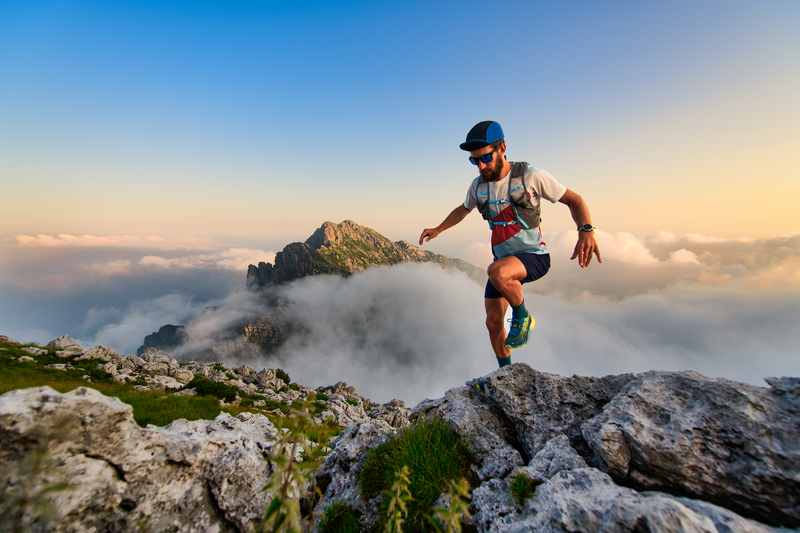
95% of researchers rate our articles as excellent or good
Learn more about the work of our research integrity team to safeguard the quality of each article we publish.
Find out more
REVIEW article
Front. Pediatr. , 04 October 2022
Sec. Neonatology
Volume 10 - 2022 | https://doi.org/10.3389/fped.2022.1008539
This article is part of the Research Topic Fetal and Neonatal Oxygen Metabolism View all 8 articles
Brain tissue temperature is a dynamic balance between heat generation from metabolism, passive loss of energy to the environment, and thermoregulatory processes such as perfusion. Perinatal brain injuries, particularly neonatal encephalopathy, and seizures, have a significant impact on the metabolic and haemodynamic state of the developing brain, and thereby likely induce changes in brain temperature. In healthy newborn brains, brain temperature is higher than the core temperature. Magnetic resonance spectroscopy (MRS) has been used as a viable, non-invasive tool to measure temperature in the newborn brain with a reported accuracy of up to 0.2 degrees Celcius and a precision of 0.3 degrees Celcius. This measurement is based on the separation of chemical shifts between the temperature-sensitive water peaks and temperature-insensitive singlet metabolite peaks. MRS thermometry requires transport to an MRI scanner and a lengthy single-point measurement. Optical monitoring, using near infrared spectroscopy (NIRS), offers an alternative which overcomes this limitation in its ability to monitor newborn brain tissue temperature continuously at the cot side in real-time. Near infrared spectroscopy uses linear temperature-dependent changes in water absorption spectra in the near infrared range to estimate the tissue temperature. This review focuses on the currently available methodologies and their viability for accurate measurement, the potential benefits of monitoring newborn brain temperature in the neonatal intensive care unit, and the important challenges that still need to be addressed.
In term infants, neonatal encephalopathy (NE) is the most common form of perinatal brain injury in both high-income countries (HIC) as well as low- and middle-income countries (LMIC). Hypoxic-ischemic encephalopathy (HIE) affects 1–6 per 1,000 live births and remains a major cause of morbidity and mortality in term infants (1–3). Seizures are the most common presentation of neonatal neurological emergencies with an incidence of 1.9 to 2.2 per 1,000 live births (3). They are associated with further neuronal injury, poor neurological outcome, increased brain injury on MRI (4), and increased risk of epilepsy (5). Current research focuses on identifying optimal neuroprotective therapies for both these conditions to improve neurodevelopmental outcomes. A detailed understanding of the pathophysiological changes relating to brain metabolism and perfusion and their impact on brain tissue in real-time is a key factor in the assessment of both injury severity and its evolution.
Brain temperature is determined by the balance of heat production and heat removal, and it is influenced by multiple factors including cerebral metabolism, brain tissue injury, cerebral blood flow (CBF), body-brain temperature difference, various medications and infection (6–9). The brain and/or body temperature elevation is often associated with brain injury as seen in traumatic brain injury, adult stroke, and NE (10–13). The magnitude of temperature elevation has been shown to correlate with infarct size and severity in adults and is a risk factor for poor clinical outcomes (13–15). With significant changes in cerebral metabolism and perfusion following hypoxic-ischaemic injury and during neonatal seizures, it is likely to have brain temperature perturbation following perinatal brain injury and real-time brain temperature monitoring therefore might be an important biomarker.
The newborn brain suffers significant hemodynamic and metabolic derangements following hypoxic-ischaemic brain injury which causes a series of neurotoxic and neurochemical cascades over a period of several hours, days, and weeks post-injury (16). Early pre-clinical and clinical studies using phosphorous (31P) magnetic resonance spectroscopy (MRS) described the evolution of primary and secondary energy failure with a reduction in high energy phosphates and a rise in cerebral lactate following injury (17, 18). During the initial insult, a proportion of cells undergo primary cell death, and the neuronal supply of high energy metabolites such as adenosine triphosphate (ATP) is exhausted, also termed “primary energy failure.” Following successful resuscitation, the brain enters a latent phase lasting for ∼6–24 h which is characterized by the partial recovery of cerebral oxidative metabolism and cerebral blood flow, (CBF) although a degree of hypoperfusion continues (19, 20). The brain then enters a period of “secondary energy failure” (SEF) characterized by mitochondrial impairment and subsequent cell death with associated cerebral autoregulatory disturbance and brain hyperperfusion (21). Hypoxia-ischaemia induces significant cerebral inflammation with the production of proinflammatory cytokines (22) and activation of complement (23) that can further potentially be related to increased brain temperature. The concept of secondary energy failure (SEF) is a hallmark of NE and the primary target of current therapeutic hypothermia (TH) treatment. Abnormal autoregulatory control of cerebral haemodynamics with a combination of vasodilatation and vasoparalysis (24) is common after NE.
NE remains the major etiological factor for the development of neonatal seizures and TH reduces the seizure burden following NE (25). Up to 75% of infants with NE can develop seizures (26) which are defined as transient symptoms of excessive or synchronous neuronal activity in the brain (27–29). Mitochondrial metabolism is closely related to neuronal activity. Studies using 31P MRS have revealed a drop in high-energy phosphates by one-third and an increase in mitochondrial oxidative phosphorylation by 45% during neonatal seizure (30), indicating a depleted cerebral energy state. Electroclinical and electrographic seizures produce an increase in cerebral blood flow velocity (CBFV) (31), likely to be due to excessive demand for glucose and oxygen but may still be insufficient to meet the pathological demands. A prolonged increase in cerebral blood flow is also likely to contribute to cerebral oedema and vasoparesis with accompanying loss of autoregulatory mechanisms. Cerebral autoregulation has been noted to be absent both during the seizures themselves and between seizures (32).
