- 1Departments of Pediatrics, Hershey, PA, United States
- 2Obstetrics and Gynecology, The Pennsylvania State University College of Medicine, Hershey, PA, United States
Pulmonary surfactant proteins have many roles in surfactant- related functions and innate immunity. One of these proteins is the surfactant protein A (SP-A) that plays a role in both surfactant-related processes and host defense and is the focus in this review. SP-A interacts with the sentinel host defense cell in the alveolus, the alveolar macrophage (AM), to modulate its function and expression profile under various conditions, as well as other alveolar epithelial cells such as the Type II cell. Via these interactions, SP-A has an impact on the alveolar microenvironment. SP-A is also important for surfactant structure and function. Much of what is understood of the function of SP-A and its various roles in lung health has been learned from SP-A knockout (KO) mouse experiments, as reviewed here. A vast majority of this work has been done with infection models that are bacterial, viral, and fungal in nature. Other models have also been used, including those of bleomycin-induced lung injury and ozone-induced oxidative stress either alone or in combination with an infectious agent, bone marrow transplantation, and other. In addition, models investigating the effects of SP-A on surfactant components or surfactant structure have contributed important information. SP-A also appears to play a role in pathways involved in sex differences in response to infection and/or oxidative stress, as well as at baseline conditions. To date, this is the first review to provide a comprehensive report of the functions of SP-A as learned through KO mice.
Introduction
Pulmonary surfactant has many critical roles in respiratory function. One is to decrease surface tension at the air-liquid interface, preventing alveolar lung collapse at low lung volumes. The administration of exogenous surfactant containing surfactant proteins B and C (SP-B and SP-C) is used routinely to treat prematurely born infants at risk for respiratory distress syndrome (RDS) (1). Pulmonary surfactant is a lipoprotein complex consisting of lipids, primarily phospholipids, and several proteins, SP-A, SP-B, SP-C and SP-D (the latter co-isolates with surfactant). These proteins collectively play various roles in surfactant-related functions and innate immunity/host defense (2). The focus of this review is on SP-A actions as learned from studies of SP-A knockout (KO) mice.
SP-A has been shown to be involved in surfactant-related function or structure and in the regulation of inflammatory processes and innate host defense. SP-A interacts in the alveolar space with the sentinel resident cell in alveolar host defense, the alveolar macrophage (AM). Via its interaction with the AM, SP-A affects the status and functions of AM under baseline conditions or in response to various insults. In addition, SP-A either via its interaction with the AM or other cells in the alveolus, imparts changes in the alveolar microenvironment where the AM resides that, in turn, may have an impact on AM baseline status and its overall activity in response to various insults (2).
Humans have two SFTPA genes and these have been identified with extensive genetic and epigenetic variability and human SP-A protein variants have been shown to differentially affect AM and alveolar epithelial type II cells, as well as the alveolar microenvironment. These differential effects have been observed both under baseline conditions and in response to various insults, as assessed by studies of bronchoalveolar lavage samples, alveolar cells, or other. However, the differential effects of the human SP-A protein variants have been reviewed recently elsewhere and will not be discussed here (2). The specific focus of this review is on knowledge gained, with regards to SP-A function, via comparison studies of SP-A KO and wild type (WT) mice or KO mice expressing an SP-A transgene. The direct and indirect roles of SP-A in combating various bacterial and viral pathogens or other types of insults, as well as the various SP-A-mediated activities that may affect surfactant structure, or activities of various surfactant components are noted.
Bacterial Pathogens
Following the generation of SP-A KO mice via gene targeting techniques, the initial studies showed that these mice survived and bred normally under controlled housing conditions. Lung morphology and characteristics of surfactant and its components largely were not much different from WT mice, other than a scarcity of tubular myelin, an extracellular structural form of surfactant, and the observation that higher minimum surface tension was observed at low surfactant concentrations in KO (3). However, bacterial clearance in the KO was less efficient, pointing to a role for SP-A in innate immunity (4). Since then, infection studies with a multitude of bacterial strains have been examined to gain insight into the role of SP-A in host defense. The evidence from these studies indicates that the involvement of SP-A has a positive role in innate immune response.
The alveolar macrophage serves as the primary phagocyte of the innate immune system in the lung. Many of the interactions of SP-A involve this important population of cells. In a study of Staphylococcus aureus infection, SP-A has been found to bind to the C1q receptor on monocytes (5), as well as to interact with SP-R210 on AM (6). SP-A-opsonized bacteria are shown to lead to macrophage activation and secretion of tumor necrosis factor (TNF)-alpha (5, 6). Moreover, the S. aureus extracellular adherence protein (Eap) is a critical protein ligand for SP-A-mediated binding to S. aureus and necessary for the in vivo clearance of acute S. aureus infection (7). Veith et al. showed that in an S. aureus infection model, SP-A KO mice compared to WT showed a significantly lower number of phagocytes, mostly AM, filled with staphylococci at early post-infection intervals (8).
In infection with Escherichia coli, there has been evidence that SP-A enhances the binding and deacylation of E. coli lipopolysaccharide (LPS) by AM (9). In an infection model with non-typeable Haemophilus influenzae or Group B streptococcus infection, SP-A KO mice, in comparison to WT, showed a decrease in bacterial clearance, decreased association and phagocytosis of bacteria, as well as decreased superoxide and hydrogen peroxide production by AM. Increased lung pathology and increased total cells and polymorphonuclear neutrophils (PMNs) in bronchoalveolar lavage (BAL) were observed as well as an increase in levels of pro-inflammatory cytokines, TNF-α, interleukin 1 beta (IL-1β), interleukin 6 (IL-6), and macrophage inflammatory protein (MIP)-2 in lung homogenates (10). LeVine et al. had also conducted prior studies looking at a Group B streptococcus infection model. In one study, SP-A KO mice compared to WT showed an increase in pulmonary infiltration at 6 and 24 h post-infection, increased number of bacteria in lung homogenates, increased dissemination of bacteria to the spleen, and decreased association of bacteria with AMs (11). Another study by this group showed that in SP-A KO mice compared to WT, there was a decreased phagocytosis of bacteria by macrophages, decreased superoxide production in BAL, and rescue by exogenous SP-A increased clearance of bacteria (12).
In a Pseudomonas aeruginosa infection model, in SP-A KO mice compared to WT, there was a decreased clearance of bacteria, increased lung inflammation and bacterial burden in lungs, and decreased phagocytosis of bacteria by AMs (13, 14). In one study the SPA-4 peptide, derived from the C-terminal TLR-4 interacting region of SP-A, was administered in P. aeruginosa infected mice, and the pro-phagocytic and anti-inflammatory activity of the SPA-4 peptide led to reduced bacterial burden and decreased levels of cytokines and chemokines (15).
Other data indicate a large impact on the overall host defense system by SP-A. Ali et al. showed in their K. pneumoniae infection model that in the SP-A KO mice compared to WT ~75% of 32 host defense proteins were lower in BAL of uninfected mice, indicating that the uninfected mice hold the potential for increased susceptibility to infection. However, higher levels of more than two-thirds of the identified proteins at 4 h post-infection were observed in KO vs. WT, which was almost the exact opposite of the untreated mice (16), indicating, perhaps, an attempt by the KO to compensate for its baseline deficits. However, in terms of the significant changes in protein levels, more significant changes were observed in WT infected compared to KO infected. In an otitis media model of infection with non-typeable Haemophilus influenzae, SP-A was shown to modulate the expression of pro-inflammatory markers, IL-6 and IL-1β, and these peaked at higher levels in SP-A KO mice (17).
