- Laboratory of Human Lymphohematopoiesis, Imagine Institute, INSERM UMR 1163, Université de Paris, Paris, France
Autosomal dominant gain-of-function mutations in the PIK3CD gene encoding the catalytic subunit p110δ of phosphoinositide 3-kinase-δ (PI3K-δ) or autosomal dominant loss-of-function mutations in the PIK3R1 gene encoding the p85α, p55α and p50α regulatory subunits cause Activated PI3-kinase-δ syndrome (APDS; referred as type 1 APDS and type 2 APDS, respectively). Consequences of these mutations are PI3K-δ hyperactivity. Clinical presentation described for both types of APDS patients is very variable, ranging from mild or asymptomatic features to profound combined immunodeficiency. Massive lymphoproliferation, bronchiectasis, increased susceptibility to bacterial and viral infections and, at a lesser extent, auto-immune manifestations and occurrence of cancer, especially B cell lymphoma, have been described for both types of APDS patients. Here, we review clinical presentation and treatment options as well as fundamental immunological and biological features associated to PI3K-δ increased signaling.
Introduction
Class IA PI3Kinase (PI3K) molecules are composed of a p110 catalytic subunit and a regulatory subunit. The function of class IA PI3Ks is to convert phosphatidylinositol 4,5-bisphosphate into phosphatidylinositol 3,4,5-trisphosphate (PIP3), an important phospholipid secondary messenger. The genes PIK3CA, PIK3CB and PIK3CD encode for the class IA PI3K catalytic subunits p110α, p110β, and p110δ, respectively. P110δ is described to be predominantly expressed in leukocytes. Three genes encode for class1A regulatory subunits. The gene PIK3R1 encodes due to the usage of different first exons the regulatory subunits p85α, p55α and p50α. The genes PIK3R2 and PIK3R3 encode each one regulatory subunit p85β and p55γ, respectively (1). Each of the catalytic subunits can bind to any of the regulatory subunits and responds to extracellular signals. The regulatory unit is required for proper activity of the catalytic unit since it regulates its stability, its cellular localization, and its kinase activity. Activation of the PI3K pathway through several membrane receptors, including T cell receptor/B cell receptor, cytokine receptors and co-stimulatory membrane molecules, lead to phosphorylation of downstream molecules, among them AKT and ribosomal protein S6 (Figure 1).
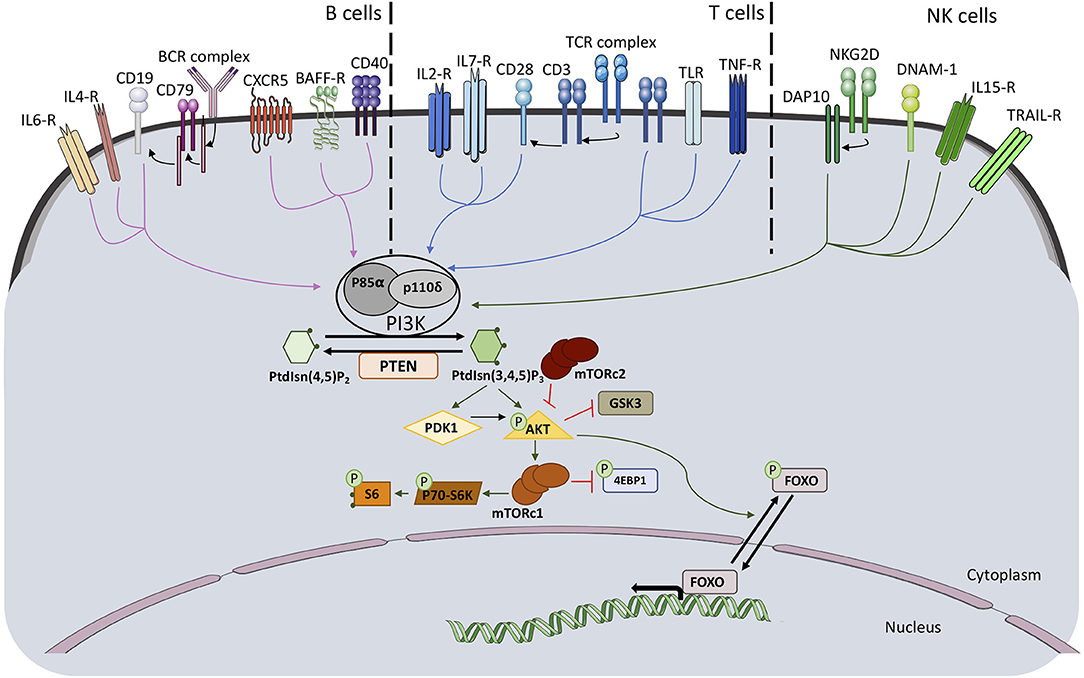
Figure 1. Schematic representation of PI3K pathway activation and downstream signaling molecules in B, T, and NK cells.
Studies of primary immunodeficient (PID) patients demonstrated the requirement of a strict balance in the PI3K pathway for optimal immune responses. On one side, bi-allelic loss-of-function (LOF) mutations in PI3KCD and PIK3R1 leading to absent or decreased p110δ or p85α expression have been reported as responsible for a combined immunodeficiency or an agammaglobulinemia, respectively (2–7). On the other side, hyperactivation of the PI3Kδ pathway leads to a complex immunodeficiency: two independent reports in 2013-2014 described the identification of heterozygous missense mutations in PIK3CD E1021K (8)– which appears as the most frequent – and N334K, E525K (9) using whole exome sequencing (WES). They provided proof that these mutations were autosomal dominant gain-of-function (GOF) and lead to increased PI3Kδ signaling responsible for a lymphoproliferation-associated primary combined immunodeficiency syndrome (Activated PI3-kinase-δ syndrome (APDS; also referred as APDS1); OMIM: # 615513; immunodeficiency 14; IMD14; also referred as p110-delta-activating mutation causing senescent T cells, lymphadenopathy, and immunodeficiency; (PASLI also referred as PASLI-CD) (8, 9). Of note, in 2006, the heterozygous mutation E1021K in the PIK3CD gene had been already identified based on targeted Sanger-sequencing in DNA from a unique patient affected by an humoral immunodeficiency but without any study of the PI3K pathway (10). Following the two landmark papers, numerous case reports completed the phenotypical features and genetic changes. Further APDS1 -causing gene modifications have been described [E81K (11, 12), G124D (11, 12); R405C (13), C416R (14), Y524N (15, 16), Y524S (17), Y524D (18), E525A (19), R929C (20), E1025G (16, 21)]. Moreover, PID patients carrying additional heterozygous missense variations in PIK3CD possibly disease- associated have been reported (R108L) (18, 22), (R512W) (23), (P658L) (18, 24). All these missense mutations are located next to the kinase domain, in the adapter-binding domain, the linker between adapter-binding domain and RAS binding domain, the protein kinase C homology-2 domain, and the helical domain of p110 δ (25). T cell blasts isolated from APDS1 patients exhibited higher PIP3 levels before and after stimulation with antibodies to CD3 and CD28 compared with healthy controls (8). Higher levels of phosphorylated Akt and reduced levels of Foxo1 were also observed (9). Moreover, addition of p110δ inhibitor rescued this phenotype providing a further proof of increased PI3Kδ signaling.