Previous studies with near-infrared spectroscopy (NIRS) during neonatal seizures have also described changes in cerebral hemodynamics and oxygenation (33–36). NIRS measures concentration changes in oxygenated [Δ(HbO2)] and deoxygenated haemoglobin [Δ(HHb)] which can then be used to derive changes in total haemoglobin [Δ(HbT)] and haemoglobin difference [Δ(HbD)]. Changes in [Δ(HbT)] and [Δ(HbD)] represent changes in cerebral blood volume and oxygenation respectively. In addition, cerebral oximeters can also measure absolute brain tissue saturation (or cerebral oxygenation) as an absolute percentage measurement of the mixed arterial and venous saturation. A seizure typically increases both cerebral blood flow and cerebral metabolic rate (37). Any increase in cerebral blood flow may not always be sufficient to match the cerebral metabolic demand during prolonged seizures, indicating cerebral blood flow-metabolism uncoupling (38) which can lead to reduced cerebral oxygenation. Previous studies in preterm infants suggested a 10% reduction in cerebral oxygenation to be of clinical concern (39), while animal studies using NIRS have found mixed cerebral oxygenation of 40%–50% (40, 41) to be the limits below which significant cerebral hypoxia with poor neurologic outcome occurs. Our previous work showed a rapid increase in the change in the oxidation state of cytochrome-c-oxidase [Δ(oxCCO)], noted at the onset of a seizure episode along with a rise in the baseline aEEG indicating an increase in neuronal activation and energy demand. Cytochrome-c-oxidase is the terminal electron acceptor in the mitochondrial electron transfer chain and is responsible for most of the ATP production during oxidative metabolism. Progressive decline in the [Δ(oxCCO)] baseline during seizures suggests a progressive decrease in mitochondrial oxidative metabolism (42). Neuronal energy demand rapidly increases at the onset of seizures reflected by a rapid increase in the mean aEEG activity coinciding with a rise in Δ[oxCCO].
Several different methodologies have been evaluated so far for brain temperature measurements (Table 1). Proton MRS (43–50), microwave radiometry (51–53) and ultrasound thermometry (54). Other approaches are still under experimental evaluation in animals, for example, non-invasive wearable sensors to assess deep brain temperature based on skin thermal conductivity (55), or invasive optical fibre-based thermometry (56) Finally, there have been computationally based approaches to estimate brain temperature changes from traditional brain recordings such as MRI using mathematical models of brain temperature (57, 58) that consider the brain’s non-equilibrium thermodynamic nature between rest and functional activity. However, in clinical practice, these approaches remain to be tested. None of these methods is feasible for long-term ambulatory clinical use in newborn infants and requires large cost-intensive equipment. Optical methods for brain temperature measurement provide an option for use in clinical settings, even in ambulatory settings, given their ease of handling through their portability and cost-efficiency. Optical thermometry can furthermore, determine the efficacy of hypothermia, noninvasively and continuously throughout TH in HIE.
MRS thermometry has been used most for non-invasive and in-vivo brain temperature measurement in the neonatal population (47, 49, 59, 60). Clinical MRS reveals the prominent peaks of N-acetyl-aspartate (NAA), Choline (Cho) and Creatine (Cr). The much larger water peak is usually artificially suppressed as it obscures the visibility of the other spectral peaks. The relative position of a peak in the spectrum is described by its chemical shift. MRS thermometry is possible because of the temperature dependence of the water chemical shift relative to the temperature-insensitive chemical shifts of NAA, Cho and Cr. Careful measurement of the chemical shift separation between the water and reference-metabolite peaks, along with suitable calibration, can yield brain temperature estimation with an accuracy of ±0.5 °C and precision of 0.3 °C (59, 61, 62).
Non-invasive local temperature measurement using proton MRS has been demonstrated in vivo for many applications. The chemical shift of water is approximately linearly dependent on temperature in the physiological range (Hindman). So, by measuring the chemical shift separation between water and one or more reference metabolites, the absolute temperature can be inferred. Figure 1 describes the basic methodology. Spectra must first be obtained that show the un-suppressed water peak and the reference peaks. This can be done by acquiring a single un-suppressed spectrum and removing the water signal in post-processing to reveal the metabolite signals (50). Alternatively, this can be achieved by acquiring a water spectrum and a water-suppressed metabolite spectrum sequentially without changing the receiver frequency (49). By fitting an assumed line shape function to the water and metabolite peaks, the chemical shift separation in ppm can be measured and converted into temperature using an appropriate calibration.
Figure 1. Brain temperature measurement using proton magnetic resonance spectroscopy. (A) Indicates the chemical shift separation between the water peak and a reference metabolite (NAA), (B) represents the calculation of brain temperature using a calibration based on the water-NAA chemical shift separation.
The absolute change in the water peak chemical shift with temperature is very small, about 0.01 ppm per degree Celcius. Therefore, to measure temperature with a precision of 0.5 °C it is necessary to be able to measure the chemical shift separation to a precision of 0.005 pm. Compare this with a typical water linewidth in vivo of about 0.05 ppm. Despite this challenge, accuracies of 0.2–0.5 °C have been reported (59, 61–63).
In-vivo MRS temperature measurement has been reported using both single voxel methods (49, 50, 59, 63, 64) and spectroscopic imaging (65–68). A challenge for both types of methods is obtaining a good shim to make the magnetic field as uniform as possible and thus minimise the water and metabolite linewidths. The movement of the subject during acquisition can make this even more challenging. Single voxel methods may be easier to implement to obtain a high precision measurement. In order to obtain sufficient signal to make the measurement, many repeated data acquisitions are averaged together. These can be compared and corrected for artefacts due to subject motion, resulting in an improved final measurement (50). However, single voxel acquisitions are limited to reporting temperature from a single location. Spectroscopic imaging methods allow the spatial variation of temperature to be mapped, but it can be more challenging to obtain a high-quality shim over an extended spatial region.
The link between the measured chemical shift separation between the water and reference peaks, and temperature is the calibration data. Numerous calibrations have been published (59, 60, 69, 70). Changes in protein and ionic concentration have been shown to alter temperature calibration curves (71, 72). The apparent measured temperature decreases with ionic concentration by about 1 degree C per 100 mmol and increases with protein concentration (72). The calibration data, therefore, show a dependence on the conditions under which they were collected, and it is important to select an appropriate calibration for the desired application (73).
More than one reference peak can be used to make the temperature measurement. This can increase the precision of the measurement and the resilience of the measurement to pathological changes to the spectrum composition (59). Care must be taken when combining data from more than one reference peak so that the calibrations are internally consistent with each other (50). The choice of calibration will affect the level of systematic error in the absolute temperature measurement and so some care must be taken in interpreting MRS-derived temperature data. It is likely that temperature variation over time or relative spatial temperature differences are more reliable measurements than the absolute values themselves.
