- 1Neonatology, EGA Institute for Women's Health, University College London, London, United Kingdom
- 2Medical Physics and Biomedical Engineering, University College London, London, United Kingdom
- 3Edinburgh Neuroscience & Centre for Clinical Brain Sciences, The University of Edinburgh, Edinburgh, United Kingdom
Neonatal encephalopathy (NE) in term and near-term infants is a significant global health problem; the worldwide burden of disease remains high despite the introduction of therapeutic hypothermia. Assessment of injury severity and effective management in the neonatal intensive care unit (NICU) relies on multiple monitoring modalities from systemic to brain-specific. Current neuromonitoring tools provide information utilized for seizure management, injury stratification, and prognostication, whilst systemic monitoring ensures multi-organ dysfunction is recognized early and supported wherever needed. The neuromonitoring technologies currently used in NE however, have limitations in either their availability during the active treatment window or their reliability to prognosticate and stratify injury confidently in the early period following insult. There is therefore a real need for a neuromonitoring tool that provides cot side, early and continuous monitoring of brain health which can reliably stratify injury severity, monitor response to current and emerging treatments, and prognosticate outcome. The clinical use of near-infrared spectroscopy (NIRS) technology has increased in recent years. Research studies within this population have also increased, alongside the development of both instrumentation and signal processing techniques. Increasing use of commercially available cerebral oximeters in the NICU, and the introduction of advanced optical measurements using broadband NIRS (BNIRS), frequency domain NIRS (FDNIRS), and diffuse correlation spectroscopy (DCS) have widened the scope by allowing the direct monitoring of oxygen metabolism and cerebral blood flow, both key to understanding pathophysiological changes and predicting outcome in NE. This review discusses the role of optical neuromonitoring in NE and why this modality may provide the next significant piece of the puzzle toward understanding the real time state of the injured newborn brain.
Background: Neonatal Encephalopathy
Neonatal encephalopathy (NE) resulting from intrapartum related events is a global health problem accounting for a quarter of neonatal deaths world-wide (1). It is the second most common cause of preventable childhood disability and this has not improved significantly over the past two decades despite hypothermia treatment (2, 3). In high income settings NE affects between 1 and 3/1,000 live births whereas the incidence is up to 10 times higher in mid and low income settings (1–3).
Following an intrapartum hypoxic-ischaemic event, the brain suffers significant hemodynamic and metabolic derangements which are dynamic, evolving over a period of hours, days and weeks due to the neurotoxic and neurochemical cascade that ensues (4). Early pre-clinical and clinical studies using phosphorous (31P) nuclear magnetic resonance spectroscopy (MRS) described the evolution of secondary energy failure with a reduction in high energy phosphates and a rise in cerebral lactate over hours and days following birth (5, 6). During the initial insult a proportion of cells undergo primary cell death and the neuronal supply of high energy metabolites such as adenosine triphosphate (ATP) is exhausted, also termed “primary energy failure.” Following successful resuscitation the brain enters a latent phase lasting for ~6–24 h which is characterized by the partial recovery of cerebral oxidative metabolism and cerebral blood flow, although a degree of hypoperfusion continues. The brain then enters a period of “secondary energy failure” (SEF) characterized by mitochondrial impairment and cell death with associated cerebral autoregulatory disturbance and brain hyperperfusion. The concept of secondary energy failure (SEF) is a hallmark of NE and the primary target of current therapeutic hypothermia treatment (5–8) (Figure 1).
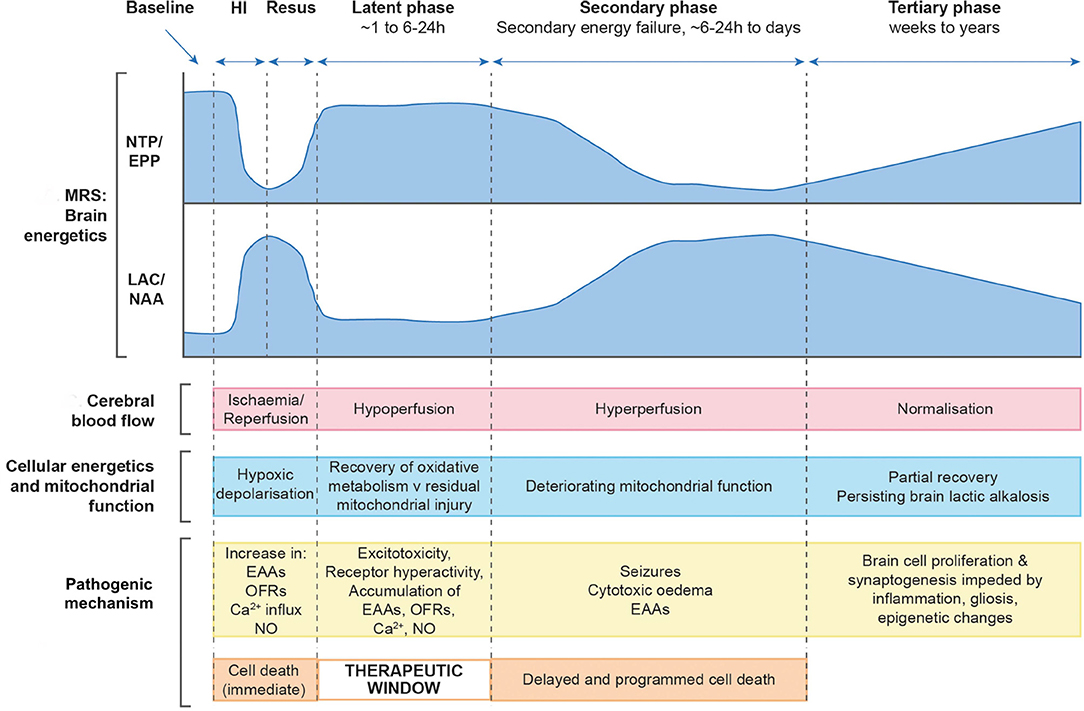
Figure 1. Schematic diagram illustrating the different pathological phases of cerebral injury after hypoxic- ischaemic (HI) insult. Changes in magnetic resonance spectroscopy derived markers of brain energetics (NTP/EPP from 31P MRS and Lac/NAA from 1H MRS, NTP, nucleotide triphosphate; EPP, exchangeable phosphate pool; Lac, lactate; NAA, N acetyl aspartate) with biphasic pattern of energy failure, cerebral blood flow, cellular energetics and mitochondrial function, pathogenic mechanisms occurring at each phase and cell damage with associated therapeutic window. Image modified from Hassell et al. (9).
Therapeutic hypothermia (TH) has been the standard of care for over 10 years for moderate to severe NE in high resource settings like the UK. Animal models post hypoxic-ischaemic (HI) insult have shown that hypothermia reduces energy expenditure and neuronal loss through multiple parallel pathways (8, 10–12). Despite this therapy a significant number of surviving infants with NE suffer moderate to severe disabilities including cerebral palsy, developmental delay, epilepsy, and visual impairment (13, 14). In parallel with the developments in search of new neuroprotective agents to improve outcome, it is essential that neuromonitoring platforms offer accurate and timely information on brain health in order to stratify injury severity, direct clinical care, identify eligible patients for new treatments, and assess their response to them, and ultimately provide vital prognosis of outcome.
Current Neuromonitoring in the Neonatal Intensive Care Unit (NICU)
Most neonatal centers providing specialist management for infants with NE are currently equipped with essential neuromonitoring tools consisting of some form of neurophysiological monitoring and cranial ultrasound, with or without additional optical monitoring (Figure 2).
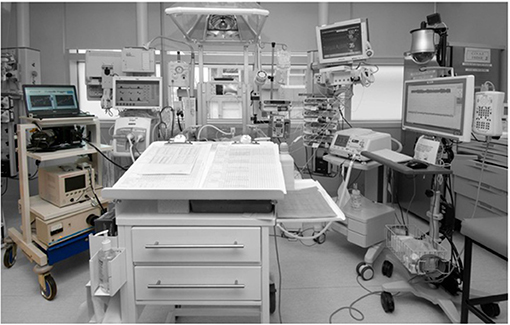
Figure 2. Optimal continuous neuromonitoring in the NICU with video EEG (right), systemic monitoring (right back) and NIRS monitoring (left—a commercial oximeter is used here with a purpose built broadband NIRS system).
Neurophysiological Monitoring: EEG and aEEG
Amplitude integrated electroencephalograph (aEEG) and electroencephalograph (EEG) have vital roles in the monitoring and management of babies with NE. The information provided assists clinicians in assessing injury severity, tracking progress and determining prognosis with respect to neurological outcome. There are advantages and disadvantages to the use of both neurophysiological tools in NE. aEEG is a filtered and compressed recording of electrocortical activity and displays background amplitude and pattern continuously. Cot side interpretation is typically carried out by neonatologists using pattern recognition and voltage classification (15, 16). A meta-analysis on aEEG use in term infants with NE is available and supports the accurate prognostic ability of background pattern on aEEG to predict neurodevelopmental outcome (17). With the introduction of TH as treatment for NE however, the timing for optimal prognostic accuracy of aEEG is now delayed until 48 h compared with <6 h in the normothermic era (18). Seizure burden is another important and independent prognostic marker in NE (16, 19, 20) and whilst aEEG monitoring is used for seizure detection, a high proportion of short and single electrical seizures may go undetected compared with continuous multi-channel EEG (21–23) which is the gold standard in seizure diagnosis and monitoring of background electrical brain activity (24). A major limitation of continuous multi-channel EEG however, is that probe placement is more complex and interpretation requires prompt and specialist neurophysiology input, something only a few tertiary NICUs can provide as part of their NE management. Most tertiary neonatal units will have access to aEEG and EEG “on demand” but not continuous cot side EEG.
Cranial Ultrasound
Cranial ultrasound is a simple, non-invasive and cot side imaging modality that is performed serially in NE in an effort to track the progression of injury. Assessment may demonstrate cerebral oedema or in more severe cases increased echogenicity in the basal ganglia and thalamus (BGT). Studies assessing predictive accuracy however, have shown it to be low with a sensitivity of 79% and specificity of 55% for abnormal neurological outcome at 18 months (25).
Transcranial Doppler (TCD) ultrasound is also performed serially in NE to measure the Pourcelot resistance index (RI) (26), calculated from the measurement of peak systolic and end diastolic flow velocity in the anterior cerebral artery. An abnormally low RI in NE suggests cerebral vasodilatation thought to be secondary to cerebral vasoparesis occurring during the phase of secondary energy failure (27, 28). A resistance index of 0.55 or less between 24 and 72 h after birth had a high predictive value for abnormal outcome following NE in the pre-cooling era, (26) however, this has significantly changed following the introduction of TH with the positive predictive value falling from a mean of 84% in normothermia to 60% during TH when measured from 24 h of life (29).