Type 2 APDS (also referred as APDS2 and PASLI-R1); OMIM: # 616005; immunodeficiency 36; IMD36 is caused by autosomal dominant LOF mutations in PIK3R1 (26, 27). The firstly described APDS2 mutation has been identified through WES in DNA of a PID patient presenting with a clinical and immunological phenotype reminiscent to that of APDS1 (26). Although a missense N564K variant has been reported (20), the vast majority of disease causing APDS2 mutations affect the splice donor or splice acceptor sites of exon 11 leading to an alternative splice product in which exon 11 (encoding part of the p110δ interacting domain) is deleted (28–32) (of note, the first non-coding exon was not counted in the initial description (26), thus exon skipping of coding exon 10 (in fact exon11) had been reported). The aberrant splice product enables the expression of a shortened mutant p85α (and p50α and p55α) protein lacking part of the iSH2 domain (Δ434_475) and as further consequence an hyperactivation of p110δ in APDS2 patients' lymphocytes (26, 27). Use of pharmacological PI3K delta inhibitor in lymphocytes from APDS2 patients indicated that especially the regulation of p110δ is disturbed by the mutant protein p85αΔ434_475. Structural studies using hydrogen-deuterium exchange mass spectrometry indicated that the APDS2 mutant protein p85αΔ434_475 disrupts inhibitory interactions of the nSH2, iSH2 and cSH2 domains especially within the p85αΔ434_475/p110δ complex, resulting in substantial basal activation of p110δ in contrast to only minimally activity of p110α within the p85αΔ434_475/p110α complex (33). Thus, although p85α is ubiquitously expressed, its detrimental activity is especially associated to the predominantly leucocyte expressed p110δ subunit, explaining why APDS2 resembles APDS1.
Clinical Phenotype and Infectious Complications
Both APDS1 and APDS2 are inherited in an autosomal dominant pattern. Familial and sporadic cases associated to de novo mutations are documented. Paternal and maternal gonadal mosaicism has been described or suggested for APDS1 explaining puzzling inheritance pattern (35, 36). A recent report described the coexistence of uniparental disomy and the PIK3CD E1021K mutation resulting in cells expressing only the mutant p110δ (37).
Clinical features of both APDS1 and APDS2 are highly variable, even in the same family, and range from profound combined immunodeficiency (associated to lymphoproliferation, severe bacterial and viral infections from childhood) to isolated humoral defects Table 1. Exceptional asymptomatic patients have been reported (34). In several cohort studies, nearly all APDS patients are described as suffering from early-onset, recurrent and severe respiratory infections (38) including sinusitis, nasopharyngitis, tonsillitis, otitis media, mastoiditis, pneumonia and pulmonary empyema (14). The most common respiratory pathogens reported in both types of APDS were Haemophilus influenzae and Pneumococcus pneumoniae. However, infections with less common pathogens as Staphylococcus aureus, Pseudomonas aeruginosa, Moraxella catarrhalis and Klebsiella species were also reported (32, 34). In addition to the predominant respiratory infections, other infections affect APDS patients at lesser frequency: ocular infections, most commonly reported as (chronic) conjunctivitis but also as dacryocystitis and orbital cellulitis, have been diagnosed in several APDS patients of both types (32, 34). Skin abscesses due to Staphylococcus aureus infections have also been described (32, 34). In contrast, invasive bacterial infections are very rare (2 patients) (32). The bacterial infections reported in APDS are those commonly observed in humoral deficiencies (39).
Evidence for an associated T cell and innate immune defect is provided by the frequency of viral or opportunistic infections (40): asymptomatic chronic EBV and CMV viremia (detected by PCR) has been reported for both types of APDS as well as disseminated lymphadenitis associated to CMV infections (32, 34). Persistent, severe or recurrent herpes virus infections are common in both types of APDS (41). Severe infections by Varicella zoster virus, or syncytial respiratory virus as well as molluscum contagiosum and warts, indicating pox virus and papilloma virus infections, respectively, have been reported for both types of APDS (32, 34). Chronic viral hepatitis related either to hepatitis B or C infection was reported in APDS2. Cryptosporidium parvum associated to diarrhea was reported for 2 APDS1 patients (34) whereas Giardia intestinalis was reported for 2 APDS2 patient (29, 32). Single cases of Toxoplasmosis infections were reported for both types of APDS. Episode of chronic mucocutaneous candidiasis were reported for both types of APDS patients (32, 34). Persistent granulomatous skin lesions at BCG vaccination injection sites have also been reported for both types of APDS (32, 34).
Benign lymphoproliferation manifesting as chronic or reactive lymphadenopathy, splenomegaly, hepatomegaly (typically in association) or gut infiltration is one of the whole marks for both types of APDS, reported in 75% of APDS1 and 89% of APDS2 patients (32, 34). Both types of APDS predispose to different types of B cell lymphoma (EBV+ and EBV-), especially classical Hodgkin lymphoma, diffuse large B cell lymphoma and marginal zone B cell lymphoma (8, 14, 25, 32, 42, 43).
Both types of APDS present with autoimmune manifestations, occurring in most cases after the first decade of life (44), predominantly as cytopenias and glomerulonephritis. As reported by the ESID APDS registry, 30% of APDS patients had autoimmune cytopenias, (44) such as hemolytic anemia, Evans syndrome and thrombocytopenic purpura (34). Additionally, autoimmune/inflammatory conditions reported include autoimmune thyroiditis, glomerulonephritis, sclerosing cholangitis, nephrotic syndrome, insulin-dependent diabetes, exocrine pancreatic insufficiency, autoimmune hepatitis, chronic arthritis, Sjogren syndrome, chronic eczema and autoimmune pericarditis (32, 34, 44–46).
