- 1Neonatal Intensive Care Unit, Fondazione IRCCS Ca' Granda Ospedale Maggiore Policlinico, Milan, Italy
- 2Department of Clinical Sciences and Community Health, Università degli Studi di Milano, Milan, Italy
- 3Angelo Bianchi Bonomi Hemophilia and Thrombosis Center, Fondazione IRCCS Ca' Granda Ospedale Maggiore Policlinico, Milan, Italy
- 4Neonatal Intensive Care Unit, Fondazione IRCCS Policlinico San Matteo, Pavia, Italy
Extracorporeal membrane oxygenation (ECMO) is a life-saving support for cardio-respiratory function. Over the last 50 years, the extracorporeal field has faced huge technological progress. However, despite the improvements in technique and materials, coagulation problems are still the main contributor to morbidity and mortality of ECMO patients. Indeed, the incidence and survival rates of the main hemorrhagic and thrombotic complications in neonatal respiratory ECMO are relevant. The main culprit is related to the intrinsic nature of ECMO: the contact phase activation. The exposure of the human blood to the non-endothelial surface triggers a systemic inflammatory response syndrome, which chronically activates the thrombin generation and ultimately leads to coagulative derangements. Pre-existing illness-related hemostatic dysfunction and the peculiarity of the neonatal clotting balance further complicate the picture. Systemic anticoagulation is the management's mainstay, aiming to prevent thrombosis within the circuit and bleeding complications in the patient. Although other agents (i.e., direct thrombin inhibitors) have been recently introduced, unfractionated heparin (UFH) is the standard of care worldwide. Currently, there are multiple tests exploring ECMO-induced coagulopathy. A combination of the parameters mentioned above and the evaluation of the patient's underlying clinical context should be used to provide a goal-directed antithrombotic strategy. However, the ideal algorithm for monitoring anticoagulation is currently unknown, resulting in a large inter-institutional diagnostic variability. In this review, we face the features of the available monitoring tests and approaches, mainly focusing on the role of point-of-care (POC) viscoelastic assays in neonatal ECMO. Current gaps in knowledge and areas that warrant further study will also be addressed.
Introduction
The role of extracorporeal membrane oxygenation (ECMO) in the neonatal population has been clearly established in the last decades (1). Furthermore, it has been demonstrated ECMO supports cardiac and pulmonary reversible diseases once conventional management has failed (2). In comparison, extracorporeal cardiopulmonary resuscitation (ECPR) represents a minor neonatal ECMO indication (2). In 2021 in Europe, 261 neonatal ECMO have been performed, 148 for pulmonary, 88 for cardiac, and 25 for ECPR indications (3).
Despite technological improvements, such as miniaturization and simplification of circuits, new coating systems, new vascular accesses, low hemolytic centrifugal pumps, and increasingly efficient artificial membranes, hemostatic management remains a real challenge during ECMO (4). Thrombosis and bleeding frequently occur, even in the same patient. Coagulation problems are the main leading cause of mortality and morbidity, as reported by the Extracorporeal Life Support Organization (ELSO) registry in 2021 (5). In neonatal pulmonary ECMO, the most common hemostatic complications reported are brain hemorrhage (intraventricular or intra-extra parenchymal, respectively, 2.9 and 3.3%), peripheral cannula site bleeding (4.3%), surgical site bleeding (6.3%), clots in the circuit components (29.2%), moderate or severe hemolysis (10%), pulmonary hemorrhage (3.4%), gastrointestinal bleeding (1.4%) (5).
The ideal ECMO running should consist of an “unclotted” circuit in a “clotting” patient. However, patient and circuit factors make obtaining this hemostatic balance difficult. Hence, the real ECMO running is an “unclotted” patient and a “clotted” circuit.
The exposure of human blood to the non-endothelial surface of the circuit determines platelet aggregation, continuous activation of the coagulation cascade, and the chronic generation of thrombin. Over time, this leads to coagulation problems, especially in a prothrombotic trend (6). Moreover, the continuous platelet activation results in down-regulation of the receptors and degranulation, making platelets hyporeactive and dysfunctional, representing an important cause of bleeding (6).
The neonatal hemostatic system is intrinsically unstable, being in developmental evolution in the first days and weeks of life (7, 8). This means it could be more susceptible to hemostatic alterations and less capable of facing bleeding or thrombotic triggers. Moreover, the pre-existing conditions and comorbidities that led the patient to ECMO may have triggered a systemic inflammatory response, contributing to the coagulation derangements (9–12).
Unfractionated heparin (UFH) is the mainstay of hemostatic treatment (13, 14). Hemostatic function monitoring allows for dynamically adjusting the heparin dose to maintain a correct balance between anticoagulation and bleeding (13). Regrettably, there is not a single reliable test, but a combination of different laboratory examinations helps to adjust the antithrombotic therapy (4, 14, 15). Each method measures different functional and quantitative hemostatic variables and may not be comparable straightaway: activated partial thromboplastin time (APTT), anti-factor Xa, and antithrombin (AT) levels are plasma-based tests, activated clotting time (ACT), and viscoelastic assays (thrombo-elastography/thrombo-elastometry) are performed on whole blood. Mainly, thromboembolic events frequently occur, although traditional coagulation tests are in the targeted ranges (15). In addition, reference ranges for neonatal age are lacking for some tests. All these factors contribute to the sizeable inter-institutional variability in diagnostic test use and management.
This review discusses the relative pros and cons of the different monitoring approaches. Next, we address the challenges in hemostatic diagnostics and anticoagulation management, focusing on the role of point-of-care (POC) viscoelastic assays in neonatal ECMO. We conclude by discussing current gaps in knowledge, emerging technologies, and areas that warrant further study.
Why do we need to worry about hemostasis in neonates on ECMO?
Patient-related factors
Developmental hemostasis
The concept of “developmental hemostasis” refers to the maturation of the coagulation system from fetal to adult life (7, 8, 16–18). Newborns have reduced levels (around 50% of adults) of most procoagulant factors, especially the vitamin-K dependent (FII, FIX, FX, FVII), factor XI, XII, prekallikrein, and high molecular weight kininogen (19–21). Moreover, anticoagulant factors vitamin K dependent (protein C and protein S) and antithrombin are also reduced. Similarly, the clot lysis is reduced (6, 12). With the reduction in pro- and anticoagulant factors, the hemostatic system of a healthy term neonate is then considered balanced (18, 22–24). On the other hand, factors VIII, XIII, fibrinogen, and von Willebrand factor (vWf) levels are similar or even increased at birth compared to adult life (21).
Primary hemostasis is also different in the neonatal age. The function is considered somewhat impaired even in a normal or slightly reduced platelet count at birth (25–27). Despite this described “hyporeactivity,” the functional tests, such as bleeding time and closure time (CT) of the platelet function analyzer (PFA-100), are often shortened in newborns (25, 28). Term newborns have CT shorter than preterm ones accordingly to the hyporeactivity of the preterm platelets (29). Primary hemostasis is maintained through higher hematocrit levels, mean corpuscular volume (MCV) of neonatal erythrocytes, vWf, and a large amount of high molecular weight multimers of vWf, which counterbalance platelets hyporeactivity (26, 27, 30).
Then, this developing hemostatic system is intrinsically susceptible to bleeding or thrombosis in critical neonates, depending on the underlying disease and the systemic response to different triggers (12). In this scenario, ECMO may further complicate the picture by disrupting this delicate balance due to the increased risk of dilutional coagulopathy, reducing thrombin generation, and rising bleeding risk (Table 1). In addition, neonates are more prone to heparin resistance secondary to low antithrombin concentrations (6).
Pre-existing conditions and comorbidities
A strict link between inflammatory response caused by infection or injury and activation of the coagulation cascade is well-described (9). Therefore, a disruption in one of these mechanisms may affect the entire balance, leading to excessive inflammatory response or coagulopathy (Table 1) (10).
Inflammation determines the activation of primary and secondary hemostasis in the endothelial lumen, decreases the action of natural anticoagulants, and inhibits the antifibrinolytic system through cytokine release. Also, natural anticoagulants reduce the endothelial response to inflammatory mediators (9, 11). Therefore, critically ill newborns who have systemic inflammatory response syndrome often have associated coagulopathy (12).
The baseline coagulation status and the platelet count may vary depending on the underlying pathology, as newborns affected by meconium aspiration syndrome have different hemostatic profile compared with septic or cardiopathic ones.
For example, children with cyanotic congenital heart disease (CHD) have thrombocytopenia secondary to increased destruction of peripheral platelets and reduced platelet production despite the increased erythropoietic stimulus (11, 31). The pathogenetic mechanism is not entirely clear. However, it appears to be due to the inhibition of megakaryocyte differentiation consequent to chronic hypoxia and right-to-left shunt in the Botallo arterial duct, allowing blood to bypass the pulmonary bed, where megakaryocytes break down into platelets (32–34). Furthermore, the increased risk of bleeding in these patients is due not only to thrombocytopenia but also to reduced levels of large multimers of vWf (11, 32). In addition, bone marrow suppression in sepsis or immune reactions may also determine thrombocytopenia (35).
Circuit-related factors
The ECMO-circuit
All components of the ECMO circuit, such as cannulas, tubes, blood pump, and membrane oxygenator, are artificial non-biological surfaces (12, 36). Therefore, blood exposure to these foreign biomaterials activates the coagulation cascade and the inflammatory response (Table 1) (35, 37).
Some advances in technology have been obtained in the last years: 1. biomimetic tubes, with heparin-coated or nitric oxide-bonded surfaces, are thought to reduce cellular activation, release of proinflammatory mediators, and platelets activation; 2. biopassive materials for circuit lines, such as phosphorylcholine or poly-2-methoxy-ethyl acrylate (PMEA), prevent the thrombogenic response, with a reduction in platelets and complement activation; 3. endothelialization of the circuit, in vivo or in vitro, could be the future for ECMO circuits, as endothelium is the key element which physiologically regulates inflammation and coagulation within the body (4). However, these technologies still do not avoid the need for systemic anticoagulation, and they are still not widely available soon as part of ECMO circuits (4).
After the start of ECMO, plasma proteins, including albumin, factor XII, fibrinogen, and kallikrein, are immediately attached to the non-endothelial surface, followed by vWf and glycoproteins (6, 13, 36). Factor XII is then activated to factor XIIa, which activates the intrinsic pathway of the coagulation cascade, with consequent thrombin generation (6).
Von Willebrand factor binds and activates circulating platelets, producing exposure of tissue factor (TF) and activation of the extrinsic coagulation pathway (13). Kallikrein activates systemic inflammatory response via the complement system. The complement cascade determines further activation of platelets, polymorphonuclear cells, and expression of cytokines which further increase the proinflammatory state (12, 36). Fibrinolysis is then activated to limit clot formation and thrombosis (13). In reaction to the massive procoagulant state, overactivation of fibrinolysis determines the consumption of clotting factors, impaired platelet function, and thrombocytopenia (21). In neonates, contact and complement activation seem to predominate in the first 24 h, while after 72 h of ECMO start, clotting, and fibrinolytic system play the leading role (Table 1) (12, 35).