Sex Differences at Baseline Conditions and in Response to Infection and/or Other Stimuli
Several models have been used to investigate sex differences at baseline conditions and in response to bacterial infection and/or other stimuli in the presence or absence of SP-A. Mikerov et al. (18) designed a number of studies using a model that involved exposure to filtered air or ozone prior to Klebsiella pneumoniae infection. In one study, there was an increased susceptibility to infection as depicted by decreased animal survival and phagocytic index of AMs in SP-A KO mice compared to WT. Although infected females showed a better survival than males, if the animals were exposed to ozone prior to infection females were more affected than males in both KO and WT mice (18). This was further described in a subsequent study where, in SP-A KO mice compared to WT, there was a decreased clearance of bacteria from the lung. Ozone-exposed females were more affected than males; however, in the absence of ozone-induced oxidative stress, males were more predisposed to have a higher level of dissemination of infection compared to females (19). In another study, via the use of a multianalyte immunoassay that measured 59 different cytokines, chemokines and other proteins, Mikerov et al. showed that wild type male mice exhibited a more exuberant response to infection alone and females exhibited a more robust response to infection with prior ozone exposure (20). Sex differences have been observed by histopathologic evaluation of lung and extrapulmonary tissues. In response to K. pneumoniae infection, more pronounced lesions were observed in extrapulmonary tissues (liver and spleen) in males compared to females. But if infection was preceded by ozone exposure (an oxidative stress), females exhibited a more excessive inflammatory response in lung than males (21). Sex differences in the AM proteome of KO and WT mice at baseline conditions (22, 23) showed that proteins such as the major vault protein, chaperonin subunit β CCT2 and Rho GDPα dissociation inhibitor, shown to interact with the estrogen receptor (24–26), were increased in females (22, 23).
Sex differences also have been observed after K. pneumoniae infection in gene expression in AM from KO and SP-A KO mice expressing an SP-A transgene (27). In the same type of mice (i.e., KO expressing an SP-A transgene), sex differences were observed: (1) in the AM (28–30) or type II epithelial cell (31) miRNome and in the miRNA-targeted genes after ozone exposure; (2) in AM cytoskeleton and cell morphology (32); (3) in the AM KO proteome after rescue with human SP-A variants at baseline conditions (33); and (4) in the BAL proteome in response to insults (34). A recent study by Xu et al. showed that AM from KO mice are more oxidized than AM from KO mice expressing an SP-A transgene after ozone exposure, as assessed by optical redox imaging analysis (35). No redox sex-dependent differences were observed in SP-A KO mice after ozone exposure, but in the presence of the SP-A transgene sex-specific differences were observed in the redox status with males exhibiting a higher mitochondrial reactive oxygen species (ROS) level (35). Furthermore, in a K. pneumoniae model, with or without subsequent methacholine, sex differences were observed in KO in certain airway function readouts (36). These together implicate sex as a variable and potentially a role for sex hormones.
Durrani et al. showed that gonadectomy of WT mice eliminated (females) or minimized (males) sex differences in survival after infection with or without ozone-exposure prior to infection. Moreover, sex-hormone treatment of gonadectomized mice resulted in a survival pattern similar to that of the non-gonadectomized WT (37). Gandhi et al. (38) explored in SP-A KO mice, the impact of ozone, sex, and gonadal hormones on BAL characteristics in infection with K. pneumoniae. In the infected KO mice, no sex differences were observed and after prior ozone exposure only limited sex differences at the later time points were observed in BAL parameters compared to WT under identical conditions. Furthermore, survival experiments with gonadectomized infected SP-A KO mice with or without prior ozone exposure showed no sex-specificity (38). This indicates that in the absence of SP-A and sex hormones, different mechanisms may be operative than those in WT mice that bring about sex-specific survival (18, 37). Of interest, in a model of K. pneumoniae infection, SP-A was found to bind to the Man α1 man sequence in the capsular polysaccharide of the K21a serotype of K. pneumoniae (39); this may be an important SP-A-mediated step in the process of providing host defense against K. pneumoniae infection.
Viral Pathogens
Along with the extensive study of bacterial pathogens and SP-A, there have been numerous studies that investigated interactions of viral pathogens and SP-A. Distinct interactions have been identified with the Respiratory Syncytial Virus (RSV) F protein and SP-A (40). In an RSV infection model, SP-A KO mice compared to WT mice showed an increase in pulmonary infiltration, particularly PMNs after infection, an increased RSV burden in lung homogenates, an increase in the levels of pro-inflammatory cytokines, such as TNF-α and IL-6, and decreased superoxide and hydrogen peroxide generation by AMs. However, SP-A KO mice rescued with exogenous SP-A reduced viral titers and inflammatory cells in the lung (41). In an infection model with adenovirus, the SP-A KO mice compared to WT, showed a decreased clearance of adenovirus, increased lung inflammation and cytokines, and inflammatory cells in BAL, and a decreased uptake of adenovirus by AMs. Rescue by exogenous SP-A improved viral clearance and decreased inflammation and neutrophils in BAL (42).
Numerous studies have investigated interactions of SP-A with Influenza A virus (IAV). LeVine et al. showed that in SP-A KO mice compared to WT, there was a decreased clearance of IAV and an increase in pulmonary inflammation, whereas there was an increased viral clearance and decreased lung inflammation in exogenous SP-A-rescued KO mice. In addition, the SP-A-rescued mice showed a decreased myeloperoxidase activity in isolated PMNs from BAL, increased B and activated T lymphocytes in the lung and spleen, increased T helper (Th) 1 responses [interferon-γ, interleukin (IL)-2, and IgG2a] and decreased Th2 responses (IL-4, IL-10, and IgG1) in the lungs, 7 days after infection (43). Another study by Li et al. showed that in SP-A KO mice compared to WT after infection with a β-resistant strain of Influenza A virus, there was decreased survival and the mice had exaggerated early inflammation with increased MIP-2 protein levels and a greater influx of neutrophils (44). Hawgood et al. showed that rescue of SP-A KO mice with SP-A partially neutralized influenza A infection (39% neutralization) (45). A proposed mechanism for preventing viral dissemination was described for the influenza A infected mice. This involved binding of the sialylated asparagine 187 residue of SP-A to hemagglutinin that blocked access of neuraminidase to surface bound substrates (43). Furthermore, Watson et al. has reviewed the structural similarity of trimeric collectins of SP-A and SP-D with trimeric viral fusion proteins of RSV, IAV, and HIV and noted their therapeutic potential against infectious and inflammatory diseases (46).
Other Infectious Agents
In an allergy model, following exposure to Aspergillus fumigatus antigen, it was shown that rescue of SP-A KO mice with SP-A, compared to KO controls, led to a decrease in peripheral eosinophilia and eosinophil peroxidase (EPO) activity and a decrease in IL-5, IL-2, and IL-10 on day 10. The ratio of interferon (IFN)-γ to IL-4 did not change significantly, but a decreased infiltration of eosinophils on lung histopathology on both days 4 and 10 after exposure was observed (47). Via the use of SP-A and inducible nitric oxide synthase (iNOS) double knockout mice it has been shown that lung injury and surfactant abnormalities observed after mycoplasma infection depend, in part, on these two molecules, SP-A and iNOS (48). Furthermore, a model with Mycoplasma pneumoniae infection showed SP-A to specifically bind the lipid A moiety of rough lipopolysaccharides desaturated phosphatidyl glycerol on the surface of M. pneumoniae (49). Another group demonstrated that mice lacking SP-A have increased airway hyperresponsiveness during M. pneumoniae infection vs. WT mice through TNF- α (50) or compared to KO expressing a specific human SP-A transgene (51). Furthermore, with this model it was shown that TNF-α activation of mast cells (MC) through the TNF receptor, but not the MC-derived TNF-α, lead to augmented airway hyperresponsiveness during M. pneumoniae infection when SP-A is absent. Moreover, M. pneumoniae infected KO mice engrafted with TNF-α negative or TNF receptor negative MC have decreased mucus production compared to mice with WT MCs. This work indicates a potential role of mast cells as secondary responders to TNF-α in host defense (52).
