- 1Department of Neonatology, Wilhelmina Children’s Hospital, University Medical Center Utrecht, Utrecht, Netherlands
- 2Monash Newborn, Monash Medical Centre, Melbourne, VIC, Australia
Cerebral oxygenation is not always reflected by systemic arterial oxygenation. Therefore, regional cerebral oxygen saturation (rScO2) monitoring with near-infrared spectroscopy (NIRS) is of added value in neonatal intensive care. rScO2 represents oxygen supply to the brain, while cerebral fractional tissue oxygen extraction, which is the ratio between rScO2 and systemic arterial oxygen saturation, reflects cerebral oxygen utilization. The balance between oxygen supply and utilization provides insight in neonatal cerebral (patho-)physiology. This review highlights the potential and limitations of cerebral oxygenation monitoring with NIRS in the neonatal intensive care unit.
Introduction
It has been nearly 8 years, since our research group published a review on the value and pitfalls of cerebral oxygenation monitoring with near-infrared spectroscopy (NIRS) in neonatology (1). Since then, research into cerebral NIRS has taken an impressive flight. The importance of cerebral oxygenation and perfusion monitoring has been increasingly recognized in neonatal intensive care. In this review, the development of cerebral NIRS monitoring over the past years is summarized.
Value of Cerebral Oxygenation Monitoring
Unfortunately, brain injury is still common in preterm neonates and can lead to a wide range of complications later in life, such as behavioral, attentional, cognitive, sensorimotor or language impairments, and epilepsy (2). Both the increasing number of preterm infants and improved survival rates contribute to the prevalence of neonatal brain injury (3, 4). Preterm infants are particularly susceptible to brain injury as the brain undergoes rapid development during the last trimester of pregnancy. During this period, the brain does not only increase in volume but also undergoes increasing gyri- and sulcification and myelination and improves connectivity (5). Pre-oligodendrocytes and axons mature, the transient subplate neurons appear, and the cerebellum develops and matures. Throughout this process, the brain is using substantial amounts of oxygen (2, 6, 7). Cerebral pathology can present as white matter injury, such as periventricular leukomalacia, or as periventricular–intraventricular hemorrhage (PIVH) (2). Inadequate or fluctuating cerebral perfusion and oxygenation can result in brain injury (8). Hyperoxia, hypoxia, and fluctuations in cerebral oxygenation, indicative of poor cerebral autoregulation, can adversely affect brain development (9–12).
Vital parameters such as blood pressure, heart rate, and pulse oximetry [arterial oxygen saturation (SaO2)] are important to assess the condition of the neonate but do not directly assess brain oxygenation (13, 14). NIRS-monitored regional cerebral oxygen saturation (rScO2) is a non-invasive and elegant method to monitor global brain oxygenation. rScO2 monitoring can be used at bedside for extended periods of time (up to several days) without side effects. Other methods that examine the brain, such as cranial ultrasound or MRI, do not allow for continuous monitoring. NIRS can be used even in the sickest infants and requires minimal handling of the infant. The device can be used at bedside in the NICU as well as during surgery or transportation (15). NIRS can easily be combined with monitoring of cerebral electrical activity by amplitude-integrated electro-encephalography (aEEG).
NIRS Technique
The NIRS technique is based on the relative transparency of biological tissue to light. Neonatal cerebral tissue can easily be penetrated by NIR light (700–1,000 nm) due to thin overlaying layers of skin and skull. An emitter sends light of the near-infrared spectrum through cerebral tissue in a semi-curved shape to a detector, approximately 2–3 cm in depth (16). Oxygenated (O2Hb) and deoxygenated (HHb) hemoglobin absorb the NIR light at different wavelengths, together accounting for total Hb (THb = O2Hb + HHb). Differences in NIR light absorption are detected by the sensor and used to calculate the concentrations of Ohb and HHb according to the modified law of Lambert–Beer. The ratio between O2Hb and HHb is expressed as the rScO2 or tissue oxygenation index (TOI), depending on the manufacturer of the NIRS device. Previous research has shown good correlation between TOI and rScO2 (17, 18). The NIR light is absorbed by HHb and O2Hb in both arterial and venous vessels, in a 25:75% ratio, and thus NRS reflects mainly cerebral venous oxygen saturation (19). The NIR light is absorbed by both superficial tissues and the cerebral cortex. When two or more detectors are used, the deeper signal reflecting cerebral cortex oxygenation can be distinguished from the superficial signal, reducing the influence of scattering. The technical details of NIRS are beyond the scope of this review but are well described elsewhere (20–23). Most commercially available devices utilize the continuous-wave technique, which measures the attenuation (or absorption) of NIR light from a continuous light source to calculate oxygenation (24). Other NIRS techniques, such as time-resolved spectroscopy and frequency-resolved spectroscopy, are now able to assess cerebral blood volume and quantify absolute concentrations of HHb and O2Hb, respectively. However, these techniques have not yet been proved practically useful in neonatal care (24).
Sensors and Devices
Today, there are several different NIRS devices and sensors commercially available. A number of comparative studies have shown that the overall correlation between NIRS devices is acceptable, although they differ in technique and algorithm (25–27). Smaller and more flexible sensors have been designed for neonatal use. However, these neonatal sensors measure 10% higher compared to the adult sensors (28, 29). Since the upper limit of most devices is set to 95%, high cerebral oxygenation values as measured by the neonatal sensors are shown as a flat line in which all variation is lost, as demonstrated in Figure 1A. NIRS device and sensor type must be taken into account when NIRS monitoring of cerebral oxygenation is applied in clinical care. Reference values for the neonatal sensor have been published (see below) (29).
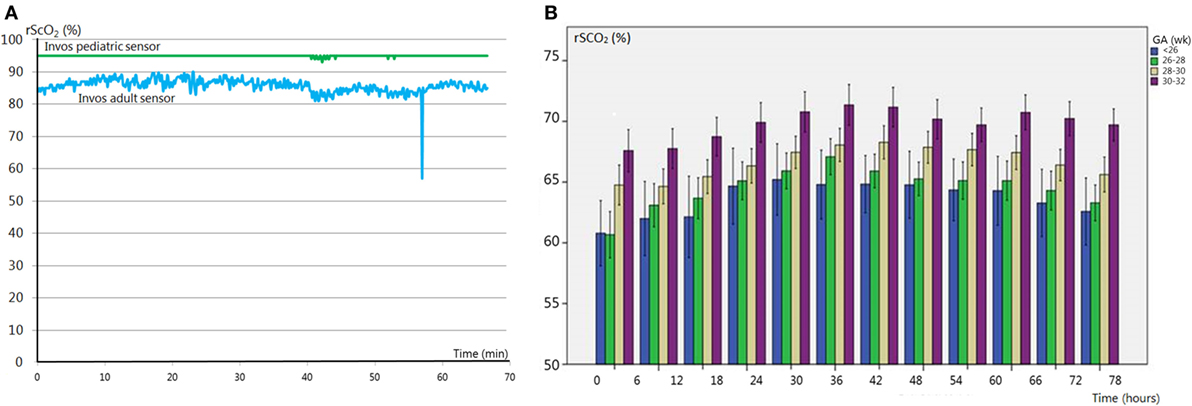
Figure 1. (A) Regional cerebral oxygen saturation (rScO2) monitored with an adult (blue line) and pediatric (green line) sensor. Hyperoxia values are untraceable with the pediatric sensor due to the cutoff value of 95%. (B) Reference values [stratified for gestational age (GA)] of rScO2 in premature infants (GA < 32 weeks). Adapted from Ref. (29).