Inflammation and endothelial dysfunction
Proinflammatory cytokines are secreted in response to the patient's illness and exposure to the ECMO circuit. Inflammation and hypercoagulability are strictly connected: increased levels of interleukin (IL) 6 are associated with ECMO-related multiorgan failure, while some soluble cytokines (IL-6, IL-8, tumor necrosis factor-α) may contribute to the endothelial damage (36). Turbulent blood flow and increased shear stress generated by the ECMO circuit promote further cellular damage, platelet activation, and hemolysis (12, 35). Endothelial dysfunction may lead to continuous platelet and coagulation activation, resulting in consumption coagulopathy (Table 1) (12).
Thrombin generation
Thrombin generation results from the coagulation cascade via TF and contact system. Thrombin is central in the coagulation pathway, as it converts fibrinogen to fibrin and activates factors XIII, V, VIII, XI, and platelets. The production of a small amount of thrombin determines an explosive amplification of the entire process (12, 36). Unfractionated heparin inhibits clot formation but is not totally efficacious in inhibiting thrombin generation and coagulation within the circuit (12, 36). Moreover, neonates on ECMO showed persistent coagulation activation, represented by increased thrombin generation and fibrinolysis (Table 1) (38).
Platelets and von Willebrand factor
Platelet alterations involve both platelet count and platelet function. They are well-described during ECMO and may lead to bleeding and thrombotic complications (35). Thrombocytopenia may result from underlying conditions, such as sepsis, which is especially common in neonatal intensive care units (NICUs), where its incidence is 18–35% (39). In addition, hemodilution from the ECMO priming volume may exacerbate the pre-existing reduced platelet count (35). Usually, platelet count decreases >40% within the first 1–2 h of ECMO start (14).
Functional impairment is determined by platelet activation, which occurs at the start of ECMO, resulting from contact with the circuit's surface, shear stress, and turbulent flow within the tubes. Activated platelets adhere to the ECMO oxygenator and endothelium, increasing the thrombotic risk (12, 35). In addition, platelet microparticles, fragments of activated platelet membrane released during shear stress, contribute to thrombus formation as they are involved in vascular injury and promote prothrombotic and proinflammatory conditions (12, 35, 40, 41). Their procoagulant properties are mediated by membrane phosphatidylserine exposure, which activates the contact-dependent coagulation pathway. Elevated levels of microparticles are found in many thrombotic states, such as arterial thrombosis, idiopathic thrombocytopenic purpura, and thrombotic thrombocytopenia. Furthermore, platelet microparticles are involved in cell-to-cell communication, modulating innate and adaptative immunity, and cancer-related angiogenesis (40, 41).
The dysfunctional platelet activation determines platelet exhaustion and decreased aggregation, resulting from degranulation, receptor inhibition, and down-regulation. Therefore, underlying patient conditions, consumption, and hemodilution result in thrombocytopenia (36, 42). The degree of thrombocytopenia is correlated with the patient's illness severity and platelet count at the time of starting ECMO rather than with the duration (4). Often, platelet transfusions are needed to correct the severe thrombocytopenia and reduce the bleeding risk (14).
Moreover, drugs with platelet inhibitory properties, such as milrinone, nitric oxide, and histamine-2-receptor blockers, are usually prescribed during ECMO, thus potentially interfering with the hemostatic balance (Table 1) (36, 43, 44).
Shear stress determines disruption of high molecular weight vWf multimers, causing the “acquired von Willebrand syndrome” (AVWS), which consists of a functional defect in vWf, associated with bleeding (12, 35, 45, 46).
This condition is well described in patients with mechanical valves and ventricular assist devices (VADs) (47). However, literature on pediatric patients is scarce, despite evidence suggesting this phenomenon is common during ECMO (47, 48). Acquired von Willebrand syndrome might occur in 100% of the patients under ECMO support, and it is reversible at the weaning (47). Due to the pivotal role of high-weight vWf multimers in neonatal primary hemostasis, AVWS might be more pronounced in newborns than adults as they counterbalance platelet hyporeactivity (46). Therefore, diagnosis of AVWS in case of bleeding should always be considered.
Hemolysis
Hemolysis is increased in neonates during ECMO compared to older children and adults due to turbulent flow through the smaller cannulas; fetal hemoglobin, which makes red blood cells more susceptible to mechanical stress; elevated hematocrit, and hemoglobin concentration (Table 1) (12, 49, 50). In addition, red blood cells are destroyed by mechanical damage in the circuit pump and complement activation (14). Plasma-free hemoglobin levels >50 mg/dl are suggestive of hemolysis, even if the measurement of plasma-free hemoglobin is not standardized across centers (12, 49, 50). Hemolysis contributes to renal failure, lead to the need for circuit component change, and has been associated with thrombotic events, transfusions, and mortality (49, 51). The explanation for hemolysis complications could be the overwhelming haptoglobin and hemopexin, which bind free hemoglobin. Then, the free hemoglobin binds the endogenous nitric oxide, determining vasoconstriction (4).
How can we achieve the hemostatic balance in neonates on ECMO?
Unfractionated heparin and antithrombin
During ECMO, anticoagulation is mandatory to maintain the circuit components' patency and avoid thrombosis while increasing the patient's risk of bleeding (6, 14). Therefore, continuous infusion of UFH is the standard of care in pediatric and neonatal ECMO. However, the evidence is mainly derived from adult population studies, and data on pharmacokinetics and pharmacodynamics of UFH in children and neonates are scanty (13, 14). In a survey conducted in the United States (US), 94% of the centers used continuous UFH infusion, while the remaining 6% used bivalirudin, a direct thrombin inhibitor (52). This differs from a previous survey, in which 100% of the centers used UFH (53).
Unfractionated heparin binds antithrombin and increases its activity by 2,000- to 3,000-fold (36). Unfractionated heparin-antithrombin complex inhibits free thrombin and prevents further thrombin generation, whereas thrombin bound to fibrin or subendothelial matrix is unaffected by UFH (12–14, 36). As a result, bounded thrombin will still contribute to thrombin generation, increasing the need for heparin (21).
The advantage of UFH is the low cost, speed in action, familiarity with use, reversibility of its effect through a specific antidote, and anti-inflammatory properties (tissue regeneration, reduction in producing reactive oxygen species, and cardiovascular protection) (12, 13, 36). Furthermore, high heparin infusion dose has been associated with lower hemolysis and mortality rate (12, 50, 54).
Unfractionated heparin binds AT and other plasma proteins, such as acute phase reactant proteins, platelet factor 4, and high molecular weight multimers of vWf (13). The plasma concentration of all these proteins is increased due to the activation of the hemostatic system and the inflammatory response during ECMO, resulting in a reduction of UFH bioavailability and anticoagulant effect (12, 13, 21). In neonates, UFH has a variable effect related to the increase in the volume of distribution, reduction of circulating AT levels, and renal function (12, 13). Moreover, heparin has an age-dependent mechanism of action (4, 55–57). As a result, neonates require greater doses of heparin than adults to achieve thrombin inhibition (21). Therefore, UFH response should be closely monitored through aPTT or ACT (13). In addition, despite being rarely described in neonates, heparin may induce immune-mediated thrombocytopenia due to antibodies against the complex platelet factor 4-heparin (12, 58).
Usually, a heparin bolus of 50–100 U/kg is administered at ECMO start, followed by a continuous infusion at 20–50 U/kg/h (2, 59).
Normal levels of AT are required for UFH to work appropriately, as it inhibits factor IIa, factor Xa, and other serine proteases involved in the coagulation pathway. As stated above, neonates and children have physiologically lower AT levels that reach adult values around 3–6 months of age (8, 13, 16). Moreover, circulating levels of AT seem to decrease over time in ECMO (12, 36, 60–63). For these reasons, according to a survey by Bembea et al. in 2013, 51% of the ECMO centers routinely measure and supplement recombinant human antithrombin (rhAT) (53). In a more recent survey conducted in the US, 73% of the centers reported occasional measuring of antithrombin and 51% empirically administering rhAT (52). Extracorporeal Life Support Organization suggests maintaining AT levels between 80 and 120% (64). However, using rhAT in the neonatal population may increase the bleeding risk, as procoagulant factors are physiologically reduced (13, 36, 60).
Research studies on rhAT replacement therapy showed different and controversial results regarding outcomes (mortality, circuit failure, thrombotic, and hemorrhagic events), diagnostic and therapeutic correlations (UFH infusion, ACT levels, blood product use) (60, 61, 65–69). Indeed, the rhAT administration during ECMO is not routine in the neonatal population, and current clinical practice depends on the single-center protocol.
Direct thrombin inhibitors
Direct thrombin inhibitors in ECMO are represented by bivalirudin and argatroban. They have been studied in adult patients, whereas data in newborns and children are scanty and derived from case reports or case series (70–73).
Direct thrombin inhibitors have some advantages compared to UFH, as they can inhibit free and bound thrombin. Indeed, their action is independent of AT, and they do not bind to plasma proteins (12, 36, 74). In addition, a complete thrombin inhibition may reduce coagulation factors consumption, as thrombin-mediated hemostasis activation should be reduced. On the other hand, disadvantages are represented by the lack of inhibition of the contact pathway of the coagulation cascade and their lack of reversibility (12, 36, 74).
Bivalirudin is the most common direct thrombin inhibitor used because of its short half-life (25 min). Still, it has no reversal agent, and its dose must be adjusted in case of renal impairment. Moreover, its dose interval described in the literature is wide (0.05–1.6 mg/kg/h), lacking linearity in the upper side of the therapeutic range when monitored with APTT (4).
However, in recent years, evidence about the use of bivalirudin has been rising in pediatrics. Safety and efficacy have been successfully evaluated in neonatal and pediatric patients as periprocedural anticoagulation or anticoagulation in patients subjected to thrombosis (75–77). Side effects, such as bleeding, were not increased; otherwise, bivalirudin has been associated with more prolonged life of the ECMO circuit and even reduced need for red blood cell transfusions (78, 79). Dosing and monitoring are still a matter of debate. Neonates showed in vitro an increased response to bivalirudin in comparison to older children and adults, with a 2-fold reduction in thrombin generation (80).
To our knowledge, a single study exploring the use of bivalirudin in neonatal ECMO has been published. The authors confirmed its safety for thrombosis and bleeding and found that an increased dose of bivalirudin was necessary to maintain stable anticoagulation as a tolerance or tachyphylaxis mechanism developed. Furthermore, there was no significant correlation between monitored bivalirudin dose with APTT and TEG-R (81).
