- 1Chongqing Key Laboratory of Translational Medical Research in Cognitive Development and Learning and Memory Disorders, Ministry of Education Key Laboratory of Child Development and Disorders, National Clinical Research Center for Child Health and Disorders, China International Science and Technology Cooperation Base of Child Development and Critical Disorders, Children's Hospital of Chongqing Medical University, Chongqing, China
- 2Institute of Aging, Key Laboratory of Alzheimer's Disease of Zhejiang Province, Zhejiang Provincial Clinical Research Center for Mental Disorders, School of Mental Health and Kangning Hospital, The Second Affiliated Hospital and Yuying Children's Hospital, Wenzhou Medical University, Wenzhou, China
- 3Oujiang Laboratory (Zhejiang Lab for Regenerative Medicine, Vision and Brain Health), Wenzhou, China
Neuroinflammation is a leading cause of secondary neuronal injury in neonatal hypoxic-ischemic encephalopathy (HIE). Regulation of neuroinflammation may be beneficial for treatment of HIE and its secondary complications. Gallic acid (GA) has been shown to have anti-inflammatory and antioxidant effects. In this report we found that oxygen-glucose deprivation and/reoxygenation (OGD/R)-induced cell death, and the generation of excessive reactive oxygen species (ROS) and inflammatory cytokines by microglia were inhibited by GA treatment. Furthermore, GA treatment reduced neuroinflammation and neuronal loss, and alleviated motor and cognitive impairments in rats with hypoxic-ischemic brain damage (HIBD). Together, our results reveal that GA is an effective regulator of neuroinflammation and has potential as a pharmaceutical intervention for HIE therapy.
Introduction
Neonatal hypoxic-ischemic encephalopathy (HIE) is a cerebral hypoxic-ischemic injury caused by perinatal asphyxia with an incidence of approximately 1–8/1,000 (1). Although the incidence of HIE has decreased as improvements have been made in prenatal and neonatal care, HIE remains the leading cause of neonatal death, with survivors later showing severe neurological sequelae such as cerebral palsy, epilepsy, and cognitive impairment (2–6). Treatment of patients with HIE and its sequelae places a heavy burden on families and society, so identifying more effective ways to improve the quality of life of HIE patients and reducing the incidence of sequelae is urgently needed.
Previous studies have shown that HIE induces primary neuronal death and delayed neuronal death. In HIE, delayed neuronal death in the brain is mainly caused by excitotoxicity, oxidative stress, and inflammation (7). As the main immune cells in the brain, microglial cells are activated rapidly after HIE and produce excessive oxidative reactive products such as reactive oxygen species (ROS) and various proinflammatory cytokines, such as interleukin-1β (IL-1β), interleukin-6 (IL-6), and tumor necrosis factor-α (TNF-α), resulting in neuroinflammation and leading to the destruction of the blood-brain barrier and infiltration of peripheral inflammatory cells into the central nervous system, which aggravates neuroinflammation and subsequent neuronal death (8–10). Therefore, controlling the inflammatory response has become a potential approach for the clinical treatment of HIE.
Gallic acid (GA) is a common polyhydroxy phenolic compound in plants. Studies have shown that GA has anti-inflammatory and antioxidant effects and exerts a neuroprotective effect in different models of neurological disease (11–14), but little is known about its effect on HIE. Since neuroinflammation plays an essential role in the occurrence and development of HIE and its sequelae, we hypothesize that GA can alleviate brain damage in HIE by reducing inflammation. In this study, we found that GA could inhibit microglia-mediated neuroinflammation and oxidative stress after OGD/R and reduce neuroinflammation and neuronal loss to improve motor and cognitive abilities in a hypoxic-ischemic brain damage (HIBD) model.
Materials and methods
Cell culture
BV2 cells were cultured in DMEM containing 10% FBS (Excell Bio, FSP500) and placed in a 37 °C incubator with 5% CO2. GA (MCE, HY-N0523) was dissolved in DMSO to generate a 100 mg/ml stock solution and diluted with PBS to 100 mM before use. To establish a model of oxygen glucose deprivation/reoxygenation (OGD/R), BV2 cells were cultured in a hypoxic incubator and treated with glucose-free DMEM (Gibco, A1443001) with or without GA for 4 h. Afterward, the medium was replaced with normal medium with or without GA, and the cells were placed in a normal incubator for 1 h to simulate the reperfusion process. Control cells were cultured for the same duration under normal conditions.
CCK8 assay
Cell viability was measured by a Cell Counting Kit-8 (MCE, HY-K0301) according to the manufacturer's instructions. BV2 cells were seeded into 96-well plates at an appropriate density, and after treatment, 10 µl of CCK8 solution was added to each well. The absorbance at 450 nm was measured with a microplate reader after incubation for 1 h.
Flow cytometry
ROS levels were measured by flow cytometry. Briefly, cells were collected, incubated with 10 µM DCFH-DA (Beyotime, S0033S) for 20 min, washed with PBS 3 times and then analyzed by flow cytometry.
Real-time quantitative PCR (qPCR)
RNA was extracted using a BioTeke kit (BioTeke, RP1202), and the RNA was then reverse-transcribed into cDNA (Takara, RR047A). TB Green Premix Ex Taq (Takara, RR820A) and gene-specific primers were used to amplify the cDNA. The following primers for mouse were used: β-actin (forward, 5′-ACTGTCGAGTCGCGTCC and reverse 5′-CTGACCCATTCCCACCATCA); IL-1β (forward, 5′-TGCCACCTTTTGACAGTGATG and reverse 5′-ATGTGCTGCTGCGAGATTTG); IL-6 (forward, 5′-GAGCCCACCAAGAACGATAG and reverse 5′-GTTGTCACCAGCATCAGTCC). The following primers for rat were used: β-actin (forward, 5′-GTCCACCCGCGAGTACAACCTTCT and reverse 5′-TCCTTCTGACCCATACCCACCATC); IL-1β (forward, 5′- TGAGGCTGACAGACCCCAAAAGAT and reverse 5′-GCTCCACGGGCAAGACATAGGTAG); IL-6 (forward, 5′-AGCCACTGCCTTCCCTACTTCA and reverse 5′-GCCATTGCACAACTCTTTTCTCA). The data were processed by the 2−ΔΔCT method.
