- 1Department of Pediatrics, West China Second University Hospital, Sichuan University, Chengdu, China
- 2Key Laboratory of Birth Defects and Related Diseases of Women and Children (Sichuan University), Ministry of Education, West China Second University Hospital, Sichuan University, Chengdu, China
Objective: There are mutual influences between intestine and lung, that propose a concept of the gut-lung axis, but the mechanism is still unclear. Microbial colonization in early life plays an important role in regulating intestinal and lung function. In order to explore the characteristics of early microbiota on the gut-lung axis, we studied the correlation between intestinal and pharyngeal microbiota on day 1 and day 28 after birth in premature neonates.
Methods: Thirteen neonates born at 26–32 weeks gestational age (GA) hospitalized at the neonatal intensive care unit (NICU) of the West China Second Hospital of Sichuan University were enrolled in this study. Stool samples and pharyngeal swabs samples were collected from each neonate on the first day (T1) and the 28th day (T28) after birth. Total bacterial DNA was extracted and sequenced using the Illumina MiSeq Sequencing System based on the V3–V4 hyper-variable regions of the 16S rRNA gene. Based on the sequencing results, the composition of the intestinal and pharyngeal microbiota was compared and analyzed.
Results: At T1, the difference in microbial composition between intestine and pharynx was not statistically significant. The intestinal microbiota was mainly composed of Unidentified Enterobacteriaceae, Ralstonia, Streptococcus, Fusobacterium, Ureaplasma, etc. The pharyngeal microbiota was mainly composed of Ureaplasma, Bacteroides, Fusobacterium, etc. Ureaplasma and Fusobacterium were detected in both intestine and pharynx. At T28, there was a significant difference in microbial composition between intestine and pharynx (p < 0.001). The intestinal microbiota was mainly composed of Unidentified Clostridiales, Klebsiella, Unidentified Enterobacteriaceae, Enterobacter, Streptococcus, etc. Pharyngeal microbiota was mainly composed of Streptococcus, Rothia, etc. Streptococcus was detected in both intestine and pharynx.
Conclusions: The intestine and pharynx of premature neonates have a unique microbial composition, and share some common microbiota. Whether these microbiotas play a role in the mechanism of gut-lung crosstalk needs further study.
Introduction
Intestinal microbiota plays an important role in human health and disease (1). In recent years, numerous studies have shown that infant intestinal microbiota affects the growth and development of children (2, 3), and are associated with neonatal sepsis, neonatal necrotizing enterocolitis (4), childhood obesity (5), asthma, eczema (6), and diabetes (7), hypertension (8), and other diseases in adulthood. It is commonly believed that the uterus is sterile and the colonization of microbiota began after birth. But recent research results suggested that the intestinal microbiota could colonize in neonates before delivery. The concept of sterile uterus has been challenged, but the results are still controversial and worth further study (9, 10). The initial colonization of microbiota in the lung may affect the development of the respiratory system and even lead to disease (11, 12). Chronic respiratory diseases can affect the composition of the intestinal microbiota, and in turn, intestinal microbiota may affected the function of the respiratory system (13, 14). These results suggest that there is a crosstalk between the respiratory system and the intestine, which proposes a concept of the gut-lung axis (15). There are many studies on the gut-lung axis, but the mechanism is still unclear (16). Therefore, it is very crucial to study the microbial characteristics of the intestine and lung for further exploring the mechanism of the gut-lung axis.
The pharynx connects the oral cavity, nasal cavity, lower respiratory tract, and digestive tract. It communicates with the outside world and is exposed to a variety of exogenous and endogenous microbes. Thus, the pharynx is an ecological niche for potentially pathogenic microbe, which may cause local inflammation or lead to lung diseases (17). Previous studies have shown that there was a large amount of overlap between the pharyngeal microbiota and the respiratory microbiota (18), so the pharyngeal microbiota could, to some extent, reflect the characteristics of the respiratory microbiota. Besides, the pharyngeal swab is a fairly accurate method to define the composition of the respiratory microbiota. Therefore, studying the composition and characteristic of pharyngeal microbiota may open a window for exploring respiratory microbiota.
