- 1Department of Pediatrics, University of Texas Southwestern Medical Center, Dallas, TX, United States
- 2Department of Psychiatry, University of Texas Southwestern Medical Center, Dallas, TX, United States
Cerebrovascular pressure autoregulation promotes stable cerebral blood flow (CBF) across a range of arterial blood pressures. Cerebral autoregulation (CA) is a developmental process that reaches maturity around term gestation and can be monitored prenatally with both Doppler ultrasound and magnetic resonance imaging (MRI) techniques. Postnatally, there are key advantages and limitations to assessing CA with Doppler ultrasound, MRI, and near-infrared spectroscopy. Here we review these CBF monitoring techniques as well as their application to both fetal and neonatal populations at risk of perturbations in CBF. Specifically, we discuss CBF monitoring in fetuses with intrauterine growth restriction, anemia, congenital heart disease, neonates born preterm and those with hypoxic-ischemic encephalopathy. We conclude the review with insights into the future directions in this field with an emphasis on collaborative science and precision medicine approaches.
Highlights
- Cerebral autoregulation is a developmental process that can be disrupted in neonates with congenital heart disease, hypoxic-ischemic encephalopathy, and those born preterm.
- Novel methods to assess cerebral autoregulation in these populations can be used to target patient-specific hemodynamic parameters.
Introduction
Cerebral autoregulation (CA) is the physiologic adaptation in cerebrovascular resistance across a range of cerebral perfusion pressure (CPP; typically estimated using mean arterial pressure, MAP) in order to promote stable cerebral blood flow (CBF). The maturation and differences in these response mechanisms in fetuses and neonates, particularly those at highest risk for cerebrovascular abnormalities, is poorly understood. Early studies in the chronically instrumented fetal sheep helped shape our current understanding of cerebral hemodynamics in the fetus (1–6). Many of these findings have not yet been confirmed in the human fetus owing to the lack of non-invasive methods other than Doppler ultrasound, which has key limitations, until recent innovations in advanced fetal MRI. This review will explore data on both traditional and novel methods for monitoring CBF and CA in the fetus and neonates with a specific focus on those with pathology that makes them vulnerable to perturbations in CBF (Figure 1).
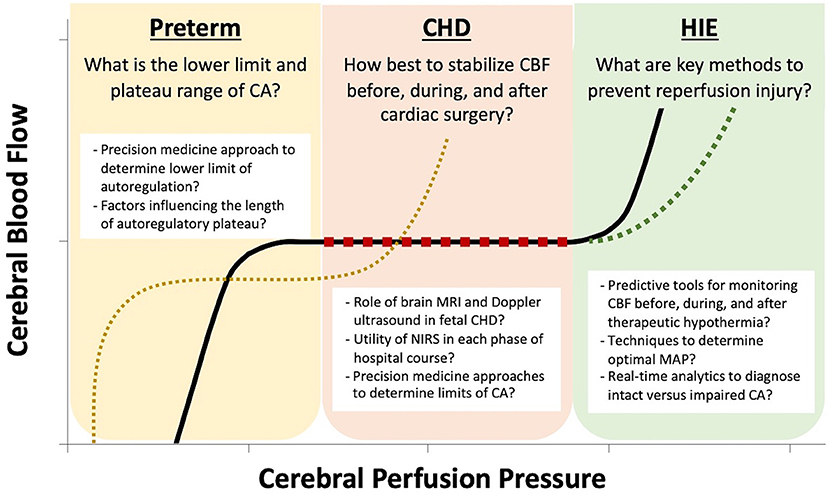
Figure 1. The key questions regarding cerebral autoregulation (CA) are unique to each neonatal pathophysiology. In the preterm neonate, due to immature mechanisms of cerebrovascular adaptation, there is a limited plateau of stable cerebral blood flow (CBF) and there is no strong evidence to guide the optimal lower limit of cerebral perfusion pressure (CPP) by gestational age. In the neonate with congenital heart disease (CHD), postnatal hypoxia and stabilization of CBF before, during, and after cardiac surgery are the prominent challenges. For neonates with hypoxic ischemic encephalopathy (HIE), avoidance of reperfusion injury plays a key role in limiting neurologic damage.
Physiology of Cerebral Blood Flow
CBF is highly related to cerebral metabolism and arterial blood gases. Cerebral vasculature is particularly sensitive to the partial pressure of carbon dioxide (PaCO2), and this reactivity is present in the late-gestation fetus and neonate as well (7). Vascular sensitivity to PaCO2 occurs throughout the cerebrovasculature, from the major feeding vessels in the neck to the parenchymal and pial arterioles (8). Hypercapnia has the well-known effect of vasodilation while hypocapnia results in cerebrovascular constriction, both of which occur through changes in the local extracellular pH surrounding smooth muscle cells of vessels (9). The sensitivity of the cerebrovasculature to hypoxia is less pronounced and is mediated through adenosine and nitric oxide synthase. Nitric oxide (NO) is a potent vasodilator of cerebrovasculature and is produced by a variety of cell types within the central nervous system, with many studies showing NO-mediated fluctuations in cerebrovascular tone result from not only hypoxia, but multiple stimuli including hypercapnia, cytokines, and activation of the parasympathetic fibers surrounding vessels (10).
Fetal cerebral arteries differ in both structure and function from their adult counterparts (11). The development of the vascular smooth muscle cell layers form around 20–24 weeks gestation and are first evident in vessels that later become the pial arteries (11). The appearance of the muscularis layer of cerebral arteries progresses from pial vessels inwards and concludes around term gestation (12). Pial vessels are traditionally considered the primary site of CBF autoregulation. Capillary-level regulation of CBF may also occur through poorly understood mechanisms involving pericytes (13, 14), which are the cells that make up the blood-brain barrier. Evidence of their potential ability to act as regulators of CBF is mounting. They are known to be contractile, are in direct contact with vascular endothelial cells, and have been shown to migrate alongside the vascular endothelial cells in the developing brain (15).
CBF is known to increase as gestation progresses owing to the increasing metabolic demands (16) and by the third trimester accounts for approximately 25% of combined ventricular output (17). Fetuses have wide minute to minute fluctuations in CBF (4, 18) with a progressive increase in cerebrovascular diastolic flow as pregnancy advances (19). This increase in diastolic flow comes with a slight but steady decline in cerebrovascular resistance that coincides with rapid fetal brain development, but in normal pregnancy remains at a higher resistance than that found in the umbilical arteries throughout gestation (20).
CBF during the transition from fetal to neonatal life is a topic of active investigation, particularly for vulnerable neonatal populations. In the healthy neonate, intact mechanisms of CBF autoregulation ensure steady cerebral oxygenation and glucose delivery at transition. At birth, the low-resistance vascular bed of the placenta is disconnected from systemic circulation while pulmonary vascular resistance falls precipitously with initiation of ventilation. When these events happen concurrently, as expected, the net change in MAP and CBF is minimal. Problems in maintenance of CBF arise when the normal drop in pulmonary vascular resistance does not occur or other factors lead to hemodynamic instability.
Postnatal CBF continues to increase throughout the first 3 years of life with a higher contribution from the posterior circulation compared to adults that peaks in toddlerhood (21). In healthy term infants, CBF is greatest in the brainstem, insula, thalamus and basal ganglia (22). Global CBF estimates in the postnatal period depend greatly on the method used and vary significantly with measurements of 13 ml/100 g/min reported by arterial spin labeling MRI (22), an average of 18 ml/100 g/min by sonographic flowmetry (16), as much as 23 ml/100 g/min by positron emission tomography (23), and approximately 18 ml/100 g/min by near-infrared spectroscopy (NIRS) (24).
Fetal and Neonatal CBF Monitoring Techniques
CBF is a complex physiologic system with multiple factors that influence its assessment in the evolving maturation from fetal to neonatal life. The techniques used to monitor CBF have also evolved significantly, but each has key benefits and limitations. These techniques are described in detail in the following section and summarized in Table 1.
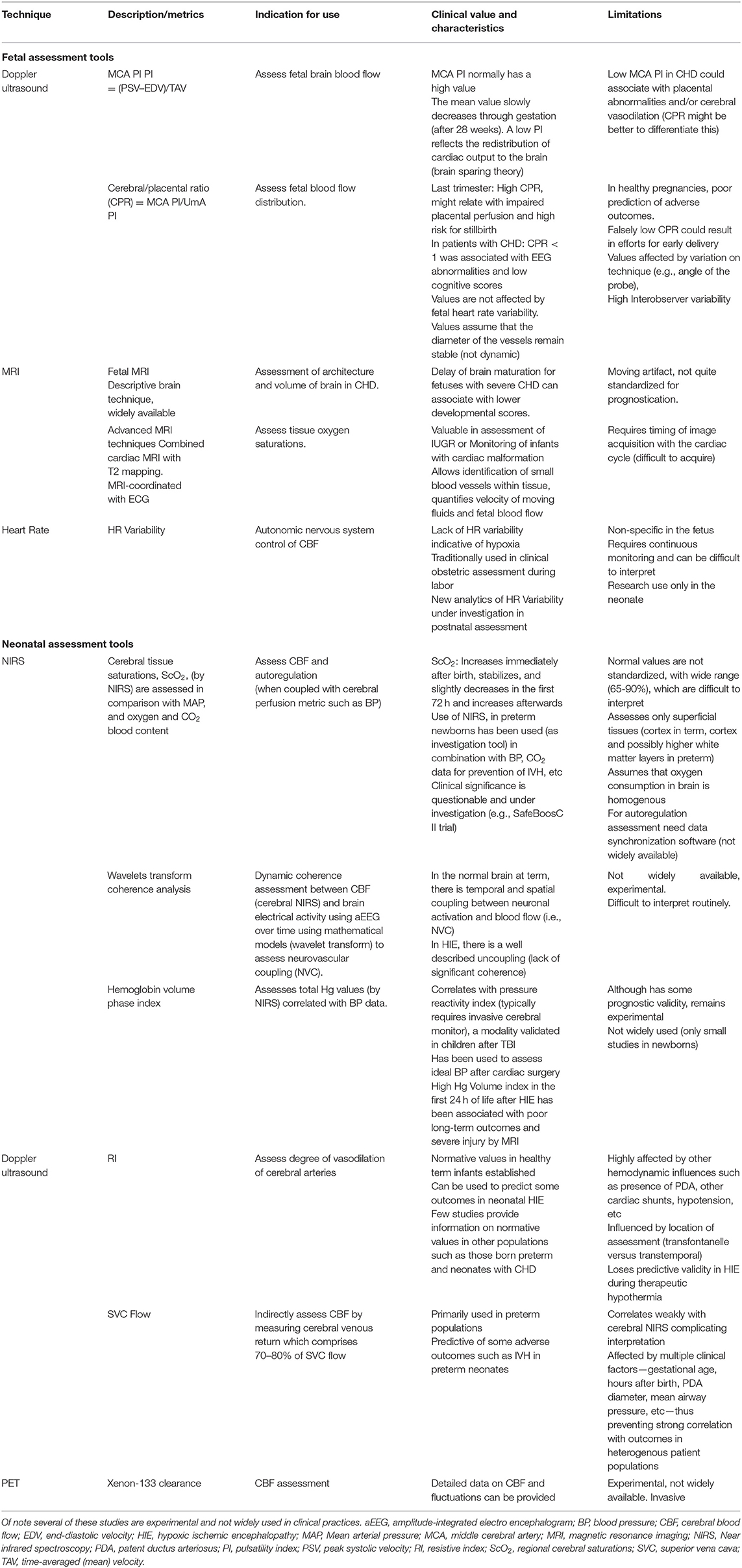
Table 1. A brief description, indication for use, clinical significance, and limitations of key diagnostic studies in newborns at risk for cerebral injury.
