- 1Fondazione IRCCS Ca' Granda Ospedale Maggiore Policlinico, NICU, Milan, Italy
- 2Department of Clinical Sciences and Community Health, Università degli Studi di Milano, Milan, Italy
- 3Department of Pediatrics—ICU, General University Hospital, 1st Faculty of Medicine Charles University, Prague, Czechia
- 4Department of Pharmacology, General University Hospital, 1st Faculty of Medicine Charles University, Prague, Czechia
- 5Intensive Care and Department of Pediatric Surgery, Erasmus MC-Sophia Children's Hospital, Rotterdam, Netherlands
- 6Division of Neonatology, Department of Pediatrics, Erasmus MC Sophia Children's Hospital, University Medical Center Rotterdam, Rotterdam, Netherlands
- 7Department of Development and Regeneration, KU Leuven, Leuven, Belgium
Extracorporeal membrane oxygenation (ECMO) is a lifesaving support technology for potentially reversible neonatal cardiac and/or respiratory failure. As the survival and the overall outcome of patients rely on the treatment and reversal of the underlying disease, effective and preferentially evidence-based pharmacotherapy is crucial to target recovery. Currently limited data exist to support the clinicians in their every-day intensive care prescribing practice with the contemporary ECMO technology. Indeed, drug dosing to optimize pharmacotherapy during neonatal ECMO is a major challenge. The impact of the maturational changes of the organ function on both pharmacokinetics (PK) and pharmacodynamics (PD) has been widely established over the last decades. Next to the developmental pharmacology, additional non-maturational factors have been recognized as key-determinants of PK/PD variability. The dynamically changing state of critical illness during the ECMO course impairs the achievement of optimal drug exposure, as a result of single or multi-organ failure, capillary leak, altered protein binding, and sometimes a hyperdynamic state, with a variable effect on both the volume of distribution (Vd) and the clearance (Cl) of drugs. Extracorporeal membrane oxygenation introduces further PK/PD perturbation due to drug sequestration and hemodilution, thus increasing the Vd and clearance (sequestration). Drug disposition depends on the characteristics of the compounds (hydrophilic vs. lipophilic, protein binding), patients (age, comorbidities, surgery, co-medications, genetic variations), and circuits (roller vs. centrifugal-based systems; silicone vs. hollow-fiber oxygenators; renal replacement therapy). Based on the potential combination of the above-mentioned drug PK/PD determinants, an integrated approach in clinical drug prescription is pivotal to limit the risks of over- and under-dosing. The understanding of the dose-exposure-response relationship in critically-ill neonates on ECMO will enable the optimization of dosing strategies to ensure safety and efficacy for the individual patient. Next to in vitro and clinical PK data collection, physiologically-based pharmacokinetic modeling (PBPK) are emerging as alternative approaches to provide bedside dosing guidance. This article provides an overview of the available evidence in the field of neonatal pharmacology during ECMO. We will identify the main determinants of altered PK and PD, elaborate on evidence-based recommendations on pharmacotherapy and highlight areas for further research.
Background
Extracorporeal membrane oxygenation (ECMO) is an established life-saving support technique for critically-ill neonates with severe cardio-respiratory failure (1, 2). Being a bridge, ECMO buys the time for cure, in part related to drugs to treat and possibly reverse the underlying disease while protecting the failing respiratory/circulatory systems from iatrogenic negative effects with long term consequences (2). Indeed, sustained and maximal mechanical ventilation may lead to hemodynamic compromise and ventilation-induced lung injury, as a result of oxygen toxicity, baro- bio-, and volu-trauma (3). Generally, these critically-ill neonates are exposed to polypharmacy, as they require anticoagulants to maintain the hemostatic balance within the ECMO circuit, analgo-sedatives to ensure patient comfort, cardiovascular agents to sustain hemodynamics, anti-infectives to prevent or treat infections, and possibly other drugs to manage underlying specific conditions or complications (4, 5).
As in many of these patients the survival and overall outcome rely on medications, effective pharmacotherapy is essential to improve care and minimize side effects (5). Adequate drug dosing is based on the understanding of two concepts: (1) pharmacokinetics (PK), which explores “what the body does to the drug” and provides the drug concentration-time profile, through the evaluation of absorption, distribution, metabolism, and excretion (ADME); (2) pharmacodynamics, which represents “what the drug does to the body” and estimates action and side-effects of a given medication, based on dose and patient profile (6, 7). The main drivers of drug PK are volume of distribution (Vd), which describes the dose required to produce the desired peak concentration and clearance (Cl), which is the volume of fluid cleared of drug from the body per unit of time. Both Vd and Cl are primary determinants of drug half-life (7). Safe and efficient prescription in neonatal ECMO depends upon the knowledge of the above-mentioned concepts and the understanding of the determinants affecting drug PK and PD in the complex context of patient immaturity, critical illness, (multi)organ failure and need for supportive extracorporeal circuits (8).
Neonatal age is by itself a window of pharmacological vulnerability (9). Drug PK and PD prediction, based on time-dependent maturational changes (age, weight) is the cornerstone of developmental pharmacology (10, 11). Additionally, critical illness may contribute to impaired drug exposure, as a result of multiple organ failure and changes in physiology, such as hyperdynamic state, increased vascular permeability, catabolism, and altered protein binding (8, 12). The need for ECMO further complicates the issue, through the sequestration of drugs into the circuit and the induction of PK specific variability (4, 13, 14).
Although physicochemical properties can be used to predict the drugs' bioavailability while on ECMO (15), the pharmacotherapy in this setting remains too empirical, as a result of limited evidence due to the lack of clinical studies and ever-evolving technology.
Because of this, treating a critically-ill neonate on ECMO is challenging and requires an integrated approach, to limit the risks of under treatment or toxicity. In this review, we will discuss current knowledge of ECMO-induced PK perturbations, and subsequently discuss the relevance of these PK findings for analgo-sedatives and antimicrobial and antiviral drugs, to end with a discussion on approaches to further optimize neonatal pharmacotherapy. However, pharmacotherapy for neonates on ECMO still needs to be integrated with the physiological maturation occurring in early infancy.
The Role of Developmental Pharmacology on Drug Disposition
In neonates the evolving physiological maturation has a dynamic impact on clinical pharmacology, thus resulting in inter- and intra- individual variability in drug exposure (PK) and drug effect (PD) (9). Growth, weight, body and plasma protein composition, organ maturation, and energy requirements are the main determinants of the developmental pharmacology, which integrates the knowledge of the ontogenetic changes to deliver safe and effective pharmacological treatment across the pediatric age range (10, 16). While maturational PK considers the age-related changes of the ADME process (17, 18), the maturational PD takes into account the developmental variability of specific organ function and receptor expression (11). An extensive and contemporary description of the maturational covariates of the developmental pharmacology is beyond the scope of this review and it is available elsewhere (10, 11, 19).
The Role of Non-maturational Determinants on Drug Disposition: Focus on Pre-ECMO Disease State
To objectivate non-maturational determinants and their impact on drug disposition in critically ill neonates is essential to integrate the concept of “precision dosing to optimize neonatal pharmacotherapy” defined as “personalized, individualized, tailored or precise pharmacotherapy” (20). Moreover, accuracy of drug formulations, drug prescription and new drug development is needed to tune pharmacotherapy in the vulnerable neonatal population (21). Non-maturational determinants such as (perinatal) asphyxia/hypoxia, sepsis/systemic inflammatory response syndrome (SIRS), multiple organ dysfunction syndrome (MODS) are considered as clinically relevant variables of drug disposition (22, 23). However, they are not well understood in critically ill neonates due to dynamically changing conditions in the single patient. There is a large inter individual variability in the PK/PD of frequently used medications (antimicrobials, analgosedatives, anti-convulsives, vasopressors, and inotropes) in neonates under critical illness (24) and ECMO (25, 26). These covariates are either predictable (i.e., related to development or drug = maturational determinants), partly predictable (i.e., related to treatment modality), or almost non-predictable (i.e., related to disease = non-maturational covariates). Changes in the Vd and Cl of drugs under critically ill conditions may lead to a high intra- and inter-individual PK variability (for different drugs 30–70%) resulting in either insufficient or toxic plasma concentrations of drugs (27). This may have an impact on the drug disposition and, as a consequence, both under- and over-dosing may contribute to unfavorable outcomes.
Perinatal Asphyxia—Hypoxia
Perinatal Asphyxia (PA) is defined by the American Academy of Pediatrics (AAP) and the American College of Obstetricians and Gynecologists (ACOG) as a condition of severely deficient supply of oxygen to the body (oxygen deprivation) leading to coma or death (28). In 2009, based on international guidelines, therapeutic hypothermia (HT; 33–34°C) has been recommended to be used for therapy in asphyxiated (moderate to severe) neonates (29). However, the decision to place on ECMO newborns treated for perinatal asphyxia and hypoxic ischemic encephalopathy (HIE) is based on criteria of HIE severity (30). Following perinatal asphyxia, neonates may suffer from HIE (69.4%), respiratory or acute kidney failure (AKI 47–61%), cardiac and hepatic dysfunction, whose rates in the era of HT (31) are similar to the pre-cooling period (32). Multiple organ dysfunction syndrome (MODS), defined as the presence of at least one organ dysfunction in addition to HIE, occurred in 58–88% of asphyxiated neonates (33) and contributed to higher mortality rates (20.5–72.9%) (31). MODS may complicate the course of neonatal ECMO, with a negative impact on survival (34, 35). Moreover, after out of hospital pediatric cardiac arrest, AKI is very common (64% of the enrolled cases, n = 282), and severe (41% of the enrolled cases), without difference in incidence in severe AKI between cases that either or not underwent HT (36). As a rule of thumb, asphyxia may lead to changes in drug disposition such as decreased or variable drug absorption (AUC, Ka, tmax or F), increased (or unchanged) drug distribution (Vd) and decreased drug elimination (CL) (37). However, data on PK changes under asphyxia in neonates are sparse (ceftazidime, amikacin, gentamicin, amoxicillin, and benzylpenicillin) (37–41) and the same holds true for cardiac arrest in neonates and changes in pH (42). Moreover, PK variability in asphyxiated neonates has been reviewed in relation to the impact of HT alone (43–46) or in combination to ECMO (47). Recently, for anticonvulsive drugs such as phenobarbital, which has low hepatic Cl and low protein bound drug HT was not found to be a PK covariate (48, 49), in contrast to birth weight (BW), postnatal age (PNA) (50), and disease severity (51). The disposition of other drugs during neonatal HT has been evaluated in the recent literature (52–56) and the relevant findings are summarized in Table 1.