Optical methods based on NIRS can also be used to monitor tissue temperature. These methods are promising as they are based on safe and portable instruments that can be used to monitor the temperature continuously at the cot side. In the near-infrared region (650–1000 nm), the major endogenous tissue chromophores, responsible for the light’s absorption of the tissue, are water and oxy- and deoxy- haemoglobin. The aim of NIRS is to quantify the concentrations, or change in concentrations related to a specific event, of these chromophores in tissue and its focus is to quantify the oxygenation of the tissues. However, the linear temperature-dependent changes in NIR water absorption spectra make the measurement of tissue temperature possible with NIRS, as the tissues are mostly composed of water (more than 70% of the brain tissue composition for example).
Indeed, the water absorption spectra and its temperature dependency has been well established (74, 75). These spectra can be seen in Figure 2A (left), where the water absorption peaks shift to higher wavelengths and decrease in amplitude by approximately 0.8% per degree Celsius around 740, 840, and 970 nm with decreasing temperature (74). The absorptivity temperature coefficients between 550 and 900 nm can also be seen in Figure 2B (right) (extracted from reference 76), where the 2 peaks around 740 and 840 nm are clearly visible. Using this property, the temperature of the adult arm77 was measured using broadband continuous wave NIRS (BNIRS). In that paper, Hollis et al. (77) used the technique of principal component analysis (PCA) to calibrate the temperature response of the absorption spectra of pure water to predict the temperature response of the tissues. They particularly focused on the bands at 740 and 840 nm and reported a standard error of prediction of 1.2 °C. They noted that the results could be improved if the scattering properties of the tissues were accounted for, which was not the case in that model to retrieve the changes in temperature. A similar approach was then used by Holper et al. (78), focusing on the 840 band, in order to monitor the brain temperature of piglets and neonates. Using their methodologies, the authors reported an overall mean error bias between NIRS predicted brain temperature and body temperature of 0.436 ± 0.283 °C (animal dataset) and 0.162 ± 0.149 °C (human dataset).
Figure 2. NIRS thermometry. Left (A) - NIR absorption spectra of pure water at various temperature. Extracted from Hollis et al. (74). Right (B) - Absorptivity temperature coefficients (da/dT) for the 550–900 nm. Extracted from Langford et al. (75).
Other studies using broadband diffuse optical spectroscopy (DOS) reported the measurement of the adult breas (79) and forearm (80). In these studies, the water peak at 970 nm was used to predict the temperature of tissues. The main limitation of this technique is that the strong absorption of the water at this wavelength limits its depth sensitivity, which makes it difficult to use for the monitoring of brain temperature in adults for example. However, the advantage of the use of DOS is to be able to disentangle the absorption and scattering properties of the tissues, thus reducing the impact of the scattering on the temperature measurement. Indeed, in NIRS measurements, as seen previously, it is often assumed that the changes in the optical signal came from a change in absorption only. However, in a clinical context, significant physiological changes can induce significant scattering changes which need to be considered to avoid crosstalk between scattering and absorption (81). Indeed, the scattering parameters of the tissue are mainly originating from the subcellular structures (82) but also from the cerebrospinal fluid layer in the subarachnoid space (83) and from the blood flow (84). Clinically, it has been shown that the resting scattering properties between the normal and affected areas in patients with traumatic brain injury (TBI) (85) and stroke (86) were significantly different. Moreover, dynamic changes in scattering are also detected when a large variation of blood flow is present (81). Thus, as blood flow variations are very likely in NE, changes in scattering coefficient can be expected. Therefore, one of the main challenges for NIRS instruments aiming at measuring brain temperature in a clinical context is to be able to measure both the absorption and scattering properties of the tissue in order to give accurate thermometry readings.
Finally, the brain temperature of piglets was measured using a time-domain NIRS (TD-NIRS) (87). TD-NIRS is known to be the most accurate of the NIRS techniques, can disentangle the absorption and scattering properties of tissues, and has the best depth sensitivity (88). Therefore, it makes it a great candidate to measure brain temperature even in difficult cases. Using this methodology, Bakhsheshi et al. used the bands at 740 and 840 nm in combination with the method of the PCA, introduced by Hollis et al., to monitor brain temperature in newborn piglets during cooling. The deep brain temperature (DBT) was also measured continuously with a thermocouple probe during this monitoring and the mean difference between the optical and DBT was 0.5 °C ± 1.6 °C. This methodology combined the two independent strengths of the studies previously mentioned: a scattering-free method (like DOS), increasing its accuracy, relying on the 740 and 840 nm bands (like BNIRS), and increasing its depth sensitivity.
Looking to the future, broadband TD-NIRS systems could further benefit brain temperature monitoring by NIRS techniques, as in general, the accuracy of the temperature prediction can be improved by acquiring a continuous absorption spectrum. Indeed, it allows a more accurate determination of chromophore concentrations compared to discrete wavelengths, as more chromophores can be quantified, and a more refined data analysis technique can be used. Such systems have been reported in the literature (89, 90), however, temperature monitoring was out of the scope of these studies. BNIRS is also able to monitor continuous absorption and scattering properties of light when appropriate algorithms and methodologies are used (91). Therefore, these 2 techniques appear to be good candidates in order to develop robust optical brain thermometry tools for clinical use.
The recent technological developments, notably in terms of electronics, enabled to reduce the footprint of TD-NIRS, which facilitated its use for clinical applications (88) and compact TD-NIRS systems are now available. Therefore, even though more work remains to be done in order to develop an accurate and robust optical instrument to monitor the brain temperature at the bedside, the recent technological developments make the possibility to develop small footprint instrument able to measure brain temperature now within reach. The next step will be to test the current methodologies in the clinic, as it has not been experimented so far.
Brain temperature is determined by the balance of heat production and heat removal, and it is influenced by multiple factors including cerebral metabolism, brain tissue injury, blood flow, body/brain temperature, drugs, sedation, seizures, and infection (6–9). The brain and/or body temperature elevation is often associated with brain injury as seen in traumatic brain injury, adult stroke, and HIE (10–13). The magnitude of temperature elevation correlates with infarct size and severity and is a risk factor for poor clinical outcomes in adults (13–15). MRS thermometry and mapping found the lowest body temperature at the core of tissue injury in adults with acute ischemic stroke and the highest brain temperature in the penumbral region (11).
Hypothermia decreases the metabolic demand for glucose and oxygen and attenuates secondary energy failure and neuroapoptosis (92, 93). The efficacy of TH depends on the target organ temperature and the neuroprotective effect depends on achieving the correct target temperature range (94, 95).
