- 1Department of Pediatrics, Children’s Hospital of Philadelphia, Philadelphia, PA, United States
- 2Perelman School of Medicine, University of Pennsylvania, Philadelphia, PA, United States
Kingella kingae is an emerging pediatric pathogen and is increasingly recognized as a leading etiology of septic arthritis, osteomyelitis, and bacteremia and an occasional cause of endocarditis in young children. The pathogenesis of K. kingae disease begins with colonization of the upper respiratory tract followed by breach of the respiratory epithelial barrier and hematogenous spread to distant sites of infection, primarily the joints, bones, and endocardium. As recognition of K. kingae as a pathogen has increased, interest in defining the molecular determinants of K. kingae pathogenicity has grown. This effort has identified numerous bacterial surface factors that likely play key roles in the pathogenic process of K. kingae disease, including type IV pili and the Knh trimeric autotransporter (adherence to the host), a potent RTX-family toxin (epithelial barrier breach), and multiple surface polysaccharides (complement and neutrophil resistance). Herein, we review the current state of knowledge of each of these factors, providing insights into potential approaches to the prevention and/or treatment of K. kingae disease.
Introduction
Advances in both culture-based and molecular-based diagnostics have led to increased recognition of Kingella kingae as an important pediatric pathogen and have stimulated interest by the research community in understanding the molecular determinants of K. kingae pathogenicity. Epidemiological surveillance studies have established that the predominant niche of K. kingae is the oropharynx of children ages 6 months to 4 years (1). The organism can be detected in approximately 10% of healthy children in this age range (1–3). In a subset of colonized children, K. kingae is able to produce invasive disease, primarily septic arthritis, osteomyelitis, tenosynovitis, and bacteremia and more rarely endocarditis and meningitis (2–4).
For K. kingae to produce invasive disease, it must accomplish several critical steps in the pathogenic process. The organism must first adhere to the respiratory epithelium to colonize the oropharynx. After successful colonization, the bacterium must breach the respiratory epithelial barrier and enter the bloodstream. As dissemination to the sites of invasive disease occurs through the hematogenous route, the organism must survive in the hostile intravascular environment and seed a typically sterile body site (e.g., bones, joints, or endocardium) to produce disease.
Over the past two decades, there has been a significant research effort to identify and characterize key factors produced by K. kingae that promote steps in the pathogenic process. In this review, we discuss the current state of knowledge of K. kingae virulence factors and their potential roles in both colonization and development of invasive disease. By gaining a more thorough understanding of the K. kingae factors involved in colonizing the host, transitioning from commensal to pathogen, and invading typically sterile body sites, there is potential to identify novel strategies to prevent or treat invasive K. kingae disease.
Type IV pili
Initial morphological analysis of K. kingae with transmission electron microscopy revealed the presence of long, filamentous surface fibers (5). These fibers have been identified as type IV pili (T4P) and are essential for a variety of K. kingae virulence-associated phenotypes, including a form of surface motility called twitching motility, natural competence, and adherence to human cells, including respiratory epithelial and synovial cells (6, 7). Early observations of K. kingae colony morphology identified two distinct colony types that correlate with T4P expression (5). The spreading/corroding colony type is associated with high levels of surface piliation, and the nonspreading/noncorroding colony type correlates with low levels of surface piliation (5, 6). Subsequent work established that strains lacking pili display a dome-shaped colony type (8). Among clinical isolates, the level of piliation varies and can be permanently changed following repeated sub-culture (8, 9). While the majority of oropharyngeal and non-endocarditis blood isolates are piliated, only a small proportion of joint fluid, bone, and endocarditis isolates produce surface pili (8).
K. kingae T4P biogenesis depends on production of the major pilin subunit PilA1, which is a ∼15-kDa protein that is exported to the inner membrane and polymerizes to form the T4P filament (10). The PilA1 amino acid sequence varies greatly among clinical isolates, suggesting that this protein experiences selective pressure in the human host (8). Expression of the pilA1 gene is regulated by the PilS/PilR two-component system and the alternative σ factor σ54 (10). In prototype strain 269–492, deletion of the pilR gene encoding the PilR response regulator and the rpoN gene encoding σ54 results in abrogation of PilA1 production and the absence of surface pili (10). In contrast, deletion of the pilS gene encoding the PilS sensor kinase results in only a partial decrease in PilA1 production and low levels of surface pili, suggesting that the PilR response regulator may remain active in the absence of the PilS sensor (10).
T4P biogenesis is controlled by the assembly ATPase PilF, which is a cytoplasmic protein that assembles PilA1 subunits into the pilus fiber (6). Deletion of the pilF gene abrogates surface pili but does not affect production of PilA1 (6, 7). T4P-mediated activities such as twitching motility and natural competence rely on the ability of T4P filaments to retract towards the cell surface. T4P retraction is controlled by the retraction ATPases PilT and PilU, which are cytoplasmic proteins that remove PilA1 subunits from the base of the T4P fiber (11). Disruption of the pilTU operon in strain KK03 results in increased levels of surface pili and decreased levels of adherence to epithelial cells, suggesting that pilus retraction is necessary for full-level adherence to human epithelial cells (11). Consistent with the hypothesis that pilus retraction is required for natural competence and twitching motility, disruption of the pilTU operon also results in abrogation of both natural competence and twitching motility (7, 11). The piliation phenotypes of specific K. kingae mutants are diagrammed in Figure 1.
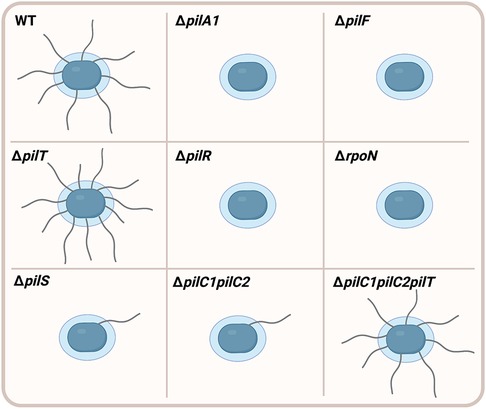
Figure 1. Piliation phenotypes in K. kingae mutants. Wild type (WT) K. kingae bacteria (blue) produce high levels of filamentous surface fibers called type IV pili. Deletion of the pilA1 gene (ΔpilA1) encoding the PilA1 major pilin subunit results in abrogation of surface pili. The pilus fiber is assembled by an assembly ATPase PilF, and deletion of the pilF gene (ΔpilF) prevents surface piliation. T4P retraction is controlled by the PilT retraction ATPase, and deletion of the pilT gene (ΔpilT) results in higher levels of surface piliation compared to WT. Expression of the pilA1 gene is controlled by the PilR/S two-component system and the σ factor σ54. Deletion of pilR (ΔpilR) and the gene encoding σ54 (ΔrpoN) abrogates surface pili. However, deletion of pilS (ΔpilS) results in very low levels of surface piliation compared to WT. Elimination of both the PilC1 and PilC2 pilus-associated adhesins (ΔpilC1pilC2) significantly decreases levels of surface pili compared to WT. However, surface piliation is restored to WT levels following additional deletion of the pilT gene (ΔpilC1pilC2pilT), suggesting that PilC1 and PilC2 promote surface piliation through counteracting retraction of the filament.