In an infection model with Pneumocystis carinii, SP-A-deficient mice compared to WT showed more severe infection and had an attenuated production of pro-inflammatory cytokines and reactive oxygen-nitrogen species (53). In a model of infection with Pneumocystis murina, although infection increased in both SP-A KO and WT mice over the study period, the overall intensity of the infection was more severe in SP-A KO mice indicating a protective role of SP-A (54). Both KO and WT cleared infection similarly but there were noted to be higher percentages of lymphocytes in SP-A KO mice and lower levels of IL-6 compared to WT, indicating that SP-A may modulate the immune response in this model (55).
Surfactant-Related Activities
As a way of brief background, the alveolus, which is the distal lung air space, is lined by epithelial cells, the Type II and Type I cells. The former are the site for the production of surfactant; surfactant is essential in the prevention of alveolar lung collapse, and via this function enables the lung to carry out its vital function of O2/CO2 exchange. The latter, the Type I cells, are responsible for the O2/CO2 exchange, which is the key function of the lung and essential for life. The alveolar space is covered by a thin liquid layer, called hypophase, and the functioning surfactant monolayer is found at the air liquid interface. Under normal conditions the only immune cell found in the hypophase is the alveolar macrophage. Also, various morphological structures of surfactant such as tubular myelin, lamellar bodies and other, as well as proteins and other are found in the hypophase. A diagrammatic presentation of this is shown below in Figure 1C.
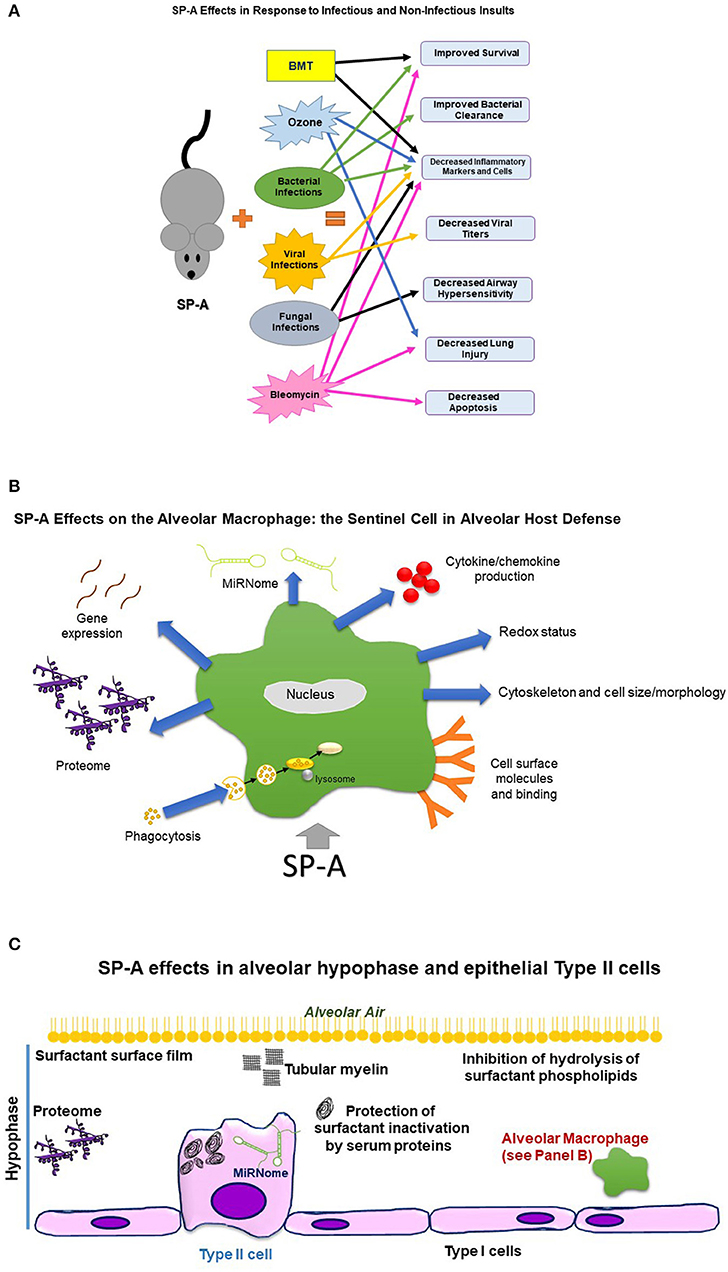
Figure 1. This figure depicts diagrammatically effects of SP-A on the organism, alveolar macrophage, and alveolar hypophase, as assessed by comparison of SP-A KO mice to WT or SP-A KO carrying a human SP-A transgene. (A) Depicts effects of SP-A on the organism in response to infectious and non-infectious insults. BMT, Bone marrow transplantation. Sex differences were observed in survival after bacterial infection, clearance of bacteria, dissemination of infection, robustness of response to infection, extrapulmonary lesions, and airway function readouts. SP-A in response to double insults, such as infection and ozone exposure, improved survival. (B) Depicts effects of SP-A on the alveolar macrophage, the sentinel alveolar host defense cell. Sex differences were observed in proteome, gene expression, miRNome, cytoskeleton, and redox status. (C) Depicts SP-A effects in the alveolar hypophase and epithelial Type II cells. Sex differences were observed in the miRNome of the epithelial Type II cells.
The effect of SP-A on various surfactant components has been evaluated in SP-A KO mice compared to WT. Alveolar and lung tissue saturated phosphatidylcholine pools were higher in KO, but the clearance of radiolabeled DPPC or SP-B from the air spaces after intratracheal injection of radiolabeled palmitic acid and choline was similar (56). Moreover, SP-A has been shown to be a necessary component for the formation of tubular myelin, an extracellular structural form of surfactant. KO mice lack tubular myelin (57, 58). However, if SP-A lacks its collagen-like region, tubular myelin is not restored in the KO mice, indicating a role for this SP-A region in tubular myelin formation. In addition, the absence of the SP-A collagen-like region results in functional defects of surfactant (59). Rescue of SP-A KO mice with surfactant from human bronchoalveolar lavage containing either both human SP-As (57) or expression of rat SP-A in Clara cells and alveolar type II cells of the SP-A KO mouse (59) restored tubular myelin forms.
Surfactant from KO mice exhibited a number of differences in its characteristics from that derived from wild type mice. These included, among others, a lower percentage of large surfactant aggregates (LA) and with cycling an increased conversion of LA (the biophysically active form of surfactant) to small vesicular forms, increased sensitivity to inactivation by serum proteins as assessed by measurements of minimum surface tension, surfactant with lower buoyant density, and other (58). Although it was previously reported that no differences between WT and KO were observed in the amount of LA (3), different methods were used. However, alterations in surfactant characteristics in KO mice compared to WT mice didn't seem to result in functional deficits, as assessed by exercise tolerance with swimming and running, and a 4-day period of hyperoxia did not affect survival and pressure-volume curves (60).
SP-A has been shown in in vitro studies to suppress synthesis of type IIA secreted phospholipases A2 (sPLA2-IIA) (61) as well as inhibit hydrolysis of surfactant phospholipids (62). The latter was shown to be the case in vivo following administration of sPLA2-IIA in mice. The SP-A KO mice exhibited a significantly higher level of surfactant phospholipid hydrolysis, indicating an SP-A inhibitory effect on this process and a potential role for SP-A in acute respiratory distress syndrome (ARDS), as changes in surfactant phospholipids become evident early in the course of ARDS. SP-A, via its ability to inhibit surfactant phospholipid hydrolysis and thus maintain, to a certain extent a well-functioning surfactant, plays a protective role in lung injury. This is of great relevance to the current Covid-19 pandemic, as sPLA2-IIA was shown to associate with markers more likely to lead to mortality after SARS-CoV-2 infection (63).