The current hypothermic strategy for NE uses rectal temperature for servo-controlled feedback to maintain a steady temperature profile. An in-vivo assessment of regional brain temperature using proton MRS during whole-body TH revealed heterogenicity of the brain temperature profile. Hypothermia effectively lower deep grey matter structures, whereas temperatures of more superficial structures in the grey matter and white matter are significantly greater than rectal temperatures (96). Findings also suggest that infants with MRI evidence of injury had overall higher and more homogenous brain temperature than those without injury (97). Non-homogenous patterns of brain temperature were also shown in other studies (50–66). In infants developing brain injury after NE, hypothermia decreased brain temperature during the first days of life but did not prevent an early increase of brain temperature (66). Wu et al. found significantly higher temperatures and brain-rectal temperature gradients in neonates with NE during TH (49). The application of using one specific baby temperature (33.5 °C) in NE for neuroprotection has improved the overall outcome of HIE but a single core temperature may not provide equal neuroprotective benefits to brain structures that have different histology, metabolic needs, and blood supply distribution. The threshold temperature to achieve neuroprotection may be unique to different brain structures. The heterogenicity of brain temperature while whole-body cooling raises the question of whether the current cooling system or methodology is the optimal way to cool. We need to explore whether there is a way to improve the homogeneity of white matter or cortical cooling and whether will this lead to improved neurological outcomes.
An animal study suggested that focal seizures produce an increase in neuronal activity and led to an elevation of local blood flow, cerebral metabolism and a significant rise in brain temperature (96). Generally, physiologic brain temperature is slightly warmer than core body temperature and subcortical structures are warmer than cortical structures (7, 67). However, injuries such as stroke can generate a brain-body temperature gradient, in which case core body temperature becomes a poor surrogate of brain temperature (98, 99). In adults with ischemic stroke, the temperature of the ipsilateral hemisphere is greater than the contralateral hemisphere (11, 61). Indeed, temperature alterations after neurologic injury, especially an increase in the brain or systemic temperature, are related to poor clinical outcomes (13, 15, 100, 101). During pathological processes such as neuroinflammation, increase metabolic demands overwhelm the brain’s already limited cooling mechanisms and drive temperature 1–2 °C higher than core body temperature (102).
In animal studies focal cooling rapidly terminates experimental neocortical seizures and histological examination of the cortex after cooling has shown no evidence of acute or delayed neuronal injury, and blood pressure and temperature remained stable (103). Animal experiments show that gentle cooling is capable of markedly reducing subsequent seizure frequency and intensity (104). The efficacy of TH in reducing seizure burden following NE has also been described (25).
Confirming brain injury at the bedside and determining the type and severity remains a challenge, as does bedside identification and monitoring of injuries likely involving ongoing processes of oxidative stress, excitotoxicity, inflammation, repair, and cell death, evolving over hours to weeks. As novel treatment strategies for neonatal brain injuries and seizures become available, the need for non-invasive and continuous bedside monitoring of disease severity and response to treatment becomes increasingly apparent. Non-invasive and continuous measurements of brain temperature in the neonatal neurocritical care set-up may permit the selection of neonatal candidates who may benefit from an adjustment in their hypothermia therapy or for additional neuroprotective therapies. Further studies are now urgently needed to establish whether optical brain monitoring can be a useful neuromonitoring tool in neonatal neurocritical care set-up. In view of the heterogeneous profile of brain temperature during TH, an option of continuous non-invasive monitoring of brain temperature at the bedside might also provide an opportunity to review and improve the current cooling methodologies in neonatal neurocritical care following NE.
Conceptualisation by SM. VV prepared the first draft, AB, FL, KHJ, IT, NJR and SM contributed to different aspects of the paper. All authors contributed to the article and approved the submitted version.
The authors are supported by The Wellcome Trust (219610/Z/19/Z), Medical Research Council (MR/S003134/1) and National Institute for Health Research University College London Hospitals Biomedical Research Center.
The authors declare that the research was conducted in the absence of any commercial or financial relationships that could be construed as a potential conflict of interest.
All claims expressed in this article are solely those of the authors and do not necessarily represent those of their affiliated organizations, or those of the publisher, the editors and the reviewers. Any product that may be evaluated in this article, or claim that may be made by its manufacturer, is not guaranteed or endorsed by the publisher.
2. De Vries LS, Jongmanas MJ. Long-term outcome after neonatal hypoxic-ischemic encephalopathy. Arch Dis Child Fetal Neonatal Ed. (2010) 95:F220–4. doi: 10.1136/adc.2008.148205
3. Gale C, Statnikov Y, Jawad S, Uthaya SN, Modi N. Brain injuries expert working group. Neonatal brain injuries in England: population-based incidence derived from routinely recorded clinical data held in the National Neonatal Research Database. Arch Dis Child Fetal Neonatal Ed. (2018) 103(4):F301–6. doi: 10.1136/archdischild-2017-313707
4. Glass HC, Glidden D, Jeremy RJ, Barkovich AJ, Ferriero DM, Miller SP. Clinical neonatal seizures are independently associated with outcome in infants at risk for hypoxic-ischemic brain injury. J Pediatr. (2009) 155(3):318–23. doi: 10.1016/j.jpeds.2009.03.040