Antiplatelet agents and hemostatic adjuncts
Antiplatelet agents, such as acetylsalicylic acid, clopidogrel, and dipyridamole, are frequently used in pediatric VAD patients (82). Therefore, they could be considered therapeutic agents in addition to anticoagulants in ECMO, although thrombocytopenia, common in neonatal ECMO, could increase the risk of bleeding. However, further studies are needed to explore their potential role in neonatal and pediatric ECMO (12, 36).
Antifibrinolytic agents, such as tranexamic acid and ε-aminocaproic acid, are reported to reduce intracranial hemorrhage (ICH) and surgical site bleeding (13, 83, 84). Rational use of these agents may derive from the increased fibrinolysis reported in pediatric and neonatal populations compared to adults (21, 36). However, they have also been associated with more frequent circuit changes and thromboembolic complications, precluding their use in pediatric and neonatal ECMO, except before, during, and after surgeries to reduce the risk of bleeding (13, 36, 85–87).
In some case reports and case series, recombinant activated factor VII (rFVIIa) has been reported to decrease bleeding and packed red blood cells (PRBCs) transfusion need, although with an increased risk of thrombosis (13, 36, 88, 89). Therefore, its use in ECMO remains off-label, and it is not recommended in children, though it could be considered in case of refractory bleeding (13, 36, 90).
Prothrombin complex concentrates (PCC) (3 or 4 Factors PCC) could be considered only in cases of life-threatening and unresponsive bleeding, as it has been studied in a few research studies. In contrast, the administration of activated prothrombin complex concentrate (APCC) has been associated with a chance of fatal thrombosis in an adult patient (13, 91).
Transfusional therapy
Blood product transfusions are often administered during neonatal ECMO. The rationale related to the use of blood transfusions products are:
• the need to prime the circuit with PRBCs and fresh frozen plasma (FFP) to avoid hemodilution;
• the need for frequent blood samples to assess coagulation status and to monitor anticoagulation therapy;
• the anemia related to hemolysis;
• bleeding from cannulation and surgical site as a possible complication (92, 93).
Although PRBCs transfusions are common, the established threshold is still a matter of debate, as hemoglobin and hematocrit targets are based primarily on expert opinion (4).
Usually, neonatal and pediatric patients receive 30–105 ml/kg/d of PRBCs (93, 94). Exposure to PRBCs has been associated with increased mortality and morbidity, impacting short- and long-term outcomes (93, 95–99). Indeed, PRBCs may contribute to hemolysis and, in turn, to oxidative stress and thrombotic risk (37, 92, 100). Moreover, oxidative stress is proportional to the volume, and the units of PRBCs transfused (92, 101). In a recent review published in 2018, the authors did not recognize a specific target during ECMO. Still, they suggest that the decision should be based on cardiorespiratory support and oxygen delivery (102).
Platelets and FFP transfusions are performed in high volumes during ECMO, around 25 and 47 ml/kg/d, respectively (92, 103). Their extensive use is driven by the risk of bleeding (104). On the other hand, their use is typically preventive rather than therapeutic, based on blood test alterations and not on evidence of clinical bleeding (105).
Scientific evidence is scarce about indications following laboratory tests for FFP and platelet transfusions (104). The most common thresholds, 80–100 × 109/L for platelets and INR 1.5–1.8 for FFP, are based on expert opinion (4, 92, 104). Depending on the protocol adopted by the single-center, plasma transfusions may also be guided by INR, prothrombin time (PT), aPTT, ACT, antithrombin, TEG R-time, ROTEM A10, and MCF, with a more liberal threshold in case of active bleeding. The laboratory test improvement after FFP or platelet transfusion is mild, both in bleeding and non-bleeding patients (104). In recent years, concern about the safety of platelet transfusion in children and newborns has been rising, as platelet transfusions have been associated with increased mortality (106–109). In conclusion, the transfusional approach is still a matter of debate. Finding a balance between bleeding risk and adverse transfusion effects is challenging, and evidence-based recommendations are lacking.
How can we monitor hemostasis in neonates on ECMO?
To date, there is not one optimal test to monitor hemostasis and titrate anticoagulation therapy in ECMO (4). Monitoring anticoagulation protocols vary across centers, depending on the expertise of the ECMO team (13). Moreover, monitoring anticoagulation is even more challenging in critically ill newborns, as hemostasis is evolving, and reference ranges are lacking for most tests (12).
A survey from Bembea et al. revealed that 97% of the respondent centers used ACT in monitoring anticoagulation, with most centers using a combination of ACT, APTT, anti-Xa, and thromboelastography (TEG) (13, 53). However, a more recent survey revealed an almost universal application of the anti-Xa assay (90% of the respondent centers) with a decrease in ACT use (65%) (52).
Today a single-test monitoring protocol is not advised, given the complexity of the task. Otherwise, the best combination is still unclear, and multiple tests mean lots of iatrogenic blood loss in a critically ill newborn (Table 2) (12).
A disadvantage common to all coagulation assays is the difference in the reagents and coagulation analyzers used in the laboratories, which makes them not well-comparable and standardized (6). As a result, correlations between laboratory test results and complications, such as thrombosis, bleeding, or mortality, have not been found in various studies (54, 110–113).
Activated clotting time
The oldest and best-known test to monitor anticoagulation is ACT, which measures the intrinsic and common pathway of the coagulation cascade (21).
Activated clotting time is a rough but straightforward bedside test performed on whole blood to assess anticoagulation adequacy (14). It measures the time for whole blood to clot when activated by kaolin, celite, or glass beads (12). It is low cost, requires a small sample size (2–3 whole blood drops), is easy to perform, and may be used even during transport (2).
Activated clotting time range targeted is usually between 180 and 220 s, with the expected rate of heparin infusion 20–50 U/kg/h (14, 21). Depending on the ACT result obtained, UFH infusion may be adjusted, and the ACT re-checked in an hour (13).
Activated clotting time remains one of the most common tests used to monitor anticoagulation in ECMO, despite its results being influenced by patient underlying coagulopathy, platelet dysfunction, AT, age, hemodilution, sample size, and temperature (21). Due to all these influencing factors, reproducibility is scarce, especially in neonates where hemostasis is continuously developing (14).
Another disadvantage of ACT is its accuracy, which decreases with the ongoing time of ECMO. However, its reliability improves when adequate clotting factor levels are maintained during the procedure (21).
To note, if the ACT is out of range with an adequate heparin infusion rate, other factors influencing hemostasis must be searched with other tests. Activated clotting time values correlate poorly with heparin levels, anti-Xa, or APTT (4, 14, 21). Activated clotting time is the test with the lowest correlation with heparin dose and is the least affected by changes in heparin doses compared to anti-Xa and APTT, especially in low heparin doses as in ECMO (113–116). In addition, a weak correlation has been found between ACT and platelet count, with the highest ACT values when the platelet count is below 100.000/mmc (111, 113).
Other ACT tests such as the i-STAT ACT are now available. In addition, ACT derived from POC instruments such as the TEG with the TF activation may be a good test for the future, despite its use not being universally spread yet (21).
In summary, the ACT is an excellent crude method to obtain immediate information about the anticoagulation of the circuit. Still, it is insufficient to monitor anticoagulation in ECMO correctly, so other tests should be considered for addiction (Table 2) (21).
Anti-factor Xa
The anti-factor Xa test is now the most popular test for UFH and low molecular weight heparin (LMWH) monitoring in many ECMO centers (14, 52). It measures the inhibition of factor Xa by heparin in plasma, detecting the rate of factor Xa inactivation by the heparin/antithrombin complexes (12, 110, 113, 117).
Unlike the ACT, which is influenced by all the other factors with a potential impact on coagulation, anti-Xa levels measure the heparin effect or concentration and the inhibition of FX conversion. This explains the weak correlation between anti-Xa levels and ACT in adult and pediatric/neonatal ECMO (4, 111). However, it has been found to have the strongest correlation with heparin dose compared to ACT, APTT, TEG, and AT levels (111, 113, 114). Otherwise, it is not affected by other parameters with hemostatic impacts, such as FVIII or fibrinogen levels (113).
The anti-factor Xa test can be performed in the absence or presence of AT. The first case measures the heparin effect, while the second measures the heparin concentration. The effective range is between 0.3 and 1.1 IU/ml, depending on the single-center, with the most common goal range used 0.3–0.7 IU/ml (2, 14, 52). In neonates, as they have low levels of AT, an anti-Xa assay in the absence of AT is more representative of what happens in vivo, otherwise may overestimate heparin activity and mask AT deficiency (12, 52).
To note, anti-Xa has poor reliability in patients with hyperbilirubinemia, increased plasma-free hemoglobin levels, and hypertriglyceridemia and is influenced by AT levels and assay type (2, 4). Other disadvantages are the training required to be performed, its high costs, and the time to run the test (114). Moreover, it has no role in evaluating thrombin generation, so it should be integrated with other tests (Table 2) (12).
Anti-Xa-based monitoring of UFH protocols showed that higher doses of heparin are needed in infants compared to older children to obtain therapeutic anti-Xa levels (52, 110). In addition, a more stable heparin dosing, with few “out of range” results, without increased complications, has been found with anti-Xa-based protocol compared to ACT-based protocol (118).
Activated partial thromboplastin time and prothrombin time
Activated partial thromboplastin time is widely recognized as a test to monitor heparin therapy in adults, even if it is poorly reliable in the acute management of ECMO (14, 21, 117).
Activated partial thromboplastin time represents the clotting time of recalcified, platelet-poor citrated plasma when activated by an intrinsic pathway activator (12, 14). Different methods and analyzers are used to determine APTT, influencing the sensitivity to heparin, and the reference ranges to use, making the test less comparable across centers (13).
Prothrombin time and activated partial thromboplastin time are often prolonged in newborns, regardless of ECMO. Therefore, they are unreliable for studying in vivo hemostasis in neonatal age (16, 18). In addition, they have low prediction capability in clinically significant bleeding (19, 22, 23). The APTT response to heparin also varies with age, with the more substantial prolongation of APTT in younger children exposed to the same heparin amounts as the older ones (4, 56, 57). Poor correlation between APTT and anti-Xa has been reported, too (119, 120). In other studies, the correlation between APTT and anti-Xa was moderate, but APTT often overestimated heparin activity (110).
Moreover, APTT is influenced by hyperbilirubinemia, hyperlipidemia, anti-phospholipid antibodies, and increased C reactive protein (12).
In adults, APTT ratio values 1.5–2.5 times above baseline moderately correlate with a heparin concentration, preventing thrombus formation (14, 21).
In summary, APTT may be used in monitoring anticoagulation in adults because it correlates well with anti-factor Xa levels, less so in children and neonates (Table 2) (14).