The concept of the gut-lung axis was born out of the observation that different lung diseases can be influenced by intestinal microenvironment changes and vice versa. The microbiota is an important factor responsible for interactions between these two sites in asthma (19). In addition, the intestinal microbiota of patients with severe bacterial pneumonia (20), cystic fibrosis (19), and influenza differs from that of healthy controls (21). Many studies show that early life is the most important period during which microbiota dysbiosis in the intestine may lead to the development of many respiratory diseases, as the intestine microbiota has a significant influence on immune cell maturation and resistance to pathogens (22).
In this study, we took premature neonates with GA <32 weeks as study subjects to analyze intestinal and pharyngeal microbial characteristics and influencing factors. Meanwhile, we studied the correlation between intestinal and pharyngeal microbiota, to provide some evidence for the role of early microbiota in the mechanism of the gut-lung axis.
Patients and Methods
Study Participants
Premature neonates who were delivered and hospitalized in the NICU of West China Second Hospital of Sichuan University from December 2019 to May 2020 were enrolled. Selection criteria were as follows: GA < 32 weeks; single birth; the birth weight of the infant appropriate for gestational age (between the 10–90th percentile of the average weight of the infant of the same gestational age) (23, 24). Exclusion criteria were as follows: infants with congenital malformations; intrauterine growth retardation; neonatal hypoxic-ischemic encephalopathy; immunodeficiency or severe infectious diseases; mother's antibiotic therapy for more than 3 days within 2 weeks before delivery; the neonate using antibiotics after birth more than 3 days within 2 weeks before delivery; the mother or the neonate used probiotics or prebiotics during perinatal period; and the guardian does not agree to participate or withdraws from the study. The following information was obtained from the medical records: duration of ruptured membranes; prenatal antibiotic use; delivery mode; GA; birth weight; gender. The study protocol was approved by the medical ethics committee of the West China Second Hospital of Sichuan University, and written informed consents were obtained from the parents or guardians of the neonates.
Sample Collection Method
Stool samples were collected from baby diapers with sterile test tubes at T1 and T28. Pharyngeal secretions samples were collected with sterile pharyngeal swabs within 30 min after birth and T28. Stool and pharyngeal swabs samples were quickly placed in a −20°C refrigerator after being collected, and stored in a −80°C refrigerator within 24 h until further processing.
DNA Extraction and Amplification
Total genome DNA from samples was extracted using Hexadecyltrimethy Ammonium Bromide (CTAB) method. DNA concentration and purity were detected by a spectrophotometer. According to the concentration, DNA was diluted to 1 ng/μl using sterile water. The bacteria genomic DNA was amplified with the 341F and 806R primers specific for the V3–V4 hypervariable regions of the 16S rDNA gene. All DNAs were amplified by following a protocol described previously (25). Samples were sequenced on an Illumina MiSeq platform according to the manufacturer's recommendations. The sequencing service was provided by Beijing Novogene Genomics Technology Co. Ltd (China).
Data Processing
Paired-end reads from the original DNA fragments are merged by using FLASH (26), which is designed to merge paired-end reads when there are overlaps between reads 1 and reads 2. Paired-end reads were assigned to each sample according to the unique barcodes. Sequences were analyzed using QIIME software package (Quantitative Insights Into Microbial Ecology), and in-house Perl scripts were used to analyze alpha (within samples) and beta (among samples) diversity. First, reads were filtered by QIIME quality filters. Then we use pick_de_novo_otus. py to pick operational taxonomic units (OTUs) by making an OTU table. Sequences with ≥97% similarity were assigned to the same OTUs. We pick representative sequences for each OTU and use the RDP classifier (27) to annotate taxonomic information for each representative sequence. In order to compute Alpha Diversity, we rarify the OTU table and calculate the Shannon index and Chao1 estimator. QIIME calculates weighted unifrac, which are phylogenetic measures of beta diversity. We used weighted uniFrac for Principal Coordinate Analysis (PCoA). LefSe tool was implemented to identify differentially abundant taxa.
Statistical Analysis
Continuous variables were reported as means ± standard deviations, and categorical data were presented as ratios or percentages. Non-parametric Wilcoxon rank-sum test was used to study differences in continuous variables and Fisher's exact tests were used to analyze categorical variables. p < 0.05 was considered statistically significant.