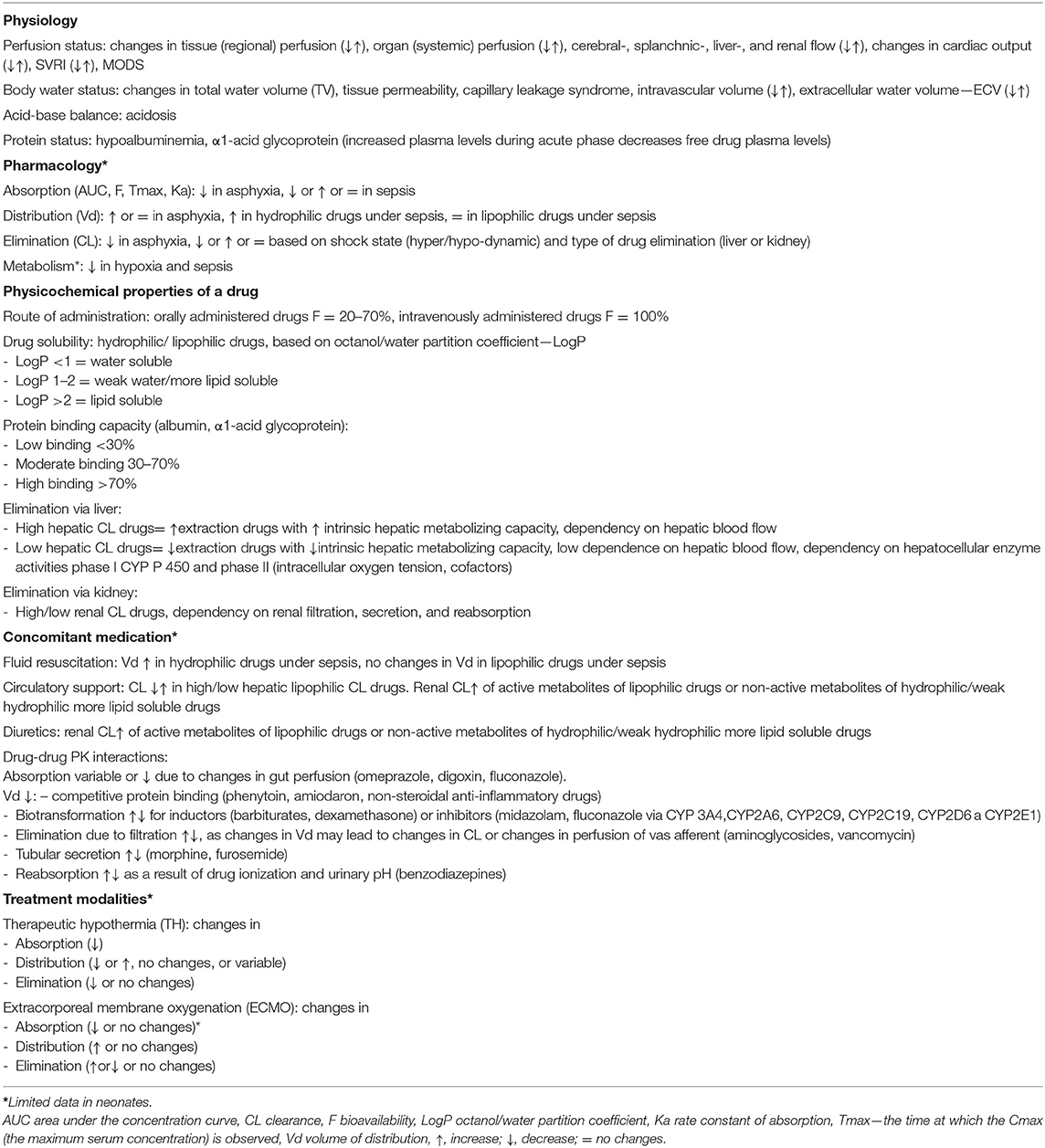
Table 1. Pre-ECMO non-maturational determinants of drug disposition and pharmacology considerations.
Sepsis/Systemic Inflammatory Response Syndrome
There is lack of consensus for the definition of sepsis in neonates (57). So far, the international consensus on pediatric sepsis and SIRS, respectively, was established to address this issue for all children (<18 years old) including term neonates (≥37 weeks completed gestation) in 2005 (58). (59) showed how mortality for MODS, in a pediatric intensive care unit, was significantly higher among term neonates compared with older children (75.4 vs. 50.9%) (59). During sepsis relevant SIRS-related physiological changes occur, which contribute to drug disposition (60). The main physiology and pharmacology considerations in sepsis/septic shock are shown in Table 1. Sepsis, and its related factors like tissue (regional) hypoperfusion, MODS (systemic) hypoperfusion, acidosis, hypoalbuminemia, SIRS, type of shock (hyperdynamic/hypodynamic), capillary leakage syndrome, or pharmacotherapy (diuretics, vasopressors, inotropic drugs) may lead to changes in PK, and therefore PD parameters (Cmax, Cmin, AUC0-24/MIC of concentration, and time dependent antibiotics, T > MIC of time-dependent antibiotics, Cmax/MIC of concentration dependent antibiotics). Moreover, sepsis and SIRS may induce a supraphysiologic renal activity, defined as augmented renal clearance (ARC) with enhanced renal pre-load and glomerular hyperfiltration (61). ARC is an established physiological response to hyperdynamic cardiovascular states in adult (61–63) and pediatric critical care patients (64, 65). However, in the neonatal period ARC has not yet been reported. In case of reduced renal functional reserve, secondary to a previously impaired kidney function or worsening organ perfusion, drug clearance may be compromised (64, 66). In children, sepsis has major impact on cytochrome P450 (CYP)3A activity (−90%), as has been illustrated with midazolam as probe drug (67). Such observations should be considered while prescribing drugs for critically ill neonates on ECMO. The interaction between the extracorporeal circuit itself with pre-ECMO disease states needs to be further characterized (68–70).
The Role of Non-maturational Determinants on Drug Disposition: Focus on ECMO
Extracorporeal membrane oxygenation interferes with the expected attainment of a drug's therapeutic level (71). In the last decades, preclinical and clinical research have provided preliminary evidence of the causative mechanisms for reduced drugs' bioavailability. Pending specific PK studies, the loading dose (LD) is usually based on Vd, while the maintenance dose (MD) is driven by the estimated Cl (72). Moreover, PK changes are strictly dependent on equipment material and circuit design (73). Most data come from ex vivo studies on silicone-based oxygenators. Technological advances have added further variability, through the introduction of ever-smaller circuits, new biocompatible coatings and poly-methyl-pentene(PMP) membrane oxygenators (74). Currently, we lack the knowledge of the interaction of contemporary neonatal ECMO circuits and pharmacotherapy. Hereby we summarize the available evidence, stemming from in vitro and in vivo studies.
Circuit-Drug Interaction
The modern neonatal circuitry includes cannulas (venous cannula for drainage and arterial cannula for reinfusion or a single double-lumen cannula, when allowed by patients' size), polyvinyl chloride conduit tubing, a centrifugal pump, and PMP hollow-fiber membrane oxygenator (75). Based on patients' conditions, an hemofilter or a continuous renal replacement therapy may be added to the circuit design (75).
Both size and material of each of the above-mentioned components may lead to significant PK changes as a result of three main mechanisms: (i) sequestration into the circuit; (ii) increased Vd; and (iii) altered Cl.
Drugs' Sequestration by the ECMO Circuit: Components and Materials
Significant extraction of medications occurs in off-patient ECMO systems as a result of a complex interaction among circuit components and specific physiochemical properties of drugs, notably molecular weight, ionization, hydrophilicity, and protein binding (13, 15). The octanol-water partition coefficient (LogP) is a measure of a drug's lipophilicity (76). The higher the LogP (>2), the higher the drug sequestration (13, 15, 77). Similarly, highly protein bound drugs are more prone to be adsorbed into the ECMO systems (14). These ex vivo findings were confirmed by in vivo ovine ECMO models (77).
Equipment matters, as different materials of oxygenators, tubing, coating, and pumps may have a variable impact on drug disposition (15, 78–81).
Pediatric membrane oxygenator technology underwent significant advancements over the last decades (82). Improvement of materials, surface area and priming volume may support pharmacotherapy. Indeed, the variability of drug adsorption by different membrane oxygenators has been acknowledged since the early 90's (71, 83). Lipophilic drugs were largely sequestered into silicone membranes, at variance with the polypropylene ones (15, 71, 83). Similarly, the last-generation polymethylpentene hollow fiber oxygenators have shown less drug adsorption when compared to silicone-based membranes, especially for the lipophilic drugs in the first hours after injection in off-patients experiments (84).
Polyvinylchloride (PVC) tubing was found to be the primary site for drug sequestration (85). According to in vitro data, fentanyl was lost to the PVC tubing by 80% after 120 min, with an additional 5% lost to the oxygenators (85). Polymethylpentene-based oxygenator had a slightly higher impact on fentanyl disposition, if compared to the microporous polypropylene-based one (85). In the same study, morphine was lost to the PVC tubing by 40% after 5 min, with almost no further adsorption by the oxygenators (85). In contrast, recombinant human albumin/heparin coating tubing showed no effect on disposition of hydrophilic drugs, such as cephalosporine and carbapenems (86). These findings were further supported in a more recent ex vivo study, which evaluated beta-lactams in ECMO circuits made up of polymethylpentene membrane, centrifugal pump, heat exchanger, and PVC tubing (87). Results confirmed that beta-lactams (except for ceftriaxone) were not sequestered into the circuit (87).
Although the impact of coating has been neglected for years, more recent in vitro studies provided evidence that surface modification may affect drug disposition to some extent (88). Coating is meant to mimic the endothelial surface to enhance biocompatibility and it is generally defined as bioactive, when it is based on heparin and nitric oxide, or biopassive, if albumin and polymers such as phosphorylcholine are used (89). In vitro results from a study specifically designed to investigate the influence of coating on morphine and fentanyl disposition have shown that the following four types of coating were inert to drug absorption: synthetic albumin, heparin-free biopassive polymer, recombinant human albumin ± heparin, and covalently bonded heparin coatings. In contrast, two other types of surface modifications were associated at 5 min with a significant reduction of morphine levels: poly2methoxylacrylate polymer and covalently bonded heparin (88). No significant differences were reported for fentanyl concentrations (88). These findings further illustrate how drug disposition results from a complex chemical and molecular interaction between individual drugs and ECMO components' individual characteristics. Indeed, electrochemical properties, namely the electric charge and degree of hydrophilicity of surface coatings, may contribute to modulate drugs' sequestration (90).
Although the influence of the type of pump itself has not been defined, centrifugal pump-based circuits with hollow-fiber membrane oxygenators have shown the least absorption for all drugs (13, 15), and this phenomenon is most pronounced for lipophilic drugs (15). Besides chemical drivers, mechanics could be advocated to affect drugs' PK, as blood is constantly exposed to variable pressures and flow-rates over the extracorporeal run (79). ECMO blood-flow is thought to affect drugs' PK (91), nevertheless the specific impact of blood-flow variability has not yet been characterized.
In addition, the type of priming solution and temperature are involved in the complex chemical mechanisms of drug loss and stability during ECMO (92), as explained in the next paragraph. Circuit age further affects pharmacotherapy: on one hand the saturation of binding sites may smooth the tubing impact on PK; on the other hand, it is not clear if the circuit acts as a reservoir, by releasing drugs back into the patient with a potential risk of cumulative effect and late toxicity (13, 71, 93). Thus far, the in vitro circuit-drug interaction has been characterized over 24 h, no data are available beyond this time frame.
ECMO-Induced Volume of Distribution Increase
The connection of a neonate to the extracorporeal circuit will affect the apparent Vd of drugs, through three main mechanisms. Firstly, as previously mentioned, the direct drug adsorption into the circuit is the driving factor (71, 93). Secondly, the haemodilution from the priming solution has been advocated for ECMO-related PK variability (92). In neonates, the priming volume of contemporary circuits approximates 250–300 ml, which equals the circulatory volume of a 3 kg neonate. Furthermore, over the course of an ECMO run, the frequent administration of blood products and crystalloids contribute to worsen the hemodilution (94). Hydrophilic drugs are the most affected, as their Vd is limited to the extracellular compartment, with no intracellular drug reservoir available for retrograde diffusion (72). The extension of the plasma compartment during the ECMO start or in critical illness affects the LD, which is the first dose needed to guarantee the therapeutic concentration (72, 95). LD is directly proportional to the enlarged Vd and, hence, should be increased accordingly (95). The priming dilution, in conjunction with electrolytes and temperature perturbations, may affect also plasma proteins, especially albumin and alpha1-acid glycoprotein, thus altering the plasmatic drug-binding (14). Hypoalbuminemia is a multifactorial process, which results from ECMO- and disease-driven physio-pathologic changes (96). The increase of unbound or free drugs may expose ECMO neonates to potential toxicity (97). Lastly, the ECMO-related physiologic changes and the underlying disease state influence Vd, as a result of the systemic inflammatory response (98–102).