Prothrombin time is a citrate plasma clotting time that investigates the clotting factors of the extrinsic and common pathway of the coagulation cascade through factor VII activation by TF and phospholipid. Prothrombin time has a strong correlation with coagulation factors levels but minimal correlation with heparin, AT, and factor XII (113). It is the most specific test for changes in clotting factors of the extrinsic and common pathways (113). It may be helpful in ECMO as it may detect the need for clotting factors supplementation in case of bleeding with normal heparin activity (Table 2).
Thromboelastography and rotational thromboelastometry
The use of viscoelastic coagulation tests (VCTs), such as thromboelastography (TEG) and rotational thromboelastometry (ROTEM), is widespread for anticoagulation monitoring in ECMO (Table 2). In a recent survey conducted in the US, 41% of the centers reported using a viscoelastic test to monitor anticoagulation (52).
They have the advantage of dynamically examining the clotting process, from detecting the first fibrin filaments to the clot lysis (21). Moreover, VCTs provide information about the coagulation factors, platelet functions and number, platelet-fibrin interaction, fibrinogen levels and activity, and fibrinolysis (14). Like ACT, they are bedside techniques, making the result available in real-time (12).
Viscoelastic coagulation tests are represented as a flat line corresponding to the liquid phase of the whole blood. Then, the line diverges into two lines with increasing amplitude until the maximum clot firmness is reached. Finally, the two lines converge simultaneously with the clot lysis (Figure 1) (121). All these steps are described by the different TEG/ROTEM parameters. They may indicate which aspect of the hemostatic process is disrupted, with a possible therapeutic approach to correct the coagulopathy.
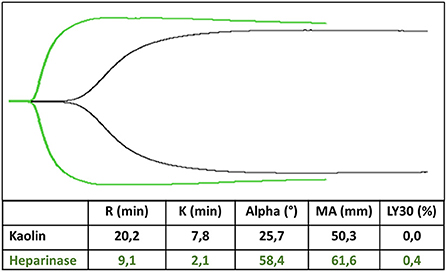
Figure 1. TEG trace in kaolin (black trace) and heparinase (green trace). R-time is prolonged 2–3-fold in kaolin compared to heparinase, whereas MA is unaffected by heparin.
Thromboelastography reaction time (R)/ROTEM Clot formation Time (CT) reflects the time from the test beginning to the first detection of fibrin filaments. The alpha angle and coagulation (K) time describe how fast the clot grows and depends on clotting factors, platelets, and fibrinogen levels. TEG Maximal Amplitude (MA)/ROTEM Maximal Clot Firmness (MCF) represents the platelets and fibrinogen activity. TEG Lysis (LY) 30/ROTEM Clot Lysis Index (CLI) 30 describes the fibrinolysis 30 min after MA/MCF (121, 122).
Rotational thromboelastometry and thromboelastography provide the same information, even with different parameter names, although the results are not interchangeable as they use different methodology and laboratory assays (123).
Thromboelastography can be performed with whole fresh blood that must be analyzed within 4–6 min, whereas if citrated blood is used, the test can be delayed for a few hours, albeit with slightly different results (121).
Activators are frequently utilized in VCTs to faster the process. Kaolin is the most used with TEG; its ROTEM equivalent is the INTEM, which uses ellagic acid and phospholipids to explore the contact-dependent coagulation pathway. Rotational thromboelastometry (ROTEM) EXTEM uses TF to analyze the extrinsic coagulation pathway. At the same time, rapid TEG activates coagulation through a combination of TF and kaolin to allow faster assessment of the hemostatic status (121, 124). Adding heparinase (Heparinase in TEG, HEPTEM in ROTEM) allows a heparin-free curve in patients subjected to anticoagulation with UFH (21, 121). Moreover, the functional fibrinogen test (FLEV-TEG in TEG, FIBTEM in ROTEM) allows for measuring the amount of fibrinogen that contributes to the clot strength. They block the platelet contribution to the MA or MCF parameter by TF and abciximab, a GPIIb/IIIa inhibitor (FLEV-TEG), and TF and cytochalasin D, an actin polymerization inhibitor (FIBTEM) (Figure 2). Their use permits discrimination between the deficit of fibrinogen and the deficit of platelets and decides which one must be administered to the patient (125–130).
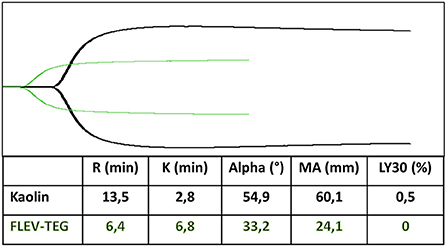
Figure 2. Functional fibrinogen test (FLEV-TEG) (green trace) shows the platelet contribution to MA parameter compared to kaolin test (black trace).
Viscoelastic coagulation tests are successfully used in adult critical care settings to guide transfusion practice, improving outcomes (121, 131, 132). In NICU, TEG and ROTEM are attractive owing to the peculiarity of the hemostatic system of the newborn, especially preterm, to assess the coagulation status and potential to drive FFP or platelets administration (19, 22, 92, 124, 133–144).
Reaction and clot formation (R and CT) time are the most important parameter when using TEG or ROTEM in ECMO, as it refers to the time needed to obtain the first fibrin filaments (Figure 1). They are influenced mainly by clotting factors and by treatment with heparin of vitamin K antagonists (121).
Therefore, anticoagulation management is usually based on R or CT time, comparing the values obtained with or without heparinase (123).
In our experience, the best way to monitor anticoagulation with TEG is to perform a heparinase TEG and a kaolin TEG simultaneously, with R time in kaolin 2–3 fold longer than R time in heparinase (R time in kaolin 15–25 min) (2, 21). The ratio between R time in kaolin and R time in heparinase drives the need to increase or decrease heparin infusion (2). Maximal amplitude parameter, which describes the maximum amplitude of the TEG trace, is a measure of platelets and fibrinogen concentration and function and may have a role in determining the need for fibrinogen or platelets administration when the functional fibrinogen test is performed (2). The same tests may be performed with ROTEM.
Studies have demonstrated a weak correlation between TEG and APTT/ACT and a strong correlation between MA and platelet count (145). In addition, qualitative platelet dysfunction during ECMO has been demonstrated in a retrospective pediatric study using Platelet-Mapping TEG. Still, the correlation between this alteration and bleeding risk is yet to be determined (146).
In a retrospective study, a reduced MCF of INTEM, EXTEM, and INTEM with heparinase showed a correlation with increased thrombotic risk. Coagulation factors and platelet consumption may explain this finding during ECMO. No correlation was found between ROTEM parameters and bleeding complications (128).
Clot formation time on INTEM and HEPTEM was found to have a moderate-strong correlation with aPTT and HaPTT for children receiving bivalirudin for anticoagulation (123).
In adults, the use of POC tests to monitor anticoagulation has been demonstrated to provide reliable and timely information about the risk of bleeding, with a good correlation with traditional coagulation tests (147). They reflect the patient's hemostatic status in mechanical circulatory support, monitor the anticoagulation and anti-aggregation therapy, and their use reduces the risk of thromboembolic and bleeding complications (15). A retrospective study on neonates affected by congenital diaphragmatic hernia revealed improved goal-directed blood product transfusions and reduced bleeding complications, especially hemothorax requiring chest tube placement or thoracotomy (148).
Further studies to standardize TEG and ROTEM are required, as reference ranges for neonatal and pediatric populations are not well-established, as well as optimal target values for ECMO patients (52, 59, 122).
Future perspectives and conclusion
Due to the complexity of the hemostatic system of the newborn and the impact of extracorporeal circulation on the hemostatic balance, anticoagulation management is a real challenge during neonatal ECMO.
Unfractionated heparin remains the mainstay of treatment, but other agents, such as antiplatelets and direct thrombin inhibitors, are of great interest and could be considered in the future (12, 36). In addition, newer anticoagulant agents, such as factor XIa and XIIa inhibitors, are now in the early stage of research studies. They might be promising in the future, as they can uncouple the antithrombotic effect from the anti-hemostatic effect (149–151).
The best strategy to monitor and titrate anticoagulation is still unknown, but in recent years, different and more reliable tests have substituted the traditional ACT and APTT. A combination of multiple techniques is probably the best option, as they explore various aspects of the coagulation status. In our experience at the neonatal ECMO center, TEG may provide helpful real-time information to drive the heparin infusion and the need for blood product transfusions, besides other tests. Of course, further studies are needed to clarify the role of every single test in the overall management and the best combination to achieve a safe balance between hemostasis and thrombosis during ECMO to obtain a shared protocol across the centers.
Author contributions
VC, GR, GSA, IA, SGu, FMa, GCe, AT, MC, AA, SGh, and GCa contributed to the study's conception and design. VC, GR, GSA, IA, SGu, FMa, SGh, and GCa contributed to the study's methodology, investigation, and data curation. VC, GR, GSA, IA, SGu, FMa, SGh, and GC wrote the original draft preparation of the manuscript. VC and GR contributed equally to this work and share the first authorship, having the right to list their name as first in their Curriculum Vitae. GCe, AT, MC, AA, SGh, GCa, and FMo provided extensive critical revision. All authors contributed to the manuscript's critical revision, read and approved the submitted version.
Funding
This study was (partially) funded by the Italian Ministry of Health—Current research IRCCS.
Conflict of interest
The authors declare that the research was conducted in the absence of any commercial or financial relationships that could be construed as a potential conflict of interest.
Publisher's note
All claims expressed in this article are solely those of the authors and do not necessarily represent those of their affiliated organizations, or those of the publisher, the editors and the reviewers. Any product that may be evaluated in this article, or claim that may be made by its manufacturer, is not guaranteed or endorsed by the publisher.
Abbreviations
ACT, activated clotting time; APTT, activated partial thromboplastin time; AT, antithrombin; CFT, clot formation time; CHD, congenital heart disease; CLI, clot lysis index; ECMO, extracorporeal membrane oxygenation; ECPR, extracorporeal cardiopulmonary resuscitation; ELSO, Extracorporeal Life Support Organization; FFP, fresh frozen plasma; ICH, intracranial hemorrhage K time, coagulation time; LMWH, low molecular weight heparin; LY, clot lysis; MA, maximal amplitude; MCF, maximal clot firmness; MCV, mean corpuscular volume; NICU, neonatal intensive care unit; PMEA, poly-2-methoxy-ethyl acrylate; PRBCs, packed red blood cells; PT, prothrombin time; RBCs, red blood cells; ROTEM, rotational thromboelastometry; R time, reaction time; TEG, thromboelastography; TF, tissue factor; UFH, un-fractioned heparin; US, United States; VAD, ventricular assist device; VCT, viscoelastic coagulation test; vWf, von Willebrand factor.
