- 1Department of Surgery, Indiana University School of Medicine (IUSM), Indianapolis, IN, United States
- 2Riley Hospital for Children, Indiana University Health, Indianapolis, IN, United States
Necrotizing enterocolitis (NEC) is a devastating condition of multi-factorial origin that affects the intestine of premature infants and results in high morbidity and mortality. Infants that survive contend with several long-term sequelae including neurodevelopmental impairment (NDI)—which encompasses cognitive and psychosocial deficits as well as motor, vision, and hearing impairment. Alterations in the gut-brain axis (GBA) homeostasis have been implicated in the pathogenesis of NEC and the development of NDI. The crosstalk along the GBA suggests that microbial dysbiosis and subsequent bowel injury can initiate systemic inflammation which is followed by pathogenic signaling cascades with multiple pathways that ultimately lead to the brain. These signals reach the brain and activate an inflammatory cascade in the brain resulting in white matter injury, impaired myelination, delayed head growth, and eventual downstream NDI. The purpose of this review is to summarize the NDI seen in NEC, discuss what is known about the GBA, explore the relationship between the GBA and perinatal brain injury in the setting of NEC, and finally, highlight the existing research into possible therapies to help prevent these deleterious outcomes.
1. Introduction
Necrotizing enterocolitis (NEC) is a devastating condition that primarily affects premature neonates and is associated with high morbidity and mortality rates (1). The pathophysiology of this disease is multifactorial and is thought to be driven by an immature intestine and immune system, microbial dysbiosis, and a cascade of inflammatory responses (1, 2) that can result in intestinal injury and necrosis, which often progress to requiring surgery and intestinal resection (2). If these neonates survive, they are faced with several downstream complications including intestinal malabsorption, chronic lung disease, and neurodevelopmental impairment (NDI) (3, 4)—much of which is mediated by the complex interplay of the gut-brain axis (GBA). Although it is understood that infants with a history of NEC go on to have worse neurodevelopmental outcomes (3), the pathogenesis of perinatal brain injury in NEC, the causes of downstream development of NDI, and the role of the GBA are not well understood.
The gut-brain axis (GBA) is defined as the interaction of several systems including: the central nervous system (CNS); the autonomic nervous system (ANS); the microbiome; and the many neural, immune, and hormonal signaling pathways that exist between them (5–9). Alterations in the neonatal microbiome and the intestinal injury seen in NEC contribute to pathogenic alterations in GBA signaling (5). This activation then triggers the downstream CNS inflammatory cascade seen in perinatal brain injury, which involves the activation of microglia—the main mediator of the innate immune system's response to brain injury (10). In addition, the stress of prematurity, maternal separation, and formula feeding can further activate the GBA in reverse and exacerbate this disease process (9). NEC usually occurs during a period of crucial and dynamic neurological development leaving the infant particularly susceptible to the pathogenesis of this disease (11), which leads to both short and long-term neurodevelopmental impairment (12). The purpose of this review is to summarize what is known about neurodevelopmental outcomes in NEC, the proposed interplay of the gut-brain axis in the pathophysiology of this disease, and to highlight research into possible therapies to help improve these detrimental outcomes.
2. Neurological and developmental delay seen in NEC
The presence of neurological changes and subsequent NDI in patients with a history of NEC is well established (13, 14). The systemic inflammatory response triggered by NEC may be mediated via bacterial products and cytokines released during intestinal injury. This, combined with the associated hypotension that is part of the systemic inflammatory response, results in signals traversing the GBA and causes well-documented white-matter injury (6, 15). Other neural changes noted include alterations of the brain parenchyma, decreased head circumference, and corresponding decreased volumes in total brain matter (16, 17). This stunted head growth and altered brain parenchyma in early infancy are detrimental to later cognitive outcomes and result in downstream NDI including: a higher incidence of cerebral palsy(CP), impaired motor function, visual and hearing impairment, and cognitive deficits (14, 16, 17).
2.1. Assessing neurodevelopmental impairment
There are a barrage of developmental screening tests for children used for early detection of developmental delays with the goal of identifying if a child has reached specific physical, cognitive, social-emotional, and behavioral milestones (18). It is important to keep in mind that these milestones are often modified by historical and cultural factors and the assessments themselves are limited by the training, availability of assessors, and the education level and socioeconomic status of parents. Despite these difficulties, there remain a series of established assessments that aim to evaluate these milestones, however, no established assessment and timing of assessments exist for looking at NDI in NEC. For this review, we will focus on describing a few assessments that are specifically targeted and validated for screening for developmental delays in high-risk populations (19, 20).
2.1.1. Ages and Stages Questionnaire -3rd edition (ASQ-3)
The ASQ-3 is a developmental screening tool that utilizes a parent-centric model. This questionnaire can be used in both general primary care and in higher-risk categories such as evaluating children that were born prematurely. The questionnaire is given at pre-determined ages (adjusted for corrected gestational age) and tracks the developmental progress of children between the ages of one month to just over 5 years. The benefit of this questionnaire is that it has an easy learning curve for administration, has several different language options, and is quick to administer (20, 21).
2.1.2. Bayley scales of infant and toddler development
The Bayley Scales of Infant and Toddler Developmental assessment is a widely used and the most psychometrically sophisticated assessment of development in infants and toddlers. This scale is advantageous because it is especially useful to screen high-risk populations such as those infants that are pre-term, have lower birth weight, or are from a lower socioeconomic status. It assesses cognition, language, motor, social-emotional, and adaptive behavior with an administration time ranging from 30 to 90 min. Most studies looking at NDI use this assessment, however, the drawbacks are that it is a difficult assessment to administer—requiring specialty training and a lengthy period of time with the patient and their families (19, 20).
2.1.3. Cognitive adaptive test/clinical linguistic auditory milestone scale (CAT/CLAMS) (20)
Like the Bayley Scales Assessment, the Cognitive Adaptive Test/Clinical Linguistic Auditory Milestone Scale (CAT/CLAMS) is another assessment that is practitioner-administered and specifically advantageous for high-risk children—especially from pre-term or low-birth-weight populations. It is a relatively newer assessment but compares favorably to the Bayley assessments and looks at language, problem-solving, and visual motor skills in children from birth to 3 years old. The CAT/CLAMS is also especially useful because it has high validity to target and identify early language delays (20, 22).
2.2. Clinical changes and neurodevelopmental impairment in NEC
A systematic review performed by Rees et al. in 2006, looked at 7,843 premature children (821 of which had NEC) and their neurodevelopmental milestones over an average of 20 months. These results demonstrated that infants with a history of NEC were more likely to have some form of neurodevelopmental impairment (NDI). Specifically, the breakdown showed that 20% of the patients with NEC developed CP, 3% developed visual impairments, 3% hearing deficits, 36% cognitive deficits, and 35% psychomotor impairments. Interestingly, when the data was further stratified, those with medical NEC were not found to have significant neurodevelopmental impairment when compared to the cohort without NEC (prematurity alone), while those in the “surgical NEC group” had a more significant impairment, worse outcomes, and higher rates of CP and psychomotor impairment overall (12). Another review analyzed a database of 12,992 very low birth weight (VLBW) infants in Israel and looked at the association of several neonatal co-morbidities (including NEC) with the risk of head growth failure (HGF)—defined as head circumference z-score that was greater than two z-scores below the mean. Overall, the risk of severe HGF was associated with a nearly 3-fold greater odds with a diagnosis of NEC. These differences are even more disparate when surgery becomes necessary, and infants diagnosed with surgical NEC had an odds ratio of 7.62 associated with the development of severe HGF (23).
The pathogenesis of NEC progresses to requiring surgery for several reasons including, free intra-abdominal air and/or clinical deterioration despite optimal medical management—often translating to worse outcomes in infants with “surgical NEC” (24). The disparity between NDI in medical and surgical NEC is further illustrated by a multi-center retrospective review of 2,948 extremely low birth weight (ELBW) infants. At a corrected age of 18 to 22 months, infants with “surgical NEC” were found to have significantly reduced weight, length, and head circumference when compared to infants without NEC or with medical NEC. On Bayley Scales of Infant Development assessments, surgical NEC, but not medical NEC, was found to be an independent risk factor for lower scores on the mental developmental index (MDI), psychomotor developmental index (PDI), and resulted in an increased risk of neuro-developmental impairment (NDI) (25). This disparity in surgical vs. medical NEC is highlighted again by a study by Martin et al. looking at a cohort of 1,155 neonates for the development of surgical or medical NEC and accompanied prognostic factors. Those who had both surgical NEC and late bacteremia had worse NDI, with the group citing an increased risk of CP [OR = 8.4 (1.9, 39)] and microcephaly [OR = 9.3 (2.2, 40)]. Like the previous study, children with medical NEC with or without late bacteremia were not at increased risk of any developmental dysfunction (26).
It is also important to note that NDI seen in early childhood testing often persists in school-aged children. Rose et al. looked at neurodevelopmental outcomes of school-aged children with a history of surgical NEC or SIP (spontaneous intestinal perforation) and compared them with matched controls (27). Although this study combined outcomes from SIP and NEC, the data still showed that the combined cohort had more abnormal motor function scores (as assessed by Movement Assessment Battery for Children) and lower intelligence quotients (IQ)-(86 ± 14 compared with 97 ± 9 in the controls) (27), supporting the hypothesis that NDI persists past infancy.
2.3. Necrotizing enterocolitis (NEC) and spontaneous intestinal perforation (SIP)
Spontaneous intestinal perforation (SIP) is a discrete entity from NEC and is characterized by an isolated perforation in the gastrointestinal tract. The presentation of an infant with SIP and NEC with perforation can be similar, however, the significant systemic inflammatory response of NEC isn't seen in SIP patients with these infants faring better after resection (28). Although NEC and SIP are often grouped together, NEC has been shown to have more significant NDI as evidenced by a retrospective study on preterm infants that compared neurodevelopmental outcomes within a cohort of NEC and SIP patients (29). A battery of neurodevelopmental assessments showed more significant abnormal findings in NEC compared to SIP in gross and fine motor skills as well as cognitive deficits (29), suggesting that the inflammatory process of NEC plays a greater role in brain injury and development of NDI.
The severity of NEC and the need for surgery demonstrating worse NDI lends itself to the question if surgery itself contributes to the NDI seen. The Necrotizing Enterocolitis Surgery Trial (NEST) looked at 310 extremely low birth weight infants (EBLW) and evaluated the difference between initial laparotomy vs. drainage on the rates of death or NDI (data collected from 18 to 22 months) in NEC and SIP. NEST ultimately determined that initial laparotomy was more likely to reduce rates of death or NDI in infants with a preoperative diagnosis of NEC when compared to placing a Penrose drain (30). This data echoes an earlier observational study in 2006 that showed that NEC (when compared to SIP) had a higher-odds of death and NDI at 18–22 months of adjusted age (31). These data indicate that surgical intervention itself, is not the primary driver of NDI, as those with worsening clinical NEC did better with more aggressive surgical intervention(laparotomy) vs. leaving a drain in place.
