- 1Kaiser Permanente South Bay, Harbor City, CA, USA
- 2Providence Little Company of Mary Medical Center, Torrance, CA, USA
Sudden infant death syndrome (SIDS) continues to be a major public health issue. Following its major decline since the “Back to Sleep” campaign, the incidence of SIDS has plateaued, with an annual incidence of about 1,500 SIDS-related deaths in the United States and thousands more throughout the world. The etiology of SIDS, the major cause of postneonatal mortality in the western world, is still poorly understood. Although sleeping in prone position is a major risk factor, SIDS continues to occur even in the supine sleeping position. The triple-risk model of Filiano and Kinney emphasizes the interaction between a susceptible infant during a critical developmental period and stressor/s in the pathogenesis of SIDS. Recent evidence ranges from dysregulated autonomic control to findings of altered neurochemistry, especially the serotonergic system that plays an important role in brainstem cardiorespiratory/thermoregulatory centers. Brainstem serotonin (5-HT) and tryptophan hydroxylase-2 (TPH-2) levels have been shown to be lower in SIDS, supporting the evidence that defects in the medullary serotonergic system play a significant role in SIDS. Pathogenic bacteria and their enterotoxins have been associated with SIDS, although no direct evidence has been established. We present a new hypothesis that the infant’s gut microbiome, and/or its metabolites, by its direct effects on the gut enterochromaffin cells, stimulates the afferent gut vagal endings by releasing serotonin (paracrine effect), optimizing autoresuscitation by modulating brainstem 5-HT levels through the microbiome–gut–brain axis, thus playing a significant role in SIDS during the critical period of gut flora development and vulnerability to SIDS. The shared similarities between various risk factors for SIDS and their relationship with the infant gut microbiome support our hypothesis. Comprehensive gut-microbiome studies are required to test our hypothesis.
Introduction
Sudden infant death syndrome (SIDS) is defined as a sudden unexplained death in the first year of life in a previously healthy infant, where the cause of death remains unidentified despite thorough investigations including a complete autopsy, death scene investigation, and review of clinical history (1). SIDS is a major cause of postneonatal infant mortality in the western world. In the United States, ~1,500 infants died of SIDS in 2013 alone, despite the steady reduction (1994–2000) in such deaths since the “Back to Sleep” campaign. The incidence of SIDS has remained fairly constant in the last decade, while the rate of other causes of ill-defined, unspecified, and sudden unexpected infant deaths has increased (1, 2). Some infant deaths, which would have been classified as SIDS in the past, are now being classified as resulting from suffocation and asphyxia. The significant reduction in SIDS rate in the past 20 years may be related to increasing diagnoses of other causes of death (1). Japan and the Netherlands have the lowest SIDS rates, at 0.09 and 0.1 per 1,000 live births, respectively, whereas New Zealand has the highest reported SIDS rate (0.8 per 1,000 live births) (3–6). The United States and UK have SIDS rates of 0.57 and 0.41 per 1,000 live births, respectively (7, 8). Prone sleeping position, a significant SIDS risk factor, cannot be easily associated with the other epidemiological risk factors related to SIDS (9).
Current Hypotheses for SIDS
Sudden infant death syndrome is a condition without a widely accepted singular pathological mechanism.
(1) Triple-risk model: this model proposes that SIDS occurs when external stressors simultaneously act upon on a susceptible infant with a vulnerable homeostatic system during a critical developmental period (10).
(2) Failed autoresuscitation: animal studies have shown that cardiorespiratory, sleep, and arousal mechanisms are abnormal following exposure to risk factors associated with SIDS or in infants who later succumb to SIDS (11, 12). Although the exact cause of SIDS is unknown, immaturity of brain stem autonomic cardiorespiratory/thermoregulatory control and failure of autoresuscitation during sleep are significant determinants of survival (11, 12). A leading SIDS hypothesis states that a structural/neurochemical brainstem abnormality results in failure of autoresuscitation following exposure to a stressor during a critical developmental period (13, 14). SIDS vulnerability is specific to failed autoresuscitation from an adverse autonomic event (AAE). The initial self-initiated gasp during such an event is dependent of optimal serotonin homeostasis in the brain, which is undermined in SIDS. Imbalance in serotonin homeostasis alters sleep rhythm, thus increasing the chances of AAE (15).
(3) Medullary serotonergic network deficiency: SIDS is associated with multiple serotonergic defects including serotonin deficiency (16–19). It has been associated with reduced serotonin in the ventral medulla, pointing to a brainstem-based autonomic dysfunction affecting sleep/arousal/cardiorespiratory reflexes (20–23). Gene polymorphisms related to serotonergic autonomic system may play a role in SIDS (24). In a recent study in neonatal rodents, loss of brain stem 5HT may explain the cardiovascular collapse during apparent severe hypoxic event in some SIDS cases (25). Recent neuropathology studies in SIDS implicate defective neurotransmitter function in the medullary arcuate nucleus, receptor immaturity of the “respiratory center” nucleus tractus solitarius (NTS), and defective function of the serotonergic raphé nuclei of the ponto-medullary ventral median septum and other brainstem serotonergic neurons (26). Abnormalities of the dorsal motor nucleus of the vagus (DMNV) have been associated with SIDS (27). In a significant number of SIDS infants, cerebellar dentate nucleus lesions may represent a developmental susceptibility leading to autonomic cardiorespiratory/arousal dysfunction and sleep-related death when exposed to homeostatic stressors (28). Cummings et al. report that, in addition to respiratory and cardiac dysfunction in normoxemic conditions, neonatal mice with reduced (by 60–70%) brainstem serotonergic neurons from early embryogenesis onward (Pet-1−/−) have major defects in autoresuscitation, a life-preserving process utilized by neonatal mammals in severely hypoxic conditions (29–33).
