- 1Department of Clinical Pharmacy, College of Pharmacy, King Saud University, Riyadh, Saudi Arabia
- 2King Khalid Hospital, Najran, Saudi Arabia
- 3Prince Sultan Military Medical City, Riyadh, Saudi Arabia
- 4Pharmaceutical Care Division, King Faisal Specialist Hospital and Research Center, Jeddah, Saudi Arabia
- 5Pharmacy Department, King Abdulaziz Medical City, Riyadh, Saudi Arabia
- 6Pharmaceutical Care Division, King Faisal Specialist Hospital and Research Center, Riyadh, Saudi Arabia
- 7Pharmaceutical Care Administration, Armed Forces Hospital Southern Region, Khamis Mushait, Saudi Arabia
- 8King Saud bin Abdulaziz University for Health Sciences, Riyadh, Saudi Arabia
- 9King Abdullah International Medical Research Center, Riyadh, Saudi Arabia
- 10Department of Clinical Pharmacy, Faculty of Pharmacy, Misr International University, Cairo, Egypt
Introduction: Vancomycin dosing in very low birth weight (VLBW) neonates is challenging. Compared with the general neonatal population, VLBW neonates are less likely to achieve the vancomycin therapeutic targets. Current dosing recommendations are based on studies of the general neonatal population, as only a very limited number of studies have evaluated vancomycin pharmacokinetics in VLBW neonates. The main aim of this study was to develop a vancomycin population pharmacokinetic model to optimize vancomycin dosing in VLBW neonates.
Methods: This multicenter study was conducted at six major hospitals in Saudi Arabia. The study included VLBW neonates who received vancomycin and had at least one vancomycin serum trough concentration measurement at a steady state. We developed a pharmacokinetic model and performed Monte Carlo simulations to develop an optimized dosing regimen for VLBW infants. We evaluated two different targets: AUC0–24 of 400–600 or 400–800 µg. h/mL. We also estimated the probability of trough concentrations >15 and 20 µg/mL.
Results: In total, we included 236 neonates, 162 in the training dataset, and 74 in the validation dataset. A one-compartment model was used, and the distribution volume was significantly associated only with weight, whereas clearance was significantly associated with weight, postmenstrual age (PMA), and serum creatinine (Scr).
Discussion: We developed dosing regimens for VLBW neonates, considering the probability of achieving vancomycin therapeutic targets, as well as different toxicity thresholds. The dosing regimens were classified according to PMA and Scr. These dosing regimens can be used to optimize the initial dose of vancomycin in VLBW neonates.
Introduction
Late-onset neonatal sepsis is a major cause of morbidity and mortality in newborns worldwide, with a mortality rate of approximately 15% in very low birth weight (VLBW) neonates (birth weight <1,500 g) (1–3). Vancomycin is a glycopeptide antibiotic frequently used to treat nosocomial gram-positive infections in neonates when methicillin-resistant Staphylococcus aureus (MRSA) or coagulase-negative staphylococci (MRCONS) are suspected. Vancomycin is considered one of the most common antimicrobial agents used in neonatal intensive care units (NICU) because of increased mortality and morbidity rates due to neonatal sepsis, compared with sepsis in children (4, 5). The optimal vancomycin dose should target an area under curve/minimum inhibitory concentration (AUC0−24/MIC) of 400 µg.h/ml, while minimizing higher AUCs0−24/MIC (>800 µg.h/ml) and troughs (>20 µg/ml) (6–10). The new IDSA treatment guidelines recommend targeting an AUC0−24 of 400–600 µg.hr/ml (assuming an MIC of 1 µg/ml) and no longer recommends trough guided monitoring. Although vancomycin has been used in clinical practice for more than 50 years, dosing in neonates remains challenging for many reasons. Most notably, these include a lack of consensus on the optimal dosing and a narrow therapeutic index (5, 10, 11–14). In addition, the rapidly changing pharmacokinetics in neonates are mainly related to changes in body water and organ maturation. Previous studies have reported that the major predictors of vancomycin pharmacokinetics in neonates include body weight, maturation [as indicated by postmenstrual age (PMA), gestational age (GA), and postnatal age (PNA)], and serum creatinine (Scr) (11, 12, 15).
Optimizing vancomycin dosing is even more challenging in VLBW neonates than in the general neonatal population and older pediatric patients. VLBW neonates exhibit limited renal elimination and higher extracellular fluid volume compared to normal weight neonates (16, 17). In our previous study, we found that VLBW neonates were less likely to achieve a vancomycin therapeutic target of an AUC0−24/MIC > 400 µg.h/ml compared to neonates born with a larger body weight (18). Similar findings were observed in the study by Stone et al. (19). This indicates that current dosing recommendations are not optimal for the VLBW neonates and there is a need for specific dosing regimens in this patient population. Most published pharmacokinetic models are in the general neonatal population. To date, only two studies evaluating vancomycin pharmacokinetics and dosing in VLBW neonates have been published (16, 17). Both of these studies included a very small sample size (<20 patients). Therefore, pharmacokinetic models must be further explored in this unique population.
Nevertheless, early optimization of vancomycin dosing to rapidly achieve adequate antibiotic exposure is extremely important. This will increase efficacy, minimize toxicity, and avoid bacterial resistance, particularly when treating invasive MRSA infections. Therefore, the main aim of this study was to develop a vancomycin population pharmacokinetic model to optimize dosing in VLBW neonates.
Methods
Patients and data collection
This multicenter observational study was conducted in six major hospitals in Saudi Arabia. Data were retrospectively collected from the electronic medical records of the following hospitals: King Saud University Medical City in Riyadh, King Faisal Specialist Hospital in Riyadh, National Guard Hospital in Riyadh, King Faisal Specialist Hospital in Jeddah, Prince Sultan Military Medical City in Riyadh, and Armed Forces Hospital Southern Region in Abha. Data were retrieved for VLBW neonates (0–30 days old) who were admitted to the NICU and received vancomycin for proven or suspected MRSA or MRCONS infections. Patients were included if they received vancomycin for more than 48 h and had at least one vancomycin serum trough concentration measurement in a steady state. The collected data included PNA, PMA, weight, Scr, vancomycin dose, frequency and concentrations. For vancomycin initial dosing recommendations, online pediatric and neonatal Lexi-Drugs® (Lexicomp, Ohio, USA) (20) was the main neonatal dosing reference at National Guard Hospital, whereas all other participating hospitals relied mainly on Micromedex NeoFax (IBM Corp., Armonk, NY, United States) (21) (Table 1). Subsequent dose adjustments were guided by the vancomycin trough concentrations. In King Saud University Medical City, both vancomycin peak and trough concentrations were obtained for therapeutic drug monitoring. In all other hospitals, the practice was to collect trough concentrations only. Peak samples were collected 1 h after the end of infusions, and trough concentrations were collected 30 min before the next dose in a steady state. Exclusion criteria were: renal failure, receiving hemodialysis, missing dosing information and those sampled before the steady state were excluded. The study was IRB approved in all six participating centers.