Two large pilus-associated proteins called PilC1 and PilC2 are essential for pilus biogenesis, twitching motility, natural competence, and adherence to human cells and extracellular matrix (ECM) proteins (6, 7, 12). These proteins belong to a conserved family of T4P-associated proteins, which includes homologs in Pseudomonas aeruginosa (PilY1), Myxococcus xanthus (PilY1), and the pathogenic Neisseria species (PilC1 and PilC2). In contrast to the near identity between the PilC1 and PilC2 proteins in Neisseria, the PilC1 and PilC2 proteins in K. kingae share limited sequence similarity, with only 7% identity and 16% similarity overall (6). Strains that produce only PilC1 or only PilC2 show no change in surface piliation or adherence to epithelial cells compared to the wild-type strain that produces both proteins (12). However, a strain lacking both PilC1 and PilC2 (ΔpilC1pilC2) has a severe defect in surface piliation (Figure 1), suggesting that PilC1 and PilC2 have important functions in pilus biogenesis (6, 7, 12). Elimination of the PilT retraction ATPase in the ΔpilC1pilC2 strain (ΔpilC1pilC2pilT) results in restoration of surface piliation, suggesting that PilC1 and PilC2 promote high levels of surface piliation through counteracting retraction of the pilus filament (7). Although the ΔpilC1pilC2pilT strain is piliated, it does not adhere to human epithelial cells, raising the hypothesis that PilC1 and PilC2 have adhesive activity (7). In support of this hypothesis, purified PilC1 and PilC2 bind to human epithelial monolayers in a dose-dependent manner and exhibit saturable binding, demonstrating that these proteins are T4P-associated adhesins (7).
Experiments with mutants producing PilC1 and PilC2 truncations and with purified PilC1 and PilC2 fragments established that the binding activity of PilC1 and PilC2 is localized to the N-terminal region of these proteins (7). Deletion of the N-terminal domain of PilC1 abrogates twitching motility and natural competence (7). In contrast, deletion of the N-terminal domain of PilC2 eliminates twitching motility but has no effect on natural competence (7). Production of the C-terminal region of either PilC1 or PilC2 is sufficient to promote surface piliation but not adherence, suggesting that the C termini of PilC1 and PilC2 are sufficient to promote pilus biogenesis (7). Similar to other members of the PilC family, the C termini region of the K. kingae PilC1 and PilC2 proteins contains a predicted β-propeller fold and a calcium-binding (Ca-binding) site (12). In contrast to studies of the Ca-binding site in P. aeruginosa PilY1, mutation of the Ca-binding sites in K. kingae PilC1 and PilC2 has no effect on surface piliation (12). Interestingly, the 9-amino acid Ca-binding site in PilC1 is required for twitching motility and adherence to human epithelial cells, while the 12-amino acid Ca-binding site in PilC2 only has a minor impact on twitching motility and no effect on adherence (12). This work has identified PilC1 and PilC2 as multi-functional T4P-associated adhesins. Further studies are needed to characterize the specific molecular mechanisms that PilC1 and PilC2 utilize to promote T4P-mediated virulence phenotypes.
Kingella NhhA homolog (Knh)
Analysis of a diverse collection of K. kingae clinical isolates revealed that a significant number of pharyngeal isolates are nonpiliated (8). As T4P are important for adherence to epithelial cells, Porsch et al. sought to uncover additional factors that may influence K. kingae adherence to epithelial cells (11). Examination of a K. kingae draft genome sequence for homologs of adhesins in other Neisseriaceae family members uncovered an open reading frame encoding a protein with C-terminal sequence similarity to the Neisseria meningitidis NhhA trimeric autotransporter. This K. kingae protein was named Knh for Kingella NhhA homolog and was confirmed to be a trimeric autotransporter through demonstration of the trimeric β-barrel in the outer membrane when produced in Escherichia coli (11). Adherence assays with human epithelial cells demonstrated that Knh is required for full-level adherence, as the adherence level mediated by T4P in the absence of Knh is reduced by approximately half (11). Dynamic adherence assays with shear stress revealed that Knh-mediated adherence is stronger than T4P-mediated adherence (13).
Further work established that the adhesive potential of Knh is dependent on post-translational modification via glycosylation (14). K. kingae encodes a homolog of the Haemophilus influenzae HMW1C glycosyltransferase that N-glycosylates Knh with glucose residues. Without glycosylation, the amount of Knh on the bacterial surface is reduced and adherence to human epithelial cells is abrogated (14).
Through analysis of the amino acid sequence of Knh, it was found that the passenger domain contains two putative adhesive domains (11). The N-terminal region is predicted to encode YadA-like head domains, based on the YadA trimeric autotransporter adhesin of Yersinia enterocolitica (15, 16). The head domains of YadA are responsible for the adhesive activity of this trimeric autotransporter, raising the possibility that these predicted domains of Knh may contain adhesive activity as well (11, 15, 16). Additionally, Knh contains an ISneck2 domain with similar architecture to the ISneck1 domain found in the adhesive regions of the H. influenzae Hia and Hsf proteins (11, 17). Further studies are necessary to experimentally confirm the adhesive domains of Knh.
RTX family pore-forming toxin
In order to invade the bloodstream, K. kingae must breach the epithelial barrier at the site of colonization. Translocation across the epithelial barrier and entry into the bloodstream likely involves multiple factors, including a repeats-in-toxin (RTX) family protein called RtxA (18). RTX family members have been identified in diverse bacterial pathogens, including Bordetella pertussis, Escherichia coli, Vibrio cholerae, Vibrio vulnificus, Moraxella spp., Pasteurella spp., Actinobacillus spp., and Aggregatibacter spp., among others (19–24). Members of this protein family are defined by the presence of nonapeptide repeats with the consensus sequence X-(L/I/F)-X-G-G-X-G-(N/D)-D in the RTX domain of the protein (25). This repeat sequence binds extracellular calcium, facilitating both folding of RTX family proteins and secretion from the organism (24, 25).
The RtxA toxin was first identified and characterized by Kehl-Fie et al. (18) and is encoded by the rtxA gene, which is conserved across all known strains of K. kingae. The rtxA gene is absent in other Kingella species except K. negevensis (26–30). RtxA is cytotoxic against a broad range of eukaryotic cells in vitro, including red blood cells, epithelial cells (HeLa, A549, FaDu, HLac-78), endothelial cells, synovial cells (SW-982, Hig-82), osteoblasts (U-2), and monocytes (THP-1, RAW264.7) (18, 24, 31–33). Mutants that lack RtxA are non-hemolytic and non-cytotoxic in vitro and are avirulent in an infant rat model of invasive disease, underscoring the role of this toxin as a major contributor to K. kingae virulence (10, 33).
Cell death is mediated by insertion of RtxA into the host cell membrane, resulting in pore formation (23, 24, 34–36). Unlike related toxins, such as the B. pertussis and E. coli RTX toxins, RtxA does not target β2-integrins in the host cell membrane. Rather, this toxin associates with membrane cholesterol, likely facilitated by a set of cholesterol recognition/interaction amino acid (CRAC) motifs in the N-terminus of the protein (24). Once in the membrane, RtxA oligomerizes, resulting in formation of a cation-selective pore that measures 1.9 nm in diameter, as demonstrated using purified RtxA and planar lipid bilayers (34).
There are five toxin-associated genes that are required for production, activation, and secretion of the K. kingae RtxA toxin, designated rtxB, rtxD, rtxC, rtxA, and tolC. These genes are present in two loci in different regions of the chromosome. In most strains with publicly available genomes, one locus contains rtxB, rtxD, and rtxC and a second locus contains rtxC, rtxA, and tolC, resulting in two copies of rtxC (23, 28). In K. kingae strain KWG-1, one locus contains all five genes, and a second locus contains rtxC, rtxA, and tolC, resulting in two copies of both rtxC and rtxA. The prevalence of the KWG-1 configuration in the K. kingae population and the phenotypic consequences of the duplication of rtxA are unknown. Both rtx loci are flanked by genes predicted to be associated with DNA transposition and have a decreased G + C content relative to the rest of the K. kingae genome (18). As a consequence, some investigators have hypothesized that the toxin may have been acquired as a part of a horizontal gene transfer event (18, 23, 28).
