- 1Pediatric Stem Cell Transplantation, Boston Children's Hospital/Dana-Farber Cancer Institute, Boston, MA, United States
- 2Department of Experimental and Clinical Pharmacology, University of Minnesota College of Pharmacy, Minneapolis, MN, United States
Access to allogenic hematopoietic cell transplantation (HCT), a potentially curative treatment for chemotherapy-resistant hematologic malignancies, can be limited if no human leukocyte antigen (HLA) identical related or unrelated donor is available. Alternative donors include Cord Blood as well as HLA-mismatched unrelated or related donors. If the goal is to minimize the number of HLA disparities, partially matched unrelated donors are more likely to share 8 or 9 of 10 HLA alleles with the recipient. However, over the last decade, there has been success with haploidentical HCT performed using the stem cells from HLA half-matched related donors. As the majority of patients have at least one eligible and motivated haploidentical donor, recruitment of haploidentical related donors is frequently more rapid than of unrelated donors. This advantage in the accessibility has historically been offset by the increased risks of graft rejection, graft-versus-host disease and delayed immune reconstitution. Various ex vivo T-cell depletion (TCD) methods have been investigated to overcome the immunological barrier and facilitate immune reconstitution after a haploidentical HCT. This review summarizes historical and contemporary clinical trials of haploidentical TCD-HCT, mainly in pediatric malignancy, and describes the evolution of these approaches with a focus on serial improvements in the kinetics of immune reconstitution. Methods of TCD discussed include in vivo as well as ex vivo positive and negative selection. In addition, haploidentical TCD as a platform for post-HCT cellular therapies is discussed. The present review highlights that, as a result of the remarkable progress over half a century, haploidentical TCD-HCT can now be considered as a preferred alternative donor option for children with hematological malignancy in need of allogeneic HCT.
Introduction
Allogeneic hematopoietic cell transplantation (HCT) is an established, potentially curative, option for some children with high-risk hematologic malignancy. HCT can mediate anti-tumor activity by virtue of cytotoxic agents in the conditioning regimen, eradication of stem cell populations with genetic predisposition to leukemia, and to different extents in different diseases, graft-versus-leukemia (GVL) activity. Historically the optimal allogeneic donor for HCT was considered to be an unaffected human leukocyte antigen (HLA)-matched related donor (MRD). However, an MRD is only available for 20%–30% of patients in need of HCT (1). Therefore, the use of alternative donors is essential to extend this curative option to the majority of patients in need. To support alternative donor transplantation, the first donor registry was established in 1974 and more than 32 million individuals have since been registered as potential HCT donors. Almost two decades later, the first umbilical cord blood bank was established in 1992. While transplant from matched unrelated donors (MUD) and umbilical cord blood (UCB) are established as options for patients lacking an MRD, they are associated with an increased risk of non-relapse mortality (NRM) largely due to an increase risk of graft vs. host disease (GvHD) and infection (2, 3). Similar to an HCT from an MRD, either bone marrow (BM) or peripheral blood stem cells (PBSC) can be collected from a MUD with potential accessibility of later repeated collection for adverse events (e.g., graft failure, relapse). However, the process is time-consuming involving identification, screening and collection of stem cells from an available and motivated donor, and may not meet the time constraints for recipients in urgent need of HCT. Moreover, HLA matched URDs are available for <30% in patients of racial/ethnic minorities in need as compared to 70%–80% of those of European descent (4). In UCB units are screened and stored frozen in banks and can be rapidly accessed. The biology of UCB allows a higher level of HLA mismatch such that recipients of racial/ethnic minorities are more likely to have an acceptable donor. While UCB unit expansion and double unit transplant are approaches to overcome the limited cell doses in UCB units, transplant with a single UCB is frequently not feasible in adolescents or young adults, and conventional UCBs do not offer the possibility of repeated infusions. Thus, there are a variety of issues that have limited the success of alternative donor transplantation, some of which can be overcome with the use of haploidentical related donors.
HCT from related individuals with a half-matched HLA profile (i.e., haploidentical HCT) can overcome the limitations of availability.
The major HLA genes are located on chromosome 6 and typically inherited without cross-over events. Thus, a patient will typically be haploidentical with each of their parents and half of their biological siblings. As a result, almost every patient has a readily available haploidentical motivated donor who can provide BM or PBSC repeatedly as necessary. However, haploidentical transplant requires specialized approaches to overcome the major immunological barrier of HLA mismatch with the potential of graft rejection mediated by recipient T cells and GvHD mediated by donor T cells. Ex vivo T-cell depletion (TCD) of grafts has been utilized in haploidentical HCT for decades to overcome this challenge (5). The first successful TCD transplantations were performed in infants with immune deficiency who were unable to reject HLA mis-matched stem cells. However, other early attempts of TCD haploidentical HCTs were complicated by graft failure. Even after engraftment was reliably achieved, the dependence on de novo, thymic derived T cell reconstitution has been associated with slow immune reconstitution. Recent advances in ex vivo TCD through sophisticated, targeted removal of specific lymphocyte subsets as well as in vivo TCD primarily with adoption of the post-transplant cyclophosphamide (PTCY) platform have led to improved outcomes. These approaches also eliminate or shorten the duration of immunosuppressive medications in the post-HCT period simultaneously offering a unique platform for adoptive cellular therapy for control of infectious complications and even underlying malignant disease in the post-HCT period. In this review, we discuss the evolution of TCD strategies in haploidentical HCT for pediatric malignancies with a focus on immune reconstitution and cell therapy post HCT.
T cell depletion for haploidentical-HCT
Evolution of ex vivo TCD
Recognized as a potentially fatal complication of haploidentical HCT since the 1970s and 1980s (6, 7), severe acute GvHD is a consequence of donor immune cell recognition of the recipient as foreign. As a strategy to prevent GvHD, T cell depletion was introduced over a half century ago and was initially successful in transplant of infants with impaired immunity. These early transplants were associated with a very limited incidence of acute and chronic GvHD, and TCD remain the most robust approach to limiting the incidence of chronic GvHD (5).
In this earliest version of ex vivo TCD, donor T cells were removed from bone marrow by sequential soybean lectin agglutination and E-rosette depletion (SBA-E-) resulting in a 3- to 4-log depletion of T cells (8). Subsequent approaches depended on the positive selection of CD34+ stem cells achieving a 5-log depletion of T cells successfully preventing GvHD and delivering high stem cell doses able to achieve reliable engraftment. However, even after issues of graft rejections were addressed, this approach was associated with an increased risk of treatment-related mortality (TRM) largely related to infection, such that prospective randomized trials failed to demonstrate improved OS (9).
Reconstitution of T cell immunity after transplant depends on homeostatic expansion of T cells infused with the graft and de novo thymic dependent T cell generation (10). Recipients of TCD HCT depend predominantly on the latter which takes a minimum of 3 months in young infants and up to several years in older adults (11, 12). A TCD HCT approach with a less complete depletion of T cells was an early attempt to solve this issue and was explored in a multi-center trial, but failed to demonstrate an advantage in disease-free survival (DFS) (13).
Subsequently techniques were developed to deplete specific populations of T cells rather than positively select CD34+ stem cells. This approach can preserve the non-T cell composition of the graft and has allowed for increasingly sophisticated approaches to graft manipulation. Removal of CD3+/CD19+ cells successfully spared NK cells in addition to progenitor cells, which decreased the incidence of fatal infections (14). More recently, ex vivo depletion strategies selectively removing TCRαβ+ T cells or Naïve CD45RA+ T cells have been explored with promising results. These more sophisticated approaches to graft manipulation have the promise to improve post-transplant immune reconstitution while preserving the protection from GvHD; however, they are resource intense and will likely not be feasible in resource limited areas. In contrast, the use of PTCY for in vivo TCD of haploidentical HCT has emerged as an approach that is broadly accessible. Understanding the kinetics of immune reconstitution after haploidentical transplants facilitated with each of these approaches will be critical to successful implementation of the broadening armamentarium of alternative donor options. Key characteristics of different ex-vivo TCD methods are highlighted in Table 1, and clinical evidence is summarized in Supplementary Table S1. Figures 1, 2 show schematic illustration of SBA-E- and CliniMACS methods, respectively.
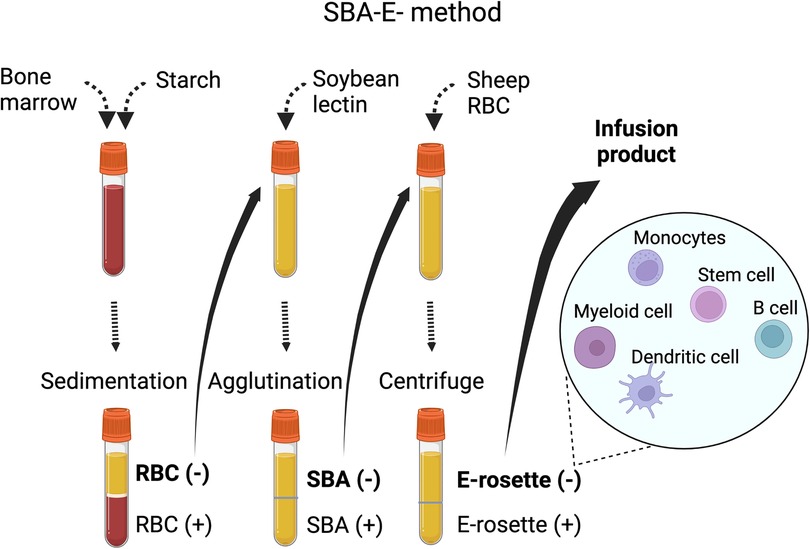
Figure 1. Soybean lectin agglutination and E-rosette (SBA-E-) method.There are four main steps in the procedure, (1) Filtered bone marrow is separated over hetastarch and the leukocyte-rich plasma (RBC-) is collected. (2) Leukocyte-rich plasma is mixed with soybean lectin, allowed to agglutinate and then settled through 5% albumin. (3) The unagglutinated (SBA-negative) population is mixed with sheep RBCs to allow R-rosette formation with T cells. After centrifugation over ficoll-hypaque, the supernatant (R-rosette negative) is collected. A final separation removes the trace of residual T cells in the SBA-E- fraction by separation of cells forming E-rosettes with neuraminidase-treated sheep RBCs.Abbreviation: SBA-E-, soybean agglutination negative and E-rosette negative; RBC, red blood cell.