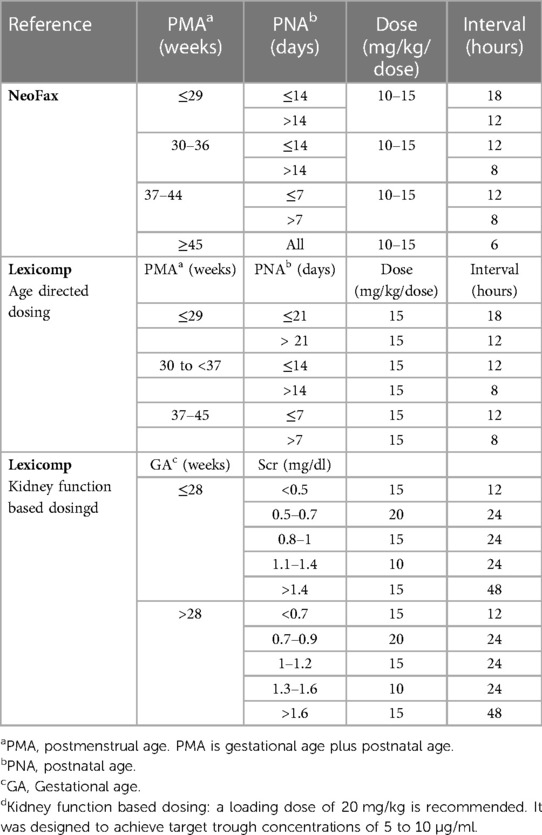
Table 1. Vancomycin dosing regimens from neonatal dosing references commonly used in participating hospitals.
Analytical assay
Three different validated methods were used for the analytical assay, as each hospital had a different system. One hospital used a chemiluminescent microparticle immunoassay with an Architect i4000SR immunoassay analyzer (Abbott), two hospitals used the kinetic interaction of microparticles in solution (KIMS) with a Cobas c system (Roche/Hitachi), and three hospitals used the homogeneous particle-enhanced turbidimetric inhibition immunoassay (PETINIA) with an Alinity c analyzer (Abbott). The assay range for the Architect immunoassay is from 0.5 to 83 µg/ml, the range for the KIMS assay is 4–80 µg/ml, and the range for the PETINIA is 1.4–100 µg/ml. For the Scr assays, four centers used the Jaffe method, and two centers used the enzymatic method. The following formula was used to convert the enzymatic assay to the Jaffe method (22):
Population pharmacokinetics
We split the data randomly into the training (70%) and validation (30%) data sets. Data splitting was performed using R statistical software. Pharmacokinetic modeling was performed using the Monolix software (2020R1) with the stochastic approximation expectation maximization algorithm.
Base model
First, we developed a structural model for vancomycin. This included testing one- and two-compartment models with linear and nonlinear elimination. For the between-subject variability in pharmacokinetic parameters, we used the following lognormal distribution:
where is the individual pharmacokinetic estimate for the ith individual, p is the median value of the pharmacokinetic parameter, and is the standard deviation for the ith individual with a mean of 0 and variance ω2. For residual unexplained variability, we tested different statistical models, including the constant, proportional, and combined error models. As different assays were used for vancomycin, we tested having different residual variability models accordingly.
Covariates
After we developed the base model, we evaluated the effect of covariates on pharmacokinetic parameters. We tested the effects of postnatal age, gestational age, PMA, weight, height, sex, Scr, presence of congenital heart disease, and VLBW vs. extremely low birth weight (ELBW) on all pharmacokinetic parameters. Covariates were tested by plotting the post hoc individual pharmacokinetic parameters (empirical Bayesian estimates) against covariates to identify possible correlations. Testing was performed stepwise using a likelihood ratio rest. The significance level was set at p < 0.05. The effect of continuous covariates was modeled using a power function as follows:
where is the individual pharmacokinetic parameter for the ith individual, P is the typical value of the pharmacokinetic parameter in the population, is the covariate value for the ith individual, and is the population mean of the covariate. The exponents for the effects of weight on total body clearance (Cl) and the volume of distribution (V) were fixed at 0.75 and 1, respectively (23–25). To determine the effect of age on Cl, we tested both power, linear, and sigmoidal functions to account for maturation (25, 26). The sigmoidal function is expressed as follows:
where hill is the Hill coefficient for clearance, and TMA50 is the PMA, where Cl is 50% of the mature value.
Model evaluation
Model evaluation and selection were guided by the objective function value, physiological plausibility, and standard diagnostic plots. The relative standard error of the pharmacokinetic estimates was also assessed, with an optimal value of <30%.
Validation
The final population pharmacokinetic model was evaluated using the validation dataset. Model performance was assessed using simulation diagnostics, Visual Predictive Check (VPC), and Normalized Prediction Distribution Error (NPDE) (27). The null hypothesis for the NPDE is that it follows a normal distribution, with a mean of 0 and a standard deviation of 1. The VPC is based on 1,000 dataset simulations, followed by a comparison between the observed data and the simulated concentrations.
Simulation
The final model was used to perform Monte Carlo simulations to determine the optimal vancomycin dose. We evaluated various doses ranging from to 10–20 mg/kg in 2.5 mg/kg increments every 6, 8, 12, 18, and 24 h. The final population pharmacokinetic model with the distribution of the pharmacokinetic parameters was embedded into the “Simulx” package in R. Simulx is part of the “mlxr” package in R and can be used to simulate the time concentration profile for different pharmacokinetic models (Lixoft, Antony, France, http://simulx.webpopix.org/). To ensure that the distribution of the significant covariates was identical to our patient population, we replicated our dataset 40 times (40 × 236 = 9,440). We simulated the time-concentration profiles for all 9,440 cases. We evaluated two targets in the simulations: (1) AUC0−24 of 400–600 µg.h/ml, as recommended in the IDSA treatment guidelines, (10) and (2) the less conservative target of AUC0−24 of 400–800 µg.h/ml, based on a prior study in pediatrics demonstrating increased nephrotoxicity for AUC0−24 > 800 µg.h/ml (28). We also estimated the probability of both trough levels >5 µg/ml and 20 µg/ml for each dosing regimen. Here, we considered troughs as the secondary marker for nephrotoxicity, as higher trough concentrations are also associated with nephrotoxicity (29–31). Prior studies in adults indicate risk of nephrotoxicity is approximately 15% for troughs between 15 and 20 µg/ml and increases to approximately 30% for troughs >20 µg/ml (30). Therefore, troughs >20 µg/ml should be always avoided, while troughs >15 µg/ml should be minimized if possible, as some patients can achieve a vancomycin therapeutic target of an AUC0−24 400–600 µg.h/ml at troughs <15 µg/ml (12, 30–32).