Little is known about the regulatory mechanisms that govern transcription of rtxA, but rtxA expression is influenced at least in part by phase variation (37). Phase variation is mediated by the phase variable type III DNA methyltransferase, ModK. Expansion or contraction of a tandem tetranucleotide repeat sequence in modK results in a frame shift that introduces a premature stop codon, generating a truncated and inactive ModK protein. Inactivation of ModK results in a subpopulation of organisms with less surface-associated RtxA (37). Phase variation of ModK also influences levels of the surface-associated heat shock proteins DnaK and GroEL.
RtxA is synthesized as a pro-toxin, which is non-cytotoxic and non-hemolytic. The pro-toxin is activated by the RtxC protein, which adds two acyl-chain moieties that play a key role in facilitating RtxA insertion into the host cell membrane. The activated RtxA toxin is then secreted by a type I secretion system (TISS) that is comprised of RtxB, RtxD, and the TolC outer membrane protein. The proposed secretion pathway for RtxA is shown in Figure 2 (18, 24, 25, 36).
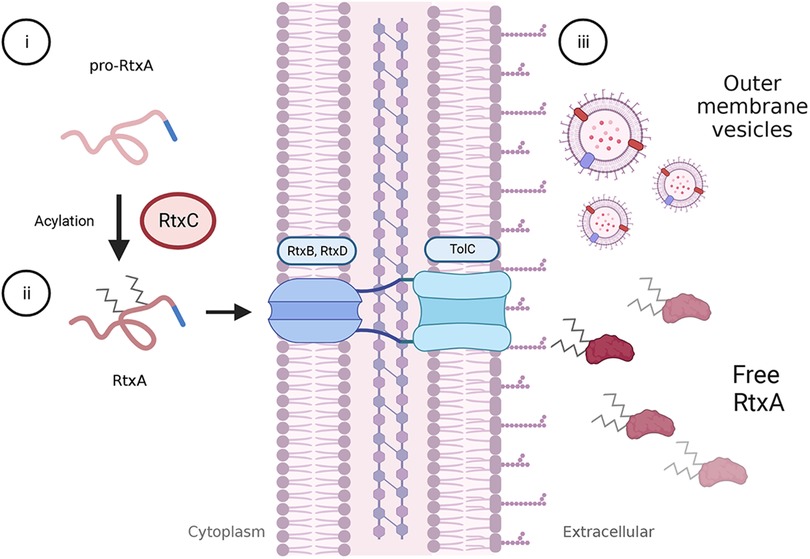
Figure 2. Activation and secretion of RtxA. The rtxA gene encodes a pro-toxin in K. kingae. Following translation, pro-RtxA remains unfolded and associates with RtxC in the cytosol, possibly near the cell membrane. (i) RtxC catalyzes the addition of an acyl-moiety onto each of two, conserved lysine residues. (ii) As with other RTX toxins, RtxA is thought to remain unfolded until it is exported from the bacterial cell via a type I secretion system comprised of RtxD and RtxB in the inner membrane and TolC in the outer membrane. Export is facilitated by a C-terminal secretion signal, and folding is likely mediated through the interaction of extracellular calcium with the RtxA calcium-binding domain. (iii) Outside of the cell, RtxA is found in a free state in spent media as well as in association with outer membrane vesicles (OMVs). It is unclear if the OMV-associated toxin is within the OMV or associated with the membrane. Both free RtxA and OMV-associated RtxA can cause host cell lysis.
Beyond secretion via the TISS, RtxA is released from the organism in outer membrane vesicles (OMV). OMV production is highly variable among clinical isolates (31, 32). Along with RtxA, K. kingae OMVs contain a host of other proteins in the outer membrane. OMVs are rapidly phagocytosed by eukaryotic cells and are cytotoxic against red blood cells and monocytes but not epithelial cells (32). Exposure to OMVs results in increased production of granulocyte-macrophage colony-stimulating factor (GM-CSF), interleukin-6 (IL-6), IL-1β, and tumor necrosis factor-α (TNF-α) from SW982 (synovial) cells and hFOB 1.19 (osteoblast) cells in vitro (31, 32). Currently, it is unclear if stimulation of these inflammatory molecules is due to RtxA or to other factors found in OMVs, such as lipopolysaccharide (LPS).
Polysaccharide capsule
Polysaccharide capsules are produced by many pathogenic bacteria and promote bacterial survival in diverse environments, providing protection against a variety of extracellular insults. Capsules are surface-exposed, lipid-anchored, highly-hydrated polymers that promote bacterial survival within a host by assisting in the evasion of host immune mechanisms, including phagocytosis and complement-mediated killing, making them attractive targets as vaccine antigens (38, 39).
The hypothesis that K. kingae is encapsulated began with qualitative examination of the mucoid colony phenotype on chocolate agar, a phenotype that is common in other bacteria that produce a polysaccharide capsule (11). A search through the draft genome sequence of K. kingae for homologs of genes responsible for encapsulation in other organisms led to discovery of a locus with similarity to the ABC-type capsule export operon in N. meningitidis, containing the ctrABCD genes. Thin-section transmission electron microscopy after staining with ferritin and visualization of Alcian blue-stained acid extracts confirmed that K. kingae produces a surface-associated polysaccharide capsule that is absent when ctrA is disrupted (11).
Further homology-based approaches and a transposon mutagenesis screen identified additional capsule synthesis and assembly genes in K. kingae. These include genes homologous to the N. meningitidis lipB and lipA genes and the E. coli kpsS and kpsC genes involved in synthesizing the poly β-Kdo linker between the capsular polysaccharide and the lipid anchor (11, 40–42) Individual disruption of the K. kingae lipB or lipA homolog results in loss of encapsulation and accumulation of capsular polysaccharide in the cytoplasm, as evidenced by the presence of lacunae in thin section electron micrographs (42). These results are consistent with studies in E. coli by Willis and Whitfield, demonstrating that KpsS adds a single β-Kdo residue onto a lipid moiety (phosphatidylglycerol) on the cytoplasmic side of the inner membrane and KpsC then incorporates additional β-Kdo residues onto the first residue (41). The transposon mutagenesis screen also identified a predicted glycosyltransferase gene necessary for capsule production in strain KK01, a stable nonspreading/noncorroding derivative of septic arthritis isolate 269–492, and designated csaA. Targeted deletion of csaA results in a complete absence of both intracellular and extracellular capsular polysaccharide, suggesting that this gene is involved in capsule synthesis (42). In contrast to other encapsulated organisms, the K. kingae ctrABCD, lipA, lipB, and csaA genes are not present at a single locus in the chromosome and instead are scattered throughout the genome, raising questions about the regulatory mechanism used to control capsule biosynthesis and export (42, 43).
To determine the composition and structure of the K. kingae polysaccharide capsule, glycosyl composition analysis and NMR spectroscopy were performed on purified capsular polysaccharide from strain KK01 (44). These studies established that the polysaccharide is composed of N-acetyl galactosamine (GalNAc) and 3-deoxy-D-manno-oct-2-ulosonic acid (Kdo), with the structure [3)-β-GalpNAc-(1→5)-β-Kdop-(2→] (44). Point mutations in the csaA capsule synthesis gene revealed that CsaA is a bifunctional enzyme (42). The N-terminal region contains a putative GalNAc transferase domain, and the middle and C-terminal region contains a putative β-Kdo transferase domain. This glycosyltransferase creates a polymer of GalNAc and Kdo and is able to create both the β-GalpNAc-(1→5)-β-Kdop linkage and the β-Kdop-(2→3)-β-GalpNAc linkage, linking the polysaccharide to the terminal β-Kdo residue of the poly β-Kdo linker and synthesizing the capsular polysaccharide (42). The interplay of each of the capsule biosynthesis gene products in strain KK01 is summarized in Figure 3.