Magnetic Resonance Imaging and Spectroscopy (MRI/MRS)
Although Magnetic Resonance Imaging (MRI) and spectroscopy (MRS) cannot be performed as part of the continuous neuromonitoring in the NICU, this is currently the optimum neuroimaging technique for detecting perinatally acquired cerebral lesions following NE, and the pattern and severity of these provide a reliable guide to prognosis (30–32). The British Association of Perinatal Medicine's (BAPM) most recent guidance for NE has indicated that all infants undergoing TH should have a brain MRI and proton MRS of basal ganglia-thalamic (BGT) region undertaken between 5 and 14 days after birth (33).
Proton (1H) MRS in the neonatal brain has prominent signal peaks related to the presence of N-acetylaspartate (NAA), choline, creatine and lactate (Lac). Metabolite peak area ratios are typically calculated using a voxel positioned in the basal ganglia and thalamic region. An increased lactate and reduced N-acetyl aspartate (high Lac/NAA peak area ratio) on (1H) MRS reflects neuronal and mitochondrial injury as well as impaired oxidative metabolism and (1H) MRS is highly predictive of outcome in babies who have undergone TH following NE (34, 35). A recent study in newborns undergoing TH for moderate to severe NE noted a threshold of 0.39 Lac/NAA peak area ratio accurately predicted 2 year motor, cognitive and language outcomes (sensitivity and specificity of 100 and 97, 90 and 97, 81 and 97%, respectively) (35). Absolute quantitation of NAA concentration is also reported to have high prognostic value [sensitivity of 1.0 (0.74–1.0) and specificity of 0.97 (0.90–1.0)], but this currently requires a much longer acquisition time and specialist facilities (34).
Optical Neuromonitoring in Neonatal Encephalopathy
As focus moves toward novel neuroprotective agents targeting underlying secondary and tertiary phases of injury, a very real need persists for a cot side neuromonitoring device in the NICU that will assess injury severity, accurately track the progression of injury from soon after birth, identify babies eligible for novel treatments, and assess their response to those treatments. Ultimately the aim of such a neuromonitoring system would be to offer robust biomarkers of injury in real time that will provide clinicians with vital information regarding severity in order to direct care, and in conjunction with current gold standard techniques such as MRI/MRS accurately inform on neurodevelopmental outcome. Brain monitoring based on optical technologies is relatively cheap, safe, transportable, easy to use and importantly, it allows continuous monitoring at the cot side making it an attractive monitoring system for the dynamic changes occurring in NE. Significant advances have occurred over the past four decades with the development of both near-infrared spectroscopy (NIRS) and diffuse correlation spectroscopy (DCS). Here we present a review of the various optical technologies and findings from their utilization in NE to date (Table 1). We also discuss developments in the instrumentation itself as well as the advances in signal processing techniques used to assess the dynamic relationships between measured parameters. Finally, we discuss current and future research aimed at furthering our ability to more accurately measure the complex pathophysiology taking place in NE and provide answers to key clinical questions in order to improve outcomes.
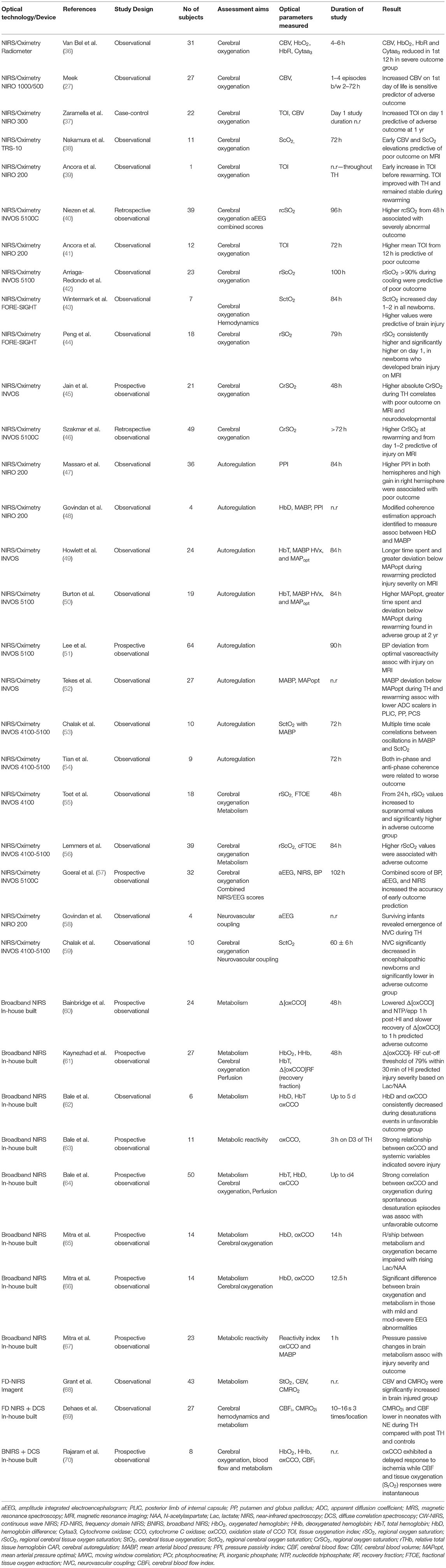
Table 1. Clinical and relevant recent pre-clinical optical studies in Neonatal Encephalopathy: characteristics.
Near-Infrared Spectroscopy: Basic Principles
Near-infrared spectroscopy (NIRS) utilizes the relative transparency of biological tissue to light in the near infrared region of 700–1,000 nm. This relative transparency allows us to measure different chromophores, substances which absorb light within the same near infrared (NIR) spectrum. Neonates are well-suited to this form of monitoring due to the relatively lower thickness of their skin and skull bones allowing better NIR light depth penetration of tissues. There are three major oxygen dependent chromophores of interest in clinical NIRS monitoring, oxyhemoglobin (HbO2), deoxyemoglobin (HHb), and the redox state of Cytochrome C oxidase (CCO). As all have different absorption spectra within the NIR region it is possible to individually measure their tissue concentration changes.
NIRS Devices and Measured Parameters
Traditional NIRS devices measure changes in hemoglobin in both its oxygenated (HbO2) and deoxygenated (HHb) forms to calculate levels of total hemoglobin (HbT=HbO2 + HHb) and hemoglobin difference (HbD= HbO2-HHb). Total hemoglobin and hemoglobin difference serve as proxy markers for cerebral blood volume (CBV) and cerebral oxygen saturation, respectively. Hemoglobin difference has also been utilized as a proxy marker for cerebral blood flow (CBF) and has been shown to be a reliable surrogate in previous studies (71, 72). Commercially available cerebral oximeters provide a further NIRS parameter called the absolute tissue oxygen saturation or cerebral oxygenation, with manufacturers using a variety of nomenclature for this parameter (StO2, TOI, rSO2, rScO2, SctO2, CrSO2). For consistency we will refer to this parameter throughout as the absolute tissue oxygen saturation. Using manufacturer specific algorithms this is delivered as an absolute percentage, calculated as a ratio of oxyhemoglobin (HbO2) to total hemoglobin (HbT), averages are calculated in the arterial and venous compartments in the measured tissue and finally weighted by their estimated arterial/venous volume ratio. These ratios are impossible to determine precisely and are most certainly dynamic with proportions likely to vary over time, between different subjects and depending on different disease states (73). For this reason approximations are used in manufacturer algorithms. Beyond commercially available NIRS brain oximetry measurements, other NIRS techniques can also be used to quantify oxidative metabolism and microvascular perfusion, to be discussed in later sections.
There are three main modes of NIRS technology utilized within both commercial and purpose-built platforms. Continuous Wave (CW-NIRS) is the earliest and most common. Using a few (2–4) discrete wavelengths of continuous light source, CW-NIRS does not differentiate between light attenuation caused by absorption and scattering. Absolute concentrations of individual chromophores are not possible for this reason and instead changes in concentrations are measured against an arbitrary baseline. CW-NIRS commercial brain oximeters that measure absolute tissue oxygen saturation employ multi-distance measurements and algorithms such as spatially resolved spectroscopy (SRS) to scale the concentration measurements of hemoglobin and obtain an absolute tissue oxygen saturation value. Frequency domain (FD-NIRS) mode modulates light at a particular frequency and the attenuation of this light and frequency phase shift is measured. Phase shift observations relate to tissue scattering allowing the differentiation from absorption and therefore measurement of absolute chromophore concentrations. This gives FD-NIRS a theoretical advantage with more consistent quantitative measurements. Time-domain (TD-NIRS) is arguably the most complex technology and uses ultrashort pulses of light, measuring the time of flight through tissue with a photon counting device. This mode enables the absolute measurement of chromophores also, through differentiation of scattering and absorption. TD-NIRS instrumentation provides superior depth penetration however, is a complex and costly technology. Of importance to any monitoring technology is high reproducibility and precision of the measured parameters. Several groups have investigated the precision of NIRS-based tissue oximeters within the neonatal population (74–79). Results of this work remain varied with groups finding both high (74) and low (76) levels of precision for the measurement of various NIRS parameters in neonatal cohorts. More recent studies however, have shown promising results with higher precision achieved by ascertaining high tissue homogeneity for each measurement (79) and by accounting for the significant contribution made by systemic fluctuations and attempting to correct for these (78).
NIRS Monitoring in Neonatal Encephalopathy
NIRS monitoring has been used widely in both pre-clinical and clinical research studies following perinatal insult. Most studies to date have utilized forms of commercial cerebral oximeters and have provided important information regarding changes in cerebral tissue oxygen saturation and hemodynamics. Cerebral oximeters are also becoming increasingly popular on neonatal units amongst clinicians due to their ease of use and their ability to produce a single absolute measurement of brain tissue oxygen saturation for comparison at the cot side. More recently, there has been renewed interest and further development in broadband NIRS (BNIRS), an extension of CW-NIRS which allows us to capture the full NIR optical spectra of brain tissue and more directly measure the intracellular oxidative metabolic state. FD-NIRS has also been utilized in recent studies in both NE (68) and the preterm population (80) for its ability to provide estimates of relative changes in the cerebral metabolic rate of oxygen (rCMRO2), a derived NIRS marker of metabolic activity. FDNIRS has since been used in combination with another optical technology, diffuse correlation spectroscopy (DCS), which directly measures microvascular blood flow to more directly calculate the metabolic parameter CMRO2i in NE (69). The advancement of signal processing techniques occurring in parallel to the technology developments has meant that disturbances in dynamic relationships such as those involved in cerebral autoregulation and metabolic reactivity can be better characterized and monitored, improving our understanding of this disease and bringing us closer to developing robust real-time cot side biomarkers of injury.