Results
In total, we included 236 patients: 162 in the training dataset, and 74 in the validation dataset. The total number of observations was 214 for the training dataset, and 97 for the validation dataset. Out of the 236 patients 36% had a culture confirmed infection. Approximately 58% of our patient population were extremely low birth weight (59% in the training data set and 55% in the validation data set). Both the training and validation data sets were similar in terms of bodyweight, PMA and Scr. The full baseline demographics of both datasets are shown in Table 2.
Population pharmacokinetics
Vancomycin pharmacokinetic data were best described using a one-compartment model with linear elimination and a proportional error model. Significant covariates identified were weight for volume of distribution (V) and weight, Scr, and PMA for clearance (Cl). Bodyweight was scaled to the median bodyweight of 0.93 kg, and the exponents for the effect of weight on V and Cl were fixed at 1 and 0.75 (23, 25, 26). The effect of PMA was best described using a sigmoidal function. The typical Cl value for a VLBW neonate weighing 0.93 kg, PMA equal to 26 weeks, and Scr of 0.6 mg/dl was 0.09 L/h, while the typical V value for a VLBW neonate weighing 0.93 kg was 0.81 L. The formulas below describe the predicted values of Cl and V:
Figure 1 shows the correlation between Cl normalized by body weight vs. Scr and PMA. The results of the final model are presented in Table 3. Diagnostic plots are shown in Figures 2–4.
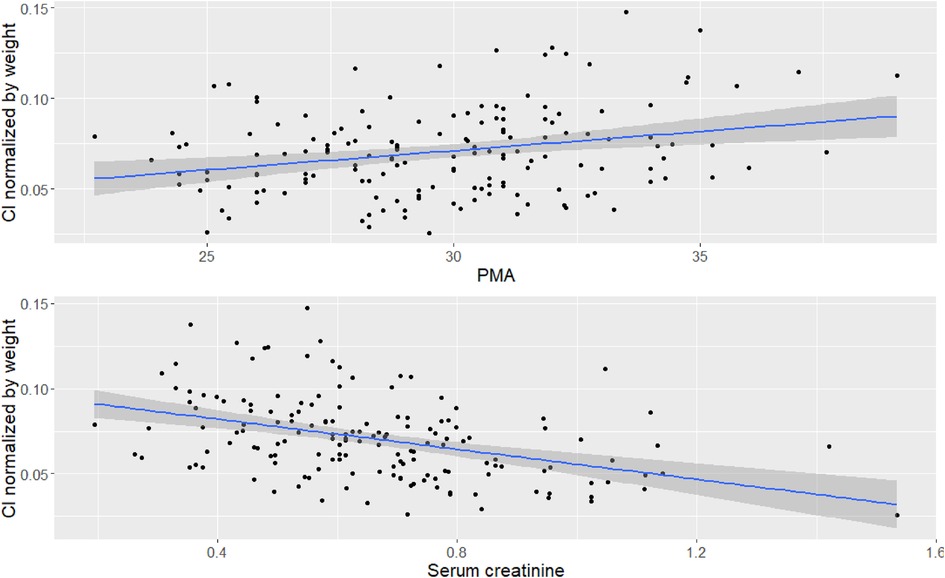
Figure 1. Correlation between covariates and Cl. Top figure is correlation between PMA and Cl normalized by bodyweight, bottom figure is correlation between Scr and Cl normalized by bodyweight.
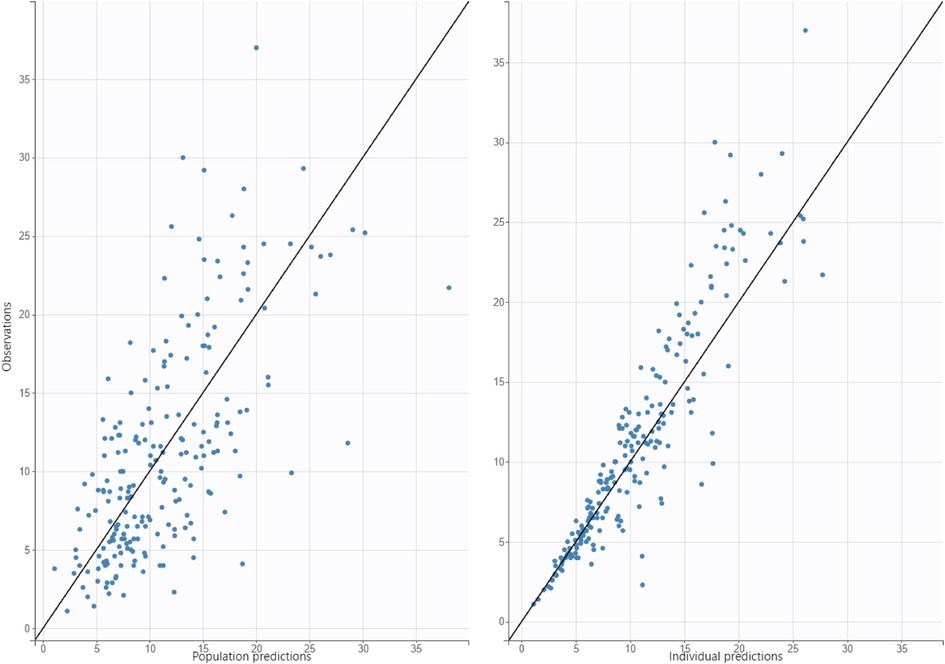
Figure 2. Diagnostic plot for observed concentrations vs. predicted concentrations, figure on left is population predictions, figure on right is individual predictions.