Clinical manifestations outside of the immune system include neurodevelopmental delay presenting as mild cognitive impairment or learning disabilities reported for both types of APDS [19% (34) and 31% (32)].
A potential difference between type 1 and type 2 APDS is the notion of growth retardation more commonly associated to APDS2 (26, 32). A few reports relate APDS2 patients associated to a SHORT syndrome (47). SHORT syndrome is a rare genetic congenital disease characterized by short stature, hyperextensibility, ocular depression, Rieger anomaly and teething delay, with no reported immunodeficiency. Up to now, it has been described as linked to heterozygous genetic missense, nonsense and frameshift mutations in the PIK3R1 gene located mostly downstream of exon 11 and associated to decreased PI3K activity (48–50). However, functional and structural studies for the SHORT mutation R649W located within the cSH2 of PIK3R1 indicate that the mutation disrupts binding to phosphorylated YXXM motifs in receptor tyrosine kinases and leads as a consequence to the activation of p110α and p110δ (51). Although these observations provide functional insights for the correlation between PI3K signaling imbalance and growth retardation, the pathophysiological mechanism of APDS2 and clinical features of SHORT syndrome needs to be further elucidated.
Disturbed B Lymphocyte Differentiation and Function in APDS
A study of a cohort of 53 APDS patients revealed variable immunoglobulin levels, with increased IgM levels (79%) and reduced total IgG levels (43%) Table 2. Fifty-eight percent of patients with normal IgG levels had, however, an IgG2 and IgG4 subclass deficiency (20, 34). Reduced IgA levels were common (50%), affecting mostly IgA2. Absent response to vaccination with the polysaccharide S. Pneumoniae vaccine (T -independent response) was reported in several studies (8, 20, 34). In contrast, T-dependent vaccine responses, for example, to Tetanus toxoid were found to be normal in several APDS patients (8, 20). For both types of APDS patients, peripheral blood (PB) immunophenotyping of B lymphocyte subsets indicated an increased frequency of transitional B cells (CD19+Ig(M)D+CD38+CD24+CD27– or CD20+CD10+CD27-), a reduced frequency of naïve B cells (IgM/IgD+CD27–) and of switched memory B cells (IgD–CD27+), contrasting with an increased frequency of plasmablasts [CD38++CD27++ or CD24-CD38++(IgD-CD27++sIgM-cIgM+)] compared to controls (27, 29, 53, 58). Morphological analysis of bone marrow (BM) aspirate smears from APDS1 patients revealed increased presence of immature lymphoid cells (21). Flow cytometric immunophenotyping showed a precursor B cell hyperplasia (based on CD10/CD20/CD19 expression) and impaired maturation of B lymphocytes (21). Further evaluation of the different progenitor B lymphocyte subsets in the BM of APDS1 patients suggested a block of B lymphocyte development starting at the preB-II (CD19+CD34−CD10+CD20dimIgM−) stage (52). Since increase in CD10+ B cell precursors in the BM coincided with increased CD10+ B cells in the peripheral blood of APDS1 patients (21, 52), the increased frequency of circulating immature/transitional B cells likely reflects the impaired BM development. Moreover, the normal BM development observed in an APDS1 patient after an hematopoietic stem cell transplantation (HSCT) suggests a B cell-intrinsic defect (52). The decreased numbers of naive and memory B cell subsets in contrast to increased numbers of plasmablasts in PB indicate further B cell differentiation defects outside the BM (20, 32, 34, 52). Increased frequencies of plasmablasts were also observed in lymph node biopsies from APDS2 patients (32).
Numerous patients have been firstly diagnosed as affected by an Ig class switch recombination (CSR) defect (14, 20, 32, 34, 42). In both types of APDS reduced frequencies of class-switched memory B cells were described (14, 20, 32, 34, 42). In vitro induced Ig CSR analyzed in different studies indicated compromised differentiation of B cells into class switched Ig (but not IgM) secreting plasmablasts. Normal and tendered to be lower expression levels of AICDA and normal B cell proliferation were described in in vitro Ig CSR cultures (9, 52). This partial Ig CSR deficiency was associated to a variable defect in the somatic hypermutation process, described to be within low-normal range on IgG and IgA transcripts (20) and normal on IgM transcripts (8).
In a mice model of Pik3cd GOF, an in vivo and in vitro defective CSR was observed associated to reduced Aicda mRNA expression. The addition of the PI3Kδ inhibitor leniolisib in the in vitro CSR cultures increased Aicda mRNA level and switching toward IgG1 (52). In contrast, normal affinity maturation was described in this model (52).
Analysis of sera from a cohort of APDS1 patients revealed high levels of self-reactive IgM antibodies against diverse self-antigens (59). All the analyzed patients also presented with an increased percentage of VH4-34hi B cells in all subsets, suggesting increased proportions of autoreactive B cells (59). In line, the analysis of a Pik3cd GOF / SWHEL BCR transgenic murine model indicated that activated PI3Kδ-signaling impaired central and peripheral B cell tolerance mechanisms (59). Secondary C1q deficiency possibly due to the consumption of C1q driven by increased apoptotic bodies in combination with elevated IgM level observed in APDS2 patients (60) could further impair peripheral B cell tolerance.
An increased frequency of IL10-producing B lymphocytes (with a transitional B cell phenotype) was reported both in APDS1 patients' PB and in a Pik3cd GOF murine model (61) suggesting that activated PI3Kδ-signaling promotes development of B10 regulatory cells.
T Lymphocyte Dysfunction in APDS
Immunophenotyping of APDS patients revealed CD4+ T cells lymphopenia, with a decrease in naive CD4+ and CD8+ T cells' count and a concomitant increase in effector memory CD8+ T cells' count resulting in normal to high counts of CD8+ T cells and a subsequent inverted CD4/CD8 ratio (8, 9, 32) Table 2.