Prenatal Doppler Ultrasound
This relationship between cerebrovascular resistance and resistance in the umbilical arteries provides the basis for fetal CBF monitoring using Doppler ultrasound. The cerebral/placental ratio (CPR) is also known as the cerebral/umbilical ratio and is a primary indicator of fetal blood flow distribution (25–30). This metric is a ratio of the relative pulsatility indices (PI) in the cerebral and placental circulations (as measured in the umbilical artery, UmA) defined by the equations,
However, limitations to this method of CA monitoring exist. In the third trimester, low fetal CPR is associated with high uterine artery Doppler indices and is thought to be a consequence of impaired placental perfusion that increases the risk for stillbirth (31). However, it may also result from cerebrovascular vasodilation, which is common in fetuses growing poorly and is discussed further in the section on intrauterine growth restriction (IUGR). The use of CPR as a screening tool in otherwise healthy pregnancies has been shown to only weakly predict adverse outcomes and there is concern that overuse of this metric may expose women and neonates to unnecessary interventions such as earlier gestational age delivery (32).
Postnatal Doppler Ultrasound
Similar to prenatal Doppler ultrasound monitoring of CBF, postnatal measurements can be useful to determine cerebrovascular dilatation as well. The primary metric used postnatally is the resistive index (RI), which is derived from the systolic and diastolic velocities in the cerebral arteries as described,
Normative values for newborn cerebral artery RI are well defined and typically fall between 0.55 and 0.8 (33, 34). Although RI is not sensitive to the angle of insonation, there are limitations to its use including variations based on location of measurement (e.g., anterior fontanelle or transtemporal) (33), and differences based on technique (35). As such, a recent systematic review concluded that there is no clear evidence to support following RI in preterm infants (36), although RI may still have utility in neonates with hypoxic-ischemic encephalopathy (HIE) (37).
MRI Techniques
Advanced magnetic resonance imaging (MRI) technologies that contribute to adult research and care are now finding utility in pregnant people and the fetus. Using advanced MR imaging techniques that combine fetal cardiac MRI with T2 mapping to determine tissue oxygen saturations have enhanced our understanding of fetal circulation (38). These experiments effectively replicate in the human fetus, those classic studies in the chronically instrumented pregnant ewe that form the basis for our current understanding of fetal and placental hemodynamics (39–42). Several key differences in the human fetus have been described by Seed and colleagues, who are pioneering the application of these new technologies (43–46).
T2 mapping is an MRI method that allows identification of small blood vessels within tissue. When combined with phase contrast MRI, which quantifies velocity of moving fluids, accurate measurements of fetal blood flow are possible. One major obstacle to this imaging is the necessity of timing image acquisition with the cardiac cycle (47–51). Cardiac gating is used to time data acquisition with specific phases of the cardiac cycle, and MRI-compatible fetal ECG devices are now available (48, 51–55). This technology has now been applied to fetal disease states including IUGR (54) as well as the fetus with cardiac malformations (38).
Near-Infrared Spectroscopy
Modern NIRS is based on the pioneering work of Professor Frans F. Jöbsis, and since his landmark study in 1977 (56), its use has expanded exponentially. NIRS has the advantage of being non-invasive, inexpensive, and continuous, therefore it is the most commonly used technique to measure regional cerebral saturation (ScO2) in neonates despite the lack of large randomized clinical trials to support its clinical impact. The NIRS signal represents the oxygen saturation of Hg in the underlying brain tissue and is derived from the difference in absorption of near-infrared light by the oxygenated and deoxygenated heme groups (57). However, the depth of tissue interrogation can be relatively low and likely only provides information on the cortex (58, 59). Using NIRS to estimate CBF relies on the assumptions that the overwhelming majority of oxygen in blood is bound to hemoglobin, total hemoglobin is proportional to cerebral blood volume, and there is uniform oxygen consumption within the brain. The NIRS ScO2 value represents a “weighted average” of arterial, venous, and capillary oxygenation with approximately 75–80% of the signal originating from the venous measurement (60). In general, ScO2 values between 65 and 90% are considered normal in healthy term neonates (61, 62).
Only when coupled with a measure of CPP can NIRS impart information about CA. Using NIRS to determine periods of intact and impaired CA requires additional data as well as careful interpretation. Systems that provide time-synchronization of the continuous signals from invasive blood pressure monitors, NIRS, peripheral oxygen saturation, heart rate, and respiratory rate are enhancing the utility of NIRS as a monitor for CA.
Wavelet transform coherence analysis was developed as an alternative tool to Fourier transform to analyze non-stationary signals. Its application to our understanding of neonatal CBF has evolved over the past 10 years (63–65). Due to the non-stationary ScO2 signal provided by NIRS, traditional Fourier transform is inadequate to create a unified analysis across the time-frequency domain without dividing the data into arbitrary “bins” or frequency intervals that require an artificial simplification of the signal into a pseudo-stationary form for analysis. The use of wavelet transform coherence analysis does not require such simplification, but instead allows analysis of signal coherence across a spectrum of the time-frequency domain. The application of wavelet transform coherence analysis has been used extensively for neonates with HIE and is further discussed in that section.
Multiple other analytic techniques utilize NIRS or its component values to determine the correlation between CBF and MAP. The cerebral oximetry index uses the regional cortical oxygen saturation and has been validated in animal models of hypotension (66, 67) followed by clinical trials in pediatric cardiac surgery (68, 69). The pressure reactivity index has been used extensively in traumatic brain injury patients and requires invasive intracranial pressure monitoring, thus, it is not suited for neonates. The hemoglobin volume phase index was developed by Lee et al. using the regional hemoglobin from the NIRS calculation to estimate CBF, and is a non-invasive correlate of the pressure reactivity index (70–72). This method has been used to establish an individualized optimal MAP and time spent below this optimal MAP correlated with adverse outcomes (73). The significant differences in the calculated limits of autoregulation based on each of these methodologies complicates their use in clinical practice and necessitates validation of each methodology in specific populations and disease states.
Methodologic Limitations of NIRS in the Neonate
Despite their widespread use, no randomized studies to date have systematically investigated potential benefits or risks associated with specific ScO2 target ranges or provided validation of these analytical tools in any neonatal population. Normative NIRS signals vary considerably by commercial sensor and by individual patient physiology. Only recently investigators have reported on the mathematical conversions to allow comparison of NIRS data between devices (74, 75). Due to these limitations, the European Society of Paediatric and Neonatal Intensive Care recommends against routine clinical use of NIRS in all children with hemodynamic instability and there is no agreement from the group on the significance of a decline in ScO2 from baseline or a lower limit of normal (76).
Other Monitoring Techniques
Early studies on CBF in neonates used a modified xenon-133 clearance method that relied on external scintillation detectors (77–79). These earlier discoveries related to the influence of Hg, hypoglycemia, and blood pressure on neonatal CBF have informed much of the contemporary investigations into neonatal CBF homeostasis. Recently developed methods to estimate CBF based on heart rate variability show promise since invasive monitoring of MAP is not always possible or desirable in all neonates (80). Heart rate variability refers to the variation in time intervals between each heartbeat and is controlled by the autonomic nervous system. This variability is affected by hypoxia and other conditions that compromise CBF, thus making the metric a possible predictor of disturbed CBF (81, 82). One key limitation to using heart rate variability as a clinical tool is correlating it with other physiologic parameters of cerebral oxygenation and the real-time data analysis required for it to be useful (83).
Another method of CBF monitoring is superior vena cava (SVC) flow which is based on the physiologic principle that cerebral venous return contributes as much as 70–80% to total SVC flow. This technique has been applied in preterm populations and may correlate with adverse outcomes (84), although it is subject to influence by multiple factors. In one study, low SVC flow was associated with lower gestational age, higher upper body vascular resistance, larger diameter patent ductus arteriosus (PDA) shunts, and higher mean airway pressure (85). Together, these variables can make interpretation of SVC flow difficult. In addition, SVC flow only correlates weakly with estimations of CBF by cerebral NIRS (86), adding complexity to its use. With further refinement this technique may contribute to the overall assessment of CBF, and is the subject of ongoing investigations to determine its predictive validity on long-term outcomes in preterm neonates (87).
Cerebral Blood Flow in Vulnerable Fetal and Neonatal Populations
Intrauterine Growth Restriction
CPR is used in pregnancies complicated by IUGR to quantify the redistribution of cardiac output with increased flow to the fetal brain (traditionally called a “brain-sparing” effect) (88, 89). However, this term may be a misnomer. In a secondary analysis of the large multicenter Prospective Observational Trial to Optimize Pediatric Health in IUGR (PORTO) Study, investigators showed that this redistribution of blood flow to the brain is associated with adverse perinatal outcomes including intraventricular hemorrhage, periventricular leukomalacia, HIE, necrotizing enterocolitis, bronchopulmonary dysplasia, sepsis, and death (90). In fact, fetuses with IUGR and CPR <1 had poorer neurodevelopmental outcomes at 3 years compared to pregnancies with abnormal umbilical artery Doppler alone in the follow up report of 375 patients from the PORTO Study (91). These studies clearly demonstrate that cerebral vasodilation does not result in normalization of brain development.
The cause of this hemodynamic disturbance is most frequently related to placental insufficiency and can lead to deleterious alterations in vasoreactivity of cerebral vasculature when prolonged (92). In fact, IUGR fetuses with normalization of MCA-PI after prolonged vasodilation are at increased risk for fetal demise (93). Postnatally, neonates who experienced IUGR have persistent vasodilation of cerebral vasculature (94). This can result in higher ScO2 values by NIRS likely due to a combination of increased cerebral oxygen delivery and a decreased cerebral tissue oxygen consumption (95). The key factor in using this technique is to not rely soley on the single timepoint measurement; rather, a trend in CPR is required to determine where on the trajectory a fetus is in regard to CA.