Results
Clinical Characteristics, and Influencing Factors of Microbial Colonization
In this study, 22 infants with GA <32 weeks in the NICU of West China Second Hospital of Sichuan University were included. Based on the exclusion criteria, 9 cases were excluded, and the remaining 13 cases were included in the final analysis (Table 1). At T1, 13 meconium samples were collected and included in the T1s group, and 13 pharyngeal swabs samples were collected and included in the T1y group. At T28, 13 stool samples were collected and included in the T28s group, and 13 pharyngeal swabs samples were collected and included in the T28y group. In total, 52 samples were collected from the enrolled neonates, 16S rRNA was amplified from 34 samples (6 samples in T1s group; 4 samples in T1y group; 13 samples in T28s group; 11 samples in T28y group; Table 2), and 2,944,688 reads were obtained. At T1, maternal antibiotics, GA, delivery mode, and gender had some effect on the microbial colonization of meconium and pharyngeal swabs, but these were not significant (Table 3).
Comparisons Between Intestinal and Pharyngeal Microbiota
At T1, 1,340 OTUs were detected in the intestine, and 2,404 OTUs were detected in the pharynx, among which 179 OTUs were commonly shared. At T28, 536 OTUs were detected in the intestine, and 1,377 OTUs were detected in the pharynx, among which, 120 OTUs were commonly shared (Figure 1A). At T1, there was no significant difference in Shannon index and Chao1 index between the T1s group and the T1y group. At T28 there was no significant difference in Shannon index between the T28s group and the T28y group; the Chao1 index of the T28y group was higher than that of the T28s group, the difference was significant (p < 0.05; Figure 1B).
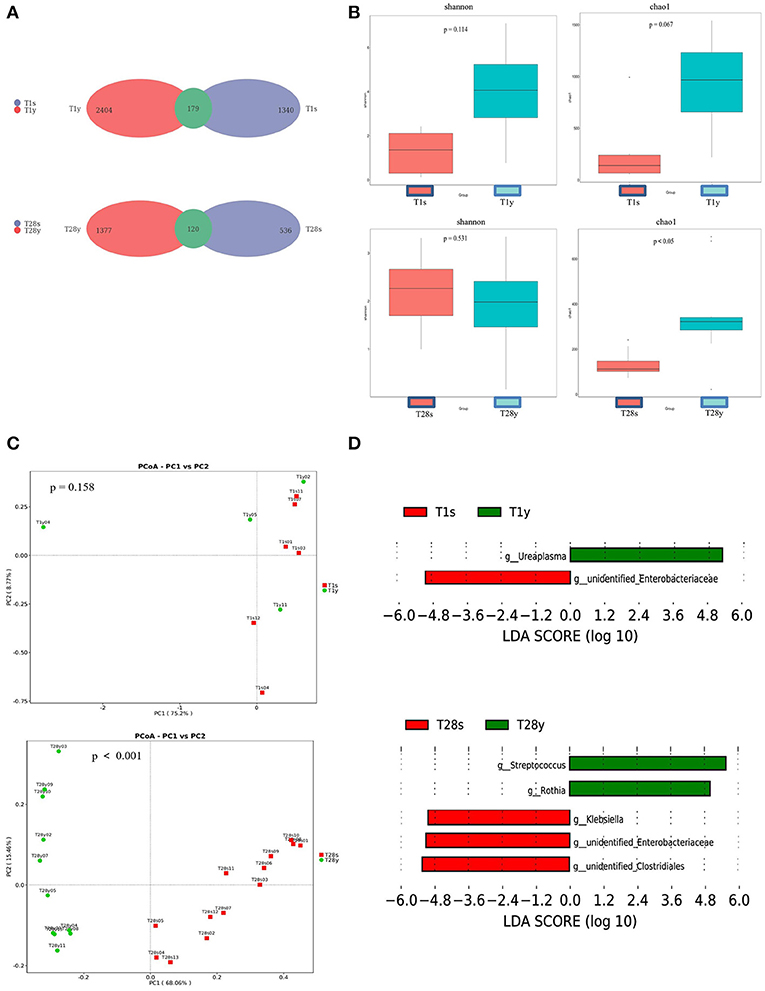
Figure 1. Comparisons between intestinal and pharyngeal microbiota. (A) OTUs were shared by the intestine and pharynx. (B) Comparison of the microbial biodiversity between intestine and pharynx, the shannon index, and chao1 were shown as estimators. (C) PCoA plot based on OTU abundance. Each point represents the intestinal or pharyngeal microbiota of a subject. (D) Histogram of the LDA scored for differentially abundant genera between intestine and pharynx. LDA scores were calculated by LDA effect size using linear discriminant analysis.