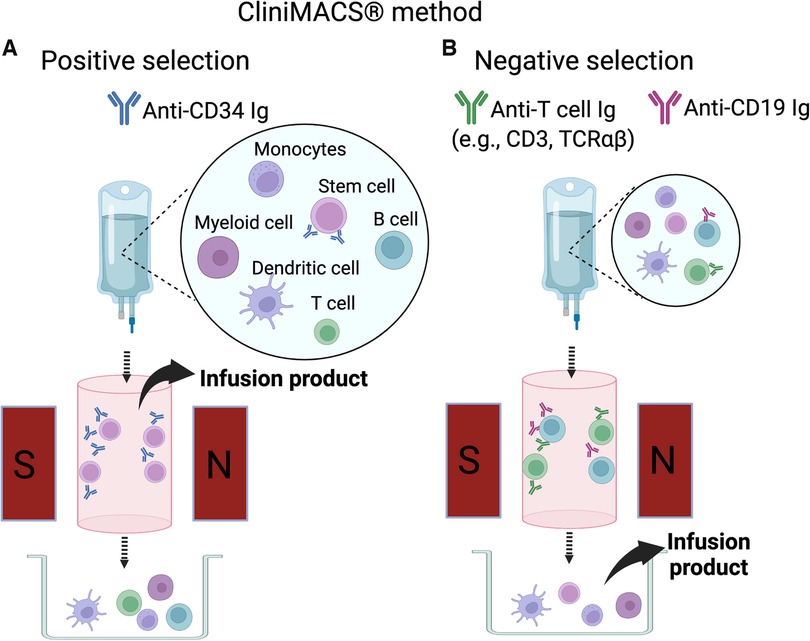
Figure 2. CliniMACS method. (A) The positive selection method (CD34+ selection). Donor marrow or G-CSF mobilized peripheral blood mononuclear cells are stained with anti-CD34 antibody conjugated with magnetic beads. CD34+ cells bound to the antibody-bead complex are captured in the magnetic field on the column. The cells retained in the magnetic field are released from the magnet and collected for infusion. (B) The negative selection method. Bone marrow or peripheral blood mononuclear cells are stained with anti-T cell antibody (e.g., anti-CD3 or anti-TCRαβ) and anti-CD19 antibody are used as antibody-magnetic bead complex. The cells bound to the antibody-bead complex are captured in the magnetic field. The cells not captured on the magnetic field of the column are collected for infusion.
T Cell Depletion by Soybean Lectin Agglutination with E-rosetting
Following the report by Dicke et al. in 1968 that splenocytes depleted of lymphoid cells by density centrifugation could engraft in irradiated mice, ex vivo TCD haploidentical HCT was introduced to the clinic in the 1980s (15). In 1981 Reisner and his colleagues at Memorial Sloan Kettering Cancer Center in New York, USA described a sequential technique to fractionate donor BM cells by initial removal of T cells by soybean lectin agglutinin, followed by elimination of residual T cells by E-rosetting (SBA-E-) with sheep red blood cells. This technique decreased donor T cells from the harvested BM graft by 1000-fold.
After durable engraftment of haploidentical TCD HCT was reported in two of three patients with primary immune deficiency, a large series confirmed this as a curative approach for infants with SCID (16, 17). However, this lectin-based TCD approach failed in patients with leukemia because of an unacceptably high incidence of graft rejection (as high as 50%) (18) mediated by the emergence of host-derived cytotoxic T cells with anti-donor specificity (19). These donor-specific host T cells can be overcome by donor T cells, but when uncontested, residual host T cells can eliminate the TCD graft. In HLA-matched sibling transplants, this barrier could be overcome by the addition of anti-thymocyte globulin (ATG) and/or thiotepa to the conditioning regimen (20).
Based on promising preclinical data (21, 22), the group at the University of Perugia, Italy used “megadose” haploidentical HCT to overcome host T cell-mediated graft rejection (23). After stem cell mobilization by G-CSF, both BM and PBSC were collected and depleted of T cells via lectin-based ex vivo TCD. These grafts contained 11.6 ± 4.7 × 106 CD34+ cells/kg and were infused to patients after intensified conditioning with cyclophosphamide, thiotepa, ATG, and total body irradiation (TBI). Among the 17 adult and pediatric patients in this study, one had graft rejection and one had acute GvHD. However, fatal infections (3 patients with CMV and 2 with sepsis) and transplant-related toxicity (3 idiopathic pneumonitis and 1 GvHD) resulted in 9 deaths. This experience revealed the unacceptably high risk of severe infection in recipients of TCD HCT which was increasingly understood to be related to a prolonged period of immune compromise.
These lectin-based approaches have largely been replaced as they were technically challenging and thus difficult to export; however, they importantly demonstrated the feasibility of TCD HCT crossing HLA barriers and set the standard for subsequent approaches.
Positive Selection of CD34+ Peripheral Blood Stem Cells
The advent of an immuno-magnetic cell separation device, CliniMACS (Meltenyi Biotec, Bergisch Gladbach, Germany) in 1999 was among the biggest breakthroughs in the field of haploidentical HCT. This device enables purification of desired stem cell subsets using microbeads bound to monoclonal antibodies recognizing specific cell surface markers. Using magnetic beads bound to anti-CD34 antibody, purity and recovery of collected CD34 cells were 98% and 75%, respectively with residual CD3 and CD19 cells of 0.03% and 1.2% (24). The University of Perugia group then investigated a protocol using CD34+ PBSC after GCSF mobilization in combination with de-escalation of conditioning regimen by replacing cyclophosphamide with fludarabine in 1999–2004 (25). Adults and children with hematologic malignancy received high-dose CD34+ cells (mean 13.8 × 106 cells/kg) following conditioning with fludarabine, thiotepa, ATG, and TBI. No post-transplant GvHD prophylaxis was given. Of the 101 patients, 94 attained primary engraftment, and all but 1 engrafted after a second transplantation. Risks of acute GvHD, chronic GvHD, and NRM were relatively low (8%, 7%, and 37%, respectively). Despite a prior theoretical concern, the risk of relapse was not increased among those in remission prior to HCT (14%). A main setback of this regimen remained delayed immune reconstitution and a high risk of fatal opportunistic infection. In this cohort 71% (27/38) of NRM was attributed to infection, especially of viruses (14 cases of CMV, 1 EBV, 1 adenovirus, and 1 HHV-6). In a recent randomized trial in adult patients with AML or MDS undergoing HLA-matched HCT, it was demonstrated that delayed immune reconstitution associated with an increased incidence of fatal infections led to a trend of higher rate of NRM (9).
These results were extended to pediatric patients in a retrospective study from the European Blood and Marrow Transplantation reporting on 102 recipients of TCD haploidentical HCT transplanted between 1995 and 2004 (77% used CD34+ selection by CliniMACS) (26). Primary and secondary graft failure were observed in 9% and 4% of patients, respectively. Acute Grade 2, Grade 3–4, and chronic GvHD occurred in 13%, 9%, and 14% of patients, respectively. Five-year leukemia-free survival, relapse, and NRM were 27%, 36%, and 37%, respectively. A trend of improved leukemia-free survival and decreased relapse was observed in recipients of a graft with a higher number of CD34 cells (adjusted p = 0.09 and p = 0.07, respectively). This study demonstrated that relapse and infection are common causes of mortality (46% and 33% of death, respectively). Aversa et al. investigated nonmyeloablative haploidentical HCT with ATG/Fludarabine/TBI 2Gy regimen followed by infusion of megadose CD34+ cells by CD3-/CD19- TCD combined with PTCY as the sole posttransplant GvHD prophylaxis (27).
In 1999 Small et al. demonstrated the improved overall and event free survival in younger recipients of TCD HCT and evaluated the risk of infection as related to attaining milestones of immune reconstitution. The importance of achieving specific milestones of immune reconstitution was again demonstrated by Admiraal et al. in 2015 who established that achieving a CD4 of 50/μl by day 100 post HCT was associated with improved outcomes (28). This milestone was validated in 2020 by van Roessel and Prockop et al. who demonstrated that those undergoing TCD HCT with any of the above methods were less likely to achieve this milestone, but those who did achieve it similarly had superior OS (12). More recently, de Koning et al. reported that pediatric patients who developed GvHD regardless of the transplant platform had improved survival if they had attained this milestone (29).
Selective Depletion of CD3+ T Cells and CD19+ B Cells
The appreciation for the essential roles played by non-T/non-B cells in the success of HCT was important in shifting from a positive selection of CD34+ HSCs to an approach in which CD3+ and CD19+ cells are removed from the graft by “negative selection”, sparing other populations including NK cells, dendritic cells, and monocytes. In particular, many preclinical studies suggested contribution of NK cells to successful engraftment, immune reconstitution, and anti-leukemia effect (30).
Diaz et al. reported experience with CD3-/CD19- haploidentical PBSC with fludarabine-based reduced intensity conditioning (RIC) among 70 children with hematologic malignancy in Spain between 2005 and 2013. Pharmacologic GvHD prophylaxis consisted of a short course of cyclosporine (tapered before Day +30) (14). Graft failure were observed in 4 patients (6%), 3 of whom were rescued by a second transplant. With a median follow-up of 22 months the probability of NRM, relapse, and disease-free survival (DFS) were 23±5%, 32±6%, and 52±6%, respectively. Recovery of NK cells were notably faster compared to recipients of CD34+ PBSC (31), and infection-related mortality was 9%. However, this regimen was associated with a higher incidence of acute and chronic GvHD (grade 2–4 acute 35±5% and chronic GvHD 46±7%). This insufficient GvHD prophylaxis was attributed to higher residual T cells as compared to CD34+ PBSC (31).
Lang et al. at University of Tübingen in Germany also investigated CD3-/CD19- haploidentical PBSC using a melphalan-based non-TBI RIC regimen in pediatric patients with hematologic malignancies treated between 2004 and 2012. Median CD34+ and CD3+ cells in the infused graft were 15 × 106/kg and 0.06 × 106/kg, and those with CD3+ cells >0.025 × 106/kg received a short course of mycophenolate mofetil (MMF). This trial had a 12% incidence of graft failure, where all were rescued by a repeated transplantation, and 5-year TRM of 20% (causes of death included bacterial infection [n = 1], GvHD [n = 2], cardiomyopathy [n = 2]). However, the incidence of relapse was high even among those who underwent the first HCT in remission (2-year relapse incidence and 3-year EFS were 38% and 46%, respectively). Acute GvHD grade 2–4 (27%) and chronic GvHD (21%) resulted in two deaths.
