- Neonatal Intensive Care Unit, Department of Surgical Sciences, Azienda Ospedaliera Universitaria (AOU) Cagliari, University of Cagliari, Cagliari, Italy
Gestational diabetes mellitus (GDM), or any degree of glucose intolerance recognized for the first time during pregnancy, is one of the diseases that most frequently aggravates the course of gestation. Missed or late diagnosis and inadequate treatment are associated with high maternal and fetal morbidity, with possible short- and long-term repercussions. Estimates on the prevalence of GDM are alarming and increasing by about 30% in the last 10–20 years. In addition, there is the negative influence of the SARS-CoV-2 emergency on the glycemic control of pregnant women, making the matter increasingly topical. To date, knowledge on the metabolic maturation of newborns is still incomplete. However, in light of the considerable progress of the theory of “developmental origins of health and disease,” the relevant role of the intrauterine environment cannot be overlooked. In fact, due to the high plasticity of the early stages of development, some detrimental metabolic alterations during fetal growth, including maternal hyperglycemia, are associated with a higher incidence of chronic diseases in adult life. In this context, metabolomic analysis which allows to obtain a detailed phenotypic portrait through the dynamic detection of all metabolites in cells, tissues and different biological fluids could be very useful for the early diagnosis and prevention of complications. Indeed, if the diagnostic timing is optimized through the identification of specific metabolites, the detailed understanding of the altered metabolic pathway could also allow better management and more careful monitoring, also from a nutritional profile, of the more fragile children. In this context, a further contribution derives from the analysis of the intestinal microbiota, the main responsible for the fecal metabolome, given its alteration in pregnancies complicated by GDM and the possibility of transmission to offspring. The purpose of this review is to analyze the available data regarding the alterations in the metabolomic profile and microbiota of the offspring of mothers with GDM in order to highlight future prospects for reducing GDM-related complications in children of mothers affected by this disorder.
Introduction
The American Diabetes Association defines gestational diabetes mellitus (GDM) as any degree of glucose intolerance recognized for the first time during pregnancy, mainly in the second or third trimester of gestation (1). Insulin resistance and ß-cellular dysfunction are critical factors in the pathophysiology of this metabolic alteration (2). The maternal risk factors are now well-known, they can be are modifiable and non-modifiable. The former includes overweight and pre-pregnancy maternal obesity, metabolic syndrome and polycystic ovary syndrome, previous pre-eclampsia in previous pregnancies and stress (3). There are also other non-modifiable conditions such as maternal age, the gravidity and parity, a genetic predisposition as well as a family history of hyperglycemia, ethnicity and social-economic status (3).
GDM, if not diagnosed and treated properly, is associated with high maternal and fetal morbidity, with possible repercussions throughout their life span (4). Indeed, data on the short and long-term impact on both the mother and the child are now numerous (2, 5). Estimates of the prevalence of glycemic changes in pregnancy are not encouraging. In fact, it is believed that, in 2017, about 21.3 million live birth, or 16.2% of them, were affected by some form of maternal hyperglycemia and that 18.4 millions of them are precisely caused by GDM (about the 86.4% of pregnancy hyperglycemia) (6). The 9th edition of the IDF Diabetes Atlas released in 2019 estimated a slight reduction in the overall prevalence of maternal hyperglycemia to 15.8%, with GDM at 12.8% (7).
These data reflect an increase of this pathology of about 30% in the last 10–20 years, which affects not only industrialized countries but also developing countries (8) with variable proportions depending on the geographical area (9). Furthermore, the data available to date would seem to confirm the negative influence of the SARS-CoV-2 emergency on the glycemic control of pregnant women, making the issue increasingly topical and crucial. In fact, a worsening of diabetes control during the COVID-19 pandemic lock-down emerged from a French retrospective study (10), probably confirming the impact of reduced physical activity, changes in eating habits and associated stress. These results were reinforced by Cauldwell et al. (11) highlighting a 33% increase in the diagnosis of GDM since the beginning of the pandemic.
To date, in light of the increasing evidence of the theory of “developmental origins of health and disease” (DOHaD), the importance of the environmental impact on the phenotypic expression of an individual has been affirmed, including the intrauterine environment. It is now known that metabolic alterations during fetal growth are correlated with the occurrence of chronic diseases in adult life, such as type 2 diabetes, atherosclerosis, hypertension and metabolic syndrome, due to the high plasticity of the early stages of development (12). In this context, maternal hyperglycemia plays a very important role as a risk factor (13–15).
Metabolomics, through the analysis of a large number of low molecular weight metabolites, makes it possible to instantly identify the changes in the various metabolic pathways resulting from the interaction between specific pathophysiological states, gene expression and the environment (16). Therefore, this technique could be very useful. On the one hand, the diagnostic timing, prognosis and management of GDM could be optimized, on the other hand it would allow the improvement of the outcomes of the children of a diabetic mother. Indeed, the understanding of the specific altered metabolic pathways and the early recognition of metabolic profiles associated with the development of diseases in adult life would allow a personalized intervention even from a nutritional point of view, with a view to an individualized medicine for the most fragile children (16).
In this context, a valid contribution can also be provided by the analysis of the microbiota, the main responsible for the fecal metabolome, which has been found to be altered in pregnancies complicated by GDM, with the possibility of transmission to the offspring (17, 18).
Epigenetics
In the scientific community, there is a growing optimism about the possibility of using the information coming from epigenetics studies as potential biomarkers to predict the probability of future adverse effects in offspring. The possibility of determining whether GDM causes an increased risk of metabolic problems in children would represent an important tool for intervention against an intergenerational cycle favoring the onset of obesity and type 2 diabetes (19). In fact, once the causal effect will be clarified, the epigenetic mechanisms could represent a possible target to prevent the negative outcomes (19). Nevertheless, the evidence from epigenetic epidemiology regarding the potential mechanism that would mediate this association is, at the moment, still not very solid (19). This is what emerged from a recent review of the scientific literature by Elliot et al. (19), which examined all human studies published in the literature regarding the association between different glycemic changes during pregnancy (including GDM and type 2 diabetes) and any epigenetic markers (DNA methylation, histone modification and miRNA). In fact, some critical issues emerged in these studies. Primarily, the small number of samples, secondly a poor replication but above all a lack of investigation of causal effects. The authors therefore conclude that further research in this area is needed with the aim to replicate what has already been observed, then the expansion of causal analysis approaches, and where possible, to investigate whether the GDM-associated epigenetic mechanisms are directly related to adverse effects in offspring even in the long term (19).
However, this analysis showed that the studies on this subject are still quite numerous and that most of them revealed alterations in DNA methylation (83% of the studies analyzed) in the cord blood, placenta and blood of the newborn (19). Overall, from the examination of the studies analyzed both by the review by Elliot et al. (19) and by that conducted in the same year period by Franzago et al. (20), it emerges that the possibility of acquiring information on the epigenetic alterations induced by an adverse intrauterine environment may represent the first step to understand the pathophysiological mechanism underlying the development of adverse effects.
An in-depth analysis of epigenetic epidemiology studies is beyond the scope of this review, nevertheless, it may be useful to understand the correlation between certain metabolic pathways and the main GDM-related epigenetic markers of some genes potentially responsible for metabolic disorders in offspring. In addition, the detection of particular epi-markings associated with glycemic changes during gestation could also be important for optimizing the interpretation of metabolomic analysis data.
Indeed, in 2013, an important impact of epigenetic labeling emerges from the study by Ruchat et al. (21). This is the first study that not only investigated the effects of GDM on the entire neonatal epigenome but also studied the possible consequences on the growth and development of the child. They showed that GDM can affect the methylation of more than 3,000 genes, both at the placental level and in the cord blood. These genes are involved in metabolic disorders as well, including diabetes, and in the regulation of energy expenditure and adiposity.
The fetal methylation state of the leptin gene (LEP) and that of adiponectin (ADIPOQ) have quite often been the subject of human epigenetics studies (20) (Table 1), due to the critical contribution of these adipokines to metabolic homeostasis (13, 22). The first study concerning the methylation of these two genes, was performed by Bouchard et al. (13, 14), in 2010. They highlighted not only the correlation between GDM and LEP methylation status, but also the difference in epigenetic labeling between the fetal and maternal side of the placenta, suggesting a different impact of maternal glycemic alterations in the placental sides resulting in LEP hyper-methylation and LEP hypo-methylation on the maternal and fetal side, respectively. In 2012 (14), they focused their attention on the methylation of ADIPOQ, highlighting a state of hypo-methylation at the level of the promoter of this gene on the fetal side of the placenta. The speculations made by the authors mainly concern the involvement of ADIPOQ and LEP in energy metabolism and in the control of insulin sensitivity. Thus, they hypothesized that these epigenetic adaptations could potentially be responsible for the metabolic disorders of the offspring over the course of life. This possible correlation is of even greater importance in light of the findings of García-Cardona et al. (23). They highlighted the presence of a negative association between methylation at the level of specific sites of LEP and ADIPOQ promoters and the combined presence of obesity, and insulin resistance, supporting the hypothesis that epigenetic modifications could be at the basis of the development of obesity and related metabolic disorders.
While, in 2018, Ott et al. investigated the alterations of adiponectin plasma, mRNA, and DNA methylation levels in GDM offspring, showing that while newborn adiponectin levels were similar between groups (GDM and controls), DNA methylation in GDM offspring was variously altered (22).