HIBD animal model and treatment
All animal studies were conducted in accordance with the Guide for the Care and Use of Laboratory Animals of the Ethics Committee of Chongqing Medical University. Animals were blindly grouped and analyzed. The experimental procedures were approved by the Animal Study Committee of the Children's Hospital of Chongqing Medical University. Seven-day-old Sprague-Dawley (SD) rats were used to construct the HIBD model, a well-validated animal model (15), as described previously (16). Rats were anesthetized by intraperitoneal injection of sodium pentobarbital at a dose of 30 mg/kg. The left carotid artery of the neonatal rats was ligated, and the rats were then placed in a hypoxic cage (8% O2 + 92% N2) at 37 °C for 2.5 h after resting for approximately 1 h. The rats in the sham group underwent left carotid artery isolation without ligation and hypoxia. After hypoxia exposure, the pups were returned to their home cages and care for by their mothers until day P21. All the rats had free access to water and food and were kept in a constant-temperature room on a 12 h light/12 h dark cycle.
The animals were randomly divided into four groups: the sham + saline, sham + GA, HIBD + saline, and HIBD + GA groups. GA was dissolved in saline to a concentration of 10 mg/mL and injected intraperitoneally at a dose of 50 mg/kg for 14 days. The first injection was performed immediately after construction of the HIBD model (Figure 1).
Rotarod test
Three weeks after HIBD model construction, the rotarod test (16) was performed (Figure 1). Two adaptative training sessions were performed first, and the rats that did not cooperate were excluded. Then, ten consecutive rotarod trials were performed the day after adaptive training. The rotarod was accelerated from 5 r/min to 60 r/min over 3 min, and the time that each rat stayed on the rotarod was recorded. The instrument was cleaned with 75% alcohol after each test.
Grasping test
Two days after the rotarod test, the rats were subjected to the grasping test (16) to evaluate muscle strength (Figure 1). The left or right forelimb of each rat was placed on the grasp meter. Then, the rats were pulled back until they could no longer grasp the meter, and the maximum tension was recorded. The measurement was repeated 10 times for each rat's unilateral forelimb.
Morris water maze (MWM) test
The MWM test (17) was performed after the grasping test to assess the spatial learning and memory of the rats (Figure 1). On the first day, the rats underwent adaptative training, during which they were placed in the water maze and allowed to swim for 60 s. A spatial learning trial was performed on each of the subsequent 5 days; the rats were placed in the water facing the pool wall at staggered entry points (1–3–2–4/4–2–3–1). The time required for the rats to find the platform was recorded. If a rat did not find the platform within 60 s, it was guided to the platform and allowed to stay on it for 10 s. On the last day, a probe test was performed. The hidden platform was removed, and the rats were placed in the water in the quadrant opposite the platform quadrant. The time the rats spent in the platform quadrant and the number of times they crossed the platform location were recorded. All the data were recorded by an Any-maze tracking system.
Immunofluorescence
After the behavioral tests, the rats were anesthetized with urethane, and the left hemisphere of the brain was fixed with 4% paraformaldehyde for several days after transcardial perfusion with 0.9% saline. Thereafter, the brain tissues were dehydrated with 20% and 30% sucrose until it sank to the bottom of the tube. Next, the brain tissues were sectioned into 20 μm slices. Six to nine slices per brain were washed in PBS, treated with blocking solution (Beyotime, P0260) for approximately 30 min and then incubated overnight at 4 °C with anti-NeuN primary antibodies (1:400, Abcam, ab177487). The slices were subsequently incubated with secondary antibodies (1:500, Invitrogen, A21206) for 90 min the next day. Images were taken with an Olympus full-slide scanner, and the number of neurons in the CA1 region was counted with ImageJ.
Statistical analysis
SPSS 19.0 software was used to analyze the data. The data in this study were all quantitative and expressed as the means ± standard errors (SEMs). Student's t test was used for comparisons between two groups, while one-way ANOVA followed by the LSD test was used for comparisons among multiple groups. Differences in data from the spatial learning trials of the MWM test were assessed by two-way repeated-measures ANOVA with matched subjects followed by the LSD multiple comparison post hoc test. P < 0.05 was considered statistically significant.
Results
GA increased the viability of Bv2 cells after OGD/R
To investigate the effect of GA on cell viability, BV2 cells were first subjected to OGD, and then CCK8 assay was performed. The results of the CCK8 assay showed that after 2, 4, 6, and 8 h of OGD, cell viability significantly decreased to 76.19% ± 1.38%, 32.95% ± 5.09%, 18.47% ± 1.43%, and 8.43% ± 2.56%, respectively (Figure 2A). OGD for 4 h was chosen as the optimal condition for GA treatment. In the absence of OGD/R, GA treatment had no effect on cell viability (Figure 2B). However, after 3 h of reoxygenation, treatment with 20, 40 or 80 µM GA markedly increased cell viability to 44.51% ± 2.22%, 45.18% ± 2%, and 41.51% ± 2.76%, respectively from 33% ± 4.96% (Figure 2B). To examine the effect of GA on cell viability after reoxygenation for different durations, cells were treated with 40 µM GA. The cell viability was decreased to 46.18% ± 4.7%, and 62.4% ± 3.93% after 1 h and 3 h of reoxygenation, respectively, and GA significantly increased the survival rate of OGD-treated cells to 52.7% ± 2.27% and 75.04% ± 5.01% after 1 h and 3 h of reoxygenation, respectively (Figure 2C). These data indicated that GA protected BV2 cells from OGD/R.