Fetal Anemia
A fetus is typically evaluated for fetal anemia (defined as Hg levels less than mean for gestational age (GA) (96) or multiples of the median (MoM) for GA) (97–99) when it is noted to have abnormal fluid collections (ascites, pericardial effusion, hydrothorax, and/or skin edema) or reduced movements. The gold standard for the diagnosis of fetal anemia is fetal blood sampling (FBS), a procedure that imparts a high risk for fetal loss, particularly in hydropic fetuses (100). Doppler ultrasonography can detect fetal anemia based on an increase in the peak velocity of systolic blood flow in the MCA (MCA-PSV), mitigating the risk of fetal loss (97, 100). This non-invasive metric relies on the fetal cerebral autoregulatory capacity to increase CBF in the setting of hypoxia, similar to that seen in fetal IUGR.
The basic principle used by Doppler for the diagnosis of fetal anemia, relates to the observed increase in blood velocity which stems from the increased fetal cardiac output. Due to relative maintenance of cardiac output for small changes in Hg, there is no strong correlation between MCA-PSV and fetal Hg within the normal range. If significant Hg decreases occur, however, the MCA-PSV increases and can be used to determine the Hg value with a fair degree of accuracy (100). An MCA-PSV > 1.5 MoM is used as a cut-off for severely anemic fetuses. In non-hydropic fetuses, sensitivity of a single measurement of MCA-PSV varies between 75 and 95%, with about 10% of false-positive results, and this increases if the study is performed after 35 weeks GA (101). Use of the multiple time points of MCA-PSV can decrease the false positive values (101). As such, for high-risk fetuses, MCA-PSV are typically performed at regular intervals depending on risk assessment. Fetuses with anemia are at risk for multiple complications and may benefit from intrauterine transfusions, underscoring the importance of assessment at regular intervals in those deemed high-risk.
Infants Born Preterm
Although much has been learned about CBF in preterm neonates, many questions remain including how variations in CBF are related to neurologic pathologies, and the role of various CBF monitoring techniques, particularly in the setting of disturbed hemodynamics such as hypotension, PDA, anemia, and changes in respiratory status. In all neonates, immediately following birth there is an initial increase in CBF followed by a slight downward trend until around 72 h of life. This rise in CBF after birth is more pronounced in preterm infants (102, 103). Immaturity of the autonomic nervous system, especially the parasympathetic control of CBF is particularly underdeveloped in preterm infants and is the presumed cause of these differences between term and preterm infants (104–106). Loss of this autonomic regulation dampens the adaptive cerebral reactivity, making preterm infants susceptible to passive cerebral circulation and putting them at risk for low CBF in the setting of hypotension (107, 108). When accompanied by PDA, this further compromises CBF and the ability to maintain intact CA (109, 110).
Respiratory status changes also affect CBF more acutely in the preterm neonate as they are prone to rapid fluctuations in PaCO2 after birth due to respiratory distress syndrome and surfactant administration. In addition, fluctuations in MAP acutely impact cerebrovascular responsiveness to PaCO2 (111). Together, these circumstances put preterm infants at increased risk of impaired CA. Dysfunction of CA has been proposed as the mechanism for preterm brain injuries such as IVH and leukomalacia (112–115). The correlation between NIRS metrics of intact vs. impaired CA and subsequent IVH was recently demonstrated in a cohort of preterm infants studied in the first 4 days of life (116). Infants who developed IVH or died showed a loss of cerebral reactivity with fluctuation in blood pressures, resulting in both hypoperfusion and reperfusion brain injury (116).
Several studies to date have asked the question of whether routine NIRS monitoring in preterm neonates improves outcomes. There is no consensus as researchers from several centers have reported opposing results (117–120). Comparisons between such studies are difficult, however, and differences are likely due to contrasting approaches in the management of preterm neonates based on ScO2 measures. Initial results in the SafeBoosC II randomized controlled trial of NIRS in neonates born extremely preterm did not show an impact on EEG outcomes (121) or neurodevelopmental outcome at age 2 years (119), but an additional Phase III randomized-controlled trial is underway (122). A practical guide to the use of NIRS in neonates was recently published by Vesoulis et al. and the authors conclude that there is a need for large studies of comparative approaches (123), which the SafeBoosC III trial will be a strong contributor. These studies must occur while equipoise still exists.
Congenital Heart Disease
Fetal CHD
In fetuses with CHD, there is mounting evidence that brain development is disrupted prenatally with lagging brain growth (38, 124, 125) and estimated growth delay of ~1 month (126–128). This observation has spurred significant interest in CBF monitoring in this population. The use of CPR presents unique challenges to interpretation in fetal CHD. In many forms of CHD, there is adaptive cerebral vasodilation attributable in some cardiac lesions to a relative fetal cerebral hypoxia. In a small study of fetuses with hypoplastic left heart syndrome (HLHS), dextro-transposition of the great arteries (D-TGA), and tetralogy of Fallot (TOF), CPR <1 was associated with reduced neural synchrony as measured by EEG (129). In addition, in those with CHD and CPR <1, cognitive scores on the Bayley Scales of Infant Development were lower at 18-months of age. The adaptive cerebral vasodilation in the fetus with CHD results in a lower MCA PI, which may or may not be accompanied by placental insufficiency (130–134). Higher rates of placental pathologies are known to occur in pregnancies complicated by fetal CHD (135–139), therefore CPR requires careful interpretation in fetuses with CHD.
Postnatal CBF in CHD—Early Adoption of NIRS
NIRS is now commonplace in most pediatric cardiac intensive care units despite the lack of specific normative parameters in neonatal CHD or randomized controlled trials to support its use. Small studies showing improved metrics of care with NIRS use, such as reduction in days on the ventilator in post-operative neonates with CHD (140) have contributed to widespread adoption of the technology. The interpretation of the NIRS signal in the setting of altered CBF in specific CHD physiologies presents unique challenges. In neonates with single-ventricle CHD, ScO2 is lower than healthy term infants at baseline [68.0 ± 9.7 (n = 28) compared to 80.6 ± 7.9, n = 16 in healthy controls; p < 0.001] (141), but depending on whether there is right or left-sided obstruction, ScO2 values can vary (142). Definitive data on whether NIRS monitoring improves patient care is a topic of active investigation (143–147), although the optimal time to answer this question has likely passed given the near-universal use of NIRS in neonates with operative CHD in the United States.
Hypoxic-Ischemic Encephalopathy
There is a sense of urgency to improve our understanding of CA in neonates with HIE as their fluctuations in CBF and maintenance of adequate CPP have been shown to correlate with secondary injury (148–154). Avoidance of post-hypoxic reperfusion injury is a key area of concern in these infants (155). Doppler assessment of cerebral RI with measurements < 0.55 indicate loss of CA and correlate with worse outcomes (156, 157). However, in the setting of active therapeutic hypothermia, RI is somewhat less predictive (positive predictive outcome 60%, negative predictive outcome, NPV 78%) (158), but in late cooling RI < 0.55 has a NPV of 86%, which increases to 89% after rewarming (159).
Many studies underway are using NIRS coupled with invasive MAP monitoring to determine the upper limits of CA in asphyxiated neonates in order to develop individualized optimal MAP parameters and improve long-term prognostication (151, 160). With adjuvant therapies on the horizon, this will enhance the impact of therapeutic hypothermia (or replace its use in those patients in whom it is contraindicated) (161–165). Wavelet transform coherence analysis is being used to differentiate those neonates labeled as mild HIE who may benefit from therapeutic hypothermia based on their inability to maintain intact CA (Figure 2). Chalak et al. has further developed the wavelet coherence analysis to quantify neurovascular coupling by analyzing the relation between regional changes in aEEG signals and MAP. This analysis may provide new avenues for classification of HIE injury that will promote a more targeted therapeutic approach, particularly due to the heterogeneity of the HIE diagnosis. Currently, these techniques are limited by the time and computationally intensive analysis required. Therefore, further development of these bedside time-synchronized hemodynamic monitoring tools to include real-time analytics for neonates with HIE is of utmost importance.
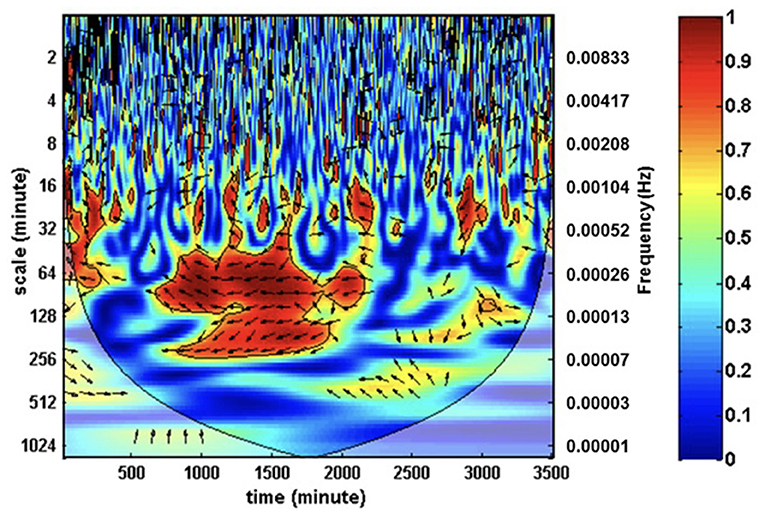
Figure 2. Wavelet transform coherence analysis can be used to characterize cross-correlations between mean arterial pressure and ScO2 as a function of a wide range of both time and frequencies. The coherence depicted by color mapping represents the correlations across the time-frequency axis and can be translated into percent coherence from 0 (least coherent, blue) to 1 (most coherent, red). In-phase coherence indicates impaired cerebral autoregulation.
Hemoglobin volume phase index, which relies on an estimated relative total hemoglobin from NIRS along with continuous MAP to assess CA, was recently used in a cohort of neonates with HIE. Those with higher hemoglobin volume phase values at 24 h of life (indicating impaired CA) had a higher rate of death or severe brain injury by MRI (166). The author's proposed cutoff of hemoglobin volume phase index had a sensitivity of 86% and specificity of 74% for death or severe brain injury by MRI. This type of early prognostication tools may facilitate the use of adjuvant therapies in specific HIE sub-populations and/or allow for improved decision-making about goal-directed care at a much earlier timepoint than standard treatments currently allow. Next steps should include larger scale multicenter prospective clinical trials to determine the generalizability of this metric.
