- Department of Neonatology, Wilhelmina Children's Hospital, University Medical Center Utrecht, Utrecht University, Utrecht, Netherlands
Worldwide neonatal hypoxic-ischemic encephalopathy (HIE) is a common cause of mortality and neurologic disability, despite the implementation of therapeutic hypothermia treatment. Advances toward new neuroprotective interventions have been limited by incomplete knowledge about secondary injurious processes such as cerebral hyperperfusion commonly observed during the first 1–5 days after asphyxia. Cerebral hyperperfusion is correlated with adverse neurodevelopmental outcome and it is a process that remains poorly understood. In order to provide an overview of the existing knowledge on the pathophysiology and highlight the gaps in current understanding of cerebral hyperperfusion in term animals and neonates with HIE, we performed a systematic research. We included papers scoping for study design, population, number of participants, study technique and relevant findings. Methodological quality was assessed using the checklist for cohort studies from The Joanna Briggs Institute. Out of 2,690 results, 34 studies were included in the final review—all prospective cohort studies. There were 14 studies of high, 17 moderate and 3 of low methodological quality. Data from the literature were analyzed in two main subjects: (1) Hemodynamic Changes subdivided into macro- and microscopic hemodynamic changes, and (2) Endogenous Pathways which was subdivided into N-methyl-D-aspartate/Mitogen activated protein kinase (NDMA/MAPK), Nitric Oxide (NO), prostanoids and other endogenous studies. Cerebral hyperperfusion in term neonates with HIE was found to be present 10–30 min after the hypoxic-ischemic event and was still present around day 10 and up to 1 month after birth. Cerebral hyperperfusion was also characterized by angiogenesis and cerebral vasodilation. Additionally, cerebral vasodilation was mediated by endogenous pathways such as MAPK through urokinase Plasminogen Activator (uPA), by neuronal NO synthase following NMDA and by prostanoid synthesis. Future research should elucidate the precise role of NMDA, MAPK and prostanoids in cerebral hyperperfusion. Moreover, research should focus on possible interventions and the effect of hypothermia on hyperperfusion. These findings should be taken into account simultaneously with brain imagining techniques, becoming a valuable asset in assessing the impact in neurodevelopmental outcome.
Highlights
- What is already known? Cerebral hyperperfusion in term neonates with hypoxic-ischemic encephalopathy is often seen after the acute phase, occurring 6–15 h after the HI-event. Cerebral hyperperfusion is correlated with adverse neurodevelopmental outcome.
- What this review adds? Cerebral hyperperfusion is present 10–30 minutes after the hypoxic-ischemic event and persisted around day 10 and up to 1 month after birth. Cerebral hyperperfusion may be characterized by angiogenesis and cerebral vasodilation. A rise in prostanoids may contribute to vasodilatation trough MAPK. Another finding is that NMDA induces vasodilatation and the cerebroprotective effects of hypothermia therapy are not mediated by NMDA.
- What is still unknown? Future research needs to be developed about the precise role of NMDA, MAPK, prostanoids in cerebral vasodilation, and possible interventions.
Introduction
Hypoxic-ischemic encephalopathy (HIE) is one of the main causes of neonatal death and developmental psychomotor disorders in the pediatric population. The incidence of HIE is ~1–8 per 1,000 live births in technically advanced countries and is up to 26 per 1,000 live births in less developed countries (1). Despite the widespread use of therapeutic hypothermia, a large proportion of infants will suffer from neurodevelopmental impairments, especially in the case of severe HIE (2). Therefore, new synergistic therapies need to be developed.
For clinicians, it is important to understand the pathophysiology of injury after the HI-event, due to its relevance in developing novel therapies and because neuroimaging has shown that subsequent brain injury evolves over the course of days, if not weeks (3). In the process of neonatal encephalopathy, a hypoxic-ischemic event, with intermitting anoxia or acute hypoxia, leads to a decreased cerebral perfusion, if the event occurs for long enough. This decreased perfusion sets a time-related pattern of injury pathways in motion, which is divided into distinct phases (4, 5). Figure 1 provides a schematic overview of the phases of injury in HIE. After the hypoxic-ischemic event, there is the acute phase of injury, which is followed by a latent phase of injury. After ~6–15 h, there is a secondary phase. A tertiary phase occurs weeks after the hypoxic-ischemic event. More extensive:
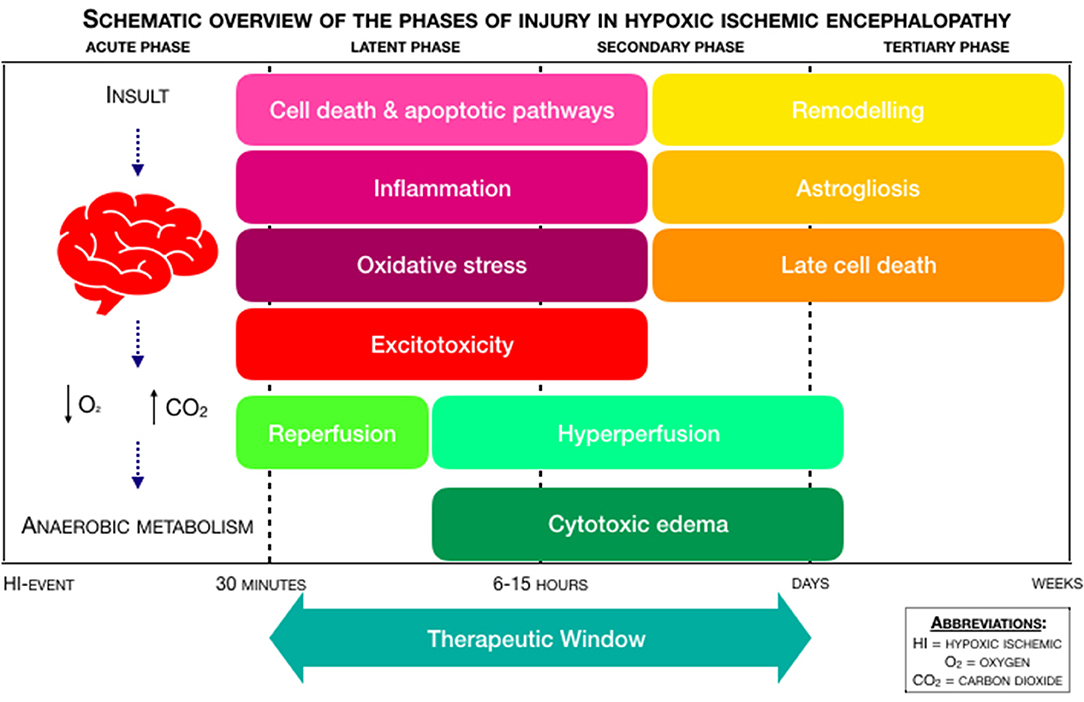
Figure 1. Schematic overview of the phases of injury in Hypoxic-Ischemic Encephalopathy (HIE), adapted from Douglas-Escobar et al. (4) and Hassell et al. (5).
- The acute phase, also known as “primary energy failure,” is characterized by anaerobic metabolism, oxidative stress, neuronal cell death, and excitotoxicity. In the process of excitotoxicity, a surplus in the amount of the excitatory amino acid glutamate leads to overstimulation of the 2-(aminomethyl)phenylacetic acid (AMPA), kainite (KA), and N-methyl-D-aspartate (NMDA) receptors. Overstimulation of AMPA and KA causes an influx of sodium (Na+) and chloride (Cl−), which leads to an increased cellular osmolality. Overstimulation of NMDA triggers the influx of calcium (Ca2+), which leads to apoptosis and necrosis (4). The mitogen activated protein kinase (MAPK) signaling pathway is overactivated after the hypoxic-ischemic event, leading to neuronal apoptosis. Prostanoids also play a role in the hypoxic-ischemic event: stimulating prostanoid-receptors may be neuroprotective and prevent neuronal cerebellar injury (4). Depending on the duration of decreased perfusion and the presence or absence of medical intervention, a partial recovery with reperfusion occurs from 30 to 60 min after the hypoxic-ischemic event (6).