(4) Neurotransmitters: neurotransmitter systems (e.g., cholinergic and GABA-ergic) have been shown to be involved in SIDS (34, 35). Reduced muscarinic cholinergic binding in the medullary arcuate nucleus (involved in cardiorespiratory control) has been shown to occur in SIDS (34). GABA neurons in the medulla help regulate homeostasis through interactions with the medullary serotonergic system (35). Significant decrease in GABA A receptor binding was found in the medullary serotonergic system in SIDS cases associated with 5-HT defects (35).
New Hypothesis
We propose a new hypothesis that the infant gut microbiome plays an important role in SIDS during the period critical to both gut flora maturation/development and vulnerability to SIDS, by modulating brainstem serotonergic system through the bidirectional microbiome–gut–brain axis, thus tilting the balance in favor of successful autoresuscitation during a sleep-related AAE. The components of our hypothesis, though individually and separately studied in the past, have never been put together as the structure of a SIDS hypothesis. The factors protective against as well as the risk factors of SIDS show some compelling circumstantial evidence of their effects on gut microbiome leading to beneficial and dysbiotic infant gut flora, respectively, with corresponding effects on brainstem serotonergic system. The plausibility of such an SIDS hypothesis would open up a new paradigm for preventative and therapeutic approaches in SIDS. Our hypothesis is the only one till date, which connects the protective/risk factors of SIDS with infant gut flora, their effect via microbiome–gut–brain axis on brainstem serotonergic system, and subsequent successful autoresuscitation (Figure 1).
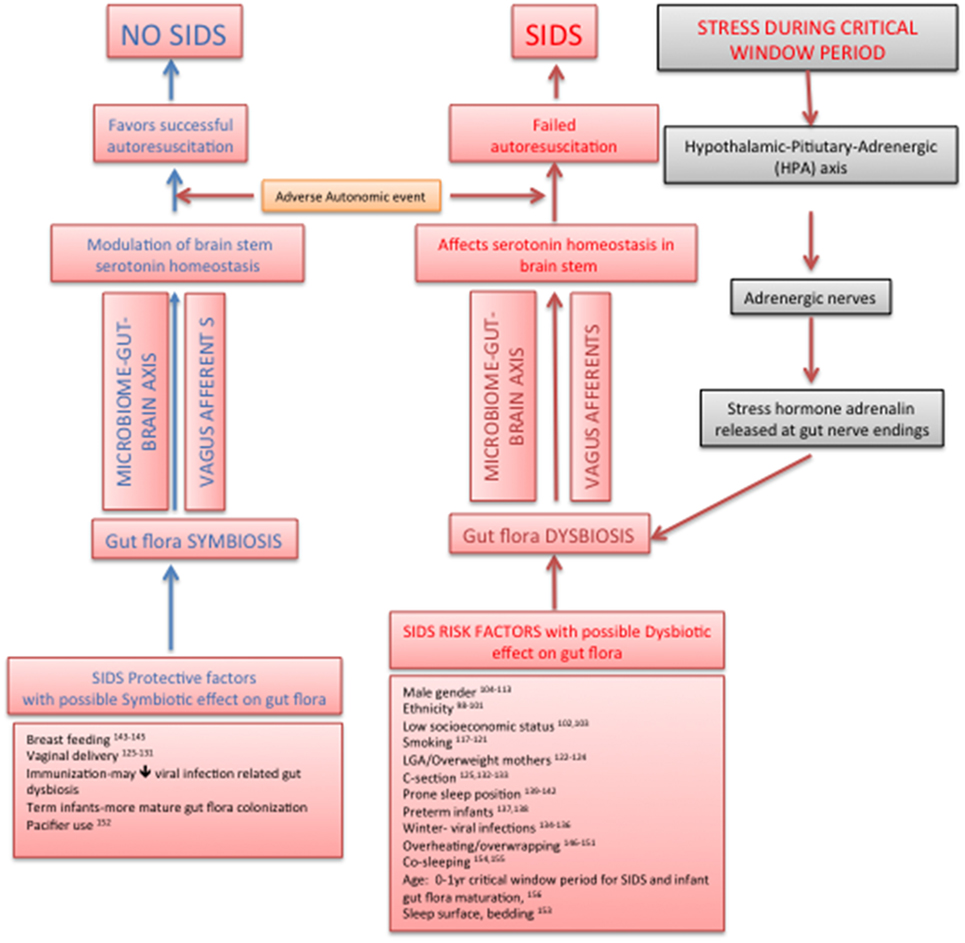
Figure 1. New sudden infant death syndrome hypothesis pathway: effect on infant gut flora on brainstem serotonin homeostasis and autoresuscitation via microbiome–gut–brain axis.
Microbiome–Gut–Brain Axis
The human adult gut has about 1014 microorganisms, 10-fold the human cells and 150-fold the amount of human DNA (36, 37). The human gut microbiome comprises more than 1,000 species, predominantly obligate anaerobes, and includes viruses, protozoa, archaea, and fungi (36–39). Gastrointestinal homeostasis has a significant role in the human general health and well-being (39–41). The concept of the brain–gut axis involves the complex bidirectional homeostatic neuronal communication through the vagus nerve that exists between the central nervous system (CNS) and the enteric nervous system (ENS) (42). Current research studies the mechanism of such communication along this axis and its relationship to normal homeostasis and disease states (42–48). The basic skeleton of the microbiome–gut–brain axis includes gut microbiome, the CNS, neuroendocrine and neuro-immune systems, ENS, sympathetic, and parasympathetic arms of the autonomic nervous system (49). The gut accounts for 95% of the body’s serotonin content. The detailed structure, integration, and functioning of the various components of the above axis have been reviewed extensively elsewhere (45).
Gut microbial colonization also plays a major role in the postnatal development of the endocrine and immune systems, which in turn support CNS function, particularly the developing serotonergic system (41, 42, 50–52). Neurotransmitters, neurohormones, and receptors are ubiquitous in nature, e.g., catecholamines corticotrophin, somatostatin, and GABA derived from bacteria (53–56). Evolutionally speaking, bacteria preceded humans in developing neurotransmitters and recognizing them (57–59). The ontogeny of neurochemicals in mammals has been postulated to arise as a consequence of bacterial lateral gene transfer (60). Thus, the gut microbiota might have played an important role in the evolution of neurodevelopment (61).