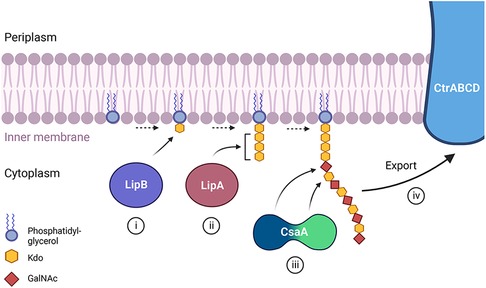
Figure 3. A proposed model depicting the role of each of the known capsule biosynthesis gene products in K. kingae strain KK01 producing capsule type a. (i) The lipB gene encodes a β-Kdo transferase that adds a single β-Kdo residue onto a lipid moiety (phosphatidylglycerol) at the cytoplasmic side of the bacterial membrane. (ii) The lipA gene encodes a β-Kdo transferase that adds multiple β-Kdo residues onto the initial β-Kdo residue and creates a short β-Kdo chain. (iii) The csaA gene encodes a bifunctional enzyme with a GalNAc transferase at the N-terminal region (blue portion) and a Kdo transferase in the middle and C-terminal region (green portion) of the protein. CsaA builds the capsular polysaccharide chain composed of GalNAc and Kdo repeating units onto the chain of β-Kdo residues, with the N-terminal region (blue) adding GalNAc and the middle and C-terminal region (green) adding Kdo. (iv) The capsular polysaccharide is then shuttled to the outer membrane through the ABC-type capsule transporter encoded by ctrABCD.
The structure of the strain KK01 capsular polysaccharide is similar to a previously reported surface polysaccharide with the structure [6)-α-D-GlcpNAc-(1→5)-β-Kdop-(2→] in K. kingae strain PYKK181 (45). With the hypothesis that the strain PYKK181 surface polysaccharide represents a distinct capsule type, Starr et al. used a PCR approach to screen a collection of 417 Israeli invasive and carrier isolates for the presence of additional capsule synthesis loci (43). Four distinct capsule synthesis loci were discovered, producing four different capsular polysaccharide types. The csa locus produces the GalNAc-Kdo capsule identified in strain KK01, designated capsule type a (42, 43). The csb locus produces the GlcNAc-Kdo capsule present in strain PYKK181, designated capsule type b (43, 45). The csc locus produces a capsule composed of ribose and Kdo with the structure [3)-β-D-Ribf-(1→2)-β-D-Ribf-(1→2)-β-D-Ribf-(1→4)-β-Kdop-(2→], designated capsule type c (43). The csd locus produces a capsule composed of galactose and GlcNAc with the structure [P-(O→3)[β-D-Galp-(1→4)]-β-D-GlcpNAc-(1→3)-α-D-GlcpNAc-1-], designated capsule type d (43). The type a capsule is identical to the capsule of Moraxella nonliquefacians strain 3828/60, a commensal in the human upper respiratory tract (46), and the type b capsule is identical to the serotype 5a capsule of Actinobacillus pleuropneumoniae, a respiratory porcine pathogen (43, 45, 47). The capsule type c and d structures are novel (43).
The analysis of Israeli invasive and carrier isolates revealed that the type a and type b capsules are enriched in invasive isolates, accounting for >95% of these isolates. In contrast, the type c and type d capsules are disproportionally present in carrier isolates (43). Analysis of an international collection of isolates from the United States, Canada, Iceland, France, Switzerland, Spain, and New Zealand also revealed dominance of the type a and type b capsule types among invasive isolates (48–50). Whether the type a and type b capsules directly confer greater pathogenicity or are instead associated with other virulence determinants is an active area of investigation.
Detailed studies have implicated the K. kingae capsule as a key determinant of K. kingae pathogenicity. As described in the sections above, colonization of the oropharynx is presumed to be initiated by adherence of the bacterium to oropharyngeal epithelial cells. Studies have shown that the capsule masks the Knh adhesin and interferes with Knh-mediated high-affinity adherence to human epithelial cells when T4P retraction is lacking (11, 13). Examination of K. kingae interactions with human epithelial cells by transmission electron microscopy using ferritin staining to highlight the capsule suggests that T4P retraction pulls the bacterium into close contact with the host cell, resulting in physical displacement of the bacterial capsule through the retractile force of the fibers and the subsequent exposure of Knh to the surface of host cells (11, 13). This capsule displacement is necessary for Knh-mediated adherence, as the capsule is deeper than Knh is long (13).
In order to survive in the bloodstream and disseminate to sites of invasive infection, K. kingae must evade potent immune mechanisms in the intravascular space. The K. kingae capsule resists human serum-mediated killing by blocking deposition of IgG and IgM antibodies and the C3b and C4b complement fragments on the bacterial surface and inhibiting activation of the classical complement pathway (51). To delineate whether capsule type influences the ability of K. kingae to resist serum-mediated killing, isogenic mutants that produce each of the four capsule types were tested in serum-bactericidal assays. These studies revealed that the presence of any of the four capsule types is sufficient to promote serum survival under in vitro conditions (51). Consistent with the in vitro results, the capsule is necessary for full virulence in the juvenile rat model of invasive disease (42, 51). Depletion of complement components C3 and C5 in the juvenile rats with cobra venom factor prior to infection restores full virulence of unencapsulated mutants, demonstrating the importance of resisting complement for virulence in the rat infection model (51).
Beyond mediating resistance to complement-mediated killing, the polysaccharide capsule plays a key role in resisting neutrophil killing. Using freshly purified human neutrophils, Muñoz et al. demonstrated that the capsule interferes with bacterial binding to neutrophils and prevents neutrophil activation and production and release of neutrophil reactive oxygen species (52).
Galactan exopolysaccharide
In addition to the polysaccharide capsule, K. kingae was found to have a distinct exopolysaccharide called galactan in cell-free extracts. Work by Bendaoud et al. demonstrated that these cell-free extracts had surfactant-like activity and inhibited biofilm formation by K. kingae and several phylogenetically diverse bacterial and fungal pathogens when coated onto an abiotic surface (45). Preliminary characterization of the extracts suggested that they contained a high abundance of DNA, prompting the name poly-DNA-containing anti-adhesive material extract or PAM extract. Selective removal of PAM extract components from K. kingae strain PYKK181 suggested that the bioactive component was carbohydrate-based, and NMR analyses revealed a galactofuranose homopolymer, or galactan, with the structure →3)-β-D-Galf-(1→6)-β-D-Galf-(1→ (45). This study also identified a five gene locus designated pamABCDE, encoding a predicted UDP-galactopyranose mutase (enzyme required to catalyze the conversion of UDP-galactopyranose to UDP-galactofuranose) and four putative glycosyltransferases (Figure 4A). Expression of the pam genes in E. coli established that production of the galactan exopolysaccharide was dependent on only the pamABC genes (45).