In order to compare the composition of the overall microbiota of the intestine and pharynx, PCoA was implemented based on the OTU level. At T1, the results of PCoA showed no difference in microbiota composition between the T1s group and the T1y group. At T28, the results of PCoA showed a significant difference in microbiota composition between the T28s group and the T28y group (p < 0.001; Figure 1C). Next, the LEfSe tool (28) was used to analyze microbiota in stool samples and pharyngeal swabs samples, and to detect potential significant differences in relative abundances between the intestinal and pharyngeal microbiota. There were significant differences in 2 genera between the T1s group and the T1y group. Ureaplasma was relatively more abundant in the T1y group, whereas Unidentified Enterobacteriaceae was relatively more abundant in the T1s group. There were significant differences in some genera between the T28s group and the T28y group. Streptococcus, Rothia were relatively more abundant in the T28y group, whereas Unidentified Clostridiales, Unidentified Enterobacteriaceae, Klebsiella were relatively more abundant in the T28s group (Figure 1D).
The Microbial Composition of the Intestine and Pharynx at the Genus Level
We analyzed the microbial composition of the intestine and pharynx at the genus level and found that all samples were dominated by a specific genus (Figure 2A). At T1, intestinal microbiota was mainly composed of Unidentified Enterobacteriaceae (18.8%), Ralstonia (16.7%), Streptococcus (16.6%), Fusobacterium (14.2%), Ureaplasma (11.9%), etc. Pharyngeal microbiota was mainly composed of Ureaplasma (38.1%), Bacteroides (14.9%), Fusobacterium (1.8%), etc. At T28, intestinal microbiota was mainly composed of Unidentified Clostridiales (30.0%), Klebsiella (19.6%), Unidentified Enterobacteriaceae (18.8%), Enterobacter (7.0%), Streptococcus (2.0%), etc. Pharyngeal microbiota was mainly composed of Streptococcus (70.7%), Rothia (17.6%), etc. At T1, the relative abundance of Ureaplasma between the T1s group and the T1y group was 11.9 vs. 38.1% (p = 0.053); The relative abundance of Unidentified Enterobacteriaceae between the T1s group and the T1y group was 18.4 vs. 0.08% (p < 0.05). At T28, the relative abundance of Streptococcus between the T28s group and the T28y group was 2.0 vs. 70.7% (p < 0.05); the relative abundance of Rothia between the T28s group and the T28y group was 0.1 vs. 17.6% (p < 0.05); the relative abundance of Unidentified Clostridiales between the T28s group and the T28y group was 30.0 vs. 0.002% (p < 0.05); the relative abundance of Unidentified Enterobacteriaceae between the T28s group and the T28y group was 18.8 vs. 0.02% (p < 0.05); the relative abundance of Klebsiella between the T28s group and the T28y group was 19.6 vs. 0.5% (p < 0.05; Figure 2B).