Monitoring Absolute Cerebral Tissue Oxygen Saturation
In healthy term infants, trends in absolute cerebral tissue oxygen saturation show levels to be lowest at birth (between 40 and 56%) (81–86), rising gradually over the first 24 h following birth to ~78% (±7.9%) (87). Over the next few weeks levels stabilize between 55 and 85% (88–90). Several studies reviewed trends in absolute cerebral tissue oxygen saturation and hemodynamics following perinatal insult in both the pre-TH and TH era. Van Bel et al. found that pre-TH, CBV and absolute cerebral tissue oxygen saturation were observed to fall 12 h after birth in more severely asphyxiated infants with these parameters stabilizing by 12–24 h (36). Higher CBV and CBF values on the 1st day of life in infants pre-TH were associated with more severe injury at neurodevelopmental follow up (27, 37). This finding was supported by a further study looking at both cooled and non-cooled infants showing CBV at 6 h after birth and absolute cerebral tissue oxygen saturation by 24 h were significantly higher in infants with adverse outcomes on MRI. By combining both parameters at 24 h, the ability to predict adverse neurological outcome on MRI between 7 and 14 d after birth was significant with a sensitivity and specificity of 100% (38). Subsequently, several clinical studies in NE over the period of TH and re-warming have found significant differences in the absolute cerebral tissue oxygen saturation values between favorable and adverse outcome groups as determined by MRI or neurodevelopmental follow up (39–46). Cerebral oximetry monitoring commenced within 6 h after birth in one study of cooled infants found absolute cerebral tissue oxygen saturation (rSO2) fell following the onset of cooling in all infants followed by a gradual rise then plateau by ~24 h. rSO2 significantly increased from day 1 to day 2 of life in both outcome groups but was consistently higher throughout the 72 h hypothermia treatment in asphyxiated newborns developing later brain injury when compared to those with favorable outcomes. This difference between groups was especially prominent during the first 10 h of hypothermia treatment suggesting an early predictive ability for later outcome using this biomarker (44). Further studies during TH have found that higher absolute cerebral tissue oxygen saturation (CrSO2, SctO2) between 24 and 36h after birth (43, 45, 46) and rewarming (46) predicted adverse neurological outcome as measured by moderate to severe injury on MRI (Figure 3) (45). Rate of rise of CrSO2 from baseline until 36 h was also significant in differentiating between outcome groups on MRI (45).
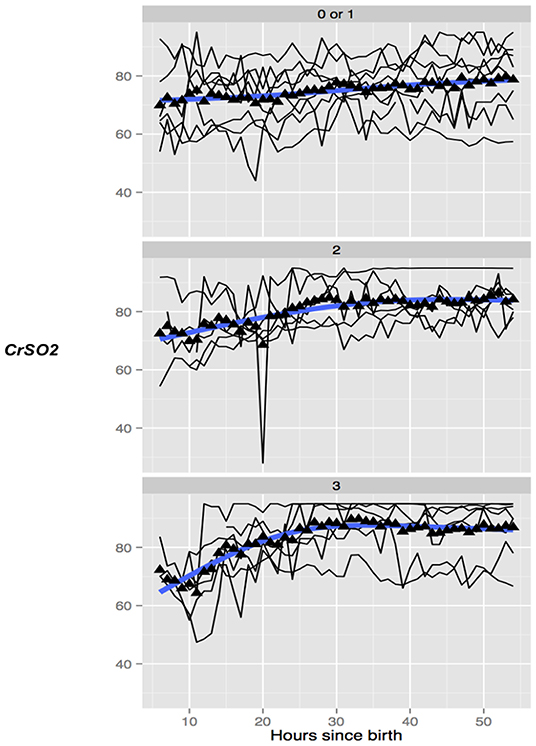
Figure 3. Trend of absolute cerebral tissue oxygen saturation (CrSO2) values in 3 groups of infants undergoing TH with none/mild (top), moderate (middle), and severe (bottom) grade of overall MRI injury. The mean values in the group are marked with a solid triangle while the individual infants are represented by thin black lines. CrSO2 in infants with no or mild MRI injury (top) remains relatively stable while in infants with moderate (middle) and severe (bottom) CrSO2 shows a trend toward an early increase. Reproduced with permission, Jain et al. (45).
The observed findings of this combined work reflect the known pathophysiological changes occurring in NE. The relative increase in absolute cerebral tissue oxygen saturation can be attributed to profound mitochondrial dysfunction during secondary energy failure leading to reduced oxygen utilization and therefore higher cerebral tissue saturations, the degree of which relates to injury severity. The elevated CBV within the first 24 h following injury reflects the hemodynamic disturbances of vasoparesis and hyperperfusion which also underpin the disease.
Monitoring Cerebral Autoregulation
Cerebral autoregulation (CAR) refers to the physiological ability of the body to maintain steady cerebral perfusion in response to changes in cerebral perfusion pressure (CPP) by modulating vascular resistance through arteriolar caliber (91). As a consequence of a hypoxic-ischaemic perinatal insult, vasoparesis occurs leading to impaired pressure autoregulation and irregularities in cerebral blood flow. This pressure passivity, or the inability to effectively regulate cerebral blood flow (CBF) in the setting of changes in systemic blood pressure has been well-documented in both animal and human studies and is implicated in the pathogenesis of secondary injury in NE (27, 28). Measuring CPP in neonates is difficult and so mean arterial blood pressure (MABP) has become a necessary surrogate. Numerous studies using NIRS monitoring have sought to characterize the autoregulatory disturbances occurring in encephalopathic newborns using MABP and proxy markers of cerebral perfusion. These are summarized in Table 2. As the relationship between variables is a complex and dynamic one, signal processing techniques used to define them have also seen steady advances in both time and frequency domain analysis techniques. Studies have compared NIRS measurements with injury outcomes to demonstrate how the degree of cerebral autoregulatory disturbance, or pressure passivity, often demonstrated by a change in reactivity limits as opposed to the loss of CAR altogether, can assist in predicting adverse outcomes. It is hypothesized that these unregulated changes in cerebral perfusion can cause uncoupling with cerebral energy metabolism resulting in further potential injury during secondary energy failure.
Using continuous NIRS and systemic monitoring Massaro et al. used spectral coherence to compare changes in MABP and HbD, a proxy for CBF, over time. An increase in coherence between these parameters demonstrated a pressure passive cerebral circulation, or disturbed autoregulation. They defined both a pressure passivity index (PPI) and gain to quantify the duration and magnitude, respectively of the passivity and found a higher PPI and gain, therefore higher duration and magnitude of cerebral pressure passivity, were predictive of adverse outcomes (death or detectable brain injury by MRI, P 0.001) (47). Govindan et al. demonstrated similar findings with a modified approach to the coherence analysis (48). Howlett et al. also conducted a NIRS-MABP study looking at changes in cerebral blood volume (CBV). They investigated a reactivity index (HVx) between CBV, measured as relative total tissue hemoglobin concentration (rTHb), and MABP to identify an optimal range of MABP (within 5 mmHg) in which the greatest pressure reactivity (MAPopt) of the cerebral vasculature was noted. A greater severity of brain injury on MRI was associated with more time spent with MABP below this MAPopt during rewarming while those with little to no injury spent more time with MABP within or above the MAPopt range (49). Further studies using the HVx reactivity index found similar results when comparing with 2-year neurodevelopmental outcomes; those infants with lower cognitive scores were found to have higher calculated MAPopt and spent more time below this range during rewarming. In contrast, infants with more favorable outcomes and higher cognitive scores had MABP with greater deviation above their calculated MAPopt (50). These results suggest a shift in the limits of optimal autoregulatory function following injury. Results of a larger study by Lee et al. during TH and rewarming comparing reactivity indices to MRI outcomes further support these findings. Infants with greatest deviation and longest time spent below their MAPopt had more pronounced injury in the white matter and paracentral gyri on MRI (51). Greater deviation below the MAPopt was also associated with restricted diffusion (low ADC values) on MRI in the posterior centrum semiovale and the posterior limb of the internal capsule (52). Chalak et al. were able to investigate more intricately the dynamic properties of cerebral autoregulation following insult. In a pilot study of infants undergoing TH and rewarming using a moving time window correlation (MWC), the relationship between MABP and absolute cerebral tissue oxygen saturation (SctO2) was quantified across multiple time scales. In infants with adverse outcomes at neurodevelopmental follow-up, large unprovoked fluctuations in MABP during TH were observed. Both in-phase and anti-phase correlations between MABP and SctO2 were seen at different time scales suggesting that infants with abnormal outcomes had two different patterns of impaired CAR dependent on the time scale studied (53). This was supported by the findings of a larger study by the same group which used wavelet coherence analysis, a time-frequency approach to characterize the same relationship between MABP and SctO2 during spontaneous oscillations in both. Cerebral autoregulation in babies with NE was found to be time-scale dependent in nature with spontaneous changes in MABP and SctO2 having both in-phase and anti-phase coherence at shorter and longer time scales, respectively. Increased coherence or cerebral pressure passivity was observed in infants with adverse outcomes on both MRI and neurodevelopment at 18–24 months and was often sustained for hours to days. In contrast, all of the neonates with normal clinical outcomes showed non-significant coherence between fluctuations in MABP and SctO2 most of the time during hypothermia (54).
These studies demonstrate a timeline in the refinement of data analysis in NIRS monitoring in order to quantify the significance of cerebral autoregulatory disturbance in neonatal encephalopathy and how this predicts injury severity and outcome. The development of NIRS based reactivity indices provides a potential physiological biomarker of injury severity which may help direct care by optimizing hemodynamic management in order to prevent secondary brain injury. Larger cohorts using agreed methods and measurements to quantify the autoregulatory disturbances however, are required before determining the full potential of these biomarkers.