Other Models
In a bleomycin-induced acute lung injury model, SP-A KO mice compared to WT, showed decreased survival, increased cytokine inflammatory response, lung edema, apoptosis, and lung injury. These observations indicate a role for SP-A in the regulation of inflammation, lung epithelial cell integrity, and apoptosis after non-infectious insults. Moreover, rescue with SP-A of SP-A KO bleomycin-treated mice improved survival to the level seen in WT (64).
In an ozone-induced oxidative stress model, SP-A KO mice compared to WT showed a larger increase in BAL total protein and an increase in LDH activity and phospholipid content. In WT mice there were more BAL PMNs and elevated MIP-2 and monocyte chemoattractant protein (MCP)-1 (65). In a model where exposure to E. coli-derived LPS combined with oxidative stress was studied, SP-A KO mice showed, in BAL, a lower number of cells at exposure to 0.5 ng of LPS but not at 2 ng of LPS, compared to WT, indicating an inability of KO to respond to low levels of LPS, but this inability was overcome in the face of a more severe insult (i.e., 2 ng LPS). After LPS and ozone exposure, KO showed a decrease in PMN recruitment, a decrease in MIP-2 levels, and an increase in phospholipids (66).
SP-A has also been shown to play a role in lung inflammation in a murine allogeneic bone marrow transplantation (BMT) model. SP-A KO mice after BMT showed increased inflammation along with decreased dynamic lung compliance and donor T-cell dependent mortality. However, treatment with SP-A improved survival and decreased inflammation in the BMT model (67). Furthermore, via the use of KO mice it was shown that SP-A is necessary for the protective effects of keratinocyte growth factor in terms of its ability to attenuate inflammation after BMT (68).
We postulate that SP-A functions by enhancing the efficiency of the alveolar macrophage in removing harmful substances or damaged cells that serve as pro-inflammatory stimuli. In the absence of SP-A these materials are not as readily cleared or neutralized, allowing these stimuli to persist and thus increasing the degree of inflammation. Thus, we contend that SP-A is not in itself anti-inflammatory, but its actions result in enhanced host defense function that ultimately lessens inflammation.
Translational Work
A summary including findings related to SP-A in human health and disease have been previously published (69). Alterations in levels of SP-A have been reported in many acute and chronic lung conditions. In diseases in adults, changes in SP-A levels have been observed, among others, in acute respiratory distress syndrome (ARDS) (70, 71), asthma (72), chronic obstructive pulmonary disease (COPD) (73), idiopathic pulmonary fibrosis (74, 75), sarcoidosis and hypersensitivity pneumonitis (75, 76), and poor outcome and early lung transplant survival (77, 78). In neonatal populations altered SP-A levels have been observed in bronchopulmonary dysplasia (BPD) (79), children with cystic fibrosis (CF) (80), infants dying from respiratory distress syndrome (RDS) (81, 82) or severity of RDS (83). These observations have led to consideration in some cases for use of SP-A as a biomarker in clinical settings. SP-A has also been found in amniotic fluid, increasing in level from 32 weeks of gestation to term (84) and then decreasing at the time of labor (85). SP-A has a postulated role in clearance of pathogens from the amniotic fluid that is part of the proposed mechanism for the increased risk for infections at preterm gestational ages (84). It has also been proposed that SP-A secreted from the mouse fetal lung serves as a hormone in parturition, and experimental evidence supports an SP-A role in the initiation of parturition as well as a delay in its absence (86). There is also further mouse evidence that the complex cascade of interactions to signal parturition may involve SP-A and platelet activating factor (PAF) (87–89). In humans, SP-A was also shown to move from the placental amnion to the reflected amnion, indicating that SP-A may play a role in protecting the integrity of the amnion and the amniotic cavity from pro-inflammatory stimuli during pregnancy (90). There is also work to indicate a potential role of SP-A in preterm birth (91). These findings highlight the key roles SP-A plays in health and disease, as well as opportunities for continuation of work to translate findings from KO mice to the human.
Summary and Comments
Research involving SP-A continues to reveal an increasing level of evidence regarding its critical role in host defense, surfactant-related functions, and other. Much has been learned by the study of SP-A KO mice. The effects of SP-A on the organism, alveolar macrophage and alveolar hypophase are diagrammatically depicted in Figures 1A–C, respectively. In general, SP-A KO mice are more susceptible to infection, whether bacterial or viral, as well as in response to other infectious and non-infectious insults. In addition, there is pathologic evidence of impaired defenses and ability to clear infection. Rescue of SP-A KO mice with exogenous SP-A has resulted in significantly improved survival and improved levels of markers of host defense or inflammation, underscoring further the importance of SP-A in host defense.
Although surfactant replacement therapy is used rather routinely in the clinical setting to treat prematurely born infants with respiratory distress syndrome, none of the widely used surfactant replacement preparations contains SP-A (1). Of interest, a major complication in prematurely born infants is infection. SP-A in animal studies is shown to be protective after infection as assessed by survival studies (18, 37, 38, 44, 92). Moreover, current experience indicates that surfactant treatment of babies with “simple” RDS or with infection is less efficacious (93, 94) or conclusions could not be reached for near-term or term infants with true or suspected bacterial infection (95). Thus, inclusion of SP-A in surfactant preparations (96) or usage by itself to treat not only premature infants, but individuals with a variety of conditions as suggested by the animal studies reviewed here warrants consideration. Moreover, the potential use of SP-A or functional fragments of SP-A for therapy (46, 72, 97) as it may relate to the current Covid-19 pandemic may be a plausible consideration. Potential scenarios for the role of SP-A in Covid-19 have been discussed elsewhere (35, 98, 99). Finally, sex differences, as observed in certain models, as well as the role of sex hormones in sex-specific outcomes, point to a continued need for further research and attention to the role of sex in study design and therapies.
Author Contributions
LD reviewed the literature and wrote much of the manuscript. DP reviewed the literature and wrote parts of the manuscript. JF planned and oversaw the entire review and contributed to all aspects of the manuscript. All authors contributed to the article and approved the submitted version.
Funding
This work was funded in part by the Evan Pugh Fund, the George Pedlow Fund, the John Ardell Pursley Memorial Research Fund, and the Center for Host Defense, Inflammation and Lung Disease (CHILD) Fund, Department of Pediatrics, Penn State College of Medicine.
Conflict of Interest
The authors declare that the research was conducted in the absence of any commercial or financial relationships that could be construed as a potential conflict of interest.
Publisher's Note
All claims expressed in this article are solely those of the authors and do not necessarily represent those of their affiliated organizations, or those of the publisher, the editors and the reviewers. Any product that may be evaluated in this article, or claim that may be made by its manufacturer, is not guaranteed or endorsed by the publisher.
Abbreviations
AM, alveolar macrophage; ARDS, acute respiratory distress syndrome; BAL, bronchoalveolar lavage; BMT, bone marrow transplantation; KO, knockout; IAV, influenza A virus; IFN, interferon; IL, interleukin; iNOS, inducible nitric oxide synthase; LA, large aggregate; LPS, lipopolysaccharide; MC, mast cell; MIP, macrophage inflammatory protein; PMN, polymorphonuclear leukocyte; RDS, respiratory distress syndrome; ROS, reactive oxygen species; RSV, respiratory syncytial virus; SP-A, -B, -C, -D, surfactant protein-A, -B, -C, -D; sPLA2, secreted Phospholipases A2; TNF, tumor necrosis factor; TLR, Toll-like receptor; WT, wild type.