Selective depletion of TCRαβ+ T cells and CD19+ B cells
More recently, further refinements of negative selection via CliniMACS have been introduced. It has been recognized since the 1990s that different subsets of T cells play critical roles in immune regulation post HCT. Several lines of evidence suggested that TCRαβ+ populations are more central than TCRγδ+ populations in causing GvHD. Biopsies of skin, liver and intestines in the presence of clinical GvHD showed tissue infiltrating lymphocytes that were almost exclusively TCRαβ+ with very few TCRγδ (32). Moreover, the number of TCRγδ cells in tissue affected by GvHD are comparable to those of controls without GvHD (33). Another study showed a correlation between higher TCRαβ cell dose in the graft and the risk of acute GvHD, while a higher dose of TCRγδ was correlated with successful engraftment (34). These findings have been confirmed in a murine model (35).
In 2007 Chaleff et al. from St. Jude Children's Research Hospital developed a technique to perform clinical-scale TCRαβ/CD19 depletion using the CliniMACS device (36). They successfully reduced the total T cell dose by 4–5 log, which is comparable to CD34+ TCD (37). The group in Rome, Italy was among the first to employ this technique depleting TCRαβ/CD19 from haploidentical PBSC in the treatment of 13 children with nonmalignant diseases (38). Lang, et al. from Tubingen, Germany subsequently reported their experience in a pediatric cohort transplanted for both malignant and non-malignant disease (39). These early single-center reports revealed a high rate of engraftment, a very low risk of visceral acute or chronic GvHD, and early reconstitution of TCR γδ+T cells and NK cells (39, 40).
These favorable results of TCRαβ/CD19 depleted HCT were confirmed in multicenter experiences. In an Italian registry of 13 centers, HCT outcomes were compared in children with malignancy who underwent myeloablative haploidentical HCT with TCRαβ-/CD19- and conventional T-cell replete HCT from matched or mismatched URDs (n = 98, 127, and 118, respectively) (41). In this first multicenter study of TCRαβ-/CD19-, all approaches showed a very low incidence of graft failure (2% each). Depletion of αβ T-cells in combination with ATG without other pharmacological GvHD prophylaxis eliminated any case of grade 3–4 or visceral acute GvHD. Incidences of grade 2 acute GvHD and extensive chronic GvHD were also substantially decreased (16% and 1% in TCRαβ-/CD19- vs. 35% and 3% in matched URD, and 44% and 12% in mismatched URD). In addition, combined ex vivo and in vivo B cell depletion with CD19- selection and a single dose of rituximab completely prevented post-transplant lymphoproliferative disease. Third, TCRαβ-/CD19- haploidentical HCT, in comparison to matched URD, was associated with higher CD34+ cell doses (14.4 vs. 5.2 × 106 cells/kg), faster neutrophil recovery (13 vs. 19 days), and faster platelet recovery (11 vs. 22 days). Overall, NRM was comparable in haploidentical TCRαβ-/CD19- HCT and matched URD HCT (6% vs. 9%). However, disease relapse remained the primary cause of failure in this relatively low-risk cohort (i.e., pediatric leukemia in remission with myeloablative regimen). Incidence of relapse was comparable between recipients of TCRαβ-/CD19- HCT and recipients of matched URD HCT (26% vs. 29%) despite a lower proportion of first complete remission in the former.
Selective depletion of naïve (CD45RA+) T cells
Another approach to deplete targeted populations of T cells is the removal of CD45RA+ cells, which selectively depletes naive T cells while retaining memory T cells. Naive T cells are known to possess higher alloreactive potential and contribute to GvHD development (42), while the latter augments diversity of T cell population against virus infection. On these bases, Bleakley et al. from Fred Hutchinson Cancer Center (Seattle, USA) developed a 2-step graft manipulation technique that incorporates a CD34+ positive component with one depleted of CD45RA+ cells (43). It is hypothesized that addback of T cells selectively depleted of CD45RA+ cells will improve immune reconstitution and reduce severe viral infection without significantly increasing the risk of GvHD. In their phase 2 single-arm trials including 138 adults with hematology malignancy undergoing MRD or MUD HCT, this method resulted in limited acute GvHD (4% and 0% for grade 3 and 4, respectively) albeit with a high incidence of acute GvHD grade 2 (71%) and remarkably infrequent chronic GvHD (total 7%, moderate 1%, severe 0%) (44). Memory T cells in the graft resulted in rapid T cell recovery and transfer of protective virus-specific immunity. Excessive rates of infection or relapse did not occur and OS was 78% at 2 years.
Triplett et al. at St. Jude Children's Research Hospital reported the efficacy and safety of CD45RA- HCT in pediatric malignancy as compared to CD3- HCT. Their regimen incorporates a CD34+ TCD graft on Day 0, followed by a CD45RA depleted stem cell infusion on Day +1, and a subsequent NK cell infusion on Day +6 (45). The CD45RA- method, in comparison to the CD3-, attained 2000-fold higher donor T cell doses and higher T-cell counts at Day +30 post-HCT (550/μl vs. 10/μl; p < 0.001), and higher T-cell diversity by Vβ spectra typing at Day +100 (p < 0.001). The CD45RA- method also resulted in a significant reduction in both the incidence and duration of any viremia (CMV, EBV, or adenovirus) in the first 6 months post-HCT. Although incidence of grade 3–4 acute GvHD were comparable in both types of TCD-HCT (23.1% vs. 22.6%), this risk was higher than that reported with other methods of TCD (e.g., CD3-CD19- or TCRαβ-/CD19-). Of note, this protocol used a short course of MMF (day <60) and total lymphoid irradiation to replace serotherapy and decrease the risk of graft rejection.
While it is clear that prevention and management of relapse is still a relevant and challenging problem that limits the success of HCT, the sophisticated graft engineering employed in haploidentical HCT can provide a unique platform for post-transplant therapies.
In vivo T cell depletion
Two approaches to support haploidentical transplant with in vivo TCD have been developed. Neither of these depend on sophisticated graft engineering and thus are easily exportable globally including to resource restricted regions. The first PTCY was pioneered by the group at Johns Hopkins Hospital (Baltimore, MD, United States), and depends on the preferential sensitivity of activated T cells to cyclophosphamide. This platform initially used a reduced intensity conditioning regimen combined with a conventional marrow graft and post-transplant (days 3 and 4) high dose cyclophosphamide combined with a CNI and MMF for GvHD prophylaxis (46) with reports of similar incidence of GvHD to that seen following a conventional MUD transplant in adults for AML (47). Subsequently myeloablative conditioning (48, 49) and the use of peripheral blood mononuclear cells (48) have all been explored as recently reviewed (50). A recent retrospective report from the EBMT on pediatric patients transplanted with PTCY based regimens for ALL reported a 2-year cumulative incidence of relapse of 25%, 37% and 50% for those transplanted in 1st, 2nd, or ≥3rd CR respectively and a 2-year OS, leukemia-free survival and GvHD-free, relapse-free survival (GRFS) of 50.8%, 38.5% and 29.2% (51).
The second approach to in vivo TCD has been termed the Beijing Protocol. For malignant disease both TBI-based on non-TBI based myeloablative regimens with high doses of ATG and GvHD prophylaxis with MMF, CSA and MTX are used with infusion of a G-CSF primed bone marrow graft. Most recently, preliminary reports suggest that this approach can be combined with a PTCY regimen. Promising results from pediatric and young adult cohorts of patients with Ph+ and Ph- ALL including those with MRD at the time of transplant (52, 53). However, beyond publications referencing a high incidence of CMV reactivation and refractory CMV infection (54), there is scant information related to immune reconstitution.
PTCY and Beijing protocols for in vivo TCD require post-transplant calcineurin inhibitor based immune suppression and are associated with distinct toxicities and sequence of immune reconstitution. The requirement for post-transplant immune suppression makes them less ideal as platforms for adoptive cellular therapy in recipients of haploidentical HCT and as such they are discussed only briefly.
Engraftment and immune reconstitution after haplo-HCT
Overall
Delayed immune reconstitution has been recognized as a major impediment to the success of T cell depleted transplantation. While an improved understanding of the contribution by different populations of the immune system to protective immunity has led to more sophisticated approaches to graft manipulation, the ability to ensure that protective immunity is reestablished in the post-transplant period remains elusive. Immune reconstitution is a complex process involving factors in the recipient (e.g., age, underlying disease), the donor (e.g., graft source cell dose, HLA match, sex match, CMV match, age) HCT regimen (e.g., conditioning regimen, in vivo T cell depletion, post-HCT GvHD prophylaxis), and post-HCT complications (e.g., CMV reactivation, GvHD) (55). The pattern of immune reconstitution after conventional MRD HCT proceeds from monocytes to neutrophils and NK cells within 1 month, with reconstitution of T cells starting at around 2–3 months, and B cells being the slowest to recover at around 1-year post HCT. In contrast after TCD HCT, T cell reconstitution, specifically CD4 T cell reconstitution is typically the last component of immunity to recover.
Monocytes
Monocytes are the first cell population that recover after an HCT. Although the kinetics and clinical implications of monocyte recovery are not as well-known as other populations, some studies suggest favorable HCT outcomes in patients who have early monocyte recovery. In a heterogenous HCT cohort with various donors (MRD, MUD, and single or double UCB) and various conditioning regimens (MAC or RIC) early recovery of monocytes at 1-month post HCT was associated with better OS, relapse, and NRM (56, 57). In TCD haploidentical HCT donor monocytes and granulocytes are depleted by CD34 positive selection but not by CD3/CD19 negative selection (<1% vs. 130 × 106 cells/kg) (58). However, another comparative study revealed similar monocyte reconstitution by TCD technique (CD34+ vs. CD3-/CD19-) or conditioning intensity (MAC vs. RIC). In this cohort of pediatric hematology malignancy, monocyte reconstitution was swift; approximately 80% achieved their age norm value at 30 days after SCT (59).