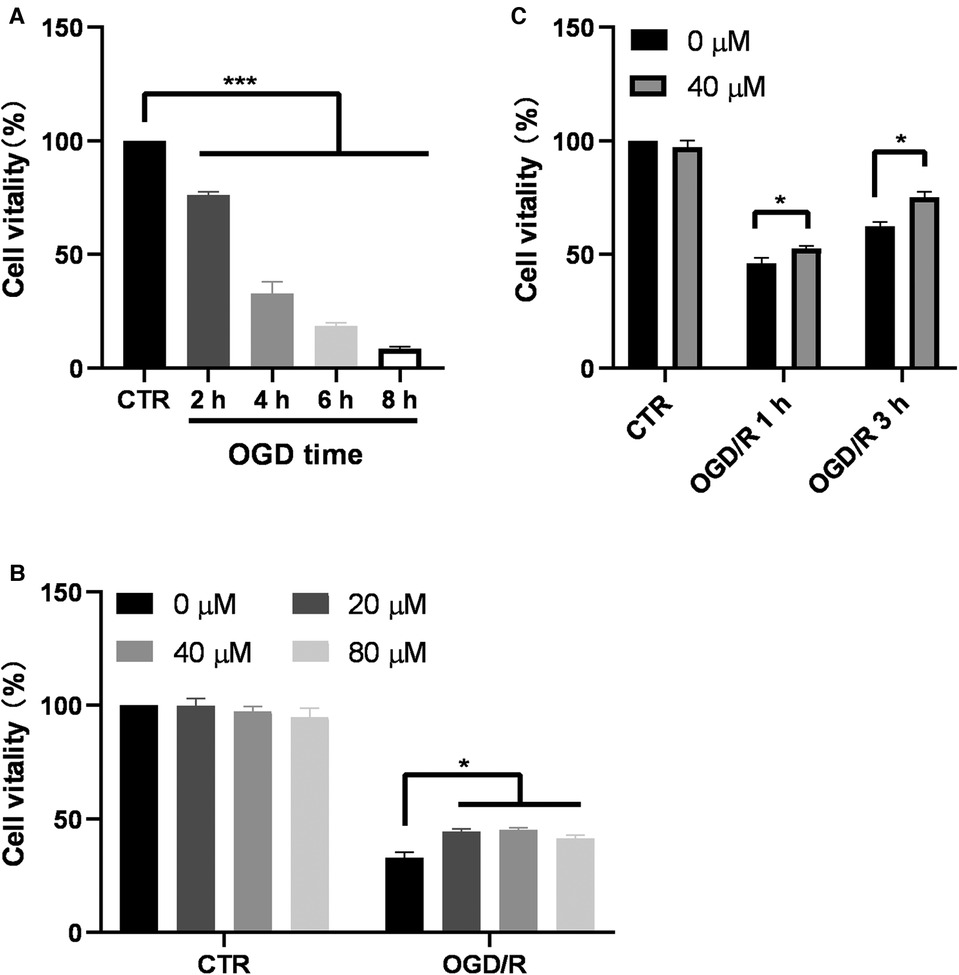
Figure 2. Ga increases BV2 cell viability after OGD/R. (A) BV2 cells were exposed to OGD for 2, 4, 6 and 8 h and the cell viability was detected by CCK-8 assay (n = 6). (B) Effect of different GA concentrations on cell viability of control cells for 7 h (CTR) and the cells treated with OGD for 4 h followed by reoxygenation for another 3 h (OGD/R). GA treatment significantly increased the survival rate of OGD cells without affecting Normal cells (n = 4). (C) GA treatment improved the cell viability after OGD for 4 h and reoxygenation for 1 and 3 h (n = 4). CTR, control. Data are presented as mean ± SEM. *P < 0.05, **P < 0.01, ***P < 0.001.
GA inhibited OGD/R-induced ROS and proinflammatory cytokine production in Bv2 cells
Considering the influence of microglial activation in the acute phase on the progression of the disease, OGD for 4 h and reperfusion for 1 h were chosen as the conditions for further study. To determine whether the protective effect of GA is related to the decreases in ROS and proinflammatory cytokine levels, the levels of ROS, IL-1β, and IL-6 in BV2 cells were measured (Figure 3). The levels of ROS, IL-1β and IL-6 mRNA significantly increased in the OGD/R group, while treatment with 40 µM GA significantly decreased their levels.
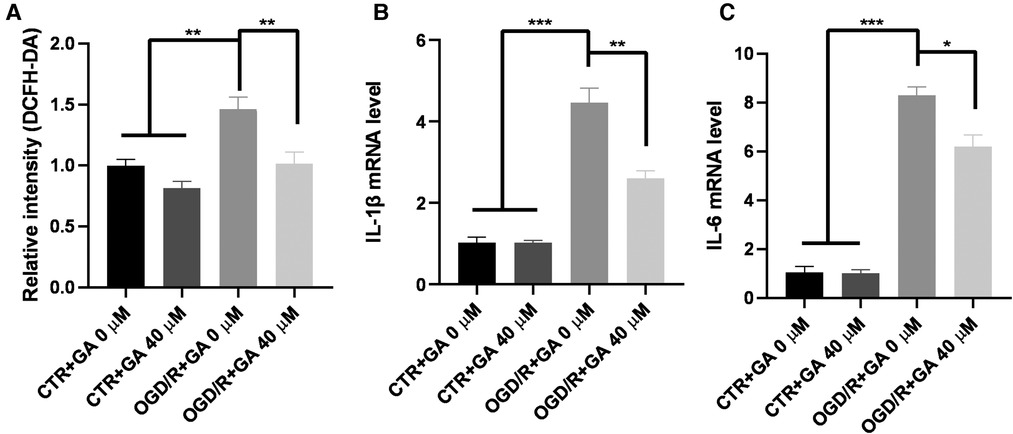
Figure 3. Ga inhibits OGD/R-induced ROS and inflammatory cytokines generation. (A) Quantification of ROS generation in BV2 cells. The mean fluorescence intensity of the control group without GA treatment was used to normalize for analysis. GA treatment reduced the overproduction of ROS induced by OGD/R (n = 5). The increased mRNA levels of IL-1β (B) and IL-6 (C) in OGD/R group were rescued with GA treatment (n = 3). Data are presented as mean ± SEM. *P < 0.05, **P < 0.01, ***P < 0.001.
GA decreased neuroinflammation in HIBD model rats
The in vitro results demonstrated that GA could exert a neuroprotective role by inhibiting OGD/R-induced ROS and proinflammatory cytokine production in BV2 cells. To determine whether GA could inhibit neonatal hypoxic-brain injury by exerting an anti-inflammatory effect, the levels of proinflammatory cytokines were also measured in vivo. HIBD model was established in 7-day-old SD rats, and RNA was extracted from the brains of rats in the different groups 48 h after modeling. The HIBD model rats exhibited higher expression of IL-1β and IL-6 (3.41 ± 0.97 and 6.25 ± 3.75 fold for IL-1β and IL-6, respectively) while the expression of IL-1β and IL-6 was decreased in response to GA treatment (1.06 ± 0.96 and 1.11 ± 0.23 fold for IL-1β and IL-6, respectively) (Figure 4).