NIRS Monitoring of the Metabolic State: Broadband NIRS
The development of secondary energy failure in NE and its relationship with the degree of injury and neurodevelopmental outcomes is well-established, indicating the importance of monitoring cerebral mitochondrial metabolism along with changes in perfusion. Multiple studies measuring absolute cerebral tissue oxygen saturation in both pre-TH and TH cohorts demonstrated that raised values particularly from 24 h after birth predicted adverse neurological outcome and increased severity of injury on MRI. These findings are explained by the onset of secondary energy failure which leads to reduced oxygen utilization by mitochondria and therefore higher cerebral tissue oxygen saturations. In order to characterize the metabolic disturbance in NE more accurately, we need to measure this metabolic disturbance directly. This has led to the development of broadband NIRS (BNIRS), a technological extension to the CW-NIRS platform. In addition to traditional hemoglobin chromophores, BNIRS technology allows us to measure changes in the redox state of another chromophore, cytochrome c oxidase (CCO). CCO is the terminal acceptor in the mitochondrial electron transport chain and is responsible for 90% of ATP production. Both the oxidized and reduced forms of CCO are chromophores with different absorption spectra in the NIR region. Accurate measurement of concentration changes in the oxidized form (oxCCO) therefore allows us to directly monitor brain metabolic state at a cellular level. CCO as a chromophore has been of interest in the NIRS community for some time as discussed in the seminal paper by Jobsis (92), however, efforts to accurately monitor concentration changes in-vivo proved challenging due to its relatively small concentration compared to that of hemoglobin, which dominates the spectral profile. Significant work was carried out by earlier research groups in the 1980's and 1990's with the development of various algorithms using different wavelength numbers aimed at accurately measuring the cytochrome c oxidase redox state in-vivo. An analysis of these algorithms (93) found that a higher number of wavelengths produced more accurate measurements. Several reviews summarizing this early work and its challenges are available (93–95). These challenges have since been resolved by the more recent development of the BNIRS technology. The instrument delivers a large number of wavelengths in the 780–900 nm region of NIR light. By using hundreds of wavelengths and excluding shorter wavelengths <780 nm, we see improved signal to noise ratios and a reduction in cross-talk artifacts between different chromophores, such as from HHb which has a large peak at 760 nm (95). Research groups have then utilized various algorithms, summarized by Matcher et al. (93) as well as the in-vivo spectra of the difference between oxidized and reduced CCO to derive a measurement of changes in its oxidation state (oxCCO) (95). Measuring changes in oxCCO therefore allows us to directly measure mitochondrial energy metabolism.
In a pre-clinical study of newborn piglets using BNIRS and (31P) MRS following an induced hypoxic-ischaemic injury, significant correlations between brain tissue changes in oxCCO and those of metabolite peak area ratios of phosphocreatine (PCr), inorganic phosphate (Pi) and nucleotide triphosphate (NTP), used to signal brain metabolic state, were noted during and after insult. The recovery of oxCCO but not HbT or HbD, was highly correlated with (31P) ratios and predictive of outcome at 48 h (60). A further pre-clinical experiment by the same group quantified the recovery in brain oxygenation (HbT and HbD) and metabolism (oxCCO) for up to 30 min following a hypoxic-ischaemic insult. The recovery fractions (RF) in BNIRS signals were then compared to (1H) MRS derived thalamic Lac/NAA as an established surrogate marker of neurodevelopmental outcome. The oxCCO RF cut-off threshold of 79% within 30 min of HI predicted injury severity with high sensitivity (100%) and specificity (93%) and offered a real-time biomarker of brain injury severity within 30 min following HI insult (61). Bale et al. described the new BNIRS instrument for clinical use (62) and carried out a feasibility study on six encephalopathic newborns. The study monitored NIRS variables for cerebral hemoglobin oxygenation and hemodynamics as well as mitochondrial oxygen utilization via oxCCO with analysis focussed on response to spontaneous oxygen desaturations. Changes in oxCCO during the desaturation events were significantly associated with injury severity (r = 0.91, p < 0.01) (62). A close correlation between oxCCO concentration changes and systemic variations was able to predict injury on (1H) MRS suggesting a higher oxygen dependency of mitochondrial metabolism in cases with unfavorable outcome (63). The relationship between cerebral oxygen delivery and mitochondrial oxidative metabolism also indicated injury severity during TH (64) and rewarming (65, 66). In the study by Mitra et al., the relationship between cerebral mitochondrial metabolism and oxygenation (measured as oxCCO and HbD, respectively) during rewarming became more impaired with rising Lac/NAA on (1H) MRS, reflective of injury severity (65). Results suggest that following severe hypoxia-ischaemia and cell death, cerebral metabolism failed to improve in spite of oxygen availability. Mitra et al. went on to develop a metabolic reactivity index using oxCCO and MABP using wavelet semblance (phase relationship) and noted a high semblance at 48 h after birth correlated well with injury severity on MRI imaging, Lac/NAA on (1H) MRS and neurodevelopmental outcomes (67).
These studies demonstrate a new potential biomarker of injury with a trend toward increased passivity of mitochondrial activity (changes in oxCCO) in response to systemic variations in the adverse outcome groups. This loss of metabolic reactivity is consistent with known pathophysiological changes of mitochondrial dysfunction and is possibly explained by lower cellular energy reserves in the more injured brain meaning CCO has less capacity to buffer changes in systemic variations (63).
Other Optical Indicators of Metabolic Disturbance in NE
Some studies have also sought to measure the metabolic changes occurring in neonatal encephalopathy using traditional cerebral oximetry. Two studies in the pre-TH and TH era, respectively (55, 56), measured the derived parameter of fractional tissue oxygen extraction (FTOE). This parameter utilizes the absolute cerebral tissue oxygen saturation (StO2, rSO2, rScO2) and systemic arterial saturations (SaO2) to represent the balance between oxygen delivery from systemic arterial supply and oxygen utilization by cerebral tissues through mitochondrial metabolic activity (FTOE= SaO2-StO2/SaO2). An increase in FTOE suggests an increase in oxygen consumption by cerebral tissue (seen as lower absolute cerebral tissue oxygen saturation) and a reduced FTOE would suggest reduced oxygen consumption or metabolic activity relative to oxygen delivery. In a pre-TH study by Toet et al., the rSO2 and FTOE remained stable in infants with normal outcomes whilst in those with an adverse outcome the rSO2 increased and the FTOE decreased after 24 h (rSO2: 65 vs. 84% at 12 and 48 h, respectively) (55). The same group carried out a further study in the TH era and similarly found that in the adverse outcome group using 18 month neurodevelopmental assessment, rScO2 reached high values from 24 h after birth onwards and values were significantly higher than in the favorable outcome group at 24, 36, 48, and 84 h postnatally. The mean FTOE value mirrored the patterns of rScO2 of both groups and became very low from 24 h of age onward in the adverse outcome group as compared with the favorable outcome group (56).
The cerebral metabolic rate of oxygen consumption (CMRO2) is another derived parameter of brain metabolic state. NIRS measurements of changes to oxy and deoxyhemoglobin as well as proxy markers for tissue blood flow combined with arteriovenous systemic oxygen saturations enable investigators to derive estimates for the relative changes in cerebral oxygen metabolism (rCMRO2). In a study by Grant et al. investigating a cohort of infants following perinatal insult in the pre-TH era, increased CBV and CMRO2 were sensitive markers in detecting evolving neonatal brain injury as evidenced on ultrasound and MRI (68). Another optical monitoring technology, diffuse correlation spectroscopy (DCS) which will be discussed in more detail, enables more direct measurement of microvascular cerebral blood flow (CBFi). Hybrid instrumentation combining DCS with FD-NIRS offers the possibility for a more accurate determination of this measurement, CMRO2i, and has been utilized so far in a single study of infants with NE undergoing TH (69). Cerebral metabolic rate of oxygen (CMRO2i) and CBF (CBFi) measurements were significantly lower than post-TH and age-matched controls (69). The difference in findings in the above two studies may be explained by a more severely affected cohort in the study by Grant et al. as well as the finding of a lower CBFi in the latter study, which was measured directly using DCS.
NIRS Monitoring in Combination With Other Neuromonitoring Tools
Several studies combined NIRS parameters with aEEG data to strengthen their ability to predict outcome in NE (56, 57). Lemmers et al. used the combined score of aEEG and absolute cerebral tissue oxygen saturation to improve the positive predictive ability for neurodevelopmental outcome to 91% from 62% and 67% individually (56), whilst Goeral et al. found similar strength in combining these two modalities, with highest predictive abilities of the combined scores between 18 h and 60 h of TH (57). Govindan et al. demonstrated the feasibility of quantifying neurovascular coupling (NVC), whereby neuronal activation produces hemodynamic changes and vice versa continuously in infants with NE undergoing therapeutic hypothermia. The coupling between simultaneous background EEG and NIRS was quantified by calculating spectral coherence between the two signals (58). Chalak et al. further investigated neurovascular coupling (NVC) using wavelet analysis of the dynamic coherence between aEEG and NIRS measured absolute cerebral tissue oxygen saturation. Significantly decreased NVC coherence was observed in encephalopathic newborns undergoing TH with adverse outcomes at 24 months compared to non-encephalopathic newborns (59).
The advantages of simultaneous NIRS monitoring in conjunction with video EEG in neonatal and pediatric epilepsy is summarized in the paper by Wallois et al. (96). The added information gained by NIRS monitoring of the metabolic and hemodynamic disturbances surrounding seizure activity may have the potential to predict seizures and/or optimize seizure management (96), which is highly advantageous in the NE population given the seizure burden in this disease and its independent contribution to neurological injury (19, 97).
Diffuse Correlation Spectroscopy
Cerebral blood flow is a vital component of brain health linking metabolic demand to oxygen delivery and in turn, oxygen utilization to by-product clearance. Its dynamic properties are integral to brain autoregulatory functioning and neurovascular coupling so the ability to measure this more directly in NE is highly desirable given the pathophysiology effecting these processes in affected newborns. Diffuse correlation spectroscopy (DCS) is a relatively new optical technology which measures fluctuations in emitted light caused by scatter occurring within moving cells in tissues, predominantly by red blood cells. DCS shares the light penetration advantages of NIRS but as it explicitly measures cell movement, it provides a direct measurement of quantities such as cerebral blood flow (CBF) (98). As the measured temporal fluctuations are directly proportional to the speed of the scatter and the tissue within which it is moving, DCS can be used to directly measure micro-vascular blood flow in the brain by providing a cerebral blood flow index (CBFi, cm2/s) (99). As the diffusing light is mostly absorbed when traversing large arteries and veins, DCS is most sensitive to the motion of blood cells in the microvasculature (98). Abnormalities in brain perfusion following a hypoxic-ischaemic insult have previously been investigated using proxy markers for large vessel blood flow provided by cerebral oximetry, such as HbD and HbT, representing CBF and CBV, respectively. By measuring local microvascular blood flow directly and continuously, DCS can capture the dynamic nature of autoregulatory disturbances which is a desirable addition to our neuromonitoring potential. The addition of a direct blood flow measurement has also had a significant impact on another all-optical measurement of metabolic state, CMRO2i.
