made by its manufacturer is not guaranteed or endorsed by the publisher.
Research integrity at Frontiers
Learn more about the work of our research integrity team to safeguard the quality of each article we publish.