- The latent phase of injury follows after the acute phase, which may last from 1 to 6 h. This latent phase is characterized by neuroinflammation and the continuation of activated apoptotic cascades. In neonates with moderate to severe HIE, the latent phase is followed up by the secondary phase, also known as “secondary energy failure” (4).
- The secondary phase occurs within ~6–15 h and is characterized by cytotoxic edema, excitotoxicity and cerebral hyperperfusion (7).
- The tertiary phase occurs in the weeks or months following primary energy failure. It involves remodeling of the injured brain, astrogliosis, and late cell death (8).
In this review, we focused on pathophysiology of cerebral hyperperfusion, often seen in the secondary phase of HI-injury, and not on the adverse outcome itself. Cranial ultrasound (cUS) and brain Magnetic Resonance Imaging (MRI) are the most used imaging techniques in neonates with HIE. Cerebral hyperperfusion seen on Arterial Spin Labeling (ASL) MRI is correlated with an adverse neurodevelopmental outcome (2, 9). To understand cerebral hyperperfusion, it is essential to elucidate when this phenomenon takes place and what the underlying mechanisms leading to hyperperfusion are. Understanding the pathophysiological mechanisms behind these observations will provide an insight into how cerebral hyperperfusion is associated with (adverse) outcome.
Given that this topic is prone to rapid changes in what is known to the scientific community, the review intents to provide a summary of the methods, models and studies already existing. With this paper we hope to create a platform that allows researchers to come up with novel ideas, using this review as a gateway for new research. This study aims to provide an overview of hemodynamic changes in cerebral hyperperfusion and endogenous pathways leading to cerebral hyperperfusion in term neonates with HIE.
Methods
Study Design
A systematic review was performed following the steps of the Preferred Reporting Items for Systematic Reviews and Meta-Analysis (PRISMA) statement (10). Prior to initiation, the systematic review protocol was registered in PROSPERO (https://www.crd.york.ac.uk/PROSPERO), registration number CRD42020152957. Because the studies in this systematic review were considered to be heterogeneous regarding study population, study technique and study outcome, we refrained from statistically pooling the data in a meta-analysis and performed a best evidence synthesis. The results of this systematic review will be described in a narrative manner.
Inclusion and Exclusion Criteria
To systematically review the pathophysiology of hyperperfusion in term neonates with hypoxic-ischemic encephalopathy, we conducted a search including papers in which term neonates (>36 weeks' GA) had a diagnosis of HIE caused by perinatal asphyxia, with or without hypothermia treatment. We also included animal studies with models of perinatal HIE at term equivalent age, because pathophysiology is more thoroughly studied in animal models. We excluded reviews, articles on preterm neonates (<36 weeks' GA), articles with pooled data on both term and preterm neonates, articles on adults and articles on neonates with main pathology that alters the brain physiology (main cerebral hemorrhage, metabolic disorders, main cardiac abnormalities or chromosomic disorders). We also excluded articles with data on NIRS or MRI when they did not report effects on vascular resistance, (re)perfusion or cerebral blood volume. No date restrictions were applied. We limited the search to English articles only. When analyzing results, the authors considered rats and mouses as “small animals” and piglets and lambs as “large animals.”
Since autoregulation is not the scope of this review, we did not include articles on this topic specifically. If the reader would like to have an in-depth overview of cerebral vascular autoregulation (CVAR) studies using NIRS in neonates and given that autoregulation is not the main focus of the review, we would like to refer to the paper of Thewissen et al. (11).
Search Strategy
The research question was defined according to the PICOTS system (Supplementary Appendix 1). The main search terms were Hypoxic-Ischemic Encephalopathy (HIE), Vasodilation and (Re-)perfusion. Besides title and abstract, MeSH-terms were used for all search terms (Supplementary Appendixes 2–4). A medical librarian with systematic review experience helped in developing the search strategy.
Data Sources, Studies Selections, and Data Extraction
Medline (PubMed) and Embase were searched from inception to December 12, 2018, with similar search strategies. Two researchers (D.K. and K.A.) independently analyzed all abstracts after removing duplicates while appraising the in- and exclusion criteria. The full text of potentially eligible studies was then assessed for inclusion using the same inclusion and exclusion criteria. If an inconsistency occurred during the abstract or full text analysis, a consensus was reached in a meeting or by the involvement of a third researcher (J.D.). Data were extracted and reviewed by D.K. and A.H. The characteristics of the included studies were recorded using data extraction forms, which included the following characteristics: study design, population, number of participants, study technique and relevant findings. Before submission of the review, the search was repeated in Medline and Embase for additional articles.
Methodological Quality
The Checklist for Cohort Studies from The Joanna Briggs Institute Critical Appraisal Tools was used to assess the methodological quality of the individual studies (12). The methodologic quality was assessed independently by two researchers (D.G.K. and A.H.), and disagreements were resolved in a meeting or by the consideration of a third researcher (J.D). We calculated the score and added up “Yes” as 1; “No” as 0 and “Unclear” as 0. Quality was calculated as the added up score, divided by the number of questions answered with “Yes,” “No” or “Unclear.” (Non-Applicable was not taken into account.) For example, 7 of 11 questions were answered with Yes and 1 question was answered with Non-Applicable. The calculated score is 7/(11–1) = 7/10 = 70%. A score of ≥80% was considered a study of high quality. A score between 60 and 80% was considered as moderate quality, whereas a score of ≤ 60% was considered to be of low quality. These cut-off values were agreed with the research team.
Best-Evidence Synthesis
A best-evidence synthesis was performed since the outcome measures of the included studies were too heterogeneous for a meta-analysis. We used the guidelines from Proper et al. (13) to synthesize the methodologic quality of the studies and to be able to reach conclusions regarding the pathophysiology of cerebral hyperperfusion. The guideline consists of the following three levels:
1. Strong evidence: consistent findings in multiple (≥2) high-quality studies;
2. Moderate evidence: consistent findings in one high-quality study and at least one low-quality study, or consistent findings in multiple low-quality studies;
3. Insufficient evidence: only one study available or inconsistent findings in multiple (≥2) studies.
Results were considered to be consistent when a minimum of 75% of the studies showed results in the same direction, which was defined according to significance (p < 0.05). If there were two or more high-quality studies, the studies of low methodologic quality were disregarded in the evidence synthesis.
Results
The search in Medline and Embase provided 3,253 results. Figure 2 provides a detailed overview of study screening and selection. The additional search in Medline and Embase before submission of the review did not provide further articles.