As seen with NIRS technology, infants make excellent populations to study with DCS due to thinner extracerebral layers and generally less hair leading to higher observed signal to noise ratio (SNR), excellent reproducibility of CBFi and greater sensitivity to cortical tissue compared to adults (100). None of the alternative technologies currently available for measuring CBF such as positron emission tomography (PET), arterial spin labeling MR (ASL-MR), single photon emission computed tomography (SPECT) or Xenon and dynamic computed tomography (CT), are suitable for monitoring at the cot side in NICU due to their impractical size or use of ionizing radiation. Thus, an opportunity exists for DCS capabilities to measure CBF easily, non-invasively, safely, and continuously at the cot side. Validation studies of CBFi have been carried out with dynamic contrast enhanced TD-NIRS (101) and BNIRS (102) using Indocyanine green (ICG) as a flow tracer, fluorescent microspheres (103) as well as ASL-MR (104). All have shown very good correlation between measurements of cerebral blood flow. DCS monitoring of CBF has been employed in a wide range of research contexts in the neonatal population including various preterm cohorts (89, 102, 105, 106), in post-op cardiac surgery (107) and in neonates and children born with severe congenital heart disease (104). In the preterm cohorts, a combination of FDNIRS with DCS simultaneously measured parameters of absolute cerebral tissue oxygen saturation (StO2), CBV and blood flow index (CBFi) to determine their relationships with gestational age and chronological age (105). Combining DCS and FD-NIRS parameters, changes in the rCMRO2 were also measured serially over the first 6 weeks of preterm life (89) and following functional activation (106). The study by Diop et al. (102) using a combined BNIRS and DCS platform successfully monitored CBFi and CMRO2 in a preterm population undergoing Indomethacin treatment for PDA with additional measurements of CBF using contrast enhanced NIRS to calibrate with DCS measurements. Whilst there was good correlation with the ICG flow tracer measurements of CBF, the continuous and non-invasive monitoring provided by DCS in the neonatal population has obvious advantages over methods requiring injections of exogenous tracers that provide few measurements. A single study by Dehaes et al. utilizing the hybrid FDNIRS-DCS device in newborns with NE has been described earlier (69) and represents an exciting direction in optical instrumentation with hybrid platforms offering the ability to simultaneously measure important parameters such as blood flow and metabolism more directly in this disease. Further hybrid examples will be discussed in new and emerging technology.
New Optical Monitors and Future Directions
New Commercial Monitors
Newer commercial devices have been developed for use particularly in the preterm population in an effort to identify variations that may precede preterm brain injury. The BabyLux (TRS NIRS+DCS) monitor has been developed to measure cerebral blood flow and absolute cerebral tissue oxygen saturation in the preterm population. The technology has integrated DCS with time-resolved (TRS) NIRS which is an evolution of CW-NIRS and offers absolute hemoglobin concentrations and tissue saturations (108). Oxyprem is a NIRS based cerebral oximeter which utilizes a self-correcting algorithm to cancel multiple in-homogeneities beneath the light source or detector and increase robustness to motion artifacts thus providing a more accurate measurement of absolute cerebral tissue oxygen saturation (StO2) (78).
Emerging Optical Research Instruments
Several research groups are pushing the boundaries of current developments in new optical systems to monitor a wider range of pathophysiological information in NE in real time. Metaox is a new commercial FDNIRS-DCS hybrid instrument. Measuring arterial oxygen saturation from pulse oximeters, quantitative measurements of Hb concentration and oxygenation using FD-NIRS and CBFi using DCS, the instrument derives the cerebral oxygen metabolism index (CMRO2i) (89, 109). Diffuse Optical Tomography (DOT) is an extension of functional NIRS (fNIRS) that combines hemodynamic information from dense optical sensor arrays and using image reconstruction techniques, can provide images of the hemodynamic correlates to neural function that are comparable to those produced by functional MR imaging (110). The UCL group in London is currently using a new dual optical platform combining BNIRS and DCS, developed in collaboration with the researchers in ICFO Barcelona, for direct measurements of mitochondrial oxidative metabolism, cerebral oxygenation and CBFi in NE. This instrument has the potential to further improve our understanding of pathophysiology following perinatal hypoxic ischaemic injury. Rajaram et al. have used a similar combined BNIRS and DCS platform in a pre-clinical piglet experiment to provide information regarding clinically significant hemodynamic events prior to the onset of brain injury, which holds great potential for use particularly in the preterm neonatal population (70). The same group have also used this dual platform to monitor cerebral hemodynamics and metabolism in a cohort of neonatal patients with post-haemorrhagic ventricular dilatation undergoing ventricular tap (111). In the NE population, this dual monitoring will pave the way for further work utilizing the combined measurements of metabolic and autoregulatory disturbances in order to develop a robust cot side biomarker of injury.
Limitations
Despite being well-suited to the neonatal population, current NIRS technology still poses some limitations. Hair, scalp oedema, movement artifact and strong light from additional sources all have the potential to cause interference with NIRS monitoring and must be carefully accounted for. Following brain insult, pathophysiological changes may show regional variation and although NIRS technology achieves good tissue penetration in neonates, spatial resolution can be limited. Research groups are currently applying other forms of NIRS technology using 3D image reconstruction such as diffuse optical tomography (DOT) which may better demonstrate spatial variations in NIRS parameters across the cortex (110, 112). Manufacturers use a range of different techniques and algorithms, most unpublished, to determine absolute cerebral tissue oxygen saturation values. Although there is good correlation between some devices, further work is needed to allow easier conversion of values from one oximeter to another and a uniform terminology is needed in order for readers to better compare findings from different studies. Various neonatal probes are also in use on the same commercial devices producing different measurements hence it is important to state the probes used with reference values for each study. There is also much variability in outcome measures between the studies described here and therefore larger cohort optical studies are needed using commonly agreed gold standard outcomes and similar methodology. Current oxCCO measurements in the hospital provide trend information of the metabolic status of the brain tissue and this trend information in combination with the continuous blood pressure measurements can derive an absolute quantification of brain metabolic autoregulation that can prognosticate infants with NE (67). Recent work in the preclinical model of HIE (61) also demonstrated that an absolute measurement of the redox state of CCO, early following hypoxic-ischaemic injury, will provide an enhanced marker of diagnosis of brain injury severity; this will need further developments in algorithms and instrumentation to deliver this in the hospital (113).
Conclusion
The understanding of pathophysiology in NE with its biphasic and continuous evolution of injury has helped shape the type of neuromonitoring tools that are most desired by researchers and clinicians alike. A reliable neuromonitoring device should be safe and easy to use at the cot side and provide continuous monitoring that can be interpreted in real time by clinicians in the hope of directing clinical care, assessing response to neuroprotective treatments and contribute valuable prognostic information of neurological outcome. Current neuromonitoring tools such as EEG/aEEG continue to play a vital role particularly in seizure detection and management but their ability to prognosticate outcome early on in disease progression has been ameliorated with the introduction of therapeutic hypothermia. MRI/MRS, whilst being the current gold standard technique in brain injury stratification and prognostication provides snapshot information days post potential early treatment windows. A real need has developed to advance the neuromonitoring potential in NE and optical technology is paving the way to fulfilling that need with significant advances in both the technology itself and data analysis of its outputs.
Near infrared spectroscopy has developed substantially over the past 4 decades with increasing focus in the last decade on its use in the monitoring of neonatal brain injury in intensive care. There have been significant advancements in both research and commercial devices along with the use of more complex signal processing techniques which allow the dynamic relationships between multiple measurements to be characterized to form real time biomarkers of injury as they are related to gold standard outcome measures. Cerebral oximetry is undergoing improvements in both the incorporated NIRS modes and the algorithms used in order to make measurements of cerebral tissue oxygen saturation more accurate and reduce known interference. The evolution of broadband NIRS has meant that a long sought after brain tissue biomarker, Cytochrome C oxidase, can now be measured to more directly monitor the oxygen utilization of mitochondria in the injured brain. The all-optical measurement of CMRO2i is also proving to be a useful measurement of brain metabolic state. Another key factor in the pathophysiology underpinning NE, altered cerebral autoregulation, has been characterized so far with NIRS monitoring by the development of advanced signal processing techniques and the formation of reactivity indices which have shown promise as potential biomarkers of injury. These reactivity indices provide further potential windows of opportunity for improving outcomes by optimizing cardiovascular stability and MABP. A further optical technology developed more recently, diffuse correlation spectroscopy, will have a major impact on the optical neuromonitoring potential in NE due to its ability to directly measure microvascular blood flow allowing more accurate calculation of autoregulatory changes and CMRO2i. When used in combination with NIRS platforms our ability to monitor brain health following injury is further increased.
The ultimate goal in neuromonitoring in NE is to identify a robust and accurate cot side biomarker, one that can be employed by neonatologists within hours of birth to track injury evolution in real time, identify eligible infants for appropriate neuroprotective treatments and assess their response, accurately stratify injury severity and provide early prognostication of outcome to assist in directing care and improving outcomes. Optical monitoring technology has shown much promise in achieving this goal and ongoing work in this area hopes to ensure a suitable biomarker of cerebral well-being is on the horizon.
Author Contributions
KH-J and SM conceptualized the review and completed the first draft. FL, IT, and NR contributed to further revision and final version of the submitted manuscript. All authors contributed to the article and approved the submitted version.
Funding
The authors are supported by The Wellcome Trust (219610/Z/19/Z) and Medical Research Council (MR/S003134/1) and National Institute for Health Research University College London Hospitals Biomedical Research Center.
Conflict of Interest
The authors declare that the research was conducted in the absence of any commercial or financial relationships that could be construed as a potential conflict of interest.