5. Glass HC, Hong KJ, Rogers EE, Jeremy RJ, Bonifacio SL, Sullivan JE, et al. Risk factors for epilepsy in children with neonatal encephalopathy. Pediatr Res. (2011) 70(5):535–40. doi: 10.1203/PDR.0b013e31822f24c7
6. Bertolizio G, Mason L, Bissonnette B. Brain temperature: heat production, elimination and clinical relevance. Paediatr Anaesth. (2011) 21:347–58. doi: 10.1111/j.1460-9592.2011.03542.x
7. Wang H, Wang B, Normoyle KP, Jackson K, Spitler K, Sharrock MF, et al. Brain temperature and its fundamental properties: a review for clinical neuroscientists. Front Neurosci. (2014) 8:307. doi: 10.3389/fnins.2014.00307
8. Yager JY, Armstrong EA, Jaharus C, Saucier DM, Wirrell EC. Preventing hyperthermia decreases brain damage following neonatal hypoxic-ischemic seizures. Brain Res. (2004) 1011:48–57. doi: 10.1016/j.brainres.2004.02.070
9. Zhu M, Ackerman JJ, Sukstanskii AL, Yablonskiy DA. How the body controls brain temperature: the temperature shielding effect of cerebral blood flow. J Appl Physiol. (2006) 101:1481–8. doi: 10.1152/japplphysiol.00319.2006
10. Millergard P, Nordstrom CH. Intracerebral temperature in neurosurgical patients. Neurosurgery. (1991) 28:709–13. doi: 10.1227/00006123-199105000-00012
11. Karaszewski B, Wardlaw JM, Marshall I, Cvoro V, Wartolowska K, Haga K, et al. Early brain temperature elevation and anaerobic metabolism in human acute ischaemic stroke. Brain. (2009) 132(Pt 4):955–64. doi: 10.1093/brain/awp010
12. Sahwab S, Spranger M, Aschoff A, Steiner T, Hacke W. Brain temperature monitoring and modulation in patients with severe MCA infraction. Neurology. (1997) 48:762–7. doi: 10.1212/WNL.48.3.762
13. Laptook A, Tyson J, Shankaran S, McDonald S, Ehrenkranz R, Fanaroff A, et al. Elevated temperature after hypoxic-ischemic encephalopathy: risk factor for adverse outcomes. Pediatrics. (2008) 122(3):491–9. doi: 10.1542/peds.2007-1673
14. Jorgensen HS, Reith J, Pedersen PM, Nakayama H, Olsen TS. Body temperature and outcome in stroke patients. Lancet. (1996) 348:193. doi: 10.1016/S0140-6736(05)66135-1
15. Reith J, Jørgensen HS, Pedersen PM, Nakayama H, Raaschou HO, Jeppesen LL, et al. Body temperature in acute stroke: relation to stroke severity, infarct size, mortality, and outcome. Lancet. (1996) 347(8999):422–5. doi: 10.1016/S0140-6736(96)90008-2
16. du Plessis AJ, Volpe JJ. Perinatal brain injury in the preterm and term newborn. Curr Opin Neurol. (2002) 15(2):151–7. doi: 10.1097/00019052-200204000-00005
17. Hope PL, Costello AM, Cady EB, Delpy DT, Tofts PS, Chu A, et al. Cerebral energy metabolism studied with phosphorus NMR spectroscopy in normal and birth-asphyxiated infants. Lancet. (1984) 2(8399):366–70. doi: 10.1016/S0140-6736(84)90539-7
18. Azzopardi D, Wyatt JS, Cady EB, Delpy DT, Baudin J, Stewart AL, et al. Prognosis of newborn infants with hypoxic-ischemic brain injury assessed by phosphorus magnetic resonance spectroscopy. Pediatr Res. (1989) 25(5):445–51. doi: 10.1203/00006450-198905000-00004
19. van Bel F, Dorrepaal CA, Benders MJ, Zeeuwe PE, van de Bor M, Berger HM. Changes in cerebral hemodynamics and oxygenation in the first 24 hours after birth asphyxia. Pediatrics. (1993) 92(3):365–72. doi: 10.1542/peds.92.3.365
20. Rosenkrantz TS, Zalneraitis EL. Prediction of survival in severely asphyxiated infants. Pediatr Neurol. (1991) 7:446–51. doi: 10.1016/0887-8994(91)90029-K
21. Meek JH, Elwell CE, McCormick DC, Edwards AD, Townsend JP, Stewart AL, et al. Abnormal cerebral haemodynamics in perinatally asphyxiated neonates related to outcome. Arch Dis Child Fetal Neonatal Ed. (1999) 81(2):F110–5. doi: 10.1136/fn.81.2.F110
22. Oygür N, Sönmez O, Saka O, Yeğin O. Predictive value of plasma and cerebrospinal fluid tumour necrosis factor-alpha and interleukin-1 beta concentrations on outcome of full term infants with hypoxic-ischaemic encephalopathy. Arch Dis Child Fetal Neonatal Ed. (1998) 79(3):F190–3. doi: 10.1136/fn.79.3.F190
23. Schultz SJ, Aly H, Hasanen BM, Khashaba MT, Lear SC, Bendon RW, et al. Complement component 9 activation, consumption, and neuronal deposition in the post-hypoxic-ischemic central nervous system of human newborn infants. Neurosci Lett. (2005) 378(1):1–6. doi: 10.1016/j.neulet.2004.12.008
24. Cope M, Delpy DT. A system for the long-term measurement of cerebral blood and tissue oxygenation in newborn infants by near infra-red transillumination. Med Biol Eng Comput. (1988) 26:289–94. doi: 10.1007/BF02447083
25. Low E, Boylan GB, Mathieson SR, Murray DM, Korotchikova I, Stevenson NJ, et al. Cooling and seizure burden in term neonates: an observational study. Arch Dis Child Fetal Neonatal Ed. (2012) 97(4):F267–72. doi: 10.1136/archdischild-2011-300716
26. Gluckman PD, Wyatt JS, Azzopardi D, Ballard R, Edwards AD, Ferriero DM, et al. Selective head cooling with mild systemic hypothermia after neonatal encephalopathy: multicentre randomised trial. Lancet. (2005) 365(9460):663–70. doi: 10.1016/S0140-6736(05)17946-X
27. Fisher RS, van Emde Boas W, Blume W, Elger C, Genton P, Lee P, et al. Epileptic seizures and epilepsy: definitions proposed by the International League Against Epilepsy (ILAE) and the International Bureau for Epilepsy (IBE). Epilepsia. (2005) 46(4):470–2. doi: 10.1111/j.0013-9580.2005.66104.x
28. Tsuchida TN, Wusthoff CJ, Shellhaas RA, Abend NS, Hahn CD, Sullivan JE, et al. American clinical neurophysiology society standardized EEG terminology and categorization for the description of continuous EEG monitoring in neonates: report of the American Clinical Neurophysiology Society critical care monitoring committee. J Clin Neurophysiol. (2013) 30(2):161–73. doi: 10.1097/WNP.0b013e3182872b24
29. Pressler RM, Cilio MR, Mizrahi EM, Moshé SL, Nunes ML, Plouin P, et al. The ILAE classification of seizures and the epilepsies: modification for seizures in the neonate. Position paper by the ILAE task force on neonatal seizures. Epilepsia. (2021) 62(3):615–28. doi: 10.1111/epi.16815