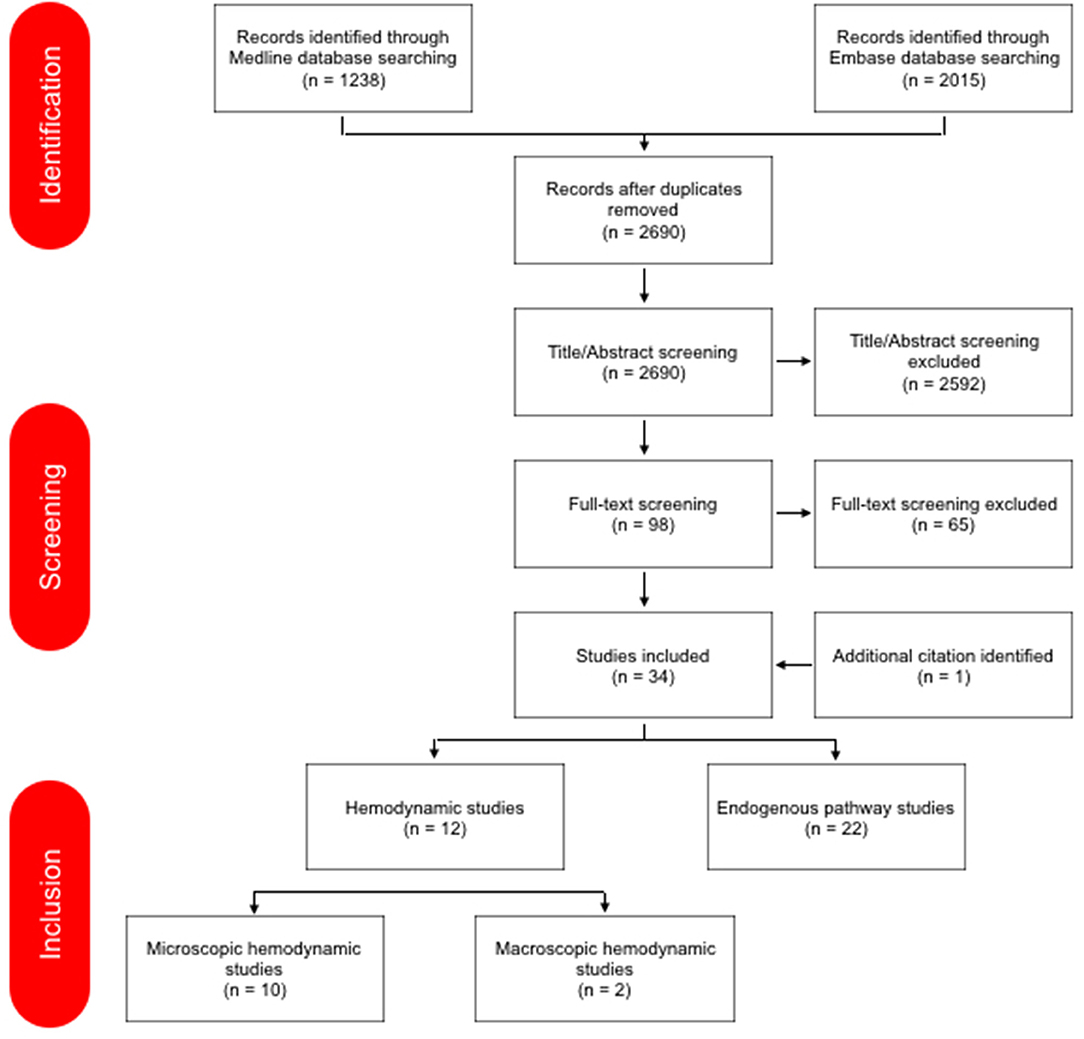
Figure 2. PRISMA flow diagram of the study selection (10).
Data from the literature were analyzed and presented below in two main subjects: (1) Hemodynamic Changes subdivided into macro- and microscopic hemodynamic changes, and (2) Endogenous Pathways which was subdivided into NDMA/MAPK, NO, prostanoids, and other endogenous studies. This makes that the review has a total of six themes.
A total of 34 studies were eligible for the review; 12 hemodynamic changes studies and 22 endogenous pathway studies. In total, 44 human newborns and 334 animals (169 large animals; 165 small animals) were included in the hemodynamic changes studies. In the endogenous pathway studies, there were no human newborns studies included. A total of 775 animals (656 large animals; 119 small animals) in the endogenous pathway studies were studied; in five studies (14–18) the number of included animals in the study was unknown. All studies were prospective cohort studies. A separate subject about human studies was not made because there were not enough human studies for that. Characteristics, relevant findings and methodological quality of each study are summarized in Tables 1, 2.
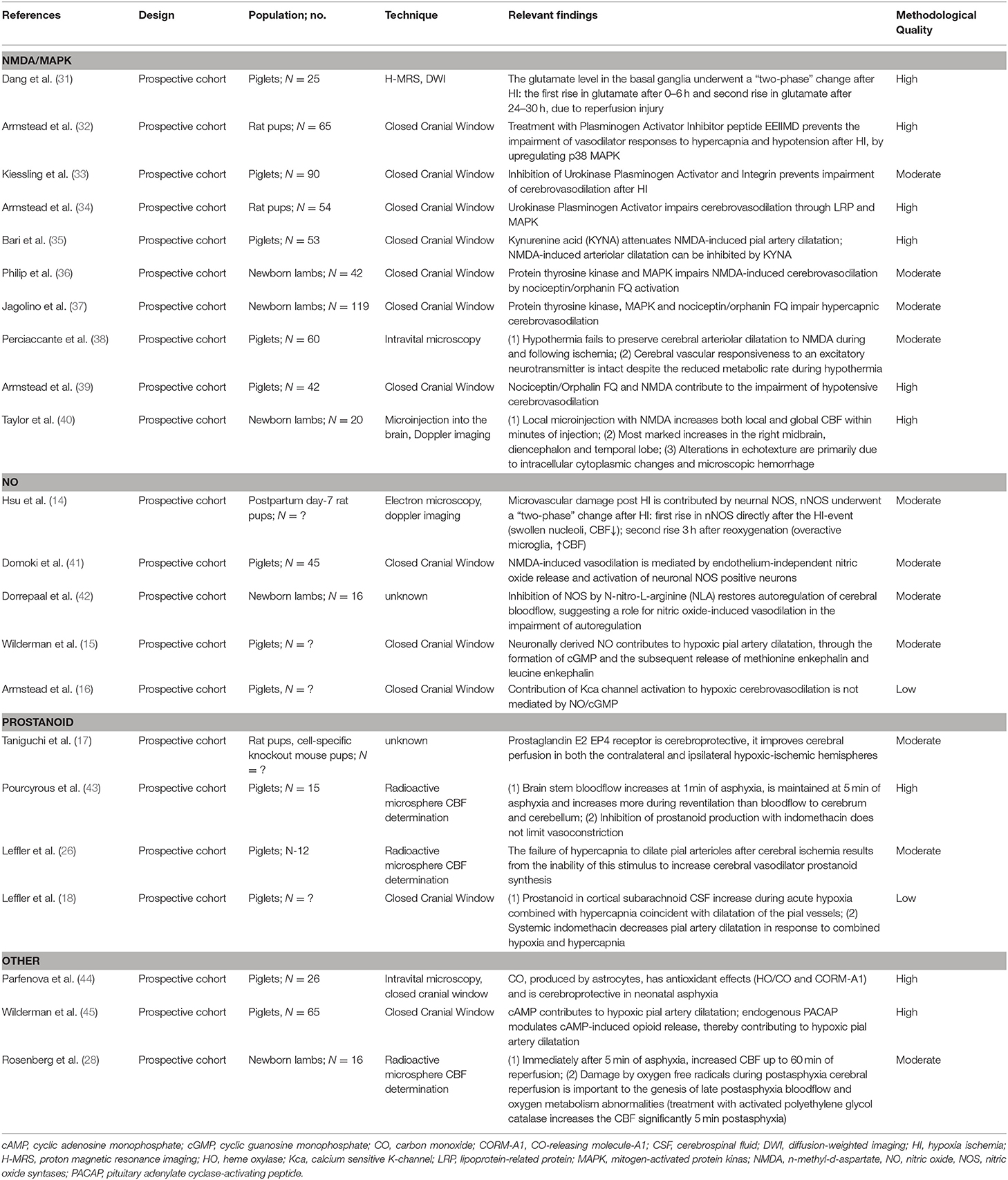
Table 2. Study characteristics endogenous pathway studies, relevant findings, and methodological quality.
There were 14 studies of high, 17 studies of moderate and three studies of low methodological quality (Tables 1, 2). Table 3 shows the critical review of the studies, presenting the questions to assess methodological quality and the answers to these questions.