ECMO-Induced Clearance Variability
Drug clearance relies on kidney and liver function, which are usually altered on ECMO, as a result of the clinical status and circuit-related factors (73, 94, 103). In the early phase of extracorporeal circulation, the SIRS releases inflammatory mediators and endogenous cytokines, thus leading to vasodilatation, increased cardiac output and renal perfusion (73, 94). In veno-arterial ECMO, non-pulsatile blood flow is associated with a reduction of the glomerular filtration rate (104). Moreover, the inflammatory state of the critically-ill is associated with the downregulation of the expression and activity of cytochrome P450 enzymes involved in the hepatic drug metabolism (67). Low clearance and consequent rise of drug levels might expose the patient to increased pharmacological effect and toxicity (67, 73).
Disposition of Analgo-Sedatives on Neonatal ECMO
During ECMO, neonates are exposed to multiple sedatives and analgesics, mostly for prolonged periods, to provide comfort, pain relief, and safety (105). The extracorporeal circuit has a large impact on sedatives and analgesics disposition, leading to high sedative needs (106–109). Drug physicochemical properties may assist in the dose prediction, which is titrated to clinical effect (5). Indeed, lipophilic agents, like fentanyl, propofol, and midazolam are highly sequestered into the circuit (15, 93, 110), especially in the first hours of bypass (84).
In the neonatal age, prolonged and sustained analgo-sedation is associated with clinical relevant adverse effects such as tolerance, dependency, impaired brain development, and iatrogenic withdrawal syndrome (105, 111). Among opioid-sparing strategies, the daily interruption of sedation and analgesia was shown to be feasible, safe, and effective (112). However, sedation targets differ among ECMO centers, ranging from deep to conscious sedation practice (5, 113). The use of alternative non-opioid agents should be preferred (73, 84). Morphine and paracetamol have a favorable PK profile (15, 84, 114), while preliminary data on α2-adrenergic agonists dexmedetomidine and clonidine suggest the need for increased dosing (81, 115).
In this section we will summarize current evidence of the disposition of sedatives and analgesics on contemporary neonatal ECMO circuits (Tables 2, 3) (15, 83, 84, 91, 93, 108, 110, 114, 115, 117–120, 134).
Benzodiazepines
Midazolam has been extensively studied in the neonatal ECMO population. Moderate sequestration into the circuit has been observed through in vitro experiments, based on both old (15, 93) and contemporary circuits (15, 84). Two PK studies are available in the neonatal ECMO population, with contrasting results. Although both described the increase of Vd, since the start of ECMO (108, 117), Mulla et al. found a constant Cl of midazolam in neonates on veno-venous ECMO, with a prolonged elimination half-life leading to drug accumulation after 48 h (108). In contrast, Ahsman et al. reported the increase of midazolam Cl over time in neonates on veno-arterial ECMO (117). These PK data suggest the need for an increased LD in the early phase (first 24–48 h) of extracorporeal support, following which dosage should be titrated down, given the risk of accumulation of midazolam and its metabolites (108, 117) and, consequently, prolonged sedation (135).
Opioids
Fentanyl is highly sequestered into the circuit (15, 92) and dose escalation is required in neonates and infants exposed to extracorporeal circuits (107, 136). Despite the technological improvements, the impact of contemporary hollow-fiber-based oxygenators remains high for lipophilic drugs, such as fentanyl and sufentanil (84). Most centers use morphine as analgesic and sedative during neonatal ECMO, because its PK profile is not significantly altered. Clinical PK studies have reported a two-fold increase of morphine Vd (91). The Cl decreased following ECMO cannulation (119, 134) but increased over time, in relation to creatinine clearance, reflecting age-related maturation of drug excretion (91). Moreover, when compared to fentanyl, morphine continuous infusions were associated with improved analgesia, reduced drug withdrawal and length of stay (137). Therefore, morphine remains the opioid of choice for neonatal ECMO. Dose adjustments need to be titrated to clinical sedo-analgesic targets, pending evidence on contemporary circuitry-related PK.
Non-opioid Analgesics
Based on preliminary in vitro studies, paracetamol has been suggested as a promising analgesic during neonatal ECMO (84, 114). However, clinical PK evaluations are needed to provide dosing recommendations.
Propofol
This highly lipophilic and protein-bound sedative-hypnotic agent is largely sequestered into the ECMO circuit (93). The drug-related toxicity and concerns for propofol infusion syndrome (PRIS) (138) call for caution in the prolonged use of this drug during neonatal ECMO.
α2-Adrenergic Agonists
Clonidine use and prescription during neonatal and pediatric ECMO is supported by a recent population PK study, which suggested higher clonidine doses, based on the increase of Vd and Cl in the specific setting of ECMO and renal replacement therapy (115). Limited in vitro data are available for dexmedetomidine, which is partially sequestered into the circuit: a LD may be required, although recommendations for its long-term use cannot be provided (81).
Disposition of Antimicrobial and Antiviral Drugs During Neonatal ECMO
Infection remains a real threat for critically-ill neonates on ECMO, with an incidence rate of 5.4 and 5.7% in respiratory and cardiac runs, and reduced survival to 51 and 19%, respectively (34). A timely and adequate antimicrobial therapy is therefore pivotal to improve outcomes (139). However, the goal to provide optimal antibiotic therapy is impaired by the ECMO-induced PK changes, which can be only partially predicted, based on current knowledge on drug-circuit-patient interaction (Figure 1) (13, 15, 103). Moreover, antimicrobial prescribing is further complicated by the lack of clinical titratable endpoints (103). Therefore, PK and PD remain the best available predictors of antimicrobial efficacy. Pending evidence-based pharmacotherapy guidelines, neonates on ECMO are still at risk of sub-optimal antibiotic exposure, contributing to treatment failure and bacterial resistance (26). In this section we will summarize current evidence of antimicrobial bioavailability on contemporary neonatal ECMO circuits (Tables 2–4) (4, 15, 92, 116, 118, 121–133).
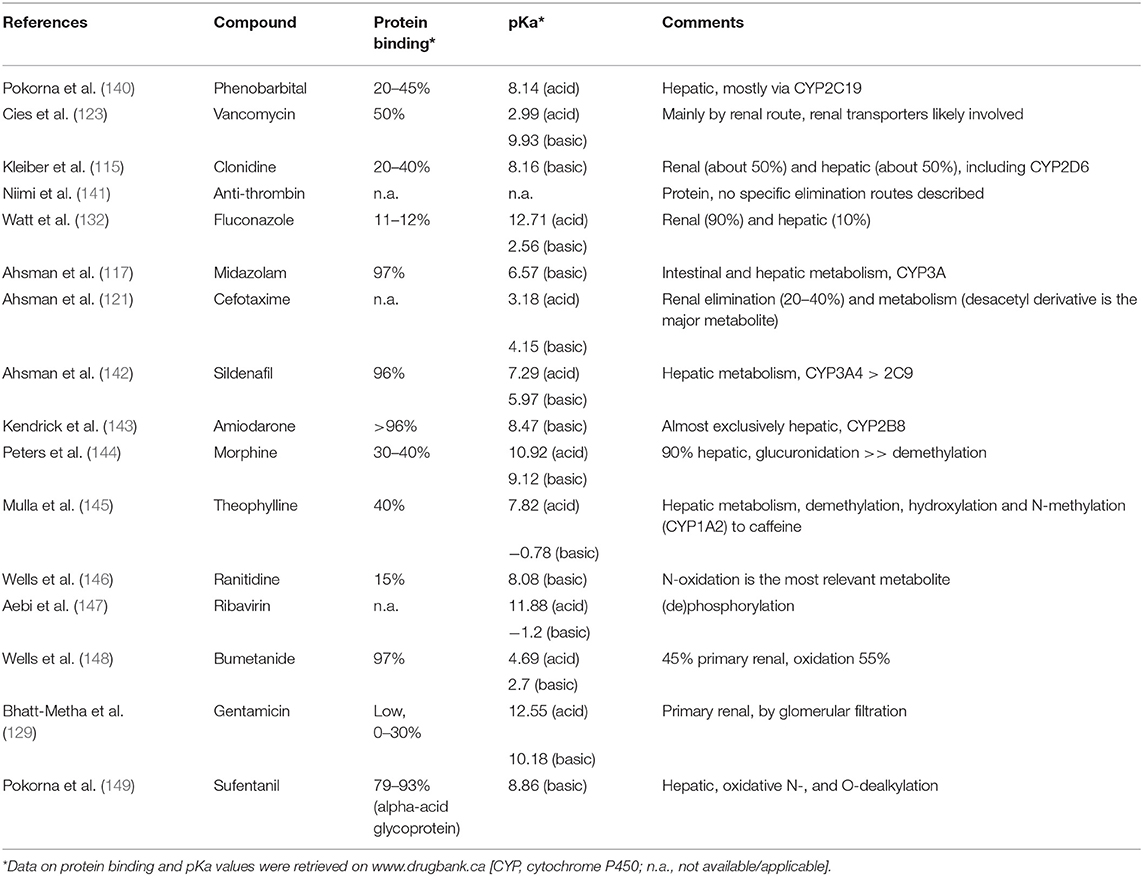
Table 4. Overview of the Pubmed (ecmo, newborn, pharmacokinetics, n = 72) search on pharmacokinetics of 16 different compounds.
Beta-Lactams
Beta-lactams are hydrophilic time-dependent antimicrobials, with a variable degree of protein binding and renal elimination (150). Their killing activity is strictly related to the time the unbound drug is above the minimum inhibitory concentration (MIC). The knowledge of ECMO-related PK changes is limited in novel circuitry and further complicated by the well-known instability (i.e., temperature) of this class of antibiotics (151). Based on in vitro observations, ampicillin showed a moderate loss in older silicon-based neonatal systems (92). The impact of contemporary circuits on cefotaxime seems negligible (15).
Although the Vd of cefotaxime was increased during ECMO, Cl was comparable to the one of non-ECMO neonates (121). Based on a neonatal PK study, standard dosing regimen of cefotaxime during ECMO provided supra-MIC plasma levels (121). Therefore, given the large therapeutic window of cefotaxime, dose adjustments are usually not needed.
Broad-spectrum carbapenem agents, such as meropenem, may be required over the neonatal ECMO course (139). The impact of the ECMO circuit on drug disposition consists of a moderate drug sequestration (15), larger Vd, and higher clearance (152). The latter factor is magnified when renal replacement therapy is added to the circuit design (122).
Glycopeptides
Vancomycin is a hydrophilic time-dependent antimicrobial, largely used in the NICUs for treatment of Gram-positive infections (118, 153). Given the narrow therapeutic window and the risk of nephrotoxicity, the PK profile of vancomycin has been extensively evaluated both in vitro and in vivo neonatal settings since the 90's (154–156). Vancomycin Cl is strictly related to renal function (155, 157) and the drug half-life was found to be prolonged in ECMO patients (156). However, these findings referred to older roller pump-based systems. Although data on contemporary circuits are limited, recent neonatal PK studies have revealed enhanced Cl, potentially leading to under-exposure (123). An empiric dosing strategy of 25–30 mg/kg/dose every 12–24 h is suggested, with a close therapeutic drug monitoring (TDM) (125).
Continuous vancomycin infusions were found to be associated with earlier and improved attainment of target concentrations compared to the intermittent modality in neonates, with no difference in terms of adverse effects (158). However, no evidence is available for the optimal infusion modality during ECMO.
Another glycopeptide antimicrobial which may be used during neonatal ECMO is teicoplanin. Although specific neonatal data of teicoplanin disposition in the extracorporeal setting are lacking, the evidence from an adult PK study suggests the need for higher doses during ECMO (159). In this prospective population PK evaluation, the predictive target attainment was reduced during ECMO for every simulated dosing, despite the Vd was lower and Cl was not affected by the extracorporeal circuit (159). Based on the hydrophilic profile of the drug, the hemodilution and protein binding could be addressed as the main drivers for teicoplanin disposition on ECMO (159).