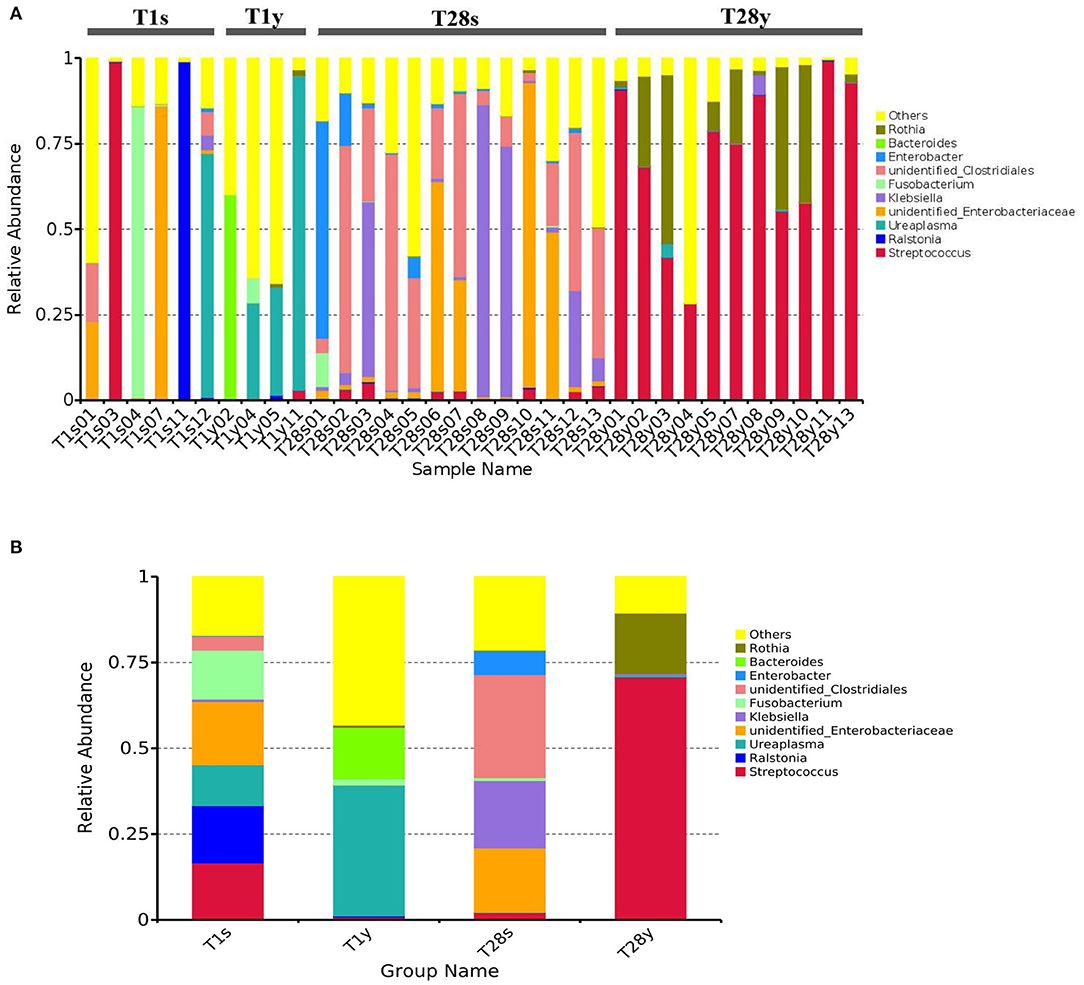
Figure 2. The composition of intestinal and pharyngeal microbiota.at the genus level. (A) The microbial composition of each sample. (B) Average microbiota of T1s, T1y, T28s, and T28y (T1s: meconium samples at T1; T1y: pharyngeal swabs samples at T1; T28s: stool samples at T28; T28y: pharyngeal swabs samples at T28).
Discussion
Microbial colonization is a complex and dynamic process. In this study, premature neonates with GA <32 weeks were selected as study subjects. Microbiota was detected in 6 meconium samples and 4 pharyngeal swabs samples at birth, which seems to be a challenge to the concept of a sterile uterus, but it needs to be determined by a larger sample size and the exclusion of more interfering factors. Maternal antibiotics, GA, delivery mode, and gender had no significant effect on the microbial detection rate of meconium and pharyngeal swabs, this is inconsistent with the results of some previous studies that the delivery mode affects the microbiota colonization of the neonates (29). The possible reason is that our sample collection was carried out immediately after birth, and the colonization of environmental microbiota has not been completed.
Through Venn diagram analysis, at T1, intestine and pharynx shared more OTUs than at T2. We may speculate that this pattern of colonization can be driven by the developing host immunity (30), or, alternatively, one pioneer group of bacteria (31) might metabolically prime the environment for replacement by a particular successor. Our observations support the hypothesis that temporal factors influence the microbial colonization of preterm infants, such as there was no significant difference in Shannon index between the T28s group and the T28y group. The Chao1 index of the T28y group was higher than that of the T28s group, and the difference was significant. The results show that the pharyngeal microbiota diversity was higher than that of the intestinal and that the low abundance and rare species are present in the community at T28y group. Through PCoA analysis, there was no significant difference in microbial composition between the T1s group and the T1y group. At T28, there was a significant difference of microbial composition between the T28s group and the T28y group. Streptococcus, Rothia were relatively more abundant in the T28y group, whereas Unidentified Clostridiales, Klebsiella, Unidentified Enterobacteriaceae, Enterobacter were relatively more abundant in the T28s group.