Macroscopic Hemodynamic Changes
Ten studies were included describing hemodynamic changes on a macrovascular level (Figure 3). Regarding small animal studies, Manole et al. (25), showed that after the hypoxic-ischemic (H-I) event cerebral blood flow (CBF) increases within 5 min and subsided after 10 min (studied with MRI). Wang et al. (23) used virtual touch tissue quantification (VTQ), histological staining and ultrasound to determine the severity of brain damage from HIE. In the asphyxia group, the diastolic velocity (Vd) was significantly increased 3 h post carotid ligation compared to the ischemia group, leading to a lower resistance index (RI) of the right MCA, indicating that the elasticity of the cerebral blood vessels decreases. This study suggests that there is a consistent correlation among histological and hemodynamic changes, VTQ values and neurodevelopmental outcome. Additionally, another small animal study showed in what regions hyperperfusion takes place. The study of Buckley et al. (21), showed hyperperfusion in both hemispheres, and more pronounced in the contralateral hemisphere (studied with diffuse correlation spectroscopy).
Moving to large animal studies, Rosenberg studied cerebral blood flow (CBF) in 1986 (28) and in 1988 (27). Rosenberg et al. (27) showed that CBF was increased 2 h after the H-I event, although the increase was attenuated. The same group (28) demonstrated that reactive hyperemia was followed by a period of hypoperfusion. Cerebral oxygen delivery increased, while cerebral oxygen consumption was significantly decreased when compared to control, suggesting mitochondrial dysfunction. Additionally, cerebral fractional oxygen extraction (CFOE) decreased. CBF increased in response to induced hypoxia at 2–5 h after HI, but the increase is attenuated and is insufficient to maintain oxygen delivery. Cerebral oxygen consumption remained stable due to a proportional increase in CFOE (27). Another large animal study showed that an increased CBF subsided after 20 min of reperfusion (26) (studied with radiolabeled microspheres).
The study of Chakkarapani et al. (24) investigated cerebral perfusion by using cerebrovascular pressure reactivity (PRx). In this study, PRx was shown to be impaired during and after a H-I event. The study mentioned that a secondary PRx peak happened after 6.5 h in the normothermia group and after 11.5 h in the hypothermia group and that this was predictive of severe neuropathology and greater insult severity. Nakamura et al. (22), showed that an increased CBF within 6 h after the hypoxic-ischemic event indicates more marked histopathological damage [studied with near-infrared time-resolved spectroscopy (TRS)].
One large animal study investigated in what regions hyperperfusion takes place. Leffler et al. (26), studied hyperperfusion with radioactive-labeled microspheres. This study showed that hyperperfusion was present in the cerebellum, diencephalon, mesencephalon, medulla and spine.
Regarding human newborns, the study of Wu et al. (20) suggested reasons why CBF was increased. Wu et al. (20) used electrical velocimetry and transcranial doppler and indicated that there was an increased cardiac output (CO) in the rewarming phase after hypoxia-ischemia, which was due to an increase in heart rate. This study also showed an increase in the peak systolic velocity of the middle cerebral artery (MCA). The study of Shaikh et al. (29) studied both macroscopic and microscopic hemodynamic changes. This study included term asphyxiated newborns treated with hypothermia. In this study, regional CBF was studied around day 10 and around 1 month of life using MRI and ASL. They found that there was an increased cerebral blood flow around day 10 in life and around 1 month of life, with no reported information between those two points.
Microscopic Hemodynamic Changes
Two articles also described hemodynamic changes on a microvascular level (29, 30). Shaikh et al. (29), the same study as in the macroscopic hemodynamic changes paragraph, studied angiogenesis in a small animal model of neonatal encephalopathy. They found that Vascular Endothelial Growth Factor (VEGF) was expressed in the first days of life of postmortem human brain tissue and rat pups after the hypoxic-ischemic event. Moreover, the count of endothelial cells was increased on 7 and 11 days after hypoxia-ischemia, indicating that there is active angiogenesis after hypoxia-ischemia (Figure 4).
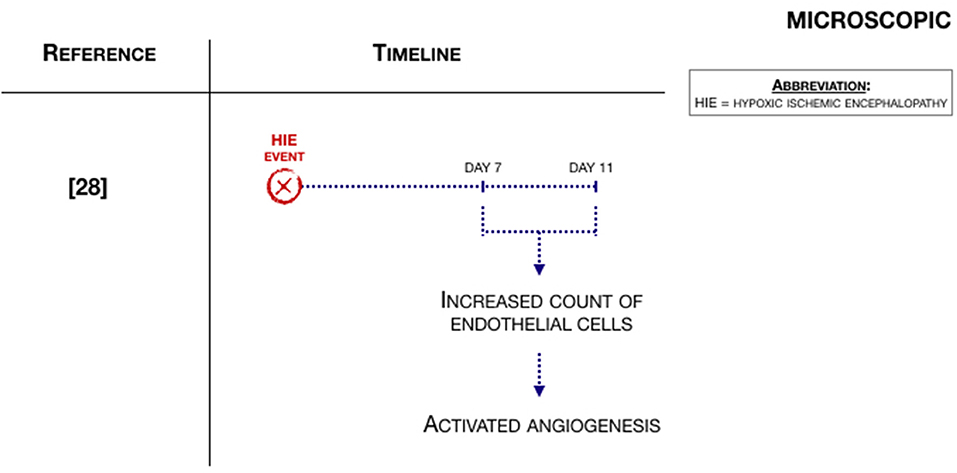
Figure 4. Microscopic hemodynamic changes associated with an HIE event (29).
Regarding large animals, Domoki et al. (30), studied microvascular hemodynamics with laser-speckle imaging. In five out of seven animals, marked cortical hyperemia was found 30 min after the initiation of hypoxia, with an increase in pial arteriolar diameters, simultaneously with arteriolar flow velocity. However, bilateral carotid artery occlusion (BCAO) could not elicit cortical ischemia and the data does not demonstrate a statistically significant effect.
Endogenous Pathways—NMDA/MAPK
In this section, we highlighted studies that described the effects of N-methyl-D-aspartate (NMDA) or mitogen activated protein kinase (MAPK). Figure 5 is a graphical representation of these pathways. The current literature shows that in the process of neuronal excitotoxicity, overstimulation of the NMDA receptor by glutamate triggers the influx of calcium, which leads to apoptosis and necrosis. The MAPK-pathway plays also an important role in neonatal HIE because the overstimulation of this pathway also leads to apoptosis and necrosis. There were three studies (32–34) that all described that Urokinase Plasminogen Activator (uPA) impairs cerebral vasodilation.
Regarding small animal studies, Armstead et al. (34) described that uPA impairs cerebral vasodilation through lipoprotein-related protein (LRP) and the ERK isoform of MAPK. Additionally, Armstead et al. (32) confirms this with a uPA inhibitor and suggests that upregulation of p38 MAPK (a class of MAPK that reacts to stress stimuli) prevents cerebral vasodilation.
Moving to large animal studies, Kiessling et al. (33) described that inhibition of uPA could prevent vasodilatation, suggesting new therapeutic possibilities for clinical practice. On top of that, three other studies (36, 37, 39) described the effects of nociceptin/orphanin FQ and of Protein Tyrosine Kinase, showing that both can partially prevent hypotensive pial dilation impairment, when coadministred with the NMDA antagonist MK801.
The Doppler imaging study of Taylor et al. (40) described that local microinjection with NMDA resulted in increased local and global CBF within minutes of injection. Bari et al. (35) found that NMDA-induced arteriolar dilatation can be inhibited by Kynurenine acid (KYNA). KYNA is a non-competitive antagonist to NMDA receptors and an antagonist to glutamate receptors, suggesting that KYNA attenuates NMDA-induced pial artery dilatation. The study of Perciaccante et al. (38), a study in which the population underwent intravital microscopy, suggests that hypothermia does not affect cerebral arteriolar dilatation to NMDA during and following ischemia, indicating that the cerebroprotective effects of hypothermia therapy are not mediated by NMDA. The study of Dang et al. (31), using proton magnetic resonance spectroscopy (1H-MRS) and diffusion-weighted imaging (DWI), showed that the glutamate level in the basal ganglia underwent a “two-phase” change after HI: first a rise in glutamate after 0–6 h and secondly a rise in glutamate after 24–30 h, due to reperfusion injury.