Validation
Regional cerebral oxygen saturation represents a mixed saturation largely determined by the venous component (75%), which is why NIRS validation studies have often focused on venous saturation (19). However, venous saturation does not reflect mixed arterial and venous saturation as NIRS does, and there is no “gold standard” to measure venous oxygen saturation (30). A good correlation has been reported between oxygen saturation in the jugular vein and NIRS-monitored cerebral oxygenation, with a mean difference of 5%, for different manufacturers (Hamamatsu, INVOS, CAS-MED) (31–34). However, the difference between jugular venous oxygen saturation and regional cerebral oxygenation may increase during hypoxia. This is presumably caused by an increased arterial contribution to the NIRS signal due to cerebral arterial vasodilatation as a response to hypoxia (35). Cerebral fractional tissue oxygen extraction (cFTOE) has been validated against central cerebral venous saturation in newborn piglets (36). Brain perfusion assessment with NIRS has been compared to perfusion assessment with MRI, which has shown strong correlations (37, 38). Both rScO2 and TOI have shown good reproducibility (39, 40).
Reference Values
Several studies have analyzed changes in rScO2 with advancing postnatal age. rScO2 is between approximately 40 and 56% directly after birth (irrespective of delivery mode) (41–43), increases up to 78% in the first 2 days after birth (44) and then slowly stabilizes during 3–6 weeks after birth with values between 55 and 85% (45–47). Several studies have published reference ranges immediately after birth, which show a gradual increase during the first 15 min of life (42, 46). A recent study by Alderliesten et al. provides reference values based on a large study cohort during the first 72 h of life in preterm infants [<32 weeks gestational age (GA); n = 999]. The data are converted into reference curves stratified for different GAs which can be used for cot side interpretation of rScO2 and cFTOE values, as shown in Figure 1B (29). These reference values, obtained with the (small) adult sensor (SomaSensor SAFB-SM, Covidien, Mansfield, MA, USA), will facilitate clinical application of cerebral oxygenation monitoring. As stated above, it is important to realize that neonatal sensors of various NIRS manufacturers display higher values (up to 10%) as compared to adult sensors (28).
Clinical Application
Gaining insight into the oxygenation of the neonatal brain can be of important clinical value, as a large share of neonatal pathology is brain associated. NIRS monitoring of cerebral oxygenation can be considered in several clinical situations as outlined below.
Cerebral Oxygenation and the Patent Ductus Arteriosus
The hemodynamically significant patent ductus arteriosus (PDA) remains a controversial topic. Clinicians and researchers are still debating whether or not it should be treated, what the best treatment strategy is and when would be the best time to intervene (48–51). Unfortunately, the brain is rarely included in this discussion. A PDA can negatively influence cerebral oxygenation. Shunting of the blood through the duct away from the brain has a profound negative effect on rScO2. This effect is independent from SaO2, which remains within normal limits during a PDA (13, 52). Cerebral oxygenation normalizes after ductal closure (13, 52). The ductal diameter is associated with cerebral oxygenation, where a larger diameter (indicating a significant left to right ductal shunt) is associated with lower rScO2 (52). Infants who need surgical PDA closure are often exposed to low rScO2 values for a longer period of time, as shown in Figure 2A, and are therefore at risk of cerebral injury (14). Additionally, a further reduction in cerebral oxygenation occurs during ductal surgery (53, 54). Weisz et al. reported an increased risk of neurodevelopmental impairment in infants after surgical ductal ligation compared to pharmaceutically treated infants (55). More specifically, underdevelopment of the cerebellar structure has been reported in infants who needed surgical closure (14). Extended episodes of low cerebral oxygenation are most likely responsible for this phenomenon (14).
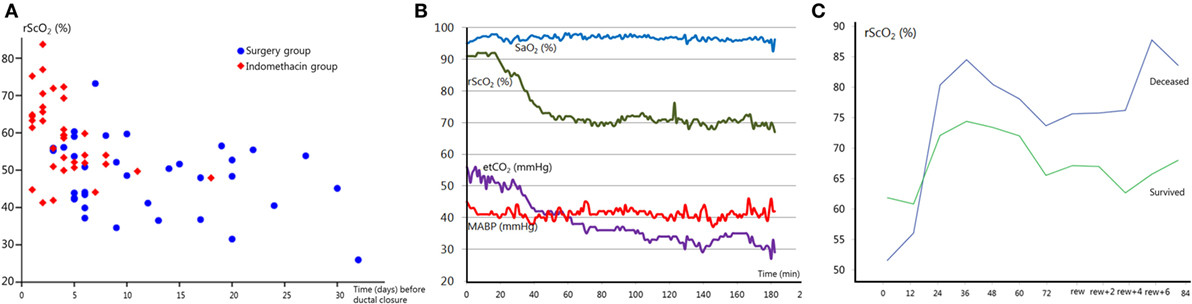
Figure 2. (A) Regional cerebral oxygen saturation (rScO2) just before ductal closure in patients treated with indomethacin (red squares) or surgery (blue circles) as a function of postnatal age in days. Note that the majority of infants requiring surgical treatment are exposed to the lowest rScO2 values for a longer period. Adapted from Ref. (14). (B) Acute end-tidal CO2 (etCO2) decrease results in a subsequent reduction in rScO2, on the contrary arterial oxygen saturation (SaO2) remains stable. MABP, mean arterial blood pressure. (C) rScO2 during hypothermia and after rewarming (rew) in two severely asphyxiated infants. The infant with an adverse outcome (blue line) showed higher rScO2 values compared to the infant that survived (green line). Cerebral fractional tissue oxygen extraction values (not shown) mirrored rScO2 values.
Cerebral Oxygenation and Respiration
Preterm infants often require respiratory support, which can affect cerebral hemodynamics and cerebral oxygenation (56, 57). An earlier study reported NIRS-monitored changes in cerebral blood flow (CBF) during continuous positive airway pressure and artificial ventilation. CBF significantly correlated with the type of respiratory support, leading to the conclusion that ventilation can impact cerebral circulation (58). Cerebral oxygenation can also be affected by the type of ventilation support during surgery (59).
Ventilation is the main regulatory mechanism of arterial carbon dioxide pressure (pCO2). pCO2 can affect the brain by altering cerebral arterial vessel diameter, where hypercapnia can induce cerebral vasodilatation and hypocapnia induces vasoconstriction (60). As such, pCO2 can affect cerebral perfusion and oxygenation, and both hyper- and hypocapnia have been associated with neuropathology (61, 62). An increase in pCO2 is accompanied by an increase in cerebral oxygen saturation with a decrease in oxygen extraction (63, 64). Acute fluctuations in pCO2, even within the normal range, appear to directly affect the neonatal brain perfusion (personal communication). The pCO2-induced changes in cerebral perfusion and oxygenation can be monitored by NIRS, as shown in Figure 2B, in order to identify and prevent pCO2-induced cerebral hypo- or hyperperfusion and brain damage.
Other factors related to respiration have also been shown to influence cerebral oxygenation. Apneas, for example, can affect brain oxygenation, and high mean airway pressure during artificial ventilation can also reduce cerebral oxygenation (1, 65–67). Also, infants with respiratory distress syndrome (RDS) have lower cerebral oxygenation and increased variance in rScO2 and cFTOE during the first 3 days after birth (68, 69). Moreover, they often have an impaired cerebral autoregulation, which may further predispose them to cerebral injury (70). Combining arterial blood pressure and cerebral oxygenation measures can help to identify (lack of) cerebral autoregulation (see below).