Gut Vagal Afferents and the Medullary Serotonergic System
Sudden infant death syndrome is associated with multiple serotonergic defects including serotonin deficiency and DMV abnormalities (15–18, 62). We briefly review the vagal afferents, brainstem respiratory neurons, and the medullary serotonergic system.
Vagal afferents outnumber vagal efferents by 10:1, which are sensitive to the paracrine effects of the enterochromaffin cells (ECC), relay through nodose and dorsal root ganglia before synapsing with second-order neurons in the spinal cord, which in turn project into the brainstem. The brainstem has major respiratory neurons concentrated into three recognizable groups comprised of four major nuclei. These include the following: (1) dorsal respiratory group (DRG) centered in the NTS; (2) ventral respiratory group that encompasses the nucleus ambiguous and the nucleus retroambigualis; (3) pre-Botzinger complex (pre-BotC) which contains putative pacemaker neurons; and (4) BotC located in and near the nucleus retrofacialis. The DRG neurons through the phrenic neurons in the cervical spinal cord control the diaphragm.
The medullary serotonergic system projects to brainstem cardiorespiratory nuclei (including the DMNV), cerebellum, and spinal cord, thus modulating cardiorespiratory protective reflexes, central chemoprotective reflexes, arousal/sleep cycles, thermoregulatory reflexes, and maintenance of upper airway patency (63).
Vagal afferents affect respiratory control as shown by altered respiratory pattern after stimulation of visceral vagal afferents in guinea pigs which died within a few hours of bilateral vagotomy; their frequency of breathing significantly decreased within minutes of the procedure (64, 65). Serotonin may regulate developmental brainstem neuronal apoptosis with its pro- or antiapoptotic effects as a result of the receptor sub-family activated (66). Animal studies have shown that the highest density of 5-HT3 receptors are found within the afferent vagal fibers of dorsal vagal complex (67, 68) and vagotomy was found to significantly reduce receptor density (69–71). Stimulation of the NTS 5-HT3 receptors leads to elevation of blood pressure and inhibition of the chemoreceptor-mediated bradycardia and the Bezold–Jarisch reflexes. As an example of sensory neural plasticity, recent rat studies have shown that glucose in the intestinal tract probably induces serotonin release from neuroendocrine cells, which activates 5HT3 on vagal afferent terminals and transmitted centrally (72–79).
Gut Microbiome Affects the Brainstem
There is emerging evidence from animal and clinical studies on the role of gut microbiome in CNS signaling.
Animal Studies
Evidence from rodent studies indicates that the gut microbiome can affect neural development, chemistry, and behaviors, e.g., emotion, pain perception, and stress responses. As rodent gut colonization pattern is similar to humans, they are subjects of choice for gut microbiome studies. CNS tryptophan concentrations are dependent on peripheral content, which suggests that gut flora might play a part in regulating peripheral and central serotonin synthesis (44, 80, 81). TPH2 is responsible for the synthesis of serotonin in brainstem raphe nuclei, which is the origin of most central serotonergic projections (82). Probiotics have been shown to modulate serotonin—a critical central neurotransmitter through multiple strain-specific mechanisms (83). Lyte et al. proposed a “delivery system” by which gut flora can communicate neurochemical messages to the brain. Gut bacteria produce and react to the same neurotransmitters (e.g., serotonin, norepinephrine, dopamine, and GABA) that play a role centrally in modulation of mood (84). Animal studies studying effects of probiotics on CNS function have been extensively and systematically reviewed elsewhere (85). In addition, we have listed few rodent studies looking at the role of pathogenic bacteria and vagus on CNS neurochemistry and behavior (52, 86–92) (Table 1).
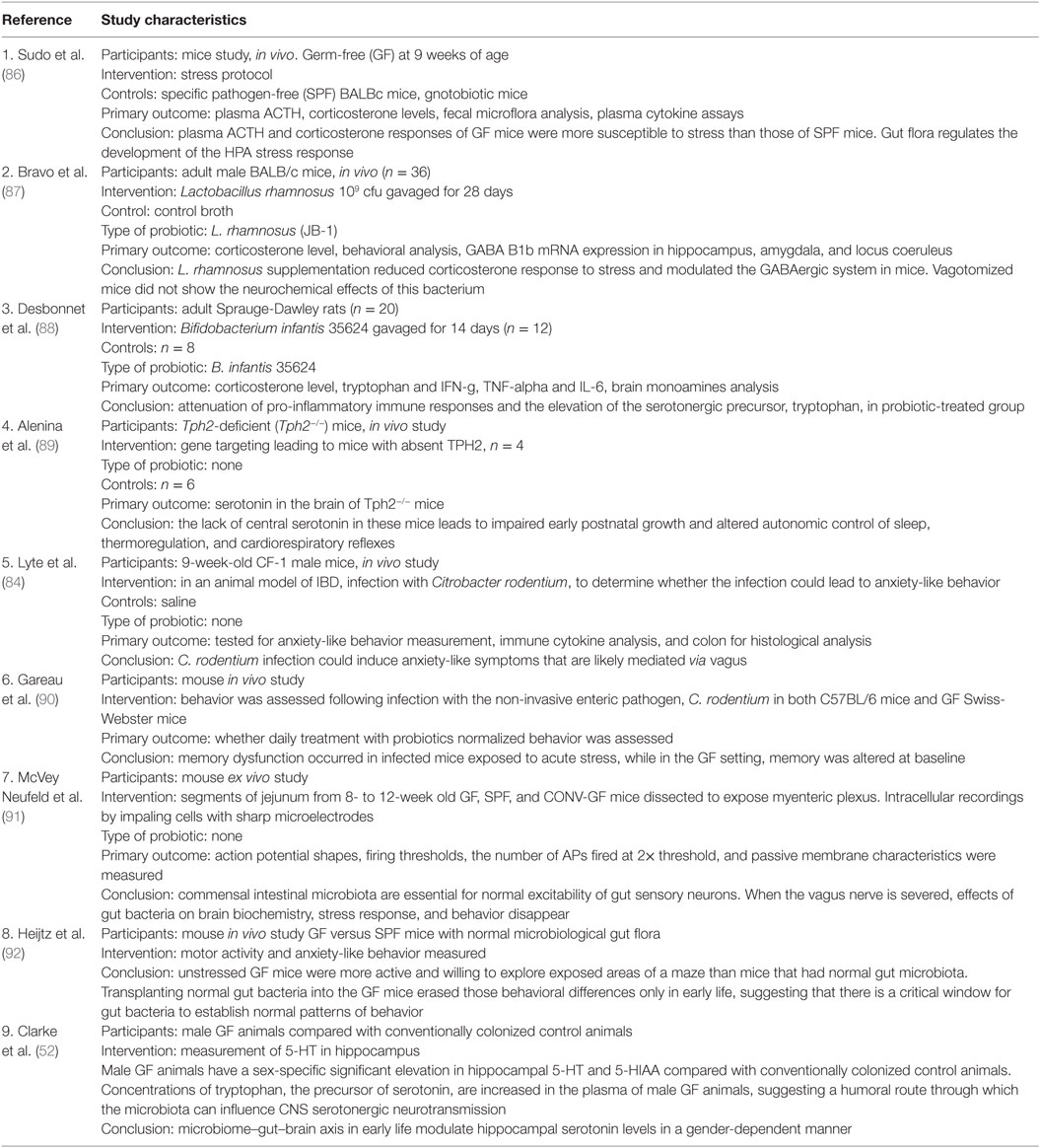
Table 1. Animal studies showing effect of gut microbiome/probiotics on the central nervous system (CNS).
Clinical Studies
Emerging evidence from clinical studies in autism indicates a relationship between gut flora and cognitive function. Researchers have reported gut flora dysbiosis with increases in Clostridium spp. in autism (93). A probiotic mixture of Lactobacillus helveticus and Bifidobacterium longum for a month has been reported to decrease anxiety and depression in healthy human (94). Other adult human clinical studies looking at probiotic effects on neurobehavior have been systematically reviewed elsewhere (85).