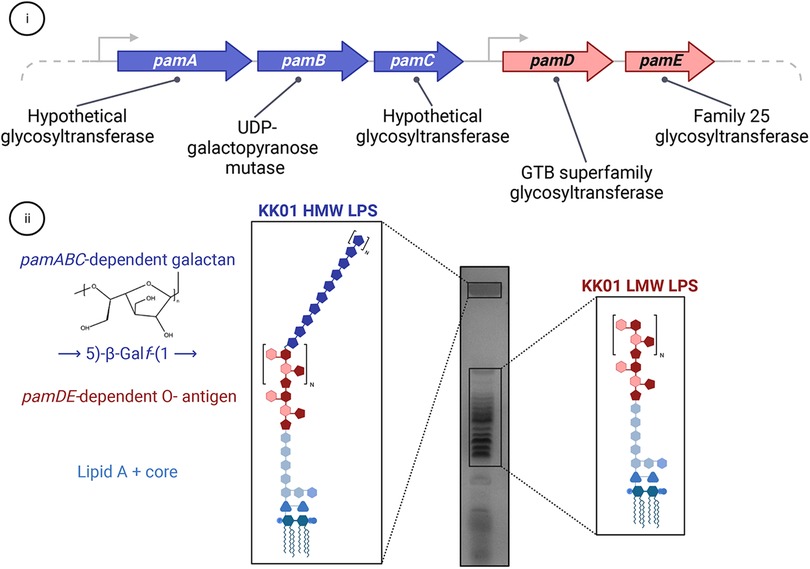
Figure 4. The relationship between the galactan exopolysaccharide and LPS in K. kingae. (i) The pamABCDE locus is essential for presentation of the galactan exopolysaccharide on the bacterial surface and contains genes encoding four predicted glycosyltransferases (pamA, pamC, pamD, and pamE) and one predicted UDP-galactopyranose mutase (pamB). (ii) The pamABC genes are necessary for synthesis of the galactan homopolymer with the structure of →5)-β-Galf-(1→, which is linked to the atypical O-antigen. The pamDE genes are required for synthesis of the atypical O-antigen. In wild type K. kingae strain KK01, the LPS migrates as two distinct modal clusters: a high molecular weight (HMW) band that contains LPS decorated with both the atypical O-antigen and galactan and a low molecular weight ladder cluster that contains LPS decorated with only the atypical O-antigen.
In addition to the →3)-β-D-Galf-(1→6)-β-D-Galf-(1→ galactan isolated from strain PYKK181, a →5)-β-Galf-(1→ galactan has been isolated from K. kingae strain KK01. These are currently the only two documented galactan structures in K. kingae, and more studies are needed to appreciate the diversity of these galactan structures across the K. kingae population structure.
Functional studies performed with strain KK01 have established that the galactan exopolysaccharide has a critical role in virulence. In particular, the galactan mediates resistance to complement-mediated serum killing, independent of the polysaccharide capsule (51). The galactan also mediates resistance to neutrophil phagocytosis and killing, again independent of the polysaccharide capsule (52). In the infant rat model of systemic K. kingae disease, mutants lacking the galactan are significantly attenuated in virulence, and mutants lacking the capsule and galactan are avirulent (51). These functions suggest that galactan must be tethered to the bacterial surface by some mechanism. Recent work has revealed that surface tethering of the galactan is dependent on the K. kingae lipopolysaccharide (LPS) atypical O-antigen (53).
LPS is a highly abundant glycolipid that is associated with the gram-negative bacterial outer membrane and promotes multiple functions related to barrier integrity and interactions with the environment (54). LPS is a tripartite molecule composed of lipid A, which is anchored in the outer membrane, a core oligosaccharide composed of non-repeating sugar residues, and in some organisms an O-antigen composed of repeating sugar units that vary greatly across bacterial species (54, 55). Initial characterization of the K. kingae LPS revealed that it varies greatly from the LPS of related species. Notably, resolution of purified LPS from wild type K. kingae revealed two modal clusters, including a low molecular weight (LMW) cluster that displays a ladder migration pattern and a high molecular weight (HMW) cluster that contains an additional polysaccharide component (Figure 4B) (53). The uniformity of the ladder migration pattern observed in the LMW LPS species suggested the presence of a repeating sugar unit, implying that K. kingae produces an atypical O-antigen. Glycosyl composition analysis and 1-D NMR revealed that the additional polysaccharide component of the HMW LPS is the galactan exopolysaccharide, implying a novel structural association between the K. kingae LPS and galactan. Homology-based approaches indicated that the pamD and pamE gene products contain folds that are shared with the GTB superfamily of glycosyltransferases and the Family 25 glycosyltransferases, respectively. Some proteins in other organisms that contain these folds are involved in LPS biosynthesis. The homology analysis of the pamD and pamE gene products and the location of the pamD and pamE genes immediately adjacent to the pamABC genes suggested that these gene products might function in K. kingae LPS biosynthesis and influence the structural association between the LPS and galactan (Figure 4A) (53). Deletion of pamD and pamE resulted in production of a truncated LPS lacking both the LMW and HMW LPS species, highlighting the essential role of the pamD and pamE genes in biosynthesis of the K. kingae atypical O-antigen (53). Furthermore, the loss of the HMW LPS species in K. kingae strains lacking the pamD and pamE genes established that surface anchoring of the galactan requires the atypical O-antigen (53).
Recent studies have begun to elucidate how the structural linkage between the LPS O-antigen and the galactan exopolysaccharide influences the function of both surface polysaccharides. In particular, initial characterization of the galactan exopolysaccharide suggested that it was able to promote resistance to cationic antimicrobial peptides (CAMPs), including polymyxin B and HNP-1 (52). However, more recent work examining mutants lacking the pamABC genes and deficient in galactan/HMW LPS production retained resistance to polymyxins, indicating that the galactan on its own is dispensable for CAMP resistance (53). Conversely, mutants lacking the pamDE genes were significantly more susceptible to polymyxins, suggesting that the LMW LPS O-antigen is a primary driver of CAMP resistance in K. kingae (53).
Identification of the novel structural relationship between the K. kingae LPS and galactan molecules has provided a mechanism for surface anchoring of the galactan exopolysaccharide and for the unique functionality of this exopolysaccharide in interactions with host immune components. More studies are needed to understand the individual roles of the pamABCDE genes in galactan and LPS biosynthesis, specifically ligation of the galactan to the growing LPS molecule and the spatiotemporal dynamics of LPS and galactan assembly and surface translocation.
Genomics
At the time of this publication, genomes for 57 strains of K. kingae are publicly available. Genomes range in size from 1.95 megabases (ATCC23330) to 2.14 megabases (KWG-1), with an average of ∼2,000 predicted open reading frames per genome. K. kingae is closely related to K. negevensis, with a number of shared virulence factors, including type IV pili, Knh, RtxA, a polysaccharide capsule, and a galactan exopolysaccharide (28–30). Amit et al. performed pulsed field gel electrophoresis (PFGE) on a collection of 181 invasive K. kingae isolates collected between 1991 and 2012 in Israel and identified 32 different clones, with five clones (clones B, H, K, N, and P) accounting for more than 70% of the invasive isolates (56). Clones K, N, and P were significantly associated with bacteremia, skeletal infections, and endocarditis, respectively, potentially reflecting different tissue tropisms (56). This study further categorized the population by applying a sequence typing scheme using the genes abcZ, adk, aroE, cpn60, gdh, and recA (56). Subsequent studies have identified 73 global sequence types (STs) comprising 12 distinct complexes (STcs). Five of these STcs account for 72% of global isolates, though their distribution is skewed based on geographic location (56–58).