References
1. Robertson B, Halliday HL. Principles of surfactant replacement. Biochim Biophys Acta. (1998) 1408:346–61. doi: 10.1016/S0925-4439(98)00080-5
2. Floros J, Thorenoor N, Tsotakos N, Phelps DS. Human surfactant protein SP-A1 and SP-A2 variants differentially affect the alveolar microenvironment, surfactant structure, regulation and function of the alveolar macrophage, and animal and human survival under various conditions. Front Immunol. (2021) 12:2889. doi: 10.3389/fimmu.2021.681639
3. Korfhagen TR, Bruno MD, Ross GF, Huelsman KM, Ikegami M, Jobe AH, et al. Altered surfactant function and structure in SP-A gene targeted mice. Proc Natl Acad Sci USA. (1996) 93:9594–9. doi: 10.1073/pnas.93.18.9594
4. Korfhagen TR, LeVine AM, Whitsett JA. Surfactant protein A (SP-A) gene targeted mice. Biochim Biophys Acta. (1998) 1408:296–302. doi: 10.1016/S0925-4439(98)00075-1
5. Geertsma MF, Nibbering PH, Haagsman HP, Daha MR, van FR. Binding of surfactant protein A to C1q receptors mediates phagocytosis of staphylococcus aureus by monocytes. Am J Physiol. (1994) 267:L578–84. doi: 10.1152/ajplung.1994.267.5.L578
6. Sever-Chroneos Z, Krupa A, Davis J, Hasan M, Yang CH, Szeliga J, et al. Surfactant protein A (SP-A)-mediated clearance of staphylococcus aureus involves binding of SP-A to the staphylococcal adhesin eap and the macrophage receptors SP-A receptor 210 and scavenger receptor class A. J Biol Chem. (2011) 286:4854–70. doi: 10.1074/jbc.M110.125567
7. van de Wetering JK, van EM, van Golde LM, Hartung T, van Strijp JA, Batenburg JJ. Characteristics of surfactant protein A and D binding to lipoteichoic acid and peptidoglycan, 2 major cell wall components of gram-positive bacteria. J Infect Dis. (2001) 184:1143–51. doi: 10.1086/323746
8. Veith NT, Tschernig T, Gutbier B, Witzenrath M, Meier C, Menger M, et al. Surfactant protein A mediates pulmonary clearance of staphylococcus aureus. Respir Res. (2014) 15:85. doi: 10.1186/s12931-014-0085-2
9. Stamme C, Wright JR. Surfactant protein A enhances the binding and deacylation of E. coli LPS by alveolar macrophages. Am J Physiol. (1999) 276:L540–7. doi: 10.1152/ajplung.1999.276.3.L540
10. LeVine AM, Whitsett JA, Gwozdz JA, Richardson TR, Fisher JH, Burhans MS, et al. Distinct effects of surfactant protein A or D deficiency during bacterial infection on the lung. J Immunol. (2000) 165:3934–40. doi: 10.4049/jimmunol.165.7.3934
11. LeVine AM, Bruno MD, Huelsman KM, Ross GF, Whitsett JA, Korfhagen TR. Surfactant protein A-deficient mice are susceptible to group B streptococcal infection. J Immunol. (1997) 158:4336–40.
12. LeVine AM, Kurak KE, Wright JR, Watford WT, Bruno MD, Ross GF, et al. Surfactant protein-A binds group B streptococcus enhancing phagocytosis and clearance from lungs of surfactant protein-A-deficient mice. Am J Respir Cell Mol Biol. (1999) 20:279–86. doi: 10.1165/ajrcmb.20.2.3303
13. LeVine AM, Kurak KE, Bruno MD, Stark JM, Whitsett JA, Korfhagen TR. Surfactant protein-A-deficient mice are susceptible to pseudomonas aeruginosa infection. Am J Respir Cell Mol Biol. (1998) 19:700–8. doi: 10.1165/ajrcmb.19.4.3254
14. Giannoni E, Sawa T, Allen L, Wiener-Kronish J, Hawgood S. Surfactant proteins A and D enhance pulmonary clearance of pseudomonas aeruginosa. Am J Respir Cell Mol Biol. (2006) 34:704–10. doi: 10.1165/rcmb.2005-0461OC
15. Awasthi S, Singh B, Ramani V, Xie J, Kosanke S. TLR4-interacting SPA4 peptide improves host defense and alleviates tissue injury in a mouse model of pseudomonas aeruginosa lung infection. PLoS ONE. (2019) 14:e0210979. doi: 10.1371/journal.pone.0210979
16. Ali M, Umstead TM, Haque R, Mikerov AN, Freeman WM, Floros J, et al. Differences in the BAL proteome after Klebsiella pneumoniae infection in wild type and SP-A-/- mice. Proteome Sci. (2010) 8:34. doi: 10.1186/1477-5956-8-34
17. Abdel-Razek O, Ni L, Yang F, Wang G. Innate immunity of surfactant protein A in experimental otitis media. Innate Immun. (2019) 25:391–400. doi: 10.1177/1753425919866006
18. Mikerov AN, Gan X, Umstead TM, Miller L, Chinchilli VM, Phelps DS, et al. Sex differences in the impact of ozone on survival and alveolar macrophage function of mice after Klebsiella pneumoniae infection. Respir Res. (2008) 9:24. doi: 10.1186/1465-9921-9-24
19. Mikerov AN, Hu S, Durrani F, Gan X, Wang G, Umstead TM, et al. Impact of sex and ozone exposure on the course of pneumonia in wild type and SP-A (-/-) mice. Microb Pathog. (2012) 52:239–49. doi: 10.1016/j.micpath.2012.01.005
20. Mikerov AN, Phelps DS, Gan X, Umstead TM, Haque R, Wang G, et al. Effect of ozone exposure and infection on bronchoalveolar lavage: sex differences in response patterns. Toxicol Lett. (2014) 230:333–44. doi: 10.1016/j.toxlet.2014.04.008
21. Mikerov AN, Cooper TK, Wang G, Hu S, Umstead TM, Phelps DS, et al. Histopathologic evaluation of lung and extrapulmonary tissues show sex differences in Klebsiella pneumoniae - infected mice under different exposure conditions. Int J Physiol Pathophysiol Pharmacol. (2011) 3:176–90.