Brain Affects Gut Microbiome
Stress induces gut permeability, which allows bacteria/bacterial antigen translocation across the epithelial barrier, thereby activating immune response and resulting in changes in the gut microbiome characteristics (95). Psychological stressors have been reported to modulate infant gut microbiome (47). Prenatal stressors have been reported to cause dysbiosis by decreasing gut Bifidobacteria and Lactobacilli in infant rhesus monkeys (96). In rodent studies, the stress of maternal separation significantly decreased stool lactobacilli on the third day, which returned to baseline by day 7 following separation (97). Stressors acting on an at-risk infant during the critical window period could affect the favorable nature of infant gut flora and consequently affect the brainstem neurotransmitters through bidirectional communication and/or gut barrier function locally.
Based on the evidence from the experimental and clinical studies discussed above, we propose that an optimal (diversity, complexity, and colony counts) gut flora interacts with ECC and modulates (possibly by its serotonin and other paracrine effects) through the afferent vagal endings to the brain stem medullary serotonergic cardiorespiratory centers in infants at risk for SIDS. Recent research in microbiome–gut–brain axis supports role of probiotics to modulate central brain neurochemistry, thus opening up a site for therapeutic targeting for central brain disorders.
Shared Risk Factors for SIDS and Gut Dysbiosis
In the following section, we report how each of the protective as well as risk factors for SIDS seems to offer evidence of promoting symbiotic (favorable) and dysbiotic gut (non-favorable) flora, respectively, during the critical window when both SIDS tends to occur and early infant gut colonization is being established.
Demographic Factors
(1) Ethnicity: studies in indigenous populations have reported a higher SIDS rate compared to the non-indigenous groups within the same countries (98). These differences may reflect differences in maternal smoking, which could affect frequency and density of colonization of infants by potentially pathogenic bacteria and induction/control of inflammatory responses (98). Maternal cigarette smoking and/or alcohol consumption may contribute to abnormal fetal medullary 5-HT development in Native American SIDS infants (99). A recent study has reported diet-related differences in gut flora composition between African-Americans and native Africans. African-Americans had higher levels of 7-α-dehydroxylating bacteria and lower levels of Lactobacillus plantarum (which produce methane and is protective against dysbiosis) (100, 101).
(2) Low socioeconomic status: SIDS has been associated with lower socioeconomic groups (102). Fecal lactobacilli numbers have been related to socioeconomic status (103). Gut flora differences related to diet, smoking status, and access to health services could be a proxy for lower socioeconomic status.
(3) Gender: SIDS shows a male preponderance. Animal studies have shown gender differences in the regulation of serotonergic system (104, 105). Estrogen has been implicated in the modulation of hippocampal serotoninergic system (106, 107). Gender influences gut microbiome (108–111) through unclear mechanisms (110) including hormone–microbe interactions (111, 112) and gender-specific immune responses (113).
(4) Genetic control: genes regulating serotonergic network, brain function and development, and cardiac function play an important role in SIDS (114). Studying the role of genetics on gut microbiome is important in understanding the pathogenesis of bacterial diseases (115, 116).
Prenatal Risk Factors
(1) Maternal smoking: SIDS is five times more common in infants born to mothers who smoked during pregnancy and three times more common in those exposed postnatally to smoking (117, 118). Cigarette smoke exposure and prone sleep position is associated with decreased 5HT1A receptors in the DMNV of SIDS infants (119). A reduction in 5HT1A receptors has been reported in the DMNV of piglets subjected to intermittent hypercapnic hypoxia and nicotine exposure (120). A recent study showed that cessation of smoking improved gut microbial diversity (121). Smoking may play a role in SIDS through its effects on infant gut flora and brainstem serotonin homeostasis.