CD8 T Lymphocytes
Despite the presence of EBV viremia in both types of APDS patients, EBV-specific CD8+ T cells were described in APDS1 patient PB. However, these cells had an effector memory phenotype (CCR7- CD45RA-) and expressed senescence-associated CD57 marker. Activation of EBV-specific CD8+ T cells showed characteristics of enhanced effector function with enhanced expression of IFNγ, Tbet and granzyme B expression compared with healthy donors' cells (9). In both non-specific and EBV-specific CD8+ T cells, expression of exhaustion markers (CD95, CD160, KLRG1, PD-1, 2B4) and senescence marker (CD57) was increased compared with healthy controls. Cytotoxicity of EBV specific CD8+ T cells against autologous EBV transformed B-LCLs was reduced (55). CD8+ effector memory T cells showed increased restimulation-induced cell death (RICD), making them more susceptible to apoptosis (55). CD57 is usually expressed by CD8+ T cells that have shortened telomeres (62). Careful study of the overall population of CD8+ T cells from young APDS patients of both types revealed shortened telomeres in these cells, even when CD57 was not expressed, suggesting an atypical senescent state (63). CD8+ T cells exhaustion and senescence phenotype have been observed in patients exhibiting chronic infection to either HIV, hepatitis B and C (64) or CMV (65) and were proposed to result from constant activation by persistent viral antigen. It is worth noting that PD-1 blockade increased virus-specific CD8+ T cell proliferation and cytokine production further indicating that exhaustion is one of the main features of APDS CD8+ T cells (66).
CD4 T Lymphocytes
Naive CD4+ T cells' counts were strongly reduced in both types of APDS patients as compared to healthy controls while memory CD4+ cells numbers appeared normal or increased. Treg levels were reported as unchanged (57). Among TCM, circulating follicular helper T cells cTFH (CD45RA- CXCR5+) frequency was found to be increased (more than 3 times) in APDS patients' PB (19, 56, 57). However, the differentiation of cTFH cells was reported to be skewed toward a Th1 pattern and away from a Th17 phenotype (57). The cTFH -Th1 cells have been described to be inefficient at promoting B cell differentiation (67). Lymph node biopsies from both types of APDS patients indicated an important hyperplasia of TFH cells (defined by expression of PD1+) present both in extrafollicular areas and germinal centers which appeared therefore disrupted by the PD1+ T cells infiltration (32, 34). Regarding the CD4+T cell compartment, analysis of cytokine production revealed an increased production of Th2 specific cytokines restricted to the memory compartment (57). Except the normal proportion of TH2 bias affecting the whole CD4+ subset, murine models are reminiscent to observations made in patients: Pik3cd GOF mice showed a decreased proportion of naïve CD4+ T cells and an increased proportion in CD4+ memory T cells, especially in TFH cells (57). Adoptive transfer of Pik3cd GOF CD4+T cells in SAP-/- mice resulted in the formation of less germinal centers, suggesting that skewed differentiation toward TFH results in a lower help to B cells and GC formation (57). Interestingly, BM chimeras of WT/Pik3cd GOF mice revealed more profound changes in differentiation states of CD4+ cells in the presence of Pik3cd GOF cells compared with control mice, suggesting that extrinsic signals drive altered differentiation of CD4+ cells (57).
NK Cells
Although numbers of NK cells have been reported as normal or decreased in the first reports, a more careful study performed in APDS1 patients revealed both NK phenotypical and functional abnormalities, which can participate to the peculiar susceptibility of patients to viral infections (54, 68). NK phenotype was found skewed toward an immature profile, with decreased expression of CD16, CD122 and CD127 and increased expression of NKG2A Table 2. Impaired NK cytotoxicity was related to decreased conjugate formation with tumoral or antibody-coated targets, decreased ERK phosphorylation and impaired polarization of the lytic granules. Interestingly, although the NK phenotype was not modified, rapamycin treatment of patients lead to partial restoration of NK cell function and improvement of the cytolytic machinery (54).
Therapeutic Approaches for Both Types of APDS
Treatment of both types of APDS consists mainly in prophylactic measures including long term antibiotics and Ig replacement therapy (32, 34, 69). More precise therapies have been initiated and investigated after the discovery of the genetic defects. Rapamycin (Sirolimus) treatment targeting mammalian target of rapamycin (mTOR), a downstream signaling component of the PI3K δ-signaling and a regulator of cell proliferation, was the first kind of precision therapy reported (9). Beneficial effects of rapamycin treatment were reported on both types on APDS especially by mitigating lymphoproliferation (27, 44). Less beneficial responses were noted for cytopenia and gastrointestinal symptoms (44). Two studies using orally administrated selective PI3K δ inhibitors, Leniolisib (70) and Seletalisib (71) reported reduction in lymphadenopathy and normalization of immune B cell sub-populations (reduction in the frequency of transitional B cells and a normalization of naïve B cell frequencies). Leniolisib was better tolerated in adult APDS1 patients (aged 17–31 years). Seletalisib was reported to have a favorable risk-benefit profile in a younger population (median age of APDS patients treated 15 years), even if two patients discontinued treatment due to increased hepatic enzyme considered to be drug related. Of note, however, PI3Kδ inhibitors harbor the risk to increase genomic instability in B cells by increasing AID expression and consequently mutations in off-Ig target genes, as shown with idelalisib in murine and human B cells (72).
Massive lymphoproliferation associated to life-threatening progressive combined immunodeficiency and autoimmunity are indications for HSCT (73–75). It appears as the only definitive cure for the lymphocyte mediated immune dysregulation in both types of APDS. Two case reports of HSCT patients reported similar survival rates of 9/11 and 7/9 patients, absence of long term severe graft vs. host disease and improvement of clinical manifestations (73, 74). None of the surviving HSCT patients required Ig replacement therapy by day 100 (76). However, the possible risks of transplant (adverse effects or engraftment failure) have to be compared to the benefit of available specific pharmaceutical treatments. These medical treatments could also be essential to allow disease remission and thus better opportunity for less risky HSCT procedure (75).
Conclusion
Studies of PID patients provided valuable insights in the underlying pathophysiological mechanisms of PI3Kδ signaling and demonstrated the requirement of a strict balance in this pathway for optimal immune responses. Delineation of the molecular basis of a lymphoproliferation-associated primary combined immunodeficiency syndrome (APDS) gave evidence that hyperactive p110δ signaling impairs B cell differentiation and maturation, T cell function and homeostasis, and NK development and function. Clinical presentation and immunological abnormalities of both types of APDS are very similar although a large heterogeneity on a patient-to-patient comparison has been noticed indicating that environmental factor(s), including infections with different pathogens, as well as other genetic “modifying” factor(s) likely contribute to the disease presentation. Clinical complications such as recurrent respiratory infections, adenopathy and intestinal problems are together with frequently reported immunological abnormalities (increased IgM serum level associated to increased frequency of transitional/immature B cells and of effector/memory CD8 T cells as well as persistent CMV and/or EBV viremia) first and major diagnostic indications to consider further investigation of the PI3Kδ signaling activation. This is evaluated through the analysis of the phosphorylation status of AKT and ribosomal protein S6 or genetic examination of the APDS related genes: PIK3CD and PIK3R1.