Aminoglycosides
Gentamicin is a hydrophilic antimicrobial with a relatively low protein binding, largely used in the NICUs for the treatment of infections due to Gram-negative bacteria (118, 153). During ECMO, gentamicin has been found to have an increased Vd, as a result of the large exogenous blood volume for circuit priming and decreased Cl, leading to a prolonged elimination half-life (4, 126, 128). The renal dysfunction, which is a common multifactorial condition during ECMO, may be considered as the main determinant of the prolonged elimination half-life of gentamicin (72). Given the concentration-dependent antimicrobial activity of aminoglycosides, it is highly recommended to perform TDM to ensure adequate antimicrobial exposure.
Antivirals
Oseltamivir is a neuraminidase inhibitor of both type A and B influenza virus (160). This drug is approved by the Food and Drug Administration (FDA) for the treatment of children older than 2 weeks of age with flu (130, 161). Oseltamivir is an oral pro-drug which is rapidly converted to oseltamivir carboxylate, the active metabolite (150, 160). Based on previous pediatric PK ECMO case series, the impact of ECMO on oseltamivir disposition is negligible with no need for dosing adjustment (131). However, oral bioavailability was reported to decrease in patients with impaired gastric motility and enteral absorption (131). Although evidence in the neonatal setting is scant, adult data support the lack of effect of ECMO on the oseltamivir's PK (162, 163).
From Fragmented Data to Integrated Knowledge
Obviously, also in neonates and children on ECMO, pharmacotherapy is a very important tool in the medical management. As a result of the large PK-PD variability, drug dosing is only to a very limited extent validated in the setting of neonatal ECMO (164). Methodological development within the field of clinical pharmacology and modeling should assist ECMO physicians to improve our practices. The other way around, modelers will need the data to get this job done.
A knowledge-driven improvement strategy necessitates sufficient understanding of human developmental biology to subsequently translate such knowledge into prediction differences in drug absorption, distribution, metabolism, and excretion (PK). Only once this PK is sufficiently well covered, an appreciation of the developmental aspects of drug-receptor or -target interactions (PD) can be considered. Physiologically-based PK (PBPK) modeling is such a structured approach to translate knowledge into prediction, but the development of such modeling techniques necessitates the collaboration of clinicians with researchers specifically skilled in modeling techniques (165). PBPK approaches provide a potent systematic way to make the most of already acquired knowledge (physiology, system knowledge) to adapt drug dosing to the needs of children on ECMO, as has recently been illustrated for fluconazole (166).
The aim is not to describe the workflow and technical details related to the development of pediatric or neonatal PBPK model (167, 168), but to illustrate how ECMO physicians and clinical researchers can contribute to improved ECMO-related pharmacotherapy in neonates and children by generating data on ECMO related (patho)-physiology, including aspects related to the initial indication to initiate ECMO, and by sharing PK datasets and data on neonatal and pediatric equipment.
PBPK Methodology
In essence, PBPK is a structured method for data integration, hypothesis testing and knowledge generation (167, 168). Moreover, one may check consistency of data obtained from different sources (in vitro, in vivo, in silico) or predict outcome (PK, PD) of future experiments, hereby enabling decision making or optimization of study design. PBPK (“so-called bottom-up”) applies mathematical models for mechanistic integration of pharmacology principles, assumptions, and data along the drug development process. It hereby integrates different types of information, such as clinical data and in silico, in vitro, and in vivo observations. PBPK hereby explicitly discriminates between physiological properties of the population (system parameters, like cardiac output, renal function, liver size, weight, plasma protein, different between populations) and compound specific (chemical, pH, solubility) properties, not different between populations (Figure 2). Using this approach, it has applications in drug development for first-in-human, first-in-child, or first in ECMO-patients, and became an established tool for drug development and regulatory needs, like e.g., data in cases with hepatic or renal impairment, drug-drug or drug-food interactions to avoid the need to recruit an impossible number of patients with very specific issues while still have sufficient confidence in the dosing regimens. The final intention is to generate dosing recommendations, or alternatively, simulations to subsequently conduct PK studies, as highlighted in Figure 2 for the specific ECMO setting (#).
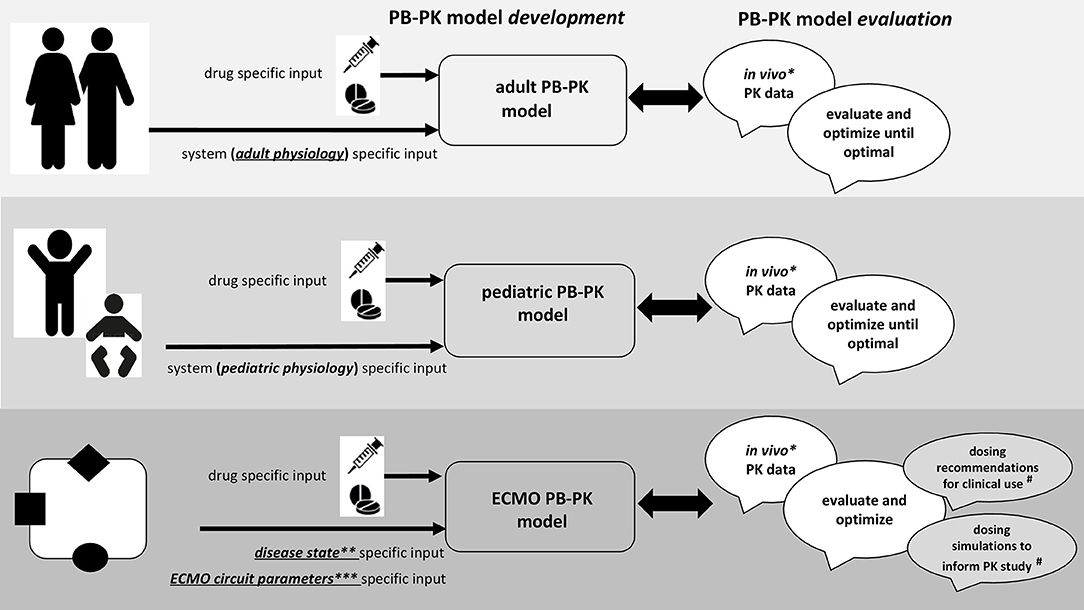
Figure 2. Integrated approach for drug prediction through physiologically-based pharmacokinetic (PBPK) models during neonatal ECMO.
Why ECMO Physicians and Clinical Researchers Are Needed to Develop Such Models
As illustrated in Figure 2, the workflow to develop and build confidence in PBPK models tailored to neonatal and pediatric ECMO pharmacotherapy necessitates availability of in vivo PK data (*), data on disease state (**), and on ECMO circuit parameters (***), and this is exactly why clinicians should become aware of the usefulness of such data beyond compound specific relevance (164, 165, 167, 168).
Availability of in vivo PK Dataset (*, Figure 2)
To illustrate that there are indeed already quite some compound specific PK observations, we conducted a structured search in PubMed on 15 January 2019 with [ECMO, newborn, and pharmacokinetics] as search terms. This resulted in 72 hits, and additional search with “infant” resulted in 79 hits, but no additional compounds. The results of this search are provided in Table 4, reporting on the compounds (n = 16) retrieved and supported by the most recent reference (115, 117, 121, 123, 132, 140–149). From a PBPK perspective, it is important to realize that these compounds are quite different when we consider protein binding, pKa (reflecting the chemical characteristics of the compound) and can be used to evaluate and optimize a variety of elimination routes, including renal, phase 1, and phase 2 processes: a perfect mix to validate the models. So PK data sharing and collaboration is an obvious need, since the data already exist and can be used to predict PK for drugs not yet evaluated or even not yet marketed (169).
Data on Disease State (**, Figure 2)
Extracorporeal membrane oxygenation is a technique to treat life threatening conditions, so data on these underlying disease conditions are also needed to further develop ECMO related P‘BPK models: it's is not just the technique, but also the reason for the technique that matters. To illustrate the feasibility to integrated (patho) physiology, we refer to PBPK models for carvedilol in children with cardiac failure (170) or intensive care adult patients with hypo-albuminemia (171). In the neonatal and pediatric ECMO, perinatal asphyxia, sepsis, or post resuscitation are common settings. It has been proven that disease affects drug PK (refer to previous section on pre-ECMO disease) (37, 67). Besides such observations, clinical researchers should also consider to build multi-center datasets (as part of the ongoing ELSO registry initiatives) on inter- and intra-patient trends of “real world” data. We hereby refer to trends in fluid retention, albumin, creatinine, heart rate, and cardiac output, energy expenditure or more specific issues like alfa-1 glycoprotein (Table 4). This is because such datasets can further feed and improve PBPK prediction, including intra-patient trends with time (8, 172). This has also recently been illustrated for e.g., alfa-1 glycoprotein maturation (173).
Data on ECMO Circuit Parameters (***)
Finally, equipment matters and data on newer extracorporeal technology need to be considered. PBPK modeling will generate further knowledge, which may guide both the development of new ECMO devices and the refinement of current technology at a biomedical engineering level.
Conclusions and Future Directions
Extracorporeal membrane oxygenation has an established role in the care of critically ill neonates. The exposure to the extracorporeal circuit impacts on drugs' disposition, potentially leading to undertreatment or toxicity, especially for drugs with a narrow therapeutic index. Non-maturational determinants (such as asphyxia/hypoxia, sepsis/SIRS, MODS) during pre-ECMO predetermine large Vd for hydrophilic drugs due to the underlying disease, while superimposed ECMO may lead to larger Vd for lipophilic and, to a lesser extent, hydrophilic drugs. Therefore, LD adjustment may be recommended to achieve optimal drug levels in neonates on ECMO. CL is influenced by renal (hydrophilic, high renal clearance drugs) and/or hepatic functions (lipophilic, high liver clearance drugs) under sepsis, asphyxia and treatment modalities (HT, ECMO), and optimal maintenance dose adjustment should be achieved on an individual basis (development, disease, genetics). Therefore, TDM is suggested to optimize LD/MD in these critically ill neonates. As drug dosing needs to be guided by PK or PD or PK/PD principles, the understanding of PK-PD changes during (pre-) ECMO will assist in the prescribing optimization and, eventually, contribute to improve patients' outcomes.
In this review we have provided an overview of the available evidence on the impact of both maturational and non-maturational determinants of PK in critically-ill neonates on ECMO. We subsequently have discussed the relevance of these determinants on the disposition of analgo-sedatives and antimicrobial and antiviral drugs during neonatal ECMO. Future efforts should be directed toward a more integrated approach, by combining existing knowledge to predict PK profile. Sparse samplings of three different periods (pre-, during, post- ECMO) may be adopted to better understand dynamically changing drug disposition. Further PK in vivo/in vitro studies will provide insights into the role of contemporary ECMO systems superimposed on maturational/non-maturational determinants.
Gathered knowledge into the maturational physiology-, illness-, and ECMO-related PK impact should be used to inform PBPK modeling, which is emerging as an alternative and powerful tool to provide bedside dosing guidance. Lastly, a prospective validation of PK/PD studies is needed by well conducted clinical trials to optimize dosing.
The final aim will be to apply pharmacotherapy in a goal-directed fashion, by reaching optimal PD outcomes through the individualization of the prescription, thus maximizing the therapeutical benefits in these vulnerable patients.
Author's Note
PP and DT represent the section of Pharmacology of the European Society of Pediatric and Neonatal Intensive Care (ESPNIC).
Author Contributions
All authors contributed conception and design of the article. GR wrote the first draft of the manuscript. PP, KA, and DT wrote sections of the manuscript. All authors contributed to manuscript critical revision, read and approved the submitted version.
Funding
PP was supported by a project MH CZ–DRO—VFN64165 and by project Progress–Charles University in the Czech Republic and by an unrestricted research grant of the Intensive Care of the Erasmus MC–Sophia Children's Hospital, Rotterdam, The Netherlands.
Conflict of Interest Statement
The authors declare that the research was conducted in the absence of any commercial or financial relationships that could be construed as a potential conflict of interest.