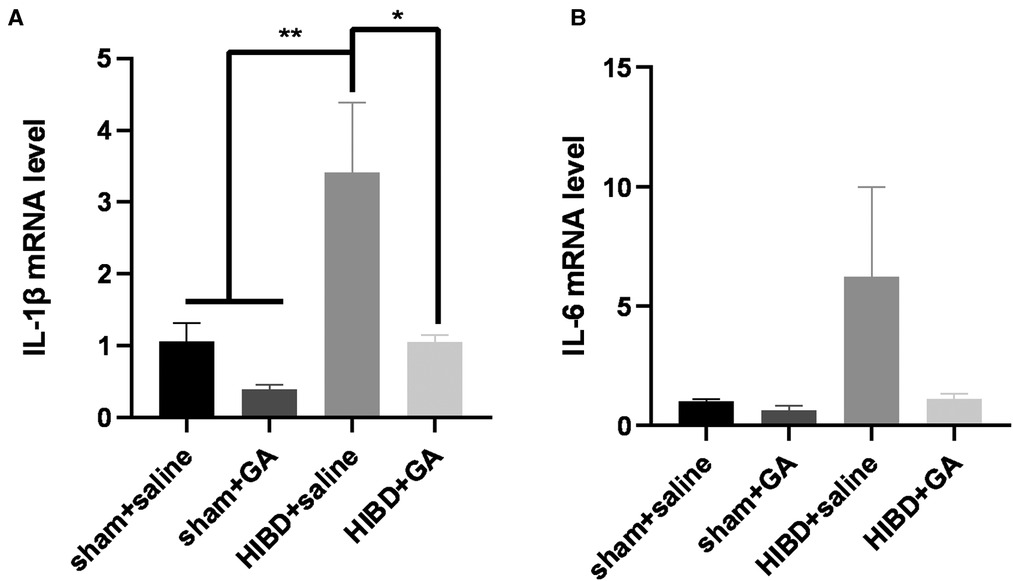
Figure 4. Ga decreases the neuroinflammation in HIBD rats. The rats were sacrificed 48 h after modeling, and RNA was extracted to detect the expression of proinflammatory cytokines. The increased mRNA levels of IL-1β (A) and IL-6 (B) in HIBD rats were rescued with GA treatment (n = 3). Data are presented as mean ± SEM. *P < 0.05.
GA reduced neuronal loss in HIBD model rats
Five weeks after HIBD rats were subjected to behavioral tests, brain tissue was sectioned for immunofluorescence staining with the neuronal marker NeuN to determine the number of neurons in the hippocampal CA1 region. The number of neurons in the CA1 region was significantly reduced in the HIBD group compared with the sham group, but was dramatically increased in response to GA treatment (108.63 ± 2.73, 114.49 ± 2.16, 77.93 ± 7.09, 108.42 ± 3.46 for sham + saline, sham + GA, HIBD + saline, and HIBD + GA, respectively) (Figure 5). The results suggested that GA protected neurons from HIBD.
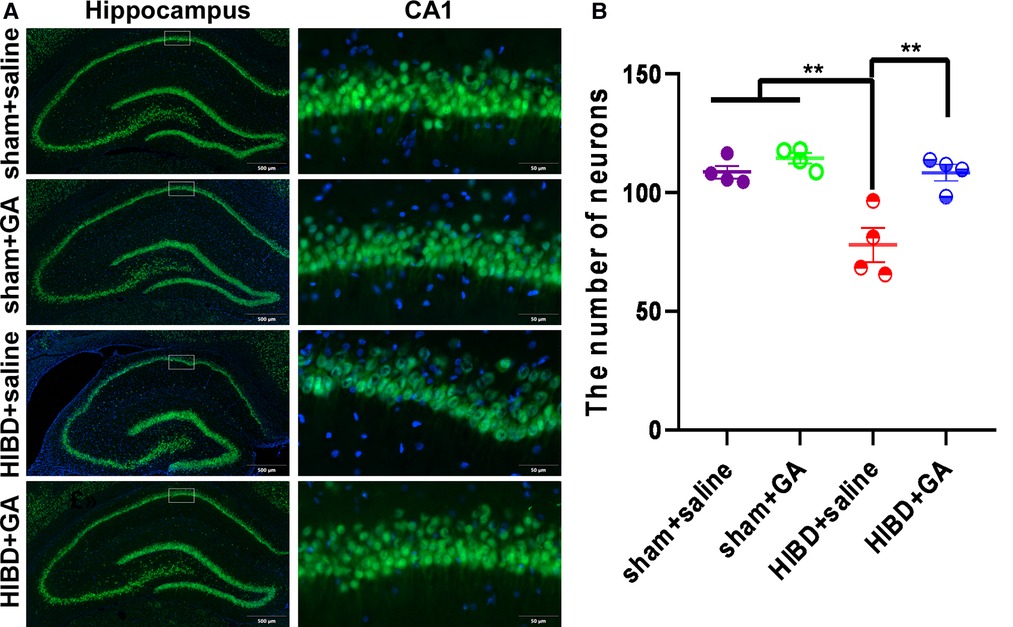
Figure 5. Ga reduces neuronal loss in CA1 region of HIBD rats. The HIBD model rats were sacrificed and the brain tissues were sectioned. The neurons were visualized by immunofluorescence staining with the neuron marker NeuN and the number of neurons in the hippocampal CA1 region were counted. (A) Representative immunofluorescence images of different groups. Scale bar: 500 μm for the left image and 50 μm for the right image (n = 4). (B) Quantification of NeuN positive cells. The number of neurons were significantly decreased in saline-treated HIBD rats compared to the rats in sham groups, which could be saved by GA treatment (n = 4). Data are presented as mean ± SEM. **P < 0.01.
GA ameliorated HIBD-induced motor dysfunction and muscle strength reduction
To test the protective effects of GA, the motor ability and muscle strength of the rats were measured by the rotarod test and grasping test. In the rotarod test, the total time that the rats in the HIBD + saline group stayed on the rotarod was significantly reduced, which was rescued by GA treatment (1200.46 ± 95.81, 1210.77 ± 89.57, 914.19 ± 67.06, and 1185.31 ± 101.44 s for sham + saline, sham + GA, HIBD + saline, HIBD + GA, respectively) (Figure 6A). The data indicated that GA treatment did not affect the motor ability of the rats in the sham group but significantly ameliorated HIBD-induced motor impairment.