The investigation of underlying molecular mechanisms for clinical manifestations outside of the immune system including neurodevelopmental delay described for both types of APDS and SHORT syndrome-like features particularly noted in APDS2 patients provide interesting research perspectives. Furthermore, a challenge for the future will be the identification of prognostic markers needed to guide treatment decisions. Natural history studies as the ESID-APDS registry in Europe or the Primary Immune Deficiency Treatment Consortium in North America should help to reach this goal.
Author Contributions
RT, NM-C, LP, AD, and SK performed literature search, conceived, prepared, and wrote the mini review manuscript. All authors contributed to the article and approved the submitted version.
Funding
The work was supported by State funding from the Agence Nationale de la Recherche under Investissments d'avenir program (ANR-10-IAHU-01), by ANR-19-CE17-0012-01 (ANR-AID), the Ligue Contre le Cancer–Comité de Paris and by INSERM. SK is a Centre National de la Recherche Scientifique staff researcher.
Conflict of Interest
SK reports grants and payments for service agreements and travel from UCB Pharma and is a designated inventor on published patent application WO2017/198590.
The remaining authors declare that the research was conducted in the absence of any commercial or financial relationships that could be construed as a potential conflict of interest.
References
1. Okkenhaug K. Signaling by the phosphoinositide 3-kinase family in immune cells. Ann Rev Immunol. (2013) 31:675–704. doi: 10.1146/annurev-immunol-032712-095946
2. Sharfe N, Karanxha A, Dadi H, Merico D, Chitayat D, Herbrick J-A, et al. Dual loss of p110δ PI3-kinase and SKAP (KNSTRN) expression leads to combined immunodeficiency and multisystem syndromic features. J Allergy Clin Immunol. (2018) 142:618–29. doi: 10.1016/j.jaci.2017.10.033
3. Sogkas G, Fedchenko M, Dhingra A, Jablonka A, Schmidt RE, Atschekzei F. Primary immunodeficiency disorder caused by phosphoinositide 3-kinase δ deficiency. J Allergy Clin Immunol. (2018) 142:1650–3.e2. doi: 10.1016/j.jaci.2018.06.039
4. Rodriguez R, Fournier B, Cordeiro DJ, Winter S, Izawa K, Martin E, et al. Concomitant PIK3CD and TNFRSF9 deficiencies cause chronic active Epstein-Barr virus infection of T cells. J Exp Med. (2019) 216:2800–18. doi: 10.1084/jem.20190678
5. Swan DJ, Aschenbrenner D, Lamb CA, Chakraborty K, Clark J, Pandey S, et al. Immunodeficiency, autoimmune thrombocytopenia and enterocolitis caused by autosomal recessive deficiency of PIK3CD-encoded phosphoinositide 3-kinase δ. Haematologica. (2019) 104:e483–6. doi: 10.3324/haematol.2018.208397
6. Conley ME, Dobbs AK, Quintana AM, Bosompem A, Wang Y-D, Coustan-Smith E, et al. Agammaglobulinemia and absent B lineage cells in a patient lacking the p85α subunit of PI3K. J Exp Med. (2012) 209:463–70. doi: 10.1084/jem.20112533
7. Tang P, Upton JEM, Barton-Forbes MA, Salvadori MI, Clynick MP, Price AK, et al. Autosomal recessive agammaglobulinemia due to a homozygous mutation in PIK3R1. J Clin Immunol. (2018) 38:88–95. doi: 10.1007/s10875-017-0462-y
8. Angulo I, Vadas O, Garçon F, Banham-Hall E, Plagnol V, Leahy TR, et al. Phosphoinositide 3-kinase δ gene mutation predisposes to respiratory infection and airway damage. Science. (2013) 342:866–71. doi: 10.1126/science.1243292
9. Lucas CL, Kuehn HS, Zhao F, Niemela JE, Deenick EK, Palendira U, et al. Dominant-activating germline mutations in the gene encoding the PI(3)K catalytic subunit p110δ result in T cell senescence and human immunodeficiency. Nat Immunol. (2014) 15:88–97. doi: 10.1038/ni.2771
10. Jou S-T, Chien Y-H, Yang Y-H, Wang T-C, Shyur S-D, Chou C-C, et al. Identification of variations in the human phosphoinositide 3-kinase p110δ gene in children with primary B-cell immunodeficiency of unknown aetiology. Int J Immunogenet. (2006) 33:361–9. doi: 10.1111/j.1744-313X.2006.00627.x
11. Heurtier L, Lamrini H, Chentout L, Deau M-C, Bouafia A, Rosain J, et al. Mutations in the adaptor-binding domain and associated linker region of p110δ cause activated PI3K-δ Syndrome 1 (APDS1). Haematologica. (2017) 102:e278–81. doi: 10.3324/haematol.2017.167601
12. Takeda AJ, Zhang Y, Dornan GL, Siempelkamp BD, Jenkins ML, Matthews HF, et al. Novel PIK3CD mutations affecting N-terminal residues of p110δ cause activated PI3Kδ syndrome (APDS) in humans. J Allergy Clin Immunol. (2017) 140:1152–6. e10. doi: 10.1016/j.jaci.2017.03.026
13. Rae W, Gao Y, Ward D, Mattocks CJ, Eren E, Williams AP. A novel germline gain-of-function variant in PIK3CD. Clin Immunol. (2017) 181:29–31. doi: 10.1016/j.clim.2017.05.020