Future Directions in CBF Monitoring
In the Fetus
CBF monitoring of the fetus will be improved significantly through advances in MRI technologies. Currently, the research scans that show the most promise for fetal and placental perfusion are limited to specific institutions that have the expertise required for these customized sequences and their interpretation. Image data sharing between institutions and countries is a major barrier. Commercialization of these MRI scanning sequences would improve their accessibility and data sharing capabilities. The development of artificial intelligence for automated region of interest assignment will be key to improve the pipeline of discovery as will user-friendly interfaces for analysis. These technologies will not replace the inexpensive and readily-available method of Doppler ultrasound for CBF monitoring anytime soon, thus studies to maximize our knowledge and utilization of this tool are also warranted. The CEPRA study (Dutch Trial Registry NTR trial NL7557) is an example of how the fetal Doppler CBF monitoring field is moving forward, the goal of which is to determine the utility of CPR as an indicator for immediate delivery in pregnant people reporting loss of fetal movement (167). These types of multicenter studies are essential to have an impact on clinical practice of fetal monitoring technologies that have already been studied for many years. In contrast, studies of CBF using fetal MRI are in their infancy and require robust sharing of data and technical expertise between centers to move the field forward.
In the Neonate
For postnatal CBF monitoring, NIRS dominates most recent research and will likely continue to be a favored technology in the neonate. However, in order to move forward with the widespread use of NIRS and its real-time analysis with other hemodynamic monitoring signals to estimate CA, significant investment is required to perform multicenter randomized controlled trials validating its ability to improve outcomes in specific neonatal populations. In neonates with CHD, equipoise is likely lost for clinical studies of NIRS since it is already widely used in these patients in cardiac intensive care units and its benefit in that population has been demonstrated in small studies. Clinical trials of NIRS and time-synchronized analytical tools are being studied in other populations, however, such as neonates with HIE (64, 65, 168) and those born preterm (121, 122, 169).
In addition, the use of NIRS to estimate the upper and lower limits of CA in vulnerable neonatal populations requires additional study and a focus on a precision-medicine approach, which is underway in ongoing clinical trials (170). The optimal MAP to maintain stable CBF are unique to individuals and highly related to their specific pathology and their hemodynamic adaptations (160). In the fetal and preterm neonatal populations the ability to autoregulate CBF is not yet fully mature. For those with CHD, the limits of CA are highly related to the degree of somatic and cerebral hypoxia that drives minute to minute changes in CBF. In each of these cases, uniform thresholds of what is considered adequate MAP are not able to provide the type of precision medicine that is needed to improve patient outcomes. Instead, new tools to analyze and integrate the multitude of data points that clinicians are tasked with interpreting are essential to allow a precision-medicine approach and move the field forward.
Technologies that integrate multiple monitoring modalities into a time synchronized visual representation will allow a new level of interpretation and improve our individualized approach to care. Specifically, in terms of CA, an accurate assessment of upper and lower limits of CA must be made for each patient in order to precisely titrate medications and interventions. Next-level monitoring tools are required to facilitate the rapid recognition of impending hemodynamic instability. Likewise, these predictive analytics will be useful to encourage faster weaning from vasoactive medications, mechanical ventilators, and other treatments. As with all technologic advances in patient monitoring, the question of whether these analytics of the future make a positive impact on patient care must be asked and carefully investigated.
Conclusions
Traditional methods of CBF monitoring facilitated our foundational understanding of the maturational process of these complex mechanisms and their behavior in the setting of perturbed hemodynamics. However, the use of animal models and reliance on technologies with significant limitations led to a period of stalled progress in the field. Recently developed techniques including those involving advanced MRI, NIRS, and novel statistical methods have sparked renewed progress. These technologies have led to a new understanding of CA as a dynamic process with limitations that are unique to not only a specific pathology, but also unique to individual patients. Progress in this field will hinge upon our ability to integrate the continuously acquired plethora of patient data into clinically useful and actionable decision-making algorithms.
Author Contributions
RL: concept, drafting, revising, and final approval. EO, NA, DA, and JW: drafting, revising, and final approval. LC: concept, revising, and final approval. All authors contributed to the article and approved the submitted version.
Funding
This work was supported by National Institute of Health (No. 1R01NS102617) to LC.
Conflict of Interest
The authors declare that the research was conducted in the absence of any commercial or financial relationships that could be construed as a potential conflict of interest.
Publisher's Note
All claims expressed in this article are solely those of the authors and do not necessarily represent those of their affiliated organizations, or those of the publisher, the editors and the reviewers. Any product that may be evaluated in this article, or claim that may be made by its manufacturer, is not guaranteed or endorsed by the publisher.
References
1. Purves MJ, James IM. Observations on the control of cerebral blood flow in the sheep fetus and newborn lamb. Circ Res. (1969) 25:651–67. doi: 10.1161/01.RES.25.6.651
2. Jones MD Jr., Sheldon RE, Peeters LL, Makowski EL, Meschia G. Regulation of cerebral blood flow in the ovine fetus. Am J Physiol. (1978) 235:H162–6. doi: 10.1152/ajpheart.1978.235.2.H162
3. Tweed WA, Cote J, Pash M, Lou H. Arterial oxygenation determines autoregulation of cerebral blood flow in the fetal lamb. Pediatr Res. (1983) 17:246–9. doi: 10.1203/00006450-198304000-00002
4. Ashwal S, Dale PS, Longo LD. Regional cerebral blood flow: studies in the fetal lamb during hypoxia, hypercapnia, acidosis, and hypotension. Pediatr Res. (1984) 18:1309–16. doi: 10.1203/00006450-198412000-00018
5. Papile LA, Rudolph AM, Heymann MA. Autoregulation of cerebral blood flow in the preterm fetal lamb. Pediatr Res. (1985) 19:159–61. doi: 10.1203/00006450-198502000-00001
6. Muller T, Lohle M, Schubert H, Bauer R, Wicher C, Antonow-Schlorke I, et al. Developmental changes in cerebral autoregulatory capacity in the fetal sheep parietal cortex. J Physiol. (2002) 539:957–67. doi: 10.1113/jphysiol.2001.012590
7. Willie CK, Tzeng YC, Fisher JA, Ainslie PN. Integrative regulation of human brain blood flow. J Physiol. (2014) 592:841–59. doi: 10.1113/jphysiol.2013.268953
8. Ainslie PN, Duffin J. Integration of cerebrovascular CO2 reactivity and chemoreflex control of breathing: mechanisms of regulation, measurement, and interpretation. Am J Physiol Regul Integr Comp Physiol. (2009) 296:R1473–95. doi: 10.1152/ajpregu.91008.2008
9. Yoon S, Zuccarello M, Rapoport RM. pCO(2) and pH regulation of cerebral blood flow. Front Physiol. (2012) 3:365. doi: 10.3389/fphys.2012.00365
10. Faraci FM, Brian J. E. Jr. Nitric oxide and the cerebral circulation. Stroke. (1994) 25:692–703. doi: 10.1161/01.STR.25.3.692
11. Kuban KC, Gilles FH. Human telencephalic angiogenesis. Ann Neurol. (1985) 17:539–48. doi: 10.1002/ana.410170603
12. Nelson MD Jr., Gonzalez-Gomez I, Gilles FH., Dyke award. The search for human telencephalic ventriculofugal arteries. AJNR Am J Neuroradiol. (1991) 12:215–22.
13. Peppiatt CM, Howarth C, Mobbs P, Attwell D. Bidirectional control of CNS capillary diameter by pericytes. Nature. (2006) 443:700–4. doi: 10.1038/nature05193
14. Hall CN, Reynell C, Gesslein B, Hamilton NB, Mishra A, Sutherland BA, et al. Capillary pericytes regulate cerebral blood flow in health and disease. Nature. (2014) 508:55–60. doi: 10.1038/nature13165
15. Armulik A, Genove G, Betsholtz C. Pericytes: developmental, physiological, pathological perspectives. Problems promises. Dev Cell. (2011) 21:193–215. doi: 10.1016/j.devcel.2011.07.001
16. Kehrer M, Krageloh-Mann I, Goelz R, Schoning M. The development of cerebral perfusion in healthy preterm and term neonates. Neuropediatrics. (2003) 34:281–6. doi: 10.1055/s-2003-44663
17. Rudolph AM, Heymann MA. Fetal and neonatal circulation and respiration. Ann Rev Physiol. (1974) 36:187–207. doi: 10.1146/annurev.ph.36.030174.001155
18. Court DJ, Parer JT, Block BS, Llanos AJ. Effects of beta-adrenergic blockade on blood flow distribution during hypoxaemia in fetal sheep. J Dev Physiol. (1984) 6:349–58.
19. Kennedy C, Grave GD, Jehle JW, Sokoloff L. Blood flow to white matter during maturation of the brain. Neurology. (1970) 20:613–8. doi: 10.1212/WNL.20.6.613
20. Di Renzo GC, Luzi G, Cucchia GC, Caserta G, Fusaro P, Perdikaris A, et al. The role of Doppler technology in the evaluation of fetal hypoxia. Early Hum Dev. (1992) 29:259–67. doi: 10.1016/0378-3782(92)90162-A
21. Schoning M, Hartig B. Age dependence of total cerebral blood flow volume from childhood to adulthood. J Cereb Blood Flow Metab. (1996) 16:827–33. doi: 10.1097/00004647-199609000-00007
22. Bouyssi-Kobar M, Murnick J, Brossard-Racine M, Chang T, Mahdi E, Jacobs M, et al. Altered cerebral perfusion in infants born preterm compared with infants born full term. J Pediatr. (2018) 193:54–61 e52. doi: 10.1016/j.jpeds.2017.09.083
23. Altman DI, Powers WJ, Perlman JM, Herscovitch P, Volpe SL, Volpe JJ. Cerebral blood flow requirement for brain viability in newborn infants is lower than in adults. Ann Neurol. (1988) 24:218–26. doi: 10.1002/ana.410240208
24. Edwards AD, Wyatt JS, Richardson C, Delpy DT, Cope M, Reynolds EO. Cotside measurement of cerebral blood flow in ill newborn infants by near infrared spectroscopy. Lancet. (1988) 2:770–1. doi: 10.1016/S0140-6736(88)92418-X
25. Gramellini D, Folli MC, Raboni S, Vadora E, Merialdi A. Cerebral-umbilical Doppler ratio as a predictor of adverse perinatal outcome. Obstet Gynecol. (1992) 79:416–20. doi: 10.1097/00006250-199203000-00018
26. Binder J, Monaghan C, Thilaganathan B, Morales-Rosello J, Khalil A. Reduced fetal movements and cerebroplacental ratio: evidence for worsening fetal hypoxemia. Ultrasound Obstet Gynecol. (2018) 51:375–80. doi: 10.1002/uog.18830
27. Kalafat E, Khalil A. Clinical significance of cerebroplacental ratio. Curr Opin Obstet Gynecol. (2018) 30:344–54. doi: 10.1097/GCO.0000000000000490
28. Monaghan C, Binder J, Thilaganathan B, Morales-Rosello J, Khalil A. Perinatal loss at term: role of uteroplacental and fetal Doppler assessment. Ultrasound Obstet Gynecol. (2018) 52:72–7. doi: 10.1002/uog.17500
29. Morales-Rosello J, Khalil A, Fornes-Ferrer V, Hervas-Marin D, Peralta-Llorens N, Rubio-Moll J, et al. The vertebroplacental ratio as an alternative to the cerebroplacental ratio in the evaluation of the fetus at the end of pregnancy. J Matern Fetal Neonatal Med. (2018) 31:70–9. doi: 10.1080/14767058.2016.1275551
30. Morales-Rosello J, Khalil A, Fornes-Ferrer V, Perales-Marin A. Accuracy of the fetal cerebroplacental ratio for the detection of intrapartum compromise in nonsmall fetuses. J Matern Fetal Neonatal Med. (2019) 32:2842–52. doi: 10.1080/14767058.2018.1450380
31. Griffin M, Seed PT, Webster L, Myers J, Mackillop L, Simpson N, et al. Diagnostic accuracy of placental growth factor and ultrasound parameters to predict the small-for-gestational-age infant in women presenting with reduced symphysis-fundus height. Ultrasound Obstet Gynecol. (2015) 46:182–90. doi: 10.1002/uog.14860
32. Winchester ML, Mccarther N, Cancino D, Fitzgerald S, Parrish M. Second trimester cerebroplacental ratio versus umbilicocerebral ratio for the prediction of adverse perinatal outcomes. J Matern Fetal Neonatal Med. (2021) 1–7. doi: 10.1080/14767058.2021.1938530 [Epub ahead of Print].