Neutrophil
Neutrophil recovery has been among the most established early indicators of HCT success which is also associated with risk of serious infection. In early experience with “megadose” TCD myeloablative haploidentical HCT by SBA-E- technique, median time to neutrophil recovery >1,000 was 11 days (range 8–19) by 10.6 × 106 ± 5.4 CD34+ cells/kg with G-CSF use (8). In a study with CD34+ positive selection method (majority by CliniMACS) for myeloablative haploidentical HCT in pediatric malignancy, median time to neutrophil >500 cells/μl was 15 days (range, 8–55) without G-CSF, with a CD34+ cell dose of 12.3 (range, 1.4–95) × 106 cells/kg (26). Similarly, with a median 7.0–9.2 × 106 CD34+ cells/kg neutrophil recovery was 13 days (range, 7–20) in the CD3-/CD19- haploidentical HCT with RIC for pediatric malignancy (14, 60). Likewise, median time to neutrophil >500 cells/μl was 13 days (range, 6–23) without G-CSF in TCRαβ-/CD19-, which was significantly faster than MUD or MMUD by T-replete methods (median 19–20 days) in myeloablative HCT in pediatric malignancy. Notably, the infused CD34+ cell dose was much higher in TCRαβ-/CD19- than in MUD or MMUD (median 14.4 vs. 5.2 vs. 4.9 × 106 cells/kg) (41). In another early-phase clinical trial median neutrophil recovery was also swift (median 10 days, range 9–13) in CD45RA- TCD method which contained median 6.4 × 106 CD34+ cells/kg (45). Together, it has been reported consistently that TCD haploidentical HCT, which commonly utilized G-CSF-mobilized PBSC, has early neutrophil recovery comparable to matched donor PBSC. PTCY with RIC conditioning using BM graft was shown to attain neutrophil recovery was 15 days (range 11–42) (46).
NK cells
NK cells are another component of innate immunity that contributes to immune reconstitution in the first month after HCT. NK cells play critical anti-tumor and anti-infection roles in the haploidentical TCD HCT where potent donor T cell-mediated effects are absent. NK cells are potentially preferable to donor T cells because of lower alloreactivity, albeit with conflicting data (30). NK cells develop self-tolerance (i.e., “licensed NK cells”) when their inhibitory surface receptor [i.e., killer-cell Ig-like receptor (KIR)] encounter matching HLA-class I ligand. These licensed NK cells can elicit potent activity against leukemia cells that do not express matching ligand (i.e., KIR receptor-ligand mismatch) (61–63). In pediatric leukemia patients undergoing haploidentical HCT NK cell reconstitution was faster in CD3-/CD19- as compared to CD34+ selection (350 vs. 180 cells/μl, respectively, on Day +60) (31), which reflects depletion of NK cells from the graft in the latter but not in the former. Similarly, TCRαβ-/CD19- method also retains NK cells in the graft and attains comparably fast reconstitution (approximately 250 cells/μl at Day 30) (64, 65). NK cell recovery is delayed in haploidentical HCT using PTCY because of elimination of donor NK cells by PTCY administration after initial proliferation. Normal NK phenotype is recovered at 9 to 12 months post HCT (66). This contributes to suppressed GVL by donor NK cells in PTCY setting.
T cells
Reconstitution of protective donor T cell function without alloreactivity is a hallmark of a successful allogeneic HCT. Higher CD3+, CD4+, or CD8+ T cells at Day +100 are associated with favorable NRM and OS (12, 67). Recently a milestone of 50 CD4 T cells/μl was defined as a predictor of improved OS, NRM and DFS in pediatric and young adult recipients of conventional TCD, and cord blood transplant (12, 28). In this study, recipients of TCD HCT less frequently attained this target CD4+ count.
Salzmann-Manrique et al. developed a mathematical model and predicted reconstitution of CD3, CD4, CD8 cells in myeloablative HCT with combined ex vivo and in vivo TCD. The predicted T cell counts at Day +90 by CD34+ vs. CD3-/CD19- methods were 154 vs. 135 cells/μl for CD3+, 31 vs. 44 cells/μl for CD4+, and 67 vs. 89 cells/μl for CD8+, respectively (59). These T cell counts correspond to 3%–16% of the counts in the age-matched healthy controls. Their infused CD3+ cell doses were 1.0 vs. 8.9 × 103 cells/kg respectively. Better T cell reconstitution was reported by Bertaina et al. with TCRαβ-/CD19- TCD combined with in vivo TCD in 98 children with pediatric malignancy (41). In comparison to CD34+ or CD3-/CD19- technique, this method results in a 3–4 log higher infused dose of γδ+ T cell with minimal αβ+ T cells (8.1 and 0.04 × 106 cells/kg respectively). At 3 months post TCRαβ-/CD19- HCT, mean CD3+ count was approximately 400 cells/μl, CD4+ was 100 cells/μl, and CD8+ was 250 cells/μl. In comparison to T cell replete URD HCT, CD3+/CD4+/CD8+ cell counts were all lower 30 days after TCRαβ-/CD19- HCT, but comparable counts were achieved by Day +180 for CD4+, and Day +365 for CD3+ and CD8+ cells. Moreover, detailed immunology analysis in TCRαβ-/CD19- by Airoldi et al. revealed that peripherally expanded infused γδ T cells are the predominant T-cell population in patients during the first weeks after HCT (91.5% of CD3+ cells) (68). Triplett et al. reported even faster T cell reconstitution and higher CD3+ cell dose in their experience with CD45RA- HCT (69). Their TCD protocol is also among rare TCD methods that do not include in vivo TCD potentially eliminating the risk of prolonged exposure to serotherapy impairing graft derived immune reconstitution. With the infusion of a median of 122 × 106 CD3+ cells/kg mean CD4+ count was approximately 150 cells/μl by Day +30 and 200 cells/μl by Day +90 in these patients. Collectively these studies illustrate that selectively depleting only subsets of T cells (i.e., TCDαβ+ or CD45RA+ cells), can translate into more rapid immune reconstitution. In haploidentical HCT with PTCY, proliferating donor T cells are depleted by infusion of PTCY, except for regulatory T cells, which are resistant to cyclophosphamide because of higher expression of detoxifying enzyme, aldehyde dehydrogenase. Without mature donor T cells, naive T cell-driven immune reconstitution is slow and T cell counts may be undetectable up to 6 weeks post HCT (70), the period with MMF in the typical PTCY regimen. In contrast regulatory T cells continue to expand and achieve the normal donor level at day 30 (71).
B cells
The B cell population is the slowest to reconstitute after HCT, and may take a few years to reach the normal levels. In Salzmann-Manrique's mathematical model CD19+ B cells in CD34+ selected pediatric myeloablative HCT are predicted to start recovering at Day +60 (45 cells/μl) and subsequently plateau from Day +90 through Day +365 (92–105 cells/μl). In contrast, B cell recovery was slower in recipients of HCT with the CD3-/CD19- method; CD19+ B cells stayed at 20–40 cells/μl from Day +60 to +365. However, in a multicenter study with TCD by TCRαβ-/CD19-, despite use of rituximab on Day -1, Bertaina et al. reported a faster recovery to approximately 80 cells/μl at Day +90 and, by Day +365, reached a level that is comparable to MUD and MMUD (approximately 400 cell/μl) (41). PTCY approaches tend to have relatively fast B cell recovery by day 49 to 77 post HCT (71, 72); however, most of these B cells are naive up to day 180 (72).
Haploidentical HCT as a platform for adoptive cell therapy
Overall
As regimens for ex vivo TCD-based haploidentical HCT require minimal post-transplant immune suppression (26, 64), this approach can provide an ideal platform for posttransplant cell therapy. As one of the major complications of ex vivo TCD HCT is delayed immune reconstitution, an appealing solution is the adoptive transfer of antigen specific or otherwise tolerized populations of T cells. Donors of haploidentical HCT (i.e., family members) are readily available for repeated apheresis for either planned or need-based post-HCT cellular therapies. Thus, various cellular therapy approaches have been explored in the setting of TCD haploidentical HCT.
Unmanipulated T cell addback
Addback of donor T cells after HCT has been investigated as a means to overcome delayed immune reconstitution and resultant high risk of fatal infection. When added to a CD34+ TCD haploidentical HCT, a Phase 1 study in adults using prophylactic DLI at a dose of 3 × 104 T cells/kg resulted in an unacceptably high rate of acute GvHD (73), but more promising in a pilot study, Oved and colleagues demonstrated that targeted add-back to achieve a dose of 1 × 105 T cells/kg with a CD3-/CD19- TCD graft was feasible and associated with acceptable incidence of GvHD (74). Alternatively, delaying infusion of T cell add-back has been explored (73, 75). Gilman et al. reported on a phase 1/2 study of 5 × 104 haploidentical T-cells/kg administered 30–42 days after transplant and followed by methotrexate with or without rituximab in pediatric recipients of CD34+ TCD haploidentical HCT. The study met the primary objective with 67% of patients having CD4+ cells >100/μl by Day +120 with acceptable rates of GvHD (Grade 2–3 11%, no Grade 4 GvHD, and 16% chronic). Central to this approach is the role that acute tissue injury induced by conditioning plays in the induction of GvHD (76). Delaying the adoptive transfer of allogeneic T cells can decrease the inflammatory stimulus that these T cells encounter. Additionally, this approach avoids exposing the adoptively transferred T cells to residual effects of serotherapy (e.g., ATG or alemtuzumab) that are commonly utilized in TCD-HCT (77, 78).
DLI with safety switch
In order to minimize the risk of GvHD associated with infusion of HLA disparate T cells, genetic engineering of donor T cells with suicide genes enables a selective in vivo depletion of donor T cells if GvHD develops.
T cells transduced with the herpes simplex virus thymidine kinase (HSVtk) can be eliminated in the event of GvHD by administration of ganciclovir, which is intracellularly converted by HSVtk to a toxic metabolite and induces arrest of DNA synthesis and subsequent cell death. A limitation of this method is the cell-cycle specificity; this ganciclovir-induced HSVtk-T cell depletion is restricted to the cells in proliferation cycle, which may allow prolonged survival of cells in the other cell-cycles. Nonetheless, the safety and activity of this method were shown in a phase 1/2 study for adults who underwent CD34+ TCD haploidentical HCT after myeloablative conditioning with ATG. No post-HCT GvHD was given. Patients received monthly doses of 106 -107/kg of HSVtk T cells starting Day +28. Twenty-two of the 28 patients (79%) attained T cell count >100 cells/μl at median of 23 days (range 13–42) after HSVtk T cell infusion. These T cells included antigen-specific T cells against CMV and EBV. Administration of gancyclovir successfully controlled all patients who developed acute (n = 10) or chronic (n = 1) GvHD.