14. Crank MC, Grossman JK, Moir S, Pittaluga S, Buckner CM, Kardava L, et al. Mutations in PIK3CD can cause hyper IgM syndrome (HIGM) associated with increased cancer susceptibility. J Clin Immunol. (2014) 34:272–6. doi: 10.1007/s10875-014-0012-9
15. Luo Y, Xia Y, Wang W, Li Z, Jin Y, Gong Y, et al. Identification of a novel de novo gain-of-function mutation of PIK3CD in a patient with activated phosphoinositide 3-kinase δ syndrome. Clin Immunol. (2018) 197:60–7. doi: 10.1016/j.clim.2018.08.007
16. Wang Y, Wang W, Liu L, Hou J, Ying W, Hui X, et al. Report of a Chinese cohort with activated phosphoinositide 3-kinase δ syndrome. J Clin Immunol. (2018) 38:854–63. doi: 10.1007/s10875-018-0568-x
17. Thauland TJ, Pellerin L, Ohgami RS, Bacchetta R, Butte MJ. Case study: mechanism for increased follicular helper T cell development in activated PI3K delta syndrome. Front Immunol. (2019) 10:753. doi: 10.3389/fimmu.2019.00753
18. Tessarin G, Rossi S, Baronio M, Gazzurelli L, Colpani M, Benvenuto A, et al. Activated phosphoinositide 3-kinase delta syndrome 1: clinical and immunological data from an Italian cohort of patients. J Clin Med. (2020) 9:3335. doi: 10.3390/jcm9103335
19. Tsujita Y, Mitsui-Sekinaka K, Imai K, Yeh T-W, Mitsuiki N, Asano T, et al. Phosphatase and tensin homolog (PTEN) mutation can cause activated phosphatidylinositol 3-kinase δ syndrome-like immunodeficiency. J Allergy Clin Immunol. (2016) 138:1672–80.e10. doi: 10.1016/j.jaci.2016.03.055
20. Wentink M, Dalm V, Lankester AC, van Schouwenburg PA, Schölvinck L, Kalina T, et al. Genetic defects in PI3Kδ affect B-cell differentiation and maturation leading to hypogammaglobulineamia and recurrent infections. Clin Immunol. (2017) 176:77–86. doi: 10.1016/j.clim.2017.01.004
21. Dulau Florea AE, Braylan RC, Schafernak KT, Williams KW, Daub J, Goyal RK, et al. Abnormal B-cell maturation in the bone marrow of patients with Germline mutations in PIK3CD. J Allergy Clin Immunol. (2017) 139:1032–5.e6. doi: 10.1016/j.jaci.2016.08.028
22. Lougaris V, Baronio M, Castagna A, Tessarin G, Rossi S, Gazzurelli L, et al. Paediatric MAS/HLH caused by a novel monoallelic activating mutation in p110δ. Clin Immunol. (2020) 219:108543. doi: 10.1016/j.clim.2020.108543
23. Kiyota K, Yoshiura K-I, Houbara R, Miyahara H, Korematsu S, Ihara K. Auto-immune disorders in a child with PIK3CD variant and 22q13 deletion. Eur J Med Genet. (2018) 61:631–3. doi: 10.1016/j.ejmg.2018.04.008
24. Lougaris V, Baronio M, Moratto D, Tampella G, Gazzurelli L, Facchetti M, et al. A novel monoallelic gain of function mutation in p110δ causing atypical activated phosphoinositide 3-kinase δ syndrome (APDS-1). Clin Immunol. (2019) 200:31–4. doi: 10.1016/j.clim.2019.01.003
25. Lucas CL, Chandra A, Nejentsev S, Condliffe AM, Okkenhaug K. PI3Kδ and primary immunodeficiencies. Nat Rev Immunol. (2016) 16:702–14. doi: 10.1038/nri.2016.93
26. Deau M-C, Heurtier L, Frange P, Suarez F, Bole-Feysot C, Nitschke P, et al. A human immunodeficiency caused by mutations in the PIK3R1 gene. J Clin Invest. (2014) 124:3923–8. doi: 10.1172/JCI75746
27. Lucas CL, Zhang Y, Venida A, Wang Y, Hughes J, McElwee J, et al. Heterozygous splice mutation in PIK3R1 causes human immunodeficiency with lymphoproliferation due to dominant activation of PI3K. J Exp Med. (2014) 211:2537–47. doi: 10.1084/jem.20141759
28. Lougaris V, Faletra F, Lanzi G, Vozzi D, Marcuzzi A, Valencic E, et al. Altered germinal center reaction and abnormal B cell peripheral maturation in PI3KR1-mutated patients presenting with HIGM-like phenotype. Clin Immunol. (2015) 159:33–6. doi: 10.1016/j.clim.2015.04.014
29. Olbrich P, Lorenz M, Cura Daball P, Lucena JM, Rensing-Ehl A, Sanchez B, et al. Activated PI3Kδ syndrome type 2: two patients, a novel mutation, and review of the literature. Pediatr Allergy Immunol. (2016) 27:640–4. doi: 10.1111/pai.12585
30. Hauck F, Magg T, Krolo A, Bilic I, Hirschmugl T, Laass M, et al. Variant PIK3R1 hypermorphic mutation and clinical phenotypes in a family with short statures, mild immunodeficiency and lymphoma. Klin Padiatr. (2017) 229:113–7. doi: 10.1055/s-0043-104218
31. Abolhassani H, Aghamohammadi A, Fang M, Rezaei N, Jiang C, Liu X, et al. Clinical implications of systematic phenotyping and exome sequencing in patients with primary antibody deficiency. Genet Med. (2019) 21:243–51. doi: 10.1038/s41436-018-0012-x