The analysis of microbial composition show that, at T1, the intestine was dominated by Unidentified Enterobacteriaceae, Ralstonia, Streptococcus, etc. among which Unidentified Enterobacteriaceae and Streptococcus are potential pathogenic microbes, and the rest are mostly pathogenic microbes. It suggests that premature neonates have very few beneficial microbes in the intestine, but a large number of potentially pathogenic microbes and pathogenic microbes, which is consistent with previous studies (32). At T1, pharyngeal microbiota was mainly composed of Ureaplasma, Bacteroides, Fusobacterium, etc, which are similar to the oropharyngeal microbiota of infants (33, 34). Ureaplasma was detected in both intestine and pharynx, the relative abundance has no significant difference between the T1s group and the T1y group. This may indicate that the origin of intestinal and pharyngeal microbiota may be the same at birth. The relative abundance and composition of the intestinal and pharyngeal microbiota was significantly different on the 28th day after birth. Streptococcus mainly existed in the pharynx, while Unidentified Clostridiales mainly existed in the intestine. We analyzed the characteristics of intestinal microbiota at different time, the results show that the intestinal microbiota colonization with beneficial bacteria is delayed in preterm infants, while the number of potentially pathogenic bacteria is high. Ureaplasma was relatively more abundant in the T1y group, Streptococcus was relatively more abundant in the T28y group. There was significant difference between early and late pharyngeal microbiota. These preliminary and explorative results suggested that the neonatal microbial colonization is a highly dynamic process, which is sensitive to environmental factors.
However, several potential limitations should be taken into consideration. Firstly the sample amount might not be adequate. Secondly, if the characteristics of neonatal microbiota and maternal microbiota were studied together, it is more meaningful to know the initial source of microbial colonization.
In conclusion, the study's results suggest that, in some neonates, the microbiota may exist in both the intestine and the pharynx at birth. There was no significant difference between intestinal and pharyngeal microbiota composition on day 1, but on day 28, site-specific microbial was already established. Despite the initial similarity of pharyngeal and gut microbiotas, the specific bacterial genera were determined in both sites. Further we hypothesize that these genera may contribute to the functioning of the gut-lung axis.
Data Availability Statement
The original contributions presented in the study are included in the article/supplementary material, further inquiries can be directed to the corresponding author/s. https://figshare.com/s/81253de749d49bcb0898.
Ethics Statement
The studies involving human participants were reviewed and approved by Ethics Committee of West China Second University Hospital, Sichuan University. Written informed consent to participate in this study was provided by the participants' legal guardian/next of kin.
Author Contributions
SY: data collection, management and analysis, and manuscript drafting and revision. LQ and JS: data collection and manuscript revision. LX: site oversight and manuscript revision. YX and HL: study concept and design, interpretation of results, manuscript revision, and final approval. All authors contributed to the article and approved the submitted version.
Funding
This work was supported by the National Key R&D Program of China (2017YFC0211705), Key R&D Program of Science and Technology Department of Sichuan Province (19ZDYF1169), and Sichuan Science and Technology Program (2019YFH0143).
Conflict of Interest
The authors declare that the research was conducted in the absence of any commercial or financial relationships that could be construed as a potential conflict of interest.