22. Phelps DS, Umstead TM, Quintero OA, Yengo CM, Floros J. In vivo rescue of alveolar macrophages from SP-A knockout mice with exogenous SP-A nearly restores a wild type intracellular proteome; actin involvement. Proteome Sci. (2011) 9:67. doi: 10.1186/1477-5956-9-67
23. Phelps DS, Umstead TM, Floros J. Sex differences in the response of the alveolar macrophage proteome to treatment with exogenous surfactant protein-A. Proteome Sci. (2012) 10:44. doi: 10.1186/1477-5956-10-44
24. Abbondanza C, Rossi V, Roscigno A, Gallo L, Belsito A, Piluso G, et al. Interaction of vault particles with estrogen receptor in the MCF-7 breast cancer cell. J Cell Biol. (1998) 141:1301–10. doi: 10.1083/jcb.141.6.1301
25. El MS, Schultz-Norton JR, Likhite VS, McLeod IX, Yates JR, Nardulli AM. Rho GDP dissociation inhibitor alpha interacts with estrogen receptor alpha and influences estrogen responsiveness. J Mol Endocrinol. (2007) 39:249–59. doi: 10.1677/JME-07-0055
26. Klimczak M, Biecek P, Zylicz A, Zylicz M. Heat shock proteins create a signature to predict the clinical outcome in breast cancer. Sci Rep. (2019) 9:7507. doi: 10.1038/s41598-019-43556-1
27. Thorenoor N, Kawasawa YI, Gandhi CK, Floros J. Sex-specific regulation of gene expression networks by surfactant protein A (SP-A) variants in alveolar macrophages in response to klebsiella pneumoniae. Front Immunol. (2020) 11:1290. doi: 10.3389/fimmu.2020.01290
28. Noutsios GT, Thorenoor N, Zhang X, Phelps DS, Umstead TM, Durrani F, et al. SP-A2 contributes to miRNA-mediated sex differences in response to oxidative stress: pro-inflammatory, anti-apoptotic, and anti-oxidant pathways are involved. Biol Sex Differ. (2017) 8:37. doi: 10.1186/s13293-017-0158-2
29. Thorenoor N, Kawasawa YI, Gandhi CK, Zhang X, Floros J. Differential impact of co-expressed SP-A1/SP-A2 protein on AM miRNome; sex differences. Front Immunol. (2019) 10:1960. doi: 10.3389/fimmu.2019.01960
30. Thorenoor N, Phelps DS, Floros J. Differential sex-dependent regulation of the alveolar macrophage miRNome of SP-A2 and co-ex (SP-A1/SP-A2) and sex differences attenuation after 18 h of ozone exposure. Antioxidants. (2020) 9:1190. doi: 10.3390/antiox9121190
31. Noutsios GT, Thorenoor N, Zhang X, Phelps DS, Umstead TM, Durrani F, et al. Major effect of oxidative stress on the male, but not female, SP-A1 type II cell miRNome. Front Immunol. (2019) 10:1514. doi: 10.3389/fimmu.2019.01514
32. Tsotakos N, Phelps DS, Yengo CM, Chinchilli VM, Floros J. Single-cell analysis reveals differential regulation of the alveolar macrophage actin cytoskeleton by surfactant proteins A1 and A2: implications of sex and aging. Biol Sex Differ. (2016) 7:18. doi: 10.1186/s13293-016-0071-0
33. Phelps DS, Umstead TM, Floros J. Sex differences in the acute in vivo effects of different human SP-A variants on the mouse alveolar macrophage proteome. J Proteomics. (2014) 108:427–44. doi: 10.1016/j.jprot.2014.06.007
34. Wang G, Umstead TM, Hu S, Mikerov AN, Phelps DS, Floros J. Differential effects of human SP-A1 and SP-A2 on the BAL proteome and signaling pathways in response to klebsiella pneumoniae and ozone exposure. Front Immunol. (2019) 10:561. doi: 10.3389/fimmu.2019.00561
35. Xu HN, Lin Z, Gandhi CK, Amatya S, Wang Y, Li LZ, et al. Sex and SP-A2 dependent NAD(H) redox alterations in mouse alveolar macrophages in response to ozone exposure: potential implications for COVID-19. Antioxidants. (2020) 9:915. doi: 10.3390/antiox9100915
36. Thorenoor N, Zhang X, Umstead TM, Scott HE, Phelps DS, Floros J. Differential effects of innate immune variants of surfactant protein-A1 (SFTPA1) and SP-A2 (SFTPA2) in airway function after Klebsiella pneumoniae infection and sex differences. Respir Res. (2018) 19:23. doi: 10.1186/s12931-018-0723-1
37. Durrani F, Phelps DS, Weisz J, Silveyra P, Hu S, Mikerov AN, et al. Gonadal hormones and oxidative stress interaction differentially affects survival of male and female mice after lung Klebsiella pneumoniae infection. Exp Lung Res. (2012) 38:165–72. doi: 10.3109/01902148.2011.654045
38. Gandhi CK, Mikerov AN, Durrani F, Umstead TM, Hu S, Wang G, et al. Impact of ozone, sex, and gonadal hormones on bronchoalveolar lavage characteristics and survival in sp-A KO mice infected with klebsiella pneumoniae. Microorganisms. (2020) 8:1354. doi: 10.3390/microorganisms8091354
39. Kabha K, Schmegner J, Keisari Y, Parolis H, Schlepper-Schaeffer J, Ofek I. SP-A enhances phagocytosis of Klebsiella by interaction with capsular polysaccharides and alveolar macrophages. Am J Physiol. (1997) 272:L344–52. doi: 10.1152/ajplung.1997.272.2.L344
40. Sano H, Nagai K, Tsutsumi H, Kuroki Y. Lactoferrin and surfactant protein A exhibit distinct binding specificity to F protein and differently modulate respiratory syncytial virus infection. Eur J Immunol. (2003) 33:2894–902. doi: 10.1002/eji.200324218
41. LeVine AM, Gwozdz J, Stark J, Bruno M, Whitsett J, Korfhagen T. Surfactant protein-A enhances respiratory syncytial virus clearance in vivo. J Clin Invest. (1999) 103:1015–21. doi: 10.1172/JCI5849
42. Harrod KS, Trapnell BC, Otake K, Korfhagen TR, Whitsett JA. SP-A enhances viral clearance and inhibits inflammation after pulmonary adenoviral infection. Am J Physiol. (1999) 277:L580–8. doi: 10.1152/ajplung.1999.277.3.L580
43. LeVine AM, Hartshorn K, Elliott J, Whitsett J, Korfhagen T. Absence of SP-A modulates innate and adaptive defense responses to pulmonary influenza infection. Am J Physiol Lung Cell Mol Physiol. (2002) 282:L563–72. doi: 10.1152/ajplung.00280.2001
44. Li G, Siddiqui J, Hendry M, Akiyama J, Edmondson J, Brown C, et al. Surfactant protein-A–deficient mice display an exaggerated early inflammatory response to a beta-resistant strain of influenza A virus. Am J Respir Cell Mol Biol. (2002) 26:277–82. doi: 10.1165/ajrcmb.26.3.4584
45. Hawgood S, Brown C, Edmondson J, Stumbaugh A, Allen L, Goerke J, et al. Pulmonary collectins modulate strain-specific influenza a virus infection and host responses. J Virol. (2004) 78:8565–72. doi: 10.1128/JVI.78.16.8565-8572.2004
46. Watson A, Phipps MJS, Clark HW, Skylaris CK, Madsen J. Surfactant proteins A and D: trimerized innate immunity proteins with an affinity for viral fusion proteins. J Innate Immun. (2019) 11:13–28. doi: 10.1159/000492974
47. Madan T, Reid KB, Singh M, Sarma PU, Kishore U. Susceptibility of mice genetically deficient in the surfactant protein (SP)-A or SP-D gene to pulmonary hypersensitivity induced by antigens and allergens of aspergillus fumigatus. J Immunol. (2005) 174:6943–54. doi: 10.4049/jimmunol.174.11.6943
48. Hickman-Davis JM, Wang Z, Fierro-Perez GA, Chess PR, Page GP, Matalon S, et al. Surfactant dysfunction in SP-A-/- and iNOS-/- mice with mycoplasma infection. Am J Respir Cell Mol Biol. (2007) 36:103–13. doi: 10.1165/rcmb.2006-0049OC
49. Kannan TR, Provenzano D, Wright JR, Baseman JB. Identification and characterization of human surfactant protein A binding protein of mycoplasma pneumoniae. Infect Immun. (2005) 73:2828–34. doi: 10.1128/IAI.73.5.2828-2834.2005
50. Ledford JG, Goto H, Potts EN, Degan S, Chu HW, Voelker DR, et al. SP-A preserves airway homeostasis during mycoplasma pneumoniae infection in mice. J Immunol. (2009) 182:7818–27. doi: 10.4049/jimmunol.0900452