More recently, the inducible caspase 9 (iCasp9) system has been explored. The iCasp9 transgene consists of a drug-binding domain and a linker to human caspase 9. Caspase 9 is activated by administration of AP1903, a small molecule that specifically binds to this receptor, and subsequently induces apoptosis of the transduced cells. Unlike HSVtk, activation of the iCasp9 safety switch is cell-cycle independent and can eliminate the target cells rapidly. Haploidentical HCT with T cells transduced with iCasp9 has been investigated by Brenner et al. at Baylor College of Medicine (Houston TX USA). In their initial clinical experience, 5 children with relapsed leukemia underwent haploidentical HCT with iCasp9-transduced T cell infusion. A single dose of AP1903, given to four patients with active GvHD, eliminated >90% of the modified T cells within 30 min after administration and resolved their GvHD without recurrence. The group later reported the sustainability of iCasp9-engineered T cells in a larger cohort of pediatric haploidentical HCT recipients (79). iCasp9 T cells continued to expand after the infusion, peaking at 9 months, and persisted for at least 2 years (80).
Elimination of alloreactive donor T cells
Another strategy to augment the T cell repertoire after CD34+ TCD haploidentical HCT without an unacceptably high risk of GvHD is ex vivo depletion of alloreactive T cells. After co-culture of donor T cells with irradiated recipient PBMCs two approaches to depletion have been explored. The first uses anti-CD25 antibody-toxin conjugate (81, 82) to remove CD25+ activated T cells. This technique successfully reduced the proportion of alloreactive T cells to <1% with a low risk of Grade 2–4 acute GvHD (0%–13%), and early reconstitution of CMV and EBV specific immunity was achieved. The second approach pioneered by Roy et al. depends on selective uptake by activated T cells of the photosensitizer TH9402 (83, 84). In their phase 1 single-center and phase 2 multicenter dose-escalation studies in adults, photo-depleted donor T cells were infused at a median of 28 days post HCT. None of the patients developed grade 3–4 acute GvHD and TRM at 1 year was lower than that in a historical cohort of recipients of CD34+ haploidentical TCD (32.2 vs. 70.3%) (83). A phase 3 randomized control trial is currently underway comparing haploidentical HCT with photodepleted T cells to T-cell replete PTCY transplant platform (NCT02999854).
Induction of anergy by blocking B7/CD28 signaling in potentially alloreactive donor T cells is another approach to minimizing the risk of GvHD. Blockade of B7 interactions by either anti-B7-antibody or a CTLA4-Ig fusion protein can induce T cell anergy in an ex vivo culture. A phase 1 dose-escalation study with administration Day +35 - Day +42 after haploidentical HCT demonstrated earlier (2–3 months vs. 9 months) reconstitution of functional virus-specific immunity at higher (103–104/kg) vs. lower (102/kg) dose levels. However, Grade 2–4 GvHD developed in 5 of 12 patients at the high doses compared to 0 of 4 at the lower dose (85). Another trial used CTLA4-Ig-induced anergy in 12 children and young adults. All 5 surviving patients attained CD4 count >400/μl by 6 months but GI acute GvHD developed in 3 patients (86). Subsequently, these authors identified expansion of regulatory T cells as potentially contributing to their results (87). Most recently, CTLA-4-Ig blockade has been approved as prophylaxis in the setting of conventional URD HCT. Collectively, anergy induction remains a promising strategy for GvHD prophylaxis.
Antigen-specific donor T cells
Infusion of selected subsets of T cells has been explored to provide more tailored T cell addback. Prophylactic or pre-emptive infusion of ex vivo engineered antigen-specific donor T cells can enhance immunity against specific infectious pathogens or leukemia cells (88–93). However, donor-recipient HLA mismatch can limit the efficacy of this approach due to the constraints of immunodominance. When antigen-specific donor T cells are restricted by HLA alleles not shared by the recipient, the donor T cells will not recognize recipient cells infected with the target virus or leukemia cells derived from the recipient. That said, haploidentical donor derived virus-specific T cells have been shown to be effective against various pathogens (e.g., CMV, EBV, adenovirus, Aspergillus) with a minimal risk of GvHD (89, 90, 93). In contrast clinical use of leukemia-specific T cells recognizing tumor associated antigens has not been as successful, likely because these cells are low frequency and predominantly naive making ex vivo expansion difficult. Nonetheless, approaches for generating tumor specific T cells from HLA identical and non-identical donors are being developed. After HLA-matched HCT, promising activity was shown for WT-1 specific donor T cells (Wilms tumor antigen 1). In preclinical studies Wiebking et al. developed a system for generating haploidentical donor derived, TCR knock-out, CD19-specific chimeric antigen T cells (94).
Optimizing regimens
Delayed CD4 cell reconstitution with higher ATG exposure post HCT infusion has been investigated by Boelens and Admiraal et al. in various types of HCT in children using ATG (28). Subsequently, individualized ATG dosing regimen informed by the previous study was tested prospectively in pediatric URD HCT and showed improved early immune reconstitution (95). Similar exposure-response correlation was reported in CD34+ TCD by the same group (96). The risk for delayed CD4 reconstitution was shown with higher ATG exposure post HCT [>100 arbitrary unit per day/milliliter (AU·day/ml)] in BM and PBSC (28), while the thresholds were much lower in UCB and CD34+ TCD (>20 and >30 AU·day/ml) (28, 96). Conversely higher ATG exposure resulted in lower risk of acute and chronic GvHD (28, 97). Another group in Japan reported a similar positive correlation between day 0 ATG exposure and risk of acute GvHD in a myeloablative haploidentical regimen (98).
Future directions and conclusion
Despite the remarkable improvement in outcomes of TCD haploidentical HCT, further research is needed to provide more potent anti-leukemia immunotherapy for very high-risk malignancy that has an ominous prognosis with conventional therapeutic options. As reviewed, novel approaches that can capitalize on the haploidentical platform, especially given that many recipients do not require pharmacologic GvHD prophylaxis in the post grafting period, are being developed. In contrast regimens with less therapy-related toxicity would be desired in patients with lower-risk of relapse. In addition TCD haploidentical HCT can potentially be offered to very different group of patients, solid organ transplant recipients. Minimal GvHD risk without posttransplant prophylaxis can make TCRαβ- TCD an ideal regimen when combined HCT/solid organ transplant from the same haploidentical donor is pursued for induction of long-term tolerance in the transplanted solid organ (99).
In summary, an iterative progress in tailored graft engineering has made TCD haploidentical HCT a more optimized therapeutic modality. Current trials are exploring approaches to improve post-HCT immune reconstitution.
With its inherent advantage in donor accessibility, this achievement has resulted in a paradigm shift in donor selection for pediatric malignancy, where haploidentical donors can now be considered as a favorable option for the majority of patients in need of HCT. In particular, the low risk of severe chronic GvHD in TCD HCT is important in children to prevent life-long consequences. TCD haploidentical HCT also offers an ideal platform for various post-HCT adoptive cellular therapy to either overcome and/or augment its effects.
Author contributions
TT and SEP generated the initial outline TT drafted the manuscript TT and SEP edited the manuscript. All authors contributed to the article and approved the submitted version.
Conflict of interest
SEP receives support for the conduct of sponsored trials from Atara Biotherapeutics, Jasper and AlloVir. Has served as a consultant to CellEvolve, Smartimmune and ADMA. Has generated IP related to the use of adoptive cell therapy for EBV and CMV with all rights assigned to Memorial Sloan Kettering Cancer Center.
Publisher's note
All claims expressed in this article are solely those of the authors and do not necessarily represent those of their affiliated organizations, or those of the publisher, the editors and the reviewers. Any product that may be evaluated in this article, or claim that may be made by its manufacturer, is not guaranteed or endorsed by the publisher.
Supplementary material
The Supplementary Material for this article can be found online at: https://www.frontiersin.org/articles/10.3389/fped.2022.987220/full#supplementary-material.