(2) Being overweight: overweight infants and mothers have a higher risk of SIDS (122). Obese human adults had less Bacteroides and more Firmicutes in their gut flora compared with lean controls (123). A recent review looked at maternal obesity-related pro-inflammatory state and its effect on maternal and in utero fetal gut microbiome and development (124).
(3) Delivery route: infants delivered by cesarean section have an increased risk of SIDS than those born by vaginal route (125). The mode of delivery has a significant effect on newborn gut flora development (126–128). The gut flora in infants born by cesarean may be altered till 6 months following delivery (129). Prolonged duration of labor during vaginal birth increases the chances of isolation of viable microbes from the stomach and mouth of the infant (130, 131). In addition to exposure to maternal flora, infants born by cesarean section acquire gut flora from their exposure to the immediate environment (132). Aseptic precautions in obstetrics and neonatal units may result in dysbiosis of the infant gut microbiome (133).
Postnatal Risks
(1) Season: SIDS is more common during winter months (134). There is an association of a viral infection in the days preceding SIDS (135). Stressors such as viral infections during winter may cause dysbiosis in infants (136). Such dysbiosis could play a role in successful autoresuscitation via microbiome–gut–brainstem pathway.
(2) Low birth weight: the rate of SIDS is higher in low birth weight infants (137). This may be related to the gut colonization patterns of very low birth weight (VLBW) infants compared with normal weight infants. In an elegant study, the initial gut colonization by Enterobacteria and Streptococci was similar in both VLBW and full-term infants; however, both microorganisms predominated for a longer period of time and the establishment of Bifidobacterium, Bacteroides, Clostridium, and Lactobacillus was delayed in VLBW infants (138).
(3) Prone sleep position: prone sleeping position has been the most important risk factor associated with SIDS (139). In addition to decreased arousal response related to prone sleeping, body temperature seems to be slightly elevated in prone infants (140, 141). Prone sleep position has been associated with Staphylococcus aureus gut colonization in SIDS. The increased risk of ingestion/inhalation of bacteria contaminating the sleeping surface during prone position, with resultant gut dysbiosis, could account for the increased risk of SIDS in such infants (22).
(4) Breastfeeding: breastfeeding has been shown to be protective against SIDS (142, 143). Breast milk oligosaccharides when fermented by gut flora to form fatty acids results in modulation of infant gut flora. Breast-fed infants show predominant proliferation of Bifidobacteria and Lactobacilli, whereas formula-fed infants show more Enterococci and Enterobacteria in their gut flora. In addition, infants who are breast-fed exclusively have been reported to have better sleep arousal patterns than formula-fed infants (144).
(5) Elevated or reduced room temperature: overheating of infants has been reported with an elevated risk of SIDS (2, 145). Animal studies have showed that the presence of certain gut flora elevates body temperature of mice and rats. Conn et al. demonstrated that Gram-positive organisms are a major source of the stimulatory effect of gut flora on normal body temperature in mice (146). Body temperature has been shown to have effects on the intestinal flora of hibernating squirrels (147, 148). Oral antibiotics have been shown to reduce nighttime body temperature in rabbits as a result of their effect on their native intestinal flora (149). These studies may help in understanding whether the increased body temperature as a risk factor for SIDS could be a result of aberrant gut flora or vice versa. Elevated body temperature associated with prone sleep position may also play a role in affecting gut flora composition (140, 141).
(6) Pacifier use: pacifier sucking has been shown to be strongly associated with the oral colonization of salivary lactobacilli (150). Thus, pacifier use might play a role in favorable oral and subsequently gut flora in infants.
(7) Sleep surface, bedding, and stuffed toys: apart from mechanical suffocation and overheating issues, these may act as fomites contributing to the infant gut flora. Sherburn et al. showed that simulated infant head movements and mattress-related factors affect aerial release of bacteria from beds (151).
(8) Co-sleeping: recent meta-analyses showed that bed sharing during sleep increases the risk of SIDS, which is further increased when combined with parental smoking, maternal alcohol consumption, and/or drug use (152). The results of a Swedish study suggest that parental skin S. aureus establish readily in the infant’s gut, perhaps due to poor competition from other gut bacteria (153). The possible role of acquiring abnormal gut flora from parents/caregivers skin/gut flora by prolonged close contact during bed sharing needs to be investigated further.
(9) Infant’s age: SIDS incidence peaks around 2–4 months of the infant’s age, and subsequently decreases by 1 year. Infant gut flora develops through a period of instability in the early months of infancy and reaches more mature adult-like microbiome by 1 year of age, the time by which SIDS disappears. Another condition, not fully explained, affecting the infant during a typical window period is infant colic, in which aberrant gut flora has been recently shown to play a role, amenable to probiotics. Infantile colic is associated with a greater extent with near-miss SIDS infants than among control infants, thereby hypothesizing that colic might play a role as a protective arousal mechanism in such infants (154). From infection/immunity standpoint, this is the time when maternal antibodies reach their nadir making infants more susceptible to infections, including from indigenous pathogenic gut flora. Introduction of supplementary foods around 6 months of age leads to more gut microbial diversity.
(10) Gestation at birth: prematurity is associated with a fourfold increased risk of SIDS (137) as well as a dysbiotic intestinal flora, and impaired gut mucosal barrier function and permeability (155–161). Lactobacillus GG has been shown to decrease the frequency of Escherichia coli K1A translocation in a neonatal rabbit model (162, 163). Extremely preterm newborns (<28 weeks) have a 5- to 10-fold higher incidence of microbial infections than term newborn (164). The preterm neonatal gut colonization is different from that in the healthy, full-term infant gut. Preterm neonates requiring intensive care are colonized by organisms such as Bifidobacteria only gradually and in a delayed fashion. Schwiertz et al. reported similar bacterial colonization patterns in preterm infants in contrast to breast-fed, full-term infants. Bacterial colonization has been observed to be similar in different preterm neonates irrespective of birth weight, feeding regime, and antibiotic therapy. The initial colonization of the newborn GI tract is highly dependent on the environment, and cross-transmission of bacteria is a serious problem in the hospital (165).