References
1. Lee AC, Kozuki N, Blencowe H, Vos T, Bahalim A, Darmstadt GL, et al. Intrapartum-related neonatal encephalopathy incidence and impairment at regional and global levels for 2010 with trends from (1990). Pediatr Res. (2013) 74(Suppl. 1):50–72. doi: 10.1038/pr.2013.206
2. Liu L, Oza S, Hogan D, Perin J, Rudan I, Lawn JE, et al. Global, regional, and national causes of child mortality in 2000–13, with projections to inform post-2015 priorities: an updated systematic analysis. Lancet. (2015) 385:430–40. doi: 10.1016/S0140-6736(14)61698-6
3. Lawn JE, Kinney M, Lee AC, Chopra M, Donnay F, Paul VK, et al. Reducing intrapartum-related deaths and disability: can the health system deliver? Int J Gynaecol Obstet. (2009) 107(Suppl 1):S123-40:S40–2. doi: 10.1016/j.ijgo.2009.07.021
4. du Plessis AJ, Volpe JJ. Perinatal brain injury in the preterm and term newborn. Curr Opin Neurol. (2002) 15:151–7. doi: 10.1097/00019052-200204000-00005
5. Hope PL, Costello AM, Cady EB, Delpy DT, Tofts PS, Chu A, et al. Cerebral energy metabolism studied with phosphorus NMR spectroscopy in normal and birth-asphyxiated infants. Lancet. (1984) 2:366–70. doi: 10.1016/S0140-6736(84)90539-7
6. Azzopardi D. Prognosis of newbron infants with hypoxic-ischaemic brain injury assessed by phosphorous magnetic resonance spectroscopy. Pediatric Res. (1989) 25:445–51. doi: 10.1203/00006450-198905000-00004
7. Lorek A, Takei Y, Cady EB, Wyatt JS, Penrice J, Edwards AD, et al. Delayed (“secondary”) cerebral energy failure after acute hypoxia-ischemia in the newborn piglet: continuous 48-hour studies by phosphorus magnetic resonance spectroscopy. Pediatric Res. (1994) 36:699–706. doi: 10.1203/00006450-199412000-00003
8. Thoresen M, Penrice J, Lorek A, Cady EB, Wylezinska M, Kirkbride V, et al. Mild hypothermia after severe transient hypoxia-ischemia ameliorates delayed cerebral energy failure in the newborn piglet. Pediatric Res. (1995) 37:667–70. doi: 10.1203/00006450-199505000-00019
9. Hassell KJ, Ezzati M, Alonso-Alconada D, Hausenloy DJ, Robertson NJ. New horizons for newborn brain protection: enhancing endogenous neuroprotection. Arch Dis Child Fetal Neonatal Ed. (2015) 100:F541–52. doi: 10.1136/archdischild-2014-306284
10. Laptook AR, Corbett RJ, Sterett R, Burns DK, Garcia D, Tollefsbol G. Modest hypothermia provides partial neuroprotection when used for immediate resuscitation after brain ischemia. Paediatric Res. (1997) 42:17–23. doi: 10.1203/00006450-199707000-00004
11. Laptook AR, Corbett RJ, Sterett R, Burns DF, Tollefsbol G, Garcia D. Modest hypothermia provides partial neuroprotection for ischemic neonatal brain. Pediatric Res. (1994) 35:436–42. doi: 10.1203/00006450-199404000-00010
12. Gunn TR, Gunn AJ, Gluckman PD. Substantial neuronal loss with prolonged selective head cooling begun 5.5h after cerebral ischemia in the fetal sheep. Pediatric Res. (1997) 41:152. doi: 10.1203/00006450-199704001-00917
13. Jacobs SE, Berg M, Hunt R, Tarnow-Mordi WO, Inder TE, Davis PG. Cooling for newborns with hypoxic ischaemic encephalopathy. Cochrane Database Syst Rev. (2013) 2013:CD003311. doi: 10.1002/14651858.CD003311.pub3
14. Edwards AD, Brocklehurst P, Gunn AJ, Halliday H, Juszczak E, Levene M, et al. Neurological outcomes at 18 months of age after moderate hypothermia for perinatal hypoxic ischaemic encephalopathy: synthesis and meta-analysis of trial data. BMJ. (2010) 340:c363. doi: 10.1136/bmj.c363
15. Hellström-Westas L, Rosén I, de Vries LS, Greisen G. Amplitude-integrated EEG classification and interpretation in preterm and term infants. Neo Rev. (2006) 7:e76–87. doi: 10.1542/neo.7-2-e76
16. Martinello K, Hart AR, Yap S, Mitra S, Robertson NJ. Management and investigation of neonatal encephalopathy: 2017 update. Arch Dis Child Fetal Neonatal Ed. (2017) 102:F346–F58. doi: 10.1136/archdischild-2015-309639
17. Awal MA, Lai MM, Azemi G, Boashash B, Colditz PB. EEG background features that predict outcome in term neonates with hypoxic ischaemic encephalopathy: A structured review. Clin Neurophysiol. (2016) 127:285–96. doi: 10.1016/j.clinph.2015.05.018
18. Thoresen M, Hellström-Westas L, Liu X, de Vries LS. Effect of hypothermia on amplitude-integrated electroencephalogram in infants with asphyxia. Pediatrics. (2010) 126:131–9. doi: 10.1542/peds.2009-2938
19. Shah DK, Wusthoff CJ, Clarke P, Wyatt JS, Ramaiah SM, Dias RJ, et al. Electrographic seizures are associated with brain injury in newborns undergoing therapeutic hypothermia. Arch Dis Child Fetal Neonatal Ed. (2014) 99:F219–24. doi: 10.1136/archdischild-2013-305206
20. Srinivasakumar P, Zempel J, Trivedi S, Wallendorf M, Rao R, Smith B, et al. Treating EEG seizures in hypoxic ischemic encephalopathy: a randomized controlled trial. Pediatrics. (2015) 136:1302–9. doi: 10.1542/peds.2014-3777
21. Mastrangelo M, Fiocchi I, Fontana P, Gorgone G, Lista G, Belcastro V. Acute neonatal encephalopathy and seizures recurrence: a combined aEEG/EEG study. Seizure. (2013) 22:703–7. doi: 10.1016/j.seizure.2013.05.006
22. Shellhaas RA, Barks AK. Impact of amplitude-integrated electroencephalograms on clinical care for neonates with seizures. Pediatr Neurol. (2012) 46:32–5. doi: 10.1016/j.pediatrneurol.2011.11.004
23. Kadivar M, Moghadam EM, Shervin Badv R, Sangsari R, Saeedy M. A comparison of conventional electroencephalography with amplitude-integrated EEG in detection of neonatal seizures. Med Devices. (2019) 12:489–96. doi: 10.2147/MDER.S214662
24. Murray DM, Boylan GB, Ali I, Ryan CA, Murphy BP, Connolly S. Defining the gap between electrographic seizure burden, clinical expression and staff recognition of neonatal seizures. Arch Dis Child Fetal Neonatal Ed. (2008) 93:F187–91. doi: 10.1136/adc.2005.086314
25. Merchant N, Azzopardi D. Early predictors of outcome in infants treated with hypothermia for hypoxic-ischaemic encephalopathy. Dev Med Child Neurol. (2015) 57:8–16. doi: 10.1111/dmcn.12726
26. Archer LN, Levene MI, Evans DH. Cerebral artery doppler ultrasonography for prediction of outcome after perinatal asphyxia. Lancet. (1986) 2:1116–8. doi: 10.1016/S0140-6736(86)90528-3
27. Meek J. Abnormal cerebral hemodynamics in perinatally asphyxiated neonates related to outcome. Arch Dis Child Fetal Neonatal Ed. (1999) 81:F110–F5. doi: 10.1136/fn.81.2.F110
28. Pryds O, Greisen G, Lou H, Friis-Hansen B. Vasoparalysis associated with brain damage in asphyxiated term infants. J Pediatrics. (1990) 117:119–25. doi: 10.1016/S0022-3476(05)72459-8
29. Elstad M, Whitelaw A, Thoresen M. Cerebral resistance index is less predictive in hypothermic encephalopathic newborns. Acta Paediatr. (2011) 100:1344–9. doi: 10.1111/j.1651-2227.2011.02327.x
30. Martinez-Biarge M, Diez-Sebastian J, Kapellou O, Gindner D, Allsop JM, Rutherford MA, et al. Predicting motor outcome and death in term hypoxic-ischemic encephalopathy. Neurology. (2011) 76:2055–61. doi: 10.1212/WNL.0b013e31821f442d
31. Cheong JL, Coleman L, Hunt RW, Lee KJ, Doyle LW, Inder TE, et al. Prognostic utility of magnetic resonance imaging in neonatal hypoxic-ischemic encephalopathy: substudy of a randomized trial. Arch Pediatr Adolesc Med. (2012) 166:634–40. doi: 10.1001/archpediatrics.2012.284
32. Rutherford M, Ramenghi LA, Edwards AD, Brocklehurst P, Halliday H, Levene M, et al. Assessment of brain tissue injury after moderate hypothermia in neonates with hypoxic-ischaemic encephalopathy: a nested substudy of a randomised controlled trial. Lancet Neurol. (2010) 9:39–45. doi: 10.1016/S1474-4422(09)70295-9
33. British Association of Perinatal Medicine (BAPM). Therapeutic Hypothermia for Neonatal Encephalopathy: A Framework for Practice (2020).
34. Lally PJ, Montaldo P, Oliveira V, Soe A, Swamy R, Bassett P, et al. Magnetic resonance spectroscopy assessment of brain injury after moderate hypothermia in neonatal encephalopathy: a prospective multicentre cohort study. Lancet Neurol. (2019) 18:35–45. doi: 10.1016/S1474-4422(18)30325-9
35. Mitra S, Kendall GS, Bainbridge A, Sokolska M, Dinan M, Uria-Avellanal C, et al. Proton magnetic resonance spectroscopy lactate/N-acetylaspartate within 2 weeks of birth accurately predicts 2-year motor, cognitive and language outcomes in neonatal encephalopathy after therapeutic hypothermia. Arch Dis Child Fetal Neonatal Ed. (2019) 104:424–32. doi: 10.1136/archdischild-2018-315478
36. van Bel F, Dorrepaal CA, Benders MJ, Zeeuwe PE, van de Bor M, Berger HM. Changes in cerebral hemodynamics and oxygenation in the first 24 hours after birth asphyxia. Pediatrics. (1993) 92:365–72.
