- 1Bone Marrow Transplant Unit, Division of Haematology and Oncology, Schneider Children's Medical Center of Israel, Petach-Tikva, Israel
- 2The Sackler Faculty of Medicine, Tel-Aviv University, Tel Aviv, Israel
- 3Department of Pediatrics, University Medical Center Ulm, Ulm, Germany
- 4St. Anna Children's Hospital, Medical University of Vienna, Vienna, Austria
- 5St. Anna Children's Cancer Research Institute (CCRI), Vienna, Austria
- 6Princess Máxima Center for Pediatric Oncology, Utrecht, Netherlands
- 7Department of Pediatric Hematology, Oncology and Stem Cell Transplantation, University Children's Hospital, University Medical Center, University of Würzburg, Würzburg, Germany
Immune reconstitution (IR) after allogeneic haematopoietic cell transplantation (HCT) represents a central determinant of the clinical post-transplant course, since the majority of transplant-related outcome parameters such as graft-vs.-host disease (GvHD), infectious complications, and relapse are related to the velocity, quantity and quality of immune cell recovery. Younger age at transplant has been identified as the most important positive prognostic factor for favourable IR post-transplant and, indeed, accelerated immune cell recovery in children is most likely the pivotal contributing factor to lower incidences of GvHD and infectious complications in paediatric allogeneic HCT. Although our knowledge about the mechanisms of IR has significantly increased over the recent years, strategies to influence IR are just evolving. In this review, we will discuss different patterns of IR during various time points post-transplant and their impact on outcome. Besides IR patterns and cellular phenotypes, recovery of antigen-specific immune cells, for example virus-specific T cells, has recently gained increasing interest, as certain threshold levels of antigen-specific T cells seem to confer protection against severe viral disease courses. In contrast, the association between IR and a possible graft-vs. leukaemia effect is less well-understood. Finally, we will present current concepts of how to improve IR and how this could change transplant procedures in the near future.
Introduction
Allogeneic haematopoietic cell transplantation (HCT) establishes a new lymphohaematopoietic system in patients who suffer from severe abnormalities of normal haematopoiesis or immune dysfunction. In the case of malignant disorders of haematopoiesis such as acute lymphoblastic leukaemia (ALL) or acute myeloid leukaemia (AML), the success of HCT critically depends on a graft-vs.-leukaemia (GvL) effect, an immunological reaction in which donor T cells track down and eliminate minimal residual leukaemic cells. HCT creates one of the deepest immunosuppressive states in medicine, sharing many features with naturally occurring states like congenital immune deficiency or human immunodeficiency (HIV) infection. For long it has been known that immune reconstitution (IR) after HCT has to recapitulate immune ontogeny but follows different pathways than nature (1–3). In normal ontogeny, lymphopoiesis begins under protected circumstances in utero, is equipped with a perfectly broad repertoire of naïve T cells at delivery, and continues to mature in early childhood when thymic tissue is most active. In contrast, lymphopoiesis post HCT is happening in an aberrant environment, where the thymus is only partially active, organs are damaged from chemotherapy and inflammation, and the body is strongly exposed to internal and external antigens. Furthermore, immune function has to be suppressed around HCT by serotherapy or immunosuppressive drugs to prevent or treat graft-vs.-host disease (GvHD)—an immune-mediated iatrogenic disorder that is caused by the artificial encounter of two immune systems in one organism. Still, the capacity to reconstitute the immune system through the generation and proliferation of immune effector cells is immense (3), and, if guided and supported by targeted interventions, immunity can be restored within months.
IR is a multidimensional process that is unique and variable among different patients (4). It may depend on the graft source, cell dose, human leukocyte antigen (HLA) barriers, conditioning of the patient prior to HCT and post-transplantation interventions, including those to prevent or treat HCT complications. The multitude of variables that influence IR post HCT have been reviewed before (5, 6) and levels of innate and adaptive immune cell reconstitution in transplanted children over time have been reported (7). The transfused graft, in addition to being a source of haematopoietic stem cells for restoration of haematopoiesis, acts as reservoir of immune cells and initiates the complex process of IR. This process is achieved by two different but complementary waves of immune cell regeneration which are closely interlocked and hard to segregate (illustrated in Figure 1). The first wave is mediated by donor lymphocytes present in the graft. Upon transfusion into a lymphodepleted host, these mature lymphocytes have the capability to expand and proliferate in response to antigenic or cytokine-mediated stimulation in a process termed homeostatic peripheral expansion (HPE), providing an early but incomplete immune defence against invading pathogens. More complete IR relies on de novo lymphopoiesis from donor-derived stem cells in the bone marrow and/or thymus, a process which can take up to several months.
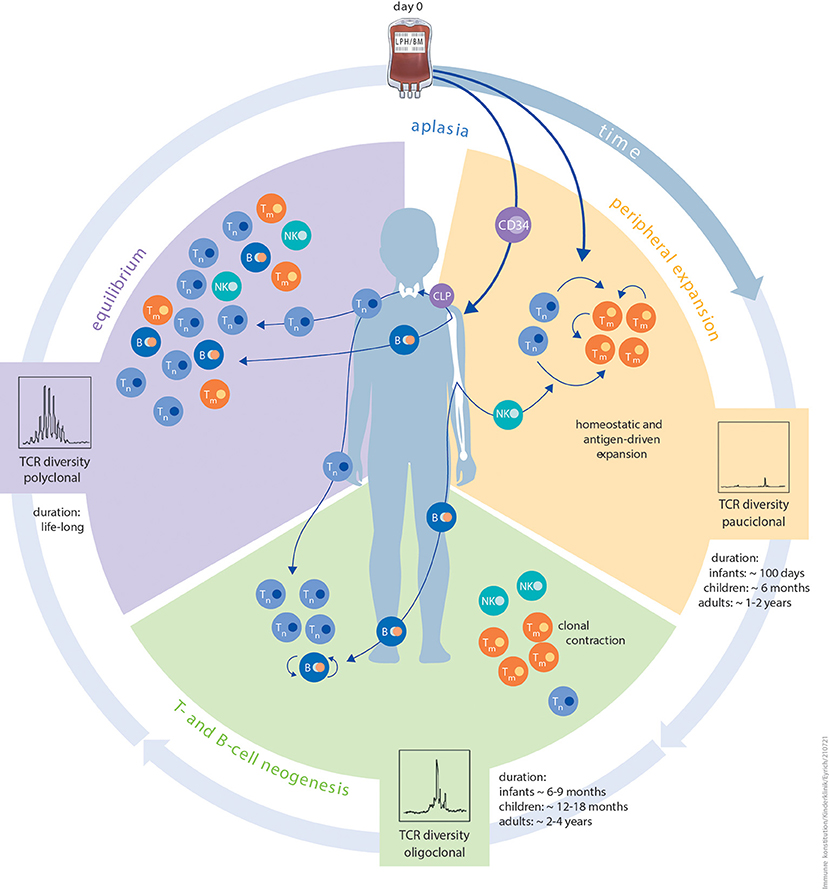
Figure 1. Schematic illustration of the different phases of immune reconstitution following HCT. The first phase peripheral expansion (orange) of IR after aplasia is dominated by homeostatic or antigen-driven peripheral expansion of graft-derived T cells. The ratio of naïve T cells to memory T cells is dependent on donor age. The quantity of regenerating T-cell numbers depends on graft size (bone marrow vs. PBSC) and in vivo (serotherapy) or in vitro T-cell depletion. Diversity of the TCR repertoire during this phase is usually dominated by expansion of singular clonotypes. The duration of this period is strictly influenced by patient age. The second phase T- and B-cell neogenesis (green) of IR is characterised by the onset of T- and B-cell neogenesis in the thymus and bone marrow. Thymic and bone marrow niches are more resilient against external stressors and more productive in infants and children than in adults. Other contributing factors are thymic tissue status, application of immunosuppression, and aGvHD or cGvHD. The risk of viral reactivation dramatically reduces as T- and B-cell neogenesis advances. The same probably applies to de novo GvHD. In this phase, immunisation with non-live vaccines is feasible. The third and final phase equilibrium (purple) of IR is a balanced and stable immune system, which is, to the best of our knowledge, maintained lifelong. Components of innate as well as adaptive immunity reach a level that is relative to patient age. Diversity of the TCR repertoire is polyclonal at this phase. Live, attenuated vaccines can be applied since positive T-cell and B-cell interactions are granted. Autoantibodies tend to disappear and risk of cGvHD is minimal. B, B cell; CLP, common lymphoid progenitor; NK, natural killer cell; TCR, T-cell receptor; Tm, memory T cell; Tn, naïve T cell.
Distinct Importance of Naïve and Memory T Cells and Significance of T-Cell Receptor Diversity
Allogeneic HCT grafts include naïve and memory T-cell subsets of which the ratio may differ tremendously between cord blood (mostly naïve cells) and bone marrow or peripheral blood (which have more memory subsets). T memory stem cells (TSCM) are of special interest as they show superior reconstitution capacity in preclinical models and contribute to peripheral reconstitution by differentiating into effectors in the early days following haploidentical HCT with post-transplant cyclophosphamide (8, 9). The abundance of naïve T cells in the graft may influence the outcome of patients after allogeneic HCT as long as thymic function has not been restored. Although total numbers of CD4+ T cells have been shown to directly correlate with survival post GvHD (10, 11), levels of naïve T cells have been identified as the most potent drivers of alloreactivity (12). In agreement, high levels of CD4+ naïve T cells (but not of CD8+ T cells) in allografts have been observed to correlate with an increased incidence of acute GvHD (aGvHD) post transplantation (13). These findings led to the initiation of clinical trials using peripheral blood stem cell (PBSC) grafts depleted of naïve T cells, which showed lower rates of aGvHD and chronic GvHD (cGvHD) in HLA-matched HCT, with no apparent increase in relapse rates (14). On the other hand, cord blood grafts (in which almost all T cells are naïve) show great anti-leukaemic potential with reduced relapse risk but a similar likelihood of developing GvHD when compared to bone marrow grafts (15), indicating that T-cell intrinsic factors are contributing to the risk of GvHD development and anti-leukemic efficacy as well. Most significant associations between IR and clinical events were described for CD4+ rather than for CD8+ T cells, maybe because CD8+ T cell numbers fluctuate more swiftly in response to infections (e.g., CMV) or other events post-transplant (16). Still, CD8+ T cells numbers have been positively associated with the likelihood to develop GvHD (17, 18), lower relapse rates as well as better overall survival (19). Furthermore, IR of CD8+ T cells is highly dependent on the graft type used for transplantation (20): Unmanipulated BM- or PBSC-grafts generally show a more rapid CD8+ than CD4+ T-cell reconstitution, due to faster homeostatic or antigen-driven expansion of memory-type CD8+ T cells. In contrast, after T-cell replete CBT frequently a rapid reappearance of thymus-derived CD4+ T cells can be observed (21, 22). However, as IR is influenced by many patient-specific and transplant-related factors, the impact of these patterns on individual outcome is hardly predictable.
Naïve CD4+ T cells in particular have been found to undergo HPE and rapidly shift toward a central memory phenotype (22). Although no side-by-side comparisons were made with other graft sources, some authors hypothesised that this CD4+ phenotype shift may be a particular characteristic of cord blood T cells (21). In a follow-up study, they showed that the transcription profile of the naïve CD4+ T cells from the cord blood grafts overlapped with the profile of foetal CD4+ T cells. Likewise, reconstituting cells that were induced in the lymphopenic environment shortly after transplant maintained these overlapping features with foetal CD4+ T cells. Interestingly, it was suggested that enhanced T-cell receptor (TCR) signalling via the transcription factor AP-1 after ligation of the TCR with self-major histocompatibility complex molecules was responsible for the rapid T-cell reconstitution (23). As expansion of T cells in lymphopenic situations is affected by the strength of TCR activation (24, 25), a skewing toward cells expressing high-affinity TCRs against host and microbiome-associated antigens during HPE may be observed. Thus, beyond monitoring numbers and phenotypes of T cells after HCT, the epigenetic programming and functional status of reconstituting naïve cells should be studies in more detail.
After an age-dependent recovery period in which HPE prevails, the thymus starts to replenish the naïve T-cell pool with new thymic emigrants. Up to this point, the diversity of the TCR repertoire is limited as new TCR recombination events do not take place in donor T cells undergoing HPE. During the first year after T-cell depleted CD34+ haploidentical HCT in children, early reconstituting T cells display a predominantly primed, activated phenotype with a severely skewed TCR repertoire (26). Nevertheless, rapidly expanding cells can differentiate into virus-specific T cells that are able to clear an infection within 2 months, as was shown in patients receiving umbilical cord blood (21). Thymopoiesis includes TCR recombination events and positive and negative selection thereby increasing TCR diversity tremendously (27). Ex vivo evaluation of thymic function is generally performed by molecular analyses of signal joint TCR excision circles (sjTRECs), which strongly correlate with flow cytometric measurements of recent thymic emigrants (CD45RA+CD27+CD31+ T cells) (28). T-cell diversity analysis can be evaluated by spectratyping the size of the β-chain complementarity determining region 3 (CDR3) (29). Nowadays, next-generation sequencing methods allow high-resolution clonotyping providing quantitative TCR assessments that can be applied to better understand clonotype dynamics during viral infections or GvHD (30) and to identify pathogenic or protective T-cell clones following HCT. In addition, screening the TCR repertoire for absence of sequences with annotated specificity for cytomegalovirus (CMV) (the public CMV repertoire) may also help to identify patients at risk for CMV reactivation and disease who may benefit from prophylactic antiviral strategies (31).
An increase in TCR diversity has been related to a better clinical outcome in multiple studies (30, 32–35). Talvensaari et al. studied TCRs in patients who underwent cord blood transplantation (harbouring an intrinsic, broad, polyclonal TCR repertoire) or bone marrow transplantation (36); they showed abnormal TCR repertoires and low TREC values during the first year after transplantation in both groups. After 2 years, TCR diversity was higher in recipients of cord blood vs. bone marrow HCT (34), suggesting a more efficient thymic regeneration pathway from cord blood lymphoid progenitors despite the lower numbers of CD34+ cells in the graft. In turn, recipients of unmanipulated bone marrow from matched sibling donors showed increased TCR diversity and faster T-cell reconstitution compared with children receiving selected CD34+ PBSCs from unrelated donors (37). In patients receiving T-cell depleted PBSCs from a matched donor or T-cell depleted haploidentical PBSCs in combination with an independent cord blood product, both GvHD and relapse were independently correlated with lower TCR repertoire diversity (35). In addition, within 6 months, adult cord blood recipients had approximately the same TCR diversity as healthy individuals, whereas recipients of T-cell-depleted PBSC grafts had much lower diversities of CD4+ and CD8+ T cells. Interestingly, these deficiencies improved 12 months post-transplant for the CD4+ but not for CD8+ T cell compartment (38). Both TCR repertoire diversity and sjTREC levels can decline during GvHD or infections as a reflection of decreased thymic output under these conditions (39, 40).
Analyses of the diversity of the TCR repertoire were mostly based on the TCR Vβ repertoire in TCRαβ+ T cells so far. However, it has been demonstrated that reconstitution of the TCRγδ repertoire is an important marker post HCT as well (41, 42). γ/δ T cells constitute up to ~10% of all T cells in blood; they are effective against virus reactivation and their presence is associated with lower relapse rates after HCT (43, 44). In line with this, Vδ2neg γδ cells isolated from CMV-reactivating patients specifically reacted with both CMV-infected cells as well as leukemic cell lines and primary myeloid leukemic and myeloma cells (45). The interplay between CMV and γδ cell subsets and the result on clinical outcome measures has not been fully elucidated yet (46). In future studies, it would be interesting to assess the predictive value of TCR diversity in specific T-cell subsets with regard to clinical outcomes in more detail, in particular regulatory T (Treg) cells. The latter subset is of special interest as in a murine model adoptively transferred Tregs at the time of HCT accelerated broadening of the TCR Vβ repertoire diversity by preventing GvHD-induced damage in the thymus and secondary lymphoid microenvironment (47).
Given the decisive impact of T-cell IR on survival chances, this issues has to be considered in the design of conditioning regimens. For instance, serotherapy (e.g., with anti-thymocyte globulin; ATG) may reduce the risk of developing GvHD and graft rejection, but dosing should be individualised (based on graft source, absolute lymphocyte count and weight) to prevent dramatically reduced T-cell IR in patients after high ATG exposure (48–51), in particular when given in combination with filgrastim (52).
Differences in Immune Reconstitution Between adults and Children
Factors affecting IR have been actively investigated for almost 30 years now. Besides other contributing factors such as stem cell dose (53), donor age (54, 55), and mixed chimerism (56), patient age at transplantation has been recognised as a prime determinant of the speed and quality of IR from the start of this research (57). The T-cell compartment (both CD4+ and CD8+) reconstitutes slower in adults than in children, which translates into a higher rate of life-threatening opportunistic infections in older patients. Storek et al. already reported in 1995 that T-cell phenotypes in adult HCT recipients were strikingly different from neonatal T cells and that these changes were more pronounced in the CD4+ compartment (58). Numerous later studies confirmed this finding and supported the notion that the second, thymus-dependent wave of T-cell reconstitution is enhanced in children (59). Prediction models of thymic output based on TREC measurements revealed that thymic reconstitution can start as early as 83 days post-transplant in infants and that each additional year of patient age adds 2 weeks to that starting point (60). Interestingly, this advantage of children with regard to improved naïve T-cell regeneration seems to confer protection against viral infections (57), non-relapse mortality and cGvHD (61) but not aGvHD and leukemic relapse, because relapse incidences in paediatric and adult ALL patients after allogeneic HCT are not strikingly different (62, 63). Whether the increased thymic output contributes to a better GvL effect is unknown. However, regeneration of functional Tregs, probably derived from thymic Treg precursors, is a prerequisite for resolution of cGvHD in children (64). Therefore, it is conceivable that the addition of new, potentially leukaemia-reactive clonotypes to the TCR repertoire is counterbalanced by the regeneration of tolerizing Tregs. Mechanistic studies addressing this issue are lacking so far. Furthermore, the precise mechanisms underlying improved thymic reconstitution (increased thymic cellularity, higher susceptibility of thymic precursors to cytokines, or enhanced influx of committed lymphoid progenitors) have not been elucidated so far. Nevertheless, enhancing thymic reconstitution in adults to achieve the same level as that observed in children is a pivotal strategy to boost IR (see below).
Another, less-examined difference between children and adults may be the better preservation of the B-cell bone marrow niche in children. Children show faster reconstitution of total numbers of B cells (56), have more B-cell precursors in regenerating bone marrow (65), and exhibit more B-cell neogenesis as measured by kappa-deleting recombination excision circles than do adults (66). Moreover, cGvHD has been demonstrated to have little impact on B-cell neogenesis and bone marrow precursor composition in children (67), which is in stark contrast to observations in adults (68, 69). Therefore, the microenvironment of the thymus as well as the bone marrow seems to be more resilient to noxious influences such as conditioning regimens and alloreactivity in children compared to in adults. These complex interactions (e.g., regenerating CD4+ T cells providing help to transitional and naïve B cells), contribute to facilitate new humoral immune responses (56) and lower production of autoantibodies (67).