32. Elkaim E, Neven B, Bruneau J, Mitsui-Sekinaka K, Stanislas A, Heurtier L, et al. Clinical and immunologic phenotype associated with activated phosphoinositide 3-kinase δ syndrome 2: A cohort study. J Allergy Clin Immunol. (2016) 138:210–8. e9. doi: 10.1016/j.jaci.2016.03.022
33. Dornan GL, Siempelkamp BD, Jenkins ML, Vadas O, Lucas CL, Burke JE. Conformational disruption of PI3Kδ regulation by immunodeficiency mutations in PIK3CD and PIK3R1. PNAS. (2017) 114:1982–7. doi: 10.1073/pnas.1617244114
34. Coulter TI, Chandra A, Bacon CM, Babar J, Curtis J, Screaton N, et al. Clinical spectrum and features of activated phosphoinositide 3-kinase δ syndrome: A large patient cohort study. J Allergy Clin Immunol. (2017) 139:597–606.e4. doi: 10.1016/j.jaci.2016.06.021
35. Segundo GRS, Takano OA, Moraes LSL, Nadaf MISV, Fernandes SJ, Ochs HD, et al. Paternal gonadal mosaicism as cause of a puzzling inheritance pattern of activated PI3-kinase delta syndrome. Ann Allergy Asthma Immunol. (2017) 119:564–6. doi: 10.1016/j.anai.2017.09.054
36. Mensa-Vilaró A, García-Morato MB, Calle-Martin O de la, Franco-Jarava C, Martínez-Saavedra MT, González-Granado LI, et al. Unexpected relevant role of gene mosaicism in patients with primary immunodeficiency diseases. J Allergy Clin Immunol. (2019) 143:359–68. doi: 10.1016/j.jaci.2018.09.009
37. Wang Y, Chen X, Yang Q, Tang W, Jia Y, Zhou L, et al. E1021K Homozygous Mutation in PIK3CD Leads to Activated PI3K-Delta Syndrome 1. J Clin Immunol. (2020) 40:378–87. doi: 10.1007/s10875-020-00749-y
38. Condliffe AM, Chandra A. Respiratory manifestations of the activated phosphoinositide 3-kinase delta syndrome. Front Immunol. (2018) 9:338. doi: 10.3389/fimmu.2018.00338
39. Durandy A, Kracker S, Fischer A. Primary antibody deficiencies. Nat Rev Immunol. (2013). 13:519–33. doi: 10.1038/nri3466
40. Chiriaco M, Brigida I, Ariganello P, Di Cesare S, Di Matteo G, Taus F, et al. The case of an APDS patient: Defects in maturation and function and decreased in vitro anti-mycobacterial activity in the myeloid compartment. Clin Immunol. (2017) 178:20–8. doi: 10.1016/j.clim.2015.12.008
41. Cohen JI. Herpesviruses in the Activated Phosphatidylinositol-3-Kinase-δ Syndrome. Front Immunol. (2018) 9:237. doi: 10.3389/fimmu.2018.00237
42. Kracker S, Curtis J, Ibrahim MAA, Sediva A, Salisbury J, Campr V, et al. Occurrence of B-cell lymphomas in patients with Activated Phosphoinositide 3-Kinase δ syndrome. J Allergy Clin Immunol. (2014) 134:233–6. doi: 10.1016/j.jaci.2014.02.020
43. Durandy A, Kracker S. Increased activation of PI3 kinase-δ predisposes to B-cell lymphoma. Blood. (2020) 135:638–43. doi: 10.1182/blood.2019002072
44. Maccari ME, Abolhassani H, Aghamohammadi A, Aiuti A, Aleinikova O, Bangs C, et al. Disease evolution and response to rapamycin in activated phosphoinositide 3-kinase δ syndrome: the european society for immunodeficiencies-activated phosphoinositide 3-kinase δ syndrome registry. Front Immunol. (2018) 9:543. doi: 10.3389/fimmu.2018.00543
45. Narayan N, Hewins P, Lane P, Rhodes B. 47 Activated PI3 kinase delta syndrome: a rare cause of inflammatory disease. Rheumatol Adv Pract. (2018) 2:rky034.010. doi: 10.1093/rap/rky034.010
46. Hartman HN, Niemela J, Hintermeyer MK, Garofalo M, Stoddard J, Verbsky JW, et al. Gain of function mutations of PIK3CD as a cause of primary sclerosing cholangitis. J Clin Immunol. (2015) 35:11–4. doi: 10.1007/s10875-014-0109-1
47. Ramirez L, Tamayo W, Ale H. APDS2 and SHORT syndrome in a teenager with pik3r1 pathogenic variant. J Clin Immunol. (2020) 40:1020–5. doi: 10.1007/s10875-020-00843-1
48. Chudasama KK, Winnay J, Johansson S, Claudi T, König R, Haldorsen I, et al. SHORT syndrome with partial lipodystrophy due to impaired phosphatidylinositol 3 kinase signaling. Am J Hum Genet. (2013) 93:150–7. doi: 10.1016/j.ajhg.2013.05.023
49. Thauvin-Robinet C, Auclair M, Duplomb L, Caron-Debarle M, Avila M, St-Onge J, et al. PIK3R1 mutations cause syndromic insulin resistance with lipoatrophy. Am J Hum Genet. (2013) 93:141–9. doi: 10.1016/j.ajhg.2013.05.019
50. Dyment DA, Smith AC, Alcantara D, Schwartzentruber JA, Basel-Vanagaite L, Curry CJ, et al. Mutations in PIK3R1 cause short syndrome. Am J Hum Genet. (2013) 93:158–66. doi: 10.1016/j.ajhg.2013.06.005
51. Dornan GL, Stariha JTB, Rathinaswamy MK, Powell CJ, Boulanger MJ, Burke JE. Defining how oncogenic and developmental mutations of PIK3R1 alter the regulation of class IA phosphoinositide 3-kinases. Structure. (2020) 28:145–56. e5. doi: 10.1016/j.str.2019.11.013
52. Avery DT, Kane A, Nguyen T, Lau A, Nguyen A, Lenthall H, et al. Germline-activating mutations in PIK3CD compromise B cell development and function. J Exp Med. (2018) 215:2073–95.