Endogenous Pathways—NO
Nitric Oxide (NO) plays a major role in vessel dilatation. According to the study of Hsu et al. (14), a study with small animals that underwent Doppler imaging, neuronal NO synthase (nNOS) suffered a “two-phase” change after HI (Figure 6). The first rise in nNOS is directly after the HI-event and is characterized by swollen nuclei and a decreased CBF. The second rise in nNOS happens 3 h after reoxygenation and is characterized by overactive microglia and an increased CBF.
In large animal models, the study of Domoki et al. (41) described the relation between NO and NMDA. This study concluded that NMDA-induced vasodilation is mediated by endothelium-independent NO release and activation of nNOS positive neurons. Dorrepaal et al. (42) showed that neuronally derived NO contributes to hypoxic pial artery dilatation (Figure 6), through the formation of cGMP (cyclic guanosine monophosphate) and the subsequent release of methionine enkephalin and leucine enkephalin. The study of Armstead et al. (16) showed that the contribution of calcium-activated potassium channels to hypoxic cerebral vasodilation is not mediated by NO/cGMP.
Endogenous Pathways—Prostanoids
Prostanoids may be neuroprotective in neonatal HIE by stimulating the prostanoid E2 receptor (Figure 7). Four studies showed the effects of prostanoids on cerebral blood flow and vasodilation. Taniguchi et al. (17) showed that activation of the prostaglandin E2 EP4 receptor in small animals improved cerebral perfusion in both hemispheres and is therefore neuroprotective. In large animal studies that considered pial vessel mechanisms, Leffler et al. (18) showed that prostanoids were increased in subarachnoid CSF during acute hypoxia combined with hypercapnia, while Pourcyrous et al. (43) showed that inhibition of prostanoid production with indomethacin decreases pial artery dilation in response to combined hypoxia and hypercapnia. In another study from the same group, Leffler et al. (46), they showed that hypercapnia didn't result in dilation of pial arterioles after cerebral ischemia, because of the inability of hypercapnia to increase cerebral vasodilator prostanoid synthesis.
Endogenous Pathways—Other
Three large animal studies did not fit the two main topics mentioned above. One study that focused on oxygen free radicals: Rosenberg et al. (47) showed that in damage by oxygen free radicals following hypoxia-ischemia, cerebral reperfusion plays a relevant role in the genesis of late postasphyxia blood flow and oxygen metabolism abnormalities. Treatment with polyethylene glycol catalase with oxygen free radicals increases the CBF significantly 5 min after the hypoxic-ischemic event, probably due to increased endothelial permeability. Another study focused on carbon monoxide (44). Carbon monoxide, produced by astrocytes, has antioxidants effects by heme oxylase/ carbon monoxide (HO/CO) and carbon monoxide release molecule-A1 (CORM-A1). HO/CO and CORM-A1 are neuroprotective in perinatal asphyxia, reducing brain oxidative stress and protecting against cerebrovascular dysfunction caused by prolonged neonatal asphyxia. The last study focused on cyclic adenosine monophosphate (cAMP): Wilderman et al. (45) concluded that cAMP contributes to hypoxic pial artery dilatation. Endogenous pituitary adenylate cyclase-activating peptide (PACAP) modulates cAMP-induced opioid release, thereby contributing to hypoxic pial artery dilatation.
Best-Evidence Synthesis
Overall, we found strong evidence in the studies regarding macroscopic and microscopic hemodynamic changes, the studies regarding NMDA/MAPK and the studies that did not fit the topics and were named in the “other” paragraph. We found moderate evidence in the studies with data on NO and prostanoids. There were no studies that reported insufficient evidence.
There were only two studies that reported data on human neonates (both high-quality studies): Wu et al. (20) and Shaikh et al. (29). Wu et al. (20) mentioned in the macroscopic hemodynamics paragraph, studied 20 human newborns that underwent electrical velocimetry and transcranial doppler. This study indicated that there was an increased cardiac output (CO) in the rewarming phase after hypoxia-ischemia, which was due to an increase in heart rate. This study also showed that the peak systolic velocity of the middle cerebral artery (MCA) was increased. Through NIRS measurements, Wu et al. (20) also mentioned that there were no changes in regional cerebral oxygen saturation (CrO2) or cerebral fractional oxygen extraction (CFOE), suggesting that cerebral flow metabolism coupling remained intact during rewarming. Shaikh et al. (29), mentioned in the microscopic hemodynamics paragraph, included 24 term asphyxiated newborns treated with hypothermia. In this study, regional CBF was studied around day 10 and around 1 month of life using MRI and ASL. They found that there was an increased cerebral blood flow around day 10 in life and around 1 month of life, with no reported information between those two points (29).
Discussion
Cerebral hyperperfusion seen in term neonates with hypoxic-ischemic encephalopathy (HIE) has been correlated with an adverse neurodevelopmental outcome (2, 9, 22–24). In order to understand how cerebral hyperperfusion is correlated with an adverse outcome, we must understand the pathophysiology. We reviewed current literature on the pathophysiology of cerebral hyperperfusion in term neonates with HIE.
Cerebral hyperperfusion may be increased up to 2–5 h after the hypoxic-ischemic event. Moreover, a secondary pressure reactivity peak may be predictive of severe neuropathology. Additionally, therapeutic hypothermia was shown to cause a 5-h delay in cerebral hyperperfusion (11.5 h instead of 6.5 h). Moreover, hyperperfusion is present in the cerebellum, diencephalon, mesencephalon, medulla and spine. Microscopic cerebral hyperperfusion was found to start 10–30 min after the initiation of hypoxia and was still observed around day 10 and around 1 month after birth. Moreover, after the hypoxic-ischemic event, VEGF is expressed and the endothelial cell count is increased, suggesting that angiogenesis is activated after hypoxia-ischemia. On the subject of NMDA and MAPK's endogenous pathway, we found that a rise in uPA can reduce cerebral vasodilation trough MAPK and that Nociceptin/Orphanin FQ contributes to the impairment of cerebral vasodilation. Moreover, NMDA induces vasodilation and hypothermia fails to preserve cerebral dilatation. Due to the reperfusion, there is a second rise in the excitatory amino acid glutamate after 24–30 h and it was also found that NMDA-induced vasodilation is mediated by nNOS through the formation of cGMP. Regarding prostanoids, they were found to be increased during hypoxia-ischemia and their synthesis' inhibition does not limit vasoconstriction. Lastly, prostanoid synthesis may contribute to vasodilation and improve perfusion by the prostaglandin E2 EP4 receptor.
This review suggests that cerebral hyperperfusion may be characterized by angiogenesis and cerebral vasodilation.
Cerebral vasodilation may be mediated by MAPK through uPA, by NMDA through nNOS and by prostanoid synthesis. Inhibition of prostanoid synthesis with indomethacin and inhibition of uPA may limit cerebral vasodilation and therefore may limit cerebral hyperperfusion. By understanding the pathophysiology, we can recognize and comprehend clinical patterns on diagnostic techniques earlier. Early diagnostics of cerebral hyperperfusion in neonates with HIE, can be beneficial for the development of new therapeutics.
There are several diagnostic techniques to detect cerebral hyperperfusion in neonates with HIE. Cranial Ultrasound (cUS) is a powerful and inexpensive alternative tool for MRI. cUS is widely available and can be repeated as often as necessary. cUS has no side effects, and, when performed by an experienced sonographer, provides a wealth of anatomical and functional information. According to Salas et al. (48), characteristic cUS findings of a term neonates with HIE are an increased echogenicity in the thalami, an enhanced gray-white matter differentiation and slit-like ventricles due to edema from the cortical structures. Duplex Ultrasonography (DUS) typically shows decreased resistance index (RI) values (<0.60), as decreased RI values are highly predictive of poor prognosis with either death or severe disability. The study of Archer et al. (49) also described that a very low RI, corresponding to “luxury hyperperfusion,” was correlated with an adverse outcome. One study in our search described a decreased RI of the right MCA in the asphyxia group, compared to the ischemia group (23). This study suggests that with hypoxic-ischemic injury, the elasticity of the brain tissue decreases, and these results are consistent with the pathological findings.