(11) Small for gestational age (SGA): it has been hypothesized that SGA infants may have a higher incidence of SIDS as a result of fetal hypoxia-induced decrease in brain serotonergic receptors (16, 166–169).
Conclusion
We have provided a new SIDS hypothesis whereby the right composition of gut flora in the early critical stage of infant development could possibly optimize or modulate serotonin homeostasis in the serotonergic cardiorespiratory/thermoregulatory brain stem nuclei by a direct communication via the vagal afferents as part of the microbiome–gut–brain axis. This may tip the balance in favor of a successful autoresuscitation response to an AAE during sleep. Investigating the role of infant microbiome using newer culture-independent techniques as well as the developmental physiology and neuropathology associated with SIDS may provide more specific strategies than those available currently to define the at risk population. As Hippocrates once stated “All diseases begin in the gut,” research on the gut flora in at risk infants would open new avenues for identifying potential biomarkers and strategies for prevention (e.g., maternal and/or early postnatal probiotic/synbiotic supplementation, diet changes) of SIDS (170).
Author Contributions
VP was involved in concept and manuscript preparation. SP was involved in editing of the final manuscript.
Conflict of Interest Statement
The authors declare that the research was conducted in the absence of any commercial or financial relationships that could be construed as a potential conflict of interest.
References
1. Moon RY, Fu L. Sudden infant death syndrome: an update. Pediatr Rev (2012) 33(7):314–20. doi:10.1542/pir.33-7-314
2. Moon RY. Task force on sudden infant death syndrome. SIDS and other sleep-related infant deaths: expansion of recommendations for a safe infant sleeping environment. Pediatrics (2011) 128(5):1030–9. doi:10.1542/peds.2011-2284
4. Central Bureau of Statistics. Netherlands (2006). Available from: http://www.cbs.nl/en-GB (accessed July 5, 2007).
5. New Zealand Health Information Service. (2003). Available from: http://www.nzhis.govt.nz (accessed July 5, 2007).
6. Hoyert DL, Mathews TJ, Menacker F, Strobino DM, Guyer B. Annual summary of vital statistics: 2004. Pediatrics (2006) 117:168–83. doi:10.1542/peds.2005-2587
7. Fleming P, Blair P, Bacon C, Berry J, editors. Sudden unexpected deaths in infancy. The CESDI SUDI Studies 1993–1996. London: The Stationary Office (2000).
8. Office for National Statistics. Report: unexplained deaths in infancy, 2005. Health Stat Q (2006) 31:82–6.
9. Guntheroth WG, Spiers PS. Sleeping prone and the risk of SIDS. J Am Med Assoc (1992) 267:2359–62. doi:10.1001/jama.1992.03480170085034
10. Filiano JJ, Kinney HC. A perspective on neuropathologic findings in victims of the sudden infant death syndrome: the triple-risk model. Biol Neonate (1994) 65(3–4):194–7. doi:10.1159/000244052
11. Galland BC, Elder DE. Sudden unexpected death in infancy: biological mechanisms. Paediatr Respir Rev (2014) 15(4):287–92. doi:10.1016/j.prrv.2014.09.003
12. Machaalani R, Waters KA. Neurochemical abnormalities in the brainstem of the sudden infant death syndrome (SIDS). Paediatr Respir Rev (2014) 15(4):293–300. doi:10.1016/j.prrv.2014.09.008
13. Poets CF, Meny RG, Chobanian MR, Bonofiglo RE. Gasping and other cardiorespiratory patterns during sudden infant deaths. Pediatr Res (1999) 45:350–4. doi:10.1203/00006450-199903000-00010
14. Sridhar R, Thach BT, Kelly DH, Henslee JA. Characterization of successful and failed autoresuscitation in human infants, including those dying of SIDS. Pediatr Pulmonol (2003) 36:113–22. doi:10.1002/ppul.10287
15. Bergmen NJ. Proposal for mechanisms of protection of supine sleep against sudden infant death syndrome: an integrated mechanism review. Pediatr Res (2015) 77(1–1):10–9. doi:10.1038/pr.2014.140
16. Paterson DS, Trachtenberg FL, Thompson EG, Belliveau RA, Beggs AH, Darnall R, et al. Multiple serotonergic brainstem abnormalities in sudden infant death syndrome. JAMA (2006) 296(17):2124–32. doi:10.1001/jama.296.17.2124
17. Penatti EM, Berniker AV, Kereshi B, Cafaro C, Kelly ML, Niblock MM, et al. Ventilatory response to hypercapnia and hypoxia after extensive lesion of medullary serotonergic neurons in newborn conscious piglets. J Appl Physiol (1985) (2006) 101(4):1177–88. doi:10.1152/japplphysiol.00376.2006
18. Paterson DS, Thompson EG, Kinney HC. Serotonergic and glutamatergic neurons at the ventral medullary surface of the human infant: observations relevant to central chemosensitivity in early human life. Auton Neurosci (2006) 124(1–2):112–24. doi:10.1016/j.autneu.2005.12.009
19. Cummings KJ, Hewitt JC, Li A, Daubenspeck JA, Nattie EE. Postnatal loss of brainstem serotonin neurones compromises the ability of neonatal rats to survive episodic severe hypoxia. J Physiol (2011) 589(Pt 21):5247–56. doi:10.1113/jphysiol.2011.214445