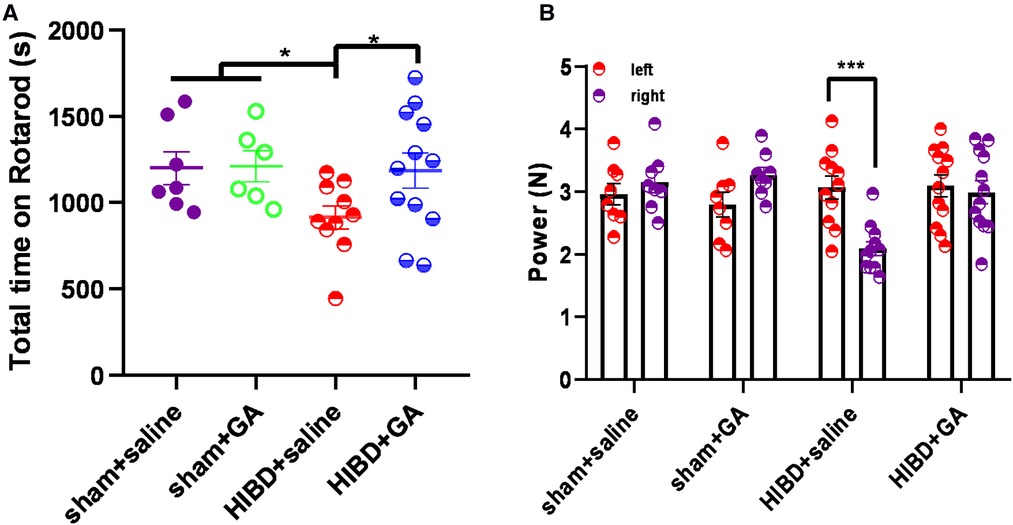
Figure 6. Ga alleviates the deficits of motor ability and muscle strength in HIBD model rats. (A) Rats were subjected to HIBD at the postnatal 7th day, GA or saline was injected daily for 2 weeks and the rotarod test was conducted at the 28th day. After the first day of adaptive training, the rats were put on the rotarod for 10 trails on the following day and the time on the rotarod was recorded. GA-treated HIBD rats increased the total time spent on the rotarod compared with the saline-treated HIBD rats (sham + saline, n = 7; sham + GA, n = 6; HIBD + saline, n = 10; HIBD + GA, n = 12). (B) The muscle strength of the left and right fore-limb was evaluated by grasping test. The imbalance muscle strength of the upper limbs caused by HIBD was saved by GA treatment (sham + saline, n = 8; sham + GA, n = 8; HIBD + saline, n = 11; HIBD + GA, n = 12). Data are presented as mean ± SEM. *P < 0.05, **P < 0.01, ***P < 0.001.
In the grasping test, the muscle strength of the right fore-limb (2.09 ± 0.11 N) was significantly decreased compared with that of the left forelimb (3.07 ± 0.18 N) in the HIBD + saline group, but no difference was observed after GA treatment (3.1 ± 0.18 N for left fore-limb and 2.99 ± 0.18 N for right fore-limb). In the sham groups, regardless of whether GA was administered, there was no difference in muscle strength between the left and right sides (2.96 ± 0.17 and 3.15 ± 0.17 N, 2.79 ± 0.2 and 3.27 ± 0.12 N for sham + saline and sham + GA, respectively) (Figure 6B).
GA alleviated HIBD-induced cognitive impairment
HIBD causes learning and memory disorders in animals (18–21). To investigate whether GA could improve cognitive functions, the spatial learning and memory of rats were evaluated by the MWM test. As shown in Figure 7A, the escape latency of rats in the HIBD + saline group was longer than that of rats in the other three groups, and the escape latency on day 5 was somewhat decreased by GA treatment (19.57 ± 3.18, 18.08 ± 3.35, 31.52 ± 3.54, and 22.61 ± 1.95 s for sham + saline, sham + GA, HIBD + saline, HIBD + GA, respectively). GA treatment also significantly increased the time spent in the platform quadrant (23.95 ± 3.09, 26.63 ± 2.17, 15.66 ± 2.19, and 22.11 ± 1.86 s for sham + saline, sham + GA, HIBD + saline, and HIBD + GA, respectively) (Figure 7D), and the number of platform crossings (2.88 ± 0.4, 2.63 ± 0.42, 1.36 ± 0.34, and 2.58 ± 0.4 times for sham + saline, sham + GA, HIBD + saline, and HIBD + GA, respectively) following HIBD. (Figure 7C). These data suggested that GA treatment alleviated HIBD-induced cognitive impairment.
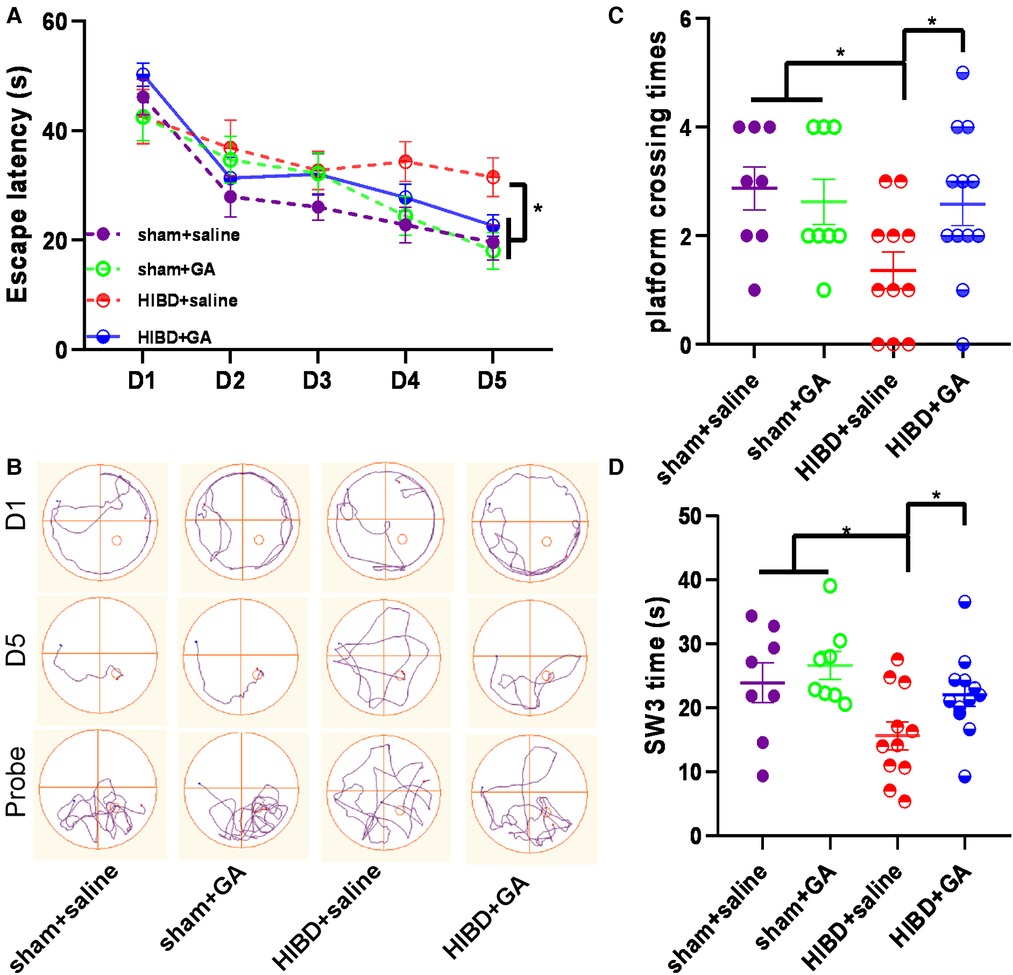
Figure 7. Ga improves the learning and memory of HIBD rats. MWM test consists of 1 day of adaptive trials and 5 days of hidden platform trials, plus a probe trial 24 h after the last hidden platform trial. Animal movement was tracked and recorded by ANY-maze tracking software (sham + saline, n = 8; sham + GA, n = 8; HIBD + saline, n = 11; HIBD + GA, n = 12). (A) In hidden platform tests, rats were trained with 4 trials per day for 5 days. GA-treated HIBD rats showed a shorter latency to escape onto the hidden platform on fifth days compared with the saline-treated HIBD rats. (B) Tracks of the rat movement in MWM test on different days. In the probe trial, the number of crossing platforms (C) and the time spent in the platform quadrant (D) of GA-treated HIBD rats were significantly more than the rats in saline-treated HIBD group. Data are presented as mean ± SEM. *P < 0.05.