Cerebral Oxygenation and Autoregulation
Cerebral autoregulation is the ability to maintain stable cerebral perfusion and oxygenation during fluctuations in blood pressure (71). Hypotension can cause a severe reduction in cerebral perfusion and impairment of cerebral autoregulation, leading to inadequate perfusion (72). Combining rScO2-monitoring with arterial blood pressure monitoring enables assessment of cerebral autoregulation (73). Prematurity is a risk factor for impaired autoregulation, and even small variations in blood pressure can affect cerebral oxygenation in clinically sick and unstable babies (48, 74). Cerebral autoregulation can indeed be affected in several clinical situations that are commonly seen in preterm infants. Our group has previously demonstrated that RDS predisposes for lack of cerebral autoregulation (70). Autoregulation might also be impaired during surgery and high concentrations of positive inotropes such as dopamine (11, 49). Impaired autoregulation has been linked to poor neurodevelopmental outcome (50). Evaluating cerebral autoregulation at bedside can identify episodes of impaired autoregulation, and appropriate measures can be initiated to stabilize cerebral perfusion and oxygenation. Cerebral autoregulation can be computed in different ways, and software to calculate autoregulation at bedside is currently being developed (51). In summary, cerebral autoregulation may become impaired, especially in the unstable (preterm) infant, predisposing these neonates to brain injury. This underlines the importance of cerebral oxygenation an autoregulation monitoring in the early neonatal period (75).
Cerebral Oxygenation and Hypotension
Cerebral oxygenation can play an important role in assessing hypotension and whether positive inotropic therapy is indicated. There is an increasing awareness that the current definitions of hypotension of prematurity do not always reflect true hypotension. Permissive hypotension is increasingly accepted, unless there are (clinical) signs of hypoperfusion (76–78). As already stated above, hypotensive treatment is not without side effects and may have adverse effects on outcome (11, 79). Cerebral oxygenation plays an important role as a marker of end-organ oxygenation and can help making decisions whether or not treatment for hypotension is indicated. Other parameters such as blood gasses, urine production, and capillary refill should be taken into account. Identifying small reductions in blood pressure that do not affect cerebral oxygenation, and systemic perfusion might prevent unnecessary treatment with inotropes (78, 80). Monitoring rScO2 and cerebral autoregulation during neonatal surgery is important to prevent hypotension-related injury to the immature brain (see also below) (81). Our research group is currently involved in a prospective study (the TOHOP study) to find an answer to the question at which stage hypotension treatment is warranted (TOHOP; http://ClinicalTrials.gov identifier: NCT01434251).
Cerebral Oxygenation and Small-for-Gestational-Age (SGA) Neonates
Preterm infants who are born SGA show higher cerebral oxygenation during the first postnatal days (29, 82). This is most likely related to the prenatal blood flow redistribution of the intrauterine growth restricted (IUGR) fetus, in an attempt to preserve oxygen supply to the brain (brain sparing effect) (83). However, this does not necessarily protect against cerebral injury, and infants born following IUGR are at an increased risk of neurodevelopmental impairment (84, 85). In case of a PDA, SGA infants demonstrated a significantly larger fall in cerebral oxygenation, as compared to AGA infants (86).
Cerebral Oxygenation and Neurodevelopmental Outcome
Disturbances in cerebral perfusion and oxygenation are major contributors to neonatal brain injury, increasing the risk of impaired neurodevelopmental outcome (8, 87). Infants are particularly susceptible to brain injury during the first 3 days after birth, when major hemodynamic transitional changes occur. A large international randomized controlled trial, the SafeboosC study (Safeguarding the brains of out smallest children), has investigated whether it is possible to reduce the hypoxic and/or hyperoxic burden on the immature brain with cerebral oxygenation monitoring, in order to prevent neurological damage and to improve outcome (88). The study has shown that disturbances in cerebral oxygenation could be identified with NIRS. A treatment protocol prescribed treatment steps to restore normal brain oxygenation. The burden of hypoxia (and hyperoxia), as expressed by the percentage of time spend outside the normal range of rScO2 (55–85%), was significantly lower in the group with (visible) NIRS monitoring as compared to the blinded control group (median 36.1 vs. 81.3%) (47). This difference was mainly due to a reduction in hypoxic episodes.
Impaired cerebral oxygenation below the threshold of 55% appears to affect neurodevelopmental outcome at 15 and 24 months corrected age (personal communication). Poor cerebral autoregulation, examined by the correlation between rScO2 and arterial blood pressure, has been be associated with an increased risk score predictive of neonatal mortality and morbidity (CRIB II) (89).
Several studies, in newborn animals and humans, showed that rScO2 values consistently below 40% (measured with adult sensors) are related to brain damage (90–92). Other clinical studies showed that low cerebral oxygen saturation immediately after birth (<15 min) is associated with PIVH (93). In accordance with these results, low cerebral oxygenation during the first 48 h after birth was associated with death or severe PIVH in a study by Cerbo et al. (94). Similarly, increased oxygen extraction cFTOE can precede development of PIVH (95, 96).
Cerebral Oxygenation and Red Blood Cell Transfusions
Several studies have shown a significant increase in cerebral oxygenation after red blood cell transfusions in anemic infants (97, 98). The infants with the lowest pre-transfusion rScO2 values seem to benefit the most from transfusions (99). Similarly, high cFTOE levels (>0.4) can indicate an imbalance between cerebral oxygen supply and demand, which may underline the need for red blood cell transfusion (100). This indicates that cerebral oxygenation monitoring might be useful as a marker to identify infants with high cFTOE and/or low rScO2 who might benefit from blood transfusions (100–102).
Cerebral Oxygenation and Neonatal Surgery
Infants with cardiac or non-cardiac anomalies may require major surgery in the first few months after birth (103). Exposure to neonatal surgery can put the immature brain at risk (104, 105). An increased risk of neurodevelopmental delay after neonatal surgery has indeed been reported (106, 107). Both the procedure as well as anesthetics can be harmful (108–110). Monitoring cerebral oxygenation during surgery to increase cerebral safety is therefore advised (111–116). Perioperative monitoring evaluates brain oxygenation pre- and postsurgery, while intraoperative monitoring can assist surgeons and anesthesiologists to optimize cerebral oxygenation during the procedure to protect the neonatal brain (113, 117, 118). During surgery, cerebral NIRS can detect episodes of hypoxia more reliably than arterial SaO2 monitoring (114, 119). Introduction of cerebral oxygenation monitoring during cardiac surgery has improved intraoperative transfusion management (120). Cerebral oxygenation monitoring can also reflect changes in vital parameters during cardio-pulmonary bypass (121).
Cerebral Oxygenation and Hypoxic-Ischemic Encephalopathy (HIE)
Previous studies have demonstrated that rScO2 is increased and cFTOE is decreased during the first days after severe birth asphyxia, and these findings have been correlated with an adverse outcome at 2 years of age (Griffiths Mental Developmental scales) (122, 123) (see also Figure 2C). NIRS monitoring combined with simultaneous assessment of aEEG background patterns has a strong prognostic value for long-term neurodevelopmental outcome. High cerebral oxygenation with an abnormal aEEG background pattern (low electrical activity) in severely asphyxiated neonates with hypothermia treatment at 12 h of age has a positive predictive value of 91%, absence of these results in a negative predictive value of 100% (123). These findings strongly suggest that NIRS monitoring of cerebral oxygenation can have an important role in the (early) prognosis of neurodevelopmental outcome. Cerebral hyperoxygenation in neonates with an adverse outcome is most likely explained by low energy metabolism after severe brain injury with low oxygen utilization, cerebral hyperperfusion, and impaired autoregulation of the cerebral vascular bed (124, 125). These findings have been confirmed in other studies, incorporating MRI (126). Cerebral oxygenation with NIRS correlates strongly with CBF as assessed by arterial spin labeled MRI in infants with severe HIE (37).