References
1. Bartlett RH, Gazzaniga AB, Jefferies MR, Huxtable RF, Haiduc N, Fong S. Extracorporeal membrane oxygenation (ECMO) cardiopulmonary support in infancy. ASAIO J. (1976) 22:80–92.
2. Bahrami KR, Van Meurs KP. ECMO for neonatal respiratory failure. Semin Perinatol. (2005) 29:15–23. doi: 10.1053/j.semperi.2005.02.004
3. Slutsky AS, Ranieri VM. Ventilator-induced lung injury. N Engl J Med. (2013) 369:2126–36. doi: 10.1056/NEJMra1208707
4. Buck ML. Pharmacokinetic changes during extracorporeal membrane oxygenation: implications for drug therapy of neonates. Clin Pharmacokinet. (2003) 42:403–17. doi: 10.2165/00003088-200342050-00001
5. Lequier L. Extracorporeal life support in pediatric and neonatal critical care: a review. J Intensive Care Med. (2004) 19:243–58. doi: 10.1177/0885066604267650
6. Blumenthal D, Brunton LL, Buxton IL, Parker KL. Goodman & Gilman's Manual of Pharmacology and Therapeutics. New York, NY: McGraw-Hill (2008).
7. Katzung BG, Trevor AJ. Basic & Clinical Pharmacology. New York, NY: Lange Medical Books/McGraw-Hill (2004).
8. Allegaert K, Simons SH, Tibboel D, Krekels EH, Knibbe CA, van den Anker JN. Non-maturational covariates for dynamic systems pharmacology models in neonates, infants, and children: filling the gaps beyond developmental pharmacology. Eur J Pharm Sci. (2017) 109: S27–31. doi: 10.1016/j.ejps.2017.05.023
9. Allegaert K, van den Anker JN. Clinical pharmacology in neonates: small size, huge variability. Neonatology. (2014) 105:344–9. doi: 10.1159/000360648
10. Kearns GL, Abdel-Rahman SM, Alander SW, Blowey DL, Leeder JS, Kauffman RE. Developmental pharmacology—drug disposition, action, and therapy in infants and children. N Engl J Med. (2003) 349:1157–67. doi: 10.1056/NEJMra035092
11. van den Anker J, Reed MD, Allegaert K, Kearns GL. Developmental changes in pharmacokinetics and pharmacodynamics. J Clin Pharmacol. (2018) 58:S10–25. doi: 10.1002/jcph.1284
12. Roberts JA, Kumar A, Lipman J. Right dose, right now: customized drug dosing in the critically ill. Crit Care Med. (2017) 45:331–6. doi: 10.1097/CCM.0000000000002210
13. Shekar K, Roberts JA, Mcdonald CI, Fisquet S, Barnett AG, Mullany DV, et al. Sequestration of drugs in the circuit may lead to therapeutic failure during extracorporeal membrane oxygenation. Crit Care. (2012) 16:R194. doi: 10.1186/cc11679
14. Shekar K, Roberts J, McDonald C, Ghassabian S, Anstey C, Wallis SC, et al. Protein-bound drugs are prone to sequestration in the extracorporeal membrane oxygenation circuit: results from an ex vivo study. Crit Care. (2015) 19:164. doi: 10.1186/s13054-015-0891-z
15. Wildschut ED, Ahsman MJ, Allegaert K, Mathot RA, Tibboel D. Determinants of drug absorption in different ECMO circuits. Intensive Care Med. (2010) 36:2109–16. doi: 10.1007/s00134-010-2041-z
16. Bartelink IH, Rademaker CM, Schobben AF, van den Anker JN. Guidelines on paediatric dosing on the basis of developmental physiology and pharmacokinetic considerations. Clin Pharmacokinet. (2006) 45:1077–97. doi: 10.2165/00003088-200645110-00003
17. Ehrnebo M, Agurell S, Jalling B, Boreus L. Age differences in drug binding by plasma proteins: studies on human foetuses, neonates and adults. Eur J Clin Pharmacol. (1971) 3:189–93. doi: 10.1007/BF00565004
18. Friis-Hansen B. Water distribution in the foetus and newborn infant. Acta Pædiatrica. (1983) 72:7–11. doi: 10.1111/j.1651-2227.1983.tb09852.x
19. Allegaert K, Mian P, N van den Anker J. Developmental pharmacokinetics in neonates: maturational changes and beyond. Curr Pharm Design. (2017) 23:5769–78. doi: 10.2174/1381612823666170926121124
20. Euteneuer JC, Kamatkar S, Fukuda T, Vinks AA, Akinbi HT. Suggestions for model-informed precision dosing to optimize neonatal drug therapy. J Clin Pharmacol. (2019) 59:168–76. doi: 10.1002/jcph.1315
21. Allegaert K, Cosaert K, van den Anker JN. Neonatal formulations: the need for a tailored, knowledge driven approach. Curr Pharm Design. (2015) 21:5674–79. doi: 10.2174/1381612821666150901110207
22. Bone RC, Sibbald WJ, Sprung CL. The ACCP-SCCM consensus conference on sepsis and organ failure. Chest. (1992) 101:1481–4. doi: 10.1378/chest.101.6.1481
23. Bodenham A, Shelly M, Park G. The altered pharmacokinetics and pharmacodynamics of drugs commonly used in critically ill patients. Clin Pharmacokinet. (1988) 14:347–73. doi: 10.2165/00003088-198814060-00003
24. De Cock RF, Piana C, Krekels EH, Danhof M, Allegaert K, Knibbe CA. The role of population PK-PD modelling in paediatric clinical research. Eur J Clin Pharmacol. (2011) 67 (suppl. 1):5–16. doi: 10.1007/s00228-009-0782-9
25. Shekar K, Roberts JA, Welch S, Buscher H, Rudham S, Burrows F, et al. ASAP ECMO: antibiotic, sedative and analgesic pharmacokinetics during extracorporeal membrane oxygenation: a multi-centre study to optimise drug therapy during ECMO. BMC Anesthesiol. (2012) 12:29. doi: 10.1186/1471-2253-12-29
26. Di Nardo M, Wildschut ED. Drugs pharmacokinetics during veno-venous extracorporeal membrane oxygenation in pediatrics. J Thorac Dis. (2018) 10 (suppl. 5):S642–52. doi: 10.21037/jtd.2017.11.02
27. Boucher BA, Wood GC, Swanson JM. Pharmacokinetic changes in critical illness. Crit Care Clin. (2006) 22:255–71. doi: 10.1016/j.ccc.2006.02.011
28. Carter BS, Haverkamp AD, Merenstein GB. The definition of acute perinatal asphyxia. Clin Perinatol. (1993) 20:287–304. doi: 10.1016/S0095-5108(18)30394-4
29. Azzopardi DV, Strohm B, Edwards AD, Dyet L, Halliday HL, Juszczak E, et al. Moderate hypothermia to treat perinatal asphyxial encephalopathy. N Engl J Med. (2009) 361:1349–58. doi: 10.1056/NEJMoa0900854
30. Jacobs SE, Berg M, Hunt R, Tarnow-Mordi WO, Inder TE, Davis PG. Cooling for newborns with hypoxic ischaemic encephalopathy. Cochrane Database Syst Rev. (2013) CD003311. doi: 10.1002/14651858.CD003311.pub3
31. Chhavi N, Zutshi K, Singh NK, Awasthi A, Goel A. Serum liver enzyme pattern in birth asphyxia associated liver injury. Pediatr Gastroenterol Hepatol Nutr. (2014) 17:162–9. doi: 10.5223/pghn.2014.17.3.162
32. Martin-Ancel A, Garcia-Alix A, Gaya F, Cabanas F, Burgueros M, Quero J. Multiple organ involvement in perinatal asphyxia. J Pediatr. (1995) 127:786–93. doi: 10.1016/S0022-3476(95)70174-5
33. Shah P, Riphagen S, Beyene J, Perlman M. Multiorgan dysfunction in infants with post-asphyxial hypoxic-ischaemic encephalopathy. Arch Dis Child Fetal Neonatal Ed. (2004) 89:F152–5. doi: 10.1136/fn.89.2.F152
34. ELSO Registry Report. International Summary January 2019. Available online at: https://www.elso.org (accessed March 30, 2019).
35. Reiterer F, Resch E, Haim M, Maurer-Fellbaum U, Riccabona M, Zobel G, et al. Neonatal extracorporeal membrane oxygenation due to respiratory failure: a single center experience over 28 years. Front Pediatr. (2018) 6:263. doi: 10.3389/fped.2018.00263
36. Cornell TT, Selewski DT, Alten JA, Askenazi D, Fitzgerald JC, Topjian A, et al. Acute kidney injury after out of hospital pediatric cardiac arrest. Resuscitation. (2018) 131:63–8. doi: 10.1016/j.resuscitation.2018.07.362
37. Cristea S, Smits A, Kulo A, Knibbe CAJ, van Weissenbruch M, Krekels EHJ, et al. Amikacin pharmacokinetics to optimize dosing in neonates with perinatal asphyxia treated with hypothermia. Antimicrob Agents Chemother. (2017) 61:e01282–17. doi: 10.1128/AAC.01282-17
38. Van Den Anker JN, Van Der Heijden BJ, Hop WC, Schoemaker RC, Broerse HM, Neijens HJ, et al. The effect of asphyxia on the pharmacokinetics of ceftazidime in the term newborn. Pediatr Res. (1995) 38:808–11. doi: 10.1203/00006450-199511000-00028
39. Bijleveld YA, de Haan TR, van der Lee HJ, Groenendaal F, Dijk PH, van Heijst A, et al. Altered gentamicin pharmacokinetics in term neonates undergoing controlled hypothermia. Br J Clin Pharmacol. (2016) 81:1067–77. doi: 10.1111/bcp.12883
40. Bijleveld YA, Mathôt R, van der Lee JH, Groenendaal F, Dijk PH, van Heijst A, et al. Population pharmacokinetics of amoxicillin in term neonates undergoing moderate hypothermia. Clin Pharmacol Therapeut. (2018) 103:458–67. doi: 10.1002/cpt.748
41. Bijleveld YA, de Haan TR, van der Lee JH, Groenendaal F, Dijk PH, van Heijst A, et al. Evaluation of a system-specific function to describe the pharmacokinetics of benzylpenicillin in term neonates undergoing moderate hypothermia. Antimicrob Agents Chemother. (2018) 62:e02311–17. doi: 10.1128/AAC.02311-17
42. Hinderling PH, Hartmann D. The pH dependency of the binding of drugs to plasma proteins in man. Therapeut Drug Monitor. (2005) 27:71–85. doi: 10.1097/00007691-200502000-00014
43. van den Broek MP, Groenendaal F, Egberts AC, Rademaker CM. Effects of hypothermia on pharmacokinetics and pharmacodynamics: a systematic review of preclinical and clinical studies. Clin Pharmacokinet. (2010) 49:277–94. doi: 10.2165/11319360-000000000-00000
44. Zanelli S, Buck M, Fairchild K. Physiologic and pharmacologic considerations for hypothermia therapy in neonates. J Perinatol. (2011) 31:377–86. doi: 10.1038/jp.2010.146
45. Zhou J, Poloyac SM. The effect of therapeutic hypothermia on drug metabolism and response: cellular mechanisms to organ function. Expert Opin Drug Metab Toxicol. (2011) 7:803–16. doi: 10.1517/17425255.2011.574127
46. Pokorna P, Wildschut ED, Vobruba V, van den Anker JN, Tibboel D. The impact of hypothermia on the pharmacokinetics of drugs used in neonates and young infants. Curr Pharmaceut Design. (2015) 21:5705–24. doi: 10.2174/1381612821666150901110929