Brain Magnetic Resonance Imaging (MRI) is one of the most used techniques in neonates with HIE and is useful to predict long-term outcomes. In the study of Wintermark et al. (2), 18 asphyxiated neonates underwent MR imaging and ASL-PI (perfusion imaging by arterial spin labeling). In neonates treated with hypothermia, hyperperfusion occurred on day 2–3 in brain areas subsequently exhibiting injury. In neonates with normothermia, hyperperfusion occurred on days 1–6. This study also suggests that early hyperperfusion is correlated with later brain injury even in infants treated with induced hypothermia. Given that this study was not reviewing the pathophysiology but only hemodynamics, we excluded this study in the results. The finding that hypothermia delays the occurrence of hyperperfusion is also found in the study of Chakkarapani et al. (24). In this study, using cerebrovascular pressure reactivity (PRx) in piglets, a secondary PRx peak was associated with severe neuropathology and with greater insult severity and happened after 6.5 h in the normothermia group and after 11.5 h in the hypothermia group.
In the study of De Vis et al. (9), where 28 neonates diagnosed with HIE were assessed using MR imaging, 1H-MRS, and ASL MRI, the main finding is that basal ganglia and thalami perfusion is higher in neonates with an adverse outcome. We have not found studies that investigated perfusion in the basal ganglia and the thalami specifically.
Limitations
There are several limitations in this systematic review. At first, when assessing the methodological quality of the included studies, we made several assumptions: we defined newborn animals as ≤ 7 days old, according to the Medline definition of “perinatal care.” Studies with newborns >7 days old were excluded. When studies did not describe the age of the participated animals, we answered questions 1 and 2 in the critical appraisal as unknown. Additionally, when gender or weight was not described in the study, we assumed that this was a normal distribution of men and female animals and a normal weight. In most of the studies, there was no baseline table for the participating animals, so we assumed that they were healthy newborns without any outcome in the beginning of the study. In agreement with the research team, a follow-up time of ≥24 h was considered to be long enough for cerebral hyperperfusion to occur. Second, because the included studies were too heterogeneous for a meta-analysis, we narratively described the studies and used Proper guidelines for the best-evidence synthesis. For a systematic review, a meta-analysis would be favorable because the precision and accuracy of estimates can be optimized as more data is used, which also means that it may increase the statistical power to detect an effect. Only a few studies in our search were studies on human neonates. The aim of this review is to give an overview of the knowledge so far and make way for new research ideas, which can come from both human and animal populations. The fact that some of the studies considered in this review were related to animal models, means that age-estimation and correlations to human newborn models may pose as a challenge for future research. Moreover, one must consider the fact that small and large animals have different pathophysiologic mechanisms, and that should be taken into account when considering the different results. Finally, in five studies (14–18) the number of animals participated in the study is unknown, therefore generalization to other animal studies was impossible. Because of various limitations, the results have to be treated with caution.
Conclusion
The main findings are that cerebral hyperperfusion is present 10–30 min after the hypoxic-ischemic event and persisted around day 10 and up to 1 month of life. Cerebral hyperperfusion may be characterized by angiogenesis and cerebral vasodilation. We found that a rise in prostanoids can reduce cerebral vasodilation trough MAPK and that Nociceptin/Orphanin FQ contributes to the impairment of cerebral vasodilation. Moreover, NMDA induces vasodilation and the cerebroprotective effects of hypothermia therapy are not mediated by NMDA. Due to reperfusion, there is a second rise in the excitatory amino acid glutamate after 24–30 h and it was also found that NMDA-induced vasodilation is mediated by nNOS through the formation of cGMP. Regarding prostanoids, they were found to be increased during hypoxia-ischemia and their synthesis' inhibition does not limit vasoconstriction. Lastly, prostanoid synthesis may contribute to vasodilation and improve perfusion by the prostaglandin E2 EP4 receptor.
Implications and Suggestions for Future Research
The exact mechanism of cerebral hyperperfusion is not known yet, but we showed why it is clinically important. To develop new therapeutics for neonatal HIE, future research about the role of NMDA and MAPK and the implications of agents that can inhibit uPA or prostanoids, such as indomethacin in cerebral vasodilation needs to be developed. Given that there were only three studies (24, 29, 38) that described the effect of hypothermia therapy on cerebral hyperperfusion, evidence is limited and therefore, future research should also focus on this. Moreover, further research is required to translate these findings into clinical practice. These findings should be taken into account simultaneously with brain imagining techniques, as they present themselves as a valuable asset in assessing the neurodevelopment throughout days/weeks after the hypoxic-ischemic event.
Data Availability Statement
The original contributions presented in the study are included in the article/Supplementary Material, further inquiries can be directed to the corresponding authors.
Author Contributions
DK contributed to the study concept, screened titles and abstracts, analyzed the data, and wrote the article. FC prepared the figures and contributed with writing the article. KA screened titles and abstracts and analyzed the data. AH, TA, and FG critically reviewed the article. MB and JD directed the project. All authors contributed to the article and approved the submitted version.
Funding
The authors acknowledged the financial support of EU H2020 MSCA-ITN-2018: INtegrating Functional Assessment measures for Neonatal Safeguard (INFANS), funded by the European Commission under Grant Agreement #813483.
Conflict of Interest
The authors declare that the research was conducted in the absence of any commercial or financial relationships that could be construed as a potential conflict of interest.
Acknowledgments
We thank Drs. Paulien Wiersma (P.W.), information specialist at Utrecht University Library, University Medical Center Utrecht, for helping with the search strategy.
Supplementary Material
The Supplementary Material for this article can be found online at: https://www.frontiersin.org/articles/10.3389/fped.2021.631258/full#supplementary-material
Supplementary Appendix 1. Review question defined according to the PICOTS system.
Supplementary Appendix 2. Summarized search strategy.
Supplementary Appendix 3. Medline search strategy.
Supplementary Appendix 4. Medline search string.
Abbreviations
AMPA, 2-(aminomethyl)phenylacetic acid; LRP, Lipoprotein-Related Protein; ASL, Arterial Spin Labeling; MABP, Mean Arterial Blood Pressure; BCAO, Bilateral Carotid Artery Occlusion; MAPK, Mitogen activated protein kinase; cAMP, Cyclic Adenosine Monophosphate; MCA, Middle Cerebral Artery; CBF, Cerebral Blood Flow; MRI, Magnetic Resonance Imaging; CFOE, Cerebral Fractional Oxygen Extraction; NIRS, Near-Infrared Spectroscopy; cGMP, Guanosine 3',5'-cyclic Monophosphate; NMDA, N-methyl-D-aspartate; CO, Cardiac Output; (n)NO, (neuronal) Nitric Oxide; CORM-A1, Carbon Monoxide Release Molecule-A1; PACAP, Pituitary Adenylate Cyclase-Activating Peptide; CrO2, Cerebral Oxygen Saturation; PRISMA, Preferred Reporting Items for Systematic Reviews and Meta-Analysis; CSF, Cerebral-Spinal Fluid; PRx, Pressure Reactivity; DWI, Diffusion-Weighted Imaging; RI, Resistance Index; GA, Gestational Age; uPA, Urokinase Plasminogen Activator; HIE, Hypoxic-Ischemic Encephalopathy; US, Ultrasound; HO/CO, Heme Oxylase/Carbon Monoxide; Vd, Diastolic Velocity; KA, Kainite; VEGF, Vascular Endothelial Growth Factor; KYNA, Kynurenine Acid; VTQ, Virtual Touch Quantification.