Limitations
Hair, dark skin, and interfering light from other sources such as phototherapy devices can pose a problem during NIRS monitoring (1). Subdural edema or hematoma below the sensor might also interfere with measurements (127) in small infants, placement of the electrode might be challenging if they also require simultaneous aEEG monitoring. The curvature of the skull and head circumference has been mentioned as potential limitations (24). However, Alderliesten et al. did not find a correlation between head circumference and rScO2, stating that influence of head curvature seems unlikely (29). As previously discussed, type of NIRS device and sensor must be taken into account when interpreting cerebral oxygenation values (28).
Conclusion
Injury to the immature brain remains a major contributor to neonatal mortality and morbidity. Monitoring vital parameters provides us with critical information concerning the condition of the infant but does not offer direct information regarding brain oxygenation and perfusion. Cerebral oxygenation monitoring with NIRS, at least during the vulnerable transition period throughout the first 3 days after birth, provides the clinician with additional important information. Several clinical conditions can affect brain oxygenation, and studies have shown that systemic oxygen saturation does not always reflect cerebral oxygenation. The assessment of neonatal brain oxygenation (and perfusion) can be extremely useful in the clinical setting. It has the potential to guide clinical management in order to prevent brain injury and to avoid unnecessary treatment. It may also provide important information regarding the infant’s prognosis.
Author Contributions
LD determined review subjects, collected, analyzed, and included the literature, drafted the initial manuscript, and approved the final manuscript and is accountable for all aspects of the manuscript. FvB and PL determined review subjects, interpreted the literature, reviewed and revised the manuscript, and approved the final manuscript and are accountable for all aspects of the manuscript.
Conflict of Interest Statement
The authors declare that the research was conducted in the absence of any commercial or financial relationships that could be construed as a potential conflict of interest.
Funding
LD was supported by the Biltema Foundation (http://www.biltemafoundation.org) and the “Vrienden van het Wilhelmina Kinderziekenhuis” (friends of the Wilhelmina Children’s Hospital; http://www.vriendenwkz.nl) Foundation.
Abbreviations
aEEG, amplitude-integrated electro-encephalography; cFTOE, cerebral fractional tissue oxygen extraction; NIRS, near-infrared spectroscopy; PDA, hemodynamically significant patent ductus arteriosus; PIVH, periventricular–intraventricular hemorrhage; RDS, respiratory distress syndrome; rScO2, regional cerebral oxygen saturation; SaO2, arterial oxygen saturation; TOI, tissue oxygenation index.
References
1. van Bel F, Lemmers P, Naulaers G. Monitoring neonatal regional cerebral oxygen saturation in clinical practice: value and pitfalls. Neonatology (2008) 94(4):237–44. doi: 10.1159/000151642
2. Volpe JJ. Brain injury in premature infants: a complex amalgam of destructive and developmental disturbances. Lancet Neurol (2009) 8(1):110–24. doi:10.1016/S1474-4422(08)70294-1
3. Martin JA, Hamilton BE, Osterman MJ. Births in the United States, 2015. NCHS Data Brief (2016) 258:1–8.
4. Younge N, Smith PB, Gustafson KE, Malcolm W, Ashley P, Cotten CM, et al. Improved survival and neurodevelopmental outcomes among extremely premature infants born near the limit of viability. Early Hum Dev (2016) 95:5–8. doi:10.1016/j.earlhumdev.2016.01.015
5. Duerden EG, Taylor MJ, Miller SP. Brain development in infants born preterm: looking beyond injury. Semin Pediatr Neurol (2013) 20(2):65–74. doi:10.1016/j.spen.2013.06.007
6. Meerman RJ, van Bel F, van Zwieten PH, Oepkes D, den Ouden L. Fetal and neonatal cerebral blood velocity in the normal fetus and neonate: a longitudinal Doppler ultrasound study. Early Hum Dev (1990) 24(3):209–17. doi:10.1016/0378-3782(90)90028-H
7. Rudolph AM, Heymann MA. Circulatory changes during growth in the fetal lamb. Circ Res (1970) 26(3):289–99. doi:10.1161/01.RES.26.3.289
8. Khwaja O, Volpe JJ. Pathogenesis of cerebral white matter injury of prematurity. Arch Dis Child Fetal Neonatal Ed (2008) 93(2):F153–61. doi:10.1136/adc.2006.108837
9. Ferriero DM. Neonatal brain injury. N Engl J Med (2004) 351(19):1985–95. doi:10.1056/NEJMra041996
10. Zaghloul N, Nasim M, Patel H, Codipilly C, Marambaud P, Dewey S, et al. Overexpression of extracellular superoxide dismutase has a protective role against hyperoxia-induced brain injury in neonatal mice. FEBS J (2012) 279(5):871–81. doi:10.1111/j.1742-4658.2012.08478.x
11. Alderliesten T, Lemmers PM, Smarius JJ, van de Vosse RE, Baerts W, van Bel F. Cerebral oxygenation, extraction, and autoregulation in very preterm infants who develop peri-intraventricular hemorrhage. J Pediatr (2013) 162(4):698–704e2. doi:10.1016/j.jpeds.2012.09.038
12. Sola A, Golombek SG, Montes Bueno MT, Lemus-Varela L, Zuluaga C, Dominguez F, et al. Safe oxygen saturation targeting and monitoring in preterm infants: can we avoid hypoxia and hyperoxia? Acta Paediatr (2014) 103(10):1009–18. doi:10.1111/apa.12692
13. Lemmers PM, Toet MC, van Bel F. Impact of patent ductus arteriosus and subsequent therapy with indomethacin on cerebral oxygenation in preterm infants. Pediatrics (2008) 121(1):142–7. doi:10.1542/peds.2007-0925
14. Lemmers PM, Benders MJ, D’Ascenzo R, Zethof J, Alderliesten T, Kersbergen KJ, et al. Patent ductus arteriosus and brain volume. Pediatrics (2016) 137(4). doi:10.1542/peds.2015-3090
15. Kenosi M, Naulaers G, Ryan CA, Dempsey EM. Current research suggests that the future looks brighter for cerebral oxygenation monitoring in preterm infants. Acta Paediatr (2015) 104(3):225–31. doi:10.1111/apa.12906
16. Brown DW, Picot PA, Naeini JG, Springett R, Delpy DT, Lee TY. Quantitative near infrared spectroscopy measurement of cerebral hemodynamics in newborn piglets. Pediatr Res (2002) 51(5):564–70. doi:10.1203/00006450-200205000-00004
17. Thavasothy M, Broadhead M, Elwell C, Peters M, Smith M. A comparison of cerebral oxygenation as measured by the NIRO 300 and the INVOS 5100 near-infrared spectrophotometers. Anaesthesia (2002) 57(10):999–1006. doi:10.1046/j.1365-2044.2002.02826.x
18. Nagdyman N, Ewert P, Peters B, Miera O, Fleck T, Berger F. Comparison of different near-infrared spectroscopic cerebral oxygenation indices with central venous and jugular venous oxygenation saturation in children. Paediatr Anaesth (2008) 18(2):160–6. doi:10.1111/j.1460-9592.2007.02365.x
19. Watzman HM, Kurth CD, Montenegro LM, Rome J, Steven JM, Nicolson SC. Arterial and venous contributions to near-infrared cerebral oximetry. Anesthesiology (2000) 93(4):947–53. doi:10.1097/00000542-200010000-00012
20. Jobsis FF. Noninvasive, infrared monitoring of cerebral and myocardial oxygen sufficiency and circulatory parameters. Science (1977) 198(4323):1264–7. doi:10.1126/science.929199
21. Edwards AD, Wyatt JS, Richardson C, Delpy DT, Cope M, Reynolds EO. Cotside measurement of cerebral blood flow in ill newborn infants by near infrared spectroscopy. Lancet (1988) 2(8614):770–1. doi:10.1016/S0140-6736(88)92418-X
22. Wray S, Cope M, Delpy DT, Wyatt JS, Reynolds EO. Characterization of the near infrared absorption spectra of cytochrome aa3 and haemoglobin for the non-invasive monitoring of cerebral oxygenation. Biochim Biophys Acta (1988) 933(1):184–92. doi:10.1016/0005-2728(88)90069-2
23. McCormick PW, Stewart M, Goetting MG, Dujovny M, Lewis G, Ausman JI. Noninvasive cerebral optical spectroscopy for monitoring cerebral oxygen delivery and hemodynamics. Crit Care Med (1991) 19(1):89–97. doi:10.1097/00003246-199101000-00020
24. Wong F. Cerebral blood flow measurements in the neonatal brain. In: Walker DW, editor. Prenatal and Postnatal Determinants of Development. New York, NY: Springer (2016). p. 69–87.