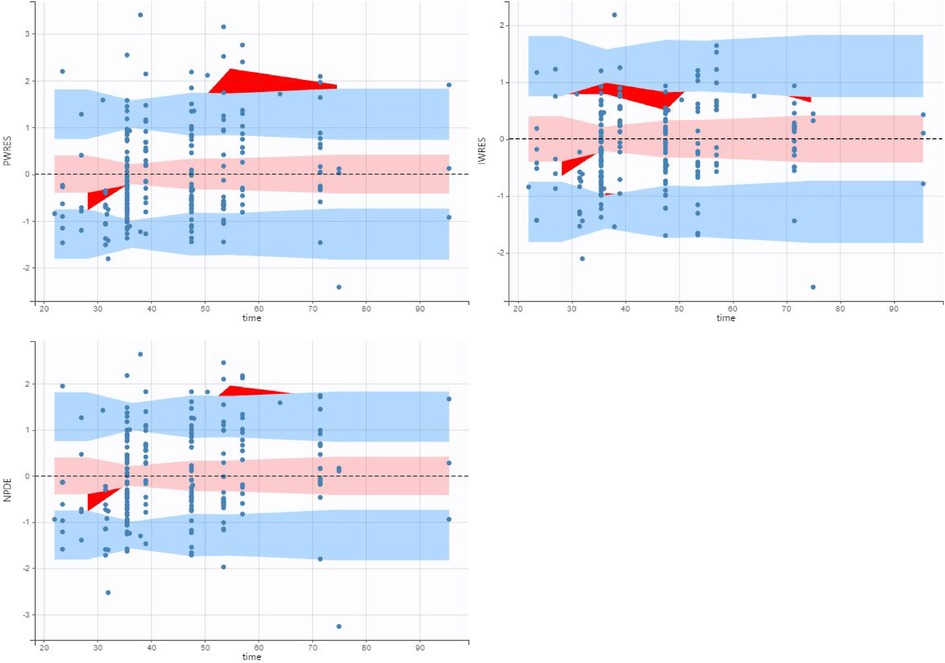
Figure 3. Population weighed residuals (top left), individual weighed residuals plot (top right) and normalized prediction distribution error plot (bottom left) for the final population pharmacokinetic model.
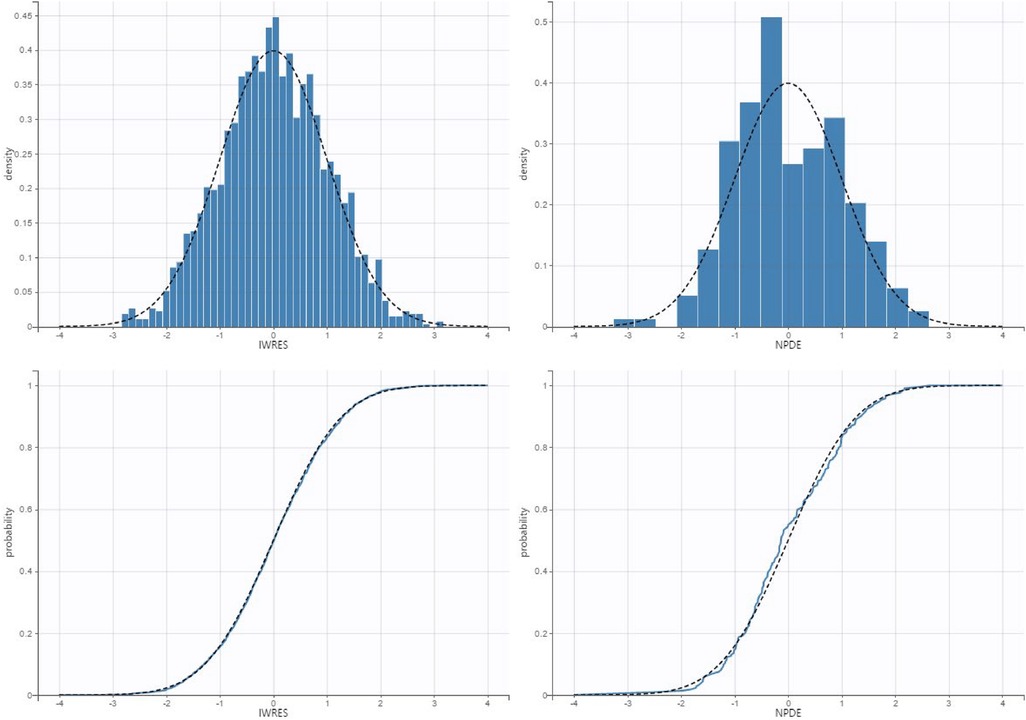
Figure 4. Qq plot and histogram of the individual weighed residuals (left) and normalized prediction errors (right).
Model validation
The validation dataset contained 97 observations from 74 participants. The diagnostic plots are shown in Figures 5 (VPC) and Figure 6 (NPDE). For the VPC, the observed and predicted medians and percentiles were similar. The mean NPDE was 0.043, and the standard deviation was 1.1, indicating that the model had limited bias and captured most of the data variability.
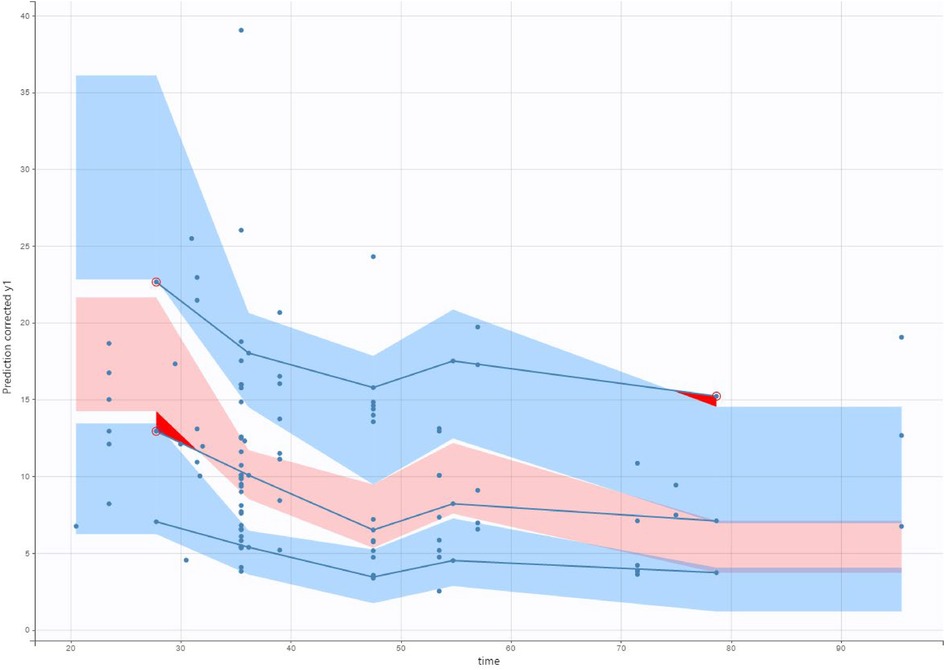
Figure 5. Visual predictive check (VPC) of our final model applied to the validation data set. The solid lines represent the 10th, 50th and 90th percentiles of observed data. The shaded regions represent the 90% confidence interval around the 10th, 50th and 90th percentiles of simulated data. The circles are observed concentrations.