In contrast to the aberrant pathways of adaptive immunity regeneration after allogeneic HCT, natural killer (NK)-cell reconstitution after HCT seems to resemble NK-cell ontogeny in early childhood, with a preponderance of immature NK cells in the early post-transplant phase (70). Type of graft manipulation (NK-replete vs. NK-depleted grafts) seems to have a greater impact on NK reconstitution than patient age, although no comparative studies directly addressing this question are available. In a heterogenous cohort of paediatric ALL patients who underwent haploidentical HCT, T-cell depletion techniques that also depleted graft-derived NK cells (i.e., CD34+ selection) resulted in faster NK-cell recovery post-transplant than techniques like CD3/CD19-depletion, which keep NK cells in the graft (71), underlining the importance of cytokine sinks such as interleukin (IL)-7 and−15 for NK-cell development (72). Especially in the haploidentical transplant setting, potential NK cell alloreactivity has gained a lot of attention. Differences in the killer-cell immunoglobuline-like (KIR) gene haplotype could lead to a donor NK cell activation caused by the lack of an inhibitory receptor on host leukemic cells. The clinical relevance of this scenario remains controversial. One study analysing 85 children with ALL transplanted with ex vivo T-cell depleted haploidentical PBSCs showed a benefit if the donor had a KIR B content score (5-year event-free survival of 51 vs. 30% in KIR B vs. KIR A haplotype, respectively) (73). However, this was not confirmed in a subsequent study of 80 children with acute leukaemias receiving TCRab/CD19-depleted haplo grafts. Here, KIR-KIR-L mismatching was not associated with any difference in leukaemia-free survival (74). For more details on that issue we refer to one of the excellent reviews published recently (75).
Immune Reconstitution and Viruses
The Complex Relationship Between Antigen Exposure and Immune Reconstitution
Exposure to infectious agents in the early post-HCT period puts the patient at increased risk for morbidity and also alters the process of IR, increasing risks of further infections and immune-mediated diseases. In order to prevent such exposure, patients are usually instructed to keep socially distanced or isolate from others, restrict their diet and take other behavioural measures in the post-HCT period to minimise their risk of encountering exogenous infections. However, as patients have already encountered infections prior to HCT, any viruses that remain latent in their body (and that are usually under tight control of the normal immune system) might become reactivated post HCT and cause significant morbidity and mortality. The best studied viral reactivation post allogeneic HCT is CMV reactivation; however, other viruses such as Epstein-Barr virus (EBV), human herpesvirus 6 (HHV-6), adenovirus and BK polyomavirus are also of clinical importance. Each virus causes a distinct pattern of disease and can appear at different levels of immunosuppression (76).
Cytomegalovirus
CMV has been considered for many decades to be the leading cause of infectious complications in recipients of bone marrow transplants (77) and, as such, serves as the prototype for the study of viral reactivation and IR post HCT. Since CMV is ubiquitous worldwide, infection usually occurs in childhood and most patients are seropositive at the time of HCT. The standard of care is to monitor CMV levels by weekly polymerase chain reaction (PCR) testing and to treat any emerging reactivation pre-emptively before clinical disease emerges. Many studies have investigated the kinetics, risk factors and clinical outcome of CMV reactivation (78). Seropositive recipients receiving a graft from a seronegative donor are at highest risk for CMV reactivation (79), reflecting the central role of specific memory T cells from the graft in controlling CMV reactivation in the early post-HCT period. Aubert et al. have shown that healthy seropositive individuals have a significant percentage (median 1.3%; range 0.29–5%) of memory CD8+ cells which are specific for the E42 epitope of the CMV pp65 protein, and that these cells are capable of mediating immune protection against CMV (80). In the context of HCT, a clear inverse correlation was found between low numbers of these cells and CMV reactivation. Interestingly, following viral reactivation, the number of E42-epitope-positive CD8+ cells increased dramatically, reflecting the ability of these cells to proliferate and expand in response to antigenic stimuli regardless of the presence of CD4+ helper cells, resulting in viral clearance.
The presence of these memory CD8+ cells immediately after HCT varies among individuals according to graft composition and the degree of T-cell depletion. In a large series published recently (81), the authors showed that patients with high peak CMV titres (>20,000 copies/mL) had significantly lower numbers of T cells (both CD4+ and CD8+) at both 1 and 3 months post HCT but these numbers increased later on, becoming high at around 6 months. Interestingly, patients who did not have reactivation of CMV (<500 copies/mL) did not show this elevation in T cells and had significantly lower numbers of T cells at 1 year post HCT. These findings are in accordance with another trial studying general IR patterns post HCT using 25 lymphocytes subsets (82). Using multivariate methods, those researchers showed that CMV reactivation and cGvHD are the major determinants of IR patterns at 1 year post HCT. Lymphocyte subsets from seropositive patients clustered differently to those from CMV seronegative patients, with increased proportions of activated, late memory effector CD8+ T cells and reduced B-cell subsets observed in seropositive patients. Due to the persistence of CMV antigens during viral latency, the long-term memory T-cell pool accumulates T cells with CMV specificity, a phenomenon called memory inflation.
Furthermore, few studies have demonstrated a bidirectional relationship between CMV reactivation and the occurrence of GvHD. While the observation that CMV reactivation is a consequence of GvHD treatment is intuitively understandable, these studies have demonstrated the converse, showing increased occurrence of GvHD following CMV reactivation (83, 84). Few hypotheses regarding this etiological relationship have been tested including induction of HLA class II expression following CMV reactivation (85), or sequence homology between CMV and human tissue peptides (86). Regardless of the biological explanation, this association as well as the above-mentioned studies regarding the impact of CMV reactivation on T-cell subpopulations, highlight the importance of CMV reactivation post HCT not only as the leading infectious agent but also as a key player in shaping the IR post HCT.
The recent introduction of Letermovir as a very efficient agent in preventing CMV reactivation post HCT, allowed us for the first time to assess IR patterns in the absence of CMV reactivation. Several groups have collected data regarding this question: Sperotto et al. have shown that patients who received prophylactic letermovir, had significantly lower CD4 and CD8 counts at 2 and 3 months post HCT, compared to patients who were treated by a standard preemptive approach (87). From a functional perspective, Zamora et al. have recently demonstrated that patients who received letermovir have significant lower levels of functional CMV-specific T cells (88). Albeit further data is definitely needed, these studies again emphasise the crucial role of CMV reactivation in shaping IR patterns post HCT.
Epstein-Barr Virus
In contrast to CMV, EBV reactivation post HCT originates usually from graft-derived donor B cells that under strong immunosuppression loose the tight control of EBV-specific T cells, resulting in a spectrum of disorders called post-transplant lymphoproliferative disease (PTLD). EBV reactivation is less common than CMV, tends to appear slightly later after HCT, and seems to require a deeper immune suppression (89). Standards for diagnosis of PTLD and treatment of EBV reactivation are less stringent than that for CMV as there is no consensus on the level of EBV copy numbers that puts the patient at high risk for PTLD. Since EBV is not targetable by antiviral drugs, a CD20 mAbs and EBV-specific T-cells remain the only available treatments so far.
D'aveni et al. profiled the immune response to EBV using the ELISpot assay at 60, 100, 180, and 360 days post HCT in 28 patients transplanted for both malignant and non-malignant indications (90). Not surprisingly, they found a correlation between general T-cell reconstitution and EBV-specific reconstitution, as well as significantly earlier and higher reconstitution in paediatric vs. adult patients. In this small series, patients with an ELISpot result of more than 1,000 spot-forming cells (SFC)/106 mononuclear cells still had the ability to clear the virus spontaneously without treatment. Similarly to the picture with CMV immunity, EBV antigenic stimulation was the strongest driver of proliferation of these cells, but this effect disappeared 1 year post HCT, suggesting that, unlike CMV, EBV reactivation has no effect on long-term IR. In a relatively large series published by Stocker et al., treatment of EBV reactivation with anti-CD20 monoclonal antibodies did not result in a different IR pattern than that observed in patients without anti CD20 treatment with the exception of delayed B-cell recovery, which normalised after 1 year post HCT (91). This delayed B-cell recovery was mirrored clinically by a higher need for immunoglobulin (Ig) G replacement in the anti-CD20 group than in the non-anti-CD20 group. Frequency of infections and clinical outcome did not differ between treatment groups.
Adenovirus
Adenovirus reactivations are of particular interest in the paediatric population (92). As no highly effective antiviral treatment against adenovirus exists, reactivation has emerged in the recent years as a major cause of morbidity and mortality after HCT in children. Admiraal et al. found that CD4+ T-cell reconstitution was the only immunological predictor of adenovirus reactivation (16). The chance of reactivation was reduced by 5% with every 10 cells/μL increase in CD4+ T cells. Furthermore, patients with early CD4+ T cell reconstitution (defined as CD4+ T cells >50 cells/μL in two consecutive samples before day +100) had a shorter duration of viraemia and, on survival analysis, had the same favourable outcome as patients without adenovirus reactivation. This is in contrast to the dismal prognosis observed in patients with adenovirus reactivation without CD4+ T-cell reconstitution.
Human Herpesvirus 6
HHV-6 is the most common virus to reactivate post HCT, but cases with clinical disease (i.e., encephalitis) are rare (93). De Koning et al. found that the only predictor of HHV-6 reactivation was CD4+ IR (94). Interestingly, HHV-6 reactivation was found to be a strong predictor of grade II–IV GvHD, and this effect vanished if CD4+ IR had occurred. Furthermore, in subsequent work, HHV-6 had a significantly negative impact on numbers of CD4+ T cells 1 year post HCT, possibly caused by the cytopathic effect of HHV-6 on thymopoiesis (95). This effect was reversed if antivirals were used.
Other Viruses
Other viral reactivations (e.g., BK polyomavirus, varicella zoster virus, and herpes simplex virus) have been less studied systematically in terms of IR, but case reports point toward a central role of T-cell immunity in controlling these reactivations following HCT (76).
Section Conclusion
To conclude this section, viral reactivations mirror the status of T-cell reconstitution. CD8+ memory T-cell populations seem to mediate protection against or clearance of CMV and EBV, whereas for other Herpesviridae such as adenovirus or HHV-6, CD4+ T cell counts are the main predictor for both reactivation and outcome. CD4+ T-cell counts are also the main predictor for long-term anti-CMV immunity. CMV reactivation is a strong stimulator of global T-cell reconstitution, with the highest effect observed 6 months post HCT. HHV-6 reactivation might have the opposite effect, with patients who experience reactivation tending to have lower T-cell counts at 6 months and 1 year post HCT. Adoptive transfer of antigen-specific T cells will probably gain widespread use in the near future, as this therapy directly targets the mechanisms behind viral reactivation.
Immune Reconstitution and Acute GVHD: The “Chicken and Egg” Dilemma
GvHD is a frequent complication of HCT. Although the incidence is lower in paediatric compared to in adult patients, GvHD significantly contributes to transplant-associated morbidity and mortality. It is broadly accepted that aGvHD and cGvHD involve different effectors and targets and have different pathologic pathways, therefore being seen as two different diseases. Nevertheless, aGVHD remains the major risk factor for development of cGvHD in the paediatric population (96, 97). This review focuses on parameters and kinetics of early IR of mainly the adaptive immune system, and therefore this chapter will cover primarily aGVHD.
In general, aGVHD is mediated by alloreactive donor T cells activated by host antigen-presenting cells followed by donor cell reactivity against a variety of target tissues of the host. aGVHD is associated with significantly impaired IR, but which is the cause and which is the effect? This question applies to a number of interacting aspects: (1) the T- and the B-cell compartment, as the antigen-presenting cells involved in aGvHD could be B cells; (2) the number and function of subpopulations of the adaptive immune system (quantity and quality); (3) HPE vs. impaired thymic production; (4) the composition of the graft and the microenvironment of the host; and (5) the effects of aGvHD itself and the administration of immunosuppressive agents for GvHD prophylaxis and treatment.
Immune cell function does not equate to cell number: it is important to distinguish between quantitative immune cell reconstitution and qualitative IR. For instance, T cells often remain dysfunctional after HCT, with a skewed TCR repertoire even after recovery to normal number (98). Hence, the normalisation of B- and T-cell numbers does not necessarily indicate reconstitution of their function and it has been suggested to differentiate between “immune reconstitution” and “immune recovery” rather than using IR alone (99).
Data regarding the influence of aGVHD on IR profiles and vice versa lack detailed information on reconstituting cell subsets and on effector functionality. Moreover, as IR is age dependent, this and other reviews are hampered by the lack of data from a primarily paediatric setting (7). Table 1 provides published data on immune cell subsets in adaptive IR and their relation to aGvHD after HCT in paediatric and adult patients (10, 11, 17–19, 49, 98, 100–110).
CD4+ T Cells
Perturbations of both HPE and thymic output contribute to impaired CD4+ T-cell reconstitution in patients with aGVHD. In this regard, patient and transplant associated aspects such age, sex, underlying disease, genetic differences between donor and host, stem cell source, and type of conditioning are influencing factors for the IR of CD4+ T cells.
In general, aGvHD is characterised by the predominance of effector CD4+ cells that are capable of secreting inflammatory cytokines and that mediate tissue damage (111). Additionally, in aGVHD allo-reactive T cells directly target both the lymphoid and the epithelial components of thymic architecture. Allo-reactive T cells further limit renewal of thymic cellularity after conditioning therapy, thereby preventing negative selection of alloreactive T cells which subsequently promote GvHD (112). Thus, IR is stuck in a vicious circle of arrested thymus regeneration and impaired de novo production of diverse T cells (113). This results in the compromised production of naïve T cells together with a shortened survival and higher susceptibility to apoptotic cell death of T cells due to the overexpression of death receptors and the under-expression of pro-survival proteins (114–116). This is accompanied by a reduced production of cytokines indispensible for thymopoiesis, which in turn leads to lower TREC levels and a distorted TCR repertoire (40, 117–119).
In pre-clinical models, it has been demonstrated that T cells from animals with GvHD were capable of significant expansion, molecular diversity and repertoire regeneration after their transfer into secondary hosts, indicating that deficits in the T-cell repertoire are not necessarily fixed but may have the capacity for normalisation once they are removed from the GvHD milieu. Therefore, the GvHD microenvironment of the host seems to be responsible for quantitative and qualitative failure of effective CD4+ T-cell reconstitution during GvHD (111, 118, 119).
In clinical studies, aGvHD correlates with aggravated skewing of the TCR repertoires of both CD4+ and CD8+ T cells as well as antigen-specific T cells. Both T- and B-cell lymphopenia and an inadequate repertoire of CD4+ and CD8+ T cells for at least 1 year after transplant increase the risk of recurrent reactivation of latent viruses, which may further contribute to a higher risk for development of aGvHD (120).
Koning et al. reported a retrospective dual-centre study of CD4+ T-cell reconstitution in paediatric patients following HCT with an aim to identify predictors of survival outcomes after aGvHD (10). Achieving CD4+ T-cell IR within 100 days after HCT did not decrease the risk of developing aGvHD but was strongly predictive for better survival outcomes (non-relapse mortality and overall survival) after moderate-to-severe aGvHD. Generally, conventional HCT grafts are associated with a higher proportion and an earlier recovery of Tregs together with greater TCR diversity when compared with T-cell-depleted grafts. Of note, de Koning et al. reported that for both cohorts (the conventional HCT and the T-cell-depleted HCT group), early CD4+ T-cell IR correlated significantly with better outcomes of aGvHD.
By means of sjTREC and beta-T-cell receptor excision circles (βTREC) quantifications, a significant but transient reduction in thymic output as well as in early thymocyte differentiation in patients with aGvHD was shown by Clave et al. in a cohort including adolescent patients after matched sibling donor HCT performed mainly for malignancies (101). Interestingly, in these patients who were <25 years old, thymic function recovered at 1 year, indicating that the impact of aGvHD on the adolescent thymus could be transient. Gabella et al. confirmed that sjTREC levels were not affected by aGvHD during long-term follow-up of adult and paediatric patients after HCT in mainly malignant diseases with myeloablative conditioning (102).
The association between cGvHD and low TREC levels indicative of poor thymic function was described by Olkinuora et al. in a prospective paediatric study: in this cohort, low TREC levels correlated with high mortality rates (121).
CD8+ T Cells
Early donor T-cell expansion is characterised by mainly CD8+ cells with a restricted repertoire and of memory cell type. The IR pattern of CD8+ T cells differs to that of CD4+ T cells, e.g., in that expanded CD8+CD28− effector memory T cells can dominate for more than 2 years post HCT (111). Expanded oligoclonal CD8+ cells are associated with an increased risk of aGvHD (18, 122, 123).
Regulatory T Cells
Tregs (CD4+25+FoxP3+) are known to maintain immune homeostasis and tolerance by inhibiting cytokine secretion and proliferation of various effector cells. They can be subdivided into naturally thymus-derived Tregs and induced Tregs differentiated from non-regulatory CD4+25+ cells. Adoptive transfer of ex vivo expanded Tregs has been shown to result in superior immune reconstitution and less GvHD in preclinical murine allotransplant models (124). Full Treg reconstitution prevents the rapid oligoclonal proliferation that gives rise to pathogenic CD4+ effector T cells, while preserving the slow homeostatic form of lymphopenia-induced peripheral expansion that repopulates a diverse peripheral T-cell pool (125). This effect is mediated through CTLA4-dependent downregulation of CD80 and CD86 on dendritic cells by Tregs.
Regarding clinical data, an association between Treg numbers and incidence of aGvHD has been established: A higher Treg content in the graft confers lower non-relapse mortality and improved overall survival (126). Magenau et al. reported that in adult and paediatric patients with aGvHD after a matched sibling or matched unrelated donor HCT, Treg frequencies were inversely correlated with aGvHD grading. Treg frequencies were measured at disease onset as the percentage of CD4+CD25brightFoxp3+ T cells out of total nucleated cells (103). Rezvani et al. were able to show that a low CD4+FOXP3+ T-cell count early after HCT (day +30) was associated with an increased risk of grade II–IV aGvHD in adult and adolescent patients who underwent HCT (104). Clinical trials with adoptive transfer of Treg are described in more detail in section Cellular Therapies. Cellular Therapies (see below).
Noteworthy, Tregs may also play a role in the graft-vs.-leukaemia reaction. In a series of 85 patients with leukemic relapses after HCT, a higher content of Helios+ Treg at day +30 within the CD4 compartment was accompanied by a higher incidence and earlier occurrence of leukemic relapse (127). In contrast, checkpoint blockade which is applied to increase antitumor immunity both in the autologous and the allogeneic setting is known to inhibit Tregs. Patients with advanced/metastatic solid tumours receiving aPD-1 and aCCR4 checkpoint inhibitor infusions had a reduced effector Treg population (128). Patients who received aCTLA4 infusions for the treatment of leukemic relapses after allo HCT showed diminished counts and less activated Tregs but exhibited a 35% likelihood of developing immune-related adverse events or GvHD. These data show that Tregs are key players in the regulation of autoimmunity and may tip the balance between GvH and GvL.