51. Ledford JG, Voelker DR, Addison KJ, Wang Y, Nikam VS, Degan S, et al. Genetic variation in SP-A2 leads to differential binding to mycoplasma pneumoniae membranes and regulation of host responses. J Immunol. (2015) 194:6123–32. doi: 10.4049/jimmunol.1500104
52. Hsia BJ, Ledford JG, Potts-Kant EN, Nikam VS, Lugogo NL, Foster WM, et al. Mast cell TNF receptors regulate responses to mycoplasma pneumoniae in surfactant protein A (SP-A)-/- mice. J Allergy Clin Immunol. (2012) 130:205–14. doi: 10.1016/j.jaci.2012.03.002
53. Atochina EN, Beck JM, Preston AM, Haczku A, Tomer Y, Scanlon ST, et al. Enhanced lung injury and delayed clearance of pneumocystis carinii in surfactant protein A-deficient mice: attenuation of cytokine responses and reactive oxygen-nitrogen species. Infect Immun. (2004) 72:6002–11. doi: 10.1128/IAI.72.10.6002-6011.2004
54. Linke M, Ashbaugh A, Koch J, Tanaka R, Walzer P. Surfactant protein a limits pneumocystis murina infection in immunosuppressed C3H/HeN mice and modulates host response during infection. Microbes Infect. (2005) 7:748–59. doi: 10.1016/j.micinf.2005.01.011
55. Linke M, Ashbaugh A, Koch J, Tanaka R, Walzer P. Efficient resolution of pneumocystis murina infection in surfactant protein A-deficient mice following withdrawal of corticosteroid-induced immunosuppression. J Med Microbiol. (2006) 55:143–7. doi: 10.1099/jmm.0.46190-0
56. Ikegami M, Korfhagen TR, Bruno MD, Whitsett JA, Jobe AH. Surfactant metabolism in surfactant protein A-deficient mice. Am J Physiol. (1997) 272:L479–85. doi: 10.1152/ajplung.1997.272.3.L479
57. Wang G, Guo X, Diangelo S, Thomas NJ, Floros J. Humanized SFTPA1 and SFTPA2 transgenic mice reveal functional divergence of SP-A1 and SP-A2: formation of tubular myelin in vivo requires both gene products. J Biol Chem. (2010) 285:11998–2010. doi: 10.1074/jbc.M109.046243
58. Ikegami M, Korfhagen TR, Whitsett JA, Bruno MD, Wert SE, Wada K, et al. Characteristics of surfactant from SP-A-deficient mice. Am J Physiol. (1998) 275:L247–54. doi: 10.1152/ajplung.1998.275.2.L247
59. Ikegami M, Elhalwagi BM, Palaniyar N, Dienger K, Korfhagen T, Whitsett JA, et al. The collagen-like region of surfactant protein A (SP-A) is required for correction of surfactant structural and functional defects in the SP-A null mouse. J Biol Chem. (2001) 276:38542–8. doi: 10.1074/jbc.M102054200
60. Ikegami M, Jobe AH, Whitsett J, Korfhagen T. Tolerance of SP-A-deficient mice to hyperoxia or exercise. J Appl Physiol 1985. (2000) 89:644–8. doi: 10.1152/jappl.2000.89.2.644
61. Wu YZ, Medjane S, Chabot S, Kubrusly FS, Raw I, Chignard M, et al. Surfactant protein-A and phosphatidylglycerol suppress type IIA phospholipase A2 synthesis via nuclear factor-kappaB. Am J Respir Crit Care Med. (2003) 168:692–9. doi: 10.1164/rccm.200304-467OC
62. Chabot S, Koumanov K, Lambeau G, Gelb MH, Balloy V, Chignard M, et al. Inhibitory effects of surfactant protein A on surfactant phospholipid hydrolysis by secreted phospholipases A2. J Immunol. (2003) 171:995–1000. doi: 10.4049/jimmunol.171.2.995
63. Snider JM, You JK, Wang X, Snider AJ, Hallmark B, Zec MM, et al. Group IIA secreted phospholipase A2 is associated with the pathobiology leading to COVID-19 mortality. J Clin Invest. (2021) 131:e149236. doi: 10.1172/JCI149236
64. Goto H, Ledford JG, Mukherjee S, Noble PW, Williams KL, Wright JR. The role of surfactant protein A in bleomycin-induced acute lung injury. Am J Respir Crit Care Med. (2010) 181:1336–44. doi: 10.1164/rccm.200907-1002OC
65. Haque R, Umstead TM, Ponnuru P, Guo X, Hawgood S, Phelps DS, et al. Role of surfactant protein-A (SP-A) in lung injury in response to acute ozone exposure of SP-A deficient mice. Toxicol Appl Pharmacol. (2007) 220:72–82. doi: 10.1016/j.taap.2006.12.017
66. Haque R, Umstead TM, Kwangmi A, Phelps DS, Floros J. Effect of low doses of lipopolysaccharide prior to ozone exposure on bronchoalveolar lavage: differences between wild type and surfactant protein A-deficient mice. Pneumon. (2009) 22:143–55.
67. Yang S, Milla C, Panoskaltsis-Mortari A, Hawgood S, Blazar BR, Haddad IY. Surfactant protein A decreases lung injury and mortality after murine marrow transplantation. Am J Respir Cell Mol Biol. (2002) 27:297–305. doi: 10.1165/rcmb.2002-0035OC
68. Haddad IY, Milla C, Yang S, Panoskaltsis-Mortari A, Hawgood S, Lacey DL, et al. Surfactant protein A is a required mediator of keratinocyte growth factor after experimental marrow transplantation. Am J Physiol Lung Cell Mol Physiol. (2003) 285:L602–10. doi: 10.1152/ajplung.00088.2003
69. Kishore U, Bernal AL, Kamran MF, Saxena S, Singh M, Sarma PU, et al. Surfactant proteins SP-A and SP-D in human health and disease. Arch Immunol Ther Exp. (2005) 53:399–417.
70. Greene KE, Wright JR, Steinberg KP, Ruzinski JT, Caldwell E, Wong WB, et al. Serial changes in surfactant-associated proteins in lung and serum before and after onset of ARDS. Am J Respir Crit Care Med. (1999) 160:1843–50. doi: 10.1164/ajrccm.160.6.9901117
71. Gregory TJ, Longmore WJ, Moxley MA, Whitsett JA, Reed CR, Fowler AA, et al. Surfactant chemical composition and biophysical activity in acute respiratory distress syndrome. J Clin Invest. (1991) 88:1976–81. doi: 10.1172/JCI115523
72. Dy ABC, Tanyaratsrisakul S, Voelker DR, Ledford JG. The emerging roles of surfactant protein-a in asthma. J Clin Cell Immunol. (2018) 9:553. doi: 10.4172/2155-9899.1000553
73. Vlachaki EM, Koutsopoulos AV, Tzanakis N, Neofytou E, Siganaki M, Drositis I, et al. Altered surfactant protein-A expression in type II pneumocytes in COPD. Chest. (2010) 137:37–45. doi: 10.1378/chest.09-1029
74. McCormack FX, King TE Jr, Voelker DR, Robinson PC, Mason RJ. Idiopathic pulmonary fibrosis. Abnormalities in the bronchoalveolar lavage content of surfactant protein A. Am Rev Respir Dis. (1991) 144:160–6. doi: 10.1164/ajrccm/144.1.160
75. Phelps DS, Umstead TM, Mejia M, Carrillo G, Pardo A, Selman M. Increased surfactant protein-A levels in patients with newly diagnosed idiopathic pulmonary fibrosis. Chest. (2004) 125:617–25. doi: 10.1378/chest.125.2.617
76. Hamm H, Luhrs J, Rotaeche J, Costabel U, Fabel H, Bartsch W. Elevated surfactant protein A in bronchoalveolar lavage fluids from sarcoidosis and hypersensitivity pneumonitis patients. Chest. (1994) 106:1766–70. doi: 10.1378/chest.106.6.1766
77. D'Ovidio F, Floros J, Aramini B, Lederer D, DiAngelo SL, Arcasoy S, et al. Donor surfactant protein A2 polymorphism and lung transplant survival. Eur Respir J. (2020) 55:1900618. doi: 10.1183/13993003.00618-2019
78. D'Ovidio F, Kaneda H, Chaparro C, Mura M, Lederer D, Di AS, et al. Pilot study exploring lung allograft surfactant protein A (SP-A) expression in association with lung transplant outcome. Am J Transplant. (2013) 13:2722–9. doi: 10.1111/ajt.12407
79. Bersani I, Speer CP, Kunzmann S. Surfactant proteins A and D in pulmonary diseases of preterm infants. Expert Rev Anti Infect Ther. (2012) 10:573–84. doi: 10.1586/eri.12.34
80. Noah TL, Murphy PC, Alink JJ, Leigh MW, Hull WM, Stahlman MT, et al. Bronchoalveolar lavage fluid surfactant protein-A and surfactant protein-D are inversely related to inflammation in early cystic fibrosis. Am J Respir Crit Care Med. (2003) 168:685–91. doi: 10.1164/rccm.200301-005OC
81. deMello DE, Phelps DS, Patel G, Floros J, Lagunoff D. Expression of the 35kDa and low molecular weight surfactant-associated proteins in the lungs of infants dying with respiratory distress syndrome. Am J Pathol. (1989) 134:1285–93.