53. Martínez-Saavedra MT, García-Gomez S, Domínguez Acosta A, Mendoza Quintana JJ, Páez JP, García-Reino EJ, et al. Gain-of-function mutation in PIK3R1 in a patient with a narrow clinical phenotype of respiratory infections. Clin Immunol. (2016) 173:117–20. doi: 10.1016/j.clim.2016.09.011
54. Ruiz-García R, Vargas-Hernández A, Chinn IK, Angelo LS, Cao TN, Coban-Akdemir Z, et al. Mutations in PI3K110δ cause impaired natural killer cell function partially rescued by rapamycin treatment. J Allergy Clin Immunol. (2018) 142:605–17. e7. doi: 10.1016/j.jaci.2017.11.042
55. Edwards ESJ, Bier J, Cole TS, Wong M, Hsu P, Berglund LJ, et al. Activating PIK3CD mutations impair human cytotoxic lymphocyte differentiation and function and EBV immunity. J Allergy Clin Immunol. (2019) 143:276–91.e6. doi: 10.1016/j.jaci.2018.04.030
56. Preite S, Cannons JL, Radtke AJ, Vujkovic-Cvijin I, Gomez-Rodriguez J, Volpi S, et al. Hyperactivated PI3Kδ promotes self and commensal reactivity at the expense of optimal humoral immunity. Nat Immunol. (2018) 19:986–1000. doi: 10.1038/s41590-018-0182-3
57. Bier J, Rao G, Payne K, Brigden H, French E, Pelham SJ, et al. Activating mutations in PIK3CD disrupt the differentiation and function of human and murine CD4+ T cells. J Allergy Clin Immunol. (2019) 144:236–53. doi: 10.1016/j.jaci.2019.01.033
58. Wallace JG, Zambrano-Rodas P, Córdova-Calderón W, Estrada-Turriate S, Mendoza-Quispe D, Limache Ontiveros Y, et al. Dysregulated actin dynamics in activated PI3Kδ syndrome. Clin Immunol. (2020) 210:108311. doi: 10.1016/j.clim.2019.108311
59. Lau A, Avery DT, Jackson K, Lenthall H, Volpi S, Brigden H, et al. Activated PI3Kδ breaches multiple B cell tolerance checkpoints and causes autoantibody production. J Exp Med. (2020) 217:e20191336. doi: 10.1084/jem.20191336
60. Hong Y, Nanthapisal S, Omoyinmi E, Olbrich P, Neth O, Speckmann C, et al. Secondary C1q Deficiency in Activated PI3Kδ Syndrome Type 2. Front Immunol. (2019) 10:2589. doi: 10.3389/fimmu.2019.02589
61. Stark A-K, Chandra A, Chakraborty K, Alam R, Carbonaro V, Clark J, et al. PI3Kδ hyper-activation promotes development of B cells that exacerbate Streptococcus pneumoniae infection in an antibody-independent manner. Nat Commun. (2018) 9:3174. doi: 10.1038/s41467-018-05674-8
62. Brenchley JM, Karandikar NJ, Betts MR, Ambrozak DR, Hill BJ, Crotty LE, et al. Expression of CD57 defines replicative senescence and antigen-induced apoptotic death of CD8+ T cells. Blood. (2003) 101:2711–20. doi: 10.1182/blood-2002-07-2103
63. Cura Daball P, Ventura Ferreira MS, Ammann S, Klemann C, Lorenz MR, Warthorst U, et al. CD57 identifies T cells with functional senescence before terminal differentiation and relative telomere shortening in patients with activated PI3 kinase delta syndrome. Immunol Cell Biol. (2018) 96:1060–71. doi: 10.1111/imcb.12169
64. Wherry EJ, Kurachi M. Molecular and cellular insights into T cell exhaustion. Nat Rev Immunol. (2015) 15:486–99. doi: 10.1038/nri3862
65. Tu W, Rao S. Mechanisms underlying T cell immunosenescence: aging and cytomegalovirus infection. Front Microbiol. (2016) 7:2111. doi: 10.3389/fmicb.2016.02111
66. Wentink MWJ, Mueller YM, Dalm VASH, Driessen GJ, van Hagen PM, van Montfrans JM, et al. Exhaustion of the CD8+ T cell compartment in patients with mutations in phosphoinositide 3-kinase delta. Front Immunol. (2018) 9:446. doi: 10.3389/fimmu.2018.00446
67. Ma CS, Wong N, Rao G, Avery DT, Torpy J, Hambridge T, et al. Monogenic mutations differentially affect the quantity and quality of T follicular helper cells in patients with human primary immunodeficiencies. J Allergy Clin Immunol. (2015) 136:993–1006.e1. doi: 10.1016/j.jaci.2015.05.036
68. Mace EM. Phosphoinositide-3-kinase signaling in human natural killer cells: new insights from primary immunodeficiency. Front Immunol. (2018) 9:445. doi: 10.3389/fimmu.2018.00445
69. Coulter TI, Cant AJ. The treatment of activated PI3Kδ syndrome. Front Immunol. (2018) 9:2043. doi: 10.3389/fimmu.2018.02043
70. Rao VK, Webster S, Dalm VASH, Šedivá A, van Hagen PM, Holland S, et al. Effective “activated PI3Kδ syndrome”–targeted therapy with the PI3Kδ inhibitor leniolisib. Blood. (2017) 130:2307–16. doi: 10.1182/blood-2017-08-801191
71. Diaz N, Juarez M, Cancrini C, Heeg M, Soler-Palacín P, Payne A, et al. Seletalisib for Activated PI3Kδ syndromes: open-label phase 1b and extension studies. J Immunol. (2020) 205:2979–87. doi: 10.4049/jimmunol.2000326
72. Compagno M, Wang Q, Pighi C, Cheong T-C, Meng F-L, Poggio T, et al. Phosphatidylinositol 3-kinase δ blockade increases genomic instability in B cells. Nature. (2017) 542:489–93. doi: 10.1038/nature21406
73. Nademi Z, Slatter MA, Dvorak CC, Neven B, Fischer A, Suarez F, et al. Hematopoietic stem cell transplant in patients with activated PI3K delta syndrome. J Allergy Clin Immunol. (2017) 139:1046–9. doi: 10.1016/j.jaci.2016.09.040
74. Okano T, Imai K, Tsujita Y, Mitsuiki N, Yoshida K, Kamae C, et al. Hematopoietic stem cell transplantation for progressive combined immunodeficiency and lymphoproliferation in patients with activated phosphatidylinositol-3-OH kinase δ syndrome type 1. J Allergy Clin Immunol. (2019) 143:266–75. doi: 10.1016/j.jaci.2018.04.032
75. Chan AY, Leiding JW, Liu X, Logan BR, Burroughs LM, Allenspach EJ, et al. Hematopoietic cell transplantation in patients with primary immune regulatory disorders (PIRD): a primary immune deficiency treatment consortium (PIDTC) survey. Front Immunol. (2020) 11:239. doi: 10.3389/fimmu.2020.00239
Keywords: PI3K signaling, PIK3CD, PIK3R1, primary immunodeficiency, lymphoproliferation
Citation: Thouenon R, Moreno-Corona N, Poggi L, Durandy A and Kracker S (2021) Activated PI3Kinase Delta Syndrome—A Multifaceted Disease. Front. Pediatr. 9:652405. doi: 10.3389/fped.2021.652405
Received: 12 January 2021; Accepted: 24 May 2021;
Published: 25 June 2021.
Edited by:
Luis Ignacio Gonzalez-Granado, University Hospital October 12, SpainReviewed by:
Eleonora Gambineri, University of Florence, ItalyLisa Renee Forbes, Baylor College of Medicine, United States
Copyright © 2021 Thouenon, Moreno-Corona, Poggi, Durandy and Kracker. This is an open-access article distributed under the terms of the Creative Commons Attribution License (CC BY). The use, distribution or reproduction in other forums is permitted, provided the original author(s) and the copyright owner(s) are credited and that the original publication in this journal is cited, in accordance with accepted academic practice. No use, distribution or reproduction is permitted which does not comply with these terms.
*Correspondence: Sven Kracker, c3Zlbi5rcmFja2VyQGluc2VybS5mcg==
†These authors have contributed equally to this work