Furthermore, Gagné-Ouellet et al. (24) investigated the association between maternal hyperglycemia, placental LEP DNA methylation, neonatal leptinemia and adiposity at 3-years-old, in 259 mother–child dyads. They observed three CpG islands associated with neonatal leptinemia, two of which, cg05136031 and cg15758240, were also associated with BMI and fat distribution at 3-years-old, respectively. Maternal glycemia was correlated with DNA methylation at cg15758240 and neonatal leptinemia. The authors speculated that DNA methylation regulation of the leptin pathway in response to maternal glycemia might be involved in programming adiposity in childhood. In addition, recently, Wang et al. (25) attempted a genome-wide identification of differentially methylated placental genes and specific GDM-associated pathways to assess the presence of correlations with fetal growth and biomarkers of metabolic health in cord blood. Results showed that although GDM was associated with changes in DNA methylation in a number of placental genes, these gene methylations were not correlated with observed biomarkers of metabolic health such as fetal growth factors, leptin and adiponectin, in cord blood. However, a trend toward a positive correlation between methylation of a CpG site in the promoter of LEP (cg05136031) and leptin in cord blood was observed, consistent with what was reported by Gagné-Ouellet et al. (24). In contrast, no correlation was observed for the cg15758240 site, unlike in the previous study. The authors conclude that the lack of significant association in the study can be partly attributed to the relatively small sample size (n = 60).
Epigenetic markings at the level of brown adipose tissue (BAT) have also aroused interest, given its potential preventive activity against obesity. Indeed, the findings by Côté et al. (26) suggest that the elevated maternal blood glucose levels during gestation may be responsible for changes in the DNA methylation status at the level of specific BAT-related genes, including PRDM16, BMP7 and PPARGC1α. It also emerged that some of these epigenetic variations (PRDM16, PPARGC1α) seem to influence the leptin levels in the cord blood with possible repercussions on the regulation of the body weight of the unborn child.
While, El Hajj et al. (27), focused their attention on a possible action at the level of white adipose tissue (WAT). They detected an important hypo-methylation of mesoderm-specific transcript (MEST) in placental tissues and it was found also in cord blood in the presence of GDM (both treated with insulin and diet therapy). The correlation of these results with the observation of an equally conspicuous hypo-methylation of MEST in obese adult patients has led to the hypothesis that an alteration of the epigenetic programming of this gene may contribute to the predisposition to obesity of the offspring of diabetic mother. In fact, the role of MEST as placental and fetal growth factor was highlighted in studies on knockout murine specimens (28). Furthermore, the investigation on genetically identical mice with different expression of MEST revealed distinct levels of development of diet-related obesity (29). Moreover, murine specimens in which the isoform-1 of MEST was hyper-expressed were characterized by a greater expansion of fat mass (30). However, the role and complexity of epigenetic variations in MEST are still to be investigated. Indeed, Karbiener et al. (31) suggested its role as an inhibitor of human adipogenesis in the early stages of adipocyte differentiation, unlike what was found in previous murine studies (32–34). Nevertheless, the positive correlation between MEST and fat mass was confirmed and higher levels of MEST emerged in WAT samples of obese adults compared to controls (31).
Equally controversial data were reported by Gagné-Ouellet et al. (15), which highlighted another DNA methylation site related to GDM at the level of the lipoproteinlipase (LPL) gene. They observed that the different degree of GDM-related methylation within the intergenic region of placental LPL (fetal side) is associated with lower birth weight and BMI before 5 years of age, according to WHO growth charts. Thus, they hypothesized that these epi-variations at the level of LPL on the fetal side of the placenta may compromise fetal growth and fat accumulation in infancy. Hence, these alterations are at the basis of metabolic dysfunctions in later life. However, these remain speculations, in fact whether this association is mediated by epigenetic mechanisms that influence the expression of the LPL gene has yet to be demonstrated.
Epigenetic epidemiology investigation concerned the possible effects on the central nervous system and its neurotransmitters, as well. The study on human placenta by Blazevic et al. (35) suggested that methylation of the gene of the serotonin transporter SLC6A4, one of the main regulators of the homeostasis of this hormone, is sensitive to maternal glycemic alterations during pregnancy. A predominant role of epigenetics, compared to genetics, was evidenced in the regulation of the expression of SLC6A4 in the human placenta. However, further investigations are needed to verify the extent to which these alterations are involved in the long-term GDM-associated effects. Another epigenetic mechanism potentially involved in the effects of GDM on fetal neurodevelopment was studied in vitro by Mishra et al. (36). They found that GDM-induced glycemic alterations decrease the expression of the sirtuins-1 gene (SIRT-1), a nutrient sensor, responsible for a decrease in placental transport of DHA through trophoblasts. This mechanism seems to have a central role in the neurodevelopmental dysfunctions observed in children of diabetic mothers (37, 38). A recent meta-analysis supported the hypothesis of the impact of GDM on the neurological development of offspring (39) conducted by the Consortium Pregnancy and Childhood Epigenetics. It analyzed 3,677 mother-infant pairs from seven distinct cohorts, including 317 pairs with GDM. They found a hypo-methylation in cord blood at the level of the OR2L13 promoter, a condition similar to that observed in subjects with autism spectrum disorder. They also discovered a hypo-methylation of CYP2E1, the upregulation of which, in the peripheral blood, is characteristic of subjects with type 1 and type 2 diabetes.
GDM-correlated complications in offspring
The numerous GDM-related adverse effects are now known despite the still weak evidence of epigenetic epidemiology in this regard. Surely the initial contribution of the Hyperglycemia and Adverse Pregnancy Outcome (HAPO) Study (5) conducted in 15 centers in 9 different countries, was decisive as it definitively established a linear association between short-term adverse effects in pregnancy and poor glycemic regulation. However, to date, the knowledge on the GDM-related offspring complications has significantly expanded to include longer-term effects (40).
Perinatal, fetal and neonatal complications
One of the best known GDM-related fetal complications is macrosomia, typically defined as a birth weight greater than the 90th percentile for gestational age or a weight >4 kg (41). In fact, there are numerous studies that report this association (5, 42–45), even if the pathophysiological mechanisms underlying this adverse effect have not yet been fully clarified (41). It has been hypothesized that maternal hyperglycemia leads to fetal hyperinsulinemia with consequent weight gain (41). This is because in the presence of maternal glycemic dysregulation, the high circulating glucose levels reach the fetus through the placenta which, however, is not crossed by maternal insulin. Then, in the second trimester of gestation, when the fetal pancreas becomes able to secrete insulin and begins to respond to hyperglycemia and releases insulin autonomously, regardless of glucose stimulation, excessive secretion of this hormone occurs. The fetus is therefore in a highly anabolic condition due to the high concentrations of glucose and hyper-insulinemia which favors weight gain (41). The correlation between GDM and macrosomia already emerged from the HAPO study (5), where an important positive association was found between maternal glycemic levels and birth weight. This correlation was then confirmed by a large retrospective study (43) conducted on 21,000 women. It showed that GDM correlates with a 48% increased risk of fetal macrosomia, a value greater than what emerged in an analysis of the literature conducted until then (15–45%) (41). However, recently, a study by Li et al. based on a multi-racial US population (46), suggested that in the presence of GDM the increase in fetal size compared to the standard, began around 20 weeks of gestation and then become significant only at 28 weeks. These data agreed with the excessive growth of the abdominal circumference found between 20 and 28 weeks of gestation by Sovio et al. (47). This finding highlighted the problem of poor alignment between the need for early intervention timings and standard diagnosis times for GDM, in support of the usefulness of earlier markers. Another retrospective analysis (42) carried out in China, in 2021, on 8,844 women, confirmed the association between GDM and an increased risk of macrosomia, cesarean section and preterm birth, as observed in previous analyzes (43). Furthermore, the latter two events could also be associated with the greater vaginal infection risk in the presence of GDM. This was also found in China in a prospective study in 2015 (48), which showed that in the presence of GDM there was a higher rate of premature rupture of membrane (PROM) and chorioamnionitis. Moreover, this was in agreement with the higher GDM correlated risk of infection reported in the literature (41). Closely associated with the hyperglycemic and hyper-insulinemic state of the newborn of a diabetic mother is hypoglycemia, which is in fact described among the GDM-related adverse neonatal outcomes (41). Indeed, it is the natural consequence of the normal postnatal adaptation to different blood glucose concentrations (49).
The correlation between GDM, perinatal death and congenital anomalies is still controversial, according to a recent review of the literature by Johns et al. (40). These data were then confirmed by an Israeli case-control, retrospective and monocentric study on 526 mothers diagnosed with GDM or pre-gestational diabetes (PGDM) and their children, between 2015 and 2017 (50). Indeed, this investigation revealed that a higher incidence of intrauterine fetal death and congenital anomalies was mainly associated with PGDM, probably also due to the impact of hyperglycemia on embryogenesis during the first 8 weeks of gestation. Other short-term adverse effects associated with the presence of GDM found in the literature (41) are possible neonatal asphyxia, jaundice and kernicterus, neonatal respiratory distress syndrome and birth trauma, including brachial plexus injury and shoulder dystocia, favored by the greater adipose accumulation between the shoulders and the abdominal circumference (47). Polycythemia, hypocalcemia and hypertrophic cardiomyopathy were also found to be GDM-related complications (50, 51). Finally, a significant increase in the thickness of the interventricular septum and of the ventricular wall were found in newborns from mothers with GDM even if in the absence of echocardiographic evidence of congenital heart disease (52).
Long-term complications
The main long-term consequences on the offspring of mothers with GDM, described in the literature, concern glycemic control, obesity, cardiovascular diseases, neuro-development, neuro-psychiatric morbidity and ophthalmic problems.