33. Allison JW, Faddis LA, Kinder DL, Roberson PK, Glasier CM, Seibert JJ. Intracranial resistive index (RI) values in normal term infants during the first day of life. Pediatr Radiol. (2000) 30:618–20. doi: 10.1007/s002470000286
34. Zamora C, Tekes A, Alqahtani E, Kalayci OT, Northington F, Huisman TA. Variability of resistive indices in the anterior cerebral artery during fontanel compression in preterm and term neonates measured by transcranial duplex sonography. J Perinatol. (2014) 34:306–10. doi: 10.1038/jp.2014.11
35. Taylor GA. Effect of scanning pressure on intracranial hemodynamics during transfontanellar duplex US. Radiology. (1992) 185:763–6. doi: 10.1148/radiology.185.3.1438759
36. Camfferman FA, De Goederen R, Govaert P, Dudink J, Van Bel F, Pellicer A, et al. Diagnostic and predictive value of Doppler ultrasound for evaluation of the brain circulation in preterm infants: a systematic review. Pediatr Res. (2020) 87:50–8. doi: 10.1038/s41390-020-0777-x
37. Gerner GJ, Burton VJ, Poretti A, Bosemani T, Cristofalo E, Tekes A, et al. Transfontanellar duplex brain ultrasonography resistive indices as a prognostic tool in neonatal hypoxic-ischemic encephalopathy before and after treatment with therapeutic hypothermia. J Perinatol. (2016) 36:202–6. doi: 10.1038/jp.2015.169
38. Sun L, Macgowan CK, Sled JG, Yoo SJ, Manlhiot C, Porayette P, et al. Reduced fetal cerebral oxygen consumption is associated with smaller brain size in fetuses with congenital heart disease. Circulation. (2015) 131:1313–23. doi: 10.1161/CIRCULATIONAHA.114.013051
39. Battaglia FC, Meschia G, Makowski EL, Bowes W. The effect of maternal oxygen inhalation upon fetal oxygenation. J Clin Invest. (1968) 47:548–55. doi: 10.1172/JCI105751
40. Rosenfeld CR, Morriss F. H. Jr., Makowski EL, Meschia G, Battaglia FC. Circulatory changes in the reproductive tissues of ewes during pregnancy. Gynecol Invest. (1974) 5:252–68. doi: 10.1159/000301658
41. Rosenfeld CR. Distribution of cardiac output in ovine pregnancy. Am J Physiol. (1977) 232:H231–5. doi: 10.1152/ajpheart.1977.232.3.H231
42. Rosenfeld CR, Gant NF Jr. The chronically instrumental ewe: a model for studying vascular reactivity to angiotensin II in pregnancy. J Clin Invest. (1981) 67:486–92. doi: 10.1172/JCI110057
43. Sun L, Macgowan CK, Portnoy S, Sled JG, Yoo SJ, Grosse-Wortmann L, et al. New advances in fetal cardiovascular magnetic resonance imaging for quantifying the distribution of blood flow and oxygen transport: Potential applications in fetal cardiovascular disease diagnosis and therapy. Echocardiography. (2017) 34:1799–803. doi: 10.1111/echo.13760
44. Sorensen A, Hutter J, Seed M, Grant PE, Gowland P. T2*-weighted placental MRI: basic research tool or emerging clinical test for placental dysfunction? Ultrasound Obstet Gynecol. (2020) 55:293–302. doi: 10.1002/uog.20855
45. Sun L, Marini D, Saini B, Schrauben E, Macgowan CK, Seed M. Understanding fetal hemodynamics using cardiovascular magnetic resonance imaging. Fetal Diagn Ther. (2020) 47:354–62. doi: 10.1159/000505091
46. Sun L, Van Amerom JFP, Marini D, Portnoy S, Lee FT, Saini BS, et al. Characterization of hemodynamic patterns in human fetuses with cyanotic congenital heart disease using MRI. Ultrasound Obstet Gynecol. (2021) 58:824–36. doi: 10.1002/uog.23707
47. Chaptinel J, Yerly J, Mivelaz Y, Prsa M, Alamo L, Vial Y, et al. Fetal cardiac cine magnetic resonance imaging in utero. Sci Rep. (2017) 7:15540. doi: 10.1038/s41598-017-15701-1
48. Roy CW, Van Amerom JFP, Marini D, Seed M, Macgowan CK. Fetal cardiac MRI: a review of technical advancements. Top Magn Reson Imaging. (2019) 28:235–44. doi: 10.1097/RMR.0000000000000218
49. Tsuritani M, Morita Y, Miyoshi T, Kurosaki K, Yoshimatsu J. Fetal cardiac functional assessment by fetal heart magnetic resonance imaging. J Comput Assist Tomogr. (2019) 43:104–8. doi: 10.1097/RCT.0000000000000781
50. Dong SZ, Zhu M, Ji H, Ren JY, Liu K. Fetal cardiac MRI: a single center experience over 14-years on the potential utility as an adjunct to fetal technically inadequate echocardiography. Sci Rep. (2020) 10:12373. doi: 10.1038/s41598-020-69375-3
51. Goolaub DS, Xu J, Schrauben E, Sun L, Roy CW, Marini D, et al. Fetal flow quantification in great vessels using motion-corrected radial phase contrast MRI: comparison with cartesian. J Magn Reson Imaging. (2021) 53:540–51. doi: 10.1002/jmri.27334
52. Kording F, Yamamura J, De Sousa MT, Ruprecht C, Hedstrom E, Aletras AH, et al. Dynamic fetal cardiovascular magnetic resonance imaging using Doppler ultrasound gating. J Cardiovasc Magn Reson. (2018) 20:17. doi: 10.1186/s12968-018-0440-4
53. Tavares De Sousa M, Hecher K, Yamamura J, Kording F, Ruprecht C, Fehrs K, et al. Dynamic fetal cardiac magnetic resonance imaging in four-chamber view using Doppler ultrasound gating in normal fetal heart and in congenital heart disease: comparison with fetal echocardiography. Ultrasound Obstet Gynecol. (2019) 53:669–75. doi: 10.1002/uog.20167
54. Goolaub DS, Marini D, Seed M, Macgowan CK. Human fetal blood flow quantification with magnetic resonance imaging and motion compensation. J Vis Exp. (2021) 167. doi: 10.36227/techrxiv.15112944
55. Lloyd DFA, Van Poppel MPM, Pushparajah K, Vigneswaran TV, Zidere V, Steinweg J, et al. Analysis of 3-dimensional arch anatomy, vascular flow, and postnatal outcome in cases of suspected coarctation of the aorta using fetal cardiac magnetic resonance imaging. Circ Cardiovasc Imaging. (2021) e012411. doi: 10.1161/CIRCIMAGING.121.012411
56. Jobsis FF. Noninvasive, infrared monitoring of cerebral and myocardial oxygen sufficiency and circulatory parameters. Science. (1977) 198:1264–7. doi: 10.1126/science.929199
57. Marin T, Moore J. Understanding near-infrared spectroscopy. Adv Neonatal Care. (2011) 11:382–8. doi: 10.1097/ANC.0b013e3182337ebb
58. Brown DW, Picot PA, Naeini JG, Springett R, Delpy DT, Lee TY. Quantitative near infrared spectroscopy measurement of cerebral hemodynamics in newborn piglets. Pediatr Res. (2002) 51:564–70. doi: 10.1203/00006450-200205000-00004
59. Dix LM, Van Bel F, Lemmers PM. Monitoring cerebral oxygenation in neonates: an update. Front Pediatr. (2017) 5:46. doi: 10.3389/fped.2017.00046
60. Watzman HM, Kurth CD, Montenegro LM, Rome J, Steven JM, Nicolson SC. Arterial and venous contributions to near-infrared cerebral oximetry. Anesthesiology. (2000) 93:947–53. doi: 10.1097/00000542-200010000-00012
61. Bernal NP, Hoffman GM, Ghanayem NS, Arca MJ. Cerebral and somatic near-infrared spectroscopy in normal newborns. J Pediatr Surg. (2010) 45:1306–10. doi: 10.1016/j.jpedsurg.2010.02.110
62. Pichler G, Binder C, Avian A, Beckenbach E, Schmolzer GM, Urlesberger B. Reference ranges for regional cerebral tissue oxygen saturation and fractional oxygen extraction in neonates during immediate transition after birth. J Pediatr. (2013) 163:1558–63. doi: 10.1016/j.jpeds.2013.07.007
63. Chalak LF, Tarumi T, Zhang R. The “neurovascular unit approach” to evaluate mechanisms of dysfunctional autoregulation in asphyxiated newborns in the era of hypothermia therapy. Early Hum Dev. (2014) 90:687–94. doi: 10.1016/j.earlhumdev.2014.06.013
64. Chalak LF, Tian F, Adams-Huet B, Vasil D, Laptook A, Tarumi T, et al. Novel wavelet real time analysis of neurovascular coupling in neonatal encephalopathy. Sci Rep. (2017) 7:45958. doi: 10.1038/srep45958
65. Chalak LF, Zhang R. New wavelet neurovascular bundle for bedside evaluation of cerebral autoregulation and neurovascular coupling in newborns with hypoxic-ischemic encephalopathy. Dev Neurosci. (2017) 39:89–96. doi: 10.1159/000457833
66. Brady KM, Lee JK, Kibler KK, Smielewski P, Czosnyka M, Easley RB, et al. Continuous time-domain analysis of cerebrovascular autoregulation using near-infrared spectroscopy. Stroke. (2007) 38:2818–25. doi: 10.1161/STROKEAHA.107.485706
67. Brady KM, Lee JK, Kibler KK, Easley RB, Koehler RC, Shaffner DH. Continuous measurement of autoregulation by spontaneous fluctuations in cerebral perfusion pressure: comparison of 3 methods. Stroke. (2008) 39:2531–7. doi: 10.1161/STROKEAHA.108.514877
68. Brady K, Joshi B, Zweifel C, Smielewski P, Czosnyka M, Easley RB, et al. Real-time continuous monitoring of cerebral blood flow autoregulation using near-infrared spectroscopy in patients undergoing cardiopulmonary bypass. Stroke. (2010) 41:1951–6. doi: 10.1161/STROKEAHA.109.575159
69. Brady KM, Mytar JO, Lee JK, Cameron DE, Vricella LA, Thompson WR, et al. Monitoring cerebral blood flow pressure autoregulation in pediatric patients during cardiac surgery. Stroke. (2010) 41:1957–62. doi: 10.1161/STROKEAHA.109.575167