The above studies demonstrate that surgical NEC has worse NDI outcomes than SIP, and it is the progression of NEC pathogenesis to requiring surgery that leads to worse NDI (not surgical intervention alone). A retrospective analysis of preterm infants by Bell et al. clarifies this issue further and looks at outcomes of patients with NEC and SIP with or without short bowel syndrome (SBS). The risk of development of moderate to severe NDI was 77% in the cohort of infants with NEC/SIP and SBS when compared to 62% of those with NEC/SIP without SBS (aRR 1.22) and 44% with no NEC, SIP, or SBS (aRR 1.6). In addition, children developing short bowel syndrome had lower cognitive, language, and motor scores on Bayley assessments than the cohort with NEC/SIP that didn't develop SBS (32). Although this study didn't differentiate between surgical NEC and SIP, it did highlight that the surgical resection of intestine—resulting in short-bowel syndrome (SBS)—is another contributing factor to the development of long-term NDI. In summary, the existing literature on NDI indicates that surgical NEC has high rates of NDI when compared to SIP, medical NEC, and prematurity alone. In addition, the development of SBS results in even higher NDI.
2.4. MRI changes in parenchyma correspond to NEC severity
The presence of increased parenchymal abnormalities in NEC patients as seen on magnetic resonance imaging (MRI) has been validated in several studies. MRIs performed on a prospective cohort of 192 premature infants at birth and repeated at 2 years old showed that infants with sepsis and/or NEC had a higher prevalence and severity of white matter abnormality, and specifically that infants with NEC had higher rates of concurrent gray matter abnormality. Unsurprisingly, infants with surgical NEC had more severe brain injury detected on MRI when compared with infants with medical NEC. When adjusted for other factors, this cohort was also found to have delayed cognitive and motor impairment (17) as demonstrated in the studies described earlier. In another study of 26 premature infants with NEC or SIP, infants with surgical NEC and SIP were found to have more significant brain injury seen on MRI, when compared with infants with medical NEC, even after adjustment for confounders (33). It is important to note that the patients with SIP were combined in the group with surgical NEC, so we are unable to extrapolate about the difference in significance between SIP and surgical NEC brain injury on MRI. More recently, another study looked at 69 infants with surgical NEC and found that 52% had some form of white matter brain injury as seen on MRI and were subsequently more likely to have a severe postoperative course. Those that survived with known white matter brain injury were found to have lower mean motor, cognitive, and language scores as well as higher rates of visual impairment at 2 years of age (34). These studies together show that NEC severity corresponds to parenchymal changes and especially white matter injury as seen on MRI. These observations along with others (17, 34) support the hypothesis that bowel injury initiates inflammation that potentially affects the developing brain (26).
3. The Gut-Brain Axis: an explanation for neurodevelopmental impairment
The gut-brain axis (GBA) is a bi-directional highway of communication involving neuro-immune-endocrine mediators that link the gut, the microbiome, and the nervous system—playing a critical role in the homeostatic processes of health and disease (9, 35). The alteration of the GBA has served as a framework for the explanation of many diseases for over three decades (36) and is now acknowledged as a crucial part of the development of the pathogenesis and downstream NDI in NEC (36, 37).
In the case of NEC, this begins as a combination of microbial dysbiosis and subsequent intestinal injury. This leads to signals traveling via the enteric nervous system (ENS) (6) residing within the intestinal wall, through the vagus nerve, and ultimately to the central nervous system (CNS) (6, 7). In addition to neural signaling, pathogenic bacteria release lipopolysaccharide (LPS) and a variety of other inflammatory mediators (such as fatty acids) into the systemic circulation. This initiates a cascade of inflammatory factors that causes systemic inflammation but also activates toll-like receptors on microglia. Activated microglia release pro-inflammatory cytokines, free-radicals, and help to activate other cells such as astrocytes as well as injure developing pre-oligodendrocytes. The combination of these insults results in white matter injury (7, 38). The interplay of this axis and its suspected role in NEC is further detailed in the following sections and is illustrated in Figure 1.
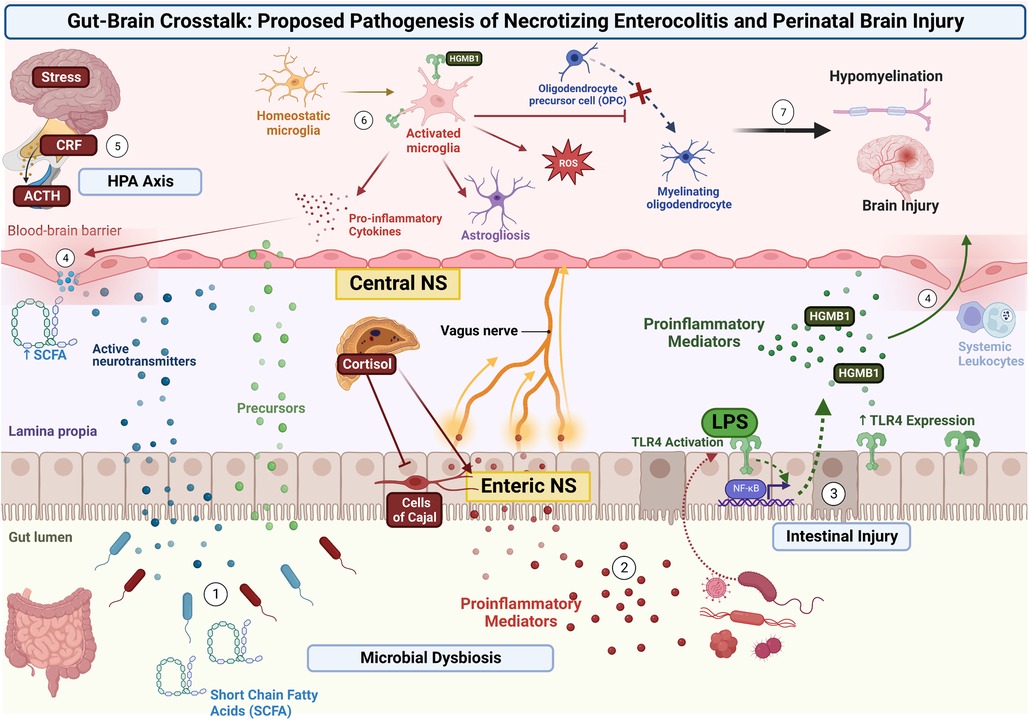
Figure 1. Proposed pathogenesis of NDI in NEC via the Gut-Brain Axis. Microbial dysbiosis and subsequent intestinal injury leads to the activation of several signaling pathways. (1) Pathogenic bacteria release signaling molecules including neurotransmitters, gasotransmitters, and short-chain fatty acids that cross the intestinal membrane and enter the systemic circulation. (2) Pathogenic bacteria release proinflammatory cytokines and other inflammatory mediators to stimulate the ENS within the intestinal wall, with signals traveling through the Vagus nerve, and ultimately to the CNS. (3) Intestinal injury and release of mediators (such as LPS) results in TLR-4 signaling and increased expression. This results in transcriptional changes via NF-κB and further release of inflammatory mediators into the systemic circulation. (4) Systemic and neural inflammatory mediators weaken the BBB and allow further passage of pro-inflammatory mediators and systemic leukocytes. (5) Concurrently, stress is processed in the limbic system of the brain, resulting in release of CRF from the hypothalamus, release of ACTH from the pituitary, and the release of cortisol from the adrenal gland. This signaling through the HPA axis and increase in systemic cortisol results in further activation of the ENS and intestinal epithelial cells, while inhibiting interstitial cells of Cajal (resulting in decreased gut motility). (6) Signals and inflammatory mediators reach the CNS and cause local brain injury as well as activate microglia via ligands (such as HMGB-1) binding to TLR4. Activated microglia release pro-inflammatory cytokines and free radicals, stimulate astrocytes, and injure developing pre-oligodendrocytes. (7) The combination of these insults results in brain injury and hypomyelination.
3.1. The role of the microbiome
The microbiome is a critical part of the GBA with microbiota influencing the CNS by interacting locally with intestinal cells and the ENS or directly via neuroendocrine and metabolic mediators (8, 9). The importance of the microbiome to the homeostasis of the GBA is best highlighted by the wealth of studies of germ-free animals which have shown a wide array of impairment or dysregulation in immune function, amino acid metabolism, hormone signaling, neurotransmission, and behavioral phenotypes when compared to their counterparts (9, 39–42).
Dysbiosis—or the change of the microbiome towards an unfavorable or pathogenic bacterial colonization—is a major contributing factor to the development of NEC (36, 43, 44). The infant microbiome is normally characterized by large amounts of Lactobacillus, Bifidobacterium, and Bacteroides in the first few weeks to one year of life which is aided by normal vaginal delivery and feeding with human breast milk (45). Although there are no causal species to the development of NEC, an overgrowth of gram-negative organisms, specifically in the Enterobacteriaceae family (46, 47) and loss of intestinal diversity can contribute to dysbiosis and the multifactorial etiology of NEC (44, 47). Additionally, several experimental models have shown the pivotal role of bacteria in the pathogenesis of NEC as germ-free animals are protected from developing NEC (46, 48).
This unfavorable change in the microbiome triggers an acute inflammatory response that leads to further disruption of the already immature intestinal barrier and is further exacerbated when pathogenic bacteria release their endotoxins and pro-inflammatory mediators or translocate across the intestinal mucosa (49). The microbiome also directly influences the brain microenvironment by the generation of neurotransmitters, short chain fatty acids (SCFAs), and cytokines as well as via direct activation of immune cells and communication with neural networks that traverse up to the CNS (35, 50, 51). Bacterial toxins such as Lipopolysaccharide (LPS) can reduce ENS activity and inhibit the function of interstitial Cells of Cajal—often referred to as the pacemaker of the intestine and important to gut motility—resulting in the ileus that is often seen in NEC (36, 52). The microbiome also is an important regulator of the hypothalamic-pituitary axis (HPA) and is important for the postnatal development of an appropriate HPA stress response in mice. Activation of this axis can result in elevated levels of systemic cortisol which can further cause intestinal injury and stimulation of the pro-inflammatory cascade (8, 42). The microbiome also plays a role in the regulation of important epithelial barriers. Changes in the microbiome can cause direct influences on the intestinal epithelium and tight junction barrier activity (8). Additionally, studies in germ-free mice have shown that a healthy microbiome is essential to the development and function of the blood brain barrier (BBB), with germ-free mice showing increased BBB permeability that persisted into adulthood. In these studies, restoration of BB integrity was seen by postnatal recolonization of the intestine with a probiotic (9, 53).