References
1. D'souza A, Fretham C, Lee SJ, Arora M, Brunner J, Chhabra S. Current use of and trends in hematopoietic cell transplantation in the United States. Biol Blood Marrow Transplant. (2020) 26(8):e177–82. doi: 10.1016/j.bbmt.2020.04.013
2. Delaney C, Gutman JA, Appelbaum FR. Cord blood transplantation for haematological malignancies: conditioning regimens double cord transplant and infectious complications. Br J Haematol. (2009) 147(2):207–16. doi: 10.1111/j.1365-2141.2009.07782.x
3. Zhang MJ, Davies SM, Camitta BM, Logan B, Tiedemann K, Eapen M. Comparison of outcomes after HLA-matched sibling and unrelated donor transplantation for children with high-risk acute lymphoblastic leukemia. Biol Blood Marrow Transplant. (2012) 18(8):1204–10. doi: 10.1016/j.bbmt.2012.01.007
4. Gragert L, Eapen M, Williams E, Freeman J, Spellman S, Baitty R. HLA Match likelihoods for hematopoietic stem-cell grafts in the U.S. Registry. N Engl J Med. (2014) 371(4):339–48. doi: 10.1056/NEJMsa1311707
5. Ho VT, Soiffer RJ. The history and future of T-cell depletion as graft-versus-host disease prophylaxis for allogeneic hematopoietic stem cell transplantation. Blood. (2001) 98(12):3192–204. doi: 10.1182/blood.V98.12.3192
6. Beatty PG, Clift RA, Mickelson EM, Nisperos BB, Flournoy N, Martin PJ. Marrow transplantation from related donors other than HLA-identical siblings. N Engl J Med. (1985) 313(13):765–71. doi: 10.1056/nejm198509263131301
7. Falk PM, Herzog P, Lubens R, Wimmer RS, Sparkes R, Naiman JL. Bone marrow transplantation between a histocompatible parent and child for acute leukemia. Transplantation. (1978) 25(2):88–90. doi: 10.1097/00007890-197802000-00011
8. Aversa F, Tabilio A, Velardi A, Cunningham I, Terenzi A, Falzetti F. Treatment of high-risk acute leukemia with T-cell–depleted stem cells from related donors with one fully mismatched HLA haplotype. N Engl J Med. (1998) 339(17):1186–93. doi: 10.1056/nejm199810223391702
9. Luznik L, Pasquini MC, Logan B, Soiffer RJ, Wu J, Devine SM. Randomized phase III BMT CTN trial of calcineurin inhibitor–free chronic graft-versus-host disease interventions in myeloablative hematopoietic cell transplantation for hematologic malignancies. J Clin Oncol. (2022) 40(4):356–68. doi: 10.1200/jco.21.02293
10. Hoare RL, Veys P, Klein N, Callard R, Standing JF. Predicting CD4 T-cell reconstitution following pediatric hematopoietic stem cell transplantation. Clin Pharmacol Ther. (2017) 102(2):349–57. doi: 10.1002/cpt.621
11. Kleinschmidt K, Lv M, Yanir A, Palma J, Lang P, Eyrich M. T-Cell-Replete versus ex vivo T-cell-depleted haploidentical haematopoietic stem cell transplantation in children with acute lymphoblastic leukaemia and other haematological malignancies. Front Pediatr. (2021) 9:794541. doi: 10.3389/fped.2021.794541
12. Van Roessel I, Prockop S, Klein E, Boulad F, Scaradavou A, Spitzer B. Early CD4+ T cell reconstitution as predictor of outcomes after allogeneic hematopoietic cell transplantation. Cytotherapy. (2020) 22(9):503–10. doi: 10.1016/j.jcyt.2020.05.005
13. Wagner JE, Thompson JS, Carter SL, Kernan NA. Effect of graft-versus-host disease prophylaxis on 3-year disease-free survival in recipients of unrelated donor bone marrow (T-cell depletion trial): a multi-centre randomised phase II–III trial. Lancet. (2005) 366(9487):733–41. doi: 10.1016/S0140-6736(05)66996-6
14. Diaz MA, Pérez-Martínez A, Herrero B, Deltoro N, Martinez I, Ramirez M. Prognostic factors and outcomes for pediatric patients receiving an haploidentical relative allogeneic transplant using CD3/CD19-depleted grafts. Bone Marrow Transplant. (2016) 51(9):1211–6. doi: 10.1038/bmt.2016.101
15. van Dicke KAHJI, van Bekkum DW. The selective elimination of immunologically competent cells from bone marrow and lymphatic cell mixtures. II. Mouse spleen cell fractionation on a discontinuous albumin gradient. Transplantation. (1968) 6(4):562–70. doi: 10.1097/00007890-196807000-00009
16. Pai SY, Logan BR, Griffith LM, Buckley RH, Parrott RE, Dvorak CC. Transplantation outcomes for severe combined immunodeficiency 2000–2009. N Engl J Med. (2014) 371(5):434–46. doi: 10.1056/NEJMoa1401177
17. Buckley RH, Schiff SE, Schiff RI, Markert ML, Williams LW, Roberts JL. Hematopoietic stem-cell transplantation for the treatment of severe combined immunodeficiency. N Engl J Med. (1999) 340(7):508–16. doi: 10.1056/nejm199902183400703
18. O'reilly RJ, Keever C, Kernan NA, Brochstein J, Collins N, Flomenberg N. HLA Nonidentical T cell depleted marrow transplants: a comparison of results in patients treated for leukemia and severe combined immunodeficiency disease. Transplant Proc. (1987) 19(6 Suppl 7):55–60.
19. Kernan NA, Flomenberg N, Dupont B, O'Reilly RJ. Graft rejection in recipients of T-cell-depleted HLA-nonidentical marrow transplants for leukemia. Identification of host-derived antidonor allocytotoxic T lymphocytes. Transplantation. (1987) 43(6):842–7. doi: 10.1097/00007890-198743060-00014
20. Reisner Y, Martelli MF. Bone marrow transplantation across HLA barriers by increasing the number of transplanted cells. Immunol Today. (1995) 16(9):437–40. doi: 10.1016/0167-5699(95)80021-2
21. Rachamim N, Gan J, Segall H, Krauthgamer R, Marcus H, Berrebi A. Tolerance induction by “megadose” hematopoietic transplants: donor-type human CD34 stem cells induce potent specific reduction of host anti-donor cytotoxic T lymphocyte precursors in mixed lymphocyte culture. Transplantation. (1998) 65(10):1386–93. doi: 10.1097/00007890-199805270-00017
22. Gur H, Krauthgamer R, Berrebi A, Klein T, Nagler A, Tabilio A. Tolerance induction by megadose hematopoietic progenitor cells: expansion of veto cells by short-term culture of purified human CD34(+) cells. Blood. (2002) 99(11):4174–81. doi: 10.1182/blood.v99.11.4174
23. Aversa F, Tabilio A, Terenzi A, Velardi A, Falzetti F, Giannoni C. Successful engraftment of T-cell-depleted haploidentical “three-loci” incompatible transplants in leukemia patients by addition of recombinant human granulocyte colony-stimulating factor-mobilized peripheral blood progenitor cells to bone marrow inoculum. Blood. (1994) 84(11):3948–55. doi: 10.1182/blood.V84.11.3948.bloodjournal84113948
24. Schumm M, Lang P, Taylor G, Kuçi S, Klingebiel T, Bühring HJ. Isolation of highly purified autologous and allogeneic peripheral CD34 + cells using the CliniMACS device. J Hematother. (1999) 8(2):209–18. doi: 10.1089/106161299320488
25. Aversa F, Terenzi A, Tabilio A, Falzetti F, Carotti A, Ballanti S. Full haplotype-mismatched hematopoietic stem-cell transplantation: a phase II study in patients with acute leukemia at high risk of relapse. J Clin Oncol. (2005) 23(15):3447–54. doi: 10.1200/jco.2005.09.117
26. Klingebiel T, Cornish J, Labopin M, Locatelli F, Darbyshire P, Handgretinger R. Results and factors influencing outcome after fully haploidentical hematopoietic stem cell transplantation in children with very high-risk acute lymphoblastic leukemia: impact of center size: an analysis on behalf of the acute leukemia and pediatric disease working parties of the European blood and marrow transplant group. Blood. (2010) 115(17):3437–46. doi: 10.1182/blood-2009-03-207001
27. Aversa F, Bachar-Lustig E, Or-Geva N, Prezioso L, Bonomini S, Manfra I. Immune tolerance induction by nonmyeloablative haploidentical HSCT combining T-cell depletion and posttransplant cyclophosphamide. Blood Advances. (2017) 1(24):2166–75. doi: 10.1182/bloodadvances.2017009423
28. Admiraal R, Van Kesteren C, Jol-Van Der Zijde CM, Lankester AC, Bierings MB, Egberts TC. Association between anti-thymocyte globulin exposure and CD4 + immune reconstitution in paediatric haemopoietic cell transplantation: a multicentre retrospective pharmacodynamic cohort analysis. Lancet Haematol. (2015) 2(5):e194–203. doi: 10.1016/s2352-3026(15)00045-9
29. De Koning C, Prockop S, Van Roessel I, Kernan N, Klein E, Langenhorst J. CD4+ T-cell reconstitution predicts survival outcomes after acute graft-versus-host-disease: a dual-center validation. Blood. (2021) 137(6):848–55. doi: 10.1182/blood.2020007905
30. Simonetta F, Alvarez M, Negrin RS. Natural killer cells in graft-versus-host-disease after allogeneic hematopoietic cell transplantation. Front Immunol. (2017) 8:465. doi: 10.3389/fimmu.2017.00465
31. Gonzalez-Vicent M, Perez A, Abad L, Sevilla J, Ramirez M, Diaz MA. Graft manipulation and reduced-intensity conditioning for allogeneic hematopoietic stem cell transplantation from mismatched unrelated and mismatched/haploidentical related donors in pediatric leukemia patients. J Pediatr Hematol Oncol. (2010) 32(3):e85–90. doi: 10.1097/MPH.0b013e3181cf813c
32. Diamond DJ, Chang KL, Jenkins KA, Forman SJ. Immunohistochemical analysis of T cell phenotypes in patients with graft-versus-host disease following allogeneic bone marrow transplantation. Transplantation. (1995) 59(10):1436–44. doi: 10.1097/00007890-199505270-00014
33. Norton J, Al-Saffar N, Sloane JP. Immunohistological study of distribution of gamma/delta lymphocytes after allogeneic bone marrow transplantation. J Clin Pathol. (1992) 45(11):1027–8. doi: 10.1136/jcp.45.11.1027
34. Kawanishi Y, Passweg J, Drobyski WR, Rowlings P, Cook-Craig A, Casper J. Effect of T cell subset dose on outcome of T cell-depleted bone marrow transplantation. Bone Marrow Transplant. (1997) 19(11):1069–77. doi: 10.1038/sj.bmt.1700807
35. Drobyski WR, Majewski D, Hanson G. Graft-facilitating doses of ex vivo activated gammadelta T cells do not cause lethal murine graft-vs.-host disease. Biol Blood Marrow Transplant. (1999) 5(4):222–30. doi: 10.1053/bbmt.1999.v5.pm10465102