Discussion
GA, a plant-derived phenolic acid with anti-inflammatory and antioxidant effects, is highly safe and has few side effects (22, 23). GA has been reported to exert a protective effect in many diseases, such as ischemic hypoxic injury in adult rats (24) or mice (25), Parkinson's disease (11), diabetes (26), and tumor (27), but little is known about its protective effect in newborn mice. HIE is not a single event, but a sustainable process. Inflammation has been considered as an important contributor in the pathophysiology of cerebral hypoxic ischemia (HI) injury (7, 28, 29). Previous studies have shown that the increased levels of inflammatory cytokines in the cerebrospinal fluid are associated with adverse neurological outcomes in children with HIE (30, 31). Neuroinflammation caused by HIE further leads to cell death and ultimately dysfunction (7, 32). Various drugs targeting neuroinflammation have been tested in animal models of perinatal brain damage. Several compounds that are already used in clinical such as melatonin and COX inhibitors have shown promising neuroprotective properties by inhibiting neuroinflammation (28). So timely inhibition of the inflammatory response in the early stage of HIE is critical to reduce injury.
Microglial cells are key immune cells that are involved in maintaining brain homeostasis (33). Previous studies have shown that microglia play a dual role in neuronal injury and recovery, which is associated with their phenotype; specifically, M1 microglia promote the release of inflammatory cytokines and aggravate injury, while M2 microglia can release anti-inflammatory cytokines to play a protective role (34, 35). HI promotes the polarization of microglia to the M1 phenotype and the release of proinflammatory factors such as TNF-α, IL-1β, and IL-6, which have toxic effects on nerve cells in the surrounding area (36, 37). In addition, excess production of ROS by activated microglia induces oxidative damage in the developing brain (38). Our study showed that OGD/R activated microglia and promoted the release of inflammatory cytokines and ROS, whereas GA treatment alleviated the damage induced by OGD/R; moreover, GA itself did not affect the inflammatory response and oxidative stress in normal BV2 cells, suggesting that GA could play a neuroprotective role by reducing neuroinflammation in OGD/R-treated BV2 cells.
Neuroinflammation caused by HI results in neuronal death, and neuronal death results in motor and cognitive impairments in both animal models and humans (39–41). Interestingly, in this study we found that GA could reduce neuroinflammation, neuronal loss and neurological dysfunction caused by HI. In addition to exerting positive effects in the HIBD model, GA has been reported to improve the cognitive function of patients with Alzheimer's disease and reduce motor dysfunction caused by lead and aflatoxin B1 exposure (13, 42–44). These evidences confirmed the neuroprotective effect of GA in HIBD.
GA was found to downregulate HI-induced proinflammatory genes in vitro and in vivo. Considering that M1 microglia can promote the release of inflammatory cytokines and aggravate injury, GA might play a protective role by affecting the polarization of microglia, thus decreasing the levels of inflammation-related genes and ROS. However, the specific mechanism through which GA regulates neuroinflammation after HI needs further in-depth study.
Conclusion
The evidence provided in this study indicates that, by inhibiting abnormal inflammatory activation, GA reduces HIBD-induced neuronal death and improves the motor and cognitive impairments following a hypoxic-ischemic insult. The data from our investigation support that GA is neuroprotective and may be a potentially effective drug for treatment of HIE and prevent its sequelae.
Data availability statement
The original contributions presented in the study are included in the article, further inquiries can be directed to the corresponding author/s.
Ethics statement
The animal study was reviewed and approved by The Ethics Committee of Chongqing Medical University.
Author contributions
XD and WS conceived and designed the experiments; XD, SL, DH, and QW performed the experiments; XD, RC, ZF, ZM, WZ, and WS analyzed and contributed reagents/materials/analysis tools. XD, RC, WZ, and WS wrote the paper. WS and WZ supervised the project. All authors reviewed the manuscript. All authors contributed to the article and approved the submitted version.
Funding
The study was partially supported by funding to Key Laboratory of Alzheimer’s Disease of Zhejiang Province and Zhejiang Provincial Clinical Research Center for Mental Disorders (WS).
Conflict of interest
The authors declare that the research was conducted in the absence of any commercial or financial relationships that could be construed as a potential conflict of interest.
Publisher's note
All claims expressed in this article are solely those of the authors and do not necessarily represent those of their affiliated organizations, or those of the publisher, the editors and the reviewers. Any product that may be evaluated in this article, or claim that may be made by its manufacturer, is not guaranteed or endorsed by the publisher.