References
1. Kurinczuk JJ, White-Koning M, Badawi N. Epidemiology of neonatal encephalopathy and hypoxic-ischaemic encephalopathy. Early Hum Dev. (2010) 86:329–38. doi: 10.1016/j.earlhumdev.2010.05.010
2. Wintermark P, Hansen A, Gregas MC, Soul J, Labrecque M, Robertson RL, et al. Brain perfusion in asphyxiated newborns treated with therapeutic hypothermia. AJNR Am J Neuroradiol. (2011) 32:2023–9. doi: 10.3174/ajnr.A2708
4. Douglas-Escobar M, Weiss MD. Hypoxic-ischemic encephalopathy: a review for the clinician. JAMA Pediatr. (2015) 169:397–403. doi: 10.1001/jamapediatrics.2014.3269
5. Hassell KJ, Ezzati M, Alonso-Alconada D, Hausenloy DJ, Robertson NJ. New horizons for newborn brain protection: enhancing endogenous neuroprotection. Arch Dis Child Fetal Neonatal Ed. (2015) 100:F541–52. doi: 10.1136/archdischild-2014-306284
6. Bennet L, Roelfsema V, Pathipati P, Quaedackers JS, Gunn AJ. Relationship between evolving epileptiform activity and delayed loss of mitochondrial activity after asphyxia measured by near-infrared spectroscopy in preterm fetal sheep. J Physiol. (2006) 572 (Pt 1):141–54. doi: 10.1113/jphysiol.2006.105197
7. Lorek A, Takei Y, Cady EB, Wyatt JS, Penrice J, Edwards AD, et al. Delayed (“secondary”) cerebral energy failure after acute hypoxia-ischemia in the newborn piglet: continuous 48-hour studies by phosphorus magnetic resonance spectroscopy. Pediatr Res. (1994) 36:699–706. doi: 10.1203/00006450-199412000-00003
8. Bennet L, Tan S, den Heuij L, Derrick M, Groenendaal F, van Bel F, et al. Cell therapy for neonatal hypoxia-ischemia and cerebral palsy. Ann Neurol. (2012) 71:589–600. doi: 10.1002/ana.22670
9. De Vis JB, Hendrikse J, Petersen ET, de Vries LS, van Bel F, Alderliesten T, et al. Arterial spin-labelling perfusion MRI and outcome in neonates with hypoxic-ischemic encephalopathy. Eur Radiol. (2015) 25:113–21. doi: 10.1007/s00330-014-3352-1
10. Liberati A, Altman DG, Tetzlaff J, Mulrow C, Gøtzsche PC, Ioannidis JPA, et al. The PRISMA statement for reporting systematic reviews and meta-analyses of studies that evaluate healthcare interventions: explanation and elaboration. BMJ. (2009) 339:b2700. doi: 10.1136/bmj.b2700
11. Thewissen L, Caicedo A, Lemmers P, Van Bel F, Van Huffel S, Naulaers G. Measuring near-infrared spectroscopy derived cerebral autoregulation in neonates: from research tool toward bedside multimodal monitoring. Front Pediatr. (2018) 6:117. doi: 10.3389/fped.2018.00117
12. Johanna Briggs Institute. Critical Appraisal Tools: Checklist for Cohort Studies. (2017). Available online at: https://joannabriggs.org/sites/default/files/2019-05/JBI_Critical_Appraisal-Checklist_for_Cohort_Studies2017_0.pdf
13. Proper KI, Singh AS, van Mechelen W, Chinapaw MJM. Sedentary behaviors and health outcomes among adults: a systematic review of prospective studies. Am J Prev Med. (2011) 40:174–82. doi: 10.1016/j.amepre.2010.10.015
14. Hsu Y-C, Chang Y-C, Lin Y-C, Sze C-I, Huang C-C, Ho C-J. Cerebral microvascular damage occurs early after hypoxia-ischemia via nNOS activation in the neonatal brain. J Cereb Blood Flow Metab Off J Int Soc Cereb Blood Flow Metab. (2014) 34:668–76. doi: 10.1038/jcbfm.2013.244
15. Wilderman MJ, Armstead WM. Role of endothelial nitric oxide synthase in hypoxia-induced pial artery dilation. J Cereb Blood Flow Metab Off J Int Soc Cereb Blood Flow Metab. (1998) 18:531–8. doi: 10.1097/00004647-199805000-00008
16. Armstead WM. Contribution of kca channel activation to hypoxic cerebrovasodilation does not involve NO. Brain Res. (1998) 799:44–8. doi: 10.1016/S0006-8993(98)00462-4
17. Taniguchi H, Anacker C, Wang Q, Andreasson K. Protection by vascular prostaglandin E2 signaling in hypoxic-ischemic encephalopathy. Exp Neurol. (2014) 255:30–7. doi: 10.1016/j.expneurol.2014.02.012
18. Leffler CW, Busija DW. Prostanoids in cortical subarachnoid cerebrospinal fluid and pial arterial diameter in newborn pigs. Circ Res. (1985) 57:689–94. doi: 10.1161/01.RES.57.5.689
19. Jinnai W, Nakamura S, Koyano K, Yamato S, Wakabayashi T, Htun Y, et al. Relationship between prolonged neural suppression and cerebral hemodynamic dysfunction during hypothermia in asphyxiated piglets. Brain Dev. (2018) 40:649–61. doi: 10.1016/j.braindev.2018.04.010
20. Wu T-W, Tamrazi B, Soleymani S, Seri I, Noori S. Hemodynamic changes during rewarming phase of whole-body hypothermia therapy in neonates with hypoxic-ischemic encephalopathy. J Pediatr. (2018) 197:68–74.e2. doi: 10.1016/j.jpeds.2018.01.067
21. Buckley EM, Patel SD, Miller BF, Franceschini MA, Vannucci SJ. In vivo monitoring of cerebral hemodynamics in the immature rat: effects of hypoxia-ischemia and hypothermia. Dev Neurosci. (2015) 37:407–16. doi: 10.1159/000381704
22. Nakamura M, Jinnai W, Hamano S, Nakamura S, Koyano K, Chiba Y, et al. Cerebral blood volume measurement using near-infrared time-resolved spectroscopy and histopathological evaluation after hypoxic-ischemic insult in newborn piglets. Int J Dev Neurosci Off J Int Soc Dev Neurosci. (2015) 42:1–9. doi: 10.1016/j.ijdevneu.2015.02.009
23. Wang S-D, Liang S-Y, Liao X-H, Deng X-F, Chen Y-Y, Liao C-Y, et al. Different extent of hypoxic-ischemic brain damage in newborn rats: histopathology, hemodynamic, virtual touch tissue quantification and neurobehavioral observation. Int J Clin Exp Pathol. (2015) 8:12177–87.
24. Chakkarapani E, Dingley J, Aquilina K, Osredkar D, Liu X, Thoresen M. Effects of xenon and hypothermia on cerebrovascular pressure reactivity in newborn global hypoxic-ischemic pig model. J Cereb Blood Flow Metab Off J Int Soc Cereb Blood Flow Metab. (2013) 33:1752–60. doi: 10.1038/jcbfm.2013.123
25. Manole MD, Foley LM, Hitchens TK, Kochanek PM, Hickey RW, Bayir H, et al. Magnetic resonance imaging assessment of regional cerebral blood flow after asphyxial cardiac arrest in immature rats. J Cereb Blood Flow Metab Off J Int Soc Cereb Blood Flow Metab. (2009) 29:197–205. doi: 10.1038/jcbfm.2008.112
26. Leffler CW, Busija DW, Mirro R, Armstead WM, Beasley DG. Effects of ischemia on brain blood flow and oxygen consumption of newborn pigs. Am J Physiol. (1989) 257(6 Pt 2):H1917–26. doi: 10.1152/ajpheart.1989.257.6.H1917
27. Rosenberg AA. Regulation of cerebral blood flow after asphyxia in neonatal lambs. Stroke. (1988) 19:239–44. doi: 10.1161/01.STR.19.2.239
28. Rosenberg AA. Cerebral blood flow and O2 metabolism after asphyxia in neonatal lambs. Pediatr Res. (1986) 20:778–82. doi: 10.1203/00006450-198608000-00016
29. Shaikh H, Lechpammer M, Jensen FE, Warfield SK, Hansen AH, Kosaras B, et al. Increased brain perfusion persists over the first month of life in term asphyxiated newborns treated with hypothermia: does it reflect activated angiogenesis? Transl Stroke Res. (2015) 6:224–33. doi: 10.1007/s12975-015-0387-9
30. Domoki F, Zolei-Szenasi D, Olah O, Toth-Szuki V, Nemeth J, Hopp B, et al. Comparison of cerebrocortical microvascular effects of different hypoxic-ischemic insults in piglets: a laser-speckle imaging study. J Physiol Pharmacol an Off J Polish Physiol Soc. (2014) 65:551–8.