3.1.1. Toll-Like receptor signaling
Toll-Like Receptors (TLRs) are pathogen-associated molecular pattern recognition receptors that participate in signaling in response to infection or disease (36). TLR-4 signaling, specifically, plays a pivotal role in the GBA and the pathogenesis of NEC. It has been shown that TLR-4 activation is unregulated in preterm infants and that TLR-4 knockout animals do not develop NEC (48, 54). Lipopolysaccharide (LPS), an endotoxin produced by pathogenic bacteria, results in an excessive TLR-4 activation in intestinal cells that causes translocation of transcription factors such as nuclear factor kappa-β (NF-kB) leading to the transcription of various pro-inflammatory cytokines and other mediators (48, 55). These mediators cause intestinal inflammation, disrupt mucosal integrity, and enter the systemic circulation. From here, these mediators initiate a systemic inflammatory response and can travel through a weakened BBB to further initiate damage and activate the brain's immune response (48, 51, 54–56).
3.1.2. Short Chain Fatty Acids(SCFAs)/neurotransmitters/gasotransmitters
Gut bacteria also independently produce metabolites that participate in the GBA. Both commensal and pathogenic bacteria produce SCFAs, such as butyrate, propionate, and acetate, that play a role in maintaining the barrier function of intestinal epithelial cells. Butyrate specifically can serve as a fuel source for colonocytes and improve tight junction integrity (35, 57). Alteration in the balance of gut SCFA is implicated in the altered function of both the intestinal barrier and BBB as well as the maintenance of homeostasis in the CNS (9). These molecules are also known to stimulate the sympathetic nervous system and influence the memory and learning process (8). SCFAs can diffuse through epithelia to exert their effects, typically through the inhibition of histone deacetylase (9, 58). In mice, intraperitoneal injections of butyrate have been shown to enhance learning, memory, and sociable behaviors while simultaneously decreasing depressive-like behaviors (9, 59–61). Studies in animal models have also shown that SCFAs can induce vagus nerve activation (9) and enhance mucosal barrier protection (9, 62). Although many studies promote the benefits of SCFA, the alteration of the homeostasis of SCFA has been implicated in certain disease processes (9). Specifically, excessive production of SCFAs has been implicated in NEC. One possible explanation for this can be secondary to overproduction of SCFAs by bacteria and poor gut motility which can in turn cause local accumulation of SCFAs (56, 63).
In addition, bacteria can both directly release neurotransmitters (such as 5-HT and GABA) (8, 9), molecules that mimic local neurotransmitters (8), and can stimulate intestinal cells to release neurotransmitters, which traverse the intestinal epithelium. These molecules or their precursors can then pass through a weakened BBB and further influence the brain (64) and CNS physiology, resulting in possible brain injury and altered development (11, 65). Gasotransmitters are another important type of signaling molecule in the GBA. In NEC, an emerging gasotransmitter of interest is H2S. Commensal bacteria such as Lactobacilli can produce hydrogen sulfide which further modulates gut motility (66). In fact, H2S has been shown to have protective effects on intestinal injury in murine models of NEC (8, 9). Although the effect of H2S on NDI has not been illustrated, there have been studies on neuroprotective effects of H2S in secondary brain injury after a TBI (67). In rats, intraperitoneal injection of NaHS, a H2S salt, resulted in improvement in TBI-induced memory impairment (67, 68) and H2S decreased TBI induced lesion volume in brains (67, 69).
3.2. Neural communications and the Hypothalamic-Pituitary Axis (HPA)
There are several neural pathways that allow the peripheral components of the GBA to communicate with the brain (36). The ANS afferent pathway starts with signaling from the lumen which traverse through the enteric nervous system (ENS) and vagal nerve to reach the CNS (8). The efferent pathway (from the CNS back to the intestinal wall) (8) often serves an anti-inflammatory function. In healthy individuals, this pathway helps to balance out or “check” the responses secondary to pro-inflammatory cytokines such as TNF-α (tumor necrosis factor alpha), signaling molecules such as HMGB1 (high mobility group box1), and inflammasomes (multi protein cytoplasmic complex that triggers cascades to enhance secretion of proinflammatory cytokines)—thereby preventing unregulated pathogenic signaling (36).
The ENS is the first access point to the afferent pathway and resides within the intestinal wall (36)—receiving signals from microbiota, immune cells in the epithelium, and altered and injured intestinal epithelium (36). Enteric signals can then communicate through the vagus nerve, dorsal root, and nodose ganglia to the CNS (6, 35, 36). The vagus nerve serves as a major pathway between microbial mediators, the ENS, and the brain, which is well supported by animal models that show the absence of neurochemical and behavioral effects with the alteration of the microbiome in vagotomized animals when compared to controls (8).
The hypothalamic-pituitary axis(HPA) is a hormonal mediator in the GBA and works alongside other signaling pathways (36). The microbiome also is a regulator of the HPA and has shown to be important to for the postnatal development of an appropriate HPA stress response in mice (8, 42). If the gut is “stressed” or there is dysbiosis, the HPA processes this information up in the limbic system. This results in corticotropin-releasing factor (CRF) from the hypothalamus, adreno-corticoid hormone(ACTH) secretion from the pituitary (8, 9) and ultimately stimulates the adrenal gland to release cortisol (9). The activation of this system then allows neural-hormonal influence of immune and intestinal epithelial cells, interstitial cells of Cajal, and ENS neurons (8, 36). Stress and signaling from the brain can drastically affect the intestine by alteration of intestinal permeability (8) and immune cell activation (8).
3.3. The brain: immune cell signaling and the brain's effector cells
The brain itself, is a complex signaling system of regions that have their own sensory and motor functions and includes the cerebral cortex, the cerebellum, the limbic system, the HPA, and the brain stem (36). Injury to different parts of the brain, can have several downstream consequences to immune functioning, neurobehavioral disorders, and intestinal disease processes (36). For the purposes of this review, we are specifically interested in neural damage as signals travel up to the brain from injury in the intestine as in the case of NEC.
Once pathogenic signals have travelled through the vagus nerve and/or inflammatory mediators have reached the CNS, the brain becomes especially susceptible to injury. This process includes activation of microglia (via TLR4 stimulation) and subsequently astrocytes and glial cells within the brain (70). Activated microglia and astrocytes migrate to sites of injury and begin the neuroinflammatory cascade by releasing pro-inflammatory cytokines such as TNF-α, interleukin-1β (IL-1β), and interleukin-6 (IL-6) (70–73). The BBB (already weakened by SCFA release) is further destabilized by the release of cytokines and the inflammatory activation of enzymes such as matrix metallopeptidases (MMPs), which allows systemic leukocytes to enter and further exacerbate injury. This interplay is believed to cause abnormalities in normal myelination and white matter injury (70, 73). At the cellular level, white matter injury is defined by alterations in the developing oligodendrocytes and specifically the pre-myelinating oligodendrocyte cell which results in hypomyelination (38, 73). Understanding these cellular and molecular processes is important for identifying future targets for prevention of poor neurodevelopmental outcomes after NEC.
4. Animal studies of NEC and neurological impairment
Although the clinical and macroscopic neurological effects of NEC on infants are clear, the microscopic changes caused by NEC in the GBA remain to be elucidated. Several animal models of NEC have been studied that show early progress in this realm (13). In mice, Sampah et al. and Nino et al. showed the onset of NEC in the intestine leads to excessive TLR4 signaling and activation of an endogenous ligand HGMB1 (high mobility group box 1) which enters the systemic circulation and activates TLR4 receptors on microglia in the brain. The microglial activation and damage in the brain was confirmed by either an increase in Iba-1(a microglial marker) staining, increased radical oxygen species accumulation, or reduced myelin basic protein (51, 54). Nino et al. further demonstrated that mice exposed to NEC had severe deficits in spatial working memory and novel object recognition memory by the time they reached postnatal day 60 (54). In another murine model of NEC, Biouss et al., showed that pups with NEC had higher brain-to-body weight ratios, thinner cortices, and increased levels of apoptosis and endoplasmic reticulum stress compared to breast-fed controls. In addition, the brains of mice with NEC had an associated reduction in the number of neurons, oligodendrocytes, and neural progenitor cells in specific regions of the brain. Finally, levels of pro-inflammatory cytokines, the density of activated microglia, and the density of astrocytes were increased in the brain, and correlated with an increase in the levels of pro-inflammatory cytokines in the gut and intestinal histologic damage (74).
Other animal models of NEC looking into the GBA remain sparse. A pig model by Brunse et al. showed that preterm pigs undergoing experimental NEC had increased BBB permeability and CNS inflammation (increased IL-6 production), but showed no effect on cerebral myelination or microglia density by day 5 (75). A rat model of NEC showed that animals with NEC demonstrated slower times to reach certain developmental milestones, increased anxiety-like behavior, and decreased cognitive function when compared to breast fed pups. These clinical observations were associated with increased numbers of “activated microglia” and decreased myelin basic protein (76).
The studies taken together highlight a few key findings of the pathophysiology of NEC: 1) intestinal inflammation and injury translates to neural changes that are proposed to occur through TLR4 signaling in the intestine, 2) endogenous ligands released from intestinal TLR4 activation go on to activate TLR4 on microglia and 3) downstream neurologic changes occur including microglial activation, increased neuroinflammation, and decreased myelination which can lead to downstream neurodevelopmental deficits.
5. Therapies in perinatal brain injury
The literature centered on therapies to prevent NDI in infants with NEC is scarce, and to date, no strong, randomized clinical trial data exist. However, there are several studies looking into therapies to prevent or ameliorate perinatal brain injury (of which NEC is a known risk factor). The following sections will summarize what is known about therapies to target perinatal brain injury which can be potentially applied to the brain injury seen in NEC.
5.1. Stem cell therapy
Mesenchymal stem cells (MSCs) are among the most widely studied stem cells because they are multi-potent cells that are relatively easy to isolate and maintain in culture (77, 78). Furthermore, they have a lower tumorigenic potential and are immune privileged with minimal host immune activation upon administration (79, 80). They have been used in various pre-clinical studies (81–84) and have been shown to reduce inflammation (85, 86), exhibit antioxidant properties (87), enhance neovascularization (88), and improve functional recovery of injured tissues. They can migrate to damaged tissues or organs in response to inflammatory mediators where they act in the local environment via secretion of paracrine mediators and interaction with surrounding cells (79, 89). The application of stem cells for the treatment of NEC, is largely still limited to pre-clinical animal studies, and very little is known about the effect of stem cells on NDI (82, 90–94). However, there are some studies that have looked at the separate effects of MSCs on the neonatal diseases of the gut, such as NEC, as well as the effects of MSCs on certain types of perinatal brain injury, including: periventricular leukomalacia (PVL), hypoxic-ischemic encephalopathy (HIE), and neonatal stroke. The combination of these findings helps us to extrapolate the connection between the effect of MSCs on the GBA in the pathogenesis of NEC (79).