References
1. Murphy DA, Hockings LE, Andrews RK, Aubron C, Gardiner EE, Pellegrino VA, et al. Extracorporeal membrane oxygenation-hemostatic complications. Transfus Med Rev. (2015) 29:90–101. doi: 10.1016/j.tmrv.2014.12.001
2. Amodeo I, Di Nardo M, Raffaeli G, Kamel S, Macchini F, Amodeo A, et al. Neonatal respiratory and cardiac ECMO in Europe. Eur J Pediatr. (2021) 180:1675–92. doi: 10.1007/s00431-020-03898-9
3. ELSO. ELSO Registry Report. European Summary, April 2022. ELSO (2022). Retrieved from: https://www.elso.org (June 11, 2022).
4. Sniderman J, Monagle P, Annich GM, MacLaren G. Hematologic concerns in extracorporeal membrane oxygenation. Res Pract Thromb Haemost. (2020) 4:455–68. doi: 10.1002/rth2.12346
5. ELSO. (2022, April 1). ELSO Registry Report. International Summary, April 2022. ELSO (2022). Retrieved from: https://www.elso.org (June 11, 2022) [Internet].
6. Kamdar A, Rintoul N, Raffini L. Anticoagulation in neonatal ECMO. Semin Perinatol. (2018) 42:122–8. doi: 10.1053/j.semperi.2017.12.008
7. Andrew M, Paes B, Milner R, Johnston M, Mitchell L, Tollefsen DM, et al. Development of the human coagulation system in the healthy premature infant. Blood. (1988) 72:1651–7. doi: 10.1182/blood.V72.5.1651.bloodjournal7251651
8. Andrew M, Paes B, Milner R, Johnston M, Mitchell L, Tollefsen DM, et al. Development of the human coagulation system in the full-term infant. Blood. (1987) 70:165–72. doi: 10.1182/blood.V70.1.165.165
9. Esmon CT. The interactions between inflammation and coagulation. Br J Haematol. (2005) 131:417–30. doi: 10.1111/j.1365-2141.2005.05753.x
10. Foley JH, Conway EM. Cross talk pathways between coagulation and inflammation. Circ Res. (2016) 118:1392–408. doi: 10.1161/CIRCRESAHA.116.306853
11. Eaton MP, Iannoli EM. Coagulation considerations for infants and children undergoing cardiopulmonary bypass. Paediatr Anaesth. (2011) 21:31–42. doi: 10.1111/j.1460-9592.2010.03467.x
12. Cashen K, Meert K, Dalton H. Anticoagulation in neonatal ECMO: an enigma despite a lot of effort! Front Pediatr. (2019). 7:366. doi: 10.3389/fped.2019.00366
13. Barton R, Ignjatovic V, Monagle P. Anticoagulation during ECMO in neonatal and paediatric patients. Thromb Res. (2019) 173:172–7. doi: 10.1016/j.thromres.2018.05.009
14. Annich GM. Extracorporeal life support: the precarious balance of hemostasis. J Thromb Haemost. (2015) 13(Suppl 1):S336–42. doi: 10.1111/jth.12963
15. Gorlinger K, Bergmann L, Dirkmann D. Coagulation management in patients undergoing mechanical circulatory support. Best Pract Res Clin Anaesthesiol. (2012) 26:179–98. doi: 10.1016/j.bpa.2012.04.003
16. Monagle P, Barnes C, Ignjatovic V, Furmedge J, Newall F, Chan A, et al. Developmental haemostasis. Impact for clinical haemostasis laboratories. Thromb Haemost. (2006) 95:362–72. doi: 10.1160/TH05-01-0047
17. Andrew M, Vegh P, Johnston M, Bowker J, Ofosu F, Mitchell L. Maturation of the hemostatic system during childhood. Blood. (1992) 80:1998–2005. doi: 10.1182/blood.V80.8.1998.bloodjournal8081998
18. Toulon P. Developmental hemostasis: laboratory and clinical implications. Int J Lab Hematol. (2016) 38(Suppl 1):66–77. doi: 10.1111/ijlh.12531
19. Tripodi A, Chantarangkul V, Mannucci PM. Acquired coagulation disorders: revisited using global coagulation/anticoagulation testing. Br J Haematol. (2009) 147:77–82. doi: 10.1111/j.1365-2141.2009.07833.x
20. Christensen RD, Baer VL, Lambert DK, Henry E, Ilstrup SJ, Bennett ST. Reference intervals for common coagulation tests of preterm infants (CME). Transfusion. (2014) 54:627–32:quiz 6. doi: 10.1111/trf.12322
21. Oliver WC. Anticoagulation coagulation management for ECMO. Semin Cardiothorac Vasc Anesth. (2009). 13:154–75. doi: 10.1177/1089253209347384
22. Tripodi A, Ramenghi LA, Chantarangkul V, De Carli A, Clerici M, Groppo M, et al. Normal thrombin generation in neonates in spite of prolonged conventional coagulation tests. Haematologica. (2008) 93:1256–9. doi: 10.3324/haematol.12566
23. Raffaeli G, Tripodi A, Cavallaro G, Cortesi V, Scalambrino E, Pesenti N, et al. Thromboelastographic profiles of healthy very low birthweight infants serially during their first month. Arch Dis Child Fetal Neonatal Ed. (2020) 105:412–8. doi: 10.1136/archdischild-2019-317860
24. Tripodi A, Raffaeli G, Scalambrino E, Padovan L, Clerici M, Chantarangkul V, et al. Procoagulant imbalance in preterm neonates detected by thrombin generation procedures. Thromb Res. (2020) 185:96–101. doi: 10.1016/j.thromres.2019.11.013
25. Strauss T, Sidlik-Muskatel R, Kenet G. Developmental hemostasis: primary hemostasis and evaluation of platelet function in neonates. Semin Fetal Neonatal Med. (2011) 16:301–4. doi: 10.1016/j.siny.2011.07.001
26. Bednarek FJ, Bean S, Barnard MR, Frelinger AL, Michelson AD. The platelet hyporeactivity of extremely low birth weight neonates is age-dependent. Thromb Res. (2009) 124:42–5. doi: 10.1016/j.thromres.2008.10.004
27. Deschmann E, Sola-Visner M, Saxonhouse MA. Primary hemostasis in neonates with thrombocytopenia. J Pediatr. (2014) 164:167–72. doi: 10.1016/j.jpeds.2013.08.037
28. Del Vecchio A, Latini G, Henry E, Christensen RD. Template bleeding times of 240 neonates born at 24 to 41 weeks gestation. J Perinatol. (2008) 28:427–31. doi: 10.1038/jp.2008.10
29. Valsami S, Kollia M, Mougiou V, Sokou R, Isaakidou E, Boutsikou M, et al. Evaluation of PFA-100 closure times in cord blood samples of healthy term and preterm neonates. Clin Chem Lab Med. (2020) 58:e113–6. doi: 10.1515/cclm-2019-0948
30. Katz JA, Moake JL, McPherson PD, Weinstein MJ, Moise KJ, Carpenter RJ, et al. Relationship between human development and disappearance of unusually large von Willebrand factor multimers from plasma. Blood. (1989) 73:1851–8. doi: 10.1182/blood.V73.7.1851.bloodjournal7371851
32. Griesman J, Karahalios D, Prendergast C. Hematologic changes in cyanotic congenital heart disease: a review. Prog Pediatr Cardiol. (2020) 56:101193. doi: 10.1016/j.ppedcard.2020.101193
33. Mukai N, Nakayama Y, Murakami S, Tanahashi T, Sessler DI, Ishii S, et al. Potential contribution of erythrocyte microRNA to secondary erythrocytosis and thrombocytopenia in congenital heart disease. Pediatr Res. (2018) 83:866–73. doi: 10.1038/pr.2017.327
34. Lill MC, Perloff JK, Child JS. Pathogenesis of thrombocytopenia in cyanotic congenital heart disease. Am J Cardiol. (2006) 98:254–8. doi: 10.1016/j.amjcard.2006.01.083
35. Cashen K, Meert K, Dalton HJ. Platelet count and function during pediatric extracorporeal membrane oxygenation. Semin Thromb Hemost. (2020) 46:357–65. doi: 10.1055/s-0040-1708542
36. Saini A, Spinella PC. Management of anticoagulation and hemostasis for pediatric extracorporeal membrane oxygenation. Clin Lab Med. (2014) 34:655–73. doi: 10.1016/j.cll.2014.06.014
37. Raffaeli G, Ghirardello S, Passera S, Mosca F, Cavallaro G. Oxidative stress and neonatal respiratory extracorporeal membrane oxygenation. Front Physiol. (2018) 9:1739. doi: 10.3389/fphys.2018.01739
38. Hundalani SG, Nguyen KT, Soundar E, Kostousov V, Bomgaars L, Moise A, et al. Age-based difference in activation markers of coagulation and fibrinolysis in extracorporeal membrane oxygenation. Pediatr Crit Care Med. (2014) 15:e198–205. doi: 10.1097/PCC.0000000000000107
39. Wiedmeier SE, Henry E, Sola-Visner MC, Christensen RD. Platelet reference ranges for neonates, defined using data from over 47,000 patients in a multihospital healthcare system. J Perinatol. (2009) 29:130–6. doi: 10.1038/jp.2008.141
40. Italiano Jr JE, Mairuhu AT, Flaumenhaft R. Clinical relevance of microparticles from platelets and megakaryocytes. Curr Opin Hematol. (2010) 17:578–84. doi: 10.1097/MOH.0b013e32833e77ee
41. Meyer AD, Gelfond JA, Wiles AA, Freishtat RJ, Rais-Bahrami K. Platelet-derived microparticles generated by neonatal extracorporeal membrane oxygenation systems. ASAIO J. (2015) 61:37–42. doi: 10.1097/MAT.0000000000000164
42. Cheung PY, Sawicki G, Salas E, Etches PC, Schulz R, Radomski MW. The mechanisms of platelet dysfunction during extracorporeal membrane oxygenation in critically ill neonates. Crit Care Med. (2000) 28:2584–90. doi: 10.1097/00003246-200007000-00067
43. Chung A, Wildhirt SM, Wang S, Koshal A, Radomski MW. Combined administration of nitric oxide gas and iloprost during cardiopulmonary bypass reduces platelet dysfunction: a pilot clinical study. J Thorac Cardiovasc Surg. (2005) 129:782–90. doi: 10.1016/j.jtcvs.2004.06.049
44. Wesley MC, McGowan FX, Castro RA, Dissanayake S, Zurakowski D, Dinardo JA. The effect of milrinone on platelet activation as determined by TEG platelet mapping. Anesth Analg. (2009) 108:1425–9. doi: 10.1213/ane.0b013e3181981fbe
45. Kubicki R, Stiller B, Kroll J, Siepe M, Beyersdorf F, Benk C, et al. Acquired von Willebrand syndrome in paediatric patients during mechanical circulatory support. Eur J Cardiothorac Surg. (2019) 55:1194–201. doi: 10.1093/ejcts/ezy408
46. Schlagenhauf A, Zieger B, Muntean W. Contact activation and acquired von Willebrand syndrome during neonatal extracorporeal circulation. J Thromb Haemost. (2020) 18:3119–21. doi: 10.1111/jth.15058
47. Ruth A, Meador M, Hui R, Loftis L, Teruya J. Acquired von Willebrand syndrome in pediatric extracorporeal membrane oxygenation patients: a single institution's experience. Pediatr Crit Care Med. (2019) 20:980–5. doi: 10.1097/PCC.0000000000002009
48. Pasala S, Fiser RT, Stine KC, Swearingen CJ, Prodhan P. von Willebrand factor multimers in pediatric extracorporeal membrane oxygenation support. ASAIO J. (2014) 60:419–23. doi: 10.1097/MAT.0000000000000084
49. Dalton HJ, Cashen K, Reeder RW, Berg RA, Shanley TP, Newth CJL, et al. Hemolysis during pediatric extracorporeal membrane oxygenation: associations with circuitry, complications, and mortality. Pediatr Crit Care Med. (2018) 19:1067–76. doi: 10.1097/PCC.0000000000001709