Already in 2001, a review of the literature by Dabelea and Pettitt (53), highlighted several scientific evidence regarding the fact that exposure to a hyperglycemic intrauterine environment may be an important risk factor, in addition to genetic predisposition, for the onset of type 2 diabetes (T2DM) in childhood and adolescence. Furthermore, several studies conducted in the following years confirmed these first evidence. Indeed, Dabelea et al. (54), also studied a multiethnic population (African American, Hispanic and non-Hispanic) aged between 10 and 22 years. It emerged that 30.4% of young people with type 2 diabetes were exposed to GDM compared to 6.3% in the unexposed group. It was also found that the association between intrauterine GDM exposure and the onset of type 2 diabetes in offspring is not explained by infant BMI, supporting possible beta-cell dysfunction in offspring (54). Similar results were found in a cohort of 18–27-year-olds with Caucasian prevalence, in which the incidence of type 2 diabetes and pre-diabetes (impaired glucose tolerance or impaired fasting glucose) was 21, 12, 11, and 4%, respectively in the offspring of women with GDM treated with diet therapy, in the children of genetically predisposed women but with normal oral glucose tolerance test (OGTT), in the offspring of mothers with type 1 diabetes and in the children of healthy controls (55). In agreement with these data, Holder et al. showed in a cohort of obese adolescents that 31.1% of subjects with normal glucose tolerance exposed to GDM developed a reduction in glucose tolerance/diabetes in the short follow-up period (2.8 years) compared to 8.6% in the non-exposed group (56). Moreover, in this investigation, they showed an inability of pancreatic beta cells to adequately compensate in response to the decrease in insulin sensitivity in the offspring of mothers with GDM.
The presence of metabolic markers of insulin resistance in the offspring of mothers with GDM has also been documented by several studies (57, 58), as well as the higher incidence of diabetes during adulthood (55).
This is confirmed by a recent (59) study, conducted on the same cohort as the HAPO study, aimed at analyzing the correlation between maternal glycemic values during gestation and the effects on glycemic control of the offspring. It proved that the intrauterine hyperglycemia was significantly associated with glucose and insulin resistance in childhood, regardless of the child's BMI and family history of diabetes. At the same time, Blotsky et al. (60) performed a retrospective cohort study on the population of Quebec and Canada which confirmed the association between GDM and the higher incidence of diabetes during childhood and adolescence. They also highlighted the need for future studies to evaluate the long-term consequences, in terms of severity and negative outcomes, in patients with pediatric diabetes resulting from a maternal history of GDM compared to other patients with childhood or juvenile diabetes.
The presence of a correlation between maternal glucose values and neonatal adiposity was already found in the HAPO Study (61), the results of which seemed to suggest that this relationship could be mediated by the production of fetal insulin. As for obesity and overweight during childhood and adolescence of children of diabetic mothers, although there is numerous scientific evidence regarding the existence of an association between BMI in childhood and GDM (62, 63), it is not yet fully understood how much the influence of maternal pre-pregnancy BMI (58, 64) and being large for gestational age infants (LGA), (58, 65) could contribute. In fact, Boerschmann et al. (60) showed that a combination of genetic predisposition, fetal over-nutrition and lifestyle contribute to the growth during infancy of the offspring of mothers with GDM. In this regard, in 2011 a systematic review and a meta-analysis of the literature showed that maternal diabetes is associated with an increase in the z score of the BMI of the offspring of diabetic mothers (66). However, it is no longer evident after correction for pre-pregnancy maternal BMI (in the limited number of studies where it is reported) (66).
Nevertheless, in the same year, Deierlein et al. (67), analyzed BMI values at the age of 3 and found that fetal exposure to high maternal glucose concentrations in the absence of pre-existing diabetes or with GDM may contribute to the development of overweight/obesity in the offspring, regardless of the maternal pre-pregnancy BMI. Similar results were found by Zhu et al. (68), which highlighted the presence of a significant association between fasting plasma glucose concentrations during pregnancy in women with GDM and the size of offspring at birth and the risk of obesity at 7 years, despite correction for pre-pregnancy maternal BMI values. In contrast, from the multinational cross-sectional study by Zhao et al. (69), on 4,740 children aged 9–11, an association, not completely independent of maternal BMI, was found between GDM and higher probabilities of childhood obesity at 9–11 years. Nonetheless, Tam et al. (62) obtained different results on 970 mother-child pairs of the HAPO Study, 7 years after childbirth. This analysis showed that maternal hyperglycemia in pregnancy is independently associated with the risk of obesity of 7-year-old offspring, exclusively for women. Finally, a large retrospective Chinese population-based study by Chen et al. showed that LGA offspring from mothers with GDM had a higher BMI z score and a higher risk of overweight from 1 to 6 years of age. However, the association was attenuated after correction for pre-pregnancy maternal BMI.
The recent systematic review and meta-analysis by Hammoud et al. (65), revealed a correlation between the presence of GDM and higher blood pressures in the offspring. This is probably at the origin of the greater occurrence of cardiovascular problems (CVD) found in GDM offspring from adolescence to adulthood (70, 71). In fact, a recent 40-year follow-up study on a large cohort of the Danish population that included both pregnant women with GDM and with type 1 and type 2 diabetes, revealed that the offspring of diabetic mothers, particularly those with a past history of CVD or other diabetes-related complications, had increased rates of CVD especially of early onset during the first decades of life (71).
The consequences of GDM on the neuro-development and neuro-psychiatric morbidity of the offspring of diabetic mothers are equally relevant (72). Specifically, the main alterations detected, concern the impairment of language development up to middle childhood (73), the mental and psychomotor development (74) as well as the development of neural systems associated with the recognition of facial expression (75), in the first years of life and the performance of explicit memory at the year of age (76). Moreover, they highlighted Worse offspring school entry assessment scores, intelligence quotient (IQ) and General Certificate of Secondary Education scores (GCSE) (77). Furthermore, in 2016, a cohort study (78), demonstrated that GDM exposure is an independent risk factor for long-term neuro-psychiatric morbidity of the offspring, also highlighting a possible correlation with autism spectrum disorders.
Regarding ophthalmic problems, a recent cohort study by Du et al. based on the Danish population (79), found that maternal diabetes during pregnancy is associated with an increased risk of high refractive error (RE) in the offspring from the neonatal period to adulthood, in all age groups (<3 years, 4–15 years and 16–25 years), especially in children of mothers who have developed diabetes-related complications. These results seem to suggest that the intrauterine ocular impairment induced by maternal diabetes may contribute to an incorrect emmetropization at an early age or to a subnormal refractive accommodation during eye growth in childhood and adulthood. These mechanisms, over time, may further contribute to the high risk of developing elevated RE (79), one of the most common vision disorders that represent the second leading cause of disability globally (80).
Metabolomics and children of diabetic mothers
A literature review was performed with the following keywords “metabolomics,” “GDM offspring/infants,” or by cross-referencing in already published reviews. The results are summarized in Table 2.
The studies regarding the analysis of the metabolomic profile of the offspring of diabetic mothers are quite limited despite their potential usefulness from a preventive point of view. In fact, through the metabolomic investigation, it would seem possible to correlate the qualitative and quantitative changes of metabolites in biological samples of offspring of mothers with previous GDM with a possible development of pathologies, in order to diagnose, prevent or implement early therapeutic strategies (89).
The first data in the literature date back to 2012 from Logan et al. (81). They studied urine samples of newborns from mothers with GDM through a 1HNMR analysis, comparing them with a control group, hypothesizing differences in metabolomic profile. The most significant differences that were observed concern the urinary concentrations of glucose, fumarate, formate, citrate and succinate: all involved in the tricarboxylic acid cycle.
In 2014, Dani et al. (82) compared the metabolomic profile of newborns of diabetic mothers with those of healthy mothers in order to evaluate the possible persistence of metabolic alterations despite a close control of the diabetes in pregnancy. They analyzed the cord blood of 30 infants from mothers with GDM and 40 controls, detecting a clear alteration of the metabolomic profile. In fact, lower concentrations of glucose and higher concentrations of pyruvate, histidine, alanine, valine, methionine, arginine, lysine, hypoxanthine, lipoproteins and lipids were detected in children of diabetic mothers. However, they found no significant clinical differences. In 2015, Peng et al. (83) analyzed the metabolomic profile of urine and meconium of 142 newborns from mothers with GDM and 197 controls. Even in this case, the metabolomic profile of the children of diabetic mothers was different from the controls. They found 14 biomarkers in meconium: 10 were endogenous and would seem to be related to gestational diabetes. While, in urine, only 3 metabolites levels were significantly different: uric acid, uridine and estrone. There were also the alterations in lipid, amino acid and purine metabolism in infants from mothers with GDM. In addition, the association between potential biomarkers and related GDM risks were analyzed by a binary logistic regression and an operating characteristic analysis (ROC). Thus, they combined 9 meconium biomarkers in a model which seems to have a great diagnostic potential for the consequences of GDM. Arginosuccinic acid, methyl-adenosine and methyl-guanosine were some of most relevant of those biomarkers.
In 2019, Shokry et al. (84), confirmed the presence of specific related GDM metabolites in the cord blood of children of diabetic mothers. Specifically, the concentrations of free carnitine, acyl-carnitine, long-chain non-esterified fatty acids (NEFA), phospholipids, some intermediates of the Krebs cycle and ß-oxidation (decreased AC2:0/AC16:0 ratios) were lower, supporting possible trans-generational metabolic effects.
Mansell et al. (86) analyzed the metabolomic profile of the cord serum as well, of some subject belonging to the pre-natal cohort of the Barwon Infant Study highlighting that in the presence of GDM there were two distinctive metabolites, vinegar-acetate and 3-hydroxybutyrate, present at higher concentrations. These findings were in agreement with associated GDM ketosis gravidarum, due to lower glucose up-take at the cellular level.
Besides, Zhao et al. (85) found a close correlation between the maternal fecal metabolome and the neonatal blood metabolome. In fact, 4 maternal fecal metabolites, lysine, putrescine, guanidinoacetate and hexadecandiodate, negatively correlated with maternal hyperglycemia, were shown to be responsible for the separation of the metabolome of the neonatal blood of children of diabetic mothers compared to controls. They also showed a correlation between these metabolites and the neonatal metabolome of children suffering from inborn errors of metabolism (IEM) (85). This supports the hypothesis of the important contribution of epigenetic regulation in pathologies with an important genetic basis. In addition, the analysis of metabolic pathways in this study (83), demonstrated a close association between these metabolites and biotin metabolism leading the authors to conclude that biotin metabolism contributes to maternal hyperglycemia as well, and therefore to changes in the neonatal blood metabolome.