5.1.1. MSCs and necrotizing enterocolitis
Over the past decade, stem cells have been studied as a potential avenue of treatment, however the therapeutic benefit of MSCs in the intestinal pathogenesis of NEC has yet to be fully explored in the clinical setting (77, 82, 95, 96). In fact, only one clinical case report shows a benefit of stem cells used in a case of surgical NEC where umbilical-cord-derived-MSCs (UC-MSCs) were given intravenously. Following administration of UC-MSCs, mesenteric doppler imaging showed improved perfusion to prior compromised portions of intestine by post-operative day 4 (97).
There are several animal studies that showcase the benefits of MSCs to mitigate the intestinal pathogenesis of NEC. In rat models of NEC, intraperitoneal(IP) injections of MSCs have shown an improvement in clinical sickness and intestinal histology injury—characterized by restoration of villi-crypt morphology and epithelium along with restoration of populations of Paneth cells, SOX9 cells, and LGR5 stem cells that occupy this crypt niche (98, 99). An adult mouse-model of ischemia and reperfusion utilized several different MSC's including umbilical cord (UC-MSC), bone-marrow (BM-MSC), and adipocyte-derived (AD-MSCs) cells and similarly showed improved overall survival, intestinal perfusion, restoration of normal intestinal histology, and a decrease in pro-inflammatory chemokines (84, 100).
5.1.2. MSCs and perinatal brain injury
Researchers have identified various causes that result in perinatal brain injury including neuronal cell death, ischemia from placental or umbilical cord disruption, accumulation of free radical oxygen species, persistent inflammatory cascades, and defective myelination of neuronal cells largely from microglia-mediated damage of pre-oligodendrocytes (101, 102). No human data exists that looks specifically at the effects of MSCs in neurodevelopmental impairment in NEC, however there are a few animal studies and clinical and preclinical trials that show promise in the field of perinatal brain injury. Oppliger et al. showed that UC-MSCs improved myelination and decreased microgliosis and astrogliosis in a rat model of white matter brain injury (103). A systemic review of 18 murine studies on the effect of neural stem cells (NSCs) on perinatal brain injury showed significantly improved motor function and cognitive function (104) consistently throughout most of the studies. In a preterm sheep model of LPS-induced white matter injury, treatment with UC-MSCs reduced cell apoptosis/inflammation, promoted oligodendrocyte survival,and attenuated astrogliosis (105). Although the overall data behind MSCs in perinatal brain injury, and specifically from inflammatory causes (not ischemia/hemorrhage), is sparse, it shows promising results, indicating that continuing to investigate the benefits of MSCs on improving NDI in NEC would be beneficial. In the following sections, we will delve into specific neonatal brain pathologies and the studies that utilize stem cells to treat them.
5.1.2.1. MSCs in neonatal stroke
The pathogenesis of neonatal stroke involves an ischemia-reperfusion injury with disruption of arterial or major venous flow. Studies in a newborn rat model of neonatal stroke by Kim et al. showed that MSCs reduced brain infarct volume and enhanced astrogliosis and ultimately improved functional test scores (106). Another rat model study of neonatal stroke by van Velthoven et al. showed that intranasally delivered MSCs reduced loss of brain matter and ultimately improved motor function (79, 107).
5.1.2.2. MSCs in Hypoxic-Ischemic Encephalopathy (HIE)
HIE is a perinatal brain injury where insufficient blood flow and oxygen is delivered to brain tissue resulting in damage and disability such as CP. Currently, therapy for HIE centers around hypothermia which prevents secondary brain injury but offers no restorative function (108). There are a few preclinical and animal studies that demonstrate the benefit of MSCs in treating HIE. In a rat model of HIE, the combination of UC-MSCs and hypothermia resulted in a reduction of the previously injured brain region and improved sensorimotor function (109). In addition, MSC therapy showed improvement in the neuro-microenvironment with decreased pro-inflammatory mediators, decreased microgliosis and astrocytosis, and decreased permeability of the BBB (79, 109). Another rat model of HIE demonstrated that intranasally delivered MSCs reduced markers of neuroinflammation and restored neuronal cell numbers (110). In a mice model of HIE, a single MSC infusion treatment directly into the cerebrum resulted in inducible gene expression that promoted growth, proliferation, and survival of neural progenitor and glial cells (107).
Early clinical trials in preterm infants suffering from HIE indicate that autologous UC-MSCs delivered intravenously showed improved Bayley III Assessment scores by 1 year of age (111, 105). UC-MSCs therapy has also been used in older children with CP in which improved cognitive effects and gross motor function have been shown (112, 79, 105). Taken together, these studies show promise in the role of MSCs in neurogenesis and repair (79).
5.1.2.3. MSCs in Periventricular Leukomalacia (PVL)
PVL has a multifactorial etiology including HIE, trauma, immature brain development, and inflammatory changes (79) and is specifically characterized by a loss of pre-oligodendrocytes, loss of normal myelination potential, and diffuse gliosis (79, 113). In a neonatal rat model of PVL, rats receiving intracerebral injections of MSCs demonstrated increased anti-myelin immunoreactivity and glial cell migration and proliferation in injured areas indicating a neuroprotective and neuro-regenerative effect (114). Two different studies of a rat model of PVL illustrated that IP injections of UC-MSCs could replicate this improvement in brain injury with increased mature oligodendrocyte counts, decreased reactive astrocytes, and activated microglia (115) as well as a demonstrated reduction in IL-1B and reversed demyelination (measured by myelin basic protein staining. Interestingly, UC-MSCs pretreated with interferon-gamma resulted in even more significant effects, indicating that MSCs can be primed to deliver their protective effects (79, 116) These studies together show that MSCs delivered in the peritoneum can participate in the GBA to deliver neuro-regenerative effects in the setting of this disease.
5.2. Extracellular vesicles (exosomes)
There is a large body of research suggesting that stem cells can exert part of their regenerative effects through the release of extracellular vesicles (EV) or exosomes. EVs carry a wide range of bioactive cargo which includes nucleic acids, lipids, proteins, and a variety of intracellular mediators including cytokines. These can then fuse with other cells and incur transcriptional and translational modifications (77, 95) and facilitate intracellular communication (70). In a neonatal rat model of NEC, McCulloh et al. isolated EVs from four types of MSCs and injected them into the peritoneal cavity and found that EVs reduced the incidence of NEC in a dose-dependent manner and reduced histologic intestinal injury (83). No studies exist that specifically tie the use of MSCs and EVs in NEC and development of NDI, however, there are a few studies that look at the effects of EVs on perinatal brain injury.
A review of the therapeutic EV studies in experimental animal models of perinatal brain injury looked at 13 studies that administered EVs from MSCs via intravenous or intranasal administration in rats, mice, and sheep. The studies overall demonstrated an improvement in myelination and neuronal deficits following brain injury, decreased secretion of pro-inflammatory factors, and reduced microglia-mediated neuroinflammation (10). In rodent models of perinatal brain injury, long-term behavioral studies also demonstrated that EV treatment not only improved early neurological deficit scores, but improved long-term changes in motor coordination, spatial learning, and several types of memory testing (70). Thomi et al. looked at an in vitro model which showed that EV administration inhibited the production of pro-inflammatory cytokines by glial cells (including activated microglia) via interference of TLR4-signaling on microglial cells which prevented degradation of NFkB inhibitor and further downstream effects (10). In studies of HIE in preterm sheep, Ophelders et al. reported that intravenous administration of bone-marrow MSC-derived EVs to the fetus improved brain function (117). Collectively, these studies showed the benefits of EVs in improving neurodevelopmental outcomes, however, more studies with consistent cell lines and administration routes must be done to confirm these findings (70).
5.3. Nutritional supplementation
Probiotics are a group of supplements that have possible neuroprotective potential. They are an amalgam of micro-organisms that can help re-colonize the gut with commensal bacteria and improve gut barrier function. Many studies have shown a benefit in the risk of NEC, but little is known about the effects on NDI. Clinical studies by Alfalfa et al. and Akar et al. showed that probiotic supplementation in preterm and VLBW infants reduced the risk, incidence, severity, and all-cause mortality in NEC, however there was no clear effect of probiotics on neurodevelopmental outcomes (118, 119). In preclinical animal studies, probiotics have been shown ameliorate brain injury by releasing inhibitors of TNF-a and NF-κB (36, 100, 101), blocking the transport of damaging bio-molecules via the GBA (36), alteration of mRNA expression in certain regions of the brain, and reducing HPA axis-induced release of cortisol (8, 120). In murine models, probiotics have been shown to alter anxiety and depression-related behavior in mice (8, 120) and in water-avoidance stress models strengthen tight junctional barrier integrity in the intestinal epithelium, which in turn attenuated the response of the HPA and ANS resulting in decreased end cortisol level and prevention of changes in the hippocampus (8, 121). Wang et al. showed that the probiotic Lactobacillus reuteri in a rodent model protected against several deleterious developmental behaviors such as cognition and anxiety and additionally prevented the increase in activated microglia and decrease in myelin basic protein that was seen in NEC (76).
Several studies have shown that early probiotic administration can help attenuate the effects of antibiotics and early life stress (9, 122–126), and prevent subsequent deleterious effects via the GBA. Cowan et al. performed studies that looked at maternal separation and early life stress in a rodent model. Pups in this model showed fear relapse and fear memories that more closely mimicked adult behavior (123). Female pups were shown to exhibit earlier onset of puberty while male pups exhibited an even later onset. Pups exposed to probiotics showed resistance to fear relapse and fear memories and restored normal onset of puberty in both sexes (124, 125). This maternal separation model also showed that by postnatal day 20, rats had hypercorticosteronemia, increased intestinal permeability, and altered gut microbiota—effects which were prevented in rats that were treated with probiotics. By postnatal day 56, rats exposed to maternal stress no longer showed serum changes in cortisol and their microbiome had largely normalized to control rats. However, the rats showed hypersensitivity when exposed to restraint stress with a significant increase in cortisol level and fecal frequency compared to controls. This hypersensitivity was also not seen in animals treated with probiotics (123). When applied to the pathophysiology of NEC and the GBA, probiotics could be a useful adjunct to attenuate the effects of early activations of brain-related circuits with fear and stress. However further studies need to be employed to look specifically at the effects of NEC and downstream NDI.