50. Lou S, MacLaren G, Best D, Delzoppo C, Butt W. Hemolysis in pediatric patients receiving centrifugal-pump extracorporeal membrane oxygenation: prevalence, risk factors, and outcomes. Crit Care Med. (2014) 42:1213–20. doi: 10.1097/CCM.0000000000000128
51. Dalton HJ, Reeder R, Garcia-Filion P, Holubkov R, Berg RA, Zuppa A, et al. Factors associated with bleeding and thrombosis in children receiving extracorporeal membrane oxygenation. Am J Respir Crit Care Med. (2017) 196:762–71. doi: 10.1164/rccm.201609-1945OC
52. Ozment CP, Scott BL, Bembea MM, Spinella PC, Pediatric ECMO (PediECMO) subgroup of the Pediatric Acute Lung Injury and Sepsis Investigators (PALISI) Network and the Extracorporeal Life Support Organization (ELSO). Anticoagulation and transfusion management during neonatal and pediatric extracorporeal membrane oxygenation: a survey of medical directors in the United States. Pediatr Crit Care Med. (2021) 22:530–41. doi: 10.1097/PCC.0000000000002696
53. Bembea MM, Annich G, Rycus P, Oldenburg G, Berkowitz I, Pronovost P. Variability in anticoagulation management of patients on extracorporeal membrane oxygenation: an international survey. Pediatr Crit Care Med. (2013) 14:e77–84. doi: 10.1097/PCC.0b013e31827127e4
54. Baird CW, Zurakowski D, Robinson B, Gandhi S, Burdis-Koch L, Tamblyn J, et al. Anticoagulation and pediatric extracorporeal membrane oxygenation: impact of activated clotting time and heparin dose on survival. Ann Thorac Surg. (2007) 83:912–9; discussion 9–20. doi: 10.1016/j.athoracsur.2006.09.054
55. Newall F, Ignjatovic V, Summerhayes R, Gan A, Butt W, Johnston L, et al. In vivo age dependency of unfractionated heparin in infants and children. Thromb Res. (2009) 123:710–4. doi: 10.1016/j.thromres.2008.07.009
56. Newall F, Johnston L, Ignjatovic V, Monagle P. Unfractionated heparin therapy in infants and children. Pediatrics. (2009) 123:e510–8. doi: 10.1542/peds.2008-2052
57. Ignjatovic V, Furmedge J, Newall F, Chan A, Berry L, Fong C, et al. Age-related differences in heparin response. Thromb Res. (2006) 118:741–5. doi: 10.1016/j.thromres.2005.11.004
58. Avila ML, Shah V, Brandao LR. Systematic review on heparin-induced thrombocytopenia in children: a call to action. J Thromb Haemost. (2013) 11:660–9. doi: 10.1111/jth.12153
59. Baumann Kreuziger L, Patricia Massicotte M. Mechanical circulatory support: balancing bleeding and clotting in high-risk patients. Hematol Am Soc Hematol Educ Progr. (2015) 2015:61–8. doi: 10.1182/asheducation-2015.1.61
60. Todd Tzanetos DR, Myers J, Wells T, Stewart D, Fanning JJ, Sullivan JE. The use of recombinant antithrombin III in pediatric and neonatal ECMO patients. ASAIO J. (2017) 63:93–8. doi: 10.1097/MAT.0000000000000476
61. Niebler RA, Christensen M, Berens R, Wellner H, Mikhailov T, Tweddell JS. Antithrombin replacement during extracorporeal membrane oxygenation. Artif Organs. (2011) 35:1024–8. doi: 10.1111/j.1525-1594.2011.01384.x
62. Perry R, Stein J, Young G, Ramanathan R, Seri I, Klee L, et al. Antithrombin III administration in neonates with congenital diaphragmatic hernia during the first three days of extracorporeal membrane oxygenation. J Pediatr Surg. (2013) 48:1837–42. doi: 10.1016/j.jpedsurg.2012.11.037
63. Stansfield BK, Wise L, Ham PB III, Patel P, Parman M, Jin C, et al. Outcomes following routine antithrombin III replacement during neonatal extracorporeal membrane oxygenation. J Pediatr Surg. (2017) 52:609–13. doi: 10.1016/j.jpedsurg.2016.10.047
64. Wild KT, Rintoul N, Kattan J, Gray B. Extracorporeal Life Support Organization (ELSO): guidelines for neonatal respiratory failure. ASAIO J. (2020) 66:463–70. doi: 10.1097/MAT.0000000000001153
65. Wong TE, Nguyen T, Shah SS, Brogan TV, Witmer CM. Antithrombin concentrate use in pediatric extracorporeal membrane oxygenation: a multicenter cohort study. Pediatr Crit Care Med. (2016) 17:1170–8. doi: 10.1097/PCC.0000000000000955
66. Wong TE, Delaney M, Gernsheimer T, Matthews DC, Brogan TV, Mazor R, et al. Antithrombin concentrates use in children on extracorporeal membrane oxygenation: a retrospective cohort study. Pediatr Crit Care Med. (2015) 16:264–9. doi: 10.1097/PCC.0000000000000322
67. Wong TE, Huang YS, Weiser J, Brogan TV, Shah SS, Witmer CM. Antithrombin concentrate use in children: a multicenter cohort study. J Pediatr. (2013) 163:1329.e1–34.e1. doi: 10.1016/j.jpeds.2013.06.036
68. Byrnes JW, Swearingen CJ, Prodhan P, Fiser R, Dyamenahalli U. Antithrombin III supplementation on extracorporeal membrane oxygenation: impact on heparin dose and circuit life. ASAIO J. (2014) 60:57–62. doi: 10.1097/MAT.0000000000000010
69. Ryerson LM, Bruce AK, Lequier L, Kuhle S, Massicotte MP, Bauman ME. Administration of antithrombin concentrate in infants and children on extracorporeal life support improves anticoagulation efficacy. ASAIO J. (2014) 60:559–63. doi: 10.1097/MAT.0000000000000099
70. Ranucci M, Ballotta A, Kandil H, Isgrò G, Carlucci C, Baryshnikova E, et al. Bivalirudin-based versus conventional heparin anticoagulation for postcardiotomy extracorporeal membrane oxygenation. Crit Care. (2011) 15:R275. doi: 10.1186/cc10556
71. Hursting MJ Dubb J, Verme-Gibboney CN. Argatroban anticoagulation in pediatric patients: a literature analysis. J Pediatr Hematol Oncol. (2006) 1:4–10. doi: 10.1097/01.mph.0000195296.48319.38
72. Scott LK, Grier LR, Conrad SA. Heparin-induced thrombocytopenia in a pediatric patient receiving extracorporeal membrane oxygenation managed with argatroban. Pediatr Crit Care Med. (2006) 7:473–5. doi: 10.1097/01.PCC.0000231946.88688.07
73. Young G, Boshkov LK, Sullivan JE, Raffini LJ, Cox DS, Boyle DA, et al. Argatroban therapy in pediatric patients requiring nonheparin anticoagulation: an open-label, safety, efficacy, and pharmacokinetic study. Pediatr Blood Cancer. (2011) 56:1103–9. doi: 10.1002/pbc.22852
74. Weitz JI, Crowther M. Direct thrombin inhibitors. Thromb Res. (2002) 106:V275–84. doi: 10.1016/S0049-3848(02)00093-2
75. O'Brien SH, Yee DL, Lira J, Goldenberg NA, Young G. UNBLOCK: an open-label, dose-finding, pharmacokinetic and safety study of bivalirudin in children with deep vein thrombosis. J Thromb Haemost. (2015). 13:1615–22. doi: 10.1111/jth.13057
76. Forbes TJ, Hijazi ZM, Young G, Ringewald JM, Aquino PM, Vincent RN, et al. Pediatric catheterization laboratory anticoagulation with bivalirudin. Catheter Cardiovasc Interv. (2011) 77:671–9. doi: 10.1002/ccd.22817
77. Buck ML. Bivalirudin as an alternative to heparin for anticoagulation in infants and children. J Pediatr Pharmacol Ther. (2015) 20:408–17. doi: 10.5863/1551-6776-20.6.408
78. Schill MR, Douds MT, Burns EL, Lahart MA, Said AS, Abarbanell AM. Is anticoagulation with bivalirudin comparable to heparin for pediatric extracorporeal life support? Results from a high-volume center. Artif Organs. (2021) 45:15–21. doi: 10.1111/aor.13758
79. Machado DS, Garvan C, Philip J, Harrington D, Spiess B, Kelly B, et al. Bivalirudin may reduce the need for red blood cell transfusion in pediatric cardiac patients on extracorporeal membrane oxygenation. ASAIO J. (2021) 67:688–96. doi: 10.1097/MAT.0000000000001291
80. Letunica N, Busuttil-Crellin X, Cowley J, Monagle P, Ignjatovic V. Age-specific differences in the in vitro anticoagulant effect of Bivalirudin in healthy neonates and children compared to adults. Thromb Res. (2020). 192:167–73. doi: 10.1016/j.thromres.2020.05.020
81. Snyder CW, Goldenberg NA, Nguyen ATH, Smithers CJ, Kays DW. A perioperative bivalirudin anticoagulation protocol for neonates with congenital diaphragmatic hernia on extracorporeal membrane oxygenation. Thromb Res. (2020) 193:198–203. doi: 10.1016/j.thromres.2020.07.043
82. Fraser Jr CD, Jaquiss RD, Rosenthal DN, Humpl T, Canter CE, Blackstone EH, et al. Prospective trial of a pediatric ventricular assist device. N Engl J Med. (2012) 367:532–41. doi: 10.1056/NEJMoa1014164
83. Horwitz JR, Cofer BR, Warner BW, Cheu HW, Lally KP. A multicenter trial of 6-aminocaproic acid (Amicar) in the prevention of bleeding in infants on ECMO. J Pediatr Surg. (1998) 33:1610–3. doi: 10.1016/S0022-3468(98)90591-7
84. Downard CD, Betit P, Chang RW, Garza JJ, Arnold JH, Wilson JM. Impact of amicar on hemorrhagic complications of ECMO: a ten-year review. J Pediatr Surg. (2003) 38:1212–6. doi: 10.1016/S0022-3468(03)00270-7
85. Basta MN, Stricker PA, Taylor JA. A systematic review of the use of antifibrinolytic agents in pediatric surgery and implications for craniofacial use. Pediatr Surg Int. (2012) 28:1059–69. doi: 10.1007/s00383-012-3167-6
86. Siemens K, Sangaran DP, Hunt BJ, Murdoch IA, Tibby SM. Antifibrinolytic drugs for the prevention of bleeding in pediatric cardiac surgery on cardiopulmonary bypass: a systematic review and meta-analysis. Anesth Analg. (2022) 134:987–1001. doi: 10.1213/ANE.0000000000005760
87. Wong JJ-M, Lam JCM, Mok YH, Lee JH. Anticoagulation in extracorporeal membrane oxygenation. J Emerg Crit Care Med. (2018) 2:12. doi: 10.21037/jeccm.2018.01.12
88. Long MT, Wagner D, Maslach-Hubbard A, Pasko DA, Baldridge P, Annich GM. Safety and efficacy of recombinant activated factor VII for refractory hemorrhage in pediatric patients on extracorporeal membrane oxygenation: a single center review. Perfusion. (2014) 29:163–70. doi: 10.1177/0267659113499782
89. Niebler RA, Punzalan RC, Marchan M, Lankiewicz MW. Activated recombinant factor VII for refractory bleeding during extracorporeal membrane oxygenation. Pediatr Crit Care Med. (2010) 11:98–102. doi: 10.1097/PCC.0b013e3181b0620b
90. ELSO Guidelines General for All Cardiopulmonary Extracorporeal Life Support Version 1.4. Ann Arbor, MI: ELSO (August 2017).