B Cells
The first B cells to emerge into the periphery following HCT are CD19+CD21lowCD38high transitional B cells; the percentage of these cells subsequently decreases while mature CD19+CD21highCD27neg naïve B cells are replenished (120). However, most paediatric studies provide information on CD19+ B cells alone (7). Generally, GvHD is correlated with impaired IR of the B-cell compartment, with regards to both numbers and function, yet most reported data are in the context of cGvHD (105). Abdel-Azim et al. observed in paediatric HCT recipients the normalisation of numbers of naïve B cells by 6 months together with a deficiency of IgM+ memory B cells and switched memory B cells (129). While the latter normalised within the first year after HCT, the deficiency of IgM memory B cells persisted for up to 2 years. They concluded that paediatric HCT recipients have impaired humoral IR, predominantly owing to a blockade of IgM memory B-cell maturation compared with earlier T cell-dependent switched memory cell IR.
Profiles of Immune Reconstitution Associated With Acute GvHD
Bae et al. reported no significant impact of aGvHD on lymphoid IR in paediatric patients who underwent HCT for malignant diseases (20). In recent research by Schultz et al. evaluating immune profiles at day +100 after HCT in correlation with National Institutes for Health (NIH)-defined GvHD, the authors described distorted patterns of IR after resolved aGvHD and late aGvHD at day +100. They then compared theses immune profiles to an immunological fingerprint of patients without any history of GvHD (immune-tolerant patients). They identified a number of different associations per group and found a progression of immune abnormalities from no cGvHD to late aGvHD, and further to the most complex pattern in cGvHD (130).
Models of immune function have been published that aim to reflect various subpopulations of immune cells and also to consider different patterns of IR (70, 131). A three-component multivariate model with a reference domain of ellipsoidal shape based on normal leukocyte subtype values from healthy children and adolescents has been created by Koenig et al. This model was used to classify paediatric patients as having high or low risk for a post-HCT events based on their IR status; significantly higher number of HCT survivors mainly after malignant diseases and various conditioning regimens fell into the low-risk vs. high-risk group during follow-up (day +200 and day +300) (132). Mellgren et al. used a principal component analysis to better analyse the process of IR after paediatric HCT. They were able to show that dysfunctional IR patterns precede severe complications such as cGvHD, relapse, and death (133). Although these reports do not provide conclusive data regarding the interaction of aGvHD and IR, they aid understanding of the interactions between variables after HCT and support a more differentiated and meaningful viewpoint on IR and transplant-related complications such as GvHD.
Haematopoietic Niche and Acute GVHD
von Bonin et al. outlined in a comprehensive review that both haematopoietic cells and cells forming the haematopoietic/progenitor niche of the bone marrow have been identified as targets in GvHD. Haematopoiesis in general and B-cell neogenesis in particular are affected by the toxic environment of GvHD, leading to a shift toward myelopoiesis (134). In terms of the in vitro composition and function of the haematopoietic microenvironment, Martinez-Jaramillo et al. found decreased numbers of myeloid, erythroid and multipotent progenitor cells in recipients of bone marrow transplants in comparison with healthy controls. Of note, progenitor levels were significantly lower in patients with GvHD (7% of normal marrow levels in patients with GvHD vs. 44% of normal marrow levels in patients without GvHD). These findings corresponded with the severely reduced numbers of fibroblastic progenitors and adherent stromal cells observed in long-term marrow culture in patients with GvHD vs. those without (135).
Immune Reconstitution and Graft-vs.-Leukaemia Effect
Immune attack of donor T cells against residual host leukaemic cells is a major pathway by which allogeneic HCT combats haematological malignancies. In general, a higher number of T cells in the graft is associated with lower relapse rates but at the cost of a higher incidence of GvHD (136). Patients with early recovery of antiviral T-cell responses have a higher probability of relapse-free survival (137), and high numbers of interferon gamma (IFNg)-reactive T cells during early IR have been shown to be associated with improved overall survival (138). However, certainly not all donor T cells contribute to the supposed GvL effect and the involved specific T-cell subpopulations are not known so far.
Since the first reports of the contribution of an immunological GvL effect on the success of HCT in the 1980s (139), the segregation of the GvL effect from GvHD has been considered the “holy grail” of HCT. In the quest to enhance the GvL effect without increasing the risk for GvHD, two general approaches have been studied. The first approach aims to discriminate subpopulations of T cells that can mediate GvL from those that mediate GvHD, thereby enabling a safer and more effective T-cell composition by graft engineering. The second approach tries to define minor histocompatibility antigens that are restricted to the haematopoietic lineage and to elicit specific T-cell responses against these antigens post HCT. Though both approaches have not yet been translated into clinical routine, progress has been achieved and some modalities are currently tested in clinical trials.
Zheng et al. have shown in a murine model of chronic myeloid leukaemia that donor memory CD4+ T cells (CD4+CD62L−CD44+CD25−) can kill leukaemic cells without causing GvHD, as opposed to the action of naïve cells that cause GvHD (140). The authors speculated that the reason for this difference is that memory T cells can generate only a limited immune response that is sufficient for GvL but not sufficient to cause GvHD (which requires a high-magnitude response and high systemic levels of cytokines to cause tissue invasion and systemic inflammation). The same group has also shown that adoptive transfer of CD8+ memory T cells from donors vaccinated against the recipient minor histocompatibility antigen H60 augmented the GvL effect without increasing GvHD (141).
Given these pre-clinical data about the central role of memory T cells in GvL, Triplett et al., conducted a clinical trial (142) in 17 paediatric patients with relapsed/refractory acute leukaemia, performing reduced intensity HCT from haploidentical donors after naïve (CD45RA+) T-cell depletion of the graft. At a median follow-up of 223 days, aGvHD rate was acceptable, and there were only two cases of relapse: both of these were in patients with advanced AML in whom primary induction had failed. Interestingly, nine patients had detectable disease at time of transplant, yet relapse rates were still low, highlighting the potential of memory T cells to mediate GvL effect. A second trial using transfer of CD45RA-depleted T cells in 35 patients with high-risk acute leukaemia confirmed low rates of cGvHD (9% with a follow-up of 932 days). aGvHD rates were similar to T-replete HCTs (66%; 95% CI 41–74%) but all cases of aGvHD were steroid responsive and no patient required second line treatment. Overall survival was 78% at 2 years, which is encouraging in this high-risk population (14). These data suggest that CD45RA− memory T cells are not devoid of any GvHD potential; however, GvHD seems more controllable for this type of HCT. The combination of CD45RA with other surface antigens such as CD276 as a depletion marker can confer superior protection against GvHD initiation (143).
Potential targets for donor T cells in the HCT setting are any polymorphic proteins of the host against which donor T cells have not been tolerized during their education in the host thymus. Recent molecular analyses have revealed that 12% of the human exome is polymorphic but only 0.5% of all single nucleotide variants (SNVs) are finally presented as HLA class I peptides (144). From this huge number of possible antigens, about 50 candidates have been biologically validated as bona fide minor histocompatibility antigens with relevance for HCT (145). Early mechanistic studies revealed that T-cell responses against minor histocompatibility antigens are oligoclonal in nature and CD4+ dominated (146, 147); one or a few minor histocompatibility antigen mismatches can be sufficient to cause GvHD and massive thymic infiltration (148). Disappointingly, a closer look at donor–host minor histocompatibility antigen disparities has not allowed clear separation between GvL and GvHD so far. The UGT2B17 truncating gene deletion has been shown to lead to increased incidence of aGvHD and reduced survival in HCT recipients (149), while HA-8 and ACC-1 SNVs in the recipient have been associated with an increased incidence of cGvHD (150). In a large retrospective analysis, Spierings et al. (151) investigated in 849 HLA-matched HCTs the impact of 10 autosomal and 10 HY-encoded minor histocompatibility antigens on GvHD and relapse incidence. Their most striking observation was a lower relapse rate and higher overall survival in patients mismatched for haematopoiesis-restricted minor histocompatibility antigens compared to patients who were matched in these antigens. Notably, this association was only given in the context of GvHD (not without).
The introduction of immune checkpoint inhibitors as an efficient method of immune-based anti-cancer therapy made its use in the context of allo HCT an intriguing way to augment the GvL effect. Pilot reports about patients with Hodgkin disease who relapsed post allo HCT have shown that this modality can be effective, though carrying the risk of occurrence of de novo GVHD (152). Davids et al. have prospectively treated 28 adult patients with relapsed haematological malignancies post HCT with aCTLA4 blockade and other 28 adult patients with aPD-1 blockade (153, 154). While some responses were noted (more in lymphoid diseases and some complete responses in extramedullary myeloid leukaemia), severe GvHD and other serious immune-mediated adverse events occurred in a significant proportion of patients. Of note only a single ALL patient was included in these studies. Further and more homogenous studies are required to better characterise patients in whom the potential benefit of immune checkpoint blockade overrides its risks. Noteworthy, in a study of 85 patients after allo HCT for various haematologic malignancies, LAG-3 and TIM-3 rather than PD-1 were overexpressed on T-cells of relapsing patients, indicating that other exhaustion markers beyond the PD-1-PD-L1 axis might be interesting and druggable targets to enhance GvL after allo HCT (127).
In summary, these data indicate that natural IR will most likely not distinguish between GvHD and GvL effects. However, adoptive transfer of minor-antigen-directed T cells, the generation of which is challenging but feasible (155, 156), in a T-cell depleted setting should be the subject of further research. Another approach to skew IR toward preferred regeneration of minor-antigen-specific T cells is the vaccination of the recipient with minor-peptide-loaded dendritic cells in combination with donor lymphocyte infusions (DLIs) (157). Given the very tight association between GvL and GvHD, a clearer separation of these two effects will only be possible by controlling IR through tailored grafts and targeted add-back of TCR specificities, e.g., antiviral T cells in the first 2–3 months to avoid or control viral reactivations followed by adoptive transfer of donor T cells reactive against leukaemic epitopes.
The Problem of Slow Immune Reconstitution
As outlined above, delayed IR—and in particular T-cell reconstitution—is associated with clinical complications following HCT. The delay of IR may be the reason or the result of these complications—probably the interaction works both ways in most instances. To optimise the outcome of HCT, slow IR should be prevented or treated. This can be performed either by avoiding factors that impede reconstitution or by using procedures that improve the reconstitution.
Avoiding Factors That Impede Immune Reconstitution
Serotherapy, total body irradiation and prophylactic immunosuppression are known inhibitors of prompt IR; however, they are indispensable elements of many conditioning regimens. Viral reactivations can impede or skew IR, as extensively discussed above. Prophylactic or pre-emptive strategies aim at avoiding viral reactivations and disease. Also mentioned above is the impact of aGvHD on IR. Prevention and treatment of aGvHD should focus on methods (e.g., selective allodepletion or extracorpeal photopheresis) that preserve T-cell function against viruses or other non-GvHD targets. Because avoidance of these detrimental factors is not always possible in clinical practise, substantial efforts have been undertaken to establish new techniques for improvement of IR (see below).
Procedures That Improve Immune Reconstitution
According to the two stages of T-cell reconstitution, efforts to improve IR in the clinical setting are based on two principles: (1) optimization of the peripheral (memory) T-cell compartment; and (2) enhancing of thymus-dependent (naïve) T-cell production. Cellular therapies are primarily based on modifications of graft composition aiming to optimise the peripheral T-cell compartment. Interventions including soluble factors and new concepts of tissue engineering may result in a better and/or faster thymic-dependent immunity. Findings from pre-clinical and clinical research in this area are summarised in Tables 2, 3, respectively, and described in more detail below (4, 14, 116, 158–194, 199–215, 220–222). Figure 2 graphically illustrates attempts to improve IR which are currently evaluated in clinical trials.
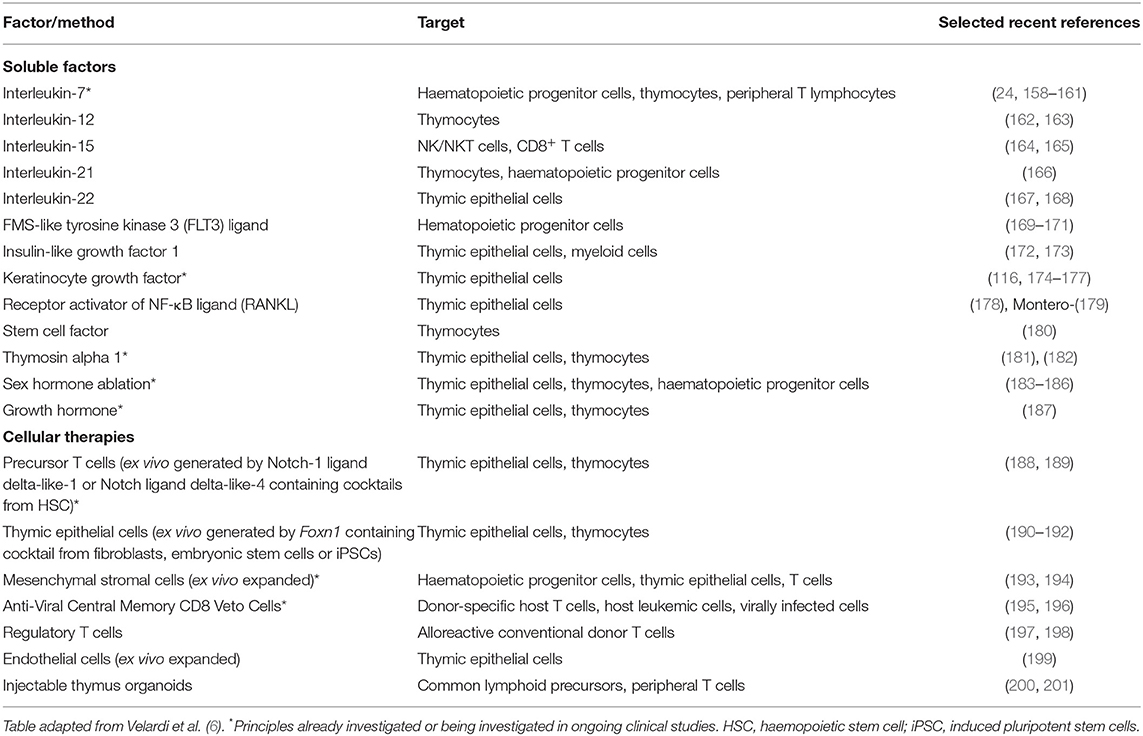
Table 2. Preclinical studies exploring soluble factors and cellular therapies to enhance T-cell function after HCT.
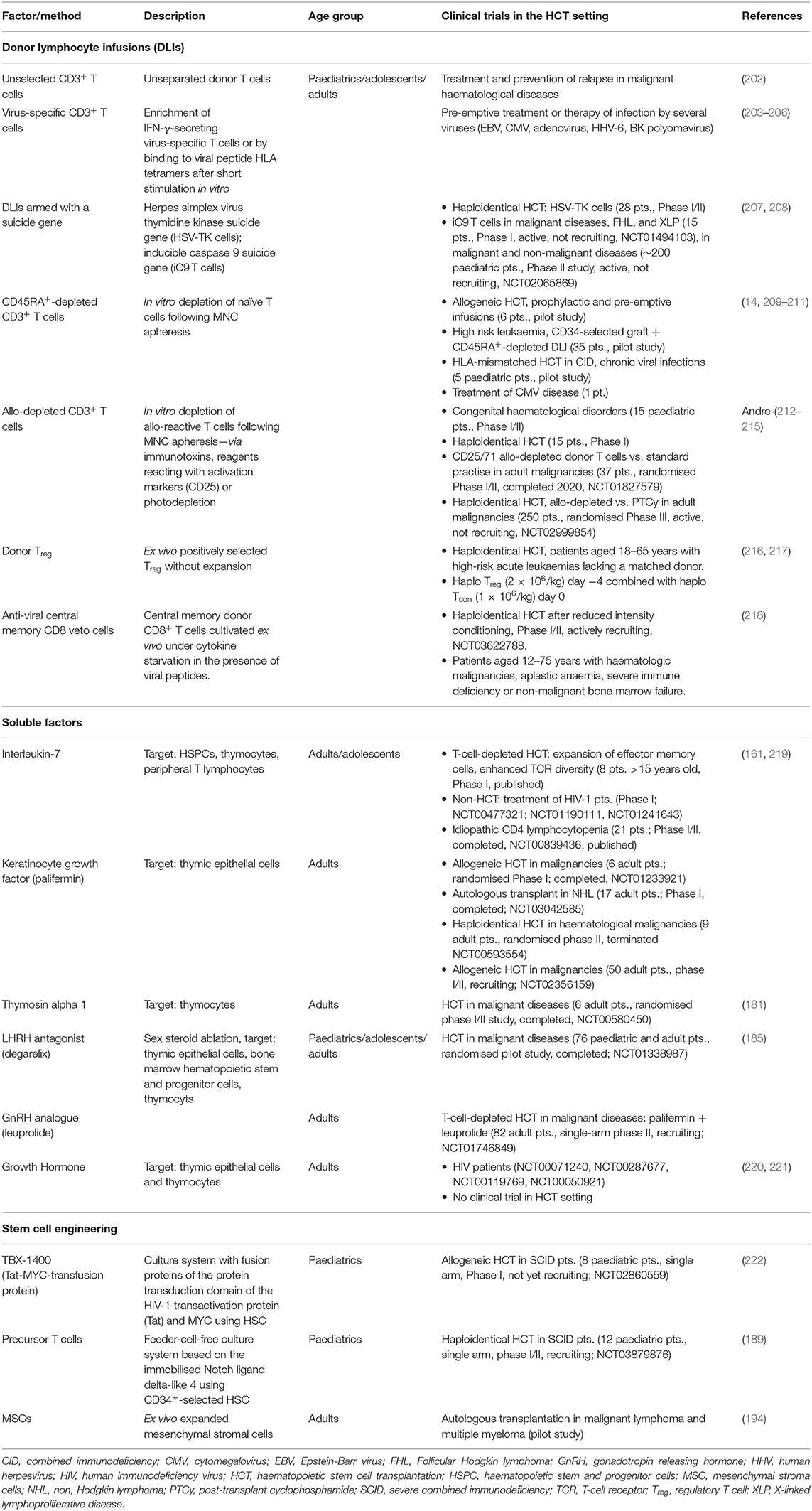
Table 3. Clinical studies investigating approaches to enhance immune reconstitution after HCT and in patients with HIV.