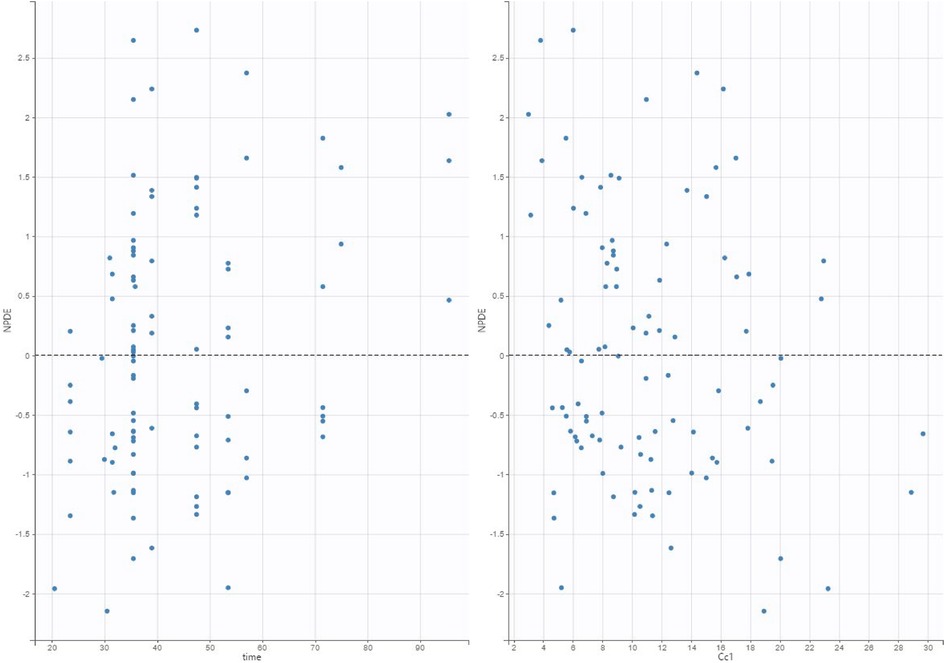
Figure 6. Normalized prediction distribution error (NPDE) plot of our final model applied to the validation data set.
Simulation
Simulations were performed for various dosing regimens based on the final population pharmacokinetic model. The simulations were performed for different age and Scr groups as follows: PMA above and below 29 weeks and Scr < 0.6, from 0.6–0.9, and >0.9–1.2 mg/dl, for a total of six groups. There were only five patients with Scr > 1.2 mg/dl; therefore, the simulations were capped at 1.2 mg/dl. The range for Scr, PMA and weight in the simulation data set was: Scr from 0.2–1.2 mg/dl, PMA from 22 to 42 weeks and for bodyweight was from 0.46 to 2.2 kg. Table 4 shows the simulations for selected dosing regimens; we only presented the doses that meet these criteria: probability of AUC0−24 > 400 µg/h/ml is >60%, and probability of AUC0−24 > 800 µg.h/ml or trough >20 µg/ml is <10% to avoid nephrotoxicity. For each group, we identified 2–4 dosing regimens that meet these criteria. Dose selection would depend on the selected target: the narrower target of AUC0−24 which is equal to 400–600 µg.h/ml, or the wider target of AUC0−24 which is equal to 400–800 µg.h/ml.
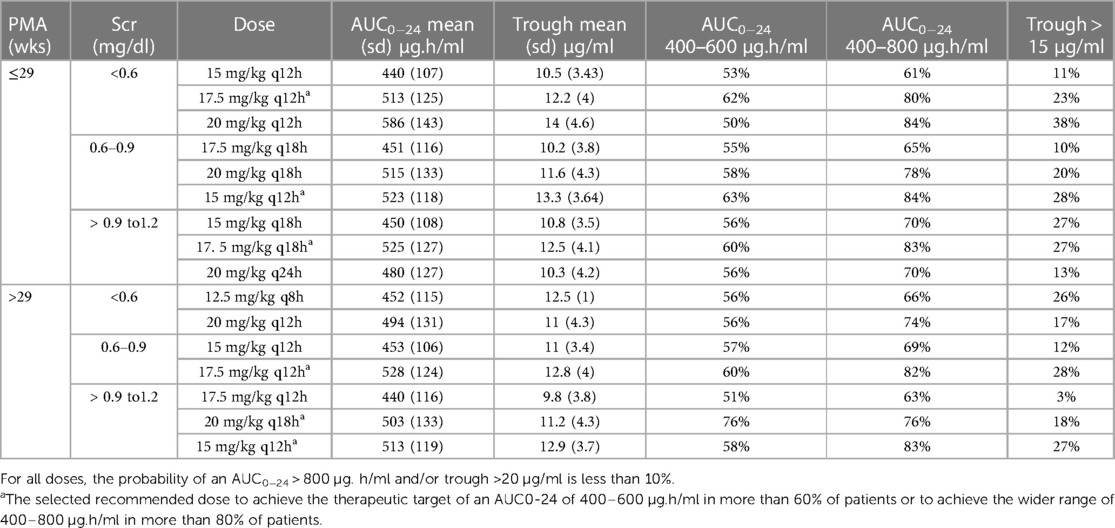
Table 4. Summary statistics of the predicted AUC and trough concentrations and Monte Carlo simulations for various dosing regimens of vancomycin in VLBW neonates.
Table 5 shows the recommended optimal dose depending on the selected target (400–600 or 400–800 µg.h/ml). The recommend doses to maintain an AUC0−24 of 400–600 µg.h/ml are expected to achieve the therapeutic target in more than 60% of patients. If we select the wider range of 400–800 µg.h/ml, the recommended doses would achieve the target in more than 80% of patients. However, the 400–800 µg.h/ml target would require higher doses and may increase the probability of elevated trough concentrations and AUCs. The only exception was for neonates with PMA > 29 weeks and Scr < 0.6 mg/dl, the highest achieved probabilities were 56% for the target AUC0−24 of 400–600 µg.h/ml and 74% for the target AUC0−24 of 400–800 µg.h/ml with the selected dosing regimen.
Discussion
Vancomycin, a drug with a narrow therapeutic window, is commonly used to treat life-threatening infections in neonates, such as MRSA. Previous clinical studies have demonstrated that vancomycin efficacy is maximized at AUC0−24/MIC > 400 µg.h/ml (33–35). Vancomycin dosing in VLBW patients is challenging. In this study, we developed a population pharmacokinetic model and identified the optimal initial dose for the VLBW neonate population. The significant covariates identified in our analysis were weight for V and weight, Scr, and PMA for Cl, consistent with prior studies in neonates (36). Also, our estimates for V and Cl are within range of previous studies (36).