Prebiotics are defined as any substrate that is utilized by the host microbiota to confer a health benefit (9, 127). A main category is dietary fiber, which includes oligosaccharides, that may provide benefits to the developing preterm brain. These indigestible food components naturally occur in breast milk (human milk oligosaccharides) and have been assigned antimicrobial, immunomodulatory, and anti-inflammatory functions (127). Prebiotics have a high relative safety profile and can help the homeostasis of the gut microbiome (128). Fresh human milk provides up to a 4% relative risk reduction in the incidence of NEC (9) and helps to colonize the gut with healthy commensal bacteria and delivers important enzymes, immunomodulatory agents, and prebiotic oligosaccharides (36). Human milk contains many protective factors including secretory IgA, lactoferrin, and various oligosaccharides including glycosaminoglycans (GAGs). These carbohydrates are highly abundant and usually are not absorbed, but instead, serve as prebiotics for commensal bacteria in the intestine. They have been shown to exhibit immuno-modulatory effects in various disease processes (36). A prominent GAG gaining clinical interest in the treatment of NEC is chondroitin sulfate (CS), which comprises over half of the normal GAG content in human milk (129, 130) and is nonexistent in most major infant formulas (131). The concentration of CS in human breast milk is higher in preterm mothers than in term mothers indicating some evolutionary importance for preterm infants (44, 131, 132). In addition, maternal health characteristics have been shown to modulate the levels and function of GAGs, indicating that some element of maternal transfer is important to the health of infants (47) that are important to immune function and the development of a healthy microbiome (36). This in turn can prevent the deleterious activation of the GBA that can result in brain injury and downstream NDI (36).
Dabydeen et al. studied the effects of a high-calorie (120% of normal) and protein diet during the first year of life. With the altered diet, infants had dramatic improvements in head growth, weight gain, and increased axonal diameters in their corticospinal tracts. Unfortunately, neurodevelopmental data were unable to be obtained as the trial was aborted due to obvious benefits in the cohort with the diet. However, the importance of nutritional supplementation as an adjunct in the treatment of NEC and the potential for reducing NDI is important to continue to investigate (133). Taken together these studies demonstrate that nutrition, prebiotics, and probiotics can be important adjuncts to the treatment of NEC and amelioration of downstream NDI.
6. Conclusion
The morbidity and mortality of NEC in infants and the downstream neurodevelopmental complications after survival is well elucidated. Studies show that of infants that survive neonatal NEC, up to 45% of children show neurodevelopmental impairment (12). The pathophysiology of NEC involves a complex signaling cascade of the Gut-Brain axis driven by dysbiosis and inflammatory signaling within the intestine. This triggers inflammatory mediators that enter the systemic circulation, participate in TLR-4 signaling, or directly communicate between neural networks involving the ENS and the vagus nerve. Together these result in downstream microglial activation, subsequent astrocytic hypertrophy/astrogliosis, and impaired functioning of pre-oligodendrocytes, which ultimately cause white matter injury and impaired myelination (5–7, 70). This cascade inhibits normal brain development and growth, which is seen as white matter abnormalities on MRI (17, 25, 34). It becomes imperative therefore to make strides in therapies to protect against brain injury and downstream NDI. Although there is no clear therapeutic intervention to improve or prevent NDI, there is some promising early research in the field of stem cells, extracellular vesicles, probiotic/prebiotic therapies, and aggressive nutrition. With the prevalence and emotional burden that NDI following NEC carries on our society, it becomes important to continue research in this field—with a specific focus on understanding gut-brain signaling and possible mechanistic targets of therapeutic and preventative interventions.
Author contributions
KM and TAM developed the concept of the manuscript, KM drafted the manuscript, and FMM, JL, WCS, JPB, and TAM provided critical revisions to the manuscript. All authors contributed to the article and approved the submitted version.
Funding
Funding for this project was received from (1) National Institute of Diabetes and Digestive and Kidney Diseases of the National Institutes of Health (R01HD105301), (2) American College of Surgeons Clowe's Memorial Research Fund, (3) Gerber Foundation, (4) Riley Children's Foundation, and (5) IU Department of Surgery.
Conflict of interest
TAM serves as a consultant for Noveome Biotherapeutics. As such, he receives consulting fees for his services. The material presented herein is not in conflict with that position.
The remaining authors declare that the research was conducted in the absence of any commercial or financial relationships that could be construed as a potential conflict of interest.
Publisher's note
All claims expressed in this article are solely those of the authors and do not necessarily represent those of their affiliated organizations, or those of the publisher, the editors and the reviewers. Any product that may be evaluated in this article, or claim that may be made by its manufacturer, is not guaranteed or endorsed by the publisher.
References
1. Jacob J, Kamitsuka M, Clark RH, Kelleher AS, Spitzer AR. Etiologies of NICU deaths. Pediatrics. (2015) 135:e59–65. doi: 10.1542/peds.2014-2967
2. Neu J, Walker WA. Necrotizing enterocolitis. N Engl J Med. (2011) 364:255–64. doi: 10.1056/NEJMra1005408
3. Rich BS, Dolgin SE. Necrotizing enterocolitis. Pediatr Rev. (2017) 38:552–9. doi: 10.1542/pir.2017-0002
4. Bazacliu C, Neu J. Necrotizing enterocolitis: long term complications. Curr Pediatr Rev. (2019) 15:115–24. doi: 10.2174/1573396315666190312093119
5. Cong X, Xu W, Romisher R, Poveda S, Forte S, Starkweather A, et al. Gut microbiome and infant health: brain-gut-microbiota axis and host genetic factors. Yale J Biol Med. (2016) 89:299–308. PMID: 27698614.27698614
6. Moschopoulos C, Kratimenos P, Koutroulis I, Shah BV, Mowes A, Bhandari V. The neurodevelopmental perspective of surgical necrotizing enterocolitis: the role of the gut-brain axis. Mediators Inflamm. (2018) 2018:7456857. doi: 10.1155/2018/7456857
7. Udit S, Gautron L. Molecular anatomy of the gut-brain axis revealed with transgenic technologies: implications in metabolic research. Front Neurosci. (2013) 7:134. doi: 10.3389/fnins.2013.00134
8. Carabotti M, Scirocco A, Maselli MA, Severi C. The gut-brain axis: interactions between enteric microbiota, central and enteric nervous systems. Ann Gastroenterol. (2015) 28:203–9. PMID: 25830558.25830558
9. Cryan JF, O'Riordan KJ, Cowan CSM, Sandhu KV, Bastiaanssen TFS, Boehme M, et al. The microbiota-gut-brain axis. Physiol Rev. (2019) 99:1877–2013. doi: 10.1152/physrev.00018.2018
10. Thomi G, Surbek D, Haesler V, Joerger-Messerli M, Schoeberlein A. Exosomes derived from umbilical cord mesenchymal stem cells reduce microglia-mediated neuroinflammation in perinatal brain injury. Stem Cell Res Ther. (2019) 10:105. doi: 10.1186/s13287-019-1207-z
11. Hickey M, Georgieff M, Ramel S. Neurodevelopmental outcomes following necrotizing enterocolitis. Semin Fetal Neonatal Med. (2018) 23:426–32. doi: 10.1016/j.siny.2018.08.005
12. Rees CM, Pierro A, Eaton S. Neurodevelopmental outcomes of neonates with medically and surgically treated necrotizing enterocolitis. Arch Dis Child Fetal Neonatal Ed. (2007) 92:F193–198. doi: 10.1136/adc.2006.099929
13. Patra A, Huang H, Bauer JA, Giannone PJ. Neurological consequences of systemic inflammation in the premature neonate. Neural Regen Res. (2017) 12:890–6. doi: 10.4103/1673-5374.208547
14. Berken JA, Chang J. Neurologic consequences of neonatal necrotizing enterocolitis. Dev Neurosci. (2022) 44:295–308. doi: 10.1159/000525378
15. Park HW, Yoon HK, Han SB, Lee BS, Sung IY, Kim KS, et al. Brain MRI measurements at a term-equivalent age and their relationship to neurodevelopmental outcomes. AJNR Am J Neuroradiol. (2014) 35:599–603. doi: 10.3174/ajnr.A3720
16. Cheong JL, Hunt RW, Anderson PJ, Howard K, Thompson DK, Wang HX, et al. Head growth in preterm infants: correlation with magnetic resonance imaging and neurodevelopmental outcome. Pediatrics. (2008) 121:e1534–1540. doi: 10.1542/peds.2007-2671
17. Shah DK, Doyle LW, Anderson PJ, Bear M, Daley AJ, Hunt RW, et al. Adverse neurodevelopment in preterm infants with postnatal sepsis or necrotizing enterocolitis is mediated by white matter abnormalities on magnetic resonance imaging at term. J Pediatr. (2008) 153:170–5, 175.e171. doi: 10.1016/j.jpeds.2008.02.033
18. Guerra NG, Williamson AA, Lucas-Molina B. Normal development: Infancy, childhood, and adolescence. In: IACAPAP e-textbook of child and adolescent mental health. Geneva: International Association for Child and Adolescent Psychiatry and Allied Professions 2012.: Rey JM (2012).
19. Assessment of Young Children The American Academy of Child and Adolescent Psychiatry. https://www.aacap.org/AACAP/Member_Resources/AACAP_Committees/Infant_and_Preschool_Committee/Assessment_of_Young_Children.aspx [11/15/2022, 2022].