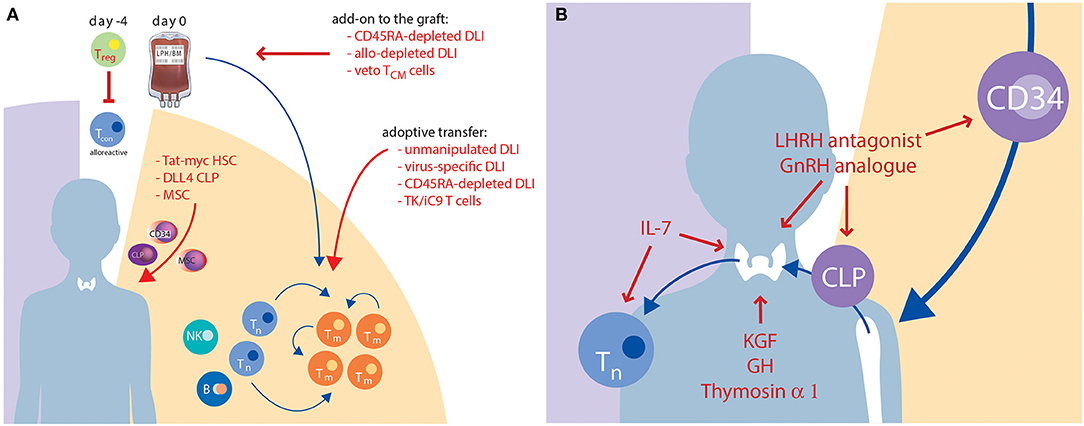
Figure 2. Current approaches to improve IR which are under clinical evaluation. This graph illustrates strategies with cellular therapies (A) or solubles factors (B) which are discussed above in sections Cellular Therapies, Soluble Factors, and Tissue Engineering. Red colour highlights the names, red arrows indicate the targets of the novel approaches. B, B cell; CLP, common lymphoid progenitor; DLL4, delta-like ligand 4; DLI, donor lymphocyte infusion; GH, growth hormone; GnRH, gonadotropine releasing hormone; HSC, haematopoietic stem cell; IL, interleukin; KGF, keratinocyte growth factor; LHRH, luteinizing hormone-releasing hormone; MSC, mesenchymal stem cell; NK, natural killer cell; TCM, central memory T cell; Tcon, conventional CD3+ T cell; Tm, memory T cell; Tn, naïve T cell; Treg, regulatory T cell; TK/iC9, thymidine kinase/inducible Caspase 9.
Cellular Therapies
Regarding cellular therapies, manipulation of the stem cell graft as well as use of DLIs are established modes to engineer T-cell immunity including anti-leukaemic effects (Figure 2A). The administration of unmanipulated donor lymphocytes is, however, complicated by a high risk of GvHD, which is even more relevant in an HLA-mismatched setting. Because of the adverse effects of GvHD on the thymus, unselected DLIs are not suitable to improve IR. Conversely, non-specific T-cell depletion of the graft, which is used particularly in HLA-mismatched HCT to avoid excessive GvHD, is complicated by delayed IR resulting in severe infectious complications and higher rates of graft rejection and relapse in patients with malignant diseases (223, 224). Advances in graft manipulation in vivo and in vitro aim to protect preferred T-cell subsets in order to maintain GvL and antiviral effects while reducing the risk of GvHD. The selective depletion of TCR-α/β lymphocytes spares the innate-like TCR-γ/δ population, thus possibly confering an improved anti-infective and antitumor response (74, 225). However, the anti-infective efficacy of TCR-γ/δ T cells is limited, and thymic-dependent IR is not improved by this procedure. Another approach using cyclophosphamide post HCT to prevent GvHD was pioneered by the Johns Hopkins group. This approach is widely used in adult patients with malignant and non-malignant diseases mainly but not exclusively in the HLA-mismatched setting (226, 227). A comparison of in vitro T-cell depleted allogeneic HCTs with post-transplant cyclophosphamide HCTs, including consideration of IR, is the topic of a separate review in this issue.
Several methods have been explored in the clinical setting to manipulate lymphocytes so that their anti-infectious activity is retained yet the risk of GvHD is reduced. The option of adoptive transfer of virus-specific T cells has already been mentioned above. Modern strategies allow rapid manufacturing of T cells against several viruses including EBV, CMV, adenovirus, HHV-6 and BK polyomavirus and are the subject of two previous reviews (203, 204). By magnetic enrichment of IFN-γ-secreting cells after short-term stimulation with viral peptide antigens, HLA-unrestricted viral-specific T cells can be produced within 1 day (205, 206). Virus-specific T cells from third-party donors are also in clinical use (228). They are usually readily available and are effective in mediating antiviral immunity without increasing the risk of GvHD (229). Another innovative approach is the generation of veto T cells with antiviral activity. This technique was developed by Reisner and colleagues and is based on the finding that T cells cultured with antigenic stimulation but under cytokine starvation are endowed with veto activity, i.e., the potential to eliminate host-vs.-graft-directed host T-cell clones, thereby facilitating donor engraftment even after reduced intensity conditioning (195) together with the preserved potential to kill host leukemic cells (196). If viral peptides are used for antigenic stimulation during in vitro culture of these cells, the veto T cells will confer graft facilitation together with improved antiviral IR in the early post-transplant phase (230). The first clinical results using the intended conditioning regimen (reduced intensity with post-transplant cyclophosphamide) followed by CD3/CD19-depleted haploidentical PBSCs were encouraging (231), and the utility of this approach in combination with veto T cell infusion is currently being investigated in a Phase I/II trial (ClinicalTrials.gov identifier: NCT03622788).
As outlined above (chapter 5.3) regulatory T cells are key regulators of alloreactivity and fast and sustained Treg reconstitution is associated with lower incidences of GvHD and lower transplant-related mortality. Thus, several investigators have established approaches for adoptive transfer of these cells. Although Treg products from third party cord blood units have been used as well, the majority of groups have relied on donor PMNCs as source of Treg. In a first feasibility trial, 28 adult patients grafted with CD34+ selected haploidentical PBSCs received on day −4 freshly isolated Tregs in a 2:1 ratio together with conventional T cells (216). Although only 2 out of 26 evaluable patients developed GvHD ≥ grade 2 and no SAEs were reported in association with the Treg infusion, TRM was 50%, making efficacy assessment difficult. In a follow-up report of the same group, 43 adult patients with AML/ALL were transplanted using the same approach (217). After switching to a less toxic preparative regimen, TRM could be reduced to 21%. Albeit patients received a mean of 1.1 ± 0.6 × 106 haploidentical CD3+/kg BW, GvHD incidences were comparable to a historical control group with fully T-cell depleted grafts. In order to increase transplantable cell numbers and to be compliant with current regulation, a GMP-compatible manufacturing process was developed, in which isolated Treg were expanded with IL-2 and rapamycin (232). After 14 days of expansion, a 9.6 fold expansion was achieved with good suppressive function of the final Treg product. This product now awaits testing in a tolerance induction protocol after haploidentical HCT.
A very intriguing yet easy to realise technique to reduce the alloreactivity of donor lymphocytes is the enrichment of memory T cells by CD45RA depletion. This technique and the first clinical results have been described in detail in the former sections of this review. An alternative and even more selective approach is selective allodepletion. Application of allodepleted T cells in vitro seems an attractive way to transfer antitumour and anti-infectious immunity from the donor to the recipient while avoiding the risk of GvHD. Reagents reacting with activation markers such as CD25, immunotoxins or a photodepletion procedure (using Kiadis Pharma technology) are methods to reduce alloreactive T cells for DLI (212–214). In two prospective randomised trials (Clinicaltrials.gov identifier: NCT02999854 and NCT01827579), such modified DLIs are currently being assessed vs. “standard” methods of haploidentical HCT, including the use of post-transplant cyclophosphamide (which was mentioned above).
Another approach for safer DLI administration involves T cells being armed with an inducible suicide gene. In a phase I-II, multicentre, non-randomised trial (ClinicalTrials.gov identifier: NCT00423124) in adult patients with high-risk haematological malignancies after haploidentical HCT, herpes-simplex thymidine kinase suicide gene expressing donor lymphocytes (HSV-TK) were infused after transplantation (207). Of the 28 patients receiving these HSV-TK cells after HCT, 22 obtained IR (i.e., CD3+ > 100/μl) at a median of 75 days (range 34–127 days) from transplantation and 23 days (range 13–42 days) from infusion. Ten patients developed aGVHD (grade I–IV) and one developed cGVHD, which was controlled by induction of the suicide gene. In another pilot study (Clinicaltrials.gov identifier: NCT01494103), 12 recipients of haploidentical HCT for different diseases including ALL, MDS, JMML, and HLH (medium age 10 years, range 2–50 years) were infused with increasing numbers of alloreplete haploidentical T cells expressing the inducible caspase 9 suicide gene (iC9-T cells) (208). All patients receiving >104/kg of alloreplete iC9-T lymphocytes achieved rapid reconstitution of immune responses toward five major pathogenic viruses and concomitant control of active infections. By administration of a chemical inducer of dimerization (AP1903/rimiducid), 86–96% of circulating CD3+CD19+ T cells were eliminated within 30 min, with no recurrence of GvHD within 90 days (208). Another Phase II trial using this approach after haploidentical HCT with CD3+ TCRα/β-depleted grafts in about 250 paediatric patients with malignant and non-malignant diseases is ongoing in Italy and the UK (Clinicaltrials.gov identifier: NCT02065869). In an interim analysis, 10.9 and 2.1% of patients developed grade II–IV and grade III–IV aGvHD, respectively. 4.6% of patients [95% CoI: 1.3–7.8] developed cGvHD (233). Of 21 patients developing GvHD, 86% responded to rimiducid, with a median time to response of 2 days. Of initial responders, 77% were still in either complete (n = 8) or partial response (n = 6) at the time of interim analysis.
Soluble Factors
Although the above methods for graft manipulation and DLI engineering show promising results in host defence, they all carry the major disadvantage of expansion of memory-type T cells in the absence of a polyclonal naïve T-cell compartment. Since dysfunction of the thymus represents the limiting factor for full T-cell recovery, strategies to accelerate naïve, polyclonal, de novo T-cell reconstitution are warranted. Strategies proposed in recent years include the stimulation of T-cell development and expansion using (1) cytokines such as IL-7, IL-12 and IL-21; (2) the administration of cytokines alongside growth factors such as stem cell factor (also known as KIT ligand), keratinocyte growth factor (KGF encoded by the fibroblast growth factor 7 gene), IL-22 and FMS-like tyrosine kinase 3 ligand; and (3) the modulation of hormone levels by suppression of sex steroids or by administration of thymosin-α1. For a recent review see Velardi et al. (6). Some of these factors have recently been explored or are currently being explored in clinical trials in the context of HCT (Figure 2B and Table 3).
Members of the common gamma-chain cytokine family IL-7 and IL-15 are involved in homeostatic expansion of T cells in the peripheral blood (234). In mice and non-human primates, administration of IL-7 seems to have a positive effect on functional T-cell recovery after HCT, with a predominant effect on naïve CD8+ cells (24, 158, 159). However, this positive effect on thymus regeneration could not be confirmed in another animal study (160). In a phase I/II clinical trial treatment of 21 adult patients with idiopathic CD4+ lymphytopenia with recombinant IL-7 (without HCT) led to an increase in the number of circulating CD4+ and CD8+ T cells and tissue-resident CD3+ T cells in the gut mucosa and bone marrow; however, enhanced thymospoiesis, measured by TRECs, was only observed in the youngest patients, aged 23 and 34 years (NCT00839436) (219). In a phase I trial, 12 patients more than 15 years of age were treated with recombinant IL-7 after TCD allo-HCT from an 8 of 8 HLA-matched donor for treatment of non-lymphoid haematologic malignancy. After a short course of IL-7, a quantitative increase of CD4+ and CD8+ effector memory T cells as well as an increase in mitogen-reactive T cells was found (NCT00684008) (161). However, there was only a limited effect on thymic output in this study as shown by minimal changes in the number of recent thymic emigrants and the levels of TRECs. An extended duration of IL-7 administration may have a greater effect on thymic function particularly in younger patients. IL-7 is currently under investigation in multiple randomised clinical trials for oncologic and infectious disorders (including human immunodeficiency virus and severe acute respiratory syndrome coronavirus 2 infection), but there are no further studies in the allogeneic HCT setting to our knowledge. Taken together, the direct impact of IL-7 on the human thymus is still unclear, but most of the effects on T-cell IR after IL-7 treatment seem to be primarily related to the expansion of peripheral T cell subsets and to the improvement of T-cell functionality. A possible impact on thymic function seems to be restricted to younger patients with more residual thymic capacity.
IL-15 has been shown to increase the number of CD8+ T cells and NK cells after transplantation in mice (164). Similarly to IL-7, IL-15 can improve lymphocyte reconstitution after T-cell-depleted HCT, but it can also worsen GvHD, which limits its use in HCT (235). For a review see Moutuou et al. (236).
Factors that stimulate the thymic niche and increase the output of recent thymic emigrants, including KGF and the luteinizing-hormone–releasing hormone (LHRH) agonist leuprolide have been identified in mouse models (237, 238). Two trials are evaluating the effects of leuprolide and the LHRH antagonist degarelix on IR following HCT (ClinicalTrials.gov identifiers: NCT01746849 and NCT01338987), but results have not yet been reported.
Human recombinant KGF (palifermin) is approved by the US Food and Drug Administration for the prevention of mucositis in patients receiving high-dose chemotherapy including conditioning for HCT. In several mouse models KGF enhanced recovery of thymic cellularity and peripheral T cell numbers after HCT, reversed thymic involution and restored thympopoiesis (116, 174). Several trials are exploring its effects on T-cell reconstitution, but results have not been reported so far (ClinicalTrials.gov identifiers: NCT01233921, NCT03042585, NCT02356159, and NCT00593554).
Thymosin-alpha1 is a low molecular weight peptide produced by thymus epithelial cells, which can increase thymocyte maturation and boost T cell function as shown in several preclinical studies. In a phase I/II clinical trial the safety and efficacy of Thymosin-alpha1 was evaluated in 6 adult recipients of haploidentical HCTs for haematologic malignancies (ClinicalTrials.gov identifiers: NCT00580450) (181). An increase of peripheral T-cell numbers, an earlier appearance of pathogen-specific T cell responses as well as a significant improvement in phagocytosis and dendritic cell function was observed (181). However, to the best of our knowledge, there are no further trials ongoing exploring Thymosin-alpha 1 in the HCT setting.
Tissue Engineering
De novo T-cell generation is dependent on the continuous seeding of the thymus by T-lymphoid precursors. These T-lymphoid precursors must differentiate from donor-derived haematopoietic stem cells in the recipient bone marrow before they can home to the thymus via the peripheral blood. Since this process is compromised after HCT by damage to the thymus caused by total body irradiation, chemotherapy, infections and predominantly GvHD prophylaxis and treatment, it may take up to 2 years before T-cell neogenesis is re-established (3, 239, 240). Adoptive transfer of in vitro generated human T-lymphoid precursors is therefore a promising approach to shortcut this pathway by targeted injection of T-lymphoid progenitors.
An US group has developed a novel approach to expand a cytokine-dependent, haematopoietic progenitor cell population ex vivo by culturing primary haematopoietic stem and progenitor cells with fusion proteins comprising the transduction domain of the HIV-1 transactivation (Tat) protein and either MYC or B-cell lymphoma 2 (BCL-2) proteins (222). In both humans and mice, the ex vivo expanded cells gave rise to a self-renewing cell population following initial transplantation in vivo; serial transplantations of this cell population were able to support haematopoiesis. Based on these laboratory studies, a clinical trial has been initiated in Israel to assess the application of TBX-1400 in patients with severe combined immunodeficiency (human donor haematopoietic stem and progenitor cells that have been treated ex vivo with the protein transduction domain of the Tat fused to MYC, ClinicalTrials.gov identifier: NCT02860559).
Several other groups have developed systems to pre-differentiate T-lymphoid progenitors out of CD34+ haematopoietic stem cells, e.g., by using the canonical Notch ligand Delta-like (DL)-1, or more recently a French group using immobilised DL-4 (241). These techniques allow the in vitro generation of large amounts of T-cell progenitor cells with high T-lymphopoietic potential. When co-transplanted together with CD34+ haematopoietic stem cells, these committed precursors led to rapid T-cell engraftment within 28 days in a humanised mouse model (242). This protocol was improved in recent years to expand CD34+ cells from granulocyte colony-stimulating factor (G-CSF)-mobilised peripheral blood as well (189). After 7 days of in vitro culture, these cells expressed T-lineage-related, thymus homing and crosstalk genes as well as markers of early lymphoid commitment but do not show any TCR rearrangement. Remarkably, in a humanised mouse model, thymic engraftment occurred 4 weeks after intrahepatic injection of such precursors in comparison to 12 weeks after injection of uncultured, CD34+-selected haematopoietic progenitor cells (189, 243). Thus, T-lymphoid progenitors seems to allow thymic engraftment just 4 weeks after transfer, a result which has to be confirmed in the human setting. Since the injected precursors do not harbour any TCR rearrangement, they should allow the generation of a polyclonal and self-tolerant T-cell repertoire without increasing the risk of GvHD. A Phase I/II clinical trial was initiated recently to evaluate the safety and efficacy of human T lymphoid progenitor transfusion after haploidentical HCT in patients with severe combined immunodeficiency (Clinical trial identifier: NCT03879876).
In the case of an entirely a functional thymus, transplantation of postnatal allogeneic thymic tissue may be another option. This procedure improved thymic output in patients with complete DiGeorge syndrome (244, 245). Although this approach has not been tested so far after HCT, it has been investigated in patients with acquired immunodeficiency syndrome (246). However, in these patients, residual host T cells led to a high rate of thymic tissue rejection. Therefore, complete T-cell depletion prior to thymus transplantation is a potential requirement if this approach is to be trialled post HCT.
In summary, several strategies to accelerate recovery of T-cell immunity after allogeneic HCT are currently under clinical evaluation. Patients with prolonged immune dysfunction caused by chemotherapy, irradiation, infection and GvHD may benefit from a multifactorial approach. The combined use of optimised graft composition, soluble factors (IL-7, KGF, Thymosin-alpha-1), T-lymphoid progenitors or, in case of complete thymic involution, thymus tissue transplantation may be able to accelerate restoration of the T-cell compartment.
Final Remarks
Studies about the reconstitution kinetics of different cellular subsets after HCT have revealed important insights about the basic principles of this treatment. They helped us to understand the artificial immune ontology after HCT as well as the pathophysiology of GvHD, viral reactivation and other transplant-related complications. By continuous efforts to dissect the phenomenon of alloreactivity, IR studies have opened the door to understand the GvL effect, at least in part. In recent years, research on IR has evolved from merely descriptive studies into a highly dynamic and innovative field which actively shapes the future design of HCT. Novel insights have fostered the continuous evolution of T-cell-depletion techniques to a level by which HCTs employing this method now yield comparable results to T-replete HCTs. Clinical trials over the coming years will show whether adoptive transfer of memory DLI, veto TCM cells or selective allodepletion approaches will give superior results. Strategies of restoring thymic cellularity by soluble factors, targeted influx of committed lymphoid progenitors or tissue engineering not only intend to lift IR kinetics of adult patients to that of an infant but will beyond that impact on ageing research since thymic involution is considered a major contributor of immune senescence.
Future studies on IR will aim to develop more precise prediction models for complications such as GvHD, viral disease or relapse. To this end, multifactorial models of IR will have to take the complex interactions around HCT into account and include not only lymphocyte subset numbers but also other factors such as graft type, graft manipulation, HLA disparity and minor histocompatibility differences. The first examples of such multidimensional IR analyses have already been published (71, 82, 132). Control over IR with targeted interventions in a timely orchestrated fashion will help to reduce transplant-related morbidity and mortality and improve GvHD-free, relapse-free survival.
Author Contributions
AY, AS, AL, SN, and ME contributed specific chapters to this review article. All authors contributed to the article and approved the submitted version.
Funding
This study received funding from the St. Anna Children's Cancer Research Institute, Vienna, Austria. The funders were not involved in the study design, collection, analysis, interpretation of data, the writing of this article, or the decision to submit it for publication.
Conflict of Interest
The authors declare that the research was conducted in the absence of any commercial or financial relationships that could be construed as a potential conflict of interest.