31. Dang Y-X, Shi K-N, Wang X-M. Early changes in glutamate metabolism and perfusion in basal ganglia following hypoxia-ischemia in neonatal piglets: a multi-sequence 3.0T MR study. Front Physiol. (2017) 8:237. doi: 10.3389/fphys.2017.00237
32. Armstead WM, Riley J, Cines DB, Higazi AA-R. PAI-1-derived peptide EEIIMD prevents hypoxia/ischemia-induced aggravation of endothelin- and thromboxane-induced cerebrovasoconstriction. Neurocrit Care. (2014) 20:111–8. doi: 10.1007/s12028-013-9906-2
33. Kiessling JW, Cines DB, Higazi AA-R, Armstead WM. Inhibition of integrin alphavbeta3 prevents urokinase plasminogen activator-mediated impairment of cerebrovasodilation after cerebral hypoxia/ischemia. Am J Physiol Heart Circ Physiol. (2009) 296:H862–7. doi: 10.1152/ajpheart.01141.2008
34. Armstead WM, Cines DB, Bdeir K, Kulikovskaya I, Stein SC, Higazi AA-R. uPA impairs cerebrovasodilation after hypoxia/ischemia through LRP and ERK MAPK. Brain Res. (2008) 1231:121–31. doi: 10.1016/j.brainres.2008.06.115
35. Bari F, Nagy K, Guidetti P, Schwarcz R, Busija DW, Domoki F. Kynurenic acid attenuates NMDA-induced pial arteriolar dilation in newborn pigs. Brain Res. (2006) 1069:39–46. doi: 10.1016/j.brainres.2005.11.033
36. Philip S, Armstead WM. Newborn pig nociceptin/orphanin FQ activates protein tyrosine kinase and mitogen activated protein kinase to impair NMDA cerebrovasodilation after ischemia. Neuroreport. (2003) 14:201–3. doi: 10.1097/00001756-200302100-00008
37. Jagolino AL, Armstead WM. PTK, MAPK, and NOC/oFQ impair hypercapnic cerebrovasodilation after hypoxia/ischemia. Am J Physiol Heart Circ Physiol. (2003) 284:H101–7. doi: 10.1152/ajpheart.00457.2002
38. Perciaccante JV, Domoki F, Puskar M, Busija DW. Effects of hypothermia on neuronal-vascular function after cerebral ischemia in piglets. Am J Physiol Regul Integr Comp Physiol. (2002) 283:R1362–7. doi: 10.1152/ajpregu.00134.2002
39. Armstead WM. NOC/oFQ and NMDA contribute to piglet hypoxic ischemic hypotensive cerebrovasodilation impairment. Pediatr Res. (2002) 51:586–91. doi: 10.1203/00006450-200205000-00007
40. Taylor GA, Trescher WA, Traystman RJ, Johnston M V. Acute experimental neuronal injury in the newborn lamb: US characterization and demonstration of hemodynamic effects. Pediatr Radiol. (1993) 23:268–75. doi: 10.1007/BF02010913
41. Domoki F, Perciaccante J V, Shimizu K, Puskar M, Busija DW, Bari F. N-methyl-D-aspartate-induced vasodilation is mediated by endothelium-independent nitric oxide release in piglets. Am J Physiol Heart Circ Physiol. (2002) 282:H1404–9. doi: 10.1152/ajpheart.00523.2001
42. Dorrepaal CA, Steendijk P, Baan J, van Bel F. Inhibition of nitric oxide synthesis following severe hypoxia-ischemia restores autoregulation of cerebral blood flow in newborn lambs. Early Hum Dev. (2001) 60:159–70. doi: 10.1016/S0378-3782(00)00104-3
43. Pourcyrous M, Leffler C, Busija D. Role of prostanoids in cerebrovascular responses to asphyxia and reventilation in newborn pigs. Am J Physiol. (1990) 259(3 Pt 2):H662–7. doi: 10.1152/ajpheart.1990.259.3.H662
44. Parfenova H, Pourcyrous M, Fedinec AL, Liu J, Basuroy S, Leffler CW. Astrocyte-produced carbon monoxide and the carbon monoxide donor CORM-A1 protect against cerebrovascular dysfunction caused by prolonged neonatal asphyxia. Am J Physiol Heart Circ Physiol. (2018) 315:H978–88. doi: 10.1152/ajpheart.00140.2018
45. Wilderman MJ, Armstead WM. Role of PACAP in the relationship between cAMP and opioids in hypoxia-induced pial artery vasodilation. Am J Physiol. (1997) 272(3 Pt 2):H1350–8. doi: 10.1152/ajpheart.1997.272.3.H1350
46. Leffler CW, Beasley DG, Busija DW. Cerebral ischemia alters cerebral microvascular reactivity in newborn pigs. Am J Physiol. (1989) 257(1 Pt 2):H266–71. doi: 10.1152/ajpheart.1989.257.1.H266
47. Rosenberg AA, Murdaugh E, White CW. The role of oxygen free radicals in postasphyxia cerebral hypoperfusion in newborn lambs. Pediatr Res. (1989) 26:215–9. doi: 10.1203/00006450-198909000-00012
48. Salas J, Tekes A, Hwang M, Northington FJ, Huisman TAGM. Head ultrasound in neonatal hypoxic-ischemic injury and its mimickers for clinicians: a review of the patterns of injury and the evolution of findings over time. Neonatology. (2018) 114:185–97. doi: 10.1159/000487913
Keywords: cerebral hyperperfusion, cerebral vasodilation, hyperemia, hypoxic-ischemic encephalopathy (HIE), neonatal encephalopathy, perinatal hypoxia-ischemia
Citation: Kleuskens DG, Gonçalves Costa F, Annink KV, van den Hoogen A, Alderliesten T, Groenendaal F, Benders MJN and Dudink J (2021) Pathophysiology of Cerebral Hyperperfusion in Term Neonates With Hypoxic-Ischemic Encephalopathy: A Systematic Review for Future Research. Front. Pediatr. 9:631258. doi: 10.3389/fped.2021.631258
Received: 19 November 2020; Accepted: 07 January 2021;
Published: 02 February 2021.
Edited by:
Francisco J. Alvarez, Hospital de Cruces, SpainReviewed by:
Raul Chavez-Valdez, Johns Hopkins Medicine, United StatesBritt Nakstad, University of Oslo, Norway
Copyright © 2021 Kleuskens, Gonçalves Costa, Annink, van den Hoogen, Alderliesten, Groenendaal, Benders and Dudink. This is an open-access article distributed under the terms of the Creative Commons Attribution License (CC BY). The use, distribution or reproduction in other forums is permitted, provided the original author(s) and the copyright owner(s) are credited and that the original publication in this journal is cited, in accordance with accepted academic practice. No use, distribution or reproduction is permitted which does not comply with these terms.
*Correspondence: Jeroen Dudink, ai5kdWRpbmtAdW1jdXRyZWNodC5ubA==