36. Chaleff S, Otto M, Barfield RC, Leimig T, Iyengar R, Martin J. A large-scale method for the selective depletion of alphabeta T lymphocytes from PBSC for allogeneic transplantation. Cytotherapy. (2007) 9(8):746–54. doi: 10.1080/14653240701644000
37. Handgretinger R. Negative depletion of CD3(+) and TcRαβ(+) T cells. Curr Opin Hematol. (2012) 19(6):434–9. doi: 10.1097/MOH.0b013e3283582340
38. Bertaina A, Romano M, Rutella S, Palumbo G, Pagliara D, Ceccarelli S. HLA Haploidentical stem cell transplantation after removal of αβ+ T lymphocytes and B lymphocytes is an effective treatment for children with life-threatening non-malignant disorders. Blood. (2012) 120(21):2018. doi: 10.1182/blood.V120.21.2018.2018
39. Lang P, Feuchtinger T, Teltschik HM, Schwinger W, Schlegel P, Pfeiffer M. Improved immune recovery after transplantation of TCRαβ/CD19-depleted allografts from haploidentical donors in pediatric patients. Bone Marrow Transplant. (2015) 50(2):S6–S10. doi: 10.1038/bmt.2015.87
40. Bertaina A, Merli P, Rutella S, Pagliara D, Bernardo ME, Masetti R. HLA-haploidentical stem cell transplantation after removal of αβ+ T and B cells in children with nonmalignant disorders. Blood. (2014) 124(5):822–6. doi: 10.1182/blood-2014-03-563817
41. Bertaina A, Zecca M, Buldini B, Sacchi N, Algeri M, Saglio F. Unrelated donor vs HLA-haploidentical α/β T-cell– and B-cell–depleted HSCT in children with acute leukemia. Blood. (2018) 132(24):2594–607. doi: 10.1182/blood-2018-07-861575
42. Anderson BE, Mcniff J, Yan J, Doyle H, Mamula M, Shlomchik MJ. Memory CD4+ T cells do not induce graft-versus-host disease. J Clin Invest. (2003) 112(1):101–8. doi: 10.1172/JCI17601
43. Bleakley M, Heimfeld S, Jones LA, Turtle C, Krause D, Riddell SR. Engineering human peripheral blood stem cell grafts that are depleted of naive T cells and retain functional pathogen-specific memory T cells. Biol Blood Marrow Transplant. (2014) 20(5):705–16. doi: 10.1016/j.bbmt.2014.01.032
44. Bleakley M, Sehgal A, Seropian S, Biernacki MA, Krakow EF, Dahlberg A. Naive T-cell depletion to prevent chronic graft-versus-host disease. J Clin Oncol. (2022) 40(11):1174–85. doi: 10.1200/jco.21.01755
45. Triplett BM, Shook DR, Eldridge P, Li Y, Kang G, Dallas M. Rapid memory T-cell reconstitution recapitulating CD45RA-depleted haploidentical transplant graft content in patients with hematologic malignancies. Bone Marrow Transplant. (2015) 50(7):968–77. doi: 10.1038/bmt.2014.324
46. Luznik L, O'donnell PV, Symons HJ, Chen AR, Leffell MS, Zahurak M. HLA-haploidentical bone marrow transplantation for hematologic malignancies using nonmyeloablative conditioning and high-dose posttransplantation cyclophosphamide. Biol Blood Marrow Transplant. (2008) 14(6):641–50. doi: 10.1016/j.bbmt.2008.03.005
47. Ciurea SO, Zhang MJ, Bacigalupo AA, Bashey A, Appelbaum FR, Aljitawi OS. Haploidentical transplant with posttransplant cyclophosphamide vs matched unrelated donor transplant for acute myeloid leukemia. Blood. (2015) 126(8):1033–40. doi: 10.1182/blood-2015-04-639831
48. Solomon SR, Sizemore CA, Sanacore M, Zhang X, Brown S, Holland HK. Haploidentical transplantation using T cell replete peripheral blood stem cells and myeloablative conditioning in patients with high-risk hematologic malignancies who lack conventional donors is well tolerated and produces excellent relapse-free survival: results of a prospective phase II trial. Biol Blood Marrow Transplant. (2012) 18(12):1859–66. doi: 10.1016/j.bbmt.2012.06.019
49. Raiola AM, Dominietto A, Ghiso A, Di Grazia C, Lamparelli T, Gualandi F. Unmanipulated haploidentical bone marrow transplantation and posttransplantation cyclophosphamide for hematologic malignancies after myeloablative conditioning. Biol Blood Marrow Transplant. (2013) 19(1):117–22. doi: 10.1016/j.bbmt.2012.08.014
50. Shah RM. Contemporary haploidentical stem cell transplant strategies in children with hematological malignancies. Bone Marrow Transplant. (2021) 56(7):1518–34. doi: 10.1038/s41409-021-01246-5
51. Ruggeri A, Galimard JE, Paina O, Fagioli F, Tbakhi A, Yesilipek A. Outcomes of unmanipulated haploidentical transplantation using post-transplant cyclophosphamide (PT-cy) in pediatric patients with acute lymphoblastic leukemia. Cell Ther Transplant. (2021) 27(5):424.e1–e9. doi: 10.1016/j.jtct.2021.01.016
52. Chang Y-J, Wang Y, Xu L-P, Zhang X-H, Chen H, Chen Y-H. Haploidentical donor is preferred over matched sibling donor for pre-transplantation MRD positive ALL: a phase 3 genetically randomized study. J Hematol Oncol. (2020) 13(1):27. doi: 10.1186/s13045-020-00860-y
53. Gao L, Zhang C, Gao L, Liu Y, Su Y, Wang S. Favorable outcome of haploidentical hematopoietic stem cell transplantation in Philadelphia chromosome-positive acute lymphoblastic leukemia: a multicenter study in southwest China. J Hematol Oncol. (2015) 8(1):90. doi: 10.1186/s13045-015-0186-5
54. Chang Y-J, Zhao X-Y, Huang X-J. Granulocyte colony-stimulating factor-primed unmanipulated haploidentical blood and marrow transplantation. Review. Front Immunol. (2019) 10:2516. doi: 10.3389/fimmu.2019.02516
55. Baumeister SHC, Rambaldi B, Shapiro RM, Romee R. Key aspects of the immunobiology of haploidentical hematopoietic cell transplantation. Front Immunol. (2020) 11:191. doi: 10.3389/fimmu.2020.00191
56. Turcotte LM, Cao Q, Cooley SA, Curtsinger J, Holtan SG, Luo X. Monocyte subpopulation recovery as predictors of hematopoietic cell transplantation outcomes. Biol Blood Marrow Transplant. (2019) 25(5):883–90. doi: 10.1016/j.bbmt.2019.01.003
57. Le Bourgeois A, Peterlin P, Guillaume T, Delaunay J, Duquesne A, Le Gouill S. Higher early monocyte and total lymphocyte counts are associated with better overall survival after standard total body irradiation cyclophosphamide and fludarabine reduced-intensity conditioning double umbilical cord blood allogeneic stem cell transplantation in adults. Biol Blood Marrow Transplant. (2016) 22(8):1473–9. doi: 10.1016/j.bbmt.2016.04.015
58. Lang P, Schumm M, Greil J, Bader P, Klingebiel T, Müller I. A comparison between three graft manipulation methods for haploidentical stem cell transplantation in pediatric patients: preliminary results of a pilot study. Klin Padiatr. (2005) 217(6):334–8. doi: 10.1055/s-2005-872529
59. Salzmann-Manrique E, Bremm M, Huenecke S, Stech M, Orth A, Eyrich M. Joint modeling of immune reconstitution post haploidentical stem cell transplantation in pediatric patients with acute leukemia comparing CD34(+)-selected to CD3/CD19-depleted grafts in a retrospective multicenter study. Front Immunol. (2018) 9:1841. doi: 10.3389/fimmu.2018.01841
60. Pérez-Martínez A, González-Vicent M, Valentín J, Aleo E, Lassaletta A, Sevilla J. Early evaluation of immune reconstitution following allogeneic CD3/CD19-depleted grafts from alternative donors in childhood acute leukemia. Bone Marrow Transplant. (2012) 47(11):1419–27. doi: 10.1038/bmt.2012.43
61. Mancusi A, Ruggeri L, Urbani E, Pierini A, Massei MS, Carotti A. Haploidentical hematopoietic transplantation from KIR ligand–mismatched donors with activating KIRs reduces nonrelapse mortality. Blood. (2015) 125(20):3173–82. doi: 10.1182/blood-2014-09-599993
62. Oevermann L, Michaelis SU, Mezger M, Lang P, Toporski J, Bertaina A. KIR B haplotype donors confer a reduced risk for relapse after haploidentical transplantation in children with ALL. Blood. (2014) 124(17):2744–7. doi: 10.1182/blood-2014-03-565069
63. Ruggeri L, Capanni M, Urbani E, Perruccio K, Shlomchik WD, Tosti A. Effectiveness of donor natural killer cell alloreactivity in mismatched hematopoietic transplants. Science. (2002) 295(5562):2097–100. doi: 10.1126/science.1068440
64. Locatelli F, Merli P, Pagliara D, Li Pira G, Falco M, Pende D. Outcome of children with acute leukemia given HLA-haploidentical HSCT after αβ T-cell and B-cell depletion. Blood. (2017) 130(5):677–85. doi: 10.1182/blood-2017-04-779769
65. Pérez-Martínez A, Ferreras C, Pascual A, Gonzalez-Vicent M, Alonso L, Badell I. Haploidentical transplantation in high-risk pediatric leukemia: a retrospective comparative analysis on behalf of the spanish working group for bone marrow transplantation in children (GETMON) and the spanish grupo for hematopoietic transplantation (GETH). Am J Hematol. (2020) 95(1):28–37. doi: 10.1002/ajh.25661
66. Russo A, Oliveira G, Berglund S, Greco R, Gambacorta V, Cieri N. NK Cell recovery after haploidentical HSCT with posttransplant cyclophosphamide: dynamics and clinical implications. Blood. (2018) 131(2):247–62. doi: 10.1182/blood-2017-05-780668
67. Bondanza A, Ruggeri L, Noviello M, Eikema DJ, Bonini C, Chabannon C. Beneficial role of CD8+ T-cell reconstitution after HLA-haploidentical stem cell transplantation for high-risk acute leukaemias: results from a clinico-biological EBMT registry study mostly in the T-cell-depleted setting. Bone Marrow Transplant. (2019) 54(6):867–76. doi: 10.1038/s41409-018-0351-x
68. Airoldi I, Bertaina A, Prigione I, Zorzoli A, Pagliara D, Cocco C. Γδ T-cell reconstitution after HLA-haploidentical hematopoietic transplantation depleted of TCR-αβ+/CD19 + lymphocytes. Blood. (2015) 125(15):2349–58. doi: 10.1182/blood-2014-09-599423
69. Triplett BM, Muller B, Kang G, Li Y, Cross SJ, Moen J. Selective T-cell depletion targeting CD45RA reduces viremia and enhances early T-cell recovery compared with CD3-targeted T-cell depletion. Transpl Infect Dis. (2018) 20(1). doi: 10.1111/tid.12823
70. Roberto A, Castagna L, Zanon V, Bramanti S, Crocchiolo R, Mclaren JE. Role of naive-derived T memory stem cells in T-cell reconstitution following allogeneic transplantation. Blood. (2015) 125(18):2855–64. doi: 10.1182/blood-2014-11-608406