Publisher's Note
All claims expressed in this article are solely those of the authors and do not necessarily represent those of their affiliated organizations, or those of the publisher, the editors and the reviewers. Any product that may be evaluated in this article, or claim that may be made by its manufacturer, is not guaranteed or endorsed by the publisher.
Acknowledgments
The authors thank Hannah Bridges for editorial assistance and Robert Wenzl, SMI Würzburg, for graphic design support with Figure 1.
References
1. Schneider LC, Antin JH, Weinstein H, Abrams JS, Pearce MK, Geha RS, et al. Lymphokine profile in bone marrow transplant recipients. Blood. (1991) 78:3076–80. doi: 10.1182/blood.V78.11.3076.bloodjournal78113076
2. Storek J, Ferrara S, Ku N, Giorgi JV, Champlin RE, Saxon A. B cell reconstitution after human bone marrow transplantation: recapitulation of ontogeny? Bone Marrow Transplant. (1993) 12:387–98.
3. Storek J, Geddes M, Khan F, Huard B, Helg C, Chalandon Y, et al. Reconstitution of the immune system after hematopoietic stem cell transplantation in humans. Semin Immunopathol. (2008) 30:425–37. doi: 10.1007/s00281-008-0132-5
4. Velardi E, Clave E, Arruda LCM, Benini F, Locatelli F, Toubert A. The role of the thymus in allogeneic bone marrow transplantation and the recovery of the peripheral T-cell compartment. Semin Immunopathol. (2021) 43:101–17. doi: 10.1007/s00281-020-00828-7
5. de Koning C, Nierkens S, Boelens JJ. Strategies before, during, and after hematopoietic cell transplantation to improve T-cell immune reconstitution. Blood. (2016) 128:2607–15. doi: 10.1182/blood-2016-06-724005
6. Velardi E, Tsai JJ, van den Brink MRM. T cell regeneration after immunological injury. Nat Rev Immunol. (2021) 21:277–91. doi: 10.1038/s41577-020-00457-z
7. de Koning C, Plantinga M, Besseling P, Boelens JJ, Nierkens S. Immune reconstitution after allogeneic hematopoietic cell transplantation in children. Biol Blood Marrow Tr. (2016) 22:195–206. doi: 10.1016/j.bbmt.2015.08.028
8. Cieri N, Oliveira G, Greco R, Forcato M, Taccioli C, Cianciotti B, et al. Generation of human memory stem T cells after haploidentical T-replete hematopoietic stem cell transplantation. Blood. (2015) 125:2865–74. doi: 10.1182/blood-2014-11-608539
9. Roberto A, Castagna L, Zanon V, Bramanti S, Crocchiolo R, McLaren JE, et al. Role of naive-derived T memory stem cells in T-cell reconstitution following allogeneic transplantation. Blood. (2015) 125:2855–64. doi: 10.1182/blood-2014-11-608406
10. de Koning C, Prockop S, van Roessel I, Kernan N, Klein E, Langenhorst J, et al. CD4+ T-cell reconstitution predicts survival outcomes after acute graft-versus-host-disease: a dual-center validation. Blood. (2021) 137:848–55. doi: 10.1182/blood.2020007905
11. van Roessel I, Prockop S, Klein E, Boulad F, Scaradavou A, Spitzer B, et al. Early CD4+T cell reconstitution as predictor of outcomes after allogeneic hematopoietic cell transplantation. Cytotherapy. (2020) 22:503–10. doi: 10.1016/j.jcyt.2020.05.005
12. Cherel M, Choufi B, Trauet J, Cracco P, Dessaint J-P, Yakoub-Agha I, et al. Naive subset develops the most important alloreactive response among human CD4+ T lymphocytes in human leukocyte antigen-identical related setting. Eur J Haematol. (2014) 92:491–6. doi: 10.1111/ejh.12283
13. Chang Y-J, Zhao X-Y, Huo M-R, Huang X-J. Expression of CD62L on donor CD4(+) T cells in allografts: correlation with graft-versus-host disease after unmanipulated allogeneic blood and marrow transplantation. J Clin Immunol. (2009) 29:696–704. doi: 10.1007/s10875-009-9293-9
14. Bleakley M, Heimfeld S, Loeb KR, Jones LA, Chaney C, Seropian S, et al. Outcomes of acute leukemia patients transplanted with naive T cell-depleted stem cell grafts. J Clin Invest. (2015) 125:2677–89. doi: 10.1172/JCI81229
15. Milano F, Appelbaum FR, Delaney C. Cord-blood transplantation in patients with minimal residual disease. N Engl J Med. (2016) 375:2204–5. doi: 10.1056/NEJMc1612872
16. Admiraal R, de Koning CCH, Lindemans CA, Bierings MB, Wensing AMJ, Versluys AB, et al. Viral reactivations and associated outcomes in the context of immune reconstitution after pediatric hematopoietic cell transplantation. J Allergy Clin Immunol. (2017) 140:1643–50.e9. doi: 10.1016/j.jaci.2016.12.992
17. Khandelwal P, Lane A, Chaturvedi V, Owsley E, Davies SM, Marmer D, et al. Peripheral blood CD38 bright CD8+ effector memory T cells predict acute graft-versus-host disease. Biol Blood Marrow Tr. (2015) 21:1215–22. doi: 10.1016/j.bbmt.2015.04.010
18. Waller EK, Logan BR, Fei M, Lee SJ, Confer D, Howard A, et al. Kinetics of immune cell reconstitution predict survival in allogeneic bone marrow and G-CSF–mobilized stem cell transplantation. Blood Adv. (2019) 3:2250–63. doi: 10.1182/bloodadvances.2018029892
19. Minculescu L, Marquart HV, Ryder LP, Andersen NS, Schjoedt I, Friis LS, et al. Improved overall survival, relapse-free-survival, and less graft-vs.-host-disease in patients with high immune reconstitution of TCR gamma delta cells 2 months after allogeneic stem cell transplantation. Front Immunol. (2019) 10:1997. doi: 10.3389/fimmu.2019.01997
20. Bae KW, Kim BE, Koh KN, Im HJ, Seo JJ. Factors influencing lymphocyte reconstitution after allogeneic hematopoietic stem cell transplantation in children. Korean J Hematol. (2012) 47:44–52. doi: 10.5045/kjh.2012.47.1.44
21. Chiesa R, Gilmour K, Qasim W, Adams S, Worth AJJ, Zhan H, et al. Omission of in vivo T-cell depletion promotes rapid expansion of naive CD4+ cord blood lymphocytes and restores adaptive immunity within 2 months after unrelated cord blood transplant. Br J Haematol. (2012) 156:656–6. doi: 10.1111/j.1365-2141.2011.08994.x
22. Fujimaki K, Maruta A, Yoshida M, Kodama F, Matsuzaki M, Fujisawa S, et al. Immune reconstitution assessed during five years after allogeneic bone marrow transplantation. Bone Marrow Transpl. (2001) 27:1275–81. doi: 10.1038/sj.bmt.1703056
23. Hiwarkar P, Hubank M, Qasim W, Chiesa R, Gilmour KC, Saudemont A, et al. Cord blood transplantation recapitulates fetal ontogeny with a distinct molecular signature that supports CD4+ T-cell reconstitution. Blood Adv. (2017) 1:2206–16. doi: 10.1182/bloodadvances.2017010827
24. Hennion-Tscheltzoff O, Leboeuf D, Gauthier S-D, Dupuis M, Assouline B, Gregoire A, et al. TCR triggering modulates the responsiveness and homeostatic proliferation of CD4+ thymic emigrants to IL-7 therapy. Blood. (2013) 121:4684–93. doi: 10.1182/blood-2012-09-458174
25. Kieper WC, Burghardt JT, Surh CD. A role for TCR affinity in regulating naive T cell homeostasis. J Immunol. (2004) 172:40–4. doi: 10.4049/jimmunol.172.1.40
26. Eyrich M, Lang P, Lal S, Bader P, Klingebiel T, Handgretinger R, et al. A prospective analysis of the pattern of immune reconstitution following transplantation of HLA-disparate hematopoietic stem cells from parental donors. Br J Haematol. (2001) 114:422–32. doi: 10.1046/j.1365-2141.2001.02934.x
27. Eyrich M, Croner T, Leiler C, Lang P, Bader P, Klingebiel T, et al. Distinct contributions of CD4+ and CD8+ naive and memory T-cell subsets to overall TCR-repertoire complexity following transplantation of T-cell depleted CD34 selected hematopoietic progenitor cells from unrelated donors. Blood. (2002) 100:1915–8. doi: 10.1182/blood-2001-11-0005
28. Adams SP, Kricke S, Ralph E, Gilmour N, Gilmour KC. A comparison of TRECs and flow cytometry for naive T cell quantification. Clin Exp Immunol. (2018) 191:198–202. doi: 10.1111/cei.13062
29. Gorski J, Yassai M, Zhu X, Kissela B, Keever C, Flomenberg N. Circulating T cell repertoire complexity in normal individuals and bone marrow recipients analyzed by CDR3 size spectratyping. Correlation with immune status. J Immunol. (1994) 152:5109–19.
30. Gkazi AS, Margetts BK, Attenborough T, Mhaldien L, Standing JF, Oakes T, et al. Clinical T cell receptor repertoire deep sequencing and analysis: an application to monitor immune reconstitution following cord blood transplantation. Front Immunol. (2018) 9:2547. doi: 10.3389/fimmu.2018.02547
31. Kuzich JA, Kankanige Y, Guinto J, Ryland G, McBean M, Wong E, et al. T cell receptor beta locus sequencing early post-allogeneic stem cell transplant identifies patients at risk of initial and recurrent cytomegalovirus infection. Bone Marrow Transpl. (2021) 56:2582–90. doi: 10.1038/s41409-021-01354-2
32. Clave E, Lisini D, Douay C, Giorgiani G, Busson M, Zecca M, et al. Thymic function recovery after unrelated donor cord blood or T-cell depleted HLA-haploidentical stem cell transplantation correlates with leukemia relapse. Front Immunol. (2013) 4:54. doi: 10.3389/fimmu.2013.00054
33. Li Y, Xu L. Evaluation of TCR repertoire diversity in patients after hematopoietic stem cell transplantation. Stem Cell Investig. (2015) 2:17. doi: 10.3978/j.issn.2306-9759.2015.09.01
34. Talvensaari K, Clave E, Douay C, Rabian C, Garderet L, Busson M, et al. A broad T-cell repertoire diversity and an efficient thymic function indicate a favorable long-term immune reconstitution after cord blood stem cell transplantation. Blood. (2002) 99:1458–64. doi: 10.1182/blood.V99.4.1458
35. Yew PY, Alachkar H, Yamaguchi R, Kiyotani K, Fang H, Yap KL, et al. Quantitative characterization of T-cell repertoire in allogeneic hematopoietic stem cell transplant recipients. Bone Marrow Transpl. (2015) 50:1227–34. doi: 10.1038/bmt.2015.133
36. Garderet L, Dulphy N, Douay C, Chalumeau N, Schaeffer V, Zilber MT, et al. The umbilical cord blood alphabeta T-cell repertoire: characteristics of a polyclonal and naive but completely formed repertoire. Blood. (1998) 91:340–6. doi: 10.1182/blood.V91.1.340.340_340_346
37. Eyrich M, Leiler C, Lang P, Schilbach K, Schumm M, Bader P, et al. A prospective comparison of immune reconstitution in pediatric recipients of positively selected CD34(+) peripheral blood stem cells from unrelated donors vs recipients of unmanipulated bone marrow from related donors. Bone Marrow Transplant. (2003) 32:379–90. doi: 10.1038/sj.bmt.1704158
38. van Heijst JWJ, Ceberio I, Lipuma LB, Samilo DW, Wasilewski GD, Gonzales AMR, et al. Quantitative assessment of T cell repertoire recovery after hematopoietic stem cell transplantation. Nat Med. (2013) 19:372–7. doi: 10.1038/nm.3100
39. Hazenberg MD, Otto SA, Cohen Stuart JW, Verschuren MC, Borleffs JC, Boucher CA, et al. Increased cell division but not thymic dysfunction rapidly affects the T-cell receptor excision circle content of the naive T cell population in HIV-1 infection. Nat Med. (2000) 6:1036–42. doi: 10.1038/79549
40. Weinberg K, Blazar BR, Wagner JE, Agura E, Hill BJ, Smogorzewska M, et al. Factors affecting thymic function after allogeneic hematopoietic stem cell transplantation. Blood. (2001) 97:1458–66. doi: 10.1182/blood.V97.5.1458
41. Fujishima N, Hirokawa M, Fujishima M, Yamashita J, Saitoh H, Ichikawa Y, et al. Skewed T cell receptor repertoire of Vdelta1(+) gammadelta T lymphocytes after human allogeneic haematopoietic stem cell transplantation and the potential role for Epstein-Barr virus-infected B cells in clonal restriction. Clin Exp Immunol. (2007) 149:70–9. doi: 10.1111/j.1365-2249.2007.03388.x
42. Hirokawa M, Horiuchi T, Kawabata Y, Kitabayashi A, Miura AB. Reconstitution of gammadelta T cell repertoire diversity after human allogeneic hematopoietic cell transplantation and the role of peripheral expansion of mature T cell population in the graft. Bone Marrow Transpl. (2000) 26:177–85. doi: 10.1038/sj.bmt.1702478
43. Godder KT, Henslee-Downey PJ, Mehta J, Park BS, Chiang KY, Abhyankar S, et al. Long term disease-free survival in acute leukemia patients recovering with increased gammadelta T cells after partially mismatched related donor bone marrow transplantation. Bone Marrow Transpl. (2007) 39:751–7. doi: 10.1038/sj.bmt.1705650
44. Lamb LS Jr, Musk P, Ye Z, van Rhee F, Geier SS, Tong JJ, et al. Human gammadelta(+) T lymphocytes have in vitro graft vs leukemia activity in the absence of an allogeneic response. Bone Marrow Transpl. (2001) 27:601–6. doi: 10.1038/sj.bmt.1702830
45. Scheper W, van Dorp S, Kersting S, Pietersma F, Lindemans C, Hol S, et al. γδT cells elicited by CMV reactivation after allo-SCT cross-recognize CMV and leukemia. Leukemia. (2013) 27:1328–38. doi: 10.1038/leu.2012.374
46. Gaballa A, Alagrafi F, Uhlin M, Stikvoort A. Revisiting the role of γδ T cells in Anti-CMV immune response after transplantation. Viruses. (2021) 13:1031. doi: 10.3390/v13061031
47. Nguyen VH, Shashidhar S, Chang DS, Ho L, Kambham N, Bachmann M, et al. The impact of regulatory T cells on T-cell immunity following hematopoietic cell transplantation. Blood. (2008) 111:945–53. doi: 10.1182/blood-2007-07-103895
48. Admiraal R, Lindemans CA, van Kesteren C, Bierings MB, Versluijs AB, Nierkens S, et al. Excellent T-cell reconstitution and survival depend on low ATG exposure after pediatric cord blood transplantation. Blood. (2016) 128:2734–41. doi: 10.1182/blood-2016-06-721936
49. Admiraal R, van Kesteren C, Jol-van der Zijde CM, Lankester AC, Bierings MB, Egberts TCG, et al. Association between anti-thymocyte globulin exposure and CD4+ immune reconstitution in paediatric haemopoietic cell transplantation: a multicentre, retrospective pharmacodynamic cohort analysis. Lancet Haematol. (2015) 2:e194–203. doi: 10.1016/S2352-3026(15)00045-9
50. Komanduri KV, St John LS, de Lima M, McMannis J, Rosinski S, McNiece I, et al. Delayed immune reconstitution after cord blood transplantation is characterized by impaired thymopoiesis and late memory T-cell skewing. Blood. (2007) 110:4543–51. doi: 10.1182/blood-2007-05-092130
51. Lindemans CA, Chiesa R, Amrolia PJ, Rao K, Nikolajeva O, de Wildt A, et al. Impact of thymoglobulin prior to pediatric unrelated umbilical cord blood transplantation on immune reconstitution and clinical outcome. Blood. (2014) 123:126–32. doi: 10.1182/blood-2013-05-502385
52. de Koning C, Gabelich J-A, Langenhorst J, Admiraal R, Kuball J, Boelens JJ, et al. Filgrastim enhances T-cell clearance by antithymocyte globulin exposure after unrelated cord blood transplantation. Blood Adv. (2018) 2:565–74. doi: 10.1182/bloodadvances.2017015487
53. Chen BJ, Cui X, Sempowski GD, Domen J, Chao NJ. Hematopoietic stem cell dose correlates with the speed of immune reconstitution after stem cell transplantation. Blood. (2004) 103:4344–52. doi: 10.1182/blood-2003-07-2534
54. Azuma E, Hirayama M, Yamamoto H, Komada Y. The role of donor age in naive T-cell recovery following allogeneic hematopoietic stem cell transplantation: the younger the better. Leuk Lymphoma. (2002) 43:735–9. doi: 10.1080/10428190290016827
55. Hirayama M, Azuma E, Jiang Q, Kobayashi M, Iwamoto S, Kumamoto T, et al. The reconstitution of CD45RBhiCD4+ naive T cells is inversely correlated with donor age in murine allogeneic haematopoietic stem cell transplantation. Br J Haematol. (2000) 111:700–7. doi: 10.1046/j.1365-2141.2000.02391.x
56. Savage WJ, Bleesing JJ, Douek D, Brown MR, Linton GM, Malech HL, et al. Lymphocyte reconstitution following non-myeloablative hematopoietic stem cell transplantation follows two patterns depending on age and donor/recipient chimerism. Bone Marrow Transplant. (2001) 28:463–71. doi: 10.1038/sj.bmt.1703176
57. Small TN, Papadopoulos EB, Boulad F, Black P, Castro Malaspina H, Childs BH, et al. Comparison of immune reconstitution after unrelated and related T-cell-depleted bone marrow transplantation: effect of patient age and donor leukocyte infusions. Blood. (1999) 93:467–80. doi: 10.1182/blood.V93.2.467
58. Storek J, Witherspoon RP, Storb R. T cell reconstitution after bone marrow transplantation into adult patients does not resemble T cell development in early life. Bone Marrow Transplant. (1995) 16:413–25.