70. Lee JK, Kibler KK, Benni PB, Easley RB, Czosnyka M, Smielewski P, et al. Cerebrovascular reactivity measured by near-infrared spectroscopy. Stroke. (2009) 40:1820–6. doi: 10.1161/STROKEAHA.108.536094
71. Diedler J, Zweifel C, Budohoski KP, Kasprowicz M, Sorrentino E, Haubrich C, et al. The limitations of near-infrared spectroscopy to assess cerebrovascular reactivity: the role of slow frequency oscillations. Anesth Analg. (2011) 113:849–57. doi: 10.1213/ANE.0b013e3182285dc0
72. Lee JK, Yang ZJ, Wang B, Larson AC, Jamrogowicz JL, Kulikowicz E, et al. Noninvasive autoregulation monitoring in a swine model of pediatric cardiac arrest. Anesth Analg. (2012) 114:825–36. doi: 10.1213/ANE.0b013e31824762d5
73. Lee JK, Brady KM, Chung SE, Jennings JM, Whitaker EE, Aganga D, et al. A pilot study of cerebrovascular reactivity autoregulation after pediatric cardiac arrest. Resuscitation. (2014) 85:1387–93. doi: 10.1016/j.resuscitation.2014.07.006
74. Kleiser S, Nasseri N, Andresen B, Greisen G, Wolf M. Comparison of tissue oximeters on a liquid phantom with adjustable optical properties. Biomed Opt Express. (2016) 7:2973–92. doi: 10.1364/BOE.7.002973
75. Kleiser S, Ostojic D, Andresen B, Nasseri N, Isler H, Scholkmann F, et al. Comparison of tissue oximeters on a liquid phantom with adjustable optical properties: an extension. Biomed Opt Express. (2018) 9:86–101. doi: 10.1364/BOE.9.000086
76. Singh Y, Villaescusa JU, Da Cruz EM, Tibby SM, Bottari G, Saxena R, et al. Recommendations for hemodynamic monitoring for critically ill children-expert consensus statement issued by the cardiovascular dynamics section of the European Society of Paediatric and Neonatal Intensive Care (ESPNIC). Crit Care. (2020) 24:620. doi: 10.1186/s13054-020-03326-2
77. Younkin DP, Reivich M, Jaggi J, Obrist W, Delivoria-Papadopoulos M. Noninvasive method of estimating human newborn regional cerebral blood flow. J Cereb Blood Flow Metab. (1982) 2:415–20. doi: 10.1038/jcbfm.1982.47
78. Younkin DP, Reivich M, Jaggi JL, Obrist WD, Delivoria-Papadopoulos M. The effect of hematocrit and systolic blood pressure on cerebral blood flow in newborn infants. J Cereb Blood Flow Metab. (1987) 7:295–9. doi: 10.1038/jcbfm.1987.66
79. Younkin D, Delivoria-Papadopoulos M, Reivich M, Jaggi J, Obrist W. Regional variations in human newborn cerebral blood flow. J Pediatr. (1988) 112:104–8. doi: 10.1016/S0022-3476(88)80131-8
80. Cardoso S, Silva MJ, Guimaraes H. Autonomic nervous system in newborns: a review based on heart rate variability. Childs Nerv Syst. (2017) 33:1053–63. doi: 10.1007/s00381-017-3436-8
81. Eventov-Friedman S, Shinwell ES, Barnea E, Flidel-Rimon O, Juster-Reicher A, Levy R. Correlation between fetal heart rate reactivity and mortality and severe neurological morbidity in extremely low birth weight infants. J Matern Fetal Neonatal Med. (2012) 25:654–5. doi: 10.3109/14767058.2011.591457
82. Bersani I, Piersigilli F, Gazzolo D, Campi F, Savarese I, Dotta A, et al. Heart rate variability as possible marker of brain damage in neonates with hypoxic ischemic encephalopathy: a systematic review. Eur J Pediatr. (2021) 180:1335–45. doi: 10.1007/s00431-020-03882-3
83. Pfurtscheller K, Bauernfeind G, Muller-Putz GR, Urlesberger B, Muller W, Pfurtscheller G. Correlation between EEG burst-to-burst intervals and HR acceleration in preterm infants. Neurosci Lett. (2008) 437:103–6. doi: 10.1016/j.neulet.2008.03.079
84. Hunt RW, Evans N, Rieger I, Kluckow M. Low superior vena cava flow and neurodevelopment at 3 years in very preterm infants. J Pediatr. (2004) 145:588–92. doi: 10.1016/j.jpeds.2004.06.056
85. Kluckow M, Evans N. Low superior vena cava flow and intraventricular haemorrhage in preterm infants. Arch Dis Child Fetal Neonatal Ed. (2000) 82:F188–94. doi: 10.1136/fn.82.3.F188
86. Moran M, Miletin J, Pichova K, Dempsey EM. Cerebral tissue oxygenation index and superior vena cava blood flow in the very low birth weight infant. Acta Paediatr. (2009) 98:43–6. doi: 10.1111/j.1651-2227.2008.01006.x
87. Bravo MC, Lopez-Ortego P, Sanchez L, Diez J, Cabanas F, Pellicer A. Randomised trial of dobutamine versus placebo for low superior vena cava flow in preterm infants: Long-term neurodevelopmental outcome. J Paediatr Child Health. (2021) 57:872–6. doi: 10.1111/jpc.15344
88. Arbeille P, Roncin A, Berson M, Patat F, Pourcelot L. Exploration of the fetal cerebral blood flow by duplex Doppler–linear array system in normal and pathological pregnancies. Ultrasound Med Biol. (1987) 13:329–37. doi: 10.1016/0301-5629(87)90166-9
89. Arbeille P, Maulik D, Fignon A, Stale H, Berson M, Bodard S, et al. Assessment of the fetal PO2 changes by cerebral and umbilical Doppler on lamb fetuses during acute hypoxia. Ultrasound Med Biol. (1995) 21:861–70. doi: 10.1016/0301-5629(95)00025-M
90. Flood K, Unterscheider J, Daly S, Geary MP, Kennelly MM, Mcauliffe FM, et al. The role of brain sparing in the prediction of adverse outcomes in intrauterine growth restriction: results of the multicenter PORTO Study. Am J Obstet Gynecol. (2014) 211:288 e281–5. doi: 10.1016/j.ajog.2014.05.008
91. Monteith C, Flood K, Pinnamaneni R, Levine TA, Alderdice FA, Unterscheider J, et al. An abnormal cerebroplacental ratio (CPR) is predictive of early childhood delayed neurodevelopment in the setting of fetal growth restriction. Am J Obstet Gynecol. (2019) 221:273.e271–3.e9. doi: 10.1016/j.ajog.2019.06.026
92. Cohen E, Baerts W, Van Bel F. Brain-sparing in intrauterine growth restriction: considerations for the neonatologist. Neonatology. (2015) 108:269–76. doi: 10.1159/000438451
93. Konje JC, Bell SC, Taylor DJ. Abnormal Doppler velocimetry and blood flow volume in the middle cerebral artery in very severe intrauterine growth restriction: is the occurence of reversal of compensatory flow too late? BJOG. (2001) 108:973–9. doi: 10.1111/j.1471-0528.2001.00222.x
94. Nishimaki S, Shima Y, Yoda H, Kawakami T, Akamatsu H. Blood flow velocities in the cerebral arteries and descending aorta in small-for-dates infants. Pediatr Radiol. (1993) 23:575–7. doi: 10.1007/BF02014966
95. Baik-Schneditz N, Pichler G, Schwaberger B, Binder-Heschl C, Mileder L, Reiss IKH, et al. Effect of intrauterine growth restriction on cerebral regional oxygen saturation in preterm and term neonates during immediate postnatal transition. Neonatology. (2020) 117:324–30. doi: 10.1159/000507583
96. Nicolaides KH, Soothill PW, Clewell WH, Rodeck CH, Mibashan RS, Campbell S. Fetal haemoglobin measurement in the assessment of red cell isoimmunisation. Lancet. (1988) 1:1073–5. doi: 10.1016/S0140-6736(88)91896-X
97. Abbasi N, Johnson JA, Ryan G. Fetal anemia. Ultrasound Obstet Gynecol. (2017) 50:145–53. doi: 10.1002/uog.17555
98. Mari G, Deter RL, Carpenter RL, Rahman F, Zimmerman R, Moise, et al. J. Jr., et al. Noninvasive diagnosis by Doppler ultrasonography of fetal anemia due to maternal red-cell alloimmunization. Collaborative group for doppler assessment of the blood velocity in anemic fetuses. N Engl J Med. (2000) 342:9–14. doi: 10.1056/NEJM200001063420102
99. Mari G, Detti L, Oz U, Zimmerman R, Duerig P, Stefos T. Accurate prediction of fetal hemoglobin by Doppler ultrasonography. Obstet Gynecol. (2002) 99:589–93. doi: 10.1097/00006250-200204000-00015
100. Society for Maternal-Fetal Medicine. Electronic Address PSO,, Mari G, Norton ME, Stone J, Berghella V, Sciscione AC, et al. Society for Maternal-Fetal Medicine (SMFM) Clinical Guideline #8: the fetus at risk for anemia–diagnosis and management. Am J Obstet Gynecol. (2015) 212:697–710. doi: 10.1016/j.ajog.2015.01.059
101. Zimmerman R, Carpenter RJ Jr., Durig P, Mari G. Longitudinal measurement of peak systolic velocity in the fetal middle cerebral artery for monitoring pregnancies complicated by red cell alloimmunisation: a prospective multicentre trial with intention-to-treat. BJOG. (2002) 109:746–52. doi: 10.1111/j.1471-0528.2002.01314.x
102. Olsen KS, Svendsen LB, Larsen FS. Validation of transcranial near-infrared spectroscopy for evaluation of cerebral blood flow autoregulation. J Neurosurg Anesthesiol. (1996) 8:280–5. doi: 10.1097/00008506-199610000-00004
103. Meek JH, Tyszczuk L, Elwell CE, Wyatt JS. Cerebral blood flow increases over the first three days of life in extremely preterm neonates. Arch Dis Child Fetal Neonatal Ed. (1998) 78:F33–7. doi: 10.1136/fn.78.1.F33
104. Clairambault J, Curzi-Dascalova L, Kauffmann F, Medigue C, Leffler C. Heart rate variability in normal sleeping full-term and preterm neonates. Early Hum Dev. (1992) 28:169–83. doi: 10.1016/0378-3782(92)90111-S