References
1. Munyaka PM, Khafipour E, Ghia JE. External influence of early childhood establishment of gut microbiota and subsequent health implications. Front Pediatr. (2014) 2:109. doi: 10.3389/fped.2014.00109
2. Robertson RC, Manges AR, Finlay BB, Prendergast AJ. The human microbiome and child growth-first 1000 days and beyond. Trends Microbiol. (2019) 27:131–47. doi: 10.1016/j.tim.2018.09.008
3. Li H, He Z, Gao D, Lv y, Zhou Q, Xiao B, et al. Characteristics of the intestinal microbiota in very low birth weight infants with extrauterine growth restriction. Front Pediatr. (2019) 7:99. doi: 10.3389/fped.2019.00099
4. Masi AC, Stewart CJ. The role of the preterm intestinal microbiome in sepsis and necrotising enterocolitis. Early Hum Dev. (2019) 138:104854. doi: 10.1016/j.earlhumdev.2019.104854
5. Subramanian S, Blanton LV, Frese SA, Charbonneau M, Mills DA, Gordon JI. Cultivating healthy growth and nutrition through the gut microbiota. Cell. (2015) 161:36–48. doi: 10.1016/j.cell.2015.03.013
6. Zimmermann P, Messina N, Mohn WW, Finlay BB, Curtis N. Association between the intestinal microbiota and allergic sensitization, eczema, and asthma: a systematic review. J Allergy Clin Immunol. (2019) 143:467–85. doi: 10.1016/j.jaci.2018.09.025
7. Qin J, Li Y, Cai Z, Li S, Zhu J, Zhang F, et al. A metagenome-wide association study of gut microbiota in type 2 diabetes. Nature. (2012) 490:55–60. doi: 10.1038/nature11450
8. Li J, Zhao F, Wang Y, Chen J, Tao J, Tian G, et al. Gut microbiota dysbiosis contributes to the development of hypertension. Microbiome. (2017) 5:14. doi: 10.1186/s40168-016-0222-x
9. Willyard C. Could baby's first bacteria take root before birth? Nature. (2018) 553:264–6. doi: 10.1038/d41586-018-00664-8
10. de Goffau MC, Lager S, Sovio U, Gaccioli F, Cook E, Peacock SJ, et al. Human placenta has no microbiome but can contain potential pathogens. Nature. (2019) 572:329–34. doi: 10.1038/s41586-019-1451-5
11. Gentle SJ, Lal CV. Predicting BPD: lessons learned from the airway microbiome of preterm infants. Front Pediatr. (2020) 7:564. doi: 10.3389/fped.2019.00564
12. Teo SM, Tang HHF, Mok D, Judd LM, Watts SC, Pham K, et al. Airway microbiota dynamics uncover a critical window for interplay of pathogenic bacteria and allergy in childhood respiratory disease. Cell Host Microbe. (2018) 24:341–52.e5. doi: 10.1016/j.chom.2018.08.005
13. Chakradhar S. A curious connection: teasing apart the link between gut microbes and lung disease. Nat Med. (2017) 23:402–4. doi: 10.1038/nm0417-402
14. Mjösberg J, Rao A. Lung inflammation originating in the gut. Science. (2018) 359:36–7. doi: 10.1126/science.aar4301
15. Ranucci G, Buccigrossi V, de Freitas MB, Guarino A, Giannattasio A. Early-life intestine microbiota and lung health in children. J Immunol Res. (2017) 2017:8450496. doi: 10.1155/2017/8450496
16. He Y, Wen Q, Yao F, Xu D, Huang Y, Wang J. Gut-lung axis: the microbial contributions and clinical implications. Crit Rev Microbiol. (2017) 43:81–95. doi: 10.1080/1040841X.2016.1176988
17. Surette MG, Bowdish DME. Loss of microbial topography precedes infection in infants. Am J Respir Crit Care Med. (2019) 200:660–2. doi: 10.1164/rccm.201903-0687ED
18. Man WH, van Houten MA, Mérelle ME, Vlieger AM, Chu MLJN, Jansen NJG, et al. Bacterial and viral respiratory tract microbiota and host characteristics in children with lower respiratory tract infections: a matched case-control study. Lancet Respir Med. (2019) 7:417–26. doi: 10.1016/S2213-2600(18)30449-1
19. Marsland BJ, Trompette A, Gollwitzer ES. The gut-lung axis in respiratory disease. Ann Am Thorac Soc. (2015) 12(Suppl. 2):S150–6.