As stated above, all known isolates of K. kingae contain the rtxA gene, which has been used as a molecular target for the clinical diagnosis of invasive K. kingae disease (28, 58, 59). In addition, all isolates contain the genetic machinery for polysaccharide capsule export, galactan exopolysaccharide biosynthesis, and type IV pili biogenesis. Production of functional T4P is critical for K. kingae natural transformation, which is presumed to be a major contributor to K. kingae genomic diversity (7). Natural transformation by exogenous DNA is influenced in K. kingae by the presence of a DNA uptake sequence (DUS), which is shared with Kingella denitrificans, but exists in different dialects in many other Neisseriaceae family members (60). A novel, rapid, PCR-based typing strategy based on the K. kingae DUS has been shown to correctly classify K. kingae isolates into MLST-derived STcs (57).
In addition to DNA acquisition via natural transformation, at least one conjugative plasmid was originally identified in strain KWG-1 (61). This plasmid has been named ISkk1, is thought to be integrated into the genome, and encodes a type IV secretion system. Additionally, ISkk1 contains several antibiotic resistance cassettes, including cassettes that encode resistance to β-lactams, sulfonamides, streptomycin, and tetracycline (61). The presence of a β-lactamase has been noted in other isolates from the United States, Israel, and Iceland (62). Based on a study of Israeli invasive and carrier isolates, ∼10% of carrier isolates (belonging to the A, F, and Ψ PFGE groups) and ∼1% of invasive isolates, produce a β-lactamase (62). Beyond ISkk1, little is known about the pangenome of K. kingae (62–65).
Conclusions
The advances in our understanding of K. kingae virulence factors detailed in this review have revealed multiple bacterial surface factors implicated in the pathogenicity of this fastidious bacterium. Several key findings have identified targets for therapeutic intervention. For example, the fact that greater than 95% of invasive disease isolates express either the type a or type b capsule raises the possibility that a glycoconjugate vaccine incorporating these two polysaccharides may be an effective approach to prevent invasive disease given the success of capsule-based conjugate vaccines in preventing morbidity and mortality due to other bacteria such as H. influenzae type b, N. meningitidis, and Streptococcus pneumoniae. In addition, it is intriguing to speculate that targeting type IV pili or Knh may be an effective strategy to prevent K. kingae colonization of the oropharynx. As we continue to gain a more complete picture of the pathogenesis of K. kingae disease, additional strategies for prevention and treatment of disease are likely to emerge.
Author contributions
EAP, KAH, DPM, NRM, and TAY wrote the manuscript. EAP, KAH, DPM, NRM, TAY, and JWS revised and edited the manuscript. All authors contributed to the article and approved the submitted version.
Acknowledgments
The figures in this manuscript were created using BioRender (https://biorender.com).
Conflict of interest
The authors declare that the research was conducted in the absence of any commercial or financial relationships that could be construed as a potential conflict of interest.
Publisher's note
All claims expressed in this article are solely those of the authors and do not necessarily represent those of their affiliated organizations, or those of the publisher, the editors and the reviewers. Any product that may be evaluated in this article, or claim that may be made by its manufacturer, is not guaranteed or endorsed by the publisher.
References
1. Yagupsky P, Dagan R, Prajgrod F, Merires M. Respiratory carriage of Kingella kingae among healthy children. Pediatr Infect Dis J. (1995) 14:673–8. doi: 10.1097/00006454-199508000-00005
2. Yagupsky P. Kingella kingae: from medical rarity to an emerging paediatric pathogen. Lancet Infect Dis. (2004) 4:358–67. doi: 10.1016/S1473-3099(04)01046-1
3. Yagupsky P. Kingella kingae: carriage, transmission, and disease. Clin Microbiol Rev. (2015) 28:54–79. doi: 10.1128/CMR.00028-14
4. Yagupsky P, Porsch E, St. Geme J 3rd. Kingella kingae: an emerging pathogen in young children. Pediatrics. (2011) 127:557–65. doi: 10.1542/peds.2010-1867
5. Froholm LO, Bovre K. Fimbriation associated with the spreading-corroding colony type in Moraxella kingii. Acta Pathol Microbiol Scand B Microbiol Immunol. (1972) 80:641–8. doi: 10.1111/j.1699-0463.1972.tb00190.x
6. Kehl-Fie TE, Miller SE, St. Geme JW III. Kingella kingae expresses type IV pili that mediate adherence to respiratory epithelial and synovial cells. J Bacteriol. (2008) 190:7157–63. doi: 10.1128/JB.00884-08
7. Sacharok AL, Porsch EA, Yount TA, Keenan O, St. Geme JW III. Kingella kingae PilC1 and PilC2 are adhesive multifunctional proteins that promote bacterial adherence, twitching motility, DNA transformation, and pilus biogenesis. PLoS Pathog. (2022) 18:e1010440. doi: 10.1371/journal.ppat.1010440
8. Kehl-Fie TE, Porsch EA, Yagupsky P, Grass EA, Obert C, Benjamin DK, et al. Examination of type IV pilus expression and pilus-associated phenotypes in Kingella kingae clinical isolates. Infect Immun. (2010) 78:1692–9. doi: 10.1128/IAI.00908-09
9. Ødum L, Frederikson W. Identification and characterization of Kingella kingae. Acta Pathol Microbiol Scand Sect B Microbiol. (1981) 89 B:311–5. doi: 10.1111/j.1699-0463.1981.tb00194_89B.x
10. Kehl-Fie TE, Porsch EA, Miller SE, St. Geme JW III. Expression of Kingella kingae type IV pili is regulated by σ54, PilS, and PilR. J Bacteriol. (2009) 191:4976–86. doi: 10.1128/JB.00123-09
11. Porsch EA, Kehl-Fie TE, St. Geme JW III. Modulation of Kingella kingae adherence to human epithelial cells by type IV Pili, capsule, and a novel trimeric autotransporter. MBio. (2012) 3:1–9. doi: 10.1128/mBio.00372-12
12. Porsch EA, Johnson MDL, Broadnax AD, Garrett CK, Redinbo MR, St. Geme JW III. Calcium binding properties of the Kingella kingae PilC1 and PilC2 proteins have differential effects on type IV pilus-mediated adherence and twitching motility. J Bacteriol. (2013) 195:886–95. doi: 10.1128/JB.02186-12
13. Kern BK, Porsch EA, St. Geme JW III. Defining the mechanical determinants of Kingella kingae adherence to host cells. J Bacteriol. (2017) 199:e00314–17. doi: 10.1128/JB.00314-17
14. Rempe KA, Spruce LA, Porsch EA, Seeholzer SH, Nørskov-Lauritsen N, St. Geme JW III. Unconventional N-linked glycosylation promotes trimeric autotransporter function in Kingella kingae and Aggregatibacter aphrophilus. MBio. (2015) 6:e01206–15. doi: 10.1128/MBIO.01206-15