59. Mackall CL, Hakim FT, Gress RE. Restoration of T-cell homeostasis after T-cell depletion. Semin Immunol. (1997) 9:339–46. doi: 10.1006/smim.1997.0091
60. Eyrich M, Wollny G, Tzaribaschev N, Dietz K, Brugger D, Bader P, et al. Onset of thymic recovery and plateau of thymic output are differentially regulated after stem cell transplantation in children. Biol Blood Marrow Transplant. (2005) 11:194–205. doi: 10.1016/j.bbmt.2004.12.001
61. Watkins BK, Horan J, Storer B, Martin PJ, Carpenter PA, Flowers ME. Recipient and donor age impact the risk of developing chronic GvHD in children after allogeneic hematopoietic transplant. Bone Marrow Transplant. (2017) 52:625–6. doi: 10.1038/bmt.2016.328
62. Nagler A, Labopin M, Houhou M, Aljurf M, Mousavi A, Hamladji R-M, et al. Outcome of haploidentical versus matched sibling donors in hematopoietic stem cell transplantation for adult patients with acute lymphoblastic leukemia: a study from the Acute Leukemia Working Party of the European Society for Blood and Marrow Transplantation. J Hematol Oncol. (2021) 14:53. doi: 10.1186/s13045-021-01065-7
63. Willasch AM, Peters C, Sedlacek P, Dalle J-H, Kitra-Roussou V, Yesilipek A, et al. Myeloablative conditioning for allo-HSCT in pediatric ALL: FTBI or chemotherapy?-A multicenter EBMT-PDWP study. Bone Marrow Transpl. (2020) 55:1540–51. doi: 10.1038/s41409-020-0854-0
64. Mahadeo KM, Masinsin B, Kapoor N, Shah AJ, Abdel-Azim H, Parkman R. Immunologic resolution of human chronic graft-versus-host disease. Biol Blood Marrow Transplant. (2014) 20:1508–15. doi: 10.1016/j.bbmt.2014.06.030
65. Bras AE, van den Heuvel-Eibrink MM, van der Sluijs-Gelling AJ, Coenen EA, Wind H, Zwaan CM, et al. No significant prognostic value of normal precursor B-cell regeneration in paediatric acute myeloid leukaemia after induction treatment. Br J Haematol. (2013) 161:861–4. doi: 10.1111/bjh.12329
66. Nakatani K, Imai K, Shigeno M, Sato H, Tezuka M, Okawa T, et al. Cord blood transplantation is associated with rapid B-cell neogenesis compared with BM transplantation. Bone Marrow Transplant. (2014) 49:1155–61. doi: 10.1038/bmt.2014.123
67. Wiegering V, Keupp A, Frietsch M, Fiessler C, Haas K, Haubitz I, et al. Role of B cells in chronic graft-versus-host disease after allogeneic stem cell transplantation in children and adolescents. Br J Haematol. (2019) 186:e133–7. doi: 10.1111/bjh.15977
68. Bohmann EM, Fehn U, Holler B, Weber D, Holler E, Herr W, et al. Altered immune reconstitution of B and T cells precedes the onset of clinical symptoms of chronic graft-versus-host disease and is influenced by the type of onset. Ann Hematol. (2017) 96:299–310. doi: 10.1007/s00277-016-2881-x
69. Glauzy S, Soret J, Fournier I, Douay C, Moins-Teisserenc H, Peffault de Latour R, et al. Impact of acute and chronic graft-versus-host disease on human B-cell generation and replication. Blood. (2014) 124:2459–62. doi: 10.1182/blood-2014-05-573303
70. Huenecke S, Cappel C, Esser R, Pfirrmann V, Salzmann-Manrique E, Betz S, et al. Development of three different nk cell subpopulations during immune reconstitution after pediatric allogeneic hematopoietic stem cell transplantation: prognostic markers in GvHD and viral infections. Front Immunol. (2017) 8:109. doi: 10.3389/fimmu.2017.00109
71. Salzmann-Manrique E, Bremm M, Huenecke S, Stech M, Orth A, Eyrich M, et al. Joint modeling of immune reconstitution post haploidentical stem cell transplantation in pediatric patients with acute leukemia comparing CD34(+)-selected to CD3/CD19-depleted grafts in a retrospective multicenter study. Front Immunol. (2018) 9:1841. doi: 10.3389/fimmu.2018.01841
72. Hangasky JA, Waldmann TA, Santi DV. Interleukin 15 pharmacokinetics and consumption by a dynamic cytokine sink. Front Immunol. (2020) 11:1813. doi: 10.3389/fimmu.2020.01813
73. Oevermann L, Michaelis SU, Mezger M, Lang P, Toporski J, Bertaina A, et al. KIR B haplotype donors confer a reduced risk for relapse after haploidentical transplantation in children with ALL. Blood. (2014) 124:2744–7. doi: 10.1182/blood-2014-03-565069
74. Locatelli F, Merli P, Pagliara D, Li Pira G, Falco M, Pende D, et al. Outcome of children with acute leukemia given HLA-haploidentical HSCT after alphabeta T-cell and B-cell depletion. Blood. (2017) 130:677–85. doi: 10.1182/blood-2017-04-779769
75. Locatelli F, Pende D, Falco M, Della Chiesa M, Moretta A, Moretta L. NK cells mediate a crucial graft-versus-leukemia effect in haploidentical-HSCT to cure high-risk acute leukemia. Trends Immunol. (2018) 39:577–90. doi: 10.1016/j.it.2018.04.009
76. Duver F, Weissbrich B, Eyrich M, Wolfl M, Schlegel PG, Wiegering V. Viral reactivations following hematopoietic stem cell transplantation in pediatric patients - a single center 11-year analysis. PLoS ONE. (2020) 15:e0228451. doi: 10.1371/journal.pone.0228451
77. Meyers JD, Flournoy N, Thomas ED. Cytomegalovirus infection and specific cell-mediated immunity after marrow transplant. J Infect Dis. (1980) 142:816–24. doi: 10.1093/infdis/142.6.816
78. Duke ER, Williamson BD, Borate B, Golob JL, Wychera C, Stevens-Ayers T, et al. CMV viral load kinetics as surrogate endpoints after allogeneic transplantation. J Clin Investig. (2021) 131:e133960. doi: 10.1172/JCI133960
79. van der Heiden PLJ, van Egmond HM, Veld SAJ, van de Meent M, Eefting M, de Wreede LC, et al. CMV seronegative donors: effect on clinical severity of CMV infection and reconstitution of CMV-specific immunity. Transpl Immunol. (2018) 49:54–8. doi: 10.1016/j.trim.2018.04.003
80. Aubert G, Hassan-Walker AF, Madrigal JA, Emery VC, Morte C, Grace S, et al. Cytomegalovirus-specific cellular immune responses and viremia in recipients of allogeneic stem cell transplants. J Infect Dis. (2001) 184:955–63. doi: 10.1086/323354
81. Leserer S, Bayraktar E, Trilling M, Bogdanov R, Arrieta-Bolaños E, Tsachakis-Mück N, et al. Cytomegalovirus kinetics after hematopoietic cell transplantation reveal peak titers with differential impact on mortality, relapse and immune reconstitution. Am J Hematol. (2021) 96:436–45. doi: 10.1002/ajh.26094
82. Itzykson R, Robin M, Moins-Teisserenc H, Delord M, Busson M, Xhaard A, et al. Cytomegalovirus shapes long-term immune reconstitution after allogeneic stem cell transplantation. Haematologica. (2015) 100:114–23. doi: 10.3324/haematol.2014.113415
83. Appleton AL, Sviland L. Pathogenesis of GVHD: role of herpes viruses. Bone Marrow Transplant. (1993) 11:349–55.
84. Cantoni N, Hirsch HH, Khanna N, Gerull S, Buser A, Bucher C, et al. Evidence for a bidirectional relationship between cytomegalovirus replication and acute graft-versus-host disease. Biol Blood Marrow Tr. (2010) 16:1309–14. doi: 10.1016/j.bbmt.2010.03.020
85. Stevanović S, van Bergen CAM, van Luxemburg-Heijs SAP, van der Zouwen B, Jordanova ES, Kruisselbrink AB, et al. HLA class II upregulation during viral infection leads to HLA-DP–directed graft-versus-host disease after CD4+ donor lymphocyte infusion. Blood. (2013) 122:1963–73. doi: 10.1182/blood-2012-12-470872
86. Hall CE, Koparde VN, Jameson-Lee M, Elnasseh AG, Scalora AF, Kobulnicky DJ, et al. Sequence homology between HLA-bound cytomegalovirus and human peptides: a potential trigger for alloreactivity. PLoS ONE. (2017) 12:e0178763. doi: 10.1371/journal.pone.0178763
87. Sperotto A, Candoni A, Gottardi M, Facchin G, Stella R, De Marchi R, et al. Cytomegalovirus prophylaxis versus pre-emptive strategy: different CD4+ and CD8+ T cell reconstitution after allogeneic hematopoietic stem cell transplantation. Transplant Cell Ther. (2021) 27:518.e1–4. doi: 10.1016/j.jtct.2021.03.003
88. Zamora D, Duke ER, Xie H, Edmison BC, Akoto B, Kiener R, et al. Cytomegalovirus-specific T-cell reconstitution following letermovir prophylaxis after hematopoietic cell transplantation. Blood. (2021) 138:34–43. doi: 10.1182/blood.2020009396
89. Clave E, Agbalika F, Bajzik V, Peffault de Latour R, Trillard M, Rabian C, et al. Epstein-barr virus (EBV) reactivation in allogeneic stem-cell transplantation: relationship between viral load, EBV-specific T-cell reconstitution and rituximab therapy. Transplantation. (2004) 77:76–84. doi: 10.1097/01.TP.0000093997.83754.2B
90. D'Aveni M, Aissi-Rothe L, Venard V, Salmon A, Falenga A, Decot V, et al. The clinical value of concomitant Epstein Barr virus (EBV)-DNA load and specific immune reconstitution monitoring after allogeneic hematopoietic stem cell transplantation. Transpl Immunol. (2011) 24:224–32. doi: 10.1016/j.trim.2011.03.002
91. Stocker N, Labopin M, Boussen I, Paccoud O, Bonnin A, Malard F, et al. Pre-emptive rituximab treatment for Epstein–Barr virus reactivation after allogeneic hematopoietic stem cell transplantation is a worthwhile strategy in high-risk recipients: a comparative study for immune recovery and clinical outcomes. Bone Marrow Transpl. (2020) 55:586–94. doi: 10.1038/s41409-019-0699-6
92. Hiwarkar P, Kosulin K, Cesaro S, Mikulska M, Styczynski J, Wynn R, et al. Management of adenovirus infection in patients after haematopoietic stem cell transplantation: state-of-the-art and real-life current approach. Rev Med Virol. (2018) 28:e1980. doi: 10.1002/rmv.1980
93. Ogata M, Oshima K, Ikebe T, Takano K, Kanamori H, Kondo T, et al. Clinical characteristics and outcome of human herpesvirus-6 encephalitis after allogeneic hematopoietic stem cell transplantation. Bone Marrow Transpl. (2017) 52:1563–70. doi: 10.1038/bmt.2017.175
94. de Koning C, Admiraal R, Nierkens S, Boelens JJ. Human herpesvirus 6 viremia affects T-cell reconstitution after allogeneic hematopoietic stem cell transplantation. Blood Adv. (2018) 2:428–32. doi: 10.1182/bloodadvances.2017012724
95. Patel SJ, Zhao G, Penna VR, Park E, Lauron EJ, Harvey IB, et al. A murine herpesvirus closely related to ubiquitous human Herpesviruses causes T-CELL DEPLETION. J Virol. (2017) 91:e02463–16. doi: 10.1128/JVI.02463-16
96. Cuvelier GDE, Nemecek ER, Wahlstrom JT, Kitko CL, Lewis VA, Schechter T, et al. Benefits and challenges with diagnosing chronic and late acute GVHD in children using the NIH consensus criteria. Blood. (2019) 134:304–16. doi: 10.1182/blood.2019000216
97. Lawitschka A, Gueclue ED, Januszko A, Körmöczi U, Rottal A, Fritsch G, et al. National institutes of health–defined chronic graft-vs.-host disease in pediatric hematopoietic stem cell transplantation patients correlates with parameters of long-term immune reconstitution. Front Immunol. (2019) 10:1879. doi: 10.3389/fimmu.2019.01879
98. Dekker L, de Koning C, Lindemans C, Nierkens S. Reconstitution of T cell subsets following allogeneic hematopoietic cell transplantation. Cancers. (2020) 12:1974. doi: 10.3390/cancers12071974
99. Forcina A, Noviello M, Carbone M, Bonini C, Bondanza A. Predicting the clinical outcome of allogeneic hematopoietic stem cell transplantation: the long and winding road toward validated immune biomarkers. Front Immunol. (2013) 4:71. doi: 10.3389/fimmu.2013.00071
100. Podgorny PJ, Liu Y, Dharmani-Khan P, Pratt LM, Jamani K, Luider J, et al. Immune cell subset counts associated with graft-versus-host disease. Biol Blood Marrow Tr. (2014) 20:450–62. doi: 10.1016/j.bbmt.2014.01.002
101. Clave E, Busson M, Douay C, Peffault de Latour R, Berrou J, Rabian C, et al. Acute graft-versus-host disease transiently impairs thymic output in young patients after allogeneic hematopoietic stem cell transplantation. Blood. (2009) 113:6477–84. doi: 10.1182/blood-2008-09-176594
102. Gaballa A, Clave E, Uhlin M, Toubert A, Arruda LCM. Evaluating thymic function after human hematopoietic stem cell transplantation in the personalized medicine era. Front Immunol. (2020) 11:1341. doi: 10.3389/fimmu.2020.01341
103. Magenau JM, Qin X, Tawara I, Rogers CE, Kitko C, Schlough M, et al. Frequency of CD4(+)CD25(hi)FOXP3(+) regulatory T cells has diagnostic and prognostic value as a biomarker for acute graft-versus-host-disease. Biol Blood Marrow Tr. (2010) 16:907–14. doi: 10.1016/j.bbmt.2010.02.026
104. Rezvani K, Mielke S, Ahmadzadeh M, Kilical Y, Savani BN, Zeilah J, et al. High donor FOXP3-positive regulatory T-cell (Treg) content is associated with a low risk of GVHD following HLA-matched allogeneic SCT. Blood. (2006) 108:1291–7. doi: 10.1182/blood-2006-02-003996
105. van der Maas NG, Berghuis D, van der Burg M, Lankester AC. B cell reconstitution and influencing factors after hematopoietic stem cell transplantation in children. Front Immunol. (2019) 10:782. doi: 10.3389/fimmu.2019.00782
106. Rubio M-T, Moreira-Teixeira L, Bachy E, Bouillié M, Milpied P, Coman T, et al. Early posttransplantation donor-derived invariant natural killer T-cell recovery predicts the occurrence of acute graft-versus-host disease and overall survival. Blood. (2012) 120:2144–54. doi: 10.1182/blood-2012-01-404673
107. Arruda LCM, Gaballa A, Uhlin M. Impact of γδ T cells on clinical outcome of hematopoietic stem cell transplantation: systematic review and meta-analysis. Blood Adv. (2019) 3:3436–48. doi: 10.1182/bloodadvances.2019000682
108. Watanabe N, Narita M, Furukawa T, Nakamura T, Yamahira A, Masuko M, et al. Kinetics of pDCs, mDCs, γδT cells and regulatory T cells in association with graft versus host disease after hematopoietic stem cell transplantation. Int J Lab Hematol. (2011) 33:378–90. doi: 10.1111/j.1751-553X.2011.01300.x
109. Kawaguchi K, Umeda K, Hiejima E, Iwai A, Mikami M, Nodomi S, et al. Influence of post-transplant mucosal-associated invariant T cell recovery on the development of acute graft-versus-host disease in allogeneic bone marrow transplantation. Int J Hematol. (2018) 108:66–75. doi: 10.1007/s12185-018-2442-2
110. Bhattacharyya A, Hanafi L-A, Sheih A, Golob JL, Srinivasan S, Boeckh MJ, et al. Graft-derived reconstitution of mucosal-associated invariant t cells after allogeneic hematopoietic cell transplantation. Biol Blood Marrow Tr. (2018) 24:242–51. doi: 10.1016/j.bbmt.2017.10.003
111. Gress RE, Emerson SG, Drobyski WR. Immune reconstitution: how it should work, what's broken, and why it matters. Biol Blood Marrow Tr. (2010) 16:S133–7. doi: 10.1016/j.bbmt.2009.10.003
112. Hauri-Hohl MM, Keller MP, Gill J, Hafen K, Pachlatko E, Boulay T, et al. Donor T-cell alloreactivity against host thymic epithelium limits T-cell development after bone marrow transplantation. Blood. (2007) 109:4080–8. doi: 10.1182/blood-2006-07-034157
113. Williams KM, Gress RE. Immune reconstitution and implications for immunotherapy following haematopoietic stem cell transplantation. Best Prac Res Clin Haematol. (2008) 21:579–96. doi: 10.1016/j.beha.2008.06.003
114. Lin M-T, Tseng L-H, Frangoul H, Gooley T, Pei J, Barsoukov A, et al. Increased apoptosis of peripheral blood T cells following allogeneic hematopoietic cell transplantation. Blood. (2000) 95:3832–9. doi: 10.1182/blood.V95.12.3832
115. Alpdogan SÖ, Lu SX, Patel N, McGoldrick S, Suh D, Budak-Alpdogan T, et al. Rapidly proliferating CD44hi peripheral T cells undergo apoptosis and delay posttransplantation T-cell reconstitution after allogeneic bone marrow transplantation. Blood. (2008) 112:4755–64. doi: 10.1182/blood-2008-02-142737
116. Min D, Taylor PA, Panoskaltsis-Mortari A, Chung B, Danilenko DM, Farrell C, et al. Protection from thymic epithelial cell injury by keratinocyte growth factor: a new approach to improve thymic and peripheral T-cell reconstitution after bone marrow transplantation. Blood. (2002) 99:4592–600. doi: 10.1182/blood.V99.12.4592
117. Krenger W, Holländer GA. The immunopathology of thymic GVHD. Semin Immunopathol. (2008) 30:439. doi: 10.1007/s00281-008-0131-6
118. Dulude G, Roy DC, Perreault C. The effect of graft-versus-host disease on T cell production and homeostasis. J Exp Med. (1999) 189:1329–42. doi: 10.1084/jem.189.8.1329
119. Gorski J, Chen X, Gendelman M, Yassai M, Krueger A, Tivol E, et al. Homeostatic expansion and repertoire regeneration of donor T cells during graft versus host disease is constrained by the host environment. Blood. (2007) 109:5502–10. doi: 10.1182/blood-2006-12-061713
120. Ogonek J, Kralj Juric M, Ghimire S, Varanasi PR, Holler E, Greinix H, et al. Immune reconstitution after allogeneic hematopoietic stem cell transplantation. Front Immunol. (2016) 7:507. doi: 10.3389/fimmu.2016.00507
121. Olkinuora H, Talvensaari K, Kaartinen T, Siitonen S, Saarinen-Pihkala U, Partanen J, et al. T cell regeneration in pediatric allogeneic stem cell transplantation. Bone Marrow Transpl. (2007) 39:149–56. doi: 10.1038/sj.bmt.1705557
122. Elfeky R, Lazareva A, Qasim W, Veys P. Immune reconstitution following hematopoietic stem cell transplantation using different stem cell sources. Expert Rev Clin Immunol. (2019) 15:735–51. doi: 10.1080/1744666X.2019.1612746