Moreover, Chen et al. (87), studied the metabolomic profile of neonatal meconium and that of maternal blood of 418 mother-child pairs from the GDM Mother and Child Study (147 GDM and 271 controls) highlighting a close correlation between the two, supporting the important impact of maternal factors on offspring metabolism. In meconium, an important alteration of the metabolic pathways of taurine, hypo-taurine, pyrimidine and beta-alanine was observed together with variations in the biosynthesis of bile acids.
While, the first work to report quantitative values of concentrations for 101 metabolites measured in the urine of newborns from diabetic mother within the first 24 h of life was performed by. Herrera-Van Oostdam et al. (88). The univariate analysis of these metabolites revealed statistical differences in 11 metabolites, while the multivariate analysis found a different metabolomic profile in neonates of GDM mothers, due to a dysregulation of the metabolism of acyl-carnitines, amino acids and polyamines, together with higher concentrations of hexadecenoyl-carnitine (C16: 1) and spermine, the most discriminant metabolites. The authors pointed out that altered spermine appears to be related to childhood obesity, oxidative stress, circulating leptin values and even possible alterations in the gut microbiota (88).
In this context, Zhao et al. (85) searched for an association between metabolomics and the data on the glycemic response during pregnancy: an analysis of greater proportions, albeit not selective, on GDM. They carried out targeted metabolomic assays on cord blood from European, Afro-Caribbean, Thai and Mexican-American children (400 for each group) whose mothers participated in the HAPO Study (Hyperglycemic and Adverse pregnancy Outcome Study). A meta-analysis of the data collected in the different study cohorts was then carried out, which showed a strong correlation between neonatal and maternal fasting metabolites at 28 weeks of gestation. However, as regards the altered maternal blood sugar, no significant correlations emerged with the amino acid metabolites but rather with a small number of lipid metabolites. Nevertheless, 3-hydroxybutyrate and its carnitine ester at the in the umbilical cord blood were associated with the maternal response to a glucose load (1-h glucose) (90).
On the other hand, there are broader implications in addition to the evaluation of the change in the metabolomic profile of the newborn of a diabetic mother. Indeed, Ott et al. (91) suggest the importance of metabolomic programming by demonstrating that several metabolites, potentially relevant for the development of the disease, are shared between mothers with GDM and their offspring even several years after delivery, regardless of other factors such as BMI or insulin sensitivity. These include carnitine, taurine, creatine, proline, sphingolipid SM (-OH) C14: 1 and glycerophospholipid PC a and C34: 3. These observations led the authors to suppose that this correlation is determined by a shared metabolic pathway rather than merely a transplacental transfer of these metabolites.
Microbiota in children of diabetic mothers
The importance of the microbiota for the onset and development of metabolic diseases is now recognized by the scientific community (92). Nevertheless, neither the individual components of the bacterial flora with protective or favoring action nor the possible intervention targets have yet been defined (93). During pregnancy, this aspect becomes even more relevant. Indeed, if, on the one hand, the maternal microbiota directly affects pregnancy by influencing the intrauterine environment, the vaginal environment and the risk of preterm birth; on the other hand, it would also seem to affect the nascent microbiota of the offspring, with equally important consequences (94, 95). In fact, it is now established that an alteration of the intestinal microbiota in the early stages of development is related to modifications of the immune system, inflammatory, allergic and metabolic diseases (96–98). Moreover, the fact that the third component of breast milk, unlike all other mammalian species, is represented by oligosaccharides (Human Milk Oligosaccharides, HMOs), specific compounds with a marked prebiotic action, supports the importance of the intestinal bacterial flora from the beginning of life (99). To date, the scientific community seems to agree that maternal health directly influences the intestinal microbiota of the fetus, also through a possible vertical mother-child transmission during pregnancy, albeit still in the absence of certainties on the precise mode of intrauterine microbial acquisition (94, 98, 100, 101). Indeed, the dogma that the human fetal environment is sterile in physiological conditions and that the first microbial colonization of the neonatal intestinal tract begins during birth, both vertically (maternal microbiome) and horizontal (environment) has been questioned (95, 98). Recent studies, through new culture-independent microbiological investigation techniques, have therefore hypothesized that the beginning of the colonization of the fetus by the microbiota begins in utero, despite the methodological limitations (95). Specifically, two main transmission routes, still under investigation, have been theorized in healthy pregnancies: a vertical ascension from the vagina and/or urinary tract and a hematogenous pathway through the placenta thanks to a translocation from the digestive tract (buccal cavity and intestine) (98). The detection of the microbial presence in meconium and amniotic fluid is added to further support the hypothesis of colonization in utero, although even in this case there is no agreement in the scientific community due to possible methodological limitations and post-natal contaminations (98).
Nowadays, there is a growing number of studies demonstrating an association between GDM and changes in the intestinal bacterial flora both in the mother (18, 102) and in the offspring (18) and specific alterations of the microbiota seem to be found both in the mother and in the progeny. Furthermore, the trend of some of these altered bacterial species appears similar in the mother-child couple, supporting not only a possible form of intergenerational transmission but also the impact of the maternal microbiota on the intestinal colonization process of the offspring (18).
At present, we have found only 5 studies in the literature conducted on the microbiota of the child of a mother with GDM (Table 3), 4 of which looked specifically at the microbial composition of meconium. We used the keywords “microbiota,” “microbiome,” “GDM infants/offspring” together with cross-referenced bibliographical references.
Among these, Wang et al. (103), analyzed the intestinal microbiota both of pregnant women with GDM and of their offspring, highlighting a significant alteration of the substantial differences compared to controls. Furthermore, they showed not only a strong correlation between some bacterial species and the oral glucose tolerance test but also the same trend in microbial variations in the mother-child pair, supporting an intergenerational analogy in the changes of the GDM-associated microbiota. Similar results were highlighted by Su et al. (93), which confirmed a correlation between the condition of maternal diabetes and microbial alterations, evidencing a decreased alpha-diversity, with some specific phyla among Proteobacteria, Firmicutes, Actinobacteria, Bacteroidetes, Chloroflexi, Acidobacteria, and totally absent Planctomycetes. Overall, it was found that offspring from mothers with GDM were characterized by an increase in Proteobacteria and Actinobacteria phyla and a decrease in Bacteroidetes and at the gender level, a decrease in Prevotella and Lactobacillus was observed. Indeed, from the correlation analysis, maternal fasting glucose levels were positively associated with a relative abundance of the phylum Actinobacteria and the genus Acinetobacter, while they showed a negative correlation with the phylum Bacteroidetes and the genus Prevotella. In this trial, 20 infants from mothers with GDM were enrolled, 15 of them were diagnosed with grade A1 and 5 with grade A2 gestational diabetes. Both groups of diabetic mothers were treated with diet therapy and exercise, however, those with more severe diabetes also received insulin therapy. The children of the latter category of mothers did not show any statistically significant variation in the microbiota compared to the control group. Herrera-Van Oostdam et al. (88) confirmed these data: they found a significant alteration of the microbial communities in meconium of neonates of mother with GDM, highlighting a reduction in alpha-diversity and at the level of phyla, a significant relative abundance of Firmicutes and a significant relative decrease of Proteobacteria.
However, these results represent a novelty in the scientific literature as the only data present up to this time were different and by Hu et al. (105). This group of researchers analyzed meconium samples from 23 neonates stratified according to maternal diabetes status (4 with pre-gestational diabetes mellitus, 5 with GDM and 13 healthy controls), showing that all samples contained a diverse microbiota, albeit with a lower diversity of species and a considerable variation from sample to sample, regardless of the type of birth (vaginal vs. cesarean). As regards the impact of maternal diabetes, only greater alpha-diversity was detected in the microbiota of the group with pre-gestational diabetes mellitus and the authors were able to conclude that the most robust predictor of the composition microbial of meconium could be the state of pre-pregnancy diabetes.
Only Ponzo et al. (104) analyzed fecal samples between the third and fifth day of life, after the expulsion of the meconium. They highlighted that the main cause of differences found in the neonatal microbiota are determined by the type of breastfeeding (breast or formula), with a greater abundance of Bifidobacterium in breastfed infants. However, it was found that some Bacteroides and some Blautia oligotypes are shared between mothers with GDM and their offspring, in support of a maternal imprinting.
The synergy of action of the metabolomic and microbiomic investigation regarding the possible prevention and management of GDM-related complications in offspring, is shown in Figure 1. While, in Table 4 we have summarized the most important biomarkers-target identified in GDM offspring correlated with metabolic alteration through epigenetic, microbiota analysis and metabolomics.
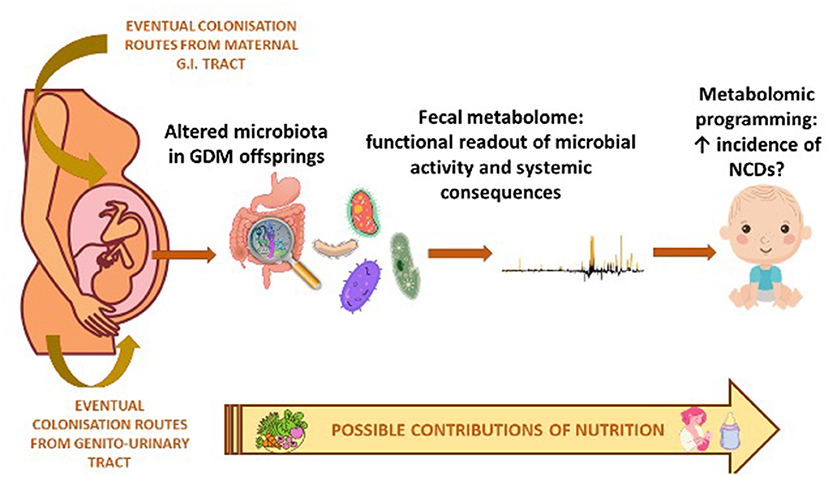
Figure 1. Synergy of action of metabolomic and microbiomic investigation in the offspring of diabetic mother.