20. Chung KF. Airway microbial dysbiosis in asthmatic patients: a target for prevention and treatment? J Allergy Clin Immunol. (2017) 139:1071–81. doi: 10.1016/j.jaci.2017.02.004
21. Qin N, Zheng B, Yao J, Guo L, Zuo J, Wu L, et al. Influence of H7N9 virus infection and associated treatment on human gut microbiota. Sci Rep. (2015) 5:14771. doi: 10.1038/srep14771
22. Sokolowska M, Frei R, Lunjani N, Akdis CA, O'Mahony L. Microbiome and asthma. Asthma Res Pract. (2018) 4:1. doi: 10.1186/s40733-017-0037-y
23. Hendrix MLE, van Kuijk SMJ, El Bahaey SE, Gerver WJM, Feron FJM, Kuin ME, et al. ‘Postnatal growth during the first five years of life in SGA and AGA neonates with reduced fetal growth'. Early Hum Dev. (2020) 151:105199. doi: 10.1016/j.earlhumdev.2020.105199
24. Gurugubelli KR, Ballambattu VB, Bobby Z. Global DNA methylation in cord blood and neurodevelopmental outcome at 18 months of age among intrauterine growth restricted and appropriate for gestational age infants. J Trop Pediatr. (2020) fmaa108. doi: 10.1093/tropej/fmaa108
25. Caporaso JG, Lauber CL, Walters WA, Berg-Lyons D, Lozupone CA, Turnbaugh PJ, et al. Global patterns of 16S rRNA diversity at a depth of millions of sequences per sample. Proc Natl Acad Sci USA. (2011) 108:4516–22. doi: 10.1073/pnas.1000080107
26. Magoč T, Salzberg SL. FLASH: fast length adjustment of short reads to improve genome assemblies. Bioinformatics. (2011) 27:2957–63. doi: 10.1093/bioinformatics/btr507
27. Wang Q, Garrity GM, Tiedje JM, Cole JR. Naive Bayesian classifier for rapid assignment of rRNA sequences into the new bacterial taxonomy. Appl Environ Microbiol. (2007) 73:5261–7. doi: 10.1128/AEM.00062-07
28. Segata N, Izard J, Waldron L, Gevers D, Miropolsky L, Garrett WS, et al. Metagenomic biomarker discovery and explanation. Genome Biol. (2011) 12:R60. doi: 10.1186/gb-2011-12-6-r60
29. Fouhy F, Watkins C, Hill CJ, O'Shea CA, Nagle B, Dempsey EM, et al. Perinatal factors affect the gut microbiota up to four years after birth. Nat Commun. (2019) 10:1517. doi: 10.1038/s41467-019-09252-4
30. Stockinger S, Hornef MW, Chassin C. Establishment of intestinal homeostasis during the neonatal period. Cell Mol Life Sci. (2011) 68:3699–712. doi: 10.1007/s00018-011-0831-2
31. Jost T, Lacroix C, Braegger CP, Chassard C. New insights in gut microbiota establishment in healthy breast fed neonates. PLoS ONE. (2012) 7:e44595. doi: 10.1371/journal.pone.0044595
32. Grier A, Qiu X, Bandyopadhyay S, Holden-Wiltse J, Kessler HA, Gill AL, et al. Impact of prematurity and nutrition on the developing gut microbiome and preterm infant growth. Microbiome. (2017) 5:158. doi: 10.1186/s40168-017-0377-0
33. Faner R, Sibila O, Agustí A, Bernasconi E, Chalmers JD, Huffnagle GB, et al. The microbiome in respiratory medicine:current challenges and future perspectives. Eur Respir J. (2017) 49:1602086. doi: 10.1183/13993003.02086-2016
Keywords: intestinal micriobiota, pharyngeal microbiota, 16S rRNA sequencing, preterm neonate, gut-lung axis
Citation: Yang S, Qiao L, Shi J, Xie L, Liu Y, Xiong Y and Liu H (2021) Clinical Study of Correlation for the Intestinal and Pharyngeal Microbiota in the Premature Neonates. Front. Pediatr. 9:632573. doi: 10.3389/fped.2021.632573
Received: 23 November 2020; Accepted: 18 January 2021;
Published: 16 February 2021.
Edited by:
Sinno Simons, Erasmus Medical Center, NetherlandsReviewed by:
Grace Tzun-Wen Shaw, Academia Sinica, TaiwanValeriy Poroyko, Covance, United States
Ewa Skarżyńska, Medical University of Warsaw, Poland
Copyright © 2021 Yang, Qiao, Shi, Xie, Liu, Xiong and Liu. This is an open-access article distributed under the terms of the Creative Commons Attribution License (CC BY). The use, distribution or reproduction in other forums is permitted, provided the original author(s) and the copyright owner(s) are credited and that the original publication in this journal is cited, in accordance with accepted academic practice. No use, distribution or reproduction is permitted which does not comply with these terms.
*Correspondence: Ying Xiong, eGlvbmd5aW5nMjQ2JiN4MDAwNDA7MTYzLmNvbQ==; Hanmin Liu, bGl1aG0mI3gwMDA0MDtzY3UuZWR1LmNu
†These authors have contributed equally to this work and share first authorship