20. Drotar D, Stancin T, Dworkin PH, Sices L, Wood S. Selecting developmental surveillance and screening tools. Pediatr in Rev. (2008) 29:e52–8. doi: 10.1542/pir.29.10.e52
21. ASQ-3 Paul H. Brookes Publishing Co. https://agesandstages.com/products-pricing/asq3/#silk-tabs-0-6
22. Rossman MJ, Hyman SL, Rorabaugh ML, Berlin LE, Allen MC, Modlin JF. The CAT/CLAMS assessment for early intervention services. Clin Pediatr (Phila). (1994) 33:404–9. doi: 10.1177/000992289403300705
23. Regev RH, Arnon S, Litmanovitz I, Bauer-Rusek S, Boyko V, Lerner-Geva L, et al. Association between neonatal morbidities and head growth from birth until discharge in very-low-birthweight infants born preterm: a population-based study. Dev Med Child Neurol. (2016) 58:1159–66. doi: 10.1111/dmcn.13153
24. Fredriksson F, Engstrand Lilja H. Survival rates for surgically treated necrotising enterocolitis have improved over the last four decades. Acta Paediatr. (2019) 108:1603–8. doi: 10.1111/apa.14770
25. Hintz SR, Kendrick DE, Stoll BJ, Vohr BR, Fanaroff AA, Donovan EF, et al. Neurodevelopmental and growth outcomes of extremely low birth weight infants after necrotizing enterocolitis. Pediatrics. (2005) 115:696–703. doi: 10.1542/peds.2004-0569
26. Martin CR, Dammann O, Allred EN, Patel S, O'Shea TM, Kuban KC, et al. Neurodevelopment of extremely preterm infants who had necrotizing enterocolitis with or without late bacteremia. J Pediatr. (2010) 157:751–6.e751. doi: 10.1016/j.jpeds.2010.05.042
27. Roze E, Ta BDP, van der Ree MH, Tanis JC, van Braeckel KNJA, Hulscher JBF, et al. Functional impairments at school age of children with necrotizing enterocolitis or spontaneous intestinal perforation. Pediatr Res. (2011) 70:619–25. doi: 10.1203/PDR.0b013e31823279b1
28. Tiwari C, Sandlas G, Jayaswal S, Shah H. Spontaneous intestinal perforation in neonates. J Neonatal Surg. (2015) 4:14–14. doi: 10.47338/jns.v4.167
29. Shin SH, Kim EK, Kim SH, Kim HY, Kim HS. Head growth and neurodevelopment of preterm infants with surgical necrotizing enterocolitis and spontaneous intestinal perforation. Children (Basel). (2021) 8(10), 833. doi: 10.3390/children8100833
30. Blakely ML, Tyson JE, Lally KP, Hintz SR, Eggleston B, Stevenson DK, et al. Initial laparotomy versus peritoneal drainage in extremely low birthweight infants with surgical necrotizing enterocolitis or isolated intestinal perforation: a multicenter randomized clinical trial. Ann Surg. (2021) 274:e370–80. doi: 10.1097/SLA.0000000000005099
31. Blakely ML, Tyson JE, Lally KP, McDonald S, Stoll BJ, Stevenson DK, et al. Laparotomy versus peritoneal drainage for necrotizing enterocolitis or isolated intestinal perforation in extremely low birth weight infants: outcomes through 18 months adjusted age. Pediatrics. (2006) 117:e680–687. doi: 10.1542/peds.2005-1273
32. Bell M, Cole CR, Hansen NI, Duncan AF, Hintz SR, Adams-Chapman I. Neurodevelopmental and growth outcomes of extremely preterm infants with short bowel syndrome. J Pediatr. (2021) 230:76–83.e75. doi: 10.1016/j.jpeds.2020.11.026
33. Merhar SL, Ramos Y, Meinzen-Derr J, Kline-Fath BM. Brain magnetic resonance imaging in infants with surgical necrotizing enterocolitis or spontaneous intestinal perforation versus medical necrotizing enterocolitis. J Pediatr. (2014) 164:410–2.e411. doi: 10.1016/j.jpeds.2013.09.055
34. Garg PM, Paschal JL, Zhang M, Pippins M, Matthews A, Adams K, et al. Brain injury in preterm infants with surgical necrotizing enterocolitis: clinical and bowel pathological correlates. Pediatr Res. (2022) 91:1182–95. doi: 10.1038/s41390-021-01614-3
35. Niemarkt HJ, De Meij TG, van Ganzewinkel CJ, de Boer NKH, Andriessen P, Hütten MC, et al. Necrotizing enterocolitis, gut Microbiota, and brain development: role of the brain-gut axis. Neonatology. (2019) 115:423–31. doi: 10.1159/000497420
36. Sherman MP, Zaghouani H, Niklas V. Gut microbiota, the immune system, and diet influence the neonatal gut-brain axis. Pediatr Res. (2015) 77:127–35. doi: 10.1038/pr.2014.161
37. Collins SM, Surette M, Bercik P. The interplay between the intestinal microbiota and the brain. Nat Rev Microbiol. (2012) 10:735–42. doi: 10.1038/nrmicro2876
38. Volpe JJ, Kinney HC, Jensen FE, Rosenberg PA. The developing oligodendrocyte: key cellular target in brain injury in the premature infant. Int J Dev Neurosci. (2011) 29:423–40. doi: 10.1016/j.ijdevneu.2011.02.012
39. Kawase T, Nagasawa M, Ikeda H, Yasuo S, Koga Y, Furuse M. Gut microbiota of mice putatively modifies amino acid metabolism in the host brain. Br J Nutr. (2017) 117:775–83. doi: 10.1017/S0007114517000678
40. Neufeld KM, Kang N, Bienenstock J, Foster JA. Reduced anxiety-like behavior and central neurochemical change in germ-free mice. Neurogastroenterol Motil. (2011) 23:255–64, e119. doi: 10.1111/j.1365-2982.2010.01620.x
41. Pan WH, Sommer F, Falk-Paulsen M, Ulas T, Best P, Fazio A, et al. Exposure to the gut microbiota drives distinct methylome and transcriptome changes in intestinal epithelial cells during postnatal development. Genome Med. (2018) 10:27. doi: 10.1186/s13073-018-0534-5
42. Sudo N, Chida Y, Aiba Y, Sonoda J, Oyama N, Yu XN, et al. Postnatal microbial colonization programs the hypothalamic-pituitary-adrenal system for stress response in mice. J Physiol. (2004) 558:263–75. doi: 10.1113/jphysiol.2004.063388
43. Mizrahi A, Barlow O, Berdon W, Blanc WA, Silverman WA. NECROTIZING ENTEROCOLITIS IN PREMATURE INFANTS. J Pediatr. (1965) 66:697–705. doi: 10.1016/S0022-3476(65)80003-8
44. Knowles TA, Hosfield BD, Pecoraro AR, Li H, Shelley WC, Markel TA. It's all in the milk: chondroitin sulfate as potential preventative therapy for necrotizing enterocolitis. Pediatr Res. (2021) 89:1373–9. doi: 10.1038/s41390-020-01125-7
45. Staude B, Oehmke F, Lauer T, Behnke J, Göpel W, Schloter M, et al. The microbiome and preterm birth: a change in paradigm with profound implications for pathophysiologic concepts and novel therapeutic strategies. Biomed Res Int. (2018) 2018:7218187. doi: 10.1155/2018/7218187
46. Morowitz MJ, Poroyko V, Caplan M, Alverdy J, Liu DC. Redefining the role of intestinal microbes in the pathogenesis of necrotizing enterocolitis. Pediatrics. (2010) 125:777–85. doi: 10.1542/peds.2009-3149
47. Bering SB. Human milk oligosaccharides to prevent gut dysfunction and necrotizing enterocolitis in preterm neonates. Nutrients. (2018) 10:1461. doi: 10.3390/nu10101461
48. Jilling T, Simon D, Lu J, Meng FJ, Li D, Schy R, et al. The roles of bacteria and TLR4 in rat and murine models of necrotizing enterocolitis. J Immunol. (2006) 177:3273–82. doi: 10.4049/jimmunol.177.5.3273
49. Shen L, Turner JR. Role of epithelial cells in initiation and propagation of intestinal inflammation. Eliminating the static: tight junction dynamics exposed. Am J Physiol-Gastrointest Liver Physiol. (2006) 290:G577–82. doi: 10.1152/ajpgi.00439.2005
50. Diaz Heijtz R. Fetal, neonatal, and infant microbiome: perturbations and subsequent effects on brain development and behavior. Semin Fetal Neonatal Med. (2016) 21:410–7. doi: 10.1016/j.siny.2016.04.012
51. Sampah MES, Hackam DJ. Prenatal immunity and influences on necrotizing enterocolitis and associated neonatal disorders. Front Immunol. (2021) 12:650709. doi: 10.3389/fimmu.2021.650709
52. Zuo DC, Choi S, Shahi PK, Kim MY, Park CG, Kim YD, et al. Inhibition of pacemaker activity in interstitial cells of cajal by LPS via NF-κB and MAP kinase. World J Gastroenterol. (2013) 19:1210–8. doi: 10.3748/wjg.v19.i8.1210
53. Braniste V, Al-Asmakh M, Kowal C, Anuar F, Abbaspour A, Tóth M, et al. The gut microbiota influences blood-brain barrier permeability in mice. Sci Transl Med. (2014) 6:263ra158. doi: 10.1126/scitranslmed.3009759
54. Niño DF, Zhou Q, Yamaguchi Y, Martin LY, Wang S, Fulton WB, et al. Cognitive impairments induced by necrotizing enterocolitis can be prevented by inhibiting microglial activation in mouse brain. Sci Transl Med. (2018) 10. doi: 10.1126/scitranslmed.aan0237
55. Hackam DJ, Sodhi CP, Good M. New insights into necrotizing enterocolitis: from laboratory observation to personalized prevention and treatment. J Pediatr Surg. (2019) 54:398–404. doi: 10.1016/j.jpedsurg.2018.06.012
56. Neu J, Pammi M. Pathogenesis of NEC: impact of an altered intestinal microbiome. Semin Perinatol. (2017) 41:29–35. doi: 10.1053/j.semperi.2016.09.015
57. Neu J, Pammi M. Necrotizing enterocolitis: the intestinal microbiome, metabolome and inflammatory mediators. Semin Fetal Neonatal Med. (2018) 23:400–5. doi: 10.1016/j.siny.2018.08.001
58. Cleophas MC, Crişan TO, Lemmers H, Toenhake-Dijkstra H, Fossati G, Jansen TL, et al. Suppression of monosodium urate crystal-induced cytokine production by butyrate is mediated by the inhibition of class I histone deacetylases. Ann Rheum Dis. (2016) 75:593–600. doi: 10.1136/annrheumdis-2014-206258
59. Bredy TW, Wu H, Crego C, Zellhoefer J, Sun YE, Barad M. Histone modifications around individual BDNF gene promoters in prefrontal cortex are associated with extinction of conditioned fear. Learn Mem. (2007) 14:268–76. doi: 10.1101/lm.500907
60. Kratsman N, Getselter D, Elliott E. Sodium butyrate attenuates social behavior deficits and modifies the transcription of inhibitory/excitatory genes in the frontal cortex of an autism model. Neuropharmacology. (2016) 102:136–45. doi: 10.1016/j.neuropharm.2015.11.003