25. Grubhofer G, Tonninger W, Keznickl P, Skyllouriotis P, Ehrlich M, Hiesmayr M, et al. A comparison of the monitors INVOS 3100 and NIRO 500 in detecting changes in cerebral oxygenation. Acta Anaesthesiol Scand (1999) 43(4):470–5. doi:10.1034/j.1399-6576.1999.430417.x
26. Cho H, Nemoto EM, Sanders M, Fernandez K, Yonas H. Comparison of two commercially available near-infrared spectroscopy instruments for cerebral oximetry. Technical note. J Neurosurg (2000) 93(2):351–4. doi:10.3171/jns.2000.93.2.0351
27. Yoshitani K, Kawaguchi M, Tatsumi K, Kitaguchi K, Furuya H. A comparison of the INVOS 4100 and the NIRO 300 near-infrared spectrophotometers. Anesth Analg (2002) 94(3):586–90. doi:10.1097/00000539-200203000-00020
28. Dix LM, van Bel F, Baerts W, Lemmers PM. Comparing near-infrared spectroscopy devices and their sensors for monitoring regional cerebral oxygen saturation in the neonate. Pediatr Res (2013) 74(5):557–63. doi:10.1038/pr.2013.133
29. Alderliesten T, Dix L, Baerts W, Caicedo A, van Huffel S, Naulaers G, et al. Reference values of regional cerebral oxygen saturation during the first 3 days of life in preterm neonates. Pediatr Res (2016) 79(1–1):55–64. doi:10.1038/pr.2015.186
30. Chieregato A, Calzolari F, Trasforini G, Targa L, Latronico N. Normal jugular bulb oxygen saturation. J Neurol Neurosurg Psychiatry (2003) 74(6):784–6. doi:10.1136/jnnp.74.6.784
31. Nagdyman N, Fleck T, Schubert S, Ewert P, Peters B, Lange PE, et al. Comparison between cerebral tissue oxygenation index measured by near-infrared spectroscopy and venous jugular bulb saturation in children. Intensive Care Med (2005) 31(6):846–50. doi:10.1007/s00134-005-2618-0
32. Daubeney PE, Pilkington SN, Janke E, Charlton GA, Smith DC, Webber SA. Cerebral oxygenation measured by near-infrared spectroscopy: comparison with jugular bulb oximetry. Ann Thorac Surg (1996) 61(3):930–4. doi:10.1016/0003-4975(95)01186-2
33. Rais-Bahrami K, Rivera O, Short BL. Validation of a noninvasive neonatal optical cerebral oximeter in veno-venous ECMO patients with a cephalad catheter. J Perinatol (2006) 26(10):628–35. doi:10.1038/sj.jp.7211573
34. Weiss M, Dullenkopf A, Kolarova A, Schulz G, Frey B, Baenziger O. Near-infrared spectroscopic cerebral oxygenation reading in neonates and infants is associated with central venous oxygen saturation. Paediatr Anaesth (2005) 15(2):102–9. doi:10.1111/j.1460-9592.2005.01404.x
35. Wong FY, Barfield CP, Campbell L, Brodecky VA, Walker AM. Validation of cerebral venous oxygenation measured using near-infrared spectroscopy and partial jugular venous occlusion in the newborn lamb. J Cereb Blood Flow Metab (2008) 28(1):74–80. doi:10.1038/sj.jcbfm.9600507
36. Naulaers G, Meyns B, Miserez M, Leunens V, Van Huffel S, Casaer P, et al. Use of tissue oxygenation index and fractional tissue oxygen extraction as non-invasive parameters for cerebral oxygenation. A validation study in piglets. Neonatology (2007) 92(2):120–6. doi:10.1159/000101063
37. Wintermark P, Hansen A, Warfield SK, Dukhovny D, Soul JS. Near-infrared spectroscopy versus magnetic resonance imaging to study brain perfusion in newborns with hypoxic-ischemic encephalopathy treated with hypothermia. Neuroimage (2014) 85(Pt 1):287–93. doi:10.1016/j.neuroimage.2013.04.072
38. Alderliesten T, De Vis JB, Lemmers PM, Hendrikse J, Groenendaal F, van Bel F, et al. Brain oxygen saturation assessment in neonates using T2-prepared blood imaging of oxygen saturation and near-infrared spectroscopy. J Cereb Blood Flow Metab (2016) 37(3):902–13. doi:10.1177/0271678X16647737
39. Menke J, Voss U, Moller G, Jorch G. Reproducibility of cerebral near infrared spectroscopy in neonates. Biol Neonate (2003) 83(1):6–11. doi:10.1159/000067006
40. Sorensen LC, Greisen G. Precision of measurement of cerebral tissue oxygenation index using near-infrared spectroscopy in preterm neonates. J Biomed Opt (2006) 11(5):054005. doi:10.1117/1.2357730
41. Almaazmi M, Schmid MB, Havers S, Reister F, Lindner W, Mayer B, et al. Cerebral near-infrared spectroscopy during transition of healthy term newborns. Neonatology (2013) 103(4):246–51. doi:10.1159/000345926
42. Urlesberger B, Kratky E, Rehak T, Pocivalnik M, Avian A, Czihak J, et al. Regional oxygen saturation of the brain during birth transition of term infants: comparison between elective cesarean and vaginal deliveries. J Pediatr (2011) 159(3):404–8. doi:10.1016/j.jpeds.2011.02.030
43. Baik N, Urlesberger B, Schwaberger B, Schmolzer GM, Mileder L, Avian A, et al. Reference ranges for cerebral tissue oxygen saturation index in term neonates during immediate neonatal transition after birth. Neonatology (2015) 108(4):283–6. doi:10.1159/000438450
44. Bailey SM, Hendricks-Munoz KD, Mally P. Cerebral, renal, and splanchnic tissue oxygen saturation values in healthy term newborns. Am J Perinatol (2014) 31(4):339–44. doi:10.1055/s-0033-1349894
45. McNeill S, Gatenby JC, McElroy S, Engelhardt B. Normal cerebral, renal and abdominal regional oxygen saturations using near-infrared spectroscopy in preterm infants. J Perinatol (2011) 31(1):51–7. doi:10.1038/jp.2010.71
46. Roche-Labarbe N, Carp SA, Surova A, Patel M, Boas DA, Grant PE, et al. Noninvasive optical measures of CBV, StO(2), CBF index, and rCMRO(2) in human premature neonates’ brains in the first six weeks of life. Hum Brain Mapp (2010) 31(3):341–52. doi:10.1002/hbm.20868
47. Hyttel-Sorensen S, Pellicer A, Alderliesten T, Austin T, van Bel F, Benders M, et al. Cerebral near infrared spectroscopy oximetry in extremely preterm infants: phase II randomised clinical trial. BMJ (2015) 350:g7635. doi:10.1136/bmj.g7635