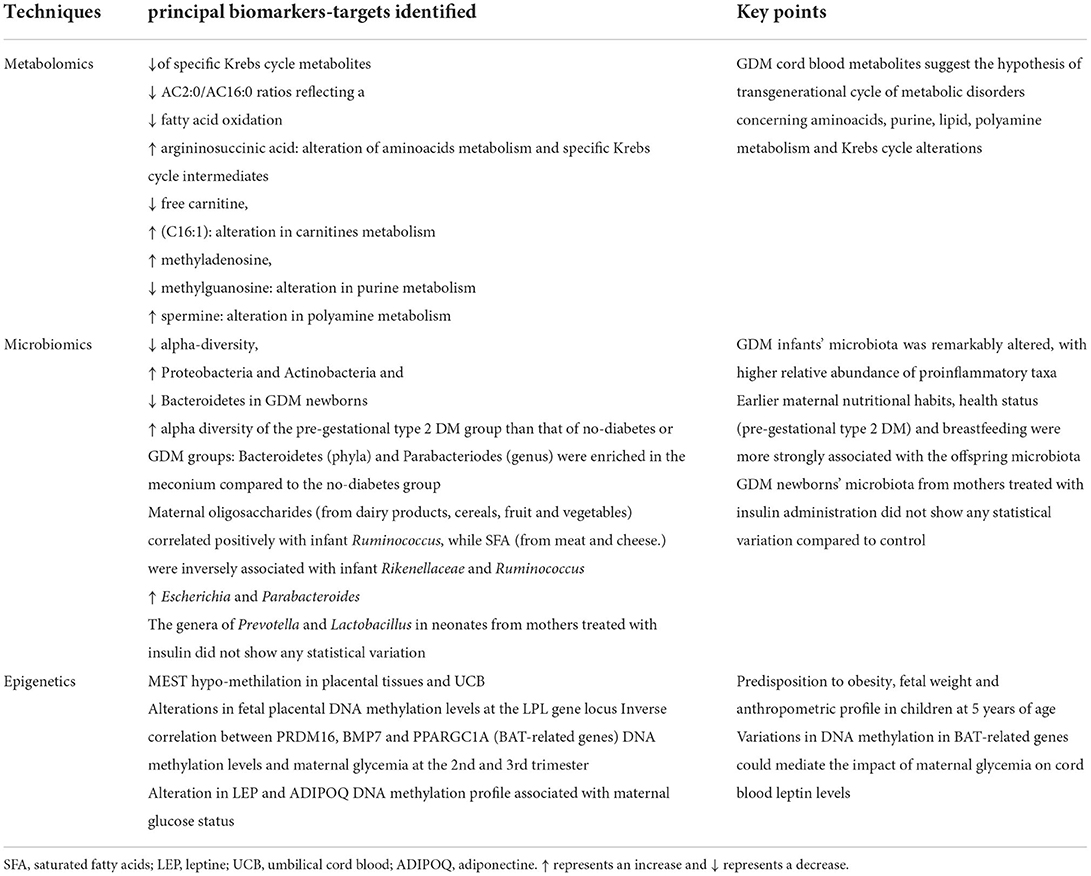
Table 4. Principal biomarkers-target identified in GDM offspring correlated with metabolic alteration.
Conclusion
In recent years, the numerous advances in the field of metabolomics have clearly highlighted its usefulness in the characterization of various physio-pathological processes (106). Indeed, metabolomics makes it possible to obtain a detailed phenotypic portrait through the dynamic detection of the set of metabolites in cells, tissues and various biological fluids. Therefore, the possibility of assessing the metabolism of the individual with respect to the various pathophysiological stimuli such as the intrauterine environment, diet and drug therapies could represent not only an important prevention tool but also an example of personalized medicine (106). As regards the GDM, the innovation of the use of metabolomic investigation is not only limited to the possibility of an early diagnosis during gestation, even before the clinical manifestation of the disease, but it could also improve the prevention and treatment of GDM-related complications in offspring. The most recent literature suggests how fetal malnutrition and the intrauterine environment can determine a permanent alteration of metabolic processes, influencing the risk of onset of chronic diseases and metabolomics could help both in the identification and management of the complex biological mechanisms that affect future health (107).
Furthermore, in pediatrics, nutritional research is mainly focused on the prevention of the development of pathologies as well as a support in case of illnesses. This is due to the fact that nutrition is a strong modulator of most biological processes that greatly affect the maintenance of health. Therefore, the integration of metabolomics and nutrition to establish individual nutritional phenotypes can help to predict the risk of disease (108), especially in categories of particularly fragile subjects, such as children of diabetic mothers.
Our review has several limitations, mainly due to the fact that the study of the impact of gestational diabetes on offspring through metabolomics and microbiomics is completely new. Thus, there is a lack of standardized and validated quantitative methods for clinical application. Hence, further studies are extremely important. In fact, it might be useful, not only to identify more precisely, through standardized biomarkers within metabolomic databases, the particular altered metabolic pathways, but also to assess the extent of metabolomic programming. Thus, the usefulness of metabolomics is that it could provides the detection of metabolites, potentially relevant for the development of diabetes-related complications, shared in the offspring of mothers with GDM both at birth and several years after delivery. This would allow us to understand more precisely the consequences of GDM on offspring that could originate not only from a transplacental transfer of certain metabolites but also be the result of a shared metabolic path.
In this context, the intestinal microbiota plays a very important role as it is the main responsible for the fecal metabolome which in turn provides a functional portrait of the microbial activity and can be used as an intermediate phenotype that mediates host-microorganisms interactions (109).
It has in fact been shown through the fecal metabolome, or secondary metabolites of microbial origin that the intestinal microbiota exerts a large effect on the biochemistry of the host (110). Therefore, it is clear that any disturbance in the composition of the intestinal microbiota can have an important impact on the health of the host through the modification of the intestinal metabolic profile (110).
Furthermore, such extensive and detailed monitoring of the metabolomic profile and microbiota would allow not only to improve the current knowledge on the overall metabolic structure of the child of a diabetic mother, but could have very important clinical implications. In fact, it would be possible to implement an effective lifestyle intervention in subjects “predestined” for the development of metabolic pathologies, preventing or delaying the pathological manifestation. This would also postpone the complications related to it, a necessary condition for controlling the enormous clinical, social and economic burden of this disease, providing a new direction in terms of early prevention and treatment of GDM. Finally, it would be desirable to manage any alterations detected also through a “personalized nutrition” in perfect harmony with the precise phenotype.
Therefore, it clearly emerges the need for the integration of multi-omics (metabolomics and microbiomics) datasets from different populations, as demonstrated by other authors (111).
Author contributions
AD wrote and revised the manuscript and original idea for this manuscript. CT and AB wrote the manuscript and revised the literature. RP and VF revised the manuscript. All authors have read and accepted the final version of this manuscript.
Conflict of interest
The authors declare that the research was conducted in the absence of any commercial or financial relationships that could be construed as a potential conflict of interest.
Publisher's note
All claims expressed in this article are solely those of the authors and do not necessarily represent those of their affiliated organizations, or those of the publisher, the editors and the reviewers. Any product that may be evaluated in this article, or claim that may be made by its manufacturer, is not guaranteed or endorsed by the publisher.
References
1. American Diabetes Association. 2. Classification and diagnosis of diabetes: standards of medical care in diabetes-2019. Diabetes Care. (2019) 42(Suppl. 1):S13–28. doi: 10.2337/dc19-S002
2. Plows JF, Stanley JL, Baker PN, Reynolds CM, Vickers MH. The pathophysiology of gestational diabetes mellitus J Mol Sci. (2018) 19:3342. doi: 10.3390/ijms19113342
3. Alejandro EU, Mamerto TP, Chung G, Villavieja A, Gaus NL, Morgan E, et al. Gestational diabetes mellitus: a harbinger of the vicious cycle of diabetes. Int J Mol Sci. (2020) 21:5003. doi: 10.3390/ijms21145003
4. Vitacolonna E, Succurro E, Lapolla A, Scavini M, Bonomo M, Di Cianni G, et al. Guidelines for the screening and diagnosis of gestational diabetes in Italy from 2010 to 2019: critical issues and the potential for improvement. Acta Diabetol. (2019) 56:1159–67. doi: 10.1007/s00592-019-01397-4
5. Metzger BE, Lowe LP, Dyer AR, Trimble ER, Chaovarindr U, Coustan DR, et al. Hyperglycemia and adverse pregnancy outcomes. N Engl J Med. (2008) 358:1991–2002. doi: 10.1056/NEJMoa0707943
6. Cho NH, Shaw JE, Karuranga S, Huang Y, da Rocha Fernandes JD, Ohlrogge AW, et al. IDF Diabetes Atlas: Global estimates of diabetes prevalence for 2017 and projections for 2045. Diabetes Res Clin Pract. (2018) 138:271–81. doi: 10.1016/j.diabres.2018.02.023