37. Zaramella P, Saraceni E, Freato F, Falcon E, Suppiej A, Milan A, et al. Can tissue oxygenation index (TOI) and cotside neurophysiological variables predict outcome in depressed/asphyxiated newborn infants?. Early Hum Dev. (2007) 83:483–9. doi: 10.1016/j.earlhumdev.2006.09.003
38. Nakamura S, Koyano K, Jinnai W, Hamano S, Yasuda S, Konishi Y, et al. Simultaneous measurement of cerebral hemoglobin oxygen saturation and blood volume in asphyxiated neonates by near-infrared time-resolved spectroscopy. Brain Dev. (2015) 37:925–32. doi: 10.1016/j.braindev.2015.04.002
39. Ancora G, Maranella E, Locatelli C, Pierantoni L, Faldella G. Changes in cerebral hemodynamics and amplitude integrated EEG in an asphyxiated newborn during and after cool cap treatment. Brain Dev. (2009) 31:442–4. doi: 10.1016/j.braindev.2008.06.003
40. Niezen CK, Bos AF, Sival DA, Meiners LC, Ter Horst HJ. Amplitude-integrated EEG and cerebral near-infrared spectroscopy in cooled, asphyxiated infants. Am J Perinatol. (2018) 35:904–10. doi: 10.1055/s-0038-1626712
41. Ancora G, Maranella E, Grandi S, Sbravati F, Coccolini E, Savini S, et al. Early predictors of short term neurodevelopmental outcome in asphyxiated cooled infants. a combined brain amplitude integrated electroencephalography and near infrared spectroscopy study. Brain Dev. (2013) 35:26–31. doi: 10.1016/j.braindev.2011.09.008
42. Arriaga-Redondo M, Arnaez J, Benavente-Fernández I, Lubián-López S, Hortigüela M, Vega-Del-Val C, et al. Lack of variability in cerebral oximetry tendency in infants with severe hypoxic-ischemic encephalopathy under hypothermia. Ther Hypothermia Temp Manag. (2019) 9:243–50. doi: 10.1089/ther.2018.0041
43. Wintermark P, Hansen A, Warfield SK, Dukhovny D, Soul JS. Near-infrared spectroscopy versus magnetic resonance imaging to study brain perfusion in newborns with hypoxic-ischemic encephalopathy treated with hypothermia. Neuroimage. (2014) 85:287–93. doi: 10.1016/j.neuroimage.2013.04.072
44. Peng S, Boudes E, Tan X, Saint-Martin C, Shevell M, Wintermark P. Does near-infrared spectroscopy identify asphyxiated newborns at risk of developing brain injury during hypothermia treatment? Am J Perinatol. (2015) 32:555–64. doi: 10.1055/s-0034-1396692
45. Jain SV, Pagano L, Gillam-Krakauer M, Slaughter JC, Pruthi S, Engelhardt B. Cerebral regional oxygen saturation trends in infants with hypoxic-ischemic encephalopathy. Early Hum Dev. (2017) 113:55–61. doi: 10.1016/j.earlhumdev.2017.07.008
46. Szakmar E, Smith J, Yang E, Volpe JJ, Inder T, El-Dib M. Association between cerebral oxygen saturation and brain injury in neonates receiving therapeutic hypothermia for neonatal encephalopathy. J Perinatol. (2021) 41:269–77. doi: 10.1038/s41372-020-00910-w
47. Massaro AN, Govindan RB, Vezina G, Chang T, Andescavage NN, Wang Y, et al. Impaired cerebral autoregulation and brain injury in newborns with hypoxic-ischemic encephalopathy treated with hypothermia. J Neurophysiol. (2015) 114:818–24. doi: 10.1152/jn.00353.2015
48. Govindan RB, Massaro AN, Andescavage NN, Chang T, du Plessis A. Cerebral pressure passivity in newborns with encephalopathy undergoing therapeutic hypothermia. Front Hum Neurosci. (2014) 8:266. doi: 10.3389/fnhum.2014.00266
49. Howlett JA, Northington FJ, Gilmore MM, Tekes A, Huisman TA, Parkinson C, et al. Cerebrovascular autoregulation and neurologic injury in neonatal hypoxic-ischemic encephalopathy. Pediatr Res. (2013) 74:525–35. doi: 10.1038/pr.2013.132
50. Burton VJ, Gerner G, Cristofalo E, Chung SE, Jennings JM, Parkinson C, et al. A pilot cohort study of cerebral autoregulation and 2-year neurodevelopmental outcomes in neonates with hypoxic-ischemic encephalopathy who received therapeutic hypothermia. BMC Neurol. (2015) 15:209. doi: 10.1186/s12883-015-0464-4
51. Lee JK, Poretti A, Perin J, Huisman T, Parkinson C, Chavez-Valdez R, et al. Optimizing cerebral autoregulation may decrease neonatal regional hypoxic-ischemic brain injury. Dev Neurosci. (2017) 39:248–56. doi: 10.1159/000452833
52. Tekes A, Poretti A, Scheurkogel MM, Huisman TA, Howlett JA, Alqahtani E, et al. Apparent diffusion coefficient scalars correlate with near-infrared spectroscopy markers of cerebrovascular autoregulation in neonates cooled for perinatal hypoxic-ischemic injury. AJNR Am J Neuroradiol. (2015) 36:188–93. doi: 10.3174/ajnr.A4083
53. Chalak LF, Tian F, Tarumi T, Zhang R. Cerebral hemodynamics in asphyxiated newborns undergoing hypothermia therapy: pilot findings using a multiple-time-scale analysis. Pediatr Neurol. (2016) 55:30–6. doi: 10.1016/j.pediatrneurol.2015.11.010
54. Tian F, Tarumi T, Liu H, Zhang R, Chalak L. Wavelet coherence analysis of dynamic cerebral autoregulation in neonatal hypoxic-ischemic encephalopathy. Neuroimage Clin. (2016) 11:124–32. doi: 10.1016/j.nicl.2016.01.020
55. Toet MC, Lemmers PMA, van Schelven LJ, van Bel F. Cerebral oxygenation and electrical activity after birth asphyxia: their relation to outcome. Paediatrics. (2006) 117:333–9. doi: 10.1542/peds.2005-0987
56. Lemmers PM, Zwanenburg RJ, Benders MJ, de Vries LS, Groenendaal F, van Bel F, et al. Cerebral oxygenation and brain activity after perinatal asphyxia: does hypothermia change their prognostic value? Pediatr Res. (2013) 74:180–5. doi: 10.1038/pr.2013.84
57. Goeral K, Urlesberger B, Giordano V, Kasprian G, Wagner M, Schmidt L, et al. Prediction of outcome in neonates with hypoxic-ischemic encephalopathy II: role of amplitude-integrated electroencephalography and cerebral oxygen saturation measured by near-infrared spectroscopy. Neonatology. (2017) 112:193–202. doi: 10.1159/000468976
58. Govindan RB, Massaro A, Chang T, Vezina G, du Plessis A. A novel technique for quantitative bedside monitoring of neurovascular coupling. J Neurosci Methods. (2016) 259:135–42. doi: 10.1016/j.jneumeth.2015.11.025
59. Chalak LF, Tian F, Adams-Huet B, Vasil D, Laptook A, Tarumi T, et al. Novel wavelet real time analysis of neurovascular coupling in neonatal encephalopathy. Sci Rep. (2017) 7:45958. doi: 10.1038/srep45958
60. Bainbridge A, Tachtsidis I, Faulkner SD, Price D, Zhu T, Baer E, et al. Brain mitochondrial oxidative metabolism during and after cerebral hypoxia-ischemia studied by simultaneous phosphorus magnetic-resonance and broadband near-infrared spectroscopy. Neuroimage. (2014) 102:173–83. doi: 10.1016/j.neuroimage.2013.08.016
61. Kaynezhad P, Mitra S, Bale G, Bauer C, Lingam I, Meehan C, et al. Quantification of the severity of hypoxic-ischemic brain injury in a neonatal preclinical model using measurements of cytochrome-c-oxidase from a miniature broadband-near-infrared spectroscopy system. Neurophotonics. (2019) 6:045009. doi: 10.1117/1.NPh.6.4.045009
62. Bale G, Mitra S, Meek J, Robertson N, Tachtsidis I. A new broadband near-infrared spectroscopy system for in-vivo measurements of cerebral cytochrome-c-oxidase changes in neonatal brain injury. Biomed Opt Express. (2014) 5:3450–66. doi: 10.1364/BOE.5.003450
63. Bale G. Interrelationship between broadband NIRS measurements of cerebral cytochrome C oxidase and systemic changes indicates injury severity in neonatal encephalopathy. Adv Exp Med Biol. (2016) 923:E3. doi: 10.1007/978-3-319-38810-6_24
64. Bale G, Mitra S, de Roever I, Sokolska M, Price D, Bainbridge A, et al. Oxygen dependency of mitochondrial metabolism indicates outcome of newborn brain injury. J Cereb Blood Flow Metab. (2019) 39:2035–47. doi: 10.1177/0271678X18777928
65. Mitra S, Bale G, Meek J, Uria-Avellanal C, Robertson NJ, Tachtsidis I. Relationship between cerebral oxygenation and metabolism during rewarming in newborn infants after therapeutic hypothermia following hypoxic-ischaemic brain injury. Adv Exp Med Biol. (2016) 923:E3. doi: 10.1007/978-3-319-38810-6_33
66. Mitra S, Bale G, de Roever I, Meek J, Robertson NJ, Tachtsidis I. Changes in brain tissue oxygenation and metabolism during rewarming after neonatal encephalopathy are related to electrical abnormality. Adv Exp Med Biol. (2020) 1232:25–31. doi: 10.1007/978-3-030-34461-0_4
67. Mitra S, Bale G, Highton D, Gunny R, Uria-Avellanal C, Bainbridge A, et al. Pressure passivity of cerebral mitochondrial metabolism is associated with poor outcome following perinatal hypoxic ischemic brain injury. J Cereb Blood Flow Metab. (2019) 39:118–30. doi: 10.1177/0271678X17733639
68. Grant PE, Roche-Labarbe N, Surova A, Themelis G, Selb J, Warren EK, et al. Increased cerebral blood volume and oxygen consumption in neonatal brain injury. J Cereb Blood Flow Metab. (2009) 29:1704–13. doi: 10.1038/jcbfm.2009.90
69. Dehaes M, Aggarwal A, Lin PY, Rosa Fortuno C, Fenoglio A, Roche-Labarbe N, et al. Cerebral oxygen metabolism in neonatal hypoxic ischemic encephalopathy during and after therapeutic hypothermia. J Cereb Blood Flow Metab. (2014) 34:87–94. doi: 10.1038/jcbfm.2013.165
70. Rajaram A, Bale G, Kewin M, Morrison LB, Tachtsidis I, St Lawrence K, et al. Simultaneous monitoring of cerebral perfusion and cytochrome c oxidase by combining broadband near-infrared spectroscopy and diffuse correlation spectroscopy. Biomed Opt Express. (2018) 9:2588–603. doi: 10.1364/BOE.9.002588
71. Soul JS, Taylor GA, Wypij D, Duplessis AJ, Volpe JJ. Noninvasive detection of changes in cerebral blood flow by near-infrared spectroscopy in a piglet model of hydrocephalus. Pediatr Res. (2000) 48:445–9. doi: 10.1203/00006450-200010000-00005
72. Tsuji M, duPlessis A, Taylor G, Crocker R, Volpe JJ. Near infrared spectroscopy detects cerebral ischemia during hypotension in piglets. Pediatr Res. (1998) 44:591–5. doi: 10.1203/00006450-199810000-00020
73. Wolf M, Naulaers G, van Bel F, Kleiser S, Greisen G. A review of near infrared spectroscopy for term and preterm newborns. J Near Infrared Spectroscopy. (2012) 20:43–55. doi: 10.1255/jnirs.972
74. Menke J, Moller G, Jorch G. Reproducibility of cerebral near infrared spectroscopy in neonates. Biol Neonate. (2003) 83:6–11. doi: 10.1159/000067006