48. Tsuji M, Saul JP, du Plessis A, Eichenwald E, Sobh J, Crocker R, et al. Cerebral intravascular oxygenation correlates with mean arterial pressure in critically ill premature infants. Pediatrics (2000) 106(4):625–32. doi:10.1542/peds.106.4.625
49. Brady KM, Mytar JO, Lee JK, Cameron DE, Vricella LA, Thompson WR, et al. Monitoring cerebral blood flow pressure autoregulation in pediatric patients during cardiac surgery. Stroke (2010) 41(9):1957–62. doi:10.1161/STROKEAHA.109.575167
50. Wong FY, Leung TS, Austin T, Wilkinson M, Meek JH, Wyatt JS, et al. Impaired autoregulation in preterm infants identified by using spatially resolved spectroscopy. Pediatrics (2008) 121(3):e604–11. doi:10.1542/peds.2007-1487
51. Caicedo A, Alderliesten T, Naulaers G, Lemmers P, van Bel F, Van Huffel S. A new framework for the assessment of cerebral hemodynamics regulation in neonates using NIRS. Adv Exp Med Biol (2016) 876:501–9. doi:10.1007/978-1-4939-3023-4_63
52. Dix L, Molenschot M, Breur J, de Vries W, Vijlbrief D, Groenendaal F, et al. Cerebral oxygenation and echocardiographic parameters in preterm neonates with a patent ductus arteriosus: an observational study. Arch Dis Child Fetal Neonatal Ed (2016). doi:10.1136/archdischild-2015-309192
53. Chock VY, Ramamoorthy C, Van Meurs KP. Cerebral oxygenation during different treatment strategies for a patent ductus arteriosus. Neonatology (2011) 100(3):233–40. doi:10.1159/000325149
54. Lemmers PM, Molenschot MC, Evens J, Toet MC, van Bel F. Is cerebral oxygen supply compromised in preterm infants undergoing surgical closure for patent ductus arteriosus? Arch Dis Child Fetal Neonatal Ed (2010) 95(6):F429–34. doi:10.1136/adc.2009.180117
55. Weisz DE, More K, McNamara PJ, Shah PS. PDA ligation and health outcomes: a meta-analysis. Pediatrics (2014) 133(4):e1024–46. doi:10.1542/peds.2013-3431
56. Cambonie G, Guillaumont S, Luc F, Vergnes C, Milesi C, Voisin M. Haemodynamic features during high-frequency oscillatory ventilation in preterms. Acta Paediatr (2003) 92(9):1068–73. doi:10.1111/j.1651-2227.2003.tb02579.x
57. Noone MA, Sellwood M, Meek JH, Wyatt JS. Postnatal adaptation of cerebral blood flow using near infrared spectroscopy in extremely preterm infants undergoing high-frequency oscillatory ventilation. Acta Paediatr (2003) 92(9):1079–84. doi:10.1111/j.1651-2227.2003.tb02581.x
58. Milan A, Freato F, Vanzo V, Chiandetti L, Zaramella P. Influence of ventilation mode on neonatal cerebral blood flow and volume. Early Hum Dev (2009) 85(7):415–9. doi:10.1016/j.earlhumdev.2009.01.008
59. Conforti A, Giliberti P, Landolfo F, Valfre L, Columbo C, Mondi V, et al. Effects of ventilation modalities on near-infrared spectroscopy in surgically corrected CDH infants. J Pediatr Surg (2016) 51(3):349–53. doi:10.1016/j.jpedsurg.2015.07.021
60. Hansen NB, Brubakk AM, Bratlid D, Oh W, Stonestreet BS. The effects of variations in PaCO2 on brain blood flow and cardiac output in the newborn piglet. Pediatr Res (1984) 18(11):1132–6. doi:10.1203/00006450-198411000-00015
61. Fabres J, Carlo WA, Phillips V, Howard G, Ambalavanan N. Both extremes of arterial carbon dioxide pressure and the magnitude of fluctuations in arterial carbon dioxide pressure are associated with severe intraventricular hemorrhage in preterm infants. Pediatrics (2007) 119(2):299–305. doi:10.1542/peds.2006-2434
62. McKee LA, Fabres J, Howard G, Peralta-Carcelen M, Carlo WA, Ambalavanan N. PaCO2 and neurodevelopment in extremely low birth weight infants. J Pediatr (2009) 155(2):217–21e1. doi:10.1016/j.jpeds.2009.02.024
63. Vanderhaegen J, Naulaers G, Vanhole C, De Smet D, Van Huffel S, Vanhaesebrouck S, et al. The effect of changes in tPCO2 on the fractional tissue oxygen extraction – as measured by near-infrared spectroscopy – in neonates during the first days of life. Eur J Paediatr Neurol (2009) 13(2):128–34. doi:10.1016/j.ejpn.2008.02.012
64. Victor S, Appleton RE, Beirne M, Marson AG, Weindling AM. Effect of carbon dioxide on background cerebral electrical activity and fractional oxygen extraction in very low birth weight infants just after birth. Pediatr Res (2005) 58(3):579–85. doi:10.1203/01.pdr.0000169402.13435.09
65. Henderson-Smart DJ, De Paoli AG. Methylxanthine treatment for apnoea in preterm infants. Cochrane Database Syst Rev (2010) 3:CD000140. doi:10.1002/14651858.CD000140
66. Petrova A, Mehta R. Near-infrared spectroscopy in the detection of regional tissue oxygenation during hypoxic events in preterm infants undergoing critical care. Pediatr Crit Care Med (2006) 7(5):449–54. doi:10.1097/01.PCC.0000235248.70482.14
67. Yamamoto A, Yokoyama N, Yonetani M, Uetani Y, Nakamura H, Nakao H. Evaluation of change of cerebral circulation by SpO2 in preterm infants with apneic episodes using near infrared spectroscopy. Pediatr Int (2003) 45(6):661–4. doi:10.1111/j.1442-200X.2003.01803.x
68. Kenosi M, O’Toole JM, Livingston V, Hawkes GA, Boylan GB, O’Halloran KD, et al. Effects of fractional inspired oxygen on cerebral oxygenation in preterm infants following delivery. J Pediatr (2015) 167(5):1007–12e1. doi:10.1016/j.jpeds.2015.07.063
69. Schwaberger B, Pichler G, Binder C, Avian A, Pocivalnik M, Urlesberger B. Even mild respiratory distress alters tissue oxygenation significantly in preterm infants during neonatal transition. Physiol Meas (2014) 35(10):2085–99. doi:10.1088/0967-3334/35/10/2085
70. Lemmers PM, Toet M, van Schelven LJ, van Bel F. Cerebral oxygenation and cerebral oxygen extraction in the preterm infant: the impact of respiratory distress syndrome. Exp Brain Res (2006) 173(3):458–67. doi:10.1007/s00221-006-0388-8