7. International Diabetes Federation. IDF. Diabetes Atlas. 9th ed. Brussels: International Diabetes Federation (2019).
8. Zhu Y, Zhang C. Prevalence of gestational diabetes and risk of progression to type 2 diabetes: a global perspective. Curr Diab Rep. (2016) 16:7. doi: 10.1007/s11892-015-0699-x
9. Yuen L, Wong VW, Simmons D. Ethnic disparities in gestational diabetes. Curr Diab Rep. (2018) 18:68. doi: 10.1007/s11892-018-1040-2
10. Ghesquière L, Garabedian C, Drumez E, Lemaître M, Cazaubiel M, Bengler C, et al. Effects of COVID-19 pandemic lockdown on gestational diabetes mellitus: a retrospective study. Diabetes Metab. (2021) 47:101201. doi: 10.1016/j.diabet.2020.09.008
11. Cauldwell M, van-de-L'Isle Y, Watt Coote I, Steer PJ. Seasonal and SARS-CoV-2 pandemic changes in the incidence of gestational diabetes. BJOG. (2021) 128:1881–7. doi: 10.1111/1471-0528.16779
12. Gillman MW, Barker D, Bier D, Cagampang F, Challis J, Fall C, et al. Meeting report on the 3rd international congress on developmental origins of health and disease (DOHaD). Pediatr Res. (2007) 61:625–9. doi: 10.1203/pdr.0b013e3180459fcd
13. Bouchard L, Thibault S, Guay SP, Santure M, Monpetit A, St-Pierre J, et al. Leptin gene epigenetic adaptation to impaired glucose metabolism during pregnancy. Diabetes Care. (2010) 33:2436–41. doi: 10.2337/dc10-1024
14. Bouchard L, Hivert MF, Guay SP, St-Pierre J, Perron P, Brisson D. Placental adiponectin gene DNA methylation levels are associated with mothers' blood glucose concentration. Diabetes. (2012) 6:1272–80. doi: 10.2337/db11-1160
15. Gagné-Ouellet V, Houde AA, Guay SP, Perron P, Gaudet D, Guérin R, et al. Placental lipoprotein lipase DNA methylation alterations are associated with gestational diabetes and body composition at 5 years of age. Epigenetics. (2017) 12:616–25. doi: 10.1080/15592294.2017.1322254
16. Mussap M, Antonucci R, Noto A, Fanos V. The role of metabolomics in neonatal and pediatric laboratory medicine. Clin Chim Acta. (2013) 426:127–38. doi: 10.1016/j.cca.2013.08.020
17. Ponzo V, Fedele D, Goitre I, Leone F, Lezo A, Monzeglio C, et al. Diet-gut microbiota interactions and gestational diabetes mellitus (GDM). Nutrients. (2019) 11:330. doi: 10.3390/nu11020330
18. Li X, Yu D, Wang Y, Yuan H, Ning X, Rui B, et al. The intestinal dysbiosis of mothers with gestational diabetes mellitus (GDM) and its impact on the gut microbiota of their newborns. Can J Infect Dis Med Microbiol. (2021) 2021:3044534. doi: 10.1155/2021/3044534
19. Elliott HR, Sharp GC, Relton CL, Lawlor DA. Epigenetics and gestational diabetes: a review of epigenetic epidemiology studies and their use to explore epigenetic mediation and improve prediction. Diabetologia. (2019) 62:2171–8. doi: 10.1007/s00125-019-05011-8
20. Franzago M, Fraticelli F, Stuppia L, Vitacolonna E. Nutrigenetics, epigenetics and gestational diabetes: consequences in mother and child. Epigenetics. (2019) 14:215–35. doi: 10.1080/15592294.2019.1582277
21. Ruchat SM, Houde AA, Voisin G, St-Pierre J, Perron P, Baillargeon JP, et al. Gestational diabetes mellitus epigenetically affects genes predominantly involved in metabolic diseases. Epigenetics. (2013) 8:935–43. doi: 10.4161/epi.25578
22. Ott R, Stupin JH, Melchior K, Schellong K, Ziska T, Dudenhausen JW, et al. Alterations of adiponectin gene expression and DNA methylation in adipose tissues and blood cells are associated with gestational diabetes and neonatal outcome. Clin Epigenetics. (2018) 10:131. doi: 10.1186/s13148-018-0567-z
23. García-Cardona MC, Huang F, García-Vivas JM, López-Camarillo C, Del Río Navarro BE, Navarro Olivos E, et al. DNA methylation of leptin and adiponectin promoters in children is reduced by the combined presence of obesity and insulin resistance. Int J Obes. (2014) 38:1457–65. doi: 10.1038/ijo.2014.30
24. Gagné-Ouellet V, Breton E, Thibeault K, Fortin CA, Cardenas A, Guérin R, et al. Mediation analysis supports a causal relationship between maternal hyperglycemia and placental DNA methylation variations at the leptin gene locus and cord blood leptin levels. Int J Mol Sci. (2020) 21:329. doi: 10.3390/ijms21010329
25. Wang WJ, Huang R, Zheng T, Du Q, Yang MN, Xu YJ, et al. Genome-wide placental gene methylations in gestational diabetes mellitus, fetal growth and metabolic health biomarkers in cord blood. Front Endocrinol. (2022) 13:875180. doi: 10.3389/fendo.2022.875180
26. Côté S, Gagné-Ouellet V, Guay SP, Allard C, Houde AA, Perron P, et al. PPARGC1α gene DNA methylation variations in human placenta mediate the link between maternal hyperglycemia and leptin levels in newborns. Clin Epigenetics. (2016) 8:72. doi: 10.1186/s13148-016-0239-9
27. El Hajj N, Pliushch G, Schneider E, Dittrich M, Müller T, Korenkov M, et al. Metabolic programming of MEST DNA methylation by intrauterine exposure to gestational diabetes mellitus. Diabetes. (2013) 62:1320–8. doi: 10.2337/db12-0289
28. Lefebvre L, Viville S, Barton SC, Ishino F, Keverne EB, Surani MA. Abnormal maternal behaviour and growth retardation associated with loss of the imprinted gene Mest. Nat Genet. (1998) 20:163–9. doi: 10.1038/2464
29. Koza RA, Nikonova L, Hogan J, Rim JS, Mendoza T, Faulk C, et al. Changes in gene expression foreshadow diet-induced obesity in genetically identical mice. PLoS Genet. (2006) 2:e81. doi: 10.1371/journal.pgen.0020081
30. Kamei Y, Suganami T, Kohda T, Ishino F, Yasuda K, Miura S, et al. Peg1/Mest in obese adipose tissue is expressed from the paternal allele in an isoform-specific manner. FEBS Lett. (2007) 581:91–6. doi: 10.1016/j.febslet.2006.12.002
31. Karbiener M, Glantschnig C, Pisani DF, Laurencikiene J, Dahlman I, Herzig S, et al. Mesoderm-specific transcript (MEST) is a negative regulator of human adipocyte differentiation. Int J Obes. (2015) 39:1733–41. doi: 10.1038/ijo.2015.121
32. Takahashi M, Kamei Y, Ezaki O. Mest/Peg1 imprinted gene enlarges adipocytes and is a marker of adipocyte size. Am J Physiol Endocrinol Metab. (2005) 288:E117–24. doi: 10.1152/ajpendo.00244.2004
33. Jung H, Lee SK, Jho EH. Mest/Peg1 inhibits Wnt signalling through regulation of LRP6 glycosylation. Biochem J. (2011) 436:263–9. doi: 10.1042/BJ20101512
34. Nikonova L, Koza RA, Mendoza T, Chao PM, Curley JP, Kozak LP. Mesoderm-specific transcript is associated with fat mass expansion in response to a positive energy balance. FASEB J. (2008) 22:3925–37. doi: 10.1096/fj.08-108266
35. Blazevic S, Horvaticek M, Kesic M, Zill P, Hranilovic D, Ivanisevic M, et al. Epigenetic adaptation of the placental serotonin transporter gene (SLC6A4) to gestational diabetes mellitus. PLoS ONE. (2017) 12:e0179934. doi: 10.1371/journal.pone.0179934
36. Mishra JS, Zhao H, Hattis S, Kumar S. Elevated glucose and insulin levels decrease DHA transfer across human trophoblasts via SIRT1-dependent mechanism. Nutrients. (2020) 12:1271. doi: 10.3390/nu12051271
37. Judge MP, Casavant SG, Dias JA, McGrath JM. Reduced DHA transfer in diabetic pregnancies: mechanistic basis and long-term neurodevelopmental implications. Nutr Rev. (2016) 74:411–20. doi: 10.1093/nutrit/nuw006
38. Devarshi PP, Grant RW, IkonteCJ, Hazels Mitmesser S. Maternal omega-3 nutrition, placental transfer and fetal brain development in gestational diabetes and preeclampsia. Nutrients. (2019) 11:1107. doi: 10.3390/nu11051107
39. Howe CG, Cox B, Fore R, Jungius J, Kvist T, Lent S, et al. Maternal gestational diabetes mellitus and newborn DNA methylation: findings from the pregnancy and childhood epigenetics consortium. Diabetes Care. (2020) 43:98–105. doi: 10.2337/dc19-0524
40. Johns EC, Denison FC, Norman JE, Reynolds RM. Gestational diabetes mellitus: mechanisms, treatment, and complications. Trends Endocrinol Metab. (2018) 29:743–54. doi: 10.1016/j.tem.2018.09.004
41. Kc K, Shakya S, Zhang H. Gestational diabetes mellitus and macrosomia: a literature review. Ann Nutr Metab. (2015) 66(Suppl. 2):14–20. doi: 10.1159/000371628
42. Wang, X, Zhang, X, Zhou, M, Juan, J, Wang X. Association of gestational diabetes mellitus with adverse pregnancy outcomes and its interaction with maternal age in Chinese urban women. J Diabetes Res. (2021) 2021:5516937. doi: 10.1155/2021/5516937
43. Srichumchit S, Luewan S, Tongsong T. Outcomes of pregnancy with gestational diabetes mellitus. Int J Gynaecol Obstet. (2015) 131:251–4. doi: 10.1016/j.ijgo.2015.05.033
44. Catalano PM, McIntyre HD, Cruickshank JK, McCance DR, Dyer AR, Metzger BE, et al. HAPO Study cooperative research group. the hyperglycemia and adverse pregnancy outcome study: associations of GDM and obesity with pregnancy outcomes. Diabetes Care. (2012) 35:780–6. doi: 10.2337/dc11-1790
45. Seghieri G, Di Cianni G, Seghieri M, Lacaria E, Corsi E, Lencioni C, et al. Risk and adverse outcomes of gestational diabetes in migrants: a population cohort study. Diabetes Res Clin Pract. (2020) 163:108128. doi: 10.1016/j.diabres.2020.108128
46. Li M, Hinkle SN, Grantz KL, Kim S, Grewal J, Grobman WA, et al. Glycaemic status during pregnancy and longitudinal measures of fetal growth in a multi-racial US population: a prospective cohort study. Lancet Diabetes Endocrinol. (2020) 8:292–300. doi: 10.1016/S2213-8587(20)30024-3
47. Sovio U, Murphy HR, Smith GC. Accelerated fetal growth prior to diagnosis of gestational diabetes mellitus: a prospective cohort study of nulliparous women. Diabetes Care. (2016) 39:982–7. doi: 10.2337/dc16-0160
48. Zhang X, Liao Q, Wang F, Li D. Association of gestational diabetes mellitus and abnormal vaginal flora with adverse pregnancy outcomes. Medicine. (2018) 97:e11891. doi: 10.1097/MD.0000000000011891
49. Sweet CB, Grayson S, Polak M. Management strategies for neonatal hypoglycemia. J Pediatr Pharmacol Ther. (2013) 18:199–208. doi: 10.5863/1551-6776-18.3.199
50. Riskin A, Itzchaki O, Bader D, Iofe A, Toropine A, Riskin-Mashiah S. Perinatal outcomes in infants of mothers with diabetes in pregnancy. Isr Med Assoc J. (2020) 22:569–75.