123. Fiorenza S, Turtle CJ. Associations between the gut microbiota, immune reconstitution, and outcomes of allogeneic hematopoietic stem cell transplantation. Immunometabolism. (2021) 3:e210004. doi: 10.20900/immunometab20210004
124. Wolf D, Bader CS, Barreras H, Copsel S, Pfeiffer BJ, Lightbourn CO, et al. Superior immune reconstitution using Treg-expanded donor cells versus PTCy treatment in preclinical HSCT models. JCI Insight. (2018) 3:e121717. doi: 10.1172/jci.insight.121717
125. Bolton HA, Zhu E, Terry AM, Guy TV, Koh W-P, Tan S-Y, et al. Selective Treg reconstitution during lymphopenia normalizes DC costimulation and prevents graft-versus-host disease. J Clin Investig. (2015) 125:3627–41. doi: 10.1172/JCI76031
126. Danby RD, Zhang W, Medd P, Littlewood TJ, Peniket A, Rocha V, et al. High proportions of regulatory T cells in PBSC grafts predict improved survival after allogeneic haematopoietic SCT. Bone Marrow Transpl. (2016) 51:110–8. doi: 10.1038/bmt.2015.215
127. Jain P, Tian X, Cordes S, Chen J, Cantilena CR, Bradley C, et al. Over-expression of PD-1 does not predict leukemic relapse after allogeneic stem cell transplantation. Biol Blood Marrow Tr. (2019) 25:216–22. doi: 10.1016/j.bbmt.2018.09.037
128. Doi T, Muro K, Ishii H, Kato T, Tsushima T, Takenoyama M, et al. A phase I study of the anti-CC chemokine receptor 4 antibody, mogamulizumab, in combination with nivolumab in patients with advanced or metastatic solid tumors. Clin Cancer Res. (2019) 25:6614–22. doi: 10.1158/1078-0432.CCR-19-1090
129. Abdel-Azim H, Elshoury A, Mahadeo KM, Parkman R, Kapoor N. Humoral immune reconstitution kinetics after allogeneic hematopoietic stem cell transplantation in children: a maturation block of IgM memory B cells may lead to impaired antibody immune reconstitution. Biol Blood Marrow Tr. (2017) 23:1437–46. doi: 10.1016/j.bbmt.2017.05.005
130. Schultz KR, Kariminia A, Ng B, Abdossamadi S, Lauener M, Nemecek ER, et al. Immune profile differences between chronic GVHD and late acute GVHD: results of the ABLE/PBMTC 1202 studies. Blood. (2020) 135:1287–98. doi: 10.1182/blood.2019003186
131. Ek T, Josefson M, Abrahamsson J. Multivariate analysis of the relation between immune dysfunction and treatment intensity in children with acute lymphoblastic leukemia. Pediatric Blood Cancer. (2011) 56:1078–87. doi: 10.1002/pbc.23043
132. Koenig M, Huenecke S, Salzmann-Manrique E, Esser R, Quaritsch R, Steinhilber D, et al. Multivariate analyses of immune reconstitution in children after allo-SCT: risk-estimation based on age-matched leukocyte sub-populations. Bone Marrow Transpl. (2010) 45:613–21. doi: 10.1038/bmt.2009.204
133. Mellgren K, Nierop AFM, Abrahamsson J. Use of multivariate immune reconstitution patterns to describe immune reconstitution after allogeneic stem cell transplantation in children. Biol Blood Marrow Tr. (2019) 25:2045–53. doi: 10.1016/j.bbmt.2019.06.018
134. von Bonin M, Bornhäuser M. Concise review: the bone marrow niche as a target of graft versus host disease. Stem Cells. (2014) 32:1420–8. doi: 10.1002/stem.1691
135. Martínez-Jaramillo G, Gómez-Morales E, Sánchez-Valle E, Mayani H. Severe hematopoietic alterations in vitro, in bone marrow transplant recipients who develop graft-versus-host disease. J Hematother Stem Cell Res. (2001) 10:347–54. doi: 10.1089/152581601750288957
136. Cutler C, Giri S, Jeyapalan S, Paniagua D, Viswanathan A, Antin JH. Acute and chronic graft-versus-host disease after allogeneic peripheral-blood stem-cell and bone marrow transplantation: a meta-analysis. J Clin Oncol. (2001) 19:3685–91. doi: 10.1200/JCO.2001.19.16.3685
137. Parkman R, Cohen G, Carter SL, Weinberg KI, Masinsin B, Guinan E, et al. Successful immune reconstitution decreases leukemic relapse and improves survival in recipients of unrelated cord blood transplantation. Biol Blood Marrow Tr. (2006) 12:919–27. doi: 10.1016/j.bbmt.2006.05.008
138. Wiegering V, Winkler B, Haubitz I, Wolfl M, Schlegel PG, Eyrich M. Lower TGFss serum levels and higher frequency of IFNgamma-producing T cells during early immune reconstitution in surviving children after allogeneic stem cell transplantation. Pediatr Blood Cancer. (2013) 60:121–8. doi: 10.1002/pbc.24208
139. Sullivan KM, Storb R, Buckner CD, Fefer A, Fisher L, Weiden PL, et al. Graft-versus-host disease as adoptive immunotherapy in patients with advanced hematologic neoplasms. N Engl J Med. (1989) 320:828–34. doi: 10.1056/NEJM198903303201303
140. Zheng H, Matte-Martone C, Li H, Anderson BE, Venketesan S, Sheng Tan H, et al. Effector memory CD4+ T cells mediate graft-versus-leukemia without inducing graft-versus-host disease. Blood. (2008) 111:2476–84. doi: 10.1182/blood-2007-08-109678
141. Li N, Matte-Martone C, Zheng H, Cui W, Venkatesan S, Tan HS, et al. Memory T cells from minor histocompatibility antigen-vaccinated and virus-immune donors improve GVL and immune reconstitution. Blood. (2011) 118:5965–76. doi: 10.1182/blood-2011-07-367011
142. Triplett BM, Shook DR, Eldridge P, Li Y, Kang G, Dallas M, et al. Rapid memory T-cell reconstitution recapitulating CD45RA-depleted haploidentical transplant graft content in patients with hematologic malignancies. Bone Marrow Transplant. (2015) 50:968–77. doi: 10.1038/bmt.2014.324
143. Hashimoto H, Kasteleiner P, Kressin J, Muller F, Buhring H-J, Handgretinger R, et al. Removal of CD276+ cells from haploidentical memory T-cell grafts significantly lowers the risk of GVHD. Bone Marrow Transpl. (2021) 56:2336–54. doi: 10.1038/s41409-021-01307-9
144. Granados DP, Sriranganadane D, Daouda T, Zieger A, Laumont CM, Caron-Lizotte O, et al. Impact of genomic polymorphisms on the repertoire of human MHC class I-associated peptides. Nat Commun. (2014) 5:3600. doi: 10.1038/ncomms4600
145. Griffioen M, van Bergen CA, Falkenburg JH. Autosomal minor histocompatibility antigens: how genetic variants create diversity in immune targets. Front Immunol. (2016) 7:100. doi: 10.3389/fimmu.2016.00100
146. Berger M, Wettstein PJ, Korngold R. T cell subsets involved in lethal graft-versus-host disease directed to immunodominant minor histocompatibility antigens. Transplantation. (1994) 57:1095–102. doi: 10.1097/00007890-199404150-00019
147. Chakraverty R, Eom HS, Sachs J, Buchli J, Cotter P, Hsu R, et al. Host MHC class II+ antigen-presenting cells and CD4 cells are required for CD8-mediated graft-versus-leukemia responses following delayed donor leukocyte infusions. Blood. (2006) 108:2106–13. doi: 10.1182/blood-2006-03-007427
148. Brochu S, Baron C, Hetu F, Roy DC, Perreault C. Oligoclonal expansion of CTLs directed against a restricted number of dominant minor histocompatibility antigens in hemopoietic chimeras. J Immunol. (1995) 155:5104–14.
149. Santos N, Rodriguez-Romanos R, Nieto JB, Buno I, Vallejo C, Jimenez-Velasco A, et al. UGT2B17 minor histocompatibility mismatch and clinical outcome after HLA-identical sibling donor stem cell transplantation. Bone Marrow Transplant. (2016) 51:79–82. doi: 10.1038/bmt.2015.207
150. Turpeinen H, Ojala PJ, Ojala K, Miettinen M, Volin L, Partanen J. Minor histocompatibility antigens as determinants for graft-versus-host disease after allogeneic haematopoietic stem cell transplantation. Int J Immunogenet. (2013) 40:495–501. doi: 10.1111/iji.12051
151. Spierings E, Kim YH, Hendriks M, Borst E, Sergeant R, Canossi A, et al. Multicenter analyses demonstrate significant clinical effects of minor histocompatibility antigens on GvHD and GvL after HLA-matched related and unrelated hematopoietic stem cell transplantation. Biol Blood Marrow Transplant. (2013) 19:1244–53. doi: 10.1016/j.bbmt.2013.06.001
152. Godfrey J, Bishop MR, Syed S, Hyjek E, Kline J. PD-1 blockade induces remissions in relapsed classical Hodgkin lymphoma following allogeneic hematopoietic stem cell transplantation. J Immuno Ther Cancer. (2017) 5:11. doi: 10.1186/s40425-017-0211-z
153. Davids MS, Kim HT, Bachireddy P, Costello C, Liguori R, Savell A, et al. Ipilimumab for patients with relapse after allogeneic transplantation. N Engl J Med. (2016) 375:143–53. doi: 10.1056/NEJMoa1601202
154. Davids MS, Kim HT, Costello C, Herrera AF, Locke FL, Maegawa RO, et al. A multicenter phase 1 study of nivolumab for relapsed hematologic malignancies after allogeneic transplantation. Blood. (2020) 135:2182–91. doi: 10.1182/blood.2019004710
155. Mutis T, Blokland E, Kester M, Schrama E, Goulmy E. Generation of minor histocompatibility antigen HA-1-specific cytotoxic T cells restricted by nonself HLA molecules: a potential strategy to treat relapsed leukemia after HLA-mismatched stem cell transplantation. Blood. (2002) 100:547–52. doi: 10.1182/blood-2002-01-0024
156. Schilbach K, Kerst G, Walter S, Eyrich M, Wernet D, Handgretinger R, et al. Cytotoxic minor histocompatibility antigen HA-1-specific CD8+ effector memory T cells: artificial APCs pave the way for clinical application by potent primary in vitro induction. Blood. (2005) 106:144–9. doi: 10.1182/blood-2004-07-2940
157. Oostvogels R, Kneppers E, Minnema MC, Doorn RC, Franssen LE, Aarts T, et al. Efficacy of host-dendritic cell vaccinations with or without minor histocompatibility antigen loading, combined with donor lymphocyte infusion in multiple myeloma patients. Bone Marrow Transplant. (2017) 52:228–37. doi: 10.1038/bmt.2016.250
158. Storek J, Gillespy T 3rd, Lu H, Joseph A, Dawson MA, Gough M, et al. Interleukin-7 improves CD4 T-cell reconstitution after autologous CD34 cell transplantation in monkeys. Blood. (2003) 101:4209–18. doi: 10.1182/blood-2002-08-2671
159. Fry TJ, Christensen BL, Komschlies KL, Gress RE, Mackall CL. Interleukin-7 restores immunity in athymic T-cell-depleted hosts. Blood. (2001) 97:1525–33. doi: 10.1182/blood.V97.6.1525
160. Andre-Schmutz I, Bonhomme D, Yates F, Malassis M, Selz F, Fischer A, et al. IL-7 effect on immunological reconstitution after HSCT depends on MHC incompatibility. Br J Haematol. (2004) 126:844–51. doi: 10.1111/j.1365-2141.2004.05134.x
161. Perales MA, Goldberg JD, Yuan J, Koehne G, Lechner L, Papadopoulos EB, et al. Recombinant human interleukin-7 (CYT107) promotes T-cell recovery after allogeneic stem cell transplantation. Blood. (2012) 120:4882–91. doi: 10.1182/blood-2012-06-437236
162. Li L, Hsu HC, Stockard CR, Yang P, Zhou J, Wu Q, et al. IL-12 inhibits thymic involution by enhancing IL-7- and IL-2-induced thymocyte proliferation. J Immunol. (2004) 172:2909–16. doi: 10.4049/jimmunol.172.5.2909
163. Chen T, Burke KA, Zhan Y, Wang X, Shibata D, Zhao Y. IL-12 facilitates both the recovery of endogenous hematopoiesis and the engraftment of stem cells after ionizing radiation. Exp Hematol. (2007) 35:203–13. doi: 10.1016/j.exphem.2006.10.002
164. Sauter CT, Bailey CP, Panis MM, Biswas CS, Budak-Alpdogan T, Durham A, et al. Interleukin-15 administration increases graft-versus-tumor activity in recipients of haploidentical hematopoietic SCT. Bone Marrow Transplant. (2013) 48:1237–42. doi: 10.1038/bmt.2013.47
165. Bailey CP, Budak-Alpdogan T, Sauter CT, Panis MM, Buyukgoz C, Jeng EK, et al. New interleukin-15 superagonist (IL-15SA) significantly enhances graft-versus-tumor activity. Oncotarget. (2017) 8:44366–78. doi: 10.18632/oncotarget.17875
166. Tormo A, Khodayarian F, Cui Y, Al-Chami E, Kanjarawi R, Noe B, et al. Interleukin-21 promotes thymopoiesis recovery following hematopoietic stem cell transplantation. J Hematol Oncol. (2017) 10:120. doi: 10.1186/s13045-017-0490-3
167. Dudakov JA, Hanash AM, Jenq RR, Young LF, Ghosh A, Singer NV, et al. Interleukin-22 drives endogenous thymic regeneration in mice. Science. (2012) 336:91–5. doi: 10.1126/science.1218004
168. Pan B, Zhang F, Lu Z, Li L, Shang L, Xia F, et al. Donor T-cell-derived interleukin-22 promotes thymus regeneration and alleviates chronic graft-versus-host disease in murine allogeneic hematopoietic cell transplant. Int Immunopharmacol. (2019) 67:194–201. doi: 10.1016/j.intimp.2018.12.023
169. Fry TJ, Sinha M, Milliron M, Chu YW, Kapoor V, Gress RE, et al. Flt3 ligand enhances thymic-dependent and thymic-independent immune reconstitution. Blood. (2004) 104:2794–800. doi: 10.1182/blood-2003-11-3789
170. Wils EJ, Braakman E, Verjans GM, Rombouts EJ, Broers AE, Niesters HG, et al. Flt3 ligand expands lymphoid progenitors prior to recovery of thymopoiesis and accelerates T cell reconstitution after bone marrow transplantation. J Immunol. (2007) 178:3551–7. doi: 10.4049/jimmunol.178.6.3551
171. Williams KM, Moore AR, Lucas PJ, Wang J, Bare CV, Gress RE. FLT3 ligand regulates thymic precursor cells and hematopoietic stem cells through interactions with CXCR4 and the marrow niche. Exp Hematol. (2017) 52:40–9. doi: 10.1016/j.exphem.2017.05.005
172. Alpdogan O, Muriglan SJ, Kappel BJ, Doubrovina E, Schmaltz C, Schiro R, et al. Insulin-like growth factor-I enhances lymphoid and myeloid reconstitution after allogeneic bone marrow transplantation. Transplantation. (2003) 75:1977–83. doi: 10.1097/01.TP.0000070167.81584.A2
173. Chu YW, Schmitz S, Choudhury B, Telford W, Kapoor V, Garfield S, et al. Exogenous insulin-like growth factor 1 enhances thymopoiesis predominantly through thymic epithelial cell expansion. Blood. (2008) 112:2836–46. doi: 10.1182/blood-2008-04-149435
174. Wang Y, Chen G, Qiao S, Ma X, Tang X, Sun A, et al. Keratinocyte growth factor enhanced immune reconstitution in murine allogeneic umbilical cord blood cell transplant. Leuk Lymphoma. (2011) 52:1556–66. doi: 10.3109/10428194.2011.573037
175. Seggewiss R, Einsele H. Hematopoietic growth factors including keratinocyte growth factor in allogeneic and autologous stem cell transplantation. Semin Hematol. (2007) 44:203–11. doi: 10.1053/j.seminhematol.2007.04.009
176. Rizwan R, Levine JE, Defor T, Ferarra JL, Weisdorf DJ, Blazar BR, et al. Peritransplant palifermin use and lymphocyte recovery after T-cell replete, matched related allogeneic hematopoietic cell transplantation. Am J Hematol. (2011) 86:879–82. doi: 10.1002/ajh.22136
177. Jacobson JM, Wang H, Bordi R, Zheng L, Gross BH, Landay AL, et al. A randomized controlled trial of palifermin (recombinant human keratinocyte growth factor) for the treatment of inadequate CD4+ T-lymphocyte recovery in patients with HIV-1 infection on antiretroviral therapy. J Acquir Immune Defic Syndr. (2014) 66:399–406. doi: 10.1097/QAI.0000000000000195
178. Lopes N, Vachon H, Marie J, Irla M. Administration of RANKL boosts thymic regeneration upon bone marrow transplantation. EMBO Mol Med. (2017) 9:835–51. doi: 10.15252/emmm.201607176
179. Montero-Herradon S, Garcia-Ceca J, Zapata AG. Altered maturation of medullary TEC in EphB-deficient thymi is recovered by RANK signaling stimulation. Front Immunol. (2018) 9:1020. doi: 10.3389/fimmu.2018.01020
180. Wils EJ, Rombouts EJ, van Mourik I, Spits H, Legrand N, Braakman E, et al. Stem cell factor consistently improves thymopoiesis after experimental transplantation of murine or human hematopoietic stem cells in immunodeficient mice. J Immunol. (2011) 187:2974–81. doi: 10.4049/jimmunol.1004209
181. Perruccio K, Bonifazi P, Topini F, Tosti A, Bozza S, Aloisi T, et al. Thymosin alpha1 to harness immunity to pathogens after haploidentical hematopoietic transplantation. Ann N Y Acad Sci. (2010) 1194:153–61. doi: 10.1111/j.1749-6632.2010.05486.x
182. Ding JH, Wang LL, Chen Z, Wang J, Yu ZP, Zhao G, et al. The role of Talpha1 on the infective patients after hematopoietic stem cell transplantation. Int J Hematol. (2013) 97:280–3. doi: 10.1007/s12185-012-1208-5
183. Velardi E, Dudakov JA, van den Brink MR. Sex steroid ablation: an immunoregenerative strategy for immunocompromised patients. Bone Marrow Transplant. (2015) 50(Suppl. 2):S77–81. doi: 10.1038/bmt.2015.101
184. Lai KP, Lai JJ, Chang P, Altuwaijri S, Hsu JW, Chuang KH, et al. Targeting thymic epithelia AR enhances T-cell reconstitution and bone marrow transplant grafting efficacy. Mol Endocrinol. (2013) 27:25–37. doi: 10.1210/me.2012-1244
185. Sutherland JS, Spyroglou L, Muirhead JL, Heng TS, Prieto-Hinojosa A, Prince HM, et al. Enhanced immune system regeneration in humans following allogeneic or autologous hemopoietic stem cell transplantation by temporary sex steroid blockade. Clin Cancer Res. (2008) 14:1138–49. doi: 10.1158/1078-0432.CCR-07-1784
186. Goldberg GL, Sutherland JS, Hammet MV, Milton MK, Heng TS, Chidgey AP, et al. Sex steroid ablation enhances lymphoid recovery following autologous hematopoietic stem cell transplantation. Transplantation. (2005) 80:1604–13. doi: 10.1097/01.tp.0000183962.64777.da