The most commonly used tertiary dosing references present vancomycin dosing recommendations for neonates considering only one or two of these variables. For example, Micromedex NeoFax recommends dosing based on a combination of PMA and PNA, pediatric and neonatal Lexicomp recommends two different dosing strategies, one based on a combination of PMA and PNA and the other based on a combination of GA and Scr (Table 1). As a result, the daily doses can differ widely by up to 50% between these studies. Studies have reported that the probability of achieving vancomycin therapeutic targets in neonates with these empiric dosing regimens is only 20%–50% (37).
To date, only two studies with very small sample size have evaluated the optimal vancomycin dosing in VLBW neonates (16, 17). Kato et al. (16) recommended a vancomycin dose of 10 mg/kg every 8 h as the initial dosage regimen for vancomycin in VLBW neonates. This model predicted vancomycin therapeutic target achievement in 86.7% of neonates. However, they developed their model based on data from only 10 VLBW neonates, and the simulations were not divided into categories according to Scr and PMA. The major difference between our findings and the Kato et al. model is that for most groups, except PMA > 29 weeks and low Scr < 0.6 mg/dl, we recommend against three times daily dosing, which would lead to elevated trough levels >20 µg/ml, possibly increasing the risk of nephrotoxicity. Sasano et al. (17) constructed a population pharmacokinetic model for vancomycin dosing based on data from 19 VLBW neonates and infants. They recommended a dose of 5–7.5 mg/kg/doseevery 12 h, especially when Scr > 0.6 mg/dl. The doses recommended by Sasano et al. are lower, compared with our results and those of other references, that is mainly because they evaluated a lower target range of AUC0−24 267–467 µg.h/ml. In our simulations, the selected doses largely depended on the target (AUC0−24 range of 400–600 or 400–800 µg.h/ml). Ideally, for each dose, target attainment should be approximately90%, and the probability of achieving toxic concentrations should be <10% (38). However, this would be challenging given the high variability in vancomycin pharmacokinetics in VLBW neonates and the narrow target for vancomycin (AUC0−24 400–600 µg.h/ml). When using the wider target of AUC0−24 400–800 µg.h/ml, dosing regimens could achieve the therapeutic target in 80–90% of patients. The upper cutoff of AUC0−24 800 µg.h/ml was based on a large previous study by Le et al. (9) in a pediatric population.
Notably, the pharmacokinetic/pharmacodynamic (PK/PD) target of vancomycin is mainly based on studies in adults extrapolated to pediatric and neonatal patients. In neonates, clinical studies identifying PK/PD targets and toxicity thresholds are lacking. Nephrotoxicity incidence in neonates is low (0.03%–6%) (39–41). Madigan et al. (41) identified probable vancomycin-related nephrotoxicity in only two out of 57 VLBW neonates. Some studies have attempted to assess the correlation between vancomycin exposure (trough and/or AUC) and nephrotoxicity; however, causal relationships are difficult to identify given the small number of events in these studies. Bhargava et al. (39) found a significant correlation between vancomycin trough concentration and nephrotoxicity. However, only three out of 110 subjects developed nephrotoxicity in this study. On the other hand, Viel-Thériault et al. (42) noted nephrotoxicity in 6% of patients, but the authors could not identify a correlation between vancomycin trough and nephrotoxicity. In a study by Tang et al.(43), increasing AUC0−24 was associated with an increase in nephrotoxicity. The authors identified a cutoff of AUC0−24 > 485 µg.h/ml as a predictor of nephrotoxicity in neonates. However, only seven out of 182 neonates developed nephrotoxicity in this study. Given the small number of nephrotoxicity events in these studies, it was difficult to draw meaningful conclusions regarding the correlation between vancomycin exposure and nephrotoxicity.
To the best of our knowledge, this is the largest study to evaluate vancomycin pharmacokinetics in neonates with VLBW. However, the limitations of our study include its retrospective nature; all data were collected from one country, and the pharmacokinetic model was built using only 1–2 samples per patient. Therefore, it is important for any center to validate any model to ensure that it fits the patient population before implementation in practice, as our model might not necessarily be generalizable to other populations. The evaluated targets were mainly based on data from adults, not neonates. Our study included only a few patients with Scr > 1.2 mg/dl; hence, our model does not apply to this population. Our study did not evaluate the clinical outcome for the patients. It is important for future studies to assess the correlation between vancomycin concentration and microbiologic and clinical outcome.
In conclusion, our population-specific model-based dosing approach shows promise for improving vancomycin target attainment in neonates with VLBW. Prospective clinical studies are needed to evaluate the benefits of this dosing regimen.
Data availability statement
The raw data supporting the conclusions of this article will be made available by the authors, without undue reservation.
Ethics statement
The studies involving human participants were reviewed and approved by Prince Sultan Military Medical City, Riyadh, Saudi Arabia King Faisal Specialist Hospital & Research Centre (Gen. Org)-Jeddah, Saudi Arabia King Abdulaziz Medical City, Ministry of National Guard Health Affairs, Riyadh, Saudi Arabia King Faisal Specialist Hospital and Research Center, Riyadh, Saudi Arabia Armed Forces Hospital Southern Region, Saudi Arabia King Saud University Medical City, Riyadh, Saudi Arabia. Written informed consent from the participants’ legal guardian/next of kin was not required to participate in this study in accordance with the national legislation and the institutional requirements.
Author contributions
AA data analysis and manuscript writing; MFAM data collection, study design and reviewed the paper; KMA data collection and data analysis; ZKA data collection, reviewed the paper and wrote part of the paper; HAM data collection, reviewed the paper and wrote part of the paper; AA study design and reviewed the paper; DAB study design and reviewed the paper; TMR data collection and data analysis; MAJ study design and reviewed the paper; AAs data collection, study design and reviewed the paper; MA study design, writing and reviewed the paper. All authors contributed to the article and approved the submitted version.
Funding
This work was funded by King Abdulaziz City for Science and Technology (KACST), Riyadh, Saudi Arabia, grant number: KACST 2-17-03-001-0014.
Conflict of interest
The authors declare that the research was conducted in the absence of any commercial or financial relationships that could be construed as a potential conflict of interest.
Publisher's note
All claims expressed in this article are solely those of the authors and do not necessarily represent those of their affiliated organizations, or those of the publisher, the editors and the reviewers. Any product that may be evaluated in this article, or claim that may be made by its manufacturer, is not guaranteed or endorsed by the publisher.