51. Hay WW Jr. Care of the infant of the diabetic mother. Curr Diab Rep. (2012) 12:4–15. doi: 10.1007/s11892-011-0243-6
52. Garg S, Sharma P, Sharma D, Behera V, Durairaj M, Dhall A. Use of fetal echocardiography for characterization of fetal cardiac structure in women with normal pregnancies and gestational diabetes mellitus. J Ultrasound Med. (2014) 33:1365–9. doi: 10.7863/ultra.33.8.1365
53. Dabelea D, Pettitt DJ. Intrauterine diabetic environment confers risks for type 2 diabetes mellitus and obesity in the offspring, in addition to genetic susceptibility. J Pediatr Endocrinol Metab. (2001) 14:1085–91. doi: 10.1515/jpem-2001-0803
54. Dabelea D, Mayer-Davis EJ, Lamichhane AP, D'Agostino RB Jr, Liese AD, Vehik KS, et al. Association of intrauterine exposure to maternal diabetes and obesity with type 2 diabetes in youth: the SEARCH case-control study. Diabetes Care. (2008) 31:1422–6. doi: 10.2337/dc07-2417
55. Clausen TD, Mathiesen ER, Hansen T, Pedersen O, Jensen DM, Lauenborg J, et al. High prevalence of type 2 diabetes and pre-diabetes in adult offspring of women with gestational diabetes mellitus or type 1 diabetes: the role of intrauterine hyperglycemia. Diabetes Care. (2008) 31:340–6. doi: 10.2337/dc07-1596
56. Holder T, Giannini C, Santoro N, Pierpont B, Shaw M, Duran E, et al. A low disposition index in adolescent offspring of mothers with gestational diabetes: a risk marker for the development of impaired glucose tolerance in youth. Diabetologia. (2014) 57:2413–20. doi: 10.1007/s00125-014-3345-2
57. Keely EJ, Malcolm JC, Hadjiyannakis S, Gaboury I, Lough G, Lawson ML. Prevalence of metabolic markers of insulin resistance in offspring of gestational diabetes pregnancies. Pediatr Diabetes. (2008) 9:53–9. doi: 10.1111/j.1399-5448.2007.00258.x
58. Boerschmann H, Pflüger M, Henneberger L, Ziegler AG, Hummel S. Prevalence and predictors of overweight and insulin resistance in offspring of mothers with gestational diabetes mellitus. Diabetes Care. (2010) 33:1845–9. doi: 10.2337/dc10-0139
59. Scholtens DM, Kuang A, Lowe LP, Hamilton J, Lawrence JM, Lebenthal Y, et al. Hyperglycemia and adverse pregnancy outcome follow-up study (HAPO FUS): maternal glycemia and childhood glucose metabolism. Diabetes Care. (2019) 42:381–92. doi: 10.2337/dc18-2021
60. Blotsky AL, Rahme, E, Dahhou, M, Nakhla, M, Dasgupta K. Gestational diabetes associated with incident diabetes in childhood and youth: a retrospective cohort study. CMAJ. (2019) 191:E410–17. doi: 10.1503/cmaj.181001
61. HAPO Study Cooperative Research Group. Hyperglycemia and adverse pregnancy outcome (HAPO) Study: associations with neonatal anthropometrics. Diabetes. (2009) 58:453–9. doi: 10.2337/db08-1112
62. Tam WH, Ma RCW, Ozaki R, Li AM, Chan MHM, Yuen LY, et al. In utero exposure to maternal hyperglycemia increases childhood cardiometabolic risk in offspring. Diabetes Care. (2017) 40:679–86. doi: 10.2337/dc16-2397
63. Pathirana MM, Lassi ZS, Roberts CT, Andraweera PH. Cardiovascular risk factors in offspring exposed to gestational diabetes mellitus in utero: systematic review and meta-analysis. J Dev Orig Health Dis. (2020) 11:599–616. doi: 10.1017/S2040174419000850
64. Chen YL, Han LL, Shi XL, Su WJ, Liu W, Wang LY, et al. Adverse pregnancy outcomes on the risk of overweight offspring: a population-based retrospective study in Xiamen, China. Sci Rep. (2020) 10:1549. doi: 10.1038/s41598-020-58423-7
65. Hammoud NM, Visser GHA, van Rossem L, Biesma DH, Wit JM, de Valk HW. Long-term BMI and growth profiles in offspring of women with gestational diabetes. Diabetologia. (2018) 61:1037–45. doi: 10.1007/s00125-018-4584-4
66. Philipps LH, Santhakumaran S, Gale C, Prior E, Logan KM, Hyde MJ, et al. The diabetic pregnancy and offspring BMI in childhood: a systematic review and meta-analysis. Diabetologia. (2011) 54:1957–66. doi: 10.1007/s00125-011-2180-y
67. Deierlein AL, Siega-Riz AM, Chantala K, Herring AH. The association between maternal glucose concentration and child BMI at age 3 years. Diabetes Care. (2011) 34:480–4. doi: 10.2337/dc10-1766
68. Zhu Y, Olsen SF, Mendola P, Yeung EH, Vaag A, Bowers K, et al. Growth and obesity through the first 7 y of life in association with levels of maternal glycemia during pregnancy: a prospective cohort study. Am J Clin Nutr. (2016) 103:794–800. doi: 10.3945/ajcn.115.121780
69. Zhao P, Liu E, Qiao Y, Katzmarzyk PT, Chaput JP, Fogelholm M, et al. Maternal gestational diabetes and childhood obesity at age 9-11: results of a multinational study. Diabetologia. (2016) 59:2339–48. doi: 10.1007/s00125-016-4062-9
70. Leybovitz-Haleluya N, Wainstock T, Landau D, Sheiner E. Maternal gestational diabetes mellitus and the risk of subsequent pediatric cardiovascular diseases of the offspring: a population-based cohort study with up to 18 years of follow up. Acta Diabetol. (2018) 55:1037–42. doi: 10.1007/s00592-018-1176-1
71. Yu Y, Arah OA, Liew Z, Cnattingius S, Olsen J, Sørensen HT, et al. Maternal diabetes during pregnancy and early onset of cardiovascular disease in offspring: population based cohort study with 40 years of follow-up. BMJ. (2019) 367:l6398. doi: 10.1136/bmj.l6398
72. Farahvar S, Walfisc A, Sheiner E. Gestational diabetes risk factors and long-term consequences for both mother and offspring: a literature review. Expert Rev Endocrinol Metab. (2019) 14:63–74. doi: 10.1080/17446651.2018.1476135
73. Dionne G, Boivin M, Séguin JR, Pérusse D, Tremblay RE. Gestational diabetes hinders language development in offspring. Pediatrics. (2008) 122:e1073–9. doi: 10.1542/peds.2007-3028
74. Camprubi Robles M, Campoy C, Garcia Fernandez L, Lopez-Pedrosa JM, Rueda R, Martin MJ. Maternal diabetes and cognitive performance in the offspring: a systematic review and meta-analysis. PLoS ONE. (2015) 10:e0142583. doi: 10.1371/journal.pone.0142583
75. Cordón IM, Georgieff MK, Nelson CA. Neural correlates of emotion processing in typically developing children and children of diabetic mothers. Dev Neuropsychol. (2009) 34:683–700. doi: 10.1080/87565640903265129
76. DeBoer T, Wewerka S, Bauer PJ, Georgieff MK, Nelson CA. Explicit memory performance in infants of diabetic mothers at 1 year of age. Dev Med Child Neurol. (2005) 47:525–31. doi: 10.1017/S0012162205001039
77. Fraser A, Nelson SM, Macdonald-Wallis C, Lawlor DA. Associations of existing diabetes, gestational diabetes, and glycosuria with offspring IQ and educational attainment: the avon longitudinal study of parents and children. Exp Diabetes Res. (2012) 2012:963735. doi: 10.1155/2012/963735
78. Nahum Sacks K, Friger M, Shoham-Vardi I, Abokaf H, Spiegel E, Sergienko R, et al. Prenatal exposure to gestational diabetes mellitus as an independent risk factor for long-term neuropsychiatric morbidity of the offspring. Am J Obstet Gynecol. (2016) 215:380.e1–7. doi: 10.1016/j.ajog.2016.03.030
79. Du J, Li J, Liu X, Liu H, Obel C, Shen H, et al. Association of maternal diabetes during pregnancy with high refractive error in offspring: a nationwide population-based cohort study. Diabetologia. (2021) 64:2466–77. doi: 10.1007/s00125-021-05526-z
81. Logan KM, Wijeyesekera AD, Perez IG, Hyde MJ, Romero MG, Jeffries S, et al. Infants of Mothers With Diabetes Have Altered Urinary Metabolic Profile at Birth. Available online at: www.neonatalsociety.ac.uk/abstracts/logank_2012_diabeticurinaryprofile.shtml (accessed December 23, 2021).