15. Tamm A, Tarkkanen AM, Korhonen TK, Kuusela P, Toivanen P, Skurnik M. Hydrophobic domains affect the collagen-binding specificity and surface polymerization as well as the virulence potential of the YadA protein of Yersinia enterocolitica. Mol Microbiol. (1993) 10:995–1011. doi: 10.1111/J.1365-2958.1993.TB00971.X
16. El Tahir Y, Kuusela P, Skurnik M. Functional mapping of the Yersinia enterocolitica adhesin YadA. Identification of eight NSVAIG – S motifs in the amino-terminal half of the protein involved in collagen binding. Mol Microbiol. (2000) 37:192–206. doi: 10.1046/J.1365-2958.2000.01992.X
17. Meng G, St. Geme JW III, Waksman G. Repetitive architecture of the Haemophilus influenzae hia trimeric autotransporter. J Mol Biol. (2008) 384:824. doi: 10.1016/J.JMB.2008.09.085
18. Kehl-Fie TE, St. Geme JW III. Identification and characterization of an RTX toxin in the emerging pathogen Kingella kingae. J Bacteriol. (2007) 189:430–6. doi: 10.1128/JB.01319-06
19. Pérez-Reytor D, Jaña V, Pavez L, Navarrete P, García K. Accessory toxins of Vibrio pathogens and their role in epithelial disruption during infection. Front Microbiol. (2018) 9:2248. doi: 10.3389/FMICB.2018.02248
20. Vojtova J, Kamanova J, Sebo P. Bordetella adenylate cyclase toxin: a swift saboteur of host defense. Curr Opin Microbiol. (2006) 9:69–75. doi: 10.1016/J.MIB.2005.12.011
21. Schaller A, Kuhn R, Kuhnert P, Nicolet J, Anderson TJ, Maclnnes JI, et al. Characterization of apxlVA, a new RTX determinant of Actinobacillus pleuropneumoniae. Microbiology. (1999) 145:2105–16. doi: 10.1099/13500872-145-8-2105
22. Frey J, Bosse JT, Chang Y-F, Cullen JM, Fenwick B, Gerlach GF, et al. Actinobacillus pleuropneumoniae RTX-toxins: uniform designation of haemolysins, cytolysins, pleurotoxin and their genes. J Gen Microbiol. (1993) 139:1723–8. doi: 10.1099/00221287-139-8-1723
23. Filipi K, Rahman WU, Osickova A, Osicka R. Kingella kingae RtxA cytotoxin in the context of other RTX toxins. Microorganisms. (2022) 10:518. doi: 10.3390/MICROORGANISMS10030518
24. Osickova A, Balashova N, Masin J, Sulc M, Roderova J, Wald T, et al. Cytotoxic activity of Kingella kingae RtxA toxin depends on post-translational acylation of lysine residues and cholesterol binding. Emerg Microbes Infect. (2018) 7:178. doi: 10.1038/s41426-018-0179-x
25. Linhartová I, Bumba L, Mašn J, Basler M, Osička R, Kamanová J, et al. RTX proteins: a highly diverse family secreted by a common mechanism. FEMS Microbiol Rev. (2010) 34:1076–112. doi: 10.1111/j.1574-6976.2010.00231.x
26. Ceroni D, Cherkaoui A, Ferey S, Kaelin A, Schrenzel J. Kingella kingae osteoarticular infections in young children: clinical features and contribution of a new specific real-time PCR assay to the diagnosis. J Pediatr Orthop. (2010) 30:301–4. doi: 10.1097/BPO.0B013E3181D4732F
27. Cherkaoui A, Ceroni D, Emonet S, Lefevre Y, Schrenzel J. Molecular diagnosis of Kingella kingae osteoarticular infections by specific real-time PCR assay. J Med Microbiol. (2009) 58:65–8. doi: 10.1099/JMM.0.47707-0
28. Opota O, Laurent S, Pillonel T, Léger M, Trachsel S, Prod’hom G, et al. Genomics of the new species Kingella negevensis: diagnostic issues and identification of a locus encoding a RTX toxin. Microbes Infect. (2017) 19:546–52. doi: 10.1016/J.MICINF.2017.08.001
29. Porsch EA, Yagupsky P, St. Geme JW III. Kingella negevensis shares multiple putative virulence factors with Kingella kingae. PLoS One. (2020) 15:e0241511. doi: 10.1371/JOURNAL.PONE.0241511
30. El Houmami N, Bakour S, Bzdrenga J, Rathored J, Seligmann H, Robert C, et al. Isolation and characterization of Kingella negevensis sp. nov., a novel Kingella species detected in a healthy paediatric population. Int J Syst Evol Microbiol. (2017) 67:2370–6. doi: 10.1099/IJSEM.0.001957
31. Pesce Viglietti AI, Sviercz FA, López CAM, Freiberger RN, Quarleri J, Delpino MV. Proinflammatory microenvironment during Kingella kingae infection modulates osteoclastogenesis. Front Immunol. (2021) 12:757827. doi: 10.3389/FIMMU.2021.757827
32. Maldonado R, Wei R, Kachlany SC, Kazi M, Balashova NV. Cytotoxic effects of Kingella kingae outer membrane vesicles on human cells. Microb Pathog. (2011) 51:22–30. doi: 10.1016/J.MICPATH.2011.03.005
33. Chang DW, Nudell YA, Lau J, Zakharian E, Balashova NV. RTX Toxin plays a key role in Kingella kingae virulence in an infant rat model. Infect Immun. (2014) 82:2318–28. doi: 10.1128/IAI.01636-14
34. Bárcena-Uribarri I, Benz R, Winterhalter M, Zakharian E, Balashova N. Pore forming activity of the potent RTX-toxin produced by pediatric pathogen Kingella kingae: characterization and comparison to other RTX-family members. Biochim Biophys Acta. (2015) 1848:1536–44. doi: 10.1016/j.bbamem.2015.03.036
35. Rahman WU, Osickova A, Klimova N, Lora J, Balashova N, Osicka R. Binding of Kingella kingae RtxA toxin depends on cell surface oligosaccharides, but not on β 2 integrins. Int J Mol Sci. (2020) 21:1–14. doi: 10.3390/IJMS21239092
36. Osickova A, Khaliq H, Masin J, Jurnecka D, Sukova A, Fiser R, et al. Acyltransferase-mediated selection of the length of the fatty acyl chain and of the acylation site governs activation of bacterial RTX toxins. J Biol Chem. (2020) 295:9268–80. doi: 10.1074/jbc.RA120.014122
37. Srikhanta YN, Fung KY, Pollock GL, Bennett-Wood V, Howden BP, Hartland EL. Phasevarion-regulated virulence in the emerging pediatric pathogen Kingella kingae. Infect Immun. (2017) 85:e00319–17. doi: 10.1128/IAI.00319-17
38. Hyams C, Camberlein E, Cohen JM, Bax K, Brown JS. The Streptococcus pneumoniae capsule inhibits complement activity and neutrophil phagocytosis by multiple mechanisms. Infect Immun. (2010) 78:704. doi: 10.1128/IAI.00881-09
39. Agarwal S, Vasudhev S, DeOliveira RB, Ram S. Inhibition of the classical pathway of complement by meningococcal capsular polysaccharides. J Immunol. (2014) 193:1855. doi: 10.4049/JIMMUNOL.1303177
40. Tzeng YL, Datta AK, Strole CA, Lobritz MA, Carlson RW, Stephens DS. Translocation and surface expression of lipidated serogroup B capsular polysaccharide in Neisseria meningitidis. Infect Immun. (2005) 73:1491–505. doi: 10.1128/IAI.73.3.1491-1505.2005
41. Willis LM, Whitfield C. Kpsc and KpsS are retaining 3-deoxy-D-manno-oct-2- ulosonic acid (Kdo) transferases involved in synthesis of bacterial capsules. Proc Natl Acad Sci. (2013) 110:20753–8. doi: 10.1073/PNAS.1312637110/-/DCSUPPLEMENTAL
42. Starr KF, Porsch EA, Seed PC, St. Geme JW III. Genetic and molecular basis of Kingella kingae encapsulation. Infect Immun. (2016) 84:1775–84. doi: 10.1128/IAI.00128-16