82. deMello DE, Heyman S, Phelps DS, Floros J. Immunogold localization of SP-A in lungs of infants dying from respiratory distress syndrome. Am J Pathol. (1993) 142:1631–40.
83. Hallman M, Merritt TA, Akino T, Bry K. Surfactant protein A, phosphatidylcholine, and surfactant inhibitors in epithelial lining fluid. Correlation with surface activity, severity of respiratory distress syndrome, and outcome in small premature infants. Am Rev Respir Dis. (1991) 144:1376–84. doi: 10.1164/ajrccm/144.6.1376
84. Miyamura K, Malhotra R, Hoppe HJ, Reid KB, Phizackerley PJ, Macpherson P, et al. Surfactant proteins A (SP-A) and D (SP-D): levels in human amniotic fluid and localization in the fetal membranes. Biochim Biophys Acta. (1994) 1210:303–7. doi: 10.1016/0005-2760(94)90233-X
85. Chaiworapongsa T, Hong JS, Hull WM, Kim CJ, Gomez R, Mazor M, et al. The concentration of surfactant protein-A in amniotic fluid decreases in spontaneous human parturition at term. J Matern Fetal Neonatal Med. (2008) 21:652–9. doi: 10.1080/14767050802215193
86. Condon JC, Jeyasuria P, Faust JM, Mendelson CR. Surfactant protein secreted by the maturing mouse fetal lung acts as a hormone that signals the initiation of parturition. Proc Natl Acad Sci USA. (2004) 101:4978–83. doi: 10.1073/pnas.0401124101
87. Montalbano AP, Hawgood S, Mendelson CR. Mice deficient in surfactant protein A (SP-A) and SP-D or in TLR2 manifest delayed parturition and decreased expression of inflammatory and contractile genes. Endocrinology. (2013) 154:483–98. doi: 10.1210/en.2012-1797
88. Mendelson CR, Gao L, Montalbano AP. Multifactorial regulation of myometrial contractility during pregnancy and parturition. Front Endocrinol. (2019) 10:714. doi: 10.3389/fendo.2019.00714
89. Gao L, Rabbitt EH, Condon JC, Renthal NE, Johnston JM, Mitsche MA, et al. Steroid receptor coactivators 1 and 2 mediate fetal-to-maternal signaling that initiates parturition. J Clin Invest. (2015) 125:2808–24. doi: 10.1172/JCI78544
90. Lee DC, Romero R, Kim CJ, Chaiworapongsa T, Tarca AL, Lee J, et al. Surfactant protein-A as an anti-inflammatory component in the amnion: implications for human pregnancy. J Immunol. (2010) 184:6479–91. doi: 10.4049/jimmunol.0903867
91. Green ES, Arck PC. Pathogenesis of preterm birth: bidirectional inflammation in mother and fetus. Semin Immunopathol. (2020) 42:413–29. doi: 10.1007/s00281-020-00807-y
92. Goto H, Mitsuhashi A, Nishioka Y. Role of surfactant protein A in non-infectious lung diseases. J Med Invest. (2014) 61:1–6. doi: 10.2152/jmi.61.1
93. Vento G, Tana M, Tirone C, Aurilia C, Lio A, Perelli S, et al. Effectiveness of treatment with surfactant in premature infants with respiratory failure and pulmonary infection. Acta Biomed. (2012) 83(Suppl. 1):33–6.
94. Herting E, Gefeller O, Land M, van SL, Harms K, Robertson B. Surfactant treatment of neonates with respiratory failure and group B streptococcal infection. Members of the collaborative european multicenter study group. Pediatrics. (2000) 106:957–64. doi: 10.1542/peds.106.5.957
95. Tan K, Lai NM, Sharma A. Surfactant for bacterial pneumonia in late preterm and term infants. Cochrane Database Syst Rev. (2012) CD008155. doi: 10.1002/14651858.CD008155.pub2
96. Floros J, Phelps DS. Human surfactant proteins (SP-) A1 and SP-A2: to include or not in surfactant replacement therapy, and if yes, both or which one? J Neonatal Biol. (2014) 3:1–4. doi: 10.4172/2167-0897.1000136
97. Younis US, Chu HW, Kraft M, Ledford JG. A 20-Mer peptide derived from the lectin domain of SP-A2 decreases tumor necrosis factor alpha production during mycoplasma pneumoniae infection. Infect Immun. (2020) 88:e00099–20. doi: 10.1128/IAI.00099-20
98. Floros J, Phelps DS. Is the role of innate immune molecules, SP-A1 and SP-A2, and of the alveolar macrophage being overlooked in covid-19 diverse outcomes? Pneumon. (2020) 33:1–5.
99. Tekos F, Skaperda Z, Goutzourelas N, Phelps DS, Floros J, Kouretas D. The importance of redox status in the frame of lifestyle approaches and the genetics of the lung innate immune molecules, SP-A1 and SP-A2, on differential outcomes of COVID-19 infection. Antioxidants. (2020) 9:784. doi: 10.3390/antiox9090784
Keywords: surfactant, host defense, SP-A, infection, injury, animal model, respiratory distress syndrome, innate immunity
Citation: Depicolzuane L, Phelps DS and Floros J (2022) Surfactant Protein-A Function: Knowledge Gained From SP-A Knockout Mice. Front. Pediatr. 9:799693. doi: 10.3389/fped.2021.799693
Received: 21 October 2021; Accepted: 03 December 2021;
Published: 07 January 2022.
Edited by:
MaryAnn Volpe, Tufts University School of Medicine, United StatesReviewed by:
John Benjamin, Vanderbilt University Medical Center, United StatesMartin Post, Hospital for Sick Children, Canada
Copyright © 2022 Depicolzuane, Phelps and Floros. This is an open-access article distributed under the terms of the Creative Commons Attribution License (CC BY). The use, distribution or reproduction in other forums is permitted, provided the original author(s) and the copyright owner(s) are credited and that the original publication in this journal is cited, in accordance with accepted academic practice. No use, distribution or reproduction is permitted which does not comply with these terms.
*Correspondence: Joanna Floros, amZsb3Jvc0Bwc3UuZWR1