91. Bui JD, Despotis GD, Trulock EP, Patterson GA, Goodnough LT. Fatal thrombosis after administration of activated prothrombin complex concentrates in a patient supported by extracorporeal membrane oxygenation who had received activated recombinant factor VII. J Thorac Cardiovasc Surg. (2002) 124:852–4. doi: 10.1067/mtc.2002.126038
92. Cavallaro G, Raffaeli G, Mosca F, Ghirardello G. ECMO neonatale e protocolli trasfusionali. Transf Med Netw. (2022). 2022:1–3.
93. Muszynski JA, Reeder RW, Hall MW, Berg RA, Shanley TP, Newth CJL, et al. RBC transfusion practice in pediatric extracorporeal membrane oxygenation support. Crit Care Med. (2018) 46:e552–9. doi: 10.1097/CCM.0000000000003086
94. Fiser RT, Irby K, Ward RM, Tang X, McKamie W, Prodhan P, et al. RBC transfusion in pediatric patients supported with extracorporeal membrane oxygenation: is there an impact on tissue oxygenation? Pediatr Crit Care Med. (2014) 15:806–13. doi: 10.1097/PCC.0000000000000222
95. Hébert PC, Wells G, Blajchman MA, Marshall J, Martin C, Pagliarello G, et al. A multicenter, randomized, controlled clinical trial of transfusion requirements in critical care. N Engl J Med. (1999) 340:409–17. doi: 10.1056/NEJM199902113400601
96. Holst LB, Haase N, Wetterslev J, Wernerman J, Guttormsen AB, Karlsson S, et al. Lower versus higher hemoglobin threshold for transfusion in septic shock. N Engl J Med. (2014) 371:1381–91. doi: 10.1056/NEJMoa1406617
97. Ghirardello S, Dusi E, Cortinovis I, Villa S, Fumagalli M, Agosti M, et al. Effects of red blood cell transfusions on the risk of developing complications or death: an observational study of a cohort of very low birth weight infants. Am J Perinatol. (2017) 34:88–95. doi: 10.1055/s-0036-1584300
98. Sawyer AA, Wise L, Ghosh S, Bhatia J, Stansfield BK. Comparison of transfusion thresholds during neonatal extracorporeal membrane oxygenation. Transfusion. (2017) 57:2115–20. doi: 10.1111/trf.14151
99. Fontana C, Raffaeli G, Pesenti N, Boggini T, Cortesi V, Manzoni F, et al. Red blood cell transfusions in preterm newborns and neurodevelopmental outcomes at 2 and 5 years of age. Blood Transfus. (2022) 20:40–9. doi: 10.2450/2020.0207-20
100. Lelubre C, Vincent JL. Red blood cell transfusion in the critically ill patient. Ann Intens Care. (2011) 1:43. doi: 10.1186/2110-5820-1-43
101. Collard KJ, Godeck S, Holley JE. Blood transfusion and pulmonary lipid peroxidation in ventilated premature babies. Pediatr Pulmonol. (2005) 39:257–61. doi: 10.1002/ppul.20190
102. Bembea MM, Cheifetz IM, Fortenberry JD, Bunchman TE, Valentine SL, Bateman ST, et al. Recommendations on the indications for RBC transfusion for the critically ill child receiving support from extracorporeal membrane oxygenation, ventricular assist, and renal replacement therapy devices from the pediatric critical care transfusion and anemia expertise initiative. Pediatr Crit Care Med. (2018) 19(9S Suppl 1):S157–62. doi: 10.1097/PCC.0000000000001600
103. Stiller B, Lemmer J, Merkle F, Alexi-Meskishvili V, Weng Y, Hubler M, et al. Consumption of blood products during mechanical circulatory support in children: comparison between ECMO and a pulsatile ventricular assist device. Intensive Care Med. (2004) 30:1814–20. doi: 10.1007/s00134-004-2352-z
104. Nellis ME, Saini A, Spinella PC, Davis PJ, Steiner ME, Tucci M, et al. Pediatric plasma and platelet transfusions on extracorporeal membrane oxygenation: a subgroup analysis of two large international point-prevalence studies and the role of local guidelines. Pediatr Crit Care Med. (2020) 21:267–75. doi: 10.1097/PCC.0000000000002160
105. Puetz J, Witmer C, Huang YS, Raffini L. Widespread use of fresh frozen plasma in US children's hospitals despite limited evidence demonstrating a beneficial effect. J Pediatr. (2012) 160:210.e1–5.e1. doi: 10.1016/j.jpeds.2011.08.013
106. Nellis ME, Karam O, Mauer E, Cushing MM, Davis PJ, Steiner ME, et al. Platelet transfusion practices in critically ill children. Crit Care Med. (2018) 46:1309–17. doi: 10.1097/CCM.0000000000003192
107. Saini A, West AN, Harrell C, Jones TL, Nellis ME, Joshi AD, et al. Platelet transfusions in the PICU: does disease severity matter? Pediatr Crit Care Med. (2018) 19:e472–8. doi: 10.1097/PCC.0000000000001653
108. Cashen K, Dalton H, Reeder RW, Saini A, Zuppa AF, Shanley TP, et al. Platelet transfusion practice and related outcomes in pediatric extracorporeal membrane oxygenation. Pediatr Crit Care Med. (2020) 21:178–85. doi: 10.1097/PCC.0000000000002102
109. Curley A, Stanworth SJ, Willoughby K, Fustolo-Gunnink SF, Venkatesh V, Hudson C, et al. Randomized trial of platelet-transfusion thresholds in neonates. N Engl J Med. (2019) 380:242–51. doi: 10.1056/NEJMoa1807320
110. Saini S, Folta AN, Harsh KL, Stanek JR, Dunn AL, O'Brien SH, et al. Anti-factor Xa-based monitoring of unfractionated heparin: clinical outcomes in a pediatric cohort. J Pediatr. (2019) 209:212.e1–9.e1. doi: 10.1016/j.jpeds.2019.02.015
111. Padhya DR, Prutsky GJ, Nemergut ME, Schears GS, Flick RP, Farah W, et al. Routine laboratory measures of heparin anticoagulation for children on extracorporeal membrane oxygenation: systematic review and meta-analysis. Thromb Res. (2019) 179:132–9. doi: 10.1016/j.thromres.2019.05.006
112. McMichael ABV, Hornik CP, Hupp SR, Gordon SE, Ozment CP. Correlation among antifactor Xa, activated partial thromboplastin time, and heparin dose and association with pediatric extracorporeal membrane oxygenation complications. ASAIO J. (2020) 66:307–13. doi: 10.1097/MAT.0000000000000986
113. Saifee NH, Brogan TV, McMullan DM, Yalon L, Matthews DC, Burke CR, et al. Monitoring hemostasis during extracorporeal life support. ASAIO J. (2020) 66:230–7. doi: 10.1097/MAT.0000000000000993
114. Liveris A, Bello RA, Friedmann P, Duffy MA, Manwani D, Killinger JS, et al. Anti-factor Xa assay is a superior correlate of heparin dose than activated partial thromboplastin time or activated clotting time in pediatric extracorporeal membrane oxygenation*. Pediatr Crit Care Med. (2014) 15:e72–9. doi: 10.1097/PCC.0000000000000028
115. Maul TM, Wolff EL, Kuch BA, Rosendorff A, Morell VO, Wearden PD. Activated partial thromboplastin time is a better trending tool in pediatric extracorporeal membrane oxygenation. Pediatr Crit Care Med. (2012) 13:e363–71. doi: 10.1097/PCC.0b013e31825b582e
116. Bembea MM, Schwartz JM, Shah N, Colantuoni E, Lehmann CU, Kickler T, et al. Anticoagulation monitoring during pediatric extracorporeal membrane oxygenation. ASAIO J. (2013) 59:63–8. doi: 10.1097/MAT.0b013e318279854a
117. Vandiver JW, Vondracek TG. Antifactor Xa levels versus activated partial thromboplastin time for monitoring unfractionated heparin. Pharmacotherapy. (2012) 32:546–58. doi: 10.1002/j.1875-9114.2011.01049.x
118. O'Meara LC, Alten JA, Goldberg KG, Timpa JG, Phillips J, Laney D, et al. Anti-xa directed protocol for anticoagulation management in children supported with extracorporeal membrane oxygenation. ASAIO J. (2015) 61:339–44. doi: 10.1097/MAT.0000000000000204
119. Chan AK, Black L, Ing C, Brandao LR, Williams S. Utility of aPTT in monitoring unfractionated heparin in children. Thromb Res. (2008) 122:135–6. doi: 10.1016/j.thromres.2007.09.007
120. Kuhle S, Eulmesekian P, Kavanagh B, Massicotte P, Vegh P, Lau A, et al. Lack of correlation between heparin dose and standard clinical monitoring tests in treatment with unfractionated heparin in critically ill children. Haematologica. (2007) 92:554–7. doi: 10.3324/haematol.10696
121. Hans GA, Besser MW. The place of viscoelastic testing in clinical practice. Br J Haematol. (2016) 173:37–48. doi: 10.1111/bjh.13930
122. Henderson N, Sullivan JE, Myers J, Wells T, Calhoun A, Berkenbosch J, et al. Use of thromboelastography to predict thrombotic complications in pediatric and neonatal extracorporeal membranous oxygenation. J Extra Corpor Technol. (2018) 50:149–54.