47. Wildschut ED, de Wildt SN, Mathot RA, Reiss IK, Tibboel D, Van den Anker J. Effect of hypothermia and extracorporeal life support on drug disposition in neonates. Semin Fetal Neonatal Med. (2013) 18:23–7. doi: 10.1016/j.siny.2012.10.002
48. Shellhaas RA, Ng CM, Dillon CH, Barks JD, Bhatt-Mehta V. Population pharmacokinetics of phenobarbital in infants with neonatal encephalopathy treated with therapeutic hypothermia. Pediatr Crit Care Med. (2013) 14:194–202. doi: 10.1097/PCC.0b013e31825bbbc2
49. van den Broek MP, Groenendaal F, Toet MC, van Straaten HL, van Hasselt JG, Huitema AD, et al. Pharmacokinetics and clinical efficacy of phenobarbital in asphyxiated newborns treated with hypothermia: a thermopharmacological approach. Clin Pharmacokinet. (2012) 51:671–9. doi: 10.1007/s40262-012-0004-y
50. Völler S, Flint RB, Stolk LM, Degraeuwe PLJ, Simons SHP, Pokorna P, et al. Model-based clinical dose optimization for phenobarbital in neonates: an illustration of the importance of data sharing and external validation. Eur J Pharmaceut Sci. (2017) 109S:S90–7. doi: 10.1016/j.ejps.2017.05.026
51. Pokorná P, Posch L, Šíma M, Klement P, Slanar O, van den Anker J, et al. Severity of asphyxia is a covariate of phenobarbital clearance in newborns undergoing hypothermia. J Maternal Fetal Neonatal Med. (2019) 32:2302–9. doi: 10.1080/14767058.2018.1432039
52. Róka A, Melinda KT, Vásárhelyi B, Machay T, Azzopardi D, Szabó M. Elevated morphine concentrations in neonates treated with morphine and prolonged hypothermia for hypoxic ischemic encephalopathy. Pediatrics. (2008) 121:e844–9. doi: 10.1542/peds.2007-1987
53. Smits A, Kulo A, van den Anker J, Allegaert K. The amikacin research program: a stepwise approach to validate dosing regimens in neonates. Expert Opin Drug Metab Toxicol. (2017) 13:157–66. doi: 10.1080/17425255.2017.1234606
54. Filippi L, la Marca G, Cavallaro G, Fiorini P, Favelli F, Malvagia S, et al. Phenobarbital for neonatal seizures in hypoxic ischemic encephalopathy: a pharmacokinetic study during whole body hypothermia. Epilepsia. (2011) 52:794–801. doi: 10.1111/j.1528-1167.2011.02978.x
55. Filippi L, la Marca G, Fiorini P, Poggi C, Cavallaro G, Malvagia S, et al. Topiramate concentrations in neonates treated with prolonged whole body hypothermia for hypoxic ischemic encephalopathy. Epilepsia. (2009) 50:2355–61. doi: 10.1111/j.1528-1167.2009.02302.x
56. de Haan TR, Bijleveld YA, van der Lee JH, Groenendaal F, van den Broek MP, Rademaker CM, et al. Pharmacokinetics and pharmacodynamics of medication in asphyxiated newborns during controlled hypothermia. The PharmaCool multicenter study. BMC Pediatr. (2012) 12:45. doi: 10.1186/1471-2431-12-45
57. Wynn JL. Defining neonatal sepsis. Curr Opin Pediatr. (2016) 28:135–40. doi: 10.1097/MOP.0000000000000315
58. Goldstein B, Giroir B, Randolph A, International Consensus Conference on Pediatric S. International pediatric sepsis consensus conference: definitions for sepsis and organ dysfunction in pediatrics. Pediatr Crit Care Med. (2005) 6:2–8. doi: 10.1097/01.PCC.0000149131.72248.E6
59. Bestati N, Leteurtre S, Duhamel A, Proulx F, Grandbastien B, Lacroix J, et al. Differences in organ dysfunctions between neonates and older children: a prospective, observational, multicenter study. Crit Care. (2010) 14:R202. doi: 10.1186/cc9323
60. Renton KW. Regulation of drug metabolism and disposition during inflammation and infection. Expert Opin Drug Metab Toxicol. (2005) 1:629–40. doi: 10.1517/17425255.1.4.629
61. Udy AA, Roberts JA, Lipman J. Implications of augmented renal clearance in critically ill patients. Nat Rev Nephrol. (2011) 7:539. doi: 10.1038/nrneph.2011.92
62. Udy AA, Roberts JA, Boots RJ, Paterson DL, Lipman J. Augmented renal clearance. Clin Pharmacokinet. (2010) 49:1–16. doi: 10.2165/11318140-000000000-00000
63. Hobbs AL, Shea KM, Roberts KM, Daley MJ. Implications of augmented renal clearance on drug dosing in critically ill patients: a focus on antibiotics. Pharmacotherapy. (2015) 35:1063–75. doi: 10.1002/phar.1653
64. Dhont E, Van Der Heggen T, De Jaeger A, Walle JV, De Paepe P, De Cock PA. Augmented renal clearance in pediatric intensive care: are we undertreating our sickest patients? Pediatr Nephrol. (2018):1–15. doi: 10.1007/s00467-018-4120-2
65. Avedissian SN, Bradley E, Zhang D, Bradley JS, Nazer LH, Tran TM, et al. Augmented renal clearance using population-based pharmacokinetic modeling in critically ill pediatric patients. Pediatr Crit Care Med. (2017) 18:e388–94. doi: 10.1097/PCC.0000000000001228
66. van den Anker JN, Knibbe CA, Tibboel D. Augmented renal clearance in critically III pediatric patients: does it impact the outcome of pharmacotherapy? Pediatr Crit Care Med. (2017) 18:901–2. doi: 10.1097/PCC.0000000000001264
67. Vet NJ, de Hoog M, Tibboel D, de Wildt SN. The effect of inflammation on drug metabolism: a focus on pediatrics. Drug Discov Today. (2011) 16:435. doi: 10.1016/j.drudis.2011.02.014
68. Varghese JM, Roberts JA, Lipman J. Antimicrobial pharmacokinetic and pharmacodynamic issues in the critically ill with severe sepsis and septic shock. Crit Care Clin. (2011) 27:19–34. doi: 10.1016/j.ccc.2010.09.006
69. Shah S, Barton G, Fischer A. Pharmacokinetic considerations and dosing strategies of antibiotics in the critically ill patient. J Intensive Care Soc. (2015) 16:147–53. doi: 10.1177/1751143714564816
70. De Paepe P, Belpaire FM, Buylaert WA. Pharmacokinetic and pharmacodynamic considerations when treating patients with sepsis and septic shock. Clin Pharmacokinet. (2002) 41:1135–51. doi: 10.2165/00003088-200241140-00002
71. Dagan O, Klein J, Gruenwald C, Bohn D, Barker G, Koren G. Preliminary studies of the effects of extracorporeal membrane oxygenator on the disposition of common pediatric drugs. Therapeut Drug Monitor. (1993) 15:263–66. doi: 10.1097/00007691-199308000-00001
72. De Cock PA, Allegaert K, Linakis MW, Sherwin CM. Antibiotic dosing in pediatric critically Ill patients. In: Antibiotic Pharmacokinetic/Pharmacodynamic Considerations in the Critically Ill. Singapore: Springer (2018). p. 239–63.
73. Wildschut ED, Ahsman MJ, Houmes RJ, Pokorna P, de Wildt SN, Mathot RA, et al. Pharmacotherapy in neonatal and pediatric extracorporeal membrane oxygenation (ECMO). Curr Drug Metab. (2012) 13:767–77. doi: 10.2174/138920012800840383
74. Rehder KJ, Turner DA, Bonadonna D, Walczak RJ, Rudder RJ, Cheifetz IM. Technological advances in extracorporeal membrane oxygenation for respiratory failure. Expert Rev Respir Med. (2012) 6:377–84. doi: 10.1586/ers.12.31
75. ELSO. Extracorporeal Life Support, The Red Book: The ELSO Red Book. 5th ed. Ann Arbor, MI: Extracorporeal Life Support Organization (2017).
76. Poole SK, Poole CF. Separation methods for estimating octanol–water partition coefficients. J Chromatogr B. (2003) 797:3–19. doi: 10.1016/j.jchromb.2003.08.032
77. Shekar K, Roberts JA, Barnett AG, Diab S, Wallis SC, Fung YL, et al. Can physicochemical properties of antimicrobials be used to predict their pharmacokinetics during extracorporeal membrane oxygenation? Illustrative data from ovine models. Crit Care. (2015) 19:437. doi: 10.1186/s13054-015-1151-y
78. Himebauch AS, Kilbaugh TJ, Zuppa AF. Pharmacotherapy during pediatric extracorporeal membrane oxygenation: a review. Expert Opin Drug Metab Toxicol. (2016) 12:1133–42. doi: 10.1080/17425255.2016.1201066
79. Park J, Shin DA, Lee S, Cho YJ, Jheon S, Lee JC, et al. Investigation of key circuit constituents affecting drug sequestration during extracorporeal membrane oxygenation treatment. ASAIO J. (2017) 63:293–8. doi: 10.1097/MAT.0000000000000489
80. Bhatt-Mehta V, Annich G. Sedative clearance during extracorporeal membrane oxygenation. Perfusion. (2005) 20:309–15. doi: 10.1191/0267659105pf827oa
81. Wagner D, Pasko D, Phillips K, Waldvogel J, Annich G. In vitro clearance of dexmedetomidine in extracorporeal membrane oxygenation. Perfusion. (2013) 28:40–6. doi: 10.1177/0267659112456894
82. Melchior RW, Sutton SW, Harris W, Dalton HJ. Evolution of membrane oxygenator technology for utilization during pediatric cardiopulmonary bypass. Pediatr Health Med Therapeut. (2016) 7:45. doi: 10.2147/PHMT.S35070
83. Rosen DA, Rosen KR, Silvasi DL. In vitro variability in fentanyl absorption by different membrane oxygenators. J Cardiothorac Anesth. (1990) 4:332–5. doi: 10.1016/0888-6296(90)90041-D
84. Raffaeli G, Allegaert K, Koch B, Cavallaro G, Mosca F, Tibboel D, et al. In vitro adsorption of analgosedative drugs in new extracorporeal membrane oxygenation circuits. Pediatr Crit Care Med. (2018) 19:e251–8. doi: 10.1097/PCC.0000000000001484
85. Preston TJ, Hodge AB, Riley JB, Leib-Sargel C, Nicol KK. In vitro drug adsorption and plasma free hemoglobin levels associated with hollow fiber oxygenators in the extracorporeal life support (ECLS) circuit. J Extra Corpor Technol. (2007) 39:234–7.
86. Tron C, Leven C, Fillâtre P, Maillard N, Nesseler N, Tattevin P, et al. Should we fear tubing adsorption of antibacterial drugs in extracorporeal membrane oxygenation? An answer for cephalosporins and carbapenems. Clin Exp Pharmacol Physiol. (2016) 43:281–3. doi: 10.1111/1440-1681.12527
87. Leven C, Fillâtre P, Petitcollin A, Verdier MC, Laurent J, Nesseler N, et al. ex vivo model to decipher the impact of extracorporeal membrane oxygenation on beta-lactam degradation kinetics. Therapeut Drug Monitor. (2017) 39:180–4. doi: 10.1097/FTD.0000000000000369
88. Preston TJ, Ratliff TM, Gomez D, Olshove VE, Nicol KK, Sargel CL, et al. Modified surface coatings and their effect on drug adsorption within the extracorporeal life support circuit. J Extra Corpor Technol. (2010) 42:199.