71. McCurdy SR, Vulic A, Symons HJ, Towlerton AM, Valdez BC, Jones RJ. Comparable and robust immune reconstitution after HLA-haploidentical or HLA-matched allogeneic transplantation (BMT) utilizing posttransplantation cyclophosphamide. Biol Blood Marrow Transplant. (2015) 21(2):S71. doi: 10.1016/j.bbmt.2014.11.075
72. Roberto A, Castagna L, Gandolfi S, Zanon V, Bramanti S, Sarina B. B-cell reconstitution recapitulates B-cell lymphopoiesis following haploidentical BM transplantation and post-transplant CY. Bone Marrow Transplant. (2015) 50(2):317–9. doi: 10.1038/bmt.2014.266
73. Lewalle P, Triffet A, Delforge A, Crombez P, Selleslag D, De Muynck H. Donor lymphocyte infusions in adult haploidentical transplant: a dose finding study. Bone Marrow Transplant. (2003) 31(1):39–44. doi: 10.1038/sj.bmt.1703779
74. Oved JH, Wang Y, Barrett DM, Levy EM, Huang Y, Monos DS. CD3+/CD19+ Depleted matched and mismatched unrelated donor hematopoietic stem cell transplant with targeted T cell addback is associated with excellent outcomes in pediatric patients with nonmalignant hematologic disorders. Biol Blood Marrow Transplant. (2019) 25(3):549–55. doi: 10.1016/j.bbmt.2018.10.003
75. Gilman AL, Leung W, Cowan MJ, Cannon M, Epstein S, Barnhart C. Donor lymphocyte infusion and methotrexate for immune recovery after T-cell depleted haploidentical transplantation. Am J Hematol. (2018) 93(2):169–78. doi: 10.1002/ajh.24949
76. Johnson BD, Truitt RL. Delayed infusion of immunocompetent donor cells after bone marrow transplantation breaks graft-host tolerance allows for persistent antileukemic reactivity without severe graft-versus-host disease. Blood. (1995) 85(11):3302–12. doi: 10.1182/blood.V85.11.3302.bloodjournal85113302
77. Admiraal R, Van Kesteren C, Jol-Van Der Zijde CM, Van Tol MJ, Bartelink IH, Bredius RG. Population pharmacokinetic modeling of thymoglobulin(®) in children receiving allogeneic-hematopoietic cell transplantation: towards improved survival through individualized dosing. Clin Pharmacokinet. (2015) 54(4):435–46. doi: 10.1007/s40262-014-0214-6
78. Admiraal R, Jol-Van Der Zijde CM, Furtado Silva JM, Knibbe CaJ, Lankester AC, Boelens JJ. Population pharmacokinetics of alemtuzumab (campath) in pediatric hematopoietic cell transplantation: towards individualized dosing to improve outcome. Clin Pharmacokinet. (2019) 58(12):1609–20. doi: 10.1007/s40262-019-00782-0
79. Di Stasi A, Tey SK, Dotti G, Fujita Y, Kennedy-Nasser A, Martinez C. Inducible apoptosis as a safety switch for adoptive cell therapy. N Engl J Med. (2011) 365(18):1673–83. doi: 10.1056/NEJMoa1106152
80. Merli P, Bertaina V, Galaverna F, Algeri M, Sinibaldi M, Strocchio L. Donor T cells genetically modified with a novel suicide gene (inducible caspase 9 iC9) expand and persist over time after post-allograft infusion in patients given αβ T-cell and B-cell depleted HLA-haploidentical allogeneic stem cell transplantation (αβ haplo-HSCT) contributing to accelerate immune recovery. Blood. (2017) 130(Supplement 1):211. doi: 10.1182/blood.V130.Suppl_1.211.211
81. Amrolia PJ, Muccioli-Casadei G, Huls HM, Durett A, Weiss H, Rooney CM. Adoptive immunotherapy with allodepleted donor T-cells improves immune reconstitution after haploidentical stem cell transplant. Biol Blood Marrow Transplant. (2006) 12(2 Supplement 1):15–6. doi: 10.1016/j.bbmt.2005.11.051
82. André-Schmutz I, Le Deist F, Hacein-Bey-Abina S, Vitetta E, Schindler J, Chedeville G. Immune reconstitution without graft-versus-host disease after haemopoietic stem-cell transplantation: a phase 1/2 study. Lancet. (2002) 360(9327):130–7. doi: 10.1016/S0140-6736(02)09413-8
83. Roy D, Walker I, Maertens J, Lewalle P, Olavarria E, Selleslag D. 10 - Phase II study of haploidentical stem cell transplantation using ex vivo photodepletion of donor lymphocyte infusions to eliminate anti-host reactivity results in low relapse rates and high survival rates: final 2 year follow-up. Cytotherapy. (2018) 20(5 Supplement):S10–1. doi: 10.1016/j.jcyt.2018.02.016
84. Roy DC, Lachance S, Cohen S, Delisle JS, Kiss T, Sauvageau G. Allodepleted T-cell immunotherapy after haploidentical haematopoietic stem cell transplantation without severe acute graft-versus-host disease (GVHD) in the absence of GVHD prophylaxis. Br J Haematol. (2019) 186(5):754–66. doi: 10.1111/bjh.15970
85. Davies JK, Brennan LL, Wingard JR, Cogle CR, Kapoor N, Shah AJ. Infusion of alloanergized donor lymphocytes after CD34-selected haploidentical myeloablative hematopoietic stem cell transplantation. Clin Cancer Res. (2018) 24(17):4098–109. doi: 10.1158/1078-0432.Ccr-18-0449
86. Guinan EC, Boussiotis VA, Neuberg D, Brennan LL, Hirano N, Nadler LM. Transplantation of anergic histoincompatible bone marrow allografts. N Engl J Med. (1999) 340(22):1704–14. doi: 10.1056/nejm199906033402202
87. Davies JK, Nadler LM, Guinan EC. Expansion of allospecific regulatory T cells after anergized mismatched bone marrow transplantation. Sci Transl Med. (2009) 1(1):1ra3. doi: 10.1126/scitranslmed.3000153
88. Perruccio K, Tosti A, Burchielli E, Topini F, Ruggeri L, Carotti A. Transferring functional immune responses to pathogens after haploidentical hematopoietic transplantation. Blood. (2005) 106(13):4397–406. doi: 10.1182/blood-2005-05-1775
89. Leen AM, Christin A, Myers GD, Liu H, Cruz CR, Hanley PJ. Cytotoxic T lymphocyte therapy with donor T cells prevents and treats adenovirus and epstein-barr virus infections after haploidentical and matched unrelated stem cell transplantation. Blood. (2009) 114(19):4283–92. doi: 10.1182/blood-2009-07-232454
90. Feuchtinger T, Opherk K, Bethge WA, Topp MS, Schuster FR, Weissinger EM. Adoptive transfer of pp65-specific T cells for the treatment of chemorefractory cytomegalovirus disease or reactivation after haploidentical and matched unrelated stem cell transplantation. Blood. (2010) 116(20):4360–7. doi: 10.1182/blood-2010-01-262089
91. Bornhäuser M, Thiede C, Platzbecker U, Kiani A, Oelschlaegel U, Babatz J. Prophylactic transfer of BCR-ABL– PR1- and WT1-reactive donor T cells after T cell–depleted allogeneic hematopoietic cell transplantation in patients with chronic myeloid leukemia. Blood. (2011) 117(26):7174–84. doi: 10.1182/blood-2010-09-308569
92. Chapuis AG, Ragnarsson GB, Nguyen HN, Chaney CN, Pufnock JS, Schmitt TM. Transferred WT1-reactive CD8+ T cells can mediate antileukemic activity and persist in post-transplant patients. Sci Transl Med. (2013) 5(174):174ra27. doi: 10.1126/scitranslmed.3004916
93. Pei X-Y, Zhao X-Y, Chang Y-J, Liu J, Xu L-P, Wang Y. Cytomegalovirus-Specific T-cell transfer for refractory cytomegalovirus infection after haploidentical stem cell transplantation: the quantitative and qualitative immune recovery for cytomegalovirus. J Infect Dis. (2017) 216(8):945–56. doi: 10.1093/infdis/jix357
94. Wiebking V, Lee CM, Mostrel N, Lahiri P, Bak R, Bao G. Genome editing of donor-derived T-cells to generate allogenic chimeric antigen receptor-modified T cells: optimizing αβ T cell-depleted haploidentical hematopoietic stem cell transplantation. Haematologica. (2021) 106(3):847–58. doi: 10.3324/haematol.2019.233882
95. Admiraal R, Nierkens S, Bierings MB, Bredius RGM, Van Vliet I, Jiang Y. Individualised dosing of anti-thymocyte globulin in paediatric unrelated allogeneic haematopoietic stem-cell transplantation (PARACHUTE): a single-arm phase 2 clinical trial. Lancet Haematol. (2022) 9(2):e111–20. doi: 10.1016/s2352-3026(21)00375-6
96. Lakkaraja M, Scordo M, Mauguen A, Cho C, Devlin S, Ruiz JD. Antithymocyte globulin exposure in CD34+ T-cell-depleted allogeneic hematopoietic cell transplantation. Blood Adv. (2022) 6(3):1054–63. doi: 10.1182/bloodadvances.2021005584
97. Admiraal R, Nierkens S, De Witte MA, Petersen EJ, Fleurke GJ, Verrest L. Association between anti-thymocyte globulin exposure and survival outcomes in adult unrelated haemopoietic cell transplantation: a multicentre retrospective pharmacodynamic cohort analysis. Lancet Haematol. (2017) 4(4):e183–91. doi: 10.1016/s2352-3026(17)30029-7
98. Teramoto M, Maruyama S, Tamaki H, Kaida K, Mayumi A, Fukunaga K. Association between the pharmacokinetics of rabbit anti-thymocyte globulin and acute graft-versus-host disease in patients who received haploidentical hematopoietic stem cell transplantation. Int J Hematol. (2022) 116(2):248–57. doi: 10.1007/s12185-022-03342-8
Keywords: immune reconsitition, cellular therapy, cliniMACS, stem cell transplant, children
Citation: Takahashi T and Prockop SE (2022) T-cell depleted haploidentical hematopoietic cell transplantation for pediatric malignancy. Front. Pediatr. 10:987220. doi: 10.3389/fped.2022.987220
Received: 5 July 2022; Accepted: 20 September 2022;
Published: 14 October 2022.
Edited by:
Miguel Angel Diaz, Niño Jesús University Children's Hospital, SpainReviewed by:
Carlos Solano, Hospital Clínico Universitario de Valencia, SpainJakob Passweg, University Hospital of Basel, Switzerland
© 2022 Takahashi and Prockop. This is an open-access article distributed under the terms of the Creative Commons Attribution License (CC BY). The use, distribution or reproduction in other forums is permitted, provided the original author(s) and the copyright owner(s) are credited and that the original publication in this journal is cited, in accordance with accepted academic practice. No use, distribution or reproduction is permitted which does not comply with these terms.
*Correspondence: Susan Prockop c3VzYW4ucHJvY2tvcEBjaGlsZHJlbnMuaGFydmFyZC5lZHU=
Specialty Section: This article was submitted to Pediatric Hematology and Hematological Malignancies, a section of the journal Frontiers in Pediatrics