75. Hyttel-Sorensen S, Hessel TW, la Cour A, Greisen G. A comparison between two NIRS oximeters (INVOS, OxyPrem) using measurement on the arm of adults and head of infants after caesarean section. Biomed Opt Express. (2014) 5:3671–83. doi: 10.1364/BOE.5.003671
76. Sorensen LC, Greisen G. Precision of measurement of cerebral tissue oxygenation index using near-infrared spectroscopy in preterm neonates. J Biomed Opt. (2006) 11:054005. doi: 10.1117/1.2357730
77. Kleiser S, Nasseri N, Andresen B, Greisen G, Wolf M. Comparison of tissue oximeters on a liquid phantom with adjustable optical properties. Biomed Opt Express. (2016) 7:2973–92. doi: 10.1364/BOE.7.002973
78. Kleiser S, Ostojic D, Nasseri N, Isler H, Bucher HU, Bassler D, et al. In vivo precision assessment of a near-infrared spectroscopy-based tissue oximeter (OxyPrem v1.3) in neonates considering systemic hemodynamic fluctuations. J Biomed Opt. (2018) 23:1–10. doi: 10.1117/1.JBO.23.6.067003
79. Arri SJ, Muehlemann T, Biallas M, Bucher HU, Wolf M. Precision of cerebral oxygenation and hemoglobin concentration measurements in neonates measured by near-infrared spectroscopy. J Biomed Opt. (2011) 16:047005. doi: 10.1117/1.3570303
80. Franceschini MA, Thaker S, Themelis G, Krishnamoorthy KK, Bortfeld H, Diamond SG, et al. Assessment of infant brain development with frequency-domain near-infrared spectroscopy. Pediatr Res. (2007) 61:546–51. doi: 10.1203/pdr.0b013e318045be99
81. Dix LML, van Bel F, Lemmers PMA. Monitoring cerebral oxygenation in neonates: an update. Front Pediatrics. (2017) 5:160. doi: 10.3389/fped.2017.00160
82. Garvey AA, Dempsey EM. Applications of near infrared spectroscopy in the neonate. Curr Opin Pediatr. (2018) 30:209–15. doi: 10.1097/MOP.0000000000000599
83. Almaazmi M, Schmid MB, Havers S, Reister F, Lindner W, Mayer B, et al. Cerebral near-infrared spectroscopy during transition of healthy term new-borns. Neonatology. (2013) 103:246–51. doi: 10.1159/000345926
84. Baik N, Urlesberger B, Schwaberger B, Schmölzer GM, Mileder L, Avian A, et al. Reference ranges for cerebral tissue oxygen saturation index in term neonates during immediate neonatal transition after birth. Neonatology. (2015) 108:283–6. doi: 10.1159/000438450
85. Urlesberger B, Kratky E, Rehak T, Pocivalnik M, Avian A, Czihak J, et al. Regional oxygen saturation of the brain during birth transition of term infants: comparison between elective cesarean and vaginal deliveries. J Pediatr. (2011) 159:404–8. doi: 10.1016/j.jpeds.2011.02.030
86. Pichler G, Binder C, Avian A, Beckenbach E, Schmölzer GM, Urlesberger B. Reference ranges for regional cerebral tissue oxygen saturation and fractional oxygen extraction in neonates during immediate transition after birth. J Pediatr. (2013) 163:1558–63. doi: 10.1016/j.jpeds.2013.07.007
87. Bailey SM, Hendricks-Munoz KD, Mally P. Cerebral, renal, and splanchnic tissue oxygen saturation values in healthy term newborns. Am J Perinatol. (2014) 31:339–44. doi: 10.1055/s-0033-1349894
88. McNeill S, Gatenby JC, McElroy S, Engelhardt B. Normal cerebral, renal and abdominal regional oxygen saturations using near-infrared spectroscopy in preterm infants. J Perinatol. (2011) 31:51–7. doi: 10.1038/jp.2010.71
89. Roche-Labarbe N, Carp SA, Surova A, Patel M, Boas DA, Grant PE, et al. Noninvasive optical measures of CBV, StO(2), CBF index, and rCMRO(2) in human premature neonates' brains in the first six weeks of life. Hum Brain Mapp. (2010) 31:341–52. doi: 10.1002/hbm.20868
90. Hyttel-Sorensen S, Pellicer A, Alderliesten T, Austin T, van Bel F, Benders M, et al. Cerebral near infrared spectroscopy oximetry in extremely preterm infants: phase II randomised clinical trial. BMJ. (2015) 350:g7635. doi: 10.1136/bmj.g7635
91. Paulson OB, Strandgaard S, Edvinsson L. Cerebral autoregulation. Cerebrovasc Brain Metab Rev. (1990) 2:161–92.
92. Jobsis FF. Noninvasive, infrared monitoring of cerebral and myocardial oxygen sufficiency and circulatory parameters. Science. (1977) 198:1264–7. doi: 10.1126/science.929199
93. Matcher SJ, Elwell CE, Cooper CE, Cope M, Delpy DT. Performance comparison of several published tissue near-infrared spectroscopy algorithms. Anal Biochem. (1995) 227:54–68. doi: 10.1006/abio.1995.1252
94. Cooper CE, Springett R. Measurement of cytochrome oxidase and mitochondrial energetics by near-infrared spectroscopy. Philos Trans R Soc Lond B Biol Sci. (1997) 352:669–76. doi: 10.1098/rstb.1997.0048
95. Bale G, Elwell CE, Tachtsidis I. From Jobsis to the present day: a review of clinical near-infrared spectroscopy measurements of cerebral cytochrome-c-oxidase. J Biomed Opt. (2016) 21:091307. doi: 10.1117/1.JBO.21.9.091307
96. Wallois F, Patil A, Heberle C, Grebe R. EEG-NIRS in epilepsy in children and neonates. Neurophysiol Clin. (2010) 40:281–92. doi: 10.1016/j.neucli.2010.08.004
97. Glass HC, Glidden D, Jeremy RJ, Barkovich AJ, Ferriero DM, Miller SP. Clinical neonatal seizures are independently associated with outcome in infants at risk for hypoxic-ischemic brain injury. J Pediatr. (2009) 155:318–23. doi: 10.1016/j.jpeds.2009.03.040
98. Durduran T, Choe R, Baker WB, Yodh AG. Diffuse optics for tissue monitoring and tomography. Rep Prog Phys. (2010) 73:076701. doi: 10.1088/0034-4885/73/7/076701
99. Durduran T, Yodh AG. Diffuse correlation spectroscopy for non-invasive, micro-vascular cerebral blood flow measurement. Neuroimage. (2014) 85:51–63. doi: 10.1016/j.neuroimage.2013.06.017
100. Buckley EM, Parthasarathy AB, Grant PE, Yodh AG, Franceschini MA. Diffuse correlation spectroscopy for measurement of cerebral blood flow: future prospects. Neurophotonics. (2014) 1:011009. doi: 10.1117/1.NPh.1.1.011009
101. Diop M, Verdecchia K, Lee T-Y, Lawrence KS. Calibration of diffuse correlation spectroscopy with a time-resolved near-infrared technique to yield absolute cerebral blood flow measurements. Biomed Opt Express. (2011) 2:2068–81. doi: 10.1364/BOE.2.002068
102. Diop M, Kishimoto J, Toronov V, Lee DS, St Lawrence K. Development of a combined broadband near-infrared and diffusion correlation system for monitoring cerebral blood flow and oxidative metabolism in preterm infants. Biomed Opt Express. (2015) 6:3907–18. doi: 10.1364/BOE.6.003907
103. Durduran TK, Kim MN, Buckley EM, Zhou C, Yu G, Choe R, et al. Diffuse optical monitoring of cerebral oxygen metabolism at the bed-side in cerebrovascular disorders. OSA: annual meeting. Front Optics. (2008). doi: 10.1364/FIO.2008.FTuD2
104. Durduran T, Zhou C, Buckley EM, Kim MN, Yu G, Choe R, et al. Optical measurement of cerebral hemodynamics and oxygen metabolism in neonates with congenital heart defects. J Biomed Opt. (2010) 15:037004. doi: 10.1117/1.3425884
105. Roche-Labarbe N, Fenoglio A, Aggarwal A, Dehaes M, Carp SA, Franceschini MA, et al. Near-infrared spectroscopy assessment of cerebral oxygen metabolism in the developing premature brain. J Cereb Blood Flow Metab. (2012) 32:481–8. doi: 10.1038/jcbfm.2011.145
106. Roche-Labarbe N, Fenoglio A, Radhakrishnan H, Kocienski-Filip M, Carp SA, Dubb J, et al. Somatosensory evoked changes in cerebral oxygen consumption measured non-invasively in premature neonates. Neuroimage. (2014) 85:279–86. doi: 10.1016/j.neuroimage.2013.01.035
107. Buckley EM, Lynch JM, Goff DA, Schwab PJ, Baker WB, Durduran T, et al. Early postoperative changes in cerebral oxygen metabolism following neonatal cardiac surgery: effects of surgical duration. J Thorac Cardiovasc Surg. (2013) 145:196–203:5 e1; discussion −5. doi: 10.1016/j.jtcvs.2012.09.057
108. Giovannella M, Contini D, Pagliazzi M, Pifferi A, Spinelli L, Erdmann R, et al. BabyLux device: a diffuse optical system integrating diffuse correlation spectroscopy and time-resolved near-infrared spectroscopy for the neuromonitoring of the premature newborn brain. Neurophotonics. (2019) 6:025007. doi: 10.1117/1.NPh.6.2.025007
109. Lin PY, Roche-Labarbe N, Dehaes M, Carp S, Fenoglio A, Barbieri B, et al. Non-invasive optical measurement of cerebral metabolism and hemodynamics in infants. J Vis Exp. (2013) 2013:e4379. doi: 10.3791/4379
110. Lee CW, Cooper RJ, Austin T. Diffuse optical tomography to investigate the newborn brain. Pediatr Res. (2017) 82:376–86. doi: 10.1038/pr.2017.107
111. Rajaram A, Yip LCM, Milej D, Suwalski M, Kewin M, Lo M, et al. Perfusion and metabolic neuromonitoring during ventricular taps in infants with post-hemorrhagic ventricular dilatation. Brain Sci. (2020) 10:452. doi: 10.3390/brainsci10070452
112. Singh H, Cooper RJ, Wai Lee C, Dempsey L, Edwards A, Brigadoi S, et al. Mapping cortical hemodynamics during neonatal seizures using diffuse optical tomography: a case study. Neuroimage Clin. (2014) 5:256–65. doi: 10.1016/j.nicl.2014.06.012
Keywords: neonatal encephalopathy, Hypoxic ischaemic encephalopathy (HIE), newborn brain injury, neonatal neuromonitoring, NIRS (near infrared spectroscopy), cerebral haemodynamics and oxygenation, cerebral metabolism, cerebral oxygenation
Citation: Harvey-Jones K, Lange F, Tachtsidis I, Robertson NJ and Mitra S (2021) Role of Optical Neuromonitoring in Neonatal Encephalopathy—Current State and Recent Advances. Front. Pediatr. 9:653676. doi: 10.3389/fped.2021.653676
Received: 15 January 2021; Accepted: 15 March 2021;
Published: 09 April 2021.
Edited by:
Eric W. Reynolds, University of Texas Health Science Center at Houston, United StatesReviewed by:
Keith St Lawrence, Western University, CanadaAdre DuPlessis, Children's National Hospital, United States
Copyright © 2021 Harvey-Jones, Lange, Tachtsidis, Robertson and Mitra. This is an open-access article distributed under the terms of the Creative Commons Attribution License (CC BY). The use, distribution or reproduction in other forums is permitted, provided the original author(s) and the copyright owner(s) are credited and that the original publication in this journal is cited, in accordance with accepted academic practice. No use, distribution or reproduction is permitted which does not comply with these terms.
*Correspondence: Subhabrata Mitra, subhabrata.mitra.13@ucl.ac.uk