References
1. Oza S, Lawn JE, Hogan DR, Mathers C, Cousens SN. Neonatal cause-of-death estimates for the early and late neonatal periods for 194 countries: 2000-2013. Bull World Health Organ. (2015) 93(1):19–28. doi: 10.2471/BLT.14.139790
2. Liu L, Johnson HL, Cousens S, Perin J, Scott S, Lawn JE. Global, regional, and national causes of child mortality: an updated systematic analysis for 2010 with time trends since 2000. Lancet. (2012) 379(9832):2151–61. doi: 10.1016/S0140-6736(12)60560-1
3. Hornik CP, Fort P, Clark RH, Watt K, Benjamin DK Jr, Smith PB. Early and late onset sepsis in very-low-birth-weight infants from a large group of neonatal intensive care units. Early Hum Dev. (2012) 88(Suppl 2):S69–S74. doi: 10.1016/S0378-3782(12)70019-1
4. Sosnin N, Curtis N, Cranswick N, Chiletti R, Gwee A. Vancomycin is commonly under-dosed in critically ill children and neonates. Br J Clin Pharmacol. (2019) 85(11):2591–8. doi: 10.1111/bcp.14084
5. Stoll BJ, Hansen N, Fanaroff AA, Wright LL, Carlo WA, Ehrenkranz RA. Late-onset sepsis in very low birth weight neonates: the experience of the NICHD neonatal research network. Pediatrics. (2002) 110(2 Pt 1):285–91. doi: 10.1542/peds.110.2.285
6. Lodise TP, Scheetz M, Carreno JJ, Chambers H, Fowler V, Holland TL. Associations between vancomycin exposure and acute kidney injury within the recommended area under the curve therapeutic exposure range among patients with methicillin-resistant. Open Forum Infect Dis. (2022) 9(2):ofab651. doi: 10.1093/ofid/ofab651
7. Chavada R, Ghosh N, Sandaradura I, Maley M, Van Hal SJ. Establishment of an AUC. Antimicrob Agents Chemother. (2017) 61(5):e02535-16. doi: 10.1128/AAC.02535-16
8. Aljefri DM, Avedissian SN, Rhodes NJ, Postelnick MJ, Nguyen K, Scheetz MH. Vancomycin area under the curve and acute kidney injury: a meta-analysis. Clin Infect Dis. (2019) 69(11):1881–7. doi: 10.1093/cid/ciz051
9. Le J, Ny P, Capparelli E, Lane J, Ngu B, Muus R. Pharmacodynamic characteristics of nephrotoxicity associated with vancomycin use in children. J Pediatric Infect Dis Soc. (2015) 4(4):e109–116. doi: 10.1093/jpids/piu110
10. Rybak MJ, Le J, Lodise TP, Levine DP, Bradley JS, Liu C. Therapeutic monitoring of vancomycin for serious methicillin-resistant Staphylococcus aureus infections: a revised consensus guideline and review by the American society of health-system pharmacists, the infectious diseases society of America, the pediatric infectious diseases society, and the society of infectious diseases pharmacists. Am J Health Syst Pharm. (2020) 77(11):835–64. doi: 10.1093/ajhp/zxaa036
11. Marqués-Miñana MR, Saadeddin A, Peris JE. Population pharmacokinetic analysis of vancomycin in neonates. A new proposal of initial dosage guideline. Br J Clin Pharmacol. (2010) 70(5):713–20. doi: 10.1111/j.1365-2125.2010.03736.x
12. Frymoyer A, Hersh AL, El-Komy MH, Gaskari S, Su F, Drover DR. Association between vancomycin trough concentration and area under the concentration-time curve in neonates. Antimicrob Agents Chemother. (2014) 58(11):6454–61. doi: 10.1128/AAC.03620-14
13. Abdulla A, Edwina AE, Flint RB, Allegaert K, Wildschut ED, Koch BC. Model-informed precision dosing of antibiotics in pediatric patients: a narrative review. Front Pediatr. (2021) 9:624639. doi: 10.3389/fped.2021.624639
14. Chow TC, Li JY, Wong JC, Poon FM, Lam HS, Lam TT. Vancomycin prescribing practices and therapeutic drug monitoring for critically ill neonatal and pediatric patients: a survey of physicians and pharmacists in Hong Kong. Front Pediatr. (2020) 8:538298. doi: 10.3389/fped.2020.538298
15. Li ZL, Liu YX, Jiao Z, Qiu G, Huang JQ, Xiao YB. Population pharmacokinetics of vancomycin in Chinese ICU neonates: initial dosage recommendations. Front Pharmacol. (2018) 9:603. doi: 10.3389/fphar.2018.00603
16. Kato H, Hagihara M, Nishiyama N, Koizumi Y, Mikamo H, Matsuura K. Assessment of optimal initial dosing regimen with vancomycin pharmacokinetics model in very low birth weight neonates. J Infect Chemother. (2017) 23(3):154–60. doi: 10.1016/j.jiac.2016.11.009
17. Sasano H, Aoki K, Arakawa R, Hanada K. Population pharmacokinetic analysis and dose regimen optimization in Japanese infants with an extremely low birth weight. Antimicrob Agents Chemother. (2021) 65(3):e02523-20. doi: 10.1128/AAC.02523-20
18. Abouelkheir M, Almohaizeie A, Almutairi A, Almuhisen S, Alqahtani S, Alsultan A. Evaluation of vancomycin individualized model-based dosing approach in neonates. Pediatr Neonatol. (2022). doi: 10.1016/j.pedneo.2022.10.006. [Epub ahead of print]36581523
19. Stone SB, Benner K, Utley A, MacLennan P, Coghill CH. Achieving vancomycin troughs within goal range in low birth weight neonates. J Pediatr Pharmacol Ther. (2021) 26(1):56–61. doi: 10.5863/1551-6776-26.1.56
20. Vancomycin.. In: Lexicomp Online, Pediatric & Neonatal Lexi-Drugs. Hudson, OH: Lexi-Comp, Inc. (2021). Available from: www.online.lexi.com (cited January 15, 2023).
21. Vancomycin.. In: IBM Micromedex Neofax. Greenwood Village, CO: IBM Corportation. (2021). Available from: www.micromedexsolutions.com (cited January 15, 2023).