References
1. McIntyre S, Nelson KB, Mulkey SB, Lechpammer M, Molloy E, Badawi N. Neonatal encephalopathy: focus on epidemiology and underexplored aspects of etiology. Semin Fetal Neonatal Med. (2021) 26:101265. doi: 10.1016/j.siny.2021.101265
2. Wang Z, Zhang P, Zhou W, Xia S, Zhou W, Zhou X, et al. Neonatal hypoxic-ischemic encephalopathy diagnosis and treatment: a national survey in China. BMC Pediatr. (2021) 21:261. doi: 10.1186/s12887-021-02737-6
3. Kharoshankaya L, Stevenson NJ, Livingstone V, Murray DM, Murphy BP, Ahearne CE, et al. Seizure burden and neurodevelopmental outcome in neonates with hypoxic-ischemic encephalopathy. Dev Med Child Neurol. (2016) 58:1242–8. doi: 10.1111/dmcn.13215
4. Lee BL, Glass HC. Cognitive outcomes in late childhood and adolescence of neonatal hypoxic-ischemic encephalopathy. Clin Exp Pediatr. (2021) 64:608–18. doi: 10.3345/cep.2021.00164
5. Natarajan G, Shankaran S, Pappas A, Bann C, Tyson JE, McDonald S, et al. Functional status at 18 months of age as a predictor of childhood disability after neonatal hypoxic-ischemic encephalopathy. Dev Med Child Neurol. (2014) 56:1052–8. doi: 10.1111/dmcn.12512
6. van Schie PE, Schijns J, Becher JG, Barkhof F, van Weissenbruch MM, Vermeulen RJ. Long-term motor and behavioral outcome after perinatal hypoxic-ischemic encephalopathy. Eur J Paediatr Neurol. (2015) 19:354–9. doi: 10.1016/j.ejpn.2015.01.005
7. Li B, Concepcion K, Meng X, Zhang L. Brain-immune interactions in perinatal hypoxic-ischemic brain injury. Prog Neurobiol. (2017) 159:50–68. doi: 10.1016/j.pneurobio.2017.10.006
8. Bartha AI, Foster-Barber A, Miller SP, Vigneron DB, Glidden DV, Barkovich AJ, et al. Neonatal encephalopathy: association of cytokines with MR spectroscopy and outcome. Pediatr Res. (2004) 56:960–6. doi: 10.1203/01.PDR.0000144819.45689.BB
9. Chen CY, Sun WZ, Kang KH, Chou HC, Tsao PN, Hsieh WS, et al. Hypoxic preconditioning suppresses glial activation and neuroinflammation in neonatal brain insults. Mediators Inflamm. (2015) 2015:632592. doi: 10.1155/2015/632592
10. Wang D, Wei Y, Tian J, He D, Zhang R, Ji X, et al. Oxiracetam mediates neuroprotection through the regulation of microglia under hypoxia-ischemia neonatal brain injury in mice. Mol Neurobiol. (2021) 58:3918–37. doi: 10.1007/s12035-021-02376-z
11. Chandrasekhar Y, Phani Kumar G, Ramya EM, Anilakumar KR. Gallic acid protects 6-OHDA induced neurotoxicity by attenuating oxidative stress in human dopaminergic cell line. Neurochem Res. (2018) 43:1150–60. doi: 10.1007/s11064-018-2530-y
12. Park CS, Lee JY, Choi HY, Lee K, Heo Y, Ju BG, et al. Gallic acid attenuates blood-spinal cord barrier disruption by inhibiting Jmjd3 expression and activation after spinal cord injury. Neurobiol Dis. (2020) 145:105077. doi: 10.1016/j.nbd.2020.105077
13. Reckziegel P, Dias VT, Benvegnú D, Boufleur N, Silva Barcelos RC, Segat HJ, et al. Locomotor damage and brain oxidative stress induced by lead exposure are attenuated by gallic acid treatment. Toxicol Lett. (2011) 203:74–81. doi: 10.1016/j.toxlet.2011.03.006
14. Shan H, Geng L, Jiang X, Song M, Wang J, Liu Z, et al. Large-scale chemical screen identifies gallic acid as a geroprotector for human stem cells. Protein Cell. (2022) 13:532–9. doi: 10.1007/s13238-021-00872-5
15. Vannucci RC, Vannucci SJ. Perinatal hypoxic-ischemic brain damage: evolution of an animal model. Dev Neurosci. (2005) 27:81–6. doi: 10.1159/000085978
16. Dai C, Liu Y, Dong Z. Tanshinone I alleviates motor and cognitive impairments via suppressing oxidative stress in the neonatal rats after hypoxic-ischemic brain damage. Mol Brain. (2017) 10:52. doi: 10.1186/s13041-017-0332-9
17. Han W, Dong X, Song X, Cheng L, Xie L, Cheng H, et al. Effects of advanced maternal age on cognitive and emotional development in offspring rats. Behav Brain Res. (2018) 353:218–26. doi: 10.1016/j.bbr.2018.04.003
18. Gu Y, Zhang Y, Bi Y, Liu J, Tan B, Gong M, et al. Mesenchymal stem cells suppress neuronal apoptosis and decrease IL-10 release via the TLR2/NFκB pathway in rats with hypoxic-ischemic brain damage. Mol Brain. (2015) 8:65. doi: 10.1186/s13041-015-0157-3
19. Gong M, Bi Y, Jiang W, Zhang Y, Chen L, Hou N, et al. Immortalized mesenchymal stem cells: an alternative to primary mesenchymal stem cells in neuronal differentiation and neuroregeneration associated studies. J Biomed Sci. (2011) 18:87. doi: 10.1186/1423-0127-18-87
20. Jiang W, Guo M, Gong M, Chen L, Bi Y, Zhang Y, et al. Vitamin A bio-modulates apoptosis via the mitochondrial pathway after hypoxic-ischemic brain damage. Mol Brain. (2018) 11:14. doi: 10.1186/s13041-018-0360-0
21. Tang Z, Cheng S, Sun Y, Zhang Y, Xiang X, Ouyang Z, et al. Early TLR4 inhibition reduces hippocampal injury at puberty in a rat model of neonatal hypoxic-ischemic brain damage via regulation of neuroimmunity and synaptic plasticity. Exp Neurol. (2019) 321:113039. doi: 10.1016/j.expneurol.2019.113039
22. Rajalakshmi K, Devaraj H, Niranjali Devaraj S. Assessment of the no-observed-adverse-effect level (NOAEL) of gallic acid in mice. Food Chem Toxicol. (2001) 39:919–22. doi: 10.1016/S0278-6915(01)00022-9
23. Variya BC, Bakrania AK, Madan P, Patel SS. Acute and 28-days repeated dose sub-acute toxicity study of gallic acid in albino mice. Regul Toxicol Pharmacol. (2019) 101:71–8. doi: 10.1016/j.yrtph.2018.11.010
24. Sun J, Li YZ, Ding YH, Wang J, Geng J, Yang H, et al. Neuroprotective effects of gallic acid against hypoxia/reoxygenation-induced mitochondrial dysfunctions in vitro and cerebral ischemia/reperfusion injury in vivo. Brain Res. (2014) 1589:126–39. doi: 10.1016/j.brainres.2014.09.039