105. Patural H, Barthelemy JC, Pichot V, Mazzocchi C, Teyssier G, Damon G, et al. Birth prematurity determines prolonged autonomic nervous system immaturity. Clin Auton Res. (2004) 14:391–5. doi: 10.1007/s10286-004-0216-9
106. Longin E, Gerstner T, Schaible T, Lenz T, Konig S. Maturation of the autonomic nervous system: differences in heart rate variability in premature vs. term infants. J Perinat Med. (2006) 34:303–8. doi: 10.1515/JPM.2006.058
107. Perlman JM, Mcmenamin JB, Volpe JJ. Fluctuating cerebral blood-flow velocity in respiratory-distress syndrome. Relation to the development of intraventricular hemorrhage. N Engl J Med. (1983) 309:204–9. doi: 10.1056/NEJM198307283090402
108. Greisen G. Autoregulation of cerebral blood flow in newborn babies. Early Hum Dev. (2005) 81:423–8. doi: 10.1016/j.earlhumdev.2005.03.005
109. Lemmers PM, Toet MC, Van Bel F. Impact of patent ductus arteriosus and subsequent therapy with indomethacin on cerebral oxygenation in preterm infants. Pediatrics. (2008) 121:142–7. doi: 10.1542/peds.2007-0925
110. Chock VY, Ramamoorthy C, Van Meurs KP. Cerebral autoregulation in neonates with a hemodynamically significant patent ductus arteriosus. J Pediatr. (2012) 160:936–42. doi: 10.1016/j.jpeds.2011.11.054
111. Jayasinghe D, Gill AB, Levene MI. CBF reactivity in hypotensive and normotensive preterm infants. Pediatr Res. (2003) 54:848–53. doi: 10.1203/01.PDR.0000088071.30873.DA
112. Tsuji M, Saul JP, Du Plessis A, Eichenwald E, Sobh J, Crocker R, et al. Cerebral intravascular oxygenation correlates with mean arterial pressure in critically ill premature infants. Pediatrics. (2000) 106:625–32. doi: 10.1542/peds.106.4.625
113. Soul JS, Hammer PE, Tsuji M, Saul JP, Bassan H, Limperopoulos C, et al. Fluctuating pressure-passivity is common in the cerebral circulation of sick premature infants. Pediatr Res. (2007) 61:467–73. doi: 10.1203/pdr.0b013e31803237f6
114. O'leary H, Gregas MC, Limperopoulos C, Zaretskaya I, Bassan H, Soul JS, et al. Elevated cerebral pressure passivity is associated with prematurity-related intracranial hemorrhage. Pediatrics. (2009) 124:302–9. doi: 10.1542/peds.2008-2004
115. Alderliesten T, Lemmers PM, Smarius JJ, Van De Vosse RE, Baerts W, Van Bel F. Cerebral oxygenation, extraction, and autoregulation in very preterm infants who develop peri-intraventricular hemorrhage. J Pediatr. (2013) 162:698–704.e692. doi: 10.1016/j.jpeds.2012.09.038
116. Hoffman SB, Cheng YJ, Magder LS, Shet N, Viscardi RM. Cerebral autoregulation in premature infants during the first 96 hours of life and relationship to adverse outcomes. Arch Dis Child Fetal Neonatal Ed. (2019) 104:F473–9. doi: 10.1136/archdischild-2018-315725
117. Hyttel-Sorensen S, Pellicer A, Alderliesten T, Austin T, Van Bel F, Benders M, et al. Cerebral near infrared spectroscopy oximetry in extremely preterm infants: phase II randomised clinical trial. BMJ. (2015) 350:g7635. doi: 10.1136/bmj.g7635
118. Alderliesten T, Van Bel F, Van Der Aa NE, Steendijk P, Van Haastert IC, De Vries LS, et al. Low cerebral oxygenation in preterm infants is associated with adverse neurodevelopmental outcome. J Pediatr. (2019) 207:109–16.e102. doi: 10.1016/j.jpeds.2018.11.038
119. Plomgaard AM, Alderliesten T, Van Bel F, Benders M, Claris O, Cordeiro M, et al. No neurodevelopmental benefit of cerebral oximetry in the first randomised trial (SafeBoosC II) in preterm infants during the first days of life. Acta Paediatr. (2019) 108:275–81. doi: 10.1111/apa.14463
120. Kalteren WS, Verhagen EA, Mintzer JP, Bos AF, Kooi EMW. Anemia and red blood cell transfusions, cerebral oxygenation, brain injury and development, and neurodevelopmental outcome in preterm infants: a systematic review. Front Pediatr. (2021) 9:644462. doi: 10.3389/fped.2021.644462
121. Plomgaard AM, Van Oeveren W, Petersen TH, Alderliesten T, Austin T, Van Bel F, et al. The SafeBoosC II randomized trial: treatment guided by near-infrared spectroscopy reduces cerebral hypoxia without changing early biomarkers of brain injury. Pediatr Res. (2016) 79:528–35. doi: 10.1038/pr.2015.266
122. Hansen ML, Pellicer A, Gluud C, Dempsey E, Mintzer J, Hyttel-Sorensen S, et al. Cerebral near-infrared spectroscopy monitoring versus treatment as usual for extremely preterm infants: a protocol for the SafeBoosC randomised clinical phase III trial. Trials. (2019) 20:811. doi: 10.1186/s13063-019-3955-6
123. Vesoulis ZA, Mintzer JP, Chock VY. Neonatal NIRS monitoring: recommendations for data capture and review of analytics. J Perinatol. (2021) 41:675–88. doi: 10.1038/s41372-021-00946-6
124. Von Rhein M, Buchmann A, Hagmann C, Dave H, Bernet V, Scheer I, et al. Severe congenital heart defects are associated with global reduction of neonatal brain volumes. J Pediatr. (2015) 167:1259–63.e1251. doi: 10.1016/j.jpeds.2015.07.006
125. Jansen FA, Van Zwet EW, Rijlaarsdam ME, Pajkrt E, Van Velzen CL, Zuurveen HR, et al. Head growth in fetuses with isolated congenital heart defects: lack of influence of aortic arch flow and ascending aorta oxygen saturation. Ultrasound Obstet Gynecol. (2016) 48:357–64. doi: 10.1002/uog.15980
126. Clouchoux C, Du Plessis AJ, Bouyssi-Kobar M, Tworetzky W, Mcelhinney DB, Brown DW, et al. Delayed cortical development in fetuses with complex congenital heart disease. Cereb Cortex. (2013) 23:2932–43. doi: 10.1093/cercor/bhs281
127. Claessens NH, Moeskops P, Buchmann A, Latal B, Knirsch W, Scheer I, et al. Delayed cortical gray matter development in neonates with severe congenital heart disease. Pediatr Res. (2016) 80:668–74. doi: 10.1038/pr.2016.145
128. Everwijn SMP, Namburete AIL, Van Geloven N, Jansen F a.R., Papageorghiou AT, et al. The association between flow and oxygenation and cortical development in fetuses with congenital heart defects using a brain-age prediction algorithm. Prenat Diagn. (2021) 41:43–51. doi: 10.1002/pd.5813
129. Williams IA, Tarullo AR, Grieve PG, Wilpers A, Vignola EF, Myers MM, et al. Fetal cerebrovascular resistance and neonatal EEG predict 18-month neurodevelopmental outcome in infants with congenital heart disease. Ultrasound Obstet Gynecol. (2012) 40:304–9. doi: 10.1002/uog.11144
130. Donofrio MT, Bremer YA, Schieken RM, Gennings C, Morton LD, Eidem BW, et al. Autoregulation of cerebral blood flow in fetuses with congenital heart disease: the brain sparing effect. Pediatr Cardiol. (2003) 24:436–43. doi: 10.1007/s00246-002-0404-0
131. Donofrio MT. The heart-brain interaction in the fetus: cerebrovascular blood flow in the developing human. Progr Pediatr Cardiol. (2006) 22:41–51. doi: 10.1016/j.ppedcard.2006.01.004
132. Garcia-Canadilla P, Rudenick P, Crispi F, Cruz-Lemini M, Palau G, Gratacos E, et al. Understanding prenatal brain sparing by flow redistribution based on a lumped model of the fetal circulation. Funct Imaging Model Heart. (2013) 7945:123–31. doi: 10.1007/978-3-642-38899-6_15
133. Cahill LS, Zhou YQ, Seed M, Macgowan CK, Sled JG. Brain sparing in fetal mice: BOLD MRI and Doppler ultrasound show blood redistribution during hypoxia. J Cereb Blood Flow Metab. (2014) 34:1082–8. doi: 10.1038/jcbfm.2014.62
134. Cahill LS, Hoggarth J, Lerch JP, Seed M, Macgowan CK, Sled JG. Fetal brain sparing in a mouse model of chronic maternal hypoxia. J Cereb Blood Flow Metab. (2019) 39:1172–84. doi: 10.1177/0271678X17750324
135. Jones HN, Olbrych SK, Smith KL, Cnota JF, Habli M, Ramos-Gonzales O, et al. Hypoplastic left heart syndrome is associated with structural and vascular placental abnormalities and leptin dysregulation. Placenta. (2015) 36:1078–86. doi: 10.1016/j.placenta.2015.08.003
136. Albalawi A, Brancusi F, Askin F, Ehsanipoor R, Wang J, Burd I, et al. Placental characteristics of fetuses with congenital heart disease. J Ultrasound Med. (2017) 36:965–72. doi: 10.7863/ultra.16.04023
137. Rychik J, Goff D, Mckay E, Mott A, Tian Z, Licht DJ, et al. Characterization of the placenta in the newborn with congenital heart disease: distinctions based on type of cardiac malformation. Pediatr Cardiol. (2018) 39:1165–71. doi: 10.1007/s00246-018-1876-x
138. Mir IN, Leon R, Chalak LF. Placental origins of neonatal diseases: toward a precision medicine approach. Pediatr Res. (2020) 89:377–83. doi: 10.1038/s41390-020-01293-6
139. Leon RL, Mir IN, Herrera CL, Sharma K, Spong CY, Twickler DM, et al. Neuroplacentology in congenital heart disease: placental connections to neurodevelopmental outcomes. Pediatr Res. (2021). doi: 10.1038/s41390-021-01521-7 [Epub ahead of Print].