123. Regling K, Saini A, Cashen K. Viscoelastic testing in pediatric mechanical circulatory support. Front Med (Lausanne). (2022) 9:854258. doi: 10.3389/fmed.2022.854258
124. Katsaras G, Sokou R, Tsantes AG, Piovani D, Bonovas S, Konstantinidi A, et al. The use of thromboelastography (TEG) and rotational thromboelastometry (ROTEM) in neonates: a systematic review. Eur J Pediatr. (2021) 180:3455–70. doi: 10.1007/s00431-021-04154-4
125. Harr JN, Moore EE, Ghasabyan A, Chin TL, Sauaia A, Banerjee A, et al. Functional fibrinogen assay indicates that fibrinogen is critical in correcting abnormal clot strength following trauma. Shock. (2013) 39:45–9. doi: 10.1097/SHK.0b013e3182787122
126. Roberts TCD, De Lloyd L, Bell SF, Cohen L, James D, Ridgway A, et al. Utility of viscoelastography with TEG 6s to direct management of haemostasis during obstetric haemorrhage: a prospective observational study. Int J Obstet Anesth. (2021) 47:103192. doi: 10.1016/j.ijoa.2021.103192
127. Selby R. “TEG talk”: expanding clinical roles for thromboelastography and rotational thromboelastometry. Hematology. (2020). 2020:67–75. doi: 10.1182/hematology.2020000090
128. Drop JG, Erdem O, Wildschut ED, van Rosmalen J, de Maat MPM, Kuiper J-W, et al. Use of rotational thromboelastometry to predict hemostatic complications in pediatric patients undergoing extracorporeal membrane oxygenation: a retrospective cohort study. Res Pract Thromb Haemost. (2021) 5:e12553. doi: 10.1002/rth2.12553
129. Burton CL, Furlong-Dillard JM, Jawad K, Feygin Y, Berkenbosch JW, Tzanetos DT. Analysis of viscoelastic testing in pediatric patients using the pediatric extracorporeal membrane oxygenation outcomes registry. ASAIO J. (2021) 67:1251–6. doi: 10.1097/MAT.0000000000001388
130. Teruya J, Hensch L, Bruzdoski K, Adachi I, Hui SR, Kostousov V. Monitoring bivalirudin therapy in children on extracorporeal circulatory support devices: thromboelastometry versus routine coagulation testing. Thromb Res. (2020) 186:54–7. doi: 10.1016/j.thromres.2019.12.007
131. Fahrendorff M, Oliveri RS, Johansson PI. The use of viscoelastic haemostatic assays in goal-directing treatment with allogeneic blood products - a systematic review and meta-analysis. Scand J Trauma Resusc Emerg Med. (2017) 25:39. doi: 10.1186/s13049-017-0378-9
132. Wikkelso A, Wetterslev J, Moller AM, Afshari A. Thromboelastography (TEG) or thromboelastometry (ROTEM) to monitor haemostatic treatment versus usual care in adults or children with bleeding. Cochrane Database Syst Rev. (2016) 2016:CD007871. doi: 10.1002/14651858.CD007871.pub3
133. Amodeo I, Raffaeli G, Vianello F, Cavallaro G, Cortesi V, Manzoni F, et al. Individualized bleeding risk assessment through thromboelastography: a case report of may-hegglin anomaly in preterm twin neonates. Children (Basel). (2021) 8:878. doi: 10.3390/children8100878
134. Amelio GS, Raffaeli G, Amodeo I, Gulden S, Cortesi V, Manzoni F, et al. Hemostatic evaluation with viscoelastic coagulation monitor: a NICU experience. Front Pediatr. (2022) 10:910646. doi: 10.3389/fped.2022.910646
135. Raffaeli G, Pesenti N, Cavallaro G, Cortesi V, Manzoni F, Amelio GS, et al. Optimizing fresh-frozen plasma transfusion in surgical neonates through thromboelastography: a quality improvement study. Eur J Pediatr. (2022) 181:2173–82. doi: 10.1007/s00431-022-04427-6
136. Theodoraki M, Sokou R, Valsami S, Iliodromiti Z, Pouliakis A, Parastatidou S, et al. Reference values of thrombolastometry parameters in healthy term neonates. Children. (2020) 7:259. doi: 10.3390/children7120259
137. Lampridou M, Sokou R, Tsantes AG, Theodoraki M, Konstantinidi A, Ioakeimidis G, et al. ROTEM diagnostic capacity for measuring fibrinolysis in neonatal sepsis. Thromb Res. (2020) 192:103–8. doi: 10.1016/j.thromres.2020.05.028
138. Konstantinidi A, Sokou R, Tsantes AG, Parastatidou S, Bonovas S, Kouskouni E, et al. Thromboelastometry variables in neonates with perinatal hypoxia. Semin Thromb Hemost. (2020) 46:428–34. doi: 10.1055/s-0040-1709473
139. Konstantinidi A, Sokou R, Parastatidou S, Lampropoulou K, Katsaras G, Boutsikou T, et al. Clinical application of thromboelastography/thromboelastometry (TEG/TEM) in the neonatal population: a narrative review. Semin Thromb Hemost. (2019) 45:449–57. doi: 10.1055/s-0039-1692210
140. Sokou R, Giallouros G, Konstantinidi A, Pantavou K, Nikolopoulos G, Bonovas S, et al. Thromboelastometry for diagnosis of neonatal sepsis-associated coagulopathy: an observational study. Eur J Pediatr. (2018) 177:355–62. doi: 10.1007/s00431-017-3072-z
141. Sokou R, Foudoulaki-Paparizos L, Lytras T, Konstantinidi A, Theodoraki M, Lambadaridis I, et al. Reference ranges of thromboelastometry in healthy full-term and pre-term neonates. Clin Chem Lab Med. (2017) 55:1592–7. doi: 10.1515/cclm-2016-0931
142. Sokou R, Tsantes AG, Konstantinidi A, Ioakeimidis G, Lampridou M, Parastatidou S, et al. Rotational thromboelastometry in neonates admitted to a neonatal intensive care unit: a large cross-sectional study. Semin Thromb Hemost. (2021) 47:875–84. doi: 10.1055/s-0041-1729964
143. Ghirardello S, Raffaeli G, Scalambrino E, Chantarangkul V, Cavallaro G, Artoni A, et al. The intra-assay reproducibility of thromboelastography in very low birth weight infants. Early Hum Dev. (2018) 127:48–52. doi: 10.1016/j.earlhumdev.2018.10.004
144. Ghirardello S, Raffaeli G, Cavallaro G, Mosca F. Reference ranges of thromboelastography in premature neonates? Still a long way to go. Early Hum Dev. (2018) 116:95–6. doi: 10.1016/j.earlhumdev.2017.12.005
145. Alexander DC, Butt WW, Best JD, Donath SM, Monagle PT, Shekerdemian LS. Correlation of thromboelastography with standard tests of anticoagulation in paediatric patients receiving extracorporeal life support. Thromb Res. (2010) 125:387–92. doi: 10.1016/j.thromres.2009.07.001
146. Saini A, Hartman ME, Gage BF, Said A, Gazit AZ, Eghtesady P, et al. Incidence of platelet dysfunction by thromboelastography-platelet mapping in children supported with ECMO: a pilot retrospective study. Front Pediatr. (2015) 3:116. doi: 10.3389/fped.2015.00116
147. Nair P, Hoechter DJ, Buscher H, Venkatesh K, Whittam S, Joseph J, et al. Prospective observational study of hemostatic alterations during adult extracorporeal membrane oxygenation (ECMO) using point-of-care thromboelastometry and platelet aggregometry. J Cardiothorac Vasc Anesth. (2015) 29:288–96. doi: 10.1053/j.jvca.2014.06.006
148. Phillips RC, Shahi N, Leopold D, Levek C, Shirek G, Hilton S, et al. Thromboelastography-guided management of coagulopathy in neonates with congenital diaphragmatic hernia supported by extracorporeal membrane oxygenation. Pediatr Surg Int. (2020) 36:1027–33. doi: 10.1007/s00383-020-04694-0
149. Kalinin DV. Factor XII(a) inhibitors: a review of the patent literature. Expert Opin Ther Pat. (2021) 31:1155–76. doi: 10.1080/13543776.2021.1945580
150. Gailani D. Making anticoagulation safer. Lancet. (2022) 399:1360–1. doi: 10.1016/S0140-6736(22)00563-3
Keywords: neonatal ECMO, developmental hemostasis, thrombosis, anticoagulation management, point-of-care tests
Citation: Cortesi V, Raffaeli G, Amelio GS, Amodeo I, Gulden S, Manzoni F, Cervellini G, Tomaselli A, Colombo M, Araimo G, Artoni A, Ghirardello S, Mosca F and Cavallaro G (2022) Hemostasis in neonatal ECMO. Front. Pediatr. 10:988681. doi: 10.3389/fped.2022.988681
Received: 07 July 2022; Accepted: 12 August 2022;
Published: 26 August 2022.
Edited by:
Rozeta Sokou, Nikaia General Hospital “Aghios Panteleimon”, GreeceReviewed by:
Ahmed S. Said, Washington University in St. Louis, United StatesSerena Valsami, Aretaeio Hospital, National and Kapodistrian University of Athens, Greece
Copyright © 2022 Cortesi, Raffaeli, Amelio, Amodeo, Gulden, Manzoni, Cervellini, Tomaselli, Colombo, Araimo, Artoni, Ghirardello, Mosca and Cavallaro. This is an open-access article distributed under the terms of the Creative Commons Attribution License (CC BY). The use, distribution or reproduction in other forums is permitted, provided the original author(s) and the copyright owner(s) are credited and that the original publication in this journal is cited, in accordance with accepted academic practice. No use, distribution or reproduction is permitted which does not comply with these terms.
*Correspondence: Giacomo Cavallaro, Z2lhY29tby5jYXZhbGxhcm8mI3gwMDA0MDtwb2xpY2xpbmljby5taS5pdA==
†These authors have contributed equally to this work and share first authorship