61. Schroeder FA, Lin CL, Crusio WE, Akbarian S. Antidepressant-like effects of the histone deacetylase inhibitor, sodium butyrate, in the mouse. Biol Psychiatry. (2007) 62:55–64. doi: 10.1016/j.biopsych.2006.06.036
62. Barcelo A, Claustre J, Moro F, Chayvialle JA, Cuber JC, Plaisancié P. Mucin secretion is modulated by luminal factors in the isolated vascularly perfused rat colon. Gut. (2000) 46:218–24. doi: 10.1136/gut.46.2.218
63. Lin J. Too much short chain fatty acids cause neonatal necrotizing enterocolitis. Med Hypotheses. (2004) 62:291–3. doi: 10.1016/S0306-9877(03)00333-5
64. Ge X, Pan J, Liu Y, Wang H, Zhou W, Wang X. Intestinal crosstalk between microbiota and serotonin and its impact on gut motility. Curr Pharm Biotechnol. (2018) 19:190–5. doi: 10.2174/1389201019666180528094202
65. Heijtz R D, Wang S, Anuar F, Qian Y, Björkholm B, Samuelsson A, et al. Normal gut microbiota modulates brain development and behavior. Proc Natl Acad Sci U S A. (2011) 108:3047–52. doi: 10.1073/pnas.1010529108
66. Drucker NA, Jensen AR, Ferkowicz M, Markel TA. Hydrogen sulfide provides intestinal protection during a murine model of experimental necrotizing enterocolitis. J Pediatr Surg. (2018) 53:1692–8. doi: 10.1016/j.jpedsurg.2017.12.003
67. Panthi S, Manandhar S, Gautam K. Hydrogen sulfide, nitric oxide, and neurodegenerative disorders. Transl Neurodegener. (2018) 7:3. doi: 10.1186/s40035-018-0108-x
68. Karimi SA, Hosseinmardi N, Janahmadi M, Sayyah M, Hajisoltani R. The protective effect of hydrogen sulfide [H(2)S] on traumatic brain injury (TBI) induced memory deficits in rats. Brain Res Bull. (2017) 134:177–82. doi: 10.1016/j.brainresbull.2017.07.014
69. Zhang M, Shan H, Wang T, Liu W, Wang Y, Wang L, et al. Dynamic change of hydrogen sulfide after traumatic brain injury and its effect in mice. Neurochem Res. (2013) 38:714–25. doi: 10.1007/s11064-013-0969-4
70. Gamage T, Fraser M. The role of extracellular vesicles in the developing brain: current perspective and promising source of biomarkers and therapy for perinatal brain injury. Front Neurosci. (2021) 15:744840. doi: 10.3389/fnins.2021.744840
71. Bianco F, Pravettoni E, Colombo A, Schenk U, Möller T, Matteoli M, et al. Astrocyte-derived ATP induces vesicle shedding and IL-1 beta release from microglia. J Immunol. (2005) 174:7268–77. doi: 10.4049/jimmunol.174.11.7268
72. Takenouchi T, Tsukimoto M, Iwamaru Y, Sugama S, Sekiyama K, Sato M, et al. Extracellular ATP induces unconventional release of glyceraldehyde-3-phosphate dehydrogenase from microglial cells. Immunol Lett. (2015) 167:116–24. doi: 10.1016/j.imlet.2015.08.002
73. Lombardi M, Parolisi R, Scaroni F, Bonfanti E, Gualerzi A, Gabrielli M, et al. Detrimental and protective action of microglial extracellular vesicles on myelin lesions: astrocyte involvement in remyelination failure. Acta Neuropathol. (2019) 138:987–1012. doi: 10.1007/s00401-019-02049-1
74. Biouss G, Antounians L, Li B, O'Connell JS, Seo S, Catania VD, et al. Experimental necrotizing enterocolitis induces neuroinflammation in the neonatal brain. J Neuroinflammation. (2019) 16:97. doi: 10.1186/s12974-019-1481-9
75. Brunse A, Abbaspour A, Sangild PT. Brain barrier disruption and region-specific neuronal degeneration during necrotizing enterocolitis in preterm pigs. Dev Neurosci. (2018) 40:198–208. doi: 10.1159/000488979
76. Wang Y, Jaggers RM, Mar P, Galley JD, Shaffer T, Rajab A, et al. Lactobacillus reuteri in its biofilm state promotes neurodevelopment after experimental necrotizing enterocolitis in rats. Brain Behav Immun Health. (2021) 14. doi: 10.1016/j.bbih.2021.100256
77. Drucker NA, McCulloh CJ, Li B, Pierro A, Besner GE, Markel TA. Stem cell therapy in necrotizing enterocolitis: current state and future directions. Semin Pediatr Surg. (2018) 27:57–64. doi: 10.1053/j.sempedsurg.2017.11.011
78. Shang Y, Guan H, Zhou F. Biological characteristics of umbilical cord mesenchymal stem cells and its therapeutic potential for hematological disorders. Front Cell Dev Biol. (2021) 9:570179. doi: 10.3389/fcell.2021.570179
79. Liau LL, Al-Masawa ME, Koh B, Looi QH, Foo JB, Lee SH, et al. The potential of mesenchymal stromal cell as therapy in neonatal diseases. Front Pediatr. (2020) 8:591693. doi: 10.3389/fped.2020.591693
80. Pittenger MF, Discher DE, Peault BM, Phinney DG, Hare JM, Caplan AI. Mesenchymal stem cell perspective: cell biology to clinical progress. NPJ Regen Med. (2019) 4:22. doi: 10.1038/s41536-019-0083-6
81. Drucker NA, Te Winkel JP, Shelley WC, Olson KR, Markel TA. Inhibiting hydrogen sulfide production in umbilical stem cells reduces their protective effects during experimental necrotizing enterocolitis. J Pediatr Surg. (2019) 54:1168–73. doi: 10.1016/j.jpedsurg.2019.02.037
82. McCulloh CJ, Olson JK, Zhou Y, Wang Y, Besner GE. Stem cells and necrotizing enterocolitis: a direct comparison of the efficacy of multiple types of stem cells. J Pediatr Surg. (2017) 52:999–1005. doi: 10.1016/j.jpedsurg.2017.03.028
83. McCulloh CJ, Olson JK, Wang Y, Zhou Y, Tengberg NH, Deshpande S, et al. Treatment of experimental necrotizing enterocolitis with stem cell-derived exosomes. J Pediatr Surg. (2018) 53:1215–20. doi: 10.1016/j.jpedsurg.2018.02.086
84. Jensen AR, Manning MM, Khaneki S, Drucker NA, Markel TA. Harvest tissue source does not alter the protective power of stromal cell therapy after intestinal ischemia and reperfusion injury. J Surg Res. (2016) 204:361–70. doi: 10.1016/j.jss.2016.05.006
85. Wang M, Zhang W, Crisostomo P, Markel T, Meldrum KK, Fu XY, et al. STAT3 Mediates bone marrow mesenchymal stem cell VEGF production. J Mol Cell Cardiol. (2007) 42:1009–15. doi: 10.1016/j.yjmcc.2007.04.010
86. Cheng Z, Zhu W, Cao K, Wu F, Li J, Wang G, et al. Anti-Inflammatory mechanism of neural stem cell transplantation in spinal cord injury. Int J Mol Sci. (2016) 17:1380. doi: 10.3390/ijms17091380
87. Dernbach E, Urbich C, Brandes RP, Hofmann WK, Zeiher AM, Dimmeler S. Antioxidative stress-associated genes in circulating progenitor cells: evidence for enhanced resistance against oxidative stress. Blood. (2004) 104:3591–7. doi: 10.1182/blood-2003-12-4103
88. Planat-Benard V, Silvestre JS, Cousin B, André M, Nibbelink M, Tamarat R, et al. Plasticity of human adipose lineage cells toward endothelial cells: physiological and therapeutic perspectives. Circulation. (2004) 109:656–63. doi: 10.1161/01.CIR.0000114522.38265.61
89. Song N, Scholtemeijer M, Shah K. Mesenchymal stem cell immunomodulation: mechanisms and therapeutic potential. Trends Pharmacol Sci. (2020) 41:653–64. doi: 10.1016/j.tips.2020.06.009
90. Ares GJ, McElroy SJ, Hunter CJ. The science and necessity of using animal models in the study of necrotizing enterocolitis. Semin Pediatr Surg. (2018) 27:29–33. doi: 10.1053/j.sempedsurg.2017.11.006
91. Sangild PT, Siggers RH, Schmidt M, Elnif J, Bjornvad CR, Thymann T, et al. Diet- and colonization-dependent intestinal dysfunction predisposes to necrotizing enterocolitis in preterm pigs. Gastroenterology. (2006) 130:1776–92. doi: 10.1053/j.gastro.2006.02.026
92. Waligora-Dupriet AJ, Dugay A, Auzeil N, Huerre M, Butel MJ. Evidence for clostridial implication in necrotizing enterocolitis through bacterial fermentation in a gnotobiotic quail model. Pediatr Res. (2005) 58:629–35. doi: 10.1203/01.PDR.0000180538.13142.84
93. Namachivayam K, Blanco CL, MohanKumar K, Jagadeeswaran R, Vasquez M, McGill-Vargas L, et al. Smad7 inhibits autocrine expression of TGF-β2 in intestinal epithelial cells in baboon necrotizing enterocolitis. Am J Physiol Gastrointest Liver Physiol. (2013) 304:G167–180. doi: 10.1152/ajpgi.00141.2012
94. Barlow B, Santulli TV, Heird WC, Pitt J, Blanc WA, Schullinger JN. An experimental study of acute neonatal enterocolitis–the importance of breast milk. J Pediatr Surg. (1974) 9:587–95. doi: 10.1016/0022-3468(74)90093-1
95. Nitkin CR, Rajasingh J, Pisano C, Besner GE, Thebaud B, Sampath V. Stem cell therapy for preventing neonatal diseases in the 21st century: current understanding and challenges. Pediatr Res. (2020) 87:265–76. doi: 10.1038/s41390-019-0425-5
96. Villamor-Martinez E, Hundscheid T, Kramer BW, Hooijmans CR, Villamor E. Stem cells as therapy for necrotizing enterocolitis: a systematic review and meta-analysis of preclinical studies. Front Pediatr. (2020) 8:578984. doi: 10.3389/fped.2020.578984
97. Akduman H, Dilli D, Ergun E, Cakmakci E, Celebi SK, Citli R, et al. Successful mesenchymal stem cell application in supraventricular tachycardia-related necrotizing enterocolitis: a case report. Fetal Pediatr Pathol. (2021) 40:250–5. doi: 10.1080/15513815.2019.1693672
98. Tayman C, Uckan D, Kilic E, Ulus AT, Tonbul A, Murat Hirfanoglu I, et al. Mesenchymal stem cell therapy in necrotizing enterocolitis: a rat study. Pediatr Res. (2011) 70:489–94. doi: 10.1203/PDR.0b013e31822d7ef2
99. Weis VG, Deal AC, Mekkey G, Clouse C, Gaffley M, Whitaker E, et al. Human placental-derived stem cell therapy ameliorates experimental necrotizing enterocolitis. Am J Physiol Gastrointest Liver Physiol. (2021) 320:G658–74. doi: 10.1152/ajpgi.00369.2020