30. Younkin DP, Delivoria-Papadopoulos M, Maris J, Donlon E, Clancy R, Chance B. Cerebral metabolic effects of neonatal seizures measured with in vivo 31P NMR spectroscopy. Ann Neurol. (1986) 20(4):513–9. doi: 10.1002/ana.410200412
31. Boylan GB, Panerai RB, Rennie JM, Evans DH, Rabe-Hesketh S, Binnie CD. Cerebral blood flow velocity during neonatal seizures. Arch Dis Child Fetal Neonatal Ed. (1999) 80(2):F105–10. doi: 10.1136/fn.80.2.F105
32. Boylan GB, Young K, Panerai RB, Rennie JM, Evans DH. Dynamic cerebral autoregulation in sick newborn infants. Pediatr Res. (2000) 48(1):12–7. doi: 10.1203/00006450-200007000-00005
33. Silas R, Sehgal A, Walker AM, Wong FY. Cerebral oxygenation during subclinical seizures in neonatal hypoxic-ischaemic encephalopathy. Eur J Paediatr Neurol. (2012) 16(3):304–7. doi: 10.1016/j.ejpn.2011.09.003
34. Wallois F, Patil A, Kongolo G, Goudjil S, Grebe R. Haemodynamic changes during seizure-like activity in a neonate: a simultaneous AC EEG-SPIR and high-resolution DC EEG recording. Neurophysiol Clin. (2009) 39:217–27. doi: 10.1016/j.neucli.2009.08.001
35. Singh H, Cooper RJ, Wai Lee C, Dempsey L, Edwards A, Brigadoi S, et al. Mapping cortical haemodynamics during neonatal seizures using diffuse optical tomography: a case study. Neuroimage Clin. (2014) 5:256–65. doi: 10.1016/j.nicl.2014.06.012
36. Mitra S, Bale G, Meek J, Tachtsidis I, Robertson NJ. Cerebral near infrared spectroscopy monitoring in term infants with hypoxic ischemic encephalopathy-A systematic review. Front Neurol. (2020) 11:393. doi: 10.3389/fneur.2020.00393
37. Zimmermann A, Domoki F, Bari F. Seizure-induced alterations in cerebrovascular function in the neonate. Dev Neurosci. (2008) 30(5):293–305. doi: 10.1159/000142735
38. Golnzalez H, Hunter CJ, Bennet L, Power GG, Gunn AJ. Cerebral oxygenation during postasphyxial seizures in near-term fetal sleep. J Cereb Blood Flow Metab. (2005) 25:911–8. doi: 10.1038/sj.jcbfm.9600087
39. Van Bel F, Lemmers P, Naulaers G. Monitoring neonatal regional cerebral oxygen saturation in clinical practice: value and pitfalls. Neonatology. (2008) 94(4):237–44. doi: 10.1159/000151642
40. Hou X, Ding H, Teng Y, Zhou C, Tang X, Li S, et al. Research on the relationship between brain anoxia at different regional oxygen saturations and brain damage using near-infrared spectroscopy. Physiol Meas. (2007) 28(10):1251–65. doi: 10.1088/0967-3334/28/10/010
41. Kurth CD, Levy WJ, McCann J. Near-infrared spectroscopy cerebral oxygen saturation thresholds for hypoxia-ischemia in piglets. J Cereb Blood Flow Metab. (2002) 22(3):335–41. doi: 10.1097/00004647-200203000-00011
42. Mitra S, Bale G, Mathieson S, Uria-Avellanal C, Meek J, Tachtsidis I, et al. Changes in cerebral oxidative metabolism during neonatal seizures following hypoxic-ischemic brain injury. Front Pediatr. (2016) 4:83. doi: 10.3389/fped.2016.00083
43. Ishigaki D, Ogasawara K, Yoshioka Y, Chida K, Sasaki M, Fujiwara S, et al. Brain temperature measured using proton MR spectroscopy detects cerebral hemodynamic impairment in patients with unilateral chronic major cerebral artery steno-occlusive disease: comparison with positron emission tomography. Stroke. (2009) 40(9):3012–6. doi: 10.1161/STROKEAHA.109.555508
44. Kickhefel A, Roland J, Weiss C, Schick F. Accuracy of real-time MR temperature mapping in the brain: a comparison of fast sequences. Phys Med. (2010) 26(4):192–201. doi: 10.1016/j.ejmp.2009.11.006
45. Murakami T, Ogasawara K, Yoshioka Y, Ishigaki D, Sasaki M, Kudo K, et al. Brain temperature measured by using proton MR spectroscopy predicts cerebral hyperperfusion after carotid endarterectomy. Radiology. (2010) 256(3):924–31. doi: 10.1148/radiol.10090930
46. Coman D, Trubel HK, Rycyna RE, Hyder F. Brain temperature and pH measured by (1)H chemical shift imaging of a thulium agent. NMR Biomed. (2009) 22(2):229–39. doi: 10.1002/nbm.1312
47. Corbett R, Laptook A, Weatherall P. Noninvasive measurements of human brain temperature using volume-localized proton magnetic resonance spectroscopy. J Cereb Blood Flow Metab. (1997) 17(4):363–9. doi: 10.1097/00004647-199704000-00001
48. Litt L, Hirai K, Basus VJ, James TL. Temperature control of respiring rat brain slices during high field NMR spectroscopy. Brain Res Brain Res Protoc. (2003) 10(3):191–8. doi: 10.1016/S1385-299X(02)00218-0
49. Wu TW, McLean C, Friedlich P, Wisnowski J, Grimm J, Panigrahy A, et al. Brain temperature in neonates with hypoxic-ischemic encephalopathy during therapeutic hypothermia. J Pediatr. (2014) 165(6):1129–34. doi: 10.1016/j.jpeds.2014.07.022
50. Bainbridge A, Kendall GS, De Vita E, Hagmann C, Kapetanakis A, Cady EB, et al. Regional neonatal brain absolute thermometry by 1H MRS. NMR Biomed. (2013) 26(4):416–23. doi: 10.1002/nbm.2879
51. Han JW, Van Leeuwen GM, Mizushina S, Van de Kamer JB, Maruyama K, Sugiura T, et al. Monitoring of deep brain temperature in infants using multi-frequency microwave radiometry and thermal modelling. Phys Med Biol. (2001) 46(7):1885–903. doi: 10.1088/0031-9155/46/7/311
52. Leroy Y, Bocquet B, Mamouni A. Non-invasive microwave radiometry thermometry. Physiol Meas. (1998) 19:127–48. doi: 10.1088/0967-3334/19/2/001
53. Stauffer PR, Snow BW, Rodrigues DB, Salahi S, Oliveira TR, Reudink D, et al. Non-invasive measurement of brain temperature with microwave radiometry: demonstration in a head phantom and clinical case. Neuroradiol J. (2014) 27(1):3–12. doi: 10.15274/NRJ-2014-10001
54. Lierke EG, Beuter K, Harr M. Ultrasound thermometry in deep tissues. In Thermological methods. VCH, Deerfield Beach: Florida (1984)
55. Dittmar A, Gehin C, Delhomme G, Boivin D, Dumont G, Mott C. A non invasive wearable sensor for the measurement of brain temperature. Conf Proc IEEE Eng Med Biol Soc. (2006) 2006:900–2. doi: 10.1109/IEMBS.2006.259429
56. Musolino S, Schartner EP, Tsiminis G, Salem A, Monro TM, Hutchinson MR. Portable optical fiber probe for in vivo brain temperature measurements. Biomed Opt Express. (2016) 7(8):3069–77. doi: 10.1364/BOE.7.003069
57. Sukstanskii AL, Yablonskiy DA. Theoretical model of temperature regulation in the brain during changes in functional activity. Proc Natl Acad Sci U S A. (2006) 103(32):12144–9. doi: 10.1073/pnas.0604376103