71. Paulson OB, Strandgaard S, Edvinsson L. Cerebral autoregulation. Cerebrovasc Brain Metab Rev (1990) 2(2):161–92.
72. Brady KM, Lee JK, Kibler KK, Smielewski P, Czosnyka M, Easley RB, et al. Continuous time-domain analysis of cerebrovascular autoregulation using near-infrared spectroscopy. Stroke (2007) 38(10):2818–25. doi:10.1161/STROKEAHA.107.485706
73. da Costa CS, Greisen G, Austin T. Is near-infrared spectroscopy clinically useful in the preterm infant? Arch Dis Child Fetal Neonatal Ed (2015) 100(6):F558–61. doi:10.1136/archdischild-2014-307919
74. Wong FY, Silas R, Hew S, Samarasinghe T, Walker AM. Cerebral oxygenation is highly sensitive to blood pressure variability in sick preterm infants. PLoS One (2012) 7(8):e43165. doi:10.1371/journal.pone.0043165
75. Soul JS, Hammer PE, Tsuji M, Saul JP, Bassan H, Limperopoulos C, et al. Fluctuating pressure-passivity is common in the cerebral circulation of sick premature infants. Pediatr Res (2007) 61(4):467–73. doi:10.1203/pdr.0b013e31803237f6
76. Dempsey EM, Barrington KJ. Evaluation and treatment of hypotension in the preterm infant. Clin Perinatol (2009) 36(1):75–85. doi:10.1016/j.clp.2008.09.003
77. Noori S, Seri I. Evidence-based versus pathophysiology-based approach to diagnosis and treatment of neonatal cardiovascular compromise. Semin Fetal Neonatal Med (2015) 20(4):238–45. doi:10.1016/j.siny.2015.03.005
78. Alderliesten T, Lemmers PM, van Haastert IC, de Vries LS, Bonestroo HJ, Baerts W, et al. Hypotension in preterm neonates: low blood pressure alone does not affect neurodevelopmental outcome. J Pediatr (2014) 164(5):986–91. doi:10.1016/j.jpeds.2013.12.042
79. Batton B, Li L, Newman NS, Das A, Watterberg KL, Yoder BA, et al. Early blood pressure, antihypotensive therapy and outcomes at 18-22 months’ corrected age in extremely preterm infants. Arch Dis Child Fetal Neonatal Ed (2016) 101(3):F201–6. doi:10.1136/archdischild-2015-308899
80. Binder-Heschl C, Urlesberger B, Schwaberger B, Koestenberger M, Pichler G. Borderline hypotension: how does it influence cerebral regional tissue oxygenation in preterm infants? J Matern Fetal Neonatal Med (2016) 29(14):2341–6. doi:10.3109/14767058.2015.1085020
81. Michelet D, Arslan O, Hilly J, Mangalsuren N, Brasher C, Grace R, et al. Intraoperative changes in blood pressure associated with cerebral desaturation in infants. Paediatr Anaesth (2015) 25(7):681–8. doi:10.1111/pan.12671
82. Cohen E, Baerts W, Alderliesten T, Derks J, Lemmers P, van Bel F. Growth restriction and gender influence cerebral oxygenation in preterm neonates. Arch Dis Child Fetal Neonatal Ed (2016) 101(2):F156–61. doi:10.1136/archdischild-2015-308843
83. Baschat AA, Harman CR. Antenatal assessment of the growth restricted fetus. Curr Opin Obstet Gynecol (2001) 13(2):161–8. doi:10.1097/00001703-200104000-00011
84. de Bie HM, Oostrom KJ, Delemarre-van de Waal HA. Brain development, intelligence and cognitive outcome in children born small for gestational age. Horm Res Paediatr (2010) 73(1):6–14. doi:10.1159/000271911
85. Baschat AA. Neurodevelopment after fetal growth restriction. Fetal Diagn Ther (2014) 36(2):136–42. doi:10.1159/000353631
86. Cohen E, Dix L, Baerts W, Alderliesten T, Lemmers P, van Bel F. Reduction in cerebral oxygenation due to patent ductus arteriosus is pronounced in small-for-gestational-age neonates. Neonatology (2016) 111(2):126–32. doi:10.1159/000448873
87. Vohr BR. Neurodevelopmental outcomes of extremely preterm infants. Clin Perinatol (2014) 41(1):241–55. doi:10.1016/j.clp.2013.09.003
88. Hyttel-Sorensen S, Austin T, van Bel F, Benders M, Claris O, Dempsey EM, et al. Clinical use of cerebral oximetry in extremely preterm infants is feasible. Dan Med J (2013) 60(1):A4533.
89. Mitra S, Czosnyka M, Smielewski P, O’Reilly H, Brady K, Austin T. Heart rate passivity of cerebral tissue oxygenation is associated with predictors of poor outcome in preterm infants. Acta Paediatr (2014) 103(9):e374–82. doi:10.1111/apa.12696
90. Hou X, Ding H, Teng Y, Zhou C, Tang X, Li S, et al. Research on the relationship between brain anoxia at different regional oxygen saturations and brain damage using near-infrared spectroscopy. Physiol Meas (2007) 28(10):1251–65. doi:10.1088/0967-3334/28/10/010
91. Kurth CD, McCann JC, Wu J, Miles L, Loepke AW. Cerebral oxygen saturation-time threshold for hypoxic-ischemic injury in piglets. Anesth Analg (2009) 108(4):1268–77. doi:10.1213/ane.0b013e318196ac8e
92. Dent CL, Spaeth JP, Jones BV, Schwartz SM, Glauser TA, Hallinan B, et al. Brain magnetic resonance imaging abnormalities after the Norwood procedure using regional cerebral perfusion. J Thorac Cardiovasc Surg (2006) 131(1):190–7. doi:10.1016/j.jtcvs.2005.10.003
93. Baik N, Urlesberger B, Schwaberger B, Schmolzer GM, Avian A, Pichler G. Cerebral haemorrhage in preterm neonates: does cerebral regional oxygen saturation during the immediate transition matter? Arch Dis Child Fetal Neonatal Ed (2015) 100(5):F422–7. doi:10.1136/archdischild-2014-307590
94. Cerbo RM, Scudeller L, Maragliano R, Cabano R, Pozzi M, Tinelli C, et al. Cerebral oxygenation, superior vena cava flow, severe intraventricular hemorrhage and mortality in 60 very low birth weight infants. Neonatology (2015) 108(4):246–52. doi:10.1159/000438452
95. Balegar KK, Stark MJ, Briggs N, Andersen CC. Early cerebral oxygen extraction and the risk of death or sonographic brain injury in very preterm infants. J Pediatr (2014) 164(3):475–80e1. doi:10.1016/j.jpeds.2013.10.041
96. Noori S, McCoy M, Anderson MP, Ramji F, Seri I. Changes in cardiac function and cerebral blood flow in relation to peri/intraventricular hemorrhage in extremely preterm infants. J Pediatr (2014) 164(2):e1–3. doi:10.1016/j.jpeds.2013.09.045
97. Koyano K, Kusaka T, Nakamura S, Nakamura M, Konishi Y, Miki T, et al. The effect of blood transfusion on cerebral hemodynamics in preterm infants. Transfusion (2013) 53(7):1459–67. doi:10.1111/j.1537-2995.2012.03953.x
98. Sandal G, Oguz SS, Erdeve O, Akar M, Uras N, Dilmen U. Assessment of red blood cell transfusion and transfusion duration on cerebral and mesenteric oxygenation using near-infrared spectroscopy in preterm infants with symptomatic anemia. Transfusion (2014) 54(4):1100–5. doi:10.1111/trf.12359
99. Seidel D, Blaser A, Gebauer C, Pulzer F, Thome U, Knupfer M. Changes in regional tissue oxygenation saturation and desaturations after red blood cell transfusion in preterm infants. J Perinatol (2013) 33(4):282–7. doi:10.1038/jp.2012.108
100. Andersen CC, Karayil SM, Hodyl NA, Stark MJ. Early red cell transfusion favourably alters cerebral oxygen extraction in very preterm newborns. Arch Dis Child Fetal Neonatal Ed (2015) 100(5):F433–5. doi:10.1136/archdischild-2014-307565
101. Banerjee J, Aladangady N. Biomarkers to decide red blood cell transfusion in newborn infants. Transfusion (2014) 54(10):2574–82. doi:10.1111/trf.12670