140. Johnson BA, Hoffman GM, Tweddell JS, Cava JR, Basir M, Mitchell ME, et al. Near-infrared spectroscopy in neonates before palliation of hypoplastic left heart syndrome. Ann Thorac Surg. (2009) 87:571–7; discussion 577–9. doi: 10.1016/j.athoracsur.2008.10.043
141. Tran NN, Kumar SR, Hodge FS, Macey PM. Cerebral autoregulation in neonates with and without congenital heart disease. Am J Crit Care. (2018) 27:410–6. doi: 10.4037/ajcc2018672
142. Altit G, Bhombal S, Tacy TA, Chock VY. End-organ saturation differences in early neonatal transition for left- versus right-sided congenital heart disease. Neonatology. (2018) 114:53–61. doi: 10.1159/000487472
143. Kasman N, Brady K. Cerebral oximetry for pediatric anesthesia: why do intelligent clinicians disagree? Paediatr Anaesth. (2011) 21:473–8. doi: 10.1111/j.1460-9592.2011.03549.x
144. Gregory A, Kohl BA. Con: near-infrared spectroscopy has not proven its clinical utility as a standard monitor in cardiac surgery. J Cardiothorac Vasc Anesth. (2013) 27:390–4. doi: 10.1053/j.jvca.2012.11.010
145. Vernick WJ, Gutsche JT. Pro: cerebral oximetry should be a routine monitor during cardiac surgery. J Cardiothorac Vasc Anesth. (2013) 27:385–9. doi: 10.1053/j.jvca.2012.12.002
146. Zacharias DG, Lilly K, Shaw CL, Pirundini P, Rizzo RJ, Body SC, et al. Survey of the clinical assessment and utility of near-infrared cerebral oximetry in cardiac surgery. J Cardiothorac Vasc Anesth. (2014) 28:308–16. doi: 10.1053/j.jvca.2013.06.003
147. Garvey AA, Dempsey EM. Applications of near infrared spectroscopy in the neonate. Curr Opin Pediatr. (2018) 30:209–15. doi: 10.1097/MOP.0000000000000599
148. Van Bel F, Dorrepaal CA, Benders MJ, Zeeuwe PE, Van De Bor M, Berger HM. Changes in cerebral hemodynamics and oxygenation in the first 24 hours after birth asphyxia. Pediatrics. (1993) 92:365–72. doi: 10.1542/peds.92.3.365
149. Meek JH, Elwell CE, Mccormick DC, Edwards AD, Townsend JP, Stewart AL, et al. Abnormal cerebral haemodynamics in perinatally asphyxiated neonates related to outcome. Arch Dis Child Fetal Neonatal Ed. (1999) 81:F110–5. doi: 10.1136/fn.81.2.F110
150. Meek JH, Tyszczuk L, Elwell CE, Wyatt JS. Low cerebral blood flow is a risk factor for severe intraventricular haemorrhage. Arch Dis Child Fetal Neonatal Ed. (1999) 81:F15–8. doi: 10.1136/fn.81.1.F15
151. Burton VJ, Gerner G, Cristofalo E, Chung SE, Jennings JM, Parkinson C, et al. A pilot cohort study of cerebral autoregulation and 2-year neurodevelopmental outcomes in neonates with hypoxic-ischemic encephalopathy who received therapeutic hypothermia. BMC Neurol. (2015) 15:209. doi: 10.1186/s12883-015-0464-4
152. Massaro AN, Govindan RB, Vezina G, Chang T, Andescavage NN, Wang Y, et al. Impaired cerebral autoregulation and brain injury in newborns with hypoxic-ischemic encephalopathy treated with hypothermia. J Neurophysiol. (2015) 114:818–24. doi: 10.1152/jn.00353.2015
153. Tekes A, Poretti A, Scheurkogel MM, Huisman TA, Howlett JA, Alqahtani E, et al. Apparent diffusion coefficient scalars correlate with near-infrared spectroscopy markers of cerebrovascular autoregulation in neonates cooled for perinatal hypoxic-ischemic injury. AJNR Am J Neuroradiol. (2015) 36:188–93. doi: 10.3174/ajnr.A4083
154. Govindan RB, Massaro A, Chang T, Vezina G, Du Plessis A. A novel technique for quantitative bedside monitoring of neurovascular coupling. J Neurosci Methods. (2016) 259:135–42. doi: 10.1016/j.jneumeth.2015.11.025
155. Rhee CJ, Da Costa CS, Austin T, Brady KM, Czosnyka M, Lee JK. Neonatal cerebrovascular autoregulation. Pediatr Res. (2018) 84:602–10. doi: 10.1038/s41390-018-0141-6
156. Archer LN, Levene MI, Evans DH. Cerebral artery Doppler ultrasonography for prediction of outcome after perinatal asphyxia. Lancet. (1986) 2:1116–8. doi: 10.1016/S0140-6736(86)90528-3
157. Low JA, Galbraith RS, Raymond MJ, Derrick EJ. Cerebral blood flow velocity in term newborns following intrapartum fetal asphyxia. Acta Paediatr. (1994) 83:1012–6. doi: 10.1111/j.1651-2227.1994.tb12973.x
158. Elstad M, Whitelaw A, Thoresen M. Cerebral resistance index is less predictive in hypothermic encephalopathic newborns. Acta Paediatr. (2011) 100:1344–9. doi: 10.1111/j.1651-2227.2011.02327.x
159. Skranes JH, Elstad M, Thoresen M, Cowan FM, Stiris T, Fugelseth D. Hypothermia makes cerebral resistance index a poor prognostic tool in encephalopathic newborns. Neonatology. (2014) 106:17–23. doi: 10.1159/000358229
160. Lee JK, Poretti A, Perin J, Huisman T, Parkinson C, Chavez-Valdez R, et al. Optimizing cerebral autoregulation may decrease neonatal regional hypoxic-ischemic brain injury. Dev Neurosci. (2017) 39:248–56. doi: 10.1159/000452833
161. Chalak LF, Rouse DJ. Neuroprotective approaches: before and after delivery. Clin Perinatol. (2011) 38:455–70. doi: 10.1016/j.clp.2011.06.012
162. Dupont T, Chalak L. Future alternative therapies in the pipeline for mild neonatal encephalopathy: Review of evidence of neuroprotection with erythropoiesis stimulating agents. Early Hum Dev. (2018) 120:95–8. doi: 10.1016/j.earlhumdev.2018.02.009
163. Juul SE, Comstock BA, Heagerty PJ, Mayock DE, Goodman AM, Hauge S, et al. High-dose erythropoietin for asphyxia and encephalopathy (HEAL): a randomized controlled trial - background, aims, study protocol. Neonatology. (2018) 113:331–8. doi: 10.1159/000486820
164. Nair J, Kumar VHS. Current and emerging therapies in the management of hypoxic ischemic encephalopathy in neonates. Children. (2018) 5:99. doi: 10.3390/children5070099
165. El Farargy MS, Soliman NA. A randomized controlled trial on the use of magnesium sulfate and melatonin in neonatal hypoxic ischemic encephalopathy. J Neonatal Perinatal Med. (2019) 12:379–84. doi: 10.3233/NPM-181830
166. Massaro AN, Lee JK, Vezina G, Glass P, O'kane A, Li R, et al. Exploratory assessment of the relationship between hemoglobin volume phase index, magnetic resonance imaging, and functional outcome in neonates with hypoxic-ischemic encephalopathy. Neurocrit Care. (2020) 35:121–9. doi: 10.1007/s12028-020-01150-8
167. Damhuis SE, Ganzevoort W, Duijnhoven RG, Groen H, Kumar S, Heazell AEP, et al. The CErebro Placental RAtio as indicator for delivery following perception of reduced fetal movements, protocol for an international cluster randomised clinical trial; the CEPRA study. BMC Pregnancy Childbirth. (2021) 21:285. doi: 10.1186/s12884-021-03760-2
168. Tian F, Sepulveda P, Kota S, Liu Y, Das Y, Liu H, et al. Regional heterogeneity of cerebral hemodynamics in mild neonatal encephalopathy measured with multichannel near-infrared spectroscopy. Pediatr Res. (2020) 89:882–8. doi: 10.1038/s41390-020-0992-5
169. Hyttel-Sorensen S, Austin T, Van Bel F, Benders M, Claris O, Dempsey E, et al. A phase II randomized clinical trial on cerebral near-infrared spectroscopy plus a treatment guideline versus treatment as usual for extremely preterm infants during the first three days of life (SafeBoosC): study protocol for a randomized controlled trial. Trials. (2013) 14:120. doi: 10.1186/1745-6215-14-120
Keywords: cerebral autoregulation, cerebroplacental Doppler, fetal MRI, congenital heart disease, hypoxic ischemia encephalopathy (HIE), near-infrared spectroscopy, neonatal brain, fetal brain
Citation: Leon RL, Ortigoza EB, Ali N, Angelis D, Wolovits JS and Chalak LF (2022) Cerebral Blood Flow Monitoring in High-Risk Fetal and Neonatal Populations. Front. Pediatr. 9:748345. doi: 10.3389/fped.2021.748345
Received: 27 July 2021; Accepted: 15 December 2021;
Published: 11 January 2022.
Edited by:
Valerie Chock, Stanford University, United StatesReviewed by:
Ana Alarcon, Hospital Sant Joan de Déu Barcelona, SpainMarek Czosnyka, University of Cambridge, United Kingdom
Zachary Andrew Vesoulis, Washington University School of Medicine in St. Louis, United States
Copyright © 2022 Leon, Ortigoza, Ali, Angelis, Wolovits and Chalak. This is an open-access article distributed under the terms of the Creative Commons Attribution License (CC BY). The use, distribution or reproduction in other forums is permitted, provided the original author(s) and the copyright owner(s) are credited and that the original publication in this journal is cited, in accordance with accepted academic practice. No use, distribution or reproduction is permitted which does not comply with these terms.
*Correspondence: Rachel L. Leon, cmFjaGVsLmxlb25AdXRzb3V0aHdlc3Rlcm4uZWR1