58. Yablonskiy DA, Ackerman JJ, Raichle ME. Coupling between changes in human brain temperature and oxidative metabolism during prolonged visual stimulation. Proc Natl Acad Sci U S A. (2000) 97(13):7603–8. doi: 10.1073/pnas.97.13.7603
59. Cady EB, Penrice J, Robertson NJ. Improved reproducibility of MRS regional brain thermometry by ‘amplitude-weighted combination’. NMR Biomed. (2011) 24(7):865–72. doi: 10.1002/nbm.1634
60. Zhu M, Bashir A, Ackerman JJ, Yablonskiy DA. Improved calibration technique for in vivo proton MRS thermometry for brain temperature measurement. Magn Reson Med. (2008) 60(3):536–41. doi: 10.1002/mrm.21699
61. Marshall I, Karaszewski B, Wardlaw JM, Cvoro V, Wartolowska K, Armitage PA, et al. Measurement of regional brain temperature using proton spectroscopic imaging: validation and application to acute ischemic stroke. Magn Reson Imaging. (2006) 24(6):699–706. doi: 10.1016/j.mri.2006.02.002
62. Weis J, Covaciu L, Rubertsson S, Allers M, Lunderquist A, Ahlström H. Noninvasive monitoring of brain temperature during mild hypothermia. Magn Reson Imaging. (2009) 27(7):923–32. doi: 10.1016/j.mri.2009.01.011
63. Kauppinen RA, Vidyasagar R, Childs C, Balanos GM, Hiltunen Y. Assessment of human brain temperature by 1H MRS during visual stimulation and hypercapnia. NMR Biomed. (2008) 21(4):388–95. doi: 10.1002/nbm.1204
64. Babourina-Brooks B, Wilson M, Arvanitis TN, Peet AC, Davies NP. MRS Water resonance frequency in childhood brain tumours: a novel potential biomarker of temperature and tumour environment. NMR Biomed. (2014) 27(10):1222–9. doi: 10.1002/nbm.3177
65. Sharma AA, Nenert R, Mueller C, Maudsley AA, Younger JW, Szaflarski JP. Repeatability and reproducibility of in-vivo brain temperature measurements. Front Hum Neurosci. (2020) 14:598435. doi: 10.3389/fnhum.2020.598435
66. Owji ZP, Gilbert G, Saint-Martin C, Wintermark P. Brain temperature is increased during the first days of life in asphyxiated newborns: developing brain injury despite hypothermia treatment. AJNR Am J Neuroradiol. (2017) 38(11):2180–6. doi: 10.3174/ajnr.A5350
67. Childs C, Hiltunen Y, Vidyasagar R, Kauppinen RA. Determination of regional brain temperature using proton magnetic resonance spectroscopy to assess brain-body temperature differences in healthy human subjects. Magn Reson Med. (2007) 57(1):59–66. doi: 10.1002/mrm.21100
68. Sun Z, Zhang J, Chen Y, Zhang Y, Zhang X, Guo H, et al. Differential temporal evolution patterns in brain temperature in different ischemic tissues in a monkey model of middle cerebral artery occlusion. J Biomed Biotechnol. (2012) 2012:980961. doi: 10.1155/2012/980961
69. Corbett RJ, Purdy PD, Laptook AR, Chaney C, Garcia D. Noninvasive measurement of brain temperature after stroke. AJNR Am J Neuroradiol. (1999) 20(10):1851–7.10588108
70. Maudsley AA, Goryawala MZ, Sheriff S. Effects of tissue susceptibility on brain temperature mapping. Neuroimage. (2017) 146:1093–101. doi: 10.1016/j.neuroimage.2016.09.062
71. Babourina-Brooks B, Simpson R, Arvanitis TN, Machin G, Peet AC, Davies NP. MRS Thermometry calibration at 3 T: effects of protein, ionic concentration and magnetic field strength. NMR Biomed. (2015) 28(7):792–800. doi: 10.1002/nbm.3303
72. Vescovo E, Levick A, Childs C, Machin G, Zhao S, Williams SR. High-precision calibration of MRS thermometry using validated temperature standards: effects of ionic strength and protein content on the calibration. NMR Biomed. (2013) 26(2):213–23. doi: 10.1002/nbm.2840
73. Verius M, Frank F, Gizewski E, Broessner G. Magnetic resonance spectroscopy thermometry at 3 tesla: importance of calibration measurements. Ther Hypothermia Temp Manag. (2019) 9(2):146–55. doi: 10.1089/ther.2018.0027
74. Hollis VS. Non-invasive monitoring of brain tissue temperature by near-infrared spectroscopy. United Kingdom: University of London, University College London (2002).
75. Langford VS, McKinley AJ, Quickenden TI. Temperature dependence of the visible-near-infrared sbsorption spectrum of liquid water. J Phys Chem A. (2001) 105:8916–21. doi: 10.1021/jp010093m
76. Bakhsheshi MF, Lee TY. Non-invasive monitoring of brain temperature by near-infrared spectroscopy. Temperature. (2014) 2(1):31–2. doi: 10.4161/23328940.2014.967156
77. Hollis VS, Binzoni T, Delpy DT. Non-invasive monitoring of brain tissue temperature by near-infrared spectroscopy. Proc. SPIE. (2001) 4250:470–81. doi: 10.1117/12.434506
78. Holper L, Mitra S, Bale G, Robertson N, Tachtsidis I. Prediction of brain tissue temperature using near-infrared spectroscopy. Neurophotonics. (2017) 4(2):021106. doi: 10.1117/1.NPh.4.2.021106
79. Chung SH, Cerussi AE, Klifa C, Baek HM, Birgul O, Gulsen G, et al. In vivo water state measurements in breast cancer using broadband diffuse optical spectroscopy. Phys Med Biol. (2008) 53(23):6713–27. doi: 10.1088/0031-9155/53/23/005
80. Chung SH, Cerussi AE, Merritt SI, Ruth J, Tromberg BJ. Non-invasive tissue temperature measurements based on quantitative diffuse optical spectroscopy (DOS) of water. Phys Med Biol. (2010) 55(13):3753–65. doi: 10.1088/0031-9155/55/13/012
81. Hammer SM, Hueber DM, Townsend DK, Huckaby LM, Alexander AM, Didier KD, et al. Effect of assuming constant tissue scattering on measured tissue oxygenation values during tissue ischemia and vascular reperfusion. J Appl Physiol. (2019) 127(1):22–30. doi: 10.1152/japplphysiol.01138.2018
82. Johnson LJ, Chung W, Hanley DF, Thakor NV. Optical scatter imaging detects mitochondrial swelling in living tissue slices. Neuroimage. (2002) 17(3):1649–57. doi: 10.1006/nimg.2002.1264
83. Okada E, Delpy DT. Near-infrared light propagation in an adult head model. II. Effect of Superficial Tissue Thickness on the Sensitivity of the Near-Infrared Spectroscopy Signal. Appl Opt. (2003) 42(16):2915–22. doi: 10.1364/ao.42.002915
84. Tomita M, Ohtomo M, Suzuki N. Contribution of the flow effect caused by shear-dependent RBC aggregation to NIR spectroscopic signals. Neuroimage. (2006) 33(1):1–10. doi: 10.1016/j.neuroimage.2006.05.042
85. Highton D, Tachtsidis I, Tucker A, Elwell C, Smith M. Near infrared light scattering changes following acute brain injury. Adv Exp Med Biol. (2016) 876:139–44. doi: 10.1007/978-1-4939-3023-4_17