187. Chen BJ, Cui X, Sempowski GD, Chao NJ. Growth hormone accelerates immune recovery following allogeneic T-cell-depleted bone marrow transplantation in mice. Exp Hematol. (2003) 31:953–8. doi: 10.1016/S0301-472X(03)00196-6
188. Zakrzewski JL, Kochman AA, Lu SX, Terwey TH, Kim TD, Hubbard VM, et al. Adoptive transfer of T-cell precursors enhances T-cell reconstitution after allogeneic hematopoietic stem cell transplantation. Nat Med. (2006) 12:1039–47. doi: 10.1038/nm1463
189. Simons L, Ma K, de Chappedelaine C, Moiranghtem RD, Elkaim E, Olivre J, et al. Generation of adult human T-cell progenitors for immunotherapeutic applications. J Allergy Clin Immunol. (2018) 141:1491–4 e4. doi: 10.1016/j.jaci.2017.10.034
190. Bredenkamp N, Ulyanchenko S, O'Neill KE, Manley NR, Vaidya HJ, Blackburn CC. An organized and functional thymus generated from FOXN1-reprogrammed fibroblasts. Nat Cell Biol. (2014) 16:902–8. doi: 10.1038/ncb3023
191. Parent AV, Russ HA, Khan IS, LaFlam TN, Metzger TC, Anderson MS, et al. Generation of functional thymic epithelium from human embryonic stem cells that supports host T cell development. Cell Stem Cell. (2013) 13:219–29. doi: 10.1016/j.stem.2013.04.004
192. Otsuka R, Wada H, Tsuji H, Sasaki A, Murata T, Itoh M, et al. Efficient generation of thymic epithelium from induced pluripotent stem cells that prolongs allograft survival. Sci Rep. (2020) 10:224. doi: 10.1038/s41598-019-57088-1
193. Choi DW, Cho KA, Lee HJ, Kim YH, Woo KJ, Park JW, et al. Cotransplantation of tonsilderived mesenchymal stromal cells in bone marrow transplantation promotes thymus regeneration and T cell diversity following cytotoxic conditioning. Int J Mol Med. (2020) 46:1166–74. doi: 10.3892/ijmm.2020.4657
194. Batorov EV, Shevela EY, Tikhonova MA, Batorova DS, Ushakova GY, Sizikova SA, et al. Mesenchymal stromal cells improve early lymphocyte recovery and T cell reconstitution after autologous hematopoietic stem cell transplantation in patients with malignant lymphomas. Cell Immunol. (2015) 297:80–6. doi: 10.1016/j.cellimm.2015.07.001
195. Ophir E, Or-Geva N, Gurevich I, Tal O, Eidelstein Y, Shezen E, et al. Murine anti-third-party central-memory CD8(+) T cells promote hematopoietic chimerism under mild conditioning: lymph-node sequestration and deletion of anti-donor T cells. Blood. (2013) 121:1220–8. doi: 10.1182/blood-2012-07-441493
196. Lask A, Ophir E, Or-Geva N, Cohen-Fredarow A, Afik R, Eidelstein Y, et al. A new approach for eradication of residual lymphoma cells by host nonreactive anti-third-party central memory CD8 T cells. Blood. (2013) 121:3033–40. doi: 10.1182/blood-2012-06-432443
197. Edinger M, Hoffmann P, Ermann J, Drago K, Fathman CG, Strober S, et al. CD4+CD25+ regulatory T cells preserve graft-versus-tumor activity while inhibiting graft-versus-host disease after bone marrow transplantation. Nat Med. (2003) 9:1144–50. doi: 10.1038/nm915
198. Riegel C, Boeld TJ, Doser K, Huber E, Hoffmann P, Edinger M. Efficient treatment of murine acute GvHD by in vitro expanded donor regulatory T cells. Leukemia. (2020) 34:895–908. doi: 10.1038/s41375-019-0625-3
199. Wertheimer T, Velardi E, Tsai J, Cooper K, Xiao S, Kloss CC, et al. Production of BMP4 by endothelial cells is crucial for endogenous thymic regeneration. Sci Immunol. (2018) 3:eaal2736. doi: 10.1126/sciimmunol.aal2736
200. Bortolomai I, Sandri M, Draghici E, Fontana E, Campodoni E, Marcovecchio GE, et al. Gene modification and three-dimensional scaffolds as novel tools to allow the use of postnatal thymic epithelial cells for thymus regeneration approaches. Stem Cells Transl Med. (2019) 8:1107–22. doi: 10.1002/sctm.18-0218
201. Shah NJ, Mao AS, Shih TY, Kerr MD, Sharda A, Raimondo TM, et al. An injectable bone marrow-like scaffold enhances T cell immunity after hematopoietic stem cell transplantation. Nat Biotechnol. (2019) 37:293–302. doi: 10.1038/s41587-019-0017-2
202. Kolb HJ, Mittermuller J, Clemm C, Holler E, Ledderose G, Brehm G, et al. Donor leukocyte transfusions for treatment of recurrent chronic myelogenous leukemia in marrow transplant patients. Blood. (1990) 76:2462–5. doi: 10.1182/blood.V76.12.2462.2462
203. Barrett AJ, Prockop S, Bollard CM. Virus-Specific T Cells: Broadening Applicability. Biol Blood Marrow Transplant. (2018) 24:13–8. doi: 10.1016/j.bbmt.2017.10.004
204. Bollard CM, Heslop HE. T cells for viral infections after allogeneic hematopoietic stem cell transplant. Blood. (2016) 127:3331–40. doi: 10.1182/blood-2016-01-628982
205. Creidy R, Moshous D, Touzot F, Elie C, Neven B, Gabrion A, et al. Specific T cells for the treatment of cytomegalovirus and/or adenovirus in the context of hematopoietic stem cell transplantation. J Allergy Clin Immunol. (2016) 138:920–4 e3. doi: 10.1016/j.jaci.2016.03.032
206. Feuchtinger T, Matthes-Martin S, Richard C, Lion T, Fuhrer M, Hamprecht K, et al. Safe adoptive transfer of virus-specific T-cell immunity for the treatment of systemic adenovirus infection after allogeneic stem cell transplantation. Br J Haematol. (2006) 134:64–76. doi: 10.1111/j.1365-2141.2006.06108.x
207. Ciceri F, Bonini C, Stanghellini MT, Bondanza A, Traversari C, Salomoni M, et al. Infusion of suicide-gene-engineered donor lymphocytes after family haploidentical haemopoietic stem-cell transplantation for leukaemia (the TK007 trial): a non-randomised phase I-II study. Lancet Oncol. (2009) 10:489–500.
208. Zhou X, Dotti G, Krance RA, Martinez CA, Naik S, Kamble RT, et al. Inducible caspase-9 suicide gene controls adverse effects from alloreplete T cells after haploidentical stem cell transplantation. Blood. (2015) 125:4103–13. doi: 10.1182/blood-2015-02-628354
209. Teschner D, Distler E, Wehler D, Frey M, Marandiuc D, Langeveld K, et al. Depletion of naive T cells using clinical grade magnetic CD45RA beads: a new approach for GVHD prophylaxis. Bone Marrow Transplant. (2014) 49:138–44. doi: 10.1038/bmt.2013.114
210. Touzot F, Neven B, Dal-Cortivo L, Gabrion A, Moshous D, Cros G, et al. CD45RA depletion in HLA-mismatched allogeneic hematopoietic stem cell transplantation for primary combined immunodeficiency: a preliminary study. J Allergy Clin Immunol. (2015) 135:1303–9 e1–3. doi: 10.1016/j.jaci.2014.08.019
211. Yuste JR, Lopez-Diaz de Cerio A, Rifon J, Moreno C, Panizo M, Inoges S. Adoptive T-cell therapy with CD45RA-depleted donor in the treatment of cytomegalovirus disease in immunocompromised non-transplant patients. Antivir Ther. (2019) 24:313–9. doi: 10.3851/IMP3307
212. Andre-Schmutz I, Le Deist F, Hacein-Bey-Abina S, Vitetta E, Schindler J, Chedeville G, et al. Immune reconstitution without graft-versus-host disease after haemopoietic stem-cell transplantation: a phase 1/2 study. Lancet. (2002) 360:130–7. doi: 10.1016/S0140-6736(02)09413-8
213. Amrolia PJ, Mucioli-Casadei G, Huls H, Heslop HE, Schindler J, Veys P, et al. Add-back of allodepleted donor T cells to improve immune reconstitution after haplo-identical stem cell transplantation. Cytotherapy. (2005) 7:116–25. doi: 10.1080/14653240510018181
214. Mielke S, Nunes R, Rezvani K, Fellowes VS, Venne A, Solomon SR, et al. A clinical-scale selective allodepletion approach for the treatment of HLA-mismatched and matched donor-recipient pairs using expanded T lymphocytes as antigen-presenting cells and a TH9402-based photodepletion technique. Blood. (2008) 111:4392–402. doi: 10.1182/blood-2007-08-104471
215. Roy DC, Lachance S, Cohen S, Delisle JS, Kiss T, Sauvageau G, et al. Allodepleted T-cell immunotherapy after haploidentical haematopoietic stem cell transplantation without severe acute graft-versus-host disease (GVHD) in the absence of GVHD prophylaxis. Br J Haematol. (2019) 186:754–66. doi: 10.1111/bjh.15970
216. Di Ianni M, Falzetti F, Carotti A, Terenzi A, Castellino F, Bonifacio E, et al. Tregs prevent GVHD and promote immune reconstitution in HLA-haploidentical transplantation. Blood. (2011) 117:3921–8. doi: 10.1182/blood-2010-10-311894
217. Martelli MF, Di Ianni M, Ruggeri L, Falzetti F, Carotti A, Terenzi A, et al. HLA-haploidentical transplantation with regulatory and conventional T-cell adoptive immunotherapy prevents acute leukemia relapse. Blood. (2014) 124:638–44. doi: 10.1182/blood-2014-03-564401
218. Reisner Y, Or-Geva N. Veto cells for safer nonmyeloablative haploidentical HSCT and CAR T cell therapy. Semin Hematol. (2019) 56:173–82. doi: 10.1053/j.seminhematol.2019.03.003
219. Sheikh V, Porter BO, DerSimonian R, Kovacs SB, Thompson WL, Perez-Diez A, et al. Administration of interleukin-7 increases CD4 T cells in idiopathic CD4 lymphocytopenia. Blood. (2016) 127:977–88. doi: 10.1182/blood-2015-05-645077
220. Napolitano LA, Schmidt D, Gotway MB, Ameli N, Filbert EL, Ng MM, et al. Growth hormone enhances thymic function in HIV-1-infected adults. J Clin Invest. (2008) 118:1085–98. doi: 10.1172/JCI32830
221. Sirohi B, Powles R, Morgan G, Treleaven J, Kulkarni S, Horton C, et al. Use of physiological doses of human growth hormone in haematological patients receiving intensive chemotherapy promotes haematopoietic recovery: a double-blind randomized, placebo-controlled study. Bone Marrow Transplant. (2007) 39:115–20. doi: 10.1038/sj.bmt.1705545
222. Bird GA, Polsky A, Estes P, Hanlon T, Hamilton H, Morton JJ, et al. Expansion of human and murine hematopoietic stem and progenitor cells ex vivo without genetic modification using MYC and Bcl-2 fusion proteins. PLoS ONE. (2014) 9:e105525. doi: 10.1371/journal.pone.0105525
223. Reisner Y, Kapoor N, Kirkpatrick D, Pollack MS, Dupont B, Good RA, et al. Transplantation for acute leukaemia with HLA-A and B nonidentical parental marrow cells fractionated with soybean agglutinin and sheep red blood cells. Lancet. (1981) 2:327–31. doi: 10.1016/S0140-6736(81)90647-4
224. Urbano-Ispizua A, Rozman C, Pimentel P, Solano C, de la Rubia J, Brunet S, et al. The number of donor CD3(+) cells is the most important factor for graft failure after allogeneic transplantation of CD34(+) selected cells from peripheral blood from HLA-identical siblings. Blood. (2001) 97:383–7. doi: 10.1182/blood.V97.2.383
225. Lang P, Feuchtinger T, Teltschik HM, Schwinger W, Schlegel P, Pfeiffer M, et al. Improved immune recovery after transplantation of TCRalphabeta/CD19-depleted allografts from haploidentical donors in pediatric patients. Bone Marrow Transpl. (2015) 50(Suppl. 2):S6–10. doi: 10.1038/bmt.2015.87
226. Nagler A, Ruggeri A. Haploidentical stem cell transplantation (HaploSCT) for patients with acute leukemia-an update on behalf of the ALWP of the EBMT. Bone Marrow Transplant. (2019) 54(Suppl. 2):713–8. doi: 10.1038/s41409-019-0610-5
227. Ruggeri A, Labopin M, Bacigalupo A, Gulbas Z, Koc Y, Blaise D, et al. Bone marrow versus mobilized peripheral blood stem cells in haploidentical transplants using posttransplantation cyclophosphamide. Cancer. (2018) 124:1428–37. doi: 10.1002/cncr.31228
228. Eiz-Vesper B, Maecker-Kolhoff B, Blasczyk R. Adoptive T-cell immunotherapy from third-party donors: characterization of donors and set up of a T-cell donor registry [Review]. Front Immunol. (2013) 3:410. doi: 10.3389/fimmu.2012.00410
229. Tzannou I, Papadopoulou A, Naik S, Leung K, Martinez CA, Ramos CA, et al. Off-the-shelf virus-specific T cells to treat BK virus, human herpesvirus 6, cytomegalovirus, epstein-barr virus, and adenovirus infections after allogeneic hematopoietic stem-cell transplantation. J Clin Oncol. (2017) 35:3547–57. doi: 10.1200/JCO.2017.73.0655
230. Or-Geva N, Reisner Y. The evolution of T-cell depletion in haploidentical stem-cell transplantation. Br J Haematol. (2016) 172:667–84. doi: 10.1111/bjh.13868
231. Aversa F, Bachar-Lustig E, Or-Geva N, Prezioso L, Bonomini S, Manfra I, et al. Immune tolerance induction by nonmyeloablative haploidentical HSCT combining T-cell depletion and posttransplant cyclophosphamide. Blood Adv. (2017) 1:2166–75. doi: 10.1182/bloodadvances.2017009423
232. Ulbar F, Villanova I, Giancola R, Baldoni S, Guardalupi F, Fabi B, et al. Clinical-grade expanded regulatory T cells are enriched with highly suppressive cells producing IL-10, granzyme B, and IL-35. Biol Blood Marrow Tr. (2020) 26:2204–10. doi: 10.1016/j.bbmt.2020.08.034
233. Elkeky R, Jacobsohn DA, Agarwal R, Naik S, Kapoor N, Krishnamurti L, et al. Administration of rimiducid following haploidentical BPX-501 cells in children with malignant or non-malignant disorders who develop graft-versus-host-disease (GvHD). Blood. (2018) 132(Suppl. 1):2207–7. doi: 10.1182/blood-2018-99-119792
234. Alpdogan O, van den Brink MR. IL-7 and IL-15: therapeutic cytokines for immunodeficiency. Trends Immunol. (2005) 26:56–64. doi: 10.1016/j.it.2004.11.002
235. Thiant S, Moutuou MM, Leboeuf D, Guimond M. Homeostatic cytokines in immune reconstitution and graft-versus-host disease. Cytokine. (2016) 82:24–32. doi: 10.1016/j.cyto.2016.01.003
236. Moutuou MM, Page G, Zaid I, Lesage S, Guimond M. Restoring T cell homeostasis after allogeneic stem cell transplantation; principal limitations and future challenges. Front Immunol. (2018) 9:1237. doi: 10.3389/fimmu.2018.01237
237. Goldberg GL, King CG, Nejat RA, Suh DY, Smith OM, Bretz JC, et al. Luteinizing hormone-releasing hormone enhances T cell recovery following allogeneic bone marrow transplantation. J Immunol. (2009) 182:5846–54. doi: 10.4049/jimmunol.0801458
238. Rossi SW, Jeker LT, Ueno T, Kuse S, Keller MP, Zuklys S, et al. Keratinocyte growth factor (KGF) enhances postnatal T-cell development via enhancements in proliferation and function of thymic epithelial cells. Blood. (2007) 109:3803–11. doi: 10.1182/blood-2006-10-049767
239. Bosch M, Dhadda M, Hoegh-Petersen M, Liu Y, Hagel LM, Podgorny P, et al. Immune reconstitution after anti-thymocyte globulin-conditioned hematopoietic cell transplantation. Cytotherapy. (2012) 14:1258–75. doi: 10.3109/14653249.2012.715243
240. Friedrich W, Honig M, Schulz A, Muller SM. Immune reconstitution in congenital disorders after HLA-haploidentical hemopoietic stem cell transplantation. Blood Cells Mol Dis. (2004) 33:291–3. doi: 10.1016/j.bcmd.2004.08.020
241. Reimann C, Six E, Dal-Cortivo L, Schiavo A, Appourchaux K, Lagresle-Peyrou C, et al. Human T-lymphoid progenitors generated in a feeder-cell-free Delta-like-4 culture system promote T-cell reconstitution in NOD/SCID/gammac(-/-) mice. Stem Cells. (2012) 30:1771–80. doi: 10.1002/stem.1145
242. Eyrich M, Schreiber SC, Wollny G, Ziegler H, Schlenker R, Koch-Buttner K, et al. Pre-differentiated human committed T-lymphoid progenitors promote peripheral T-cell re-constitution after stem cell transplantation in immunodeficient mice. Eur J Immunol. (2011) 41:3596–603. doi: 10.1002/eji.201141561
243. Simons L, Cavazzana M, Andre I. Concise review: boosting t-cell reconstitution following allogeneic transplantation-current concepts and future perspectives. Stem Cells Transl Med. (2019) 8:650–7. doi: 10.1002/sctm.18-0248
244. Davies EG, Cheung M, Gilmour K, Maimaris J, Curry J, Furmanski A, et al. Thymus transplantation for complete DiGeorge syndrome: European experience. J Allergy Clin Immunol. (2017) 140:1660–70.e16. doi: 10.1016/j.jaci.2017.03.020
245. Markert ML, Devlin BH, Chinn IK, McCarthy EA. Thymus transplantation in complete DiGeorge anomaly. Immunol Res. (2009) 44:61–70. doi: 10.1007/s12026-008-8082-5
Keywords: immune reconstitution, thymic function, peripheral expansion, T-cell receptor repertoire diversity, graft-vs.-host disease, graft-vs.-leukaemia effect, infectious complications
Citation: Yanir A, Schulz A, Lawitschka A, Nierkens S and Eyrich M (2022) Immune Reconstitution After Allogeneic Haematopoietic Cell Transplantation: From Observational Studies to Targeted Interventions. Front. Pediatr. 9:786017. doi: 10.3389/fped.2021.786017
Received: 29 September 2021; Accepted: 13 December 2021;
Published: 11 January 2022.
Edited by:
Adriana Balduzzi, Clinica Pediatrica Università degli Studi di Milano Bicocca, ItalyReviewed by:
Suhag Parikh, Emory University, United StatesShigeo Fuji, Osaka International Cancer Institute, Japan
Copyright © 2022 Yanir, Schulz, Lawitschka, Nierkens and Eyrich. This is an open-access article distributed under the terms of the Creative Commons Attribution License (CC BY). The use, distribution or reproduction in other forums is permitted, provided the original author(s) and the copyright owner(s) are credited and that the original publication in this journal is cited, in accordance with accepted academic practice. No use, distribution or reproduction is permitted which does not comply with these terms.
*Correspondence: Matthias Eyrich, ZXlyaWNoX20mI3gwMDA0MDt1a3cuZGU=