20. Duncan JR, Paterson DS, Hoffman JM. Brainstem serotonergic deficiency in sudden infant death syndrome. JAMA (2010) 303(5):430–7. doi:10.1001/jama.2010.45
21. Blood-Siegfried J. The role of infection and inflammation in sudden infant death syndrome. Immunopharmacol Immunotoxicol (2009) 31(4):516–23. doi:10.3109/08923970902814137
22. Highet AR, Berry AM, Bettelheim KA, Goldwater PN. Gut microbiome in sudden infant death syndrome (SIDS) differs from that in healthy comparison babies and offers an explanation for the risk factor of prone position. Int J Med Microbiol (2014) 304(5–6):735–41. doi:10.1016/j.ijmm.2014.05.007
23. Broadbelt KG, Rivera KD, Paterson DS, Duncan JR, Trachtenberg FL, Paulo JA, et al. Brainstem deficiency of the 14-3-3 regulator of serotonin synthesis: a proteomics analysis in the sudden infant death syndrome. Mol Cell Proteomics (2012) 11(1):M111.009530. doi:10.1074/mcp.M111.009530
24. Moon RY, Horne RS, Hauck FR. Sudden infant death syndrome. Lancet (2007) 370(9598):1578–87. doi:10.1016/S0140-6736(07)61662-6
25. Yang HT, Cummings KJ. Brain stem serotonin protects blood pressure in neonatal rats exposed to episodic anoxia. J Appl Physiol (1985) (2013) 115(12):1733–41. doi:10.1152/japplphysiol.00970.2013
26. Rubens D, Sarnat HB. Sudden infant death syndrome: an update and new perspectives of etiology. Handb Clin Neurol (2013) 112:867–74. doi:10.1016/B978-0-444-52910-7.00008-8
27. Bejjani C, Machaalani R, Waters KA. The dorsal motor nucleus of the vagus (DMNV) in sudden infant death syndrome (SIDS): pathways leading to apoptosis. Respir Physiol Neurobiol (2013) 185(2):203–10. doi:10.1016/j.resp.2012.09.001
28. Kinney HC, Cryan JB, Haynes RL, Paterson DS, Haas EA, Mena OJ, et al. Dentate gyrus abnormalities in sudden unexplained death in infants: morphological marker of underlying brain vulnerability. Acta Neuropathol (2015) 129(1):65–80. doi:10.1007/s00401-014-1357-0
29. Hendricks TJ, Fyodorov DV, Wegman LJ, Lelutiu NB, Pehek EA, Yamamoto B, et al. Pet-1 ETS gene plays a critical role in 5-HT neuron development and is required for normal anxiety-like and aggressive behavior. Neuron (2003) 37:233–47. doi:10.1016/S0896-6273(02)01167-4
30. Kiyasova V, Fernandez SP, Laine J, Stankovski L, Muzerelle A, Doly S, et al. A genetically defined morphologically and functionally unique subset of 5-HT neurons in the mouse raphe nuclei. J Neurosci (2011) 31:2756–68. doi:10.1523/JNEUROSCI.4080-10.2011
31. Fewell JE. Protective responses of the newborn to hypoxia. Respir Physiol Neurobiol (2005) 149:243–55. doi:10.1016/j.resp.2005.05.006
32. Erickson JT, Shafer G, Rossetti MD, Wilson CG, Deneris ES. Arrest of 5-HT neuron differentiation delays respiratory maturation and impairs neonatal homeostatic responses to environmental challenges. Respir Physiol Neurobiol (2007) 159:85–101. doi:10.1016/j.resp.2007.06.002
33. Cummings KJ, Commons KG, Hewitt JC, Daubenspeck JA, Kinney HC, Nattie EE. Failed heart rate recovery at a critical age in 5-HT-deficient mice exposed to episodic anoxia: implications for SIDS. J Appl Physiol (2011) 111:825–33. doi:10.1152/japplphysiol.00336.2011
34. Kinney HC, Filiano JJ, Sleeper LA, Mandell F, Valdes-Dapena M, White WF. Decreased muscarinic receptor binding in the arcuate nucleus in sudden infant death syndrome. Science (1995) 8:1446–50. doi:10.1126/science.7660131
35. Broadbelt K, Paterson DS, Belliveau RA, Trachtenberg FL, Haas EA, Stanley C, et al. Decreased GABAA receptor binding in the medullary serotonergic system in the sudden infant death syndrome. J Neuropathol Exp Neurol (2011) 70(9):799–810. doi:10.1097/NEN.0b013e31822c09bc
36. Gill SR, Pop M, Deboy R, Eckburg PB, Turnbaugh PJ, Samuel BS, et al. Metagenomic analysis of the human distal gut microbiome. Science (2006) 312:1355–9. doi:10.1126/science.1124234
37. Qin J, Li R, Raes J, Arumugam M, Burgdorf KS, Manichanh C, et al. A human gut microbial gene catalogue established by metagenomic sequencing. Nature (2010) 464:59–65. doi:10.1038/nature08821
38. Xu J, Mahowald MA, Ley RE, Lozupone CA, Hamady M, Martens EC, et al. Evolution of symbiotic bacteria in the distal human intestine. PLoS Biol (2007) 5:e156. doi:10.1371/journal.pbio.0050156
39. Eckburg PB, Bik EM, Bernstein CN, Purdom E, Dethlefsen L, Sargent M, et al. Diversity of the human intestinal microbial flora. Science (2005) 308:1635–8. doi:10.1126/science.1110591
40. Bischoff SC. ‘Gut health’: a new objective in medicine? BMC Med (2010) 9:24. doi:10.1186/1741-7015-9-24
41. Grenham S, Clarke G, Cryan J, Dinan TG. Brain-gut-microbe communication in health. Front Physiol (2011) 2:94. doi:10.3389/fphys.2011.00094
42. Cryan JF, O’Mahony SM. The microbiome-gut-brain axis: from bowel to behavior. Neurogastroenterol Motil (2001) 23:187–92. doi:10.1111/j.1365-2982.2010.01664.x