82. Dani C, Bresci C, Berti E, Ottanelli S, Mello G, Mecacci F, et al. Metabolomic profile of term infants of gestational diabetic mothers. J Matern Fetal Neonatal Med. (2014) 27:537–42. doi: 10.3109/14767058.2013.823941
83. Peng S, Zhang J, Liu L, Zhang X, Huang Q, Alamdar A, et al. Newborn meconium and urinary metabolome response to maternal gestational diabetes mellitus: a preliminary case-control study. J Proteome Res. (2015) 14:1799–809. doi: 10.1021/pr5011857
84. Shokry E, Marchioro L, Uhl O, Bermúdez MG, García-Santos JA, Segura MT, et al. Impact of maternal BMI and gestational diabetes mellitus on maternal and cord blood metabolome: results from the PREOBE cohort study. Acta Diabetol. (2019) 56:421–30. doi: 10.1007/s00592-019-01291-z
85. Zhao C, Ge J, Li X, Jiao R, Li Y, Quan H, et al. Integrated metabolome analysis reveals novel connections between maternal fecal metabolome and the neonatal blood metabolome in women with gestational diabetes mellitus. Sci Rep. (2020) 10:3660. doi: 10.1038/s41598-020-60540-2
86. Mansell T, Vlahos A, Collier F, Ponsonby AL, Vuillermin P, Ellul S, et al. The newborn metabolome: associations with gestational diabetes, sex, gestation, birth mode, and birth weight. Pediatr Res. (2022) 91:1864–73. doi: 10.1038/s41390-021-01672-7
87. Chen T, Qin Y, Chen M, Zhang Y, Wang X, Dong T, et al. Gestational diabetes mellitus is associated with the neonatal gut microbiota and metabolome. BMC Med. (2021) 19:120. doi: 10.1186/s12916-021-01991-w
88. Herrera-Van Oostdam AS, Salgado-Bustamante M, Lima-Rogel V, Oropeza-Valdez JJ, López JA, Rodríguez IDR, et al. Urinary Metabolomic Profile of Neonates Born to Women with Gestational Diabetes Mellitus. Metabolites. (2021) 11:723. doi: 10.3390/metabo11110723
89. Dessì A, Marincola FC, Fanos V. Metabolomics and the great obstetrical syndromes–GDM, PET, and IUGR. Best Pract Res Clin Obstet Gynaecol. (2015) 29:156–64. doi: 10.1016/j.bpobgyn.2014.04.023
90. Lowe WL Jr, Bain JR, Nodzenski M, Reisetter AC, Muehlbauer MJ, Stevens RD, et al. Maternal BMI and glycemia impact the fetal metabolome. Diabetes Care. (2017) 40:902–10. doi: 10.2337/dc16-2452
91. Ott R, Pawlow X, Weiß A, Hofelich A, Herbst M, Hummel N, et al. Intergenerational metabolomic analysis of mothers with a history of gestational diabetes mellitus and their offspring. Int J Mol Sci. (2020) 21:9647. doi: 10.3390/ijms21249647
92. Qin J, Li Y, Cai Z, Li S, Zhu J, Zhang F, et al. A metagenome-wide association study of gut microbiota in type 2 diabetes. Nature. (2012) 490:55–60. doi: 10.1038/nature11450
93. Su M, Nie Y, Shao R, Duan S, Jiang Y, Wang M, et al. Diversified gut microbiota in newborns of mothers with gestational diabetes mellitus. PLoS ONE. (2018) 13:e0205695. doi: 10.1371/journal.pone.0205695
94. Neri C, Serafino E, Morlando M, Familiari A. Microbiome and gestational diabetes: interactions with pregnancy outcome and long-term infant health. J Diabetes Res. (2021) 2021:9994734. doi: 10.1155/2021/9994734
95. Coscia A, Bardanzellu F, Caboni E, Fanos V, Peroni DG. When a neonate is born, so is a microbiota. Life. 2021) 11:148. doi: 10.3390/life11020148
96. Buchanan KL, Bohórquez DV. You are what you (first) eat. Front Hum Neurosci. (2018) 12:323. doi: 10.3389/fnhum.2018.00323
97. Chong CYL, Bloomfield FH, O'Sullivan JM. Factors affecting gastrointestinal microbiome development in neonates. Nutrients. (2018) 10:274. doi: 10.3390/nu10030274
98. Milani C, Duranti S, Bottacini F, Casey E, Turroni F, Mahony J, et al. The first microbial colonizers of the human gut: composition, activities, and health implications of the infant gut microbiota. Microbiol Mol Biol Rev. (2017) 81:e00036–17. doi: 10.1128/MMBR.00036-17
99. Corona L, Lussu A, Bosco A, Pintus R, Cesare Marincola F, Fanos V, et al. Human milk oligosaccharides: a comprehensive review towards metabolomics. Children. (2021) 8:804. doi: 10.3390/children8090804
100. Asnicar F, Manara S, Zolfo M, Truong DT, Scholz M, Armanini F, et al. Studying vertical microbiome transmission from mothers to infants by strain-level metagenomic profiling. mSystems. (2017) 2:e00164–16. doi: 10.1128/mSystems.00164-16
101. Ferretti P, Pasolli E, Tett A, Asnicar F, Gorfer V, Fedi S, et al. Mother-to-infant microbial transmission from different body sites shapes the developing infant gut microbiome. Cell Host Microbe. (2018) 24:133–45.e5. doi: 10.1016/j.chom.2018.06.005
102. Wu Y, Bible PW, Long S, Ming WK, Ding W, Long Y, et al. Metagenomic analysis reveals gestational diabetes mellitus-related microbial regulators of glucose tolerance. Acta Diabetol. (2020) 57:569–81. doi: 10.1007/s00592-019-01434-2
103. Wang J, Zheng J, Shi W, Du N, Xu X, Zhang Y, et al. Dysbiosis of maternal and neonatal microbiota associated with gestational diabetes mellitus. Gut. (2018) 67:1614–25. doi: 10.1136/gutjnl-2018-315988
104. Ponzo V, Ferrocino I, Zarovska A, Amenta MB, Leone F, Monzeglio C, et al. The microbiota composition of the offspring of patients with gestational diabetes mellitus (GDM). PLoS ONE. (2019) 14:e0226545. doi: 10.1371/journal.pone.0226545
105. Hu J, Nomura Y, Bashir A, Fernandez-Hernandez H, Itzkowitz S, Pei Z, et al. Diversified microbiota of meconium is affected by maternal diabetes status. PLoS ONE. (2013) 8:e78257. doi: 10.1371/journal.pone.0078257
106. Bardanzellu F, Fanos V. How could metabolomics change pediatric health? Ital J Pediatr. (2020) 46:37. doi: 10.1186/s13052-020-0807-7
107. Dessì, A, Puddu, M, Ottonello, G, Fanos V. Metabolomics and fetal-neonatal nutrition: between “not enough” and “too much”. Molecules. (2013) 18, 11724-32. doi: 10.3390/molecules181011724
108. Dessì A, Cesare Marincola F, Masili A, Gazzolo D, Fanos V. Clinical metabolomics and nutrition: the new frontier in neonatology and pediatrics. Biomed Res Int. (2014) 2014:981219. doi: 10.1155/2014/981219
109. Zierer J, Jackson MA, Kastenmüller G, Mangino M, Long T, Telenti A, et al. The fecal metabolome as a functional readout of the gut microbiome. Nat Genet. (2018) 50:790–5. doi: 10.1038/s41588-018-0135-7
110. Tarracchini C, Milani C, Longhi G, Fontana F, Mancabelli L, Pintus R, et al. Unraveling the microbiome of necrotizing enterocolitis: insights in novel microbial and metabolomic biomarkers. Microbiol Spectr. (2021) 9:e0117621. doi: 10.1128/Spectrum.01176-21
Keywords: GDM, metabolomics, microbiota, microbiome, obesity
Citation: Dessì A, Tognazzi C, Bosco A, Pintus R and Fanos V (2022) Metabolomic profiles and microbiota of GDM offspring: The key for future perspective? Front. Pediatr. 10:941800. doi: 10.3389/fped.2022.941800
Received: 12 May 2022; Accepted: 15 August 2022;
Published: 05 October 2022.
Edited by:
George Paltoglou, National and Kapodistrian University of Athens, GreeceReviewed by:
Ivana I. Kavecan, University of Novi Sad, SerbiaPrasoon Agarwal, Uppsala University, Sweden
Copyright © 2022 Dessì, Tognazzi, Bosco, Pintus and Fanos. This is an open-access article distributed under the terms of the Creative Commons Attribution License (CC BY). The use, distribution or reproduction in other forums is permitted, provided the original author(s) and the copyright owner(s) are credited and that the original publication in this journal is cited, in accordance with accepted academic practice. No use, distribution or reproduction is permitted which does not comply with these terms.
*Correspondence: Alice Bosco, YWxpY2Vib3Njbzg4JiN4MDAwNDA7Z21haWwuY29t