22. Srivastava T, Alon US, Althahabi R, Garg U. Impact of standardization of creatinine methodology on the assessment of glomerular filtration rate in children. Pediatr Res. (2009) 65(1):113–6. doi: 10.1203/PDR.0b013e318189a6e8
23. Anderson BJ, Holford NH. Mechanistic basis of using body size and maturation to predict clearance in humans. Drug Metab Pharmacokinet. (2009) 24(1):25–36. doi: 10.2133/dmpk.24.25
24. Anderson BJ, Allegaert K, Holford NH. Population clinical pharmacology of children: modelling covariate effects. Eur J Pediatr. (2006) 165(12):819–29. doi: 10.1007/s00431-006-0189-x
25. Anderson BJ, Allegaert K, Van den Anker JN, Cossey V, Holford NH. Vancomycin pharmacokinetics in preterm neonates and the prediction of adult clearance. Br J Clin Pharmacol. (2007) 63(1):75–84. doi: 10.1111/j.1365-2125.2006.02725.x
26. Holford N. Dosing in children. Clin Pharmacol Ther. (2010) 87(3):367–70. doi: 10.1038/clpt.2009.262
27. Brendel K, Comets E, Laffont C, Mentré F. Evaluation of different tests based on observations for external model evaluation of population analyses. J Pharmacokinet Pharmacodyn. (2010) 37(1):49–65. doi: 10.1007/s10928-009-9143-7
28. Le J, Bradley JS, Murray W, Romanowski GL, Tran TT, Nguyen N. Improved vancomycin dosing in children using area under the curve exposure. Pediatr Infect Dis J. (2013) 32(4):e155–63. doi: 10.1097/INF.0b013e318286378e
29. Lodise TP, Patel N, Lomaestro BM, Rodvold KA, Drusano GL. Relationship between initial vancomycin concentration-time profile and nephrotoxicity among hospitalized patients. Clin Infect Dis. (2009) 49(4):507–14. doi: 10.1086/600884
30. van Hal SJ, Paterson DL, Lodise TP. Systematic review and meta-analysis of vancomycin-induced nephrotoxicity associated with dosing schedules that maintain troughs between 15 and 20 milligrams per liter. Antimicrob Agents Chemother. (2013) 57(2):734–44. doi: 10.1128/AAC.01568-12
31. Neely MN, Youn G, Jones B, Jelliffe RW, Drusano GL, Rodvold KA. Are vancomycin troughs adequate for optimal dosing? Antimicrob Agents Chemother. (2013) 58:309–16. doi: 10.1128/AAC.01653-13
32. Alsultan A, Abouelkheir M, Albassam A, Alharbi E, Assiri A, Alqahtani S. AUC- vs. trough-guided monitoring of vancomycin in infants. Indian J Pediatr. (2020) 87(5):359–64. doi: 10.1007/s12098-019-03162-5
33. Moise-Broder PA, Forrest A, Birmingham MC, Schentag JJ. Pharmacodynamics of vancomycin and other antimicrobials in patients with Staphylococcus aureus lower respiratory tract infections. Clin Pharmacokinet. (2004) 43(13):925–42. doi: 10.2165/00003088-200443130-00005
34. Rybak MJ, Le J, Lodise TP, Levine DP, Bradley JS, Liu C. Therapeutic monitoring of vancomycin for serious methicillin-resistant Staphylococcus aureus infections: a revised consensus guideline and review by the American society of health-system pharmacists, the infectious diseases society of America, the pediatric infectious diseases society, and the society of infectious diseases pharmacists. Clin Infect Dis. (2020) 71(6):1361–4. doi: 10.1093/cid/ciaa303
35. Men P, Li HB, Zhai SD, Zhao RS. Association between the AUC0-24/MIC ratio of vancomycin and its clinical effectiveness: a systematic review and meta-analysis. PLoS One. (2016) 11(1):e0146224. doi: 10.1371/journal.pone.0146224
36. Aljutayli A, El-Haffaf I, Marsot A, Nekka F. An update on population pharmacokinetic analyses of vancomycin, part II: in pediatric patients. Clin Pharmacokinet. (2022) 61(1):47–70. doi: 10.1007/s40262-021-01050-w
37. Frymoyer A, Stockmann C, Hersh AL, Goswami S, Keizer RJ. Individualized empiric vancomycin dosing in neonates using a model-based approach. J Pediatric Infect Dis Soc. (2019) 8(2):97–104. doi: 10.1093/jpids/pix109
38. Turnidge J, Paterson DL. Setting and revising antibacterial susceptibility breakpoints. Clin Microbiol Rev. (2007) 20(3):391–408. table of contents. doi: 10.1128/CMR.00047-06
39. Bhargava V, Malloy M, Fonseca R. The association between vancomycin trough concentrations and acute kidney injury in the neonatal intensive care unit. BMC Pediatr. (2017) 17(1):50. doi: 10.1186/s12887-017-0777-0
40. Alrahahleh D, Xu S, Luig M, Kim HY, Alffenaar JW. Dosing of vancomycin and target attainment in neonates: a systematic review. Int J Antimicrob Agents. (2022) 59(2):106515. doi: 10.1016/j.ijantimicag.2021.106515
41. Madigan T, Teng CB, Koshaish J, Johnson KR, Graner KK, Banerjee R. Optimization of vancomycin dosing in very low-birth-weight preterm neonates. Am J Perinatol. (2015) 32(1):83–6. doi: 10.1055/s-0034-1376183
42. Viel-Thériault I, Martin B, Thompson-Desormeaux F, Blackburn J, Moussa A, Autmizguine J. Vancomycin drug monitoring in infants with CoNS sepsis-target attainment, microbiological response and nephrotoxicity. J Perinatol. (2020) 40(1):97–104. doi: 10.1038/s41372-019-0519-2
Keywords: pharmacokenetics, very low birth weigh neonates, vancomycin, infectious disease, pharmacodynamic
Citation: Alsultan A, Al Munjem MF, Atiq KM, Aljehani ZK, Al Muqati H, Almohaizeie A, Ballal DA, Refaei TM, Al Jeraisy M, Assiri A and Abouelkheir M (2023) Population pharmacokinetics of vancomycin in very low birth weight neonates. Front. Pediatr. 11:1093171. doi: 10.3389/fped.2023.1093171
Received: 8 November 2022; Accepted: 3 March 2023;
Published: 30 March 2023.
Edited by:
Thomas S. Murray, Yale University, United StatesReviewed by:
Michelle Rychalsky, Yale-New Haven Hospital, United StatesJiraganya J. J. Bhongsatiern, Regeneron Pharmaceuticals, Inc., United States
© 2023 Alsultan, Al Munajem, Atiq, Aljehani, Al Muqati, Almohaizeie, Ballal, Refaei, Al Jeraisy, Assiri and Abouelkheir. This is an open-access article distributed under the terms of the Creative Commons Attribution License (CC BY). The use, distribution or reproduction in other forums is permitted, provided the original author(s) and the copyright owner(s) are credited and that the original publication in this journal is cited, in accordance with accepted academic practice. No use, distribution or reproduction is permitted which does not comply with these terms.
*Correspondence: Abdullah Alsultan YWJzdWx0YW5Aa3N1LmVkdS5zYQ== Manal Abouelkheir bWFuYWwuYWJvZWxraGVpckBtaXVlZ3lwdC5lZHUuZWc=
Specialty Section: This article was submitted to Pediatric Infectious Diseases, a section of the journal Frontiers in Pediatrics