89. Silvetti S, Koster A, Pappalardo F. Do we need heparin coating for extracorporeal membrane oxygenation? New concepts and controversial positions about coating surfaces of extracorporeal circuits. Artif Organs. (2015) 39:176–9. doi: 10.1111/aor.12335
90. Myers GJ, Voorhees C, Eke B, Johnstone R. The effect of Diprivan (propofol) on phosphorylcholine surfaces during cardiopulmonary bypass—an In vitro investigation. Perfusion. (2009) 24:349–55. doi: 10.1177/0267659109353819
91. Peters JW, Anderson BJ, Simons SH, Uges DR, Tibboel D. Morphine metabolite pharmacokinetics during venoarterial extra corporeal membrane oxygenation in neonates. Clin Pharmacokinet. (2006) 45:705–14. doi: 10.2165/00003088-200645070-00005
92. Mehta NM, Halwick DR, Dodson BL, Thompson JE, Arnold JH. Potential drug sequestration during extracorporeal membrane oxygenation: results from an ex vivo experiment. Intensive Care Med. (2007) 33:1018–24. doi: 10.1007/s00134-007-0606-2
93. Mulla H, Lawson G, von Anrep C, Burke MD, Upton DU, Firmin RK, et al. In vitro evaluation of sedative drug losses during extracorporeal membrane oxygenation. Perfusion. (2000) 15:21–26. doi: 10.1177/026765910001500104
94. Shekar K, Fraser JF, Smith MT, Roberts JA. Pharmacokinetic changes in patients receiving extracorporeal membrane oxygenation. J Crit Care. (2012) 27:741.e749-18. doi: 10.1016/j.jcrc.2012.02.013
95. Pea F. Plasma pharmacokinetics of antimicrobial agents in critically ill patients. Curr Clin Pharmacol. (2013) 8:5–12. doi: 10.2174/157488413804810585
96. Brink A. Hypoalbuminaemia and altered protein binding. In: Antibiotic Pharmacokinetic/Pharmacodynamic Considerations in the Critically III. Springer (2018). p. 73–99.
97. Koch-Weser J, Sellers EM. Binding of drugs to serum albumin. N Engl J Med. (1976) 294:311–6. doi: 10.1056/NEJM197602052940605
98. Kozik DJ, Tweddell JS. Characterizing the inflammatory response to cardiopulmonary bypass in children. Ann Thorac Surg. (2006) 81:S2347–54. doi: 10.1016/j.athoracsur.2006.02.073
99. McILwain RB, Timpa JG, Kurundkar AR, Holt DW, Kelly DR, Hartman YE, et al. Plasma concentrations of inflammatory cytokines rise rapidly during ECMO-related SIRS due to the release of preformed stores in the intestine. Lab Invest. (2010) 90:128–39. doi: 10.1038/labinvest.2009.119
100. Millar JE, Fanning JP, McDonald CI, McAuley DF, Fraser JF. The inflammatory response to extracorporeal membrane oxygenation (ECMO): a review of the pathophysiology. Crit Care. (2016) 20:387. doi: 10.1186/s13054-016-1570-4
101. Raffaeli G, Ghirardello S, Passera S, Mosca F, Cavallaro G. Oxidative stress and neonatal respiratory extracorporeal membrane oxygenation. Front Physiol. (2018) 9:1739. doi: 10.3389/fphys.2018.01739
102. Peek GJ, Firmin RK. The inflammatory and coagulative response to prolonged extracorporeal membrane oxygenation. ASAIO J. (1999) 45:250–63. doi: 10.1097/00002480-199907000-00003
103. Shekar K, Roberts JA, Ghassabian S, Mullany DV, Wallis SC, Smith MT, et al. Altered antibiotic pharmacokinetics during extracorporeal membrane oxygenation: cause for concern? J Antimicrob Chemother. (2013) 68:726–27. doi: 10.1093/jac/dks435
104. Many M, Soroff H, Birtwell W, Giron F, Wise H, Deterling RA. The physiologic role of pulsatile and nonpulsatile blood flow: II. Effects on renal function. Arch Surg. (1967) 95:762–7. doi: 10.1001/archsurg.1967.01330170070009
105. Arnold JH, Truog RD, Orav EJ, Scavone JM, Hershenson MB. Tolerance and dependence in neonates sedated with fentanyl during extracorporeal membrane oxygenation. Anesthesiology. (1990) 73:1136–40. doi: 10.1097/00000542-199012000-00011
106. Mulla H, Lawson G, Woodland E, Peek GJ, Killer H, Firmin RK, et al. Effects of neonatal extracorporeal membrane oxygenation circuits on drug disposition. Curr Therapeut Res. (2000) 61:838–48. doi: 10.1016/S0011-393X(00)90010-9
107. Leuschen MP, Willett LD, Hoie EB, Bolam DL, Bussey ME, Goodrich PD, et al. Plasma fentanyl levels in infants undergoing extracorporeal membrane oxygenation. J Thorac Cardiovasc Surg. (1993) 105:885–91.
108. Mulla H, McCormack P, Lawson G, Firmin RK, Upton DR. Pharmacokinetics of midazolam in neonates undergoing extracorporeal membrane oxygenation. Anesthesiology. (2003) 99:275–82. doi: 10.1097/00000542-200308000-00008
109. Mulla H, Lawson G, Peek GJ, Firmin R, Upton DR. Plasma concentrations of midazolam in neonates receiving extracorporeal membrane oxygenation. ASAIO J. (2003) 49:41–47. doi: 10.1097/00002480-200301000-00007
110. Nasr VG, Meserve J, Pereira LM, Faraoni D, Brediger S, Goobie S, et al. Sedative and analgesic drug sequestration after a single bolus injection in an ex vivo extracorporeal membrane oxygenation infant circuit. ASAIO J. (2019) 65:187–91. doi: 10.1097/MAT.0000000000000793
111. Suresh S, Anand K. Opioid tolerance in neonates: a state-of-the-art review. Pediatr Anesth. (2001) 11:511–21. doi: 10.1046/j.1460-9592.2001.00764.x
112. Wildschut ED, Hanekamp MN, Vet NJ, Houmes RJ, Ahsman MJ, Mathot RA, et al. Feasibility of sedation and analgesia interruption following cannulation in neonates on extracorporeal membrane oxygenation. Intensive Care Med. (2010) 36:1587–91. doi: 10.1007/s00134-010-1931-4
113. DeBerry BB, Lynch JE, Chernin JM, Zwischenberger JB, Chung DH. A survey for pain and sedation medications in pediatric patients during extracorporeal membrane oxygenation. Perfusion. (2005) 20:139–43. doi: 10.1191/0267659105pf801oa
114. Gillogly A, Kilbourn C, Waldvogel J, Martin J, Annich G, Wagner D. In vitro clearance of intravenous acetaminophen in extracorporeal membrane oxygenation. Perfusion. (2013) 28:141–5. doi: 10.1177/0267659112467825
115. Kleiber N, Mathôt RA, Ahsman MJ, Wildschut ED, Tibboel D, Wildt SN. Population pharmacokinetics of intravenous clonidine for sedation during paediatric extracorporeal membrane oxygenation and continuous venovenous hemofiltration. Br J Clin Pharmacol. (2017) 83:1227–39. doi: 10.1111/bcp.13235
116. Cies JJ, Moore WS, Giliam N, Low T, Enache A, Chopra A. Impact of ex vivo extracorporeal membrane oxygenation circuitry on daptomycin. Perfusion. (2018) 33:624–9. doi: 10.1177/0267659118781761
117. Ahsman MJ, Hanekamp M, Wildschut ED, Tibboel D, Mathot RA. Population pharmacokinetics of midazolam and its metabolites during venoarterial extracorporeal membrane oxygenation in neonates. Clin Pharmacokinet. (2010) 49:407–19. doi: 10.2165/11319970-000000000-00000
119. Dagan O, Klein J, Bohn D, Koren G. Effects of extracorporeal membrane oxygenation on morphine pharmacokinetics in infants. Crit Care Med. (1994) 22:1099–101. doi: 10.1097/00003246-199407000-00008
120. Ziesenitz VC, Vaughns JD, Koch G, Mikus G, van den Anker JN. Pharmacokinetics of fentanyl and its derivatives in children: a comprehensive review. Clin Pharmacokinet. (2018) 57:125–49. doi: 10.1007/s40262-017-0569-6
121. Ahsman MJ, Wildschut ED, Tibboel D, Mathot RA. Pharmacokinetics of cefotaxime and desacetylcefotaxime in infants during extracorporeal membrane oxygenation. Antimicrob Agents Chemother. (2010) 54:1734–41. doi: 10.1128/AAC.01696-09
122. Cies JJ, Moore WS, Conley SB, Dickerman MJ, Small C, Carella D, et al. Pharmacokinetics of continuous infusion meropenem with concurrent extracorporeal life support and continuous renal replacement therapy: a case report. J Pediatr Pharmacol Therapeut. (2016) 21:92–97. doi: 10.5863/1551-6776-21.1.92
123. Cies JJ, Moore WS, Nichols K, Knoderer CA, Carella DM, Chopra A. Population pharmacokinetics and pharmacodynamic target attainment of vancomycin in neonates on extracorporeal life support. Pediatr Crit Care Med. (2017) 18:977–85. doi: 10.1097/PCC.0000000000001250
124. An SH, Lee EM, Kim JY, sun Gwak H. Vancomycin pharmacokinetics in critically ill neonates receiving extracorporeal membrane oxygenation. Eur J Hosp Pharm. (2019). doi: 10.1136/ejhpharm-2018-001720. [Epub ahead of print].
125. Moffett BS, Morris J, Galati M, Munoz F, Arikan AA. Population pharmacokinetics of vancomycin in pediatric extracorporeal membrane oxygenation. Pediatr Crit Care Med. (2018) 19:973–80. doi: 10.1097/PCC.0000000000001682
126. Munzenberger PJ, Massoud N. Pharmacokinetics of gentamicin in neonatal patients supported with extracorporeal membrane oxygenation. ASAIO Trans. (1991) 37:16–18. doi: 10.1097/00002480-199101000-00006
127. Moffett BS, Morris J, Galati M, Munoz FM, Arikan AA. Population pharmacokinetic analysis of gentamicin in pediatric extracorporeal membrane oxygenation. Therapeut Drug Monitor. (2018) 40:581–8. doi: 10.1097/FTD.0000000000000547
128. Southgate WM, DiPiro JT, Robertson AF. Pharmacokinetics of gentamicin in neonates on extracorporeal membrane oxygenation. Antimicrob Agents Chemother. (1989) 33:817–9. doi: 10.1128/AAC.33.6.817
129. Bhatt-Mehta V, Johnson CE, Schumacher RE. Gentamicin pharmacokinetics in term neonates receiving extracorporeal membrane oxygenation. Pharmacotherapy. (1992) 12:28–32.
130. Kamal MA, Acosta EP, Kimberlin DW, Gibiansky L, Jester P, Niranjan V, et al. The posology of oseltamivir in infants with influenza infection using a population pharmacokinetic approach. Clin Pharmacol Therapeut. (2014) 96:380–9. doi: 10.1038/clpt.2014.120
131. Wildschut ED, De Hoog M, Ahsman MJ, Tibboel D, Osterhaus AD, Fraaij PL. Plasma concentrations of oseltamivir and oseltamivir carboxylate in critically ill children on extracorporeal membrane oxygenation support. PLoS ONE. (2010) 5:e10938. doi: 10.1371/journal.pone.0010938
132. Watt KM, Benjamin DK, Cheifetz IM, Moorthy G, Wade KC, Smith PB, et al. Pharmacokinetics and safety of fluconazole in young infants supported with extracorporeal membrane oxygenation. Pediatr Infect Dis J. (2012) 31:1042–7. doi: 10.1097/INF.0b013e31825d3091
133. Sherwin J, Heath T, Watt K. Pharmacokinetics and dosing of anti-infective drugs in patients on extracorporeal membrane oxygenation: a review of the current literature. Clin Therapeut. (2016) 38:1976–94. doi: 10.1016/j.clinthera.2016.07.169
134. Geiduschek JM, Lynn AM, Bratton SL, Sanders JC, Levy FH, Haberkern CM, et al. Morphine pharmacokinetics during continuous infusion of morphine sulfate for infants receiving extracorporeal membrane oxygenation. Crit Care Med. (1997) 25:360–4. doi: 10.1097/00003246-199702000-00027
135. Bauer TM, Ritz R, Haberthür C, Ha HR, Hunkeler W, Sleight AJ, et al. Prolonged sedation due to accumulation of conjugated metabolites of midazolam. Lancet. (1995) 346:145–7. doi: 10.1016/S0140-6736(95)91209-6
136. Koren G, Crean P, Klein J, Goresky G, Villamater J, MacLeod S. Sequestration of fentanyl by the cardiopulmonary bypass (CPBP). Eur J Clin Pharmacol. (1984) 27:51–56. doi: 10.1007/BF02395206
137. Franck LS, Vilardi J, Durand D, Powers R. Opioid withdrawal in neonates after continuous infusions of morphine or fentanyl during extracorporeal membrane oxygenation. Am J Crit Care. (1998) 7:364.