102. Mintzer JP, Parvez B, Chelala M, Alpan G, LaGamma EF. Monitoring regional tissue oxygen extraction in neonates <1250 g helps identify transfusion thresholds independent of hematocrit. J Neonatal Perinatal Med (2014) 7(2):89–100. doi:10.3233/NPM-1477213
103. Laing S, Walker K, Ungerer J, Badawi N, Spence K. Early development of children with major birth defects requiring newborn surgery. J Paediatr Child Health (2011) 47(3):140–7. doi:10.1111/j.1440-1754.2010.01902.x
104. Morriss FH Jr, Saha S, Bell EF, Colaizy TT, Stoll BJ, Hintz SR, et al. Surgery and neurodevelopmental outcome of very low-birth-weight infants. JAMA Pediatr (2014) 168(8):746–54. doi:10.1001/jamapediatrics.2014.307
105. McCann ME, Schouten AN, Dobija N, Munoz C, Stephenson L, Poussaint TY, et al. Infantile postoperative encephalopathy: perioperative factors as a cause for concern. Pediatrics (2014) 133(3):e751–7. doi:10.1542/peds.2012-0973
106. Stolwijk LJ, Lemmers PM, Harmsen M, Groenendaal F, de Vries LS, van der Zee DC, et al. Neurodevelopmental outcomes after neonatal surgery for major noncardiac anomalies. Pediatrics (2016) 137(2):e20151728. doi:10.1542/peds.2015-1728
107. Sakamoto T. Current status of brain protection during surgery for congenital cardiac defect. Gen Thorac Cardiovasc Surg (2016) 64(2):72–81. doi:10.1007/s11748-015-0606-z
108. Rhondali O, Juhel S, Mathews S, Cellier Q, Desgranges FP, Mahr A, et al. Impact of sevoflurane anesthesia on brain oxygenation in children younger than 2 years. Paediatr Anaesth (2014) 24(7):734–40. doi:10.1111/pan.12397
109. Davidson AJ. Anesthesia and neurotoxicity to the developing brain: the clinical relevance. Paediatr Anaesth (2011) 21(7):716–21. doi:10.1111/j.1460-9592.2010.03506.x
110. Brinkman EN, Stolwijk LJ, Lemmers PM, van Wolfswinkel L, Purvis P, Sury MR, et al. A survey of the dose of inhalational agents used to maintain anaesthesia in infants. Eur J Anaesthesiol (2016). doi:10.1097/EJA.0000000000000546
111. Vanderhaegen J, De Smet D, Meyns B, Van De Velde M, Van Huffel S, Naulaers G. Surgical closure of the patent ductus arteriosus and its effect on the cerebral tissue oxygenation. Acta Paediatr (2008) 97(12):1640–4. doi:10.1111/j.1651-2227.2008.01021.x
112. Tytgat SH, Stolwijk LJ, Keunen K, Milstein DM, Lemmers PM, van der Zee DC. Brain oxygenation during laparoscopic correction of hypertrophic pyloric stenosis. J Laparoendosc Adv Surg Tech As (2015) 25(4):352–7. doi:10.1089/lap.2014.0592
113. Tytgat SH, van Herwaarden MY, Stolwijk LJ, Keunen K, Benders MJ, de Graaff JC, et al. Neonatal brain oxygenation during thoracoscopic correction of esophageal atresia. Surg Endosc (2016) 30(7):2811–7. doi:10.1007/s00464-015-4559-1
114. Koch HW, Hansen TG. Perioperative use of cerebral and renal near-infrared spectroscopy in neonates: a 24-h observational study. Paediatr Anaesth (2016) 26(2):190–8. doi:10.1111/pan.12831
115. Chock VY, Ramamoorthy C, Van Meurs KP. Cerebral autoregulation in neonates with a hemodynamically significant patent ductus arteriosus. J Pediatr (2012) 160(6):936–42. doi:10.1016/j.jpeds.2011.11.054
116. Durandy Y, Rubatti M, Couturier R. Near infrared spectroscopy during pediatric cardiac surgery: errors and pitfalls. Perfusion (2011) 26(5):441–6. doi:10.1177/0267659111408755
117. Giliberti P, Mondi V, Conforti A, Lombardi MH, Sgro S, Bozza P, et al. Near infrared spectroscopy in newborns with surgical disease. J Matern Fetal Neonatal Med (2011) 24(Suppl 1):56–8. doi:10.3109/14767058.2011.607673
118. Conforti A, Giliberti P, Mondi V, Valfre L, Sgro S, Picardo S, et al. Near infrared spectroscopy: experience on esophageal atresia infants. J Pediatr Surg (2014) 49(7):1064–8. doi:10.1016/j.jpedsurg.2014.01.010
119. Cruz SM, Akinkuotu AC, Rusin CG, Cass DL, Lee TC, Welty SE, et al. A novel multimodal computational system using near-infrared spectroscopy to monitor cerebral oxygenation during assisted ventilation in CDH patients. J Pediatr Surg (2016) 51(1):38–43. doi:10.1016/j.jpedsurg.2015.10.017
120. Lejus C, De Windt A, LeBoeuf-Pouliquen D, Le Roux C, Berard L, Asehnoune K. A retrospective study about cerebral near-infrared spectroscopy monitoring during paediatric cardiac surgery and intra-operative patient blood management. Anaesth Crit Care Pain Med (2015) 34(5):259–63. doi:10.1016/j.accpm.2015.01.007
121. Menke J, Moller G. Cerebral near-infrared spectroscopy correlates to vital parameters during cardiopulmonary bypass surgery in children. Pediatr Cardiol (2014) 35(1):155–63. doi:10.1007/s00246-013-0754-9
122. Toet MC, Lemmers PM, van Schelven LJ, van Bel F. Cerebral oxygenation and electrical activity after birth asphyxia: their relation to outcome. Pediatrics (2006) 117(2):333–9. doi:10.1542/peds.2005-0987
123. Lemmers PM, Zwanenburg RJ, Benders MJ, de Vries LS, Groenendaal F, van Bel F, et al. Cerebral oxygenation and brain activity after perinatal asphyxia: does hypothermia change their prognostic value? Pediatr Res (2013) 74(2):180–5. doi:10.1038/pr.2013.84
124. Greisen G. Cerebral blood flow and oxygenation in infants after birth asphyxia. Clinically useful information? Early Hum Dev (2014) 90(10):703–5. doi:10.1016/j.earlhumdev.2014.06.007
125. Howlett JA, Northington FJ, Gilmore MM, Tekes A, Huisman TA, Parkinson C, et al. Cerebrovascular autoregulation and neurologic injury in neonatal hypoxic-ischemic encephalopathy. Pediatr Res (2013) 74(5):525–35. doi:10.1038/pr.2013.132
126. Massaro AN, Bouyssi-Kobar M, Chang T, Vezina LG, du Plessis AJ, Limperopoulos C. Brain perfusion in encephalopathic newborns after therapeutic hypothermia. AJNR Am J Neuroradiol (2013) 34(8):1649–55. doi:10.3174/ajnr.A3422
Keywords: near-infrared spectroscopy, cerebral oxygenation, neonates, neonatal intensive care, neonatal neurology
Citation: Dix LML, van Bel F and Lemmers PMA (2017) Monitoring Cerebral Oxygenation in Neonates: An Update. Front. Pediatr. 5:46. doi: 10.3389/fped.2017.00046
Received: 20 December 2016; Accepted: 24 February 2017;
Published: 14 March 2017
Edited by:
Utpal S. Bhalala, Baylor College of Medicine, USAReviewed by:
Narendra Reddy Dereddy, Maria Fareri Children’s Hospital, USAArun Bansal, Postgraduate Institute of Medical Education and Research, India
Copyright: © 2017 Dix, van Bel and Lemmers. This is an open-access article distributed under the terms of the Creative Commons Attribution License (CC BY). The use, distribution or reproduction in other forums is permitted, provided the original author(s) or licensor are credited and that the original publication in this journal is cited, in accordance with accepted academic practice. No use, distribution or reproduction is permitted which does not comply with these terms.
*Correspondence: Petra Maria Anna Lemmers, cC5sZW1tZXJzQHVtY3V0cmVjaHQubmw=