43. Starr KF, Porsch EA, Seed PC, Heiss C, Naran R, Forsberg LS, et al. Kingella kingae expresses four structurally distinct polysaccharide capsules that differ in their correlation with invasive disease. PLoS Pathog. (2016) 12:e1005944. doi: 10.1371/journal.ppat.1005944
44. Starr KF, Porsch EA, Heiss C, Black I, Azadi P, St. Geme JW III. Characterization of the Kingella kingae polysaccharide capsule and exopolysaccharide. PLoS One. (2013) 8:75409. doi: 10.1371/journal.pone.0075409
45. Bendaoud M, Vinogradov E, Balashova NV, Kadouri DE, Kachlany SC, Kaplan JB. Broad-spectrum biofilm inhibition by Kingella kingae exopolysaccharide. J Bacteriol. (2011) 193:3879–86. doi: 10.1128/JB.00311-11
46. Reistad R, Zähringer U, Bryn K, Alstad J, Bøvre K, Jantzen E. A polysaccharide produced by a mucoid strain of Moraxella nonliquefaciens with a 2-acetamido-2-deoxy-5-O-(3-deoxy-β-d-manno-octulopyranosyl)-β-d-galactopyranosyl repeating unit. Carbohydr Res. (1993) 245:129–36. doi: 10.1016/0008-6215(93)80065-M
47. Perry MB. Structural analysis of the lipopolysaccharide of Actinobacillus (Haemophilus) pleuropneumoniae serotype 10. Biochem Cell Biol. (1990) 68:808–9. doi: 10.1139/O90-117
48. Basmaci R, Bidet P, Mallet C, Vialle R, Bonacorsi S. Distribution of Kingella kingae capsular serotypes in France assessed by a multiplex PCR assay on osteoarticular samples. J Clin Microbiol. (2018) 56:1491–509. doi: 10.1128/JCM.01491-18
49. Basmaci R, Deschamps K, Levy C, Mathy V, Corrard F, Thollot F, et al. Prevalence of Kingella kingae oropharyngeal carriage and predominance of type a and type b polysaccharide capsules among French young children. Clin Microbiol Infect. (2019) 25:114–6. doi: 10.1016/J.CMI.2018.07.033
50. Porsch EA, Starr KF, Yagupsky P, St. Geme JW. The type a and type b polysaccharide capsules predominate in an international collection of invasive Kingella kingae isolates. mSphere. (2017) 2:e00060–17. doi: 10.1128/msphere.00060-17
51. Muñoz VL, Porsch EA, St. Geme JW III. Kingella kingae surface polysaccharides promote resistance to human serum and virulence in a juvenile rat model. Infect Immun. (2018) 86:1–13. doi: 10.1128/IAI.00100-18
52. Muñoz VL, Porsch EA, St. Geme JW III. Kingella kingae surface polysaccharides promote resistance to neutrophil phagocytosis and killing. MBio. (2019) 10:e00631–19. doi: 10.1128/MBIO.00631-19
53. Montoya NR, Porsch EA, Muñoz VL, Muszyński A, Vlach J, Hahn DK, et al. Surface anchoring of the Kingella kingae galactan is dependent on the lipopolysaccharide O-antigen. MBio. (2022). doi: 10.1128/mbio.02295-22. [Epub ahead of print]36069736
54. Bertani B, Ruiz N. Function and biogenesis of lipopolysaccharides. EcoSal Plus. (2018) 8:1–19. doi: 10.1128/ecosalplus.esp-0001-2018
55. Lerouge I, Vanderleyden J. O-antigen structural variation: mechanisms and possible roles in animal/plant–microbe interactions. FEMS Microbiol Rev. (2002) 26:17–47. doi: 10.1111/j.1574-6976.2002.tb00597.x
56. Amit U, Porat N, Basmaci R, Bidet P, Bonacorsi S, Dagan R, et al. Genotyping of invasive Kingella kingae isolates reveals predominant clones and association with specific clinical syndromes. Clin Infect Dis. (2012) 55:1074–9. doi: 10.1093/cid/cis622
57. Basmaci R, Bidet P, Yagupsky P, Muñoz-Almagro C, Balashova NV, Doit C, et al. Major intercontinentally distributed sequence types of Kingella kingae and development of a rapid molecular typing tool. J Clin Microbiol. (2014) 52:3890–7. doi: 10.1128/JCM.01609-14
58. Basmaci R, Yagupsky P, Ilharreborde B, Guyot K, Porat N, Chomton M, et al. Multilocus sequence typing and rtxA toxin gene sequencing analysis of Kingella kingae isolates demonstrates genetic diversity and international clones. PLoS One. (2012) 7:e38078. doi: 10.1371/JOURNAL.PONE.0038078
59. El Houmami N, Bzdreng J, Durand GA, Minodier P, Seligmann H, Prudent E, et al. Molecular tests that target the RTX locus do not distinguish between Kingella kingae and the recently described Kingella negevensis species. J Clin Microbiol. (2017) 55:3113–22. doi: 10.1128/JCM.00736-17
60. Frye SA, Nilsen M, Tønjum T, Ambur OH. Dialects of the DNA uptake sequence in Neisseriaceae. PLOS Genet. (2013) 9:e1003458. doi: 10.1371/JOURNAL.PGEN.1003458
61. Bidet P, Basmaci R, Guglielmini J, Doit C, Jost C, Birgy A, et al. Genome analysis of Kingella kingae strain KWG1 reveals how a β-lactamase gene inserted in the chromosome of this species. Antimicrob Agents Chemother. (2016) 60:703–8. doi: 10.1128/AAC.02192-15
62. Yagupsky P, Slonim A, Amit U, Porat N, Dagan R. Beta-lactamase production by Kingella kingae in Israel is clonal and common in carriage organisms but rare among invasive strains. Eur J Clin Microbiol Infect Dis. (2013) 32:1049–53. doi: 10.1007/S10096-013-1849-1/TABLES/1
63. Basmaci R, Bidet P, Berçot B, Jost C, Kwon T, Gaumetou E, et al. First identification of a chromosomally located penicillinase gene in Kingella kingae species isolated in continental Europe. Antimicrob Agents Chemother. (2014) 58:6258–9. doi: 10.1128/AAC.03562-14
64. Basmaci R, Bidet P, Jost C, Yagupsky P, Bonacorsi S. Penicillinase-encoding gene blaTEM-1 may be plasmid borne or chromosomally located in Kingella kingae species. Antimicrob Agents Chemother. (2015) 59:1377–8. doi: 10.1128/AAC.04748-14
Keywords: kingella kingae, type IV pili, adhesin, toxin, capsule, exopolysaccharide, lipopolysaccharide, genomics
Citation: Porsch EA, Hernandez KA, Morreale DP, Montoya NR, Yount TA and St. Geme III JW (2022) Pathogenic determinants of Kingella kingae disease. Front. Pediatr. 10:1018054. doi: 10.3389/fped.2022.1018054
Received: 12 August 2022; Accepted: 16 September 2022;
Published: 11 October 2022.
Edited by:
Pablo Yagupsky, Soroka Medical Center, IsraelReviewed by:
Radim Osicka, Institute of Microbiology (ASCR), CzechiaRomain Basmaci, Hôpital Louis-Mourier, France
Pierre-Edouard Fournier, Aix-Marseille Université, France
© 2022 Porsch, Hernandez, Morreale, Montoya, Yount and St. Geme. This is an open-access article distributed under the terms of the Creative Commons Attribution License (CC BY). The use, distribution or reproduction in other forums is permitted, provided the original author(s) and the copyright owner(s) are credited and that the original publication in this journal is cited, in accordance with accepted academic practice. No use, distribution or reproduction is permitted which does not comply with these terms.
*Correspondence: Joseph W. St. Geme III c3RnZW1laWlpakBjaG9wLmVkdQ==
Specialty Section: This article was submitted to Pediatric Infectious Diseases, a section of the journal Frontiers in Pediatrics