86. Sato Y, Komuro Y, Lin L, Tang Z, Hu L, Kadowaki S, et al. Differences in tissue oxygenation, perfusion and optical properties in brain areas affected by stroke: a time-resolved NIRS study. Adv Exp Med Biol. (2018) 1072:63–7. doi: 10.1007/978-3-319-91287-5_11
87. Bakhsheshi MF, Diop M, St Lawrence K, Lee TY. Monitoring brain temperature by time-resolved near-infrared spectroscopy: pilot study. J Biomed Opt. (2014) 19(5):057005. doi: 10.1117/1.JBO.19.5.057005
88. Lange F, Tachtsidis I. Clinical brain monitoring with time domain NIRS: a review and future perspectives. Appl. Sci. (2019) 9:1612. doi: 10.3390/app9081612
89. Lange F, Dunne L, Hale L, Tachtsidis I. MAESTROS: a multiwavelength time-domain NIRS system to monitor changes in oxygenation and oxidation state of cytochrome-C-oxidase. IEEE J Sel Top Quantum Electron. (2018) 25(1):7100312. doi: 10.1109/JSTQE.2018.2833205
90. Sekar SKV, Dalla Mora A, Bargigia I, Martinenghi E, Lindner C, Farzam P, et al. Broadband (600–1350 nm) time-resolved diffuse optical spectrometer for clinical use. IEEE J. Sel. Top. Quantum Electron. (2016) 22:406–14. doi: 10.1109/JSTQE.2015.2506613
91. Kovacsova Z, Bale G, Mitra S, Lange F, Tachtsidis I. Absolute quantification of cerebral tissue oxygen saturation with multidistance broadband NIRS in the newborn brain. Biomed Opt Express. (2021) 12(2):907–25. doi: 10.1364/BOE.412088
92. Edwards AD, Yue X, Squier MV, Thoresen M, Cady EB, Penrice J, et al. Specific inhibition of apoptosis after cerebral hypoxia-ischaemia by moderate post-insult hypothermia. Biochem Biophys Res Commun. (1995) 217(3):1193–9. doi: 10.1006/bbrc.1995.2895
93. Thoresen M, Penrice J, Lorek A, Cady EB, Wylezinska M, Kirkbride V, et al. Mild hypothermia after severe transient hypoxia-ischemia ameliorates delayed cerebral energy failure in the newborn piglet. Pediatr Res. (1995) 37(5):667–70. doi: 10.1203/00006450-199505000-00019
94. Wassink G, Gunn ER, Drury PP, Bennet L, Gunn AJ. The mechanisms and treatment of asphyxial encephalopathy. Front Neurosci. (2014) 8:40. doi: 10.3389/fnins.2014.00040
95. Kerenyi A, Kelen D, Faulkner SD, Bainbridge A, Chandrasekaran M, Cady EB, et al. Systemic effects of whole-body cooling to 35 °C, 33.5 °C, and 30 °C in a piglet model of perinatal asphyxia: implications for therapeutic hypothermia. Pediatr Res. (2012) 71:573–82. doi: 10.1038/pr.2012.8
96. Yang XF, Chang JH, Rothman SM. Intracerebral temperature alterations associated with focal seizures. Epilepsy Res. (2002) 52(2):97–105. doi: 10.1016/S0920-1211(02)00193-6
97. Wu TW, Wisnowski J L, Geisler RF, Reitman A, Ho E, Tamrazi B, et al. An in vivo assessment of regional brain temperature during whole-body cooling for neonatal encephalopathy. J Pediatr. (2020) 220:73–79.e3. doi: 10.1016/j.jpeds.2020.01.019
98. Dehkharghani S, Fleischer CC, Qiu D, Yepes M, Tong F. Cerebral temperature dysregulation: MR thermographic monitoring in a nonhuman primate study of acute ischemic stroke. AJNR Am J Neuroradiol. (2017) 38:712–20. doi: 10.3174/ajnr.A5059
99. Smith CM, Adelson PD, Chang YF, Brown SD, Kochanek PM, Clark RS, et al. The brain-systemic temperature gradient is temperature-dependent in children with severe traumatic brain injury. Pediatr Crit Care Med. (2011) 12(4):449–54. doi: 10.1097/PCC.0b013e3181f390dd
100. Karaszewski B, Carpenter TK, Thomas RG, Armitage PA, Lymer GK, Marshall I, et al. Relationships between brain and body temperature, clinical and imaging outcomes after ischemic stroke. J Cereb Blood Flow Metab. (2013) 33(7):1083–9. doi: 10.1038/jcbfm.2013.52
101. Sacho RH, Vail A, Rainey T, King AT, Childs C. The effect of spontaneous alterations in brain temperature on outcome: a prospective observational cohort study in patients with severe traumatic brain injury. J Neurotrauma. (2010) 27:2157–64. doi: 10.1089/neu.2010.1384
102. Rossi S, Zanier E, Mauri I, Columbo A, Stocchetti N. Brain temperature, core temperature, and intracranial pressure in acute cerebral injury. J Neurol Neurosurg Psychiatry. (2001) 71:448–54. doi: 10.1136/jnnp.71.4.448
103. Yang XF, Rothman SM. Focal cooling rapidly terminates experimental neocortical seizures. Ann Neurol. (2001) 49(6):721–6. doi: 10.1002/ana.1021
Keywords: brain temperature, neonatal seizure, neonatal encephalopathy, hypoxic ischaemic encephalopathy, cerebral metabolism, cerebral perfusion, near infrared spectrscopy (NIRS), magnetic resonance spectroscopy (MRS)
Citation: Verma V, Lange F, Bainbridge A, Harvey-Jones K, Robertson NJ, Tachtsidis I and Mitra S (2022) Brain temperature monitoring in newborn infants: Current methodologies and prospects. Front. Pediatr. 10:1008539. doi: 10.3389/fped.2022.1008539
Received: 31 July 2022; Accepted: 15 September 2022;
Published: 4 October 2022.
Edited by:
Simone Pratesi, Careggi University Hospital, ItalyReviewed by:
Enrico Cocchi, Columbia University, United States© 2022 Verma, Lange, Bainbridge, Harvey-Jones, Robertson, Tachtsidis and Mitra. This is an open-access article distributed under the terms of the Creative Commons Attribution License (CC BY). The use, distribution or reproduction in other forums is permitted, provided the original author(s) and the copyright owner(s) are credited and that the original publication in this journal is cited, in accordance with accepted academic practice. No use, distribution or reproduction is permitted which does not comply with these terms.
*Correspondence: Subhabrata Mitra c3ViaGFicmF0YS5taXRyYS4xM0B1Y2wuYWMudWs=
Specialty Section: This article was submitted to Neonatology, a section of the journal Frontiers in Pediatrics
Disclaimer: All claims expressed in this article are solely those of the authors and do not necessarily represent those of their affiliated organizations, or those of the publisher, the editors and the reviewers. Any product that may be evaluated in this article or claim that may be made by its manufacturer is not guaranteed or endorsed by the publisher.
Research integrity at Frontiers
Learn more about the work of our research integrity team to safeguard the quality of each article we publish.