25. Sun J, Ren DD, Wan JY, Chen C, Chen D, Yang H, et al. Desensitizing mitochondrial permeability transition by ERK-cyclophilin D axis contributes to the neuroprotective effect of gallic acid against cerebral ischemia/reperfusion injury. Front Pharmacol. (2017) 8:184. doi: 10.3389/fphar.2017.00184
26. Abdel-Moneim A, Yousef AI, Abd El-Twab SM, Abdel Reheim ES, Ashour MB. Gallic acid and p-coumaric acid attenuate type 2 diabetes-induced neurodegeneration in rats. Metab Brain Dis. (2017) 32:1279–86. doi: 10.1007/s11011-017-0039-8
27. Raina K, Rajamanickam S, Deep G, Singh M, Agarwal R, Agarwal C. Chemopreventive effects of oral gallic acid feeding on tumor growth and progression in TRAMP mice. Mol Cancer Ther. (2008) 7:1258–67. doi: 10.1158/1535-7163.MCT-07-2220
28. Hagberg H, Mallard C, Ferriero DM, Vannucci SJ, Levison SW, Vexler ZS, et al. The role of inflammation in perinatal brain injury. Nat Rev Neurol. (2015) 11:192–208. doi: 10.1038/nrneurol.2015.13
29. Liu F, McCullough LD. Inflammatory responses in hypoxic ischemic encephalopathy. Acta Pharmacol Sin. (2013) 34:1121–30. doi: 10.1038/aps.2013.89
30. Sävman K, Blennow M, Gustafson K, Tarkowski E, Hagberg H. Cytokine response in cerebrospinal fluid after birth asphyxia. Pediatr Res. (1998) 43:746–51. doi: 10.1203/00006450-199806000-00006
31. Šumanović-Glamuzina D, Čulo F, Čulo MI, Konjevoda P, Jerković-Raguž M. A comparison of blood and cerebrospinal fluid cytokines (IL-1β, IL-6, IL-18, TNF-α) in neonates with perinatal hypoxia. Bosn J Basic Med Sci. (2017) 17:203–10. doi: 10.17305/bjbms.2017.1381
32. Torres-Cuevas I, Corral-Debrinski M, Gressens P. Brain oxidative damage in murine models of neonatal hypoxia/ischemia and reoxygenation. Free Radic Biol Med. (2019) 142:3–15. doi: 10.1016/j.freeradbiomed.2019.06.011
33. Nimmerjahn A, Kirchhoff F, Helmchen F. Resting microglial cells are highly dynamic surveillants of brain parenchyma in vivo. Science. (2005) 308:1314–8. doi: 10.1126/science.1110647
34. Hamzei Taj S, Kho W, Aswendt M, Collmann FM, Green C, Adamczak J. Dynamic modulation of microglia/macrophage polarization by miR-124 after focal cerebral ischemia. J Neuroimmune Pharmacol. (2016) 11:733–48. doi: 10.1007/s11481-016-9700-y
35. Zeng F, Wu Y. Custom-Made ceria nanoparticles show a neuroprotective effect by modulating phenotypic polarization of the microglia. Angew Chem Int Ed Engl. (2018) 57:5808–12. doi: 10.1002/anie.201802309
36. Seitz M, Koster C, Dzietko M, Sabir H, Serdar M, Felderhoff-Muser U, et al. Hypothermia modulates myeloid cell polarization in neonatal hypoxic-ischemic brain injury. J Neuroinflammation. (2021) 18:266. doi: 10.1186/s12974-021-02314-9
37. Li SJ, Liu W, Wang JL, Zhang Y, Zhao DJ, Wang TJ, et al. The role of TNF-alpha, IL-6, IL-10, and GDNF in neuronal apoptosis in neonatal rat with hypoxic-ischemic encephalopathy. Eur Rev Med Pharmacol Sci. (2014) 18:905–9.24706318
38. Kaur C, Rathnasamy G, Ling EA. Roles of activated microglia in hypoxia induced neuroinflammation in the developing brain and the retina. J Neuroimmune Pharmacol. (2013) 8:66–78. doi: 10.1007/s11481-012-9347-2
39. Ten VS, Bradley-Moore M, Gingrich JA, Stark RI, Pinsky DJ. Brain injury and neurofunctional deficit in neonatal mice with hypoxic-ischemic encephalopathy. Behav Brain Res. (2003) 145:209–19. doi: 10.1016/S0166-4328(03)00146-3
40. Ten VS, Wu EX, Tang H, Bradley-Moore M, Fedarau MV, Ratner VI, et al. Late measures of brain injury after neonatal hypoxia-ischemia in mice. Stroke. (2004) 35:2183–8. doi: 10.1161/01.STR.0000137768.25203.df
41. Vargha-Khadem F, Gadian DG, Mishkin M. Dissociations in cognitive memory: the syndrome of developmental amnesia. Philos Trans R Soc Lond B Biol Sci. (2001) 356:1435–40. doi: 10.1098/rstb.2001.0951
42. Korani MS, Farbood Y, Sarkaki A, Fathi Moghaddam H, Taghi Mansouri M. Protective effects of gallic acid against chronic cerebral hypoperfusion-induced cognitive deficit and brain oxidative damage in rats. Eur J Pharmacol. (2014) 733:62–7. doi: 10.1016/j.ejphar.2014.03.044
43. Yu M, Chen X, Liu J, Ma Q, Zhuo Z, Chen H, et al. Gallic acid disruption of aβ(1-42) aggregation rescues cognitive decline of APP/PS1 double transgenic mouse. Neurobiol Dis. (2019) 124:67–80. doi: 10.1016/j.nbd.2018.11.009
Keywords: hypoxic-ischemic brain damage, gallic acid, neuroprotective effect, neuroinflammation, neuronal loss
Citation: Dong X, Luo S, Hu D, Cao R, Wang Q, Meng Z, Feng Z, Zhou W and Song W (2022) Gallic acid inhibits neuroinflammation and reduces neonatal hypoxic-ischemic brain damages. Front. Pediatr. 10:973256. doi: 10.3389/fped.2022.973256
Received: 19 June 2022; Accepted: 2 December 2022;
Published: 22 December 2022.
Edited by:
Geun Hee Seol, Korea University, South KoreaReviewed by:
Funda Tuzun, Dokuz Eylül University, TurkeyEndla Katalin Anday, Drexel University, United States
© 2022 Dong, Luo, Hu, Cao, Wang, Meng, Feng, Zhou and Song. This is an open-access article distributed under the terms of the Creative Commons Attribution License (CC BY). The use, distribution or reproduction in other forums is permitted, provided the original author(s) and the copyright owner(s) are credited and that the original publication in this journal is cited, in accordance with accepted academic practice. No use, distribution or reproduction is permitted which does not comply with these terms.
*Correspondence: Weihong Song d2VpaG9uZ0B3bXUuZWR1LmNu Weihui Zhou endoQGhvc3BpdGFsLmNxbXUuZWR1LmNu
Specialty Section: This article was submitted to Neonatology, a section of the journal Frontiers in Pediatrics