138. Kam P, Cardone D. Propofol infusion syndrome. Anaesthesia. (2007) 62:690–701. doi: 10.1111/j.1365-2044.2007.05055.x
139. Bizzarro MJ, Conrad SA, Kaufman DA, Rycus P. Infections acquired during extracorporeal membrane oxygenation in neonates, children, and adults*. Pediatr Crit Care Med. (2011) 12:277–81. doi: 10.1097/PCC.0b013e3181e28894
140. Pokorná P, Šíma M, Vobruba V, Tibboel D, Slanar O. Phenobarbital pharmacokinetics in neonates and infants during extracorporeal membrane oxygenation. Perfusion. (2018) 33 (suppl. 1):80–6. doi: 10.1177/0267659118766444
141. Niimi KS, Fanning JJ. Initial experience with recombinant antithrombin to treat antithrombin deficiency in patients on extracorporeal membrane oxygenation. J Extra Corpor Technol. (2014) 46:84–90.
142. Ahsman MJ, Witjes BC, Wildschut ED, Sluiter I, Vulto AG, Tibboel D, et al. Sildenafil exposure in neonates with pulmonary hypertension after administration via a nasogastric tube. Arch Dis Child Fetal Neonatal Ed. (2010) 95:F109–14. doi: 10.1136/adc.2009.168336
143. Kendrick JG, Macready JJ, Kissoon N. Amiodarone treatment of junctional ectopic tachycardia in a neonate receiving extracorporeal membrane oxygenation. Ann Pharmacother. (2006) 40:1872–5. doi: 10.1345/aph.1H148
144. Peters JW, Anderson BJ, Simons SH, Uges DR, Tibboel D. Morphine pharmacokinetics during venoarterial extracorporeal membrane oxygenation in neonates. Intensive Care Med. (2005) 31:257–63. doi: 10.1007/s00134-004-2545-5
145. Mulla H, Nabi F, Nichani S, Lawson G, Firmin R, Upton DR. Population pharmacokinetics of theophylline during paediatric extracorporeal membrane oxygenation. Br J Clin Pharmacol. (2003) 55:23–31. doi: 10.1046/j.1365-2125.2003.01735.x
146. Wells TG, Heulitt MJ, Taylor BJ, Fasules JW, Kearns GL. Pharmacokinetics and pharmacodynamics of ranitidine in neonates treated with extracorporeal membrane oxygenation. J Clin Pharmacol. (1998) 38:402–7. doi: 10.1002/j.1552-4604.1998.tb04443.x
147. Aebi C, Headrick CL, McCracken GH Jr, Lindsay CA. Intravenous ribavirin therapy in a neonate with disseminated adenovirus infection undergoing extracorporeal membrane oxygenation: pharmacokinetics and clearance by hemofiltration. J Pediatr. (1997) 130:612–5. doi: 10.1016/S0022-3476(97)70246-4
148. Wells TG, Fasules JW, Taylor BJ, Kearns GL. Pharmacokinetics and pharmacodynamics of bumetanide in neonates treated with extracorporeal membrane oxygenation. J Pediatr. (1992) 121:974–80. doi: 10.1016/S0022-3476(05)80355-5
149. Pokorná P, Šíma M, Vobruba V, Bašková M, Posch L, Slanar O. Sufentanil pharmacokinetics in a full-term neonate treated with extracorporeal membrane oxygenation: a case report. Perfusion. 2019:0267659118824011. doi: 10.1177/0267659118824011
150. Wishart DS, Knox C, Guo AC, Cheng D, Shrivastava S, Tzur D, et al. DrugBank: a knowledgebase for drugs, drug actions and drug targets. Nucleic Acids Res. (2008) 36 (suppl. 1):D901–6. doi: 10.1093/nar/gkm958
151. Wyatt RG, Okamoto GA, Feigin RD. Stability of antibiotics in parenteral solutions. Pediatrics. (1972) 49:22–29.
152. Cies JJ, Moore WS, Dickerman MJ, Small C, Carella D, Chopra A, et al. Pharmacokinetics of continuous-infusion meropenem in a pediatric patient receiving extracorporeal life support. Pharmacotherapy. (2014) 34:e175–9. doi: 10.1002/phar.1476
153. Tripathi N, Cotten CM, Smith PB. Antibiotic use and misuse in the neonatal intensive care unit. Clin Perinatol. (2012) 39:61–68. doi: 10.1016/j.clp.2011.12.003
154. Hoie EB, Swigart SA, Leuschen MP, Willett LD, Bolam DL, Goodrich PD, et al. Vancomycin pharmacokinetics in infants undergoing extracorporeal membrane oxygenation. Clin Pharm. (1990) 9:711–5.
155. Amaker RD, DiPiro JT, Bhatia J. Pharmacokinetics of vancomycin in critically ill infants undergoing extracorporeal membrane oxygenation. Antimicrob Agents Chemother. (1996) 40:1139–42. doi: 10.1128/AAC.40.5.1139
156. Buck ML. Vancomycin pharmacokinetics in neonates receiving extracorporeal membrane oxygenation. Pharmacotherapy. (1998) 18:1082–6.
157. Mulla H, Pooboni S. Population pharmacokinetics of vancomycin in patients receiving extracorporeal membrane oxygenation. Br J Clin Pharmacol. (2005) 60:265–75. doi: 10.1111/j.1365-2125.2005.02432.x
158. Gwee A, Cranswick N, McMullan B, Perkins E, Bolisetty S, Gardiner K, et al. Continuous versus intermittent vancomycin infusions in infants: a randomized controlled trial. Pediatrics. (2019) 143:e20182179. doi: 10.1542/peds.2018-2179
159. Wi J, Noh H, Min KL, Yang S, Jin BH, Hahn J, et al. Population pharmacokinetics and dose optimization of teicoplanin during venoarterial extracorporeal membrane oxygenation. Antimicrob Agents Chemother. (2017) 61:e01015–17. doi: 10.1128/AAC.01015-17
160. Karadag-Oncel E, Ceyhan M. Oseltamivir in neonates, infants and young children: a focus on clinical pharmacology. Infect Disord Drug Targets. (2013) 13:15–24. doi: 10.2174/18715265112129990004
161. Food and Drug Administration. Tamiflu (oseltamivir phosphate) Information. Available online at: https://www.fda.gov/drugs/postmarket-drug-safety-information-patients-and-providers/tamiflu-oseltamivir-phosphate-information (accessed May 2, 2019).
162. Eyler RF, Heung M, Pleva M, Sowinski KM, Park PK, Napolitano LM, et al. Pharmacokinetics of oseltamivir and oseltamivir carboxylate in critically ill patients receiving continuous venovenous hemodialysis and/or extracorporeal membrane oxygenation. Pharmacotherapy. (2012) 32:1061–9. doi: 10.1002/phar.1151
163. Lemaitre F, Luyt CE, Roullet-Renoleau F, Nieszkowska A, Zahr N, Corvol E, et al. Impact of extracorporeal membrane oxygenation and continuous venovenous hemodiafiltration on the pharmacokinetics of oseltamivir carboxylate in critically ill patients with pandemic (H1N1) influenza. Therapeut Drug Monitor. (2012) 34:171–5. doi: 10.1097/FTD.0b013e318248672c
164. Zeilmaker GA, Pokorna P, Mian P, Wildschut ED, Knibbe CAJ, Krekels EHJ, et al. Pharmacokinetic considerations for pediatric patients receiving analgesia in the intensive care unit; targeting postoperative, ECMO and hypothermia patients. Expert Opin Drug Metab Oxicol. (2018) 14:417–28. doi: 10.1080/17425255.2018.1461836
165. Smits A, De Cock P, Vermeulen A, Allegaert K. Physiologically based pharmacokinetic (PBPK) modeling and simulation in neonatal drug development: how clinicians can contribute. Expert Opin Drug Metab Toxicol. (2019) 15:25–34. doi: 10.1080/17425255.2019.1558205
166. Watt KM, Cohen-Wolkowiez M, Barrett JS, Sevestre M, Zhao P, Brouwer KLR, et al. Physiologically based pharmacokinetic approach to determine dosing on extracorporeal life support: fluconazole in children on ECMO. CPT. (2018) 7:629–37. doi: 10.1002/psp4.12338
167. Michelet R, Bocxlaer JV, Vermeulen A. PBPK in preterm and term neonates: a review. Curr Pharm Design. (2017) 23:5943–54. doi: 10.2174/1381612823666171009143840
168. Yellepeddi V, Rower J, Liu X, Kumar S, Rashid J, Sherwin CM. State-of-the-art review on physiologically based pharmacokinetic modeling in pediatric drug development. Clin Pharmacokinet. (2018) 58:1–13. doi: 10.1007/s40262-018-0677-y
169. Anderson BJ, Merry AF. Data sharing for pharmacokinetic studies. Pediatr Anesth. (2009) 19:1005–10. doi: 10.1111/j.1460-9592.2009.03051.x
170. Rasool MF, Khalil F, Läer S. A Physiologically based pharmacokinetic drug–disease model to predict carvedilol exposure in adult and paediatric heart failure patients by incorporating pathophysiological changes in hepatic and renal blood flows. Clin Pharmacokinet. (2015) 54:943–62. doi: 10.1007/s40262-015-0253-7
171. T'jollyn H, Vermeulen A, Van Bocxlaer J, Colin P. A physiologically based pharmacokinetic perspective on the clinical utility of albumin-based dose adjustments in critically ill patients. Clin Pharmacokinet. (2018) 57:59–69. doi: 10.1007/s40262-017-0549-x
172. Coppini R, Simons SH, Mugelli A, Allegaert K. Clinical research in neonates and infants: challenges and perspectives. Pharmacol Res. (2016) 108:80–7. doi: 10.1016/j.phrs.2016.04.025
Keywords: ECMO, pharmacokinetics, pharmacodynamics, critical illness, developmental pharmacology, neonate
Citation: Raffaeli G, Pokorna P, Allegaert K, Mosca F, Cavallaro G, Wildschut ED and Tibboel D (2019) Drug Disposition and Pharmacotherapy in Neonatal ECMO: From Fragmented Data to Integrated Knowledge. Front. Pediatr. 7:360. doi: 10.3389/fped.2019.00360
Received: 21 May 2019; Accepted: 16 August 2019;
Published: 03 September 2019.
Edited by:
Paolo Biban, Integrated University Hospital Verona, ItalyReviewed by:
Gail Mary Annich, Hospital for Sick Children, CanadaRavi R. Thiagarajan, Boston Children's Hospital and Harvard Medical School, United States
Copyright © 2019 Raffaeli, Pokorna, Allegaert, Mosca, Cavallaro, Wildschut and Tibboel. This is an open-access article distributed under the terms of the Creative Commons Attribution License (CC BY). The use, distribution or reproduction in other forums is permitted, provided the original author(s) and the copyright owner(s) are credited and that the original publication in this journal is cited, in accordance with accepted academic practice. No use, distribution or reproduction is permitted which does not comply with these terms.
*Correspondence: Genny Raffaeli, Z2VubnkucmFmZmFlbGkmI3gwMDA0MDtnbWFpbC5jb20=