100. Jensen AR, Doster DL, Hunsberger EB, Manning MM, Stokes SM, Barwinska D, et al. Human adipose stromal cells increase survival and mesenteric perfusion following intestinal ischemia and reperfusion injury. Shock. (2016) 46:75–82. doi: 10.1097/SHK.0000000000000571
101. Mitsialis SA, Kourembanas S. Stem cell-based therapies for the newborn lung and brain: possibilities and challenges. Semin Perinatol. (2016) 40:138–51. doi: 10.1053/j.semperi.2015.12.002
102. Mueller M, Wolfs TG, Schoeberlein A, Gavilanes AW, Surbek D, Kramer BW. Mesenchymal stem/stromal cells-a key mediator for regeneration after perinatal morbidity? Mol Cell Pediatr. (2016) 3:6. doi: 10.1186/s40348-016-0034-x
103. Intranasal delivery of umbilical cord-derived mesenchymal stem cells preserves myelination in perinatal brain damage. Stem Cells Dev. (2016) 25:1234–42. doi: 10.1089/scd.2016.0027
104. Smith MJ, Paton MCB, Fahey MC, Jenkin G, Miller SL, Finch-Edmondson M, et al. Neural stem cell treatment for perinatal brain injury: a systematic review and meta-analysis of preclinical studies. Stem Cells Transl Med. (2021) 10:1621–36. doi: 10.1002/sctm.21-0243
105. Peng X, Song J, Li B, Zhu C, Wang X. Umbilical cord blood stem cell therapy in premature brain injury: opportunities and challenges. J Neurosci Res. (2020) 98:815–25. doi: 10.1002/jnr.24548
106. Kim ES, Ahn SY, Im GH, Sung DK, Park YR, Choi SH, et al. Human umbilical cord blood-derived mesenchymal stem cell transplantation attenuates severe brain injury by permanent middle cerebral artery occlusion in newborn rats. Pediatr Res. (2012) 72:277–84. doi: 10.1038/pr.2012.71
107. van Velthoven CT, Kavelaars A, van Bel F, Heijnen CJ. Mesenchymal stem cell transplantation changes the gene expression profile of the neonatal ischemic brain. Brain Behav Immun. (2011) 25:1342–8. doi: 10.1016/j.bbi.2011.03.021
108. Allen KA, Brandon DH. Hypoxic ischemic encephalopathy: pathophysiology and experimental treatments. Newborn Infant Nurs Rev. (2011) 11:125–33. doi: 10.1053/j.nainr.2011.07.004
109. Park WS, Sung SI, Ahn SY, Yoo HS, Sung DK, Im GH, et al. Hypothermia augments neuroprotective activity of mesenchymal stem cells for neonatal hypoxic-ischemic encephalopathy. PLoS One. (2015) 10:e0120893. doi: 10.1371/journal.pone.0120893
110. McDonald CA, Djuliannisaa Z, Petraki M, Paton MCB, Penny TR, Sutherland AE, et al. Intranasal delivery of mesenchymal stromal cells protects against neonatal hypoxic—ischemic brain injury. Int J Mol Sci. (2019) 20:2449. doi: 10.3390/ijms20102449
111. Cotten CM, Murtha AP, Goldberg RN, Grotegut CA, Smith PB, Goldstein RF, et al. Feasibility of autologous cord blood cells for infants with hypoxic-ischemic encephalopathy. J Pediatr. (2014) 164:973–9.e971. doi: 10.1016/j.jpeds.2013.11.036
112. Kang M, Min K, Jang J, Kim SC, Kang MS, Jang SJ, et al. Involvement of immune responses in the efficacy of cord blood cell therapy for cerebral palsy. Stem Cells Dev. (2015) 24:2259–68. doi: 10.1089/scd.2015.0074
113. Wang Y, Long W, Cao Y, Li J, You L, Fan Y. Mesenchymal stem cell-derived secretomes for therapeutic potential of premature infant diseases. Biosci Rep. (2020) 40. doi: 10.1042/BSR20200241
114. Chen A, Siow B, Blamire AM, Lako M, Clowry GJ. Transplantation of magnetically labeled mesenchymal stem cells in a model of perinatal brain injury. Stem Cell Res. (2010) 5:255–66. doi: 10.1016/j.scr.2010.08.004
115. Zhu LH, Bai X, Zhang N, Wang SY, Li W, Jiang L. Improvement of human umbilical cord mesenchymal stem cell transplantation on glial cell and behavioral function in a neonatal model of periventricular white matter damage. Brain Res. (2014) 1563:13–21. doi: 10.1016/j.brainres.2014.03.030
116. Morioka C, Komaki M, Taki A, Honda I, Yokoyama N, Iwasaki K, et al. Neuroprotective effects of human umbilical cord-derived mesenchymal stem cells on periventricular leukomalacia-like brain injury in neonatal rats. Inflamm Regen. (2017) 37:1. doi: 10.1186/s41232-016-0032-3
117. Ophelders DR, Wolfs TG, Jellema RK, Zwanenburg A, Andriessen P, Delhaas T, et al. Mesenchymal stromal cell-derived extracellular vesicles protect the fetal brain after hypoxia-ischemia. Stem Cells Transl Med. (2016) 5:754–63. doi: 10.5966/sctm.2015-0197
118. Alfaleh K, Anabrees J, Bassler D, Al-Kharfi T. Probiotics for prevention of necrotizing enterocolitis in preterm infants. Cochrane Database Syst Rev. (2011) 4:Cd005496. doi: 10.1002/14651858.CD005496.pub5
119. Akar M, Eras Z, Oncel MY, Arayici S, Guzoglu N, Canpolat FE, et al. Impact of oral probiotics on neurodevelopmental outcomes in preterm infants. J Matern Fetal Neonatal Med. (2017) 30:411–5. doi: 10.1080/14767058.2016.1174683
120. Bravo JA, Forsythe P, Chew MV, Escaravage E, Savignac HM, Dinan TG, et al. Ingestion of Lactobacillus strain regulates emotional behavior and central GABA receptor expression in a mouse via the vagus nerve. Proc Natl Acad Sci U S A. (2011) 108:16050–5. doi: 10.1073/pnas.1102999108
121. Ait-Belgnaoui A, Colom A, Braniste V, Ramalho L, Marrot A, Cartier C, et al. Probiotic gut effect prevents the chronic psychological stress-induced brain activity abnormality in mice. Neurogastroenterol Motil. (2014) 26:510–20. doi: 10.1111/nmo.12295
122. Callaghan BL, Cowan CS, Richardson R. Treating generational stress: effect of paternal stress on development of memory and extinction in offspring is reversed by probiotic treatment. Psychol Sci. (2016) 27:1171–80. doi: 10.1177/0956797616653103
123. Cowan CS, Callaghan BL, Richardson R. The effects of a probiotic formulation (Lactobacillus rhamnosus and L. helveticus) on developmental trajectories of emotional learning in stressed infant rats. Transl Psychiatry. (2016) 6:e823. doi: 10.1038/tp.2016.94
124. Cowan CSM, Richardson R. Early-life stress leads to sex-dependent changes in pubertal timing in rats that are reversed by a probiotic formulation. Dev Psychobiol. (2019) 61:679–87. doi: 10.1002/dev.21765
125. Cowan CSM, Stylianakis AA, Richardson R. Early-life stress, microbiota, and brain development: probiotics reverse the effects of maternal separation on neural circuits underpinning fear expression and extinction in infant rats. Dev Cogn Neurosci. (2019) 37:100627. doi: 10.1016/j.dcn.2019.100627
126. Fukui H, Oshima T, Tanaka Y, Oikawa Y, Makizaki Y, Ohno H, et al. Effect of probiotic Bifidobacterium bifidum G9-1 on the relationship between gut microbiota profile and stress sensitivity in maternally separated rats. Sci Rep. (2018) 8:12384. doi: 10.1038/s41598-018-30943-3
127. Gibson GR, Hutkins R, Sanders ME, Prescott SL, Reimer RA, Salminen SJ, et al. Expert consensus document: the international scientific association for probiotics and prebiotics (ISAPP) consensus statement on the definition and scope of prebiotics. Nat Rev Gastroenterol Hepatol. (2017) 14:491–502. doi: 10.1038/nrgastro.2017.75
128. Keunen K, van Elburg RM, van Bel F, Benders MJ. Impact of nutrition on brain development and its neuroprotective implications following preterm birth. Pediatr Res. (2015) 77:148–55. doi: 10.1038/pr.2014.171
129. Meinzen-Derr J, Poindexter B, Wrage L, Morrow AL, Stoll B, Donovan EF. Role of human milk in extremely low birth weight infants’ risk of necrotizing enterocolitis or death. J Perinatol. (2009) 29:57–62. doi: 10.1038/jp.2008.117
130. Altobelli E, Angeletti PM, Verrotti A, Petrocelli R. The impact of human milk on necrotizing enterocolitis: a systematic review and meta-analysis. Nutrients. (2020) 12:1322. doi: 10.3390/nu12051322
131. Deckelbaum RJ, Adair L, Appelbaum M, Baker GL, Baker SS, Berlin CM, et al. Institute of medicine committee on the evaluation of the addition of ingredients new to infant F. In: Carroll S, editor. Infant formula: evaluating the safety of new ingredients. Washington (DC): National Academies Press (US). Copyright 2004 by the National Academy of Sciences. All rights reserved, 2004.
132. Underwood MA. Human milk for the premature infant. Pediatr Clin North Am. (2013) 60:189–207. doi: 10.1016/j.pcl.2012.09.008
Keywords: gut-brain axis, necrotizing enterocolitis, perinatal brain injury, microbiome, neonatal brain, neurodevelopmental impairment
Citation: Manohar K, Mesfin FM, Liu J, Shelley WC, Brokaw JP and Markel TA (2023) Gut-Brain cross talk: The pathogenesis of neurodevelopmental impairment in necrotizing enterocolitis. Front. Pediatr. 11:1104682. doi: 10.3389/fped.2023.1104682
Received: 21 November 2022; Accepted: 23 January 2023;
Published: 15 February 2023.
Edited by:
Minesh Khashu, University Hospitals Dorset NHS Foundation Trust, United KingdomReviewed by:
Yuying Liu, University of Texas Health Science Center at Houston, United StatesGail Besner, Nationwide Children's Hospital, United States
© 2023 Manohar, Mesfin, Liu, Shelley, Brokaw and Markel. This is an open-access article distributed under the terms of the Creative Commons Attribution License (CC BY). The use, distribution or reproduction in other forums is permitted, provided the original author(s) and the copyright owner(s) are credited and that the original publication in this journal is cited, in accordance with accepted academic practice. No use, distribution or reproduction is permitted which does not comply with these terms.
*Correspondence: Troy A. Markel dG1hcmtlbEBpdXB1aS5lZHU=
Specialty Section: This article was submitted to Neonatology, a section of the journal Frontiers in Pediatrics