43. Lyte M. The microbial organ in the gut as a driver of homeostasis and disease. Med Hypotheses (2010) 74:634–8. doi:10.1016/j.mehy.2009.10.025
44. Forsythe P, Sudo N, Dinan T, Taylor VH, Bienenstock J. Mood and gut feelings. Brain Behav Immun (2010) 24:9–16. doi:10.1016/j.bbi.2009.05.058
45. Mayer EA. Gut feelings: the emerging biology of gut-brain communication. Nat Rev Neurosci (2011) 12:453–66. doi:10.1038/nrn3071
46. O’Hara AM, Shanahan F. The gut flora as a forgotten organ. EMBO Rep (2006) 7:688–93. doi:10.1038/sj.embor.7400731
47. Rhee SH, Pothoulakis C, Mayer EA. Principles and clinical implications of the brain-gut-enteric microbiota axis. Nat Rev Gastroenterol Hepatol (2009) 6:306–14. doi:10.1038/nrgastro.2009.35
48. Bercik P, Collins SM, Verdu EF. Microbes and the gut-brain axis. Neurogastroenterol Motil (2012) 24:405–13. doi:10.1111/j.1365-2982.2012.01906.x
49. O’Mahony SM, Hyland NP, Dinan TG, Cryan JF. Maternal separation as a model of brain-gut axis dysfunction. Psychopharmacology (Berl) (2001) 214:71–88. doi:10.1007/s00213-010-2010-9
50. Gaspar P, Cases O, Maroteaux L. The developmental role of serotonin: news from mouse molecular genetics. Nat Rev Neurosci (2003) 4:1002–12. doi:10.1038/nrn1256
51. Leonard BE. HPA and immune axes in stress: involvement of the serotonergic system. Neuroimmunomodulation (2006) 13:268–76. doi:10.1159/000104854
52. Clarke G, Grenham S, Scully P, Fitzgerald P, Moloney RD, Shanahan F, et al. The microbiome-gut-brain axis during early life regulates the hippocampal serotonergic system in a sex-dependent manner. Mol Psychiatry (2013) 18(6):666–73. doi:10.1038/mp.2012.77
53. Lenard J. Mammalian hormones in microbial cells. Trends Biochem Sci (1992) 17:147–50. doi:10.1016/0968-0004(92)90323-2
54. LeRoith D, Liotta AS, Roth J, Shiloach J, Lewis ME, Pert CB, et al. Corticotropin and beta-endorphin-like materials are native to unicellular organisms. Proc Natl Acad Sci U S A (1982) 79:2086–90. doi:10.1073/pnas.79.6.2086
55. LeRoith D, Pickens W, Vinik AI, Shiloach J. Bacillus subtilis contains multiple forms of somatostatin-like material. Biochem Biophys Res Commun (1985) 127:713–9. doi:10.1016/S0006-291X(85)80001-2
56. Guthrie GD, Nicholson-Guthrie CS, Leary HL Jr. A bacterial high-affinity GABA binding protein: isolation and characterization. Biochem Biophys Res Commun (2000) 268:65–8. doi:10.1006/bbrc.1999.1960
57. LeRoith D, Shiloach J, Berelowitz M, Berelowitz M, Holtgrefe M, Shiloach J, et al. Are messenger molecules in microbes the ancestors of the vertebrate hormones and tissue factors? Fed Proc (1983) 42:2602–7.
58. LeRoith D. Vertebrate hormones and neuropeptides in microbes: evolutionary origin of intercellular communication. In: Martini L, Ganong WF, editors. Frontiers in Neuroendocrinology (Vol. 8), New York: Raven Press (1984). p. 1–25.
59. Roth J, LeRoith D, Shiloach J, Rosenzweig JL, Lesniak MA, Havrankova J. The evolutionary origins of hormones, neurotransmitters, and other extracellular chemical messengers: implications for mammalian biology. N Engl J Med (1982) 306:523–7. doi:10.1056/NEJM198203043060907
60. Iyer LM, Aravind L, Coon SL, Klein DC, Koonin EV. Evolution of cell-cell signaling in animals: did late horizontal gene transfer from bacteria have a role? Trends Genet (2004) 20:292–9. doi:10.1016/j.tig.2004.05.007
61. Saulnier DM, Ringel Y, Heyman MB, Foster JA, Bercik P, Shulman RJ, et al. The intestinal microbiome, probiotics and prebiotics in neurogastroenterology. Gut Microbes (2013) 4(1):17–27. doi:10.4161/gmic.22973
62. Waters KA, Meehan B, Huang JQ, Gravel RA, Michaud J, Côté A. Neuronal apoptosis in sudden infant death syndrome. Pediatr Res (1999) 45(2):166–72. doi:10.1203/00006450-199902000-00002
63. Kinney HC, Thach BT. The sudden infant death syndrome. N Engl J Med (2009) 361(8):795–805. doi:10.1056/NEJMra0803836
64. Prabhakar NR, Marek W, Loeschcke HH. Altered breathing pattern elicited by stimulation of abdominal visceral afferents. J Appl Physiol (1985) 58(6):1755–60.
65. Glogowska M. The significance of afferent vagal information in the control of breathing in guinea pigs. Acta Neurobiol Exp (1975) 35:139–47.
66. Azmitia EC. Modern views on an ancient chemical: serotonin effects on cell proliferation, maturation, and apoptosis. Brain Res Bull (2001) 56(5):413–24. doi:10.1016/S0361-9230(01)00614-1
67. Doucet E, Miquel MC, Nosjean A, Verge D, Hamon M, Emerit MB. Immunolabeling of the rat central nervous system with antibodies partially selective of the short form of the 5-HT3 receptor. Neuroscience (2000) 95:881–92. doi:10.1016/S0306-4522(99)00494-7
68. Steward LJ, West KE, Kilpatrick GJ, Barnes NM. Labeling of 5-HT3 receptor recognition sites in the rat brain using the agonist radio-ligand [3H]meta-chlorophenylbiguanide. Eur J Pharmacol (1993) 243:13–8. doi:10.1016/0014-2999(93)90161-A
69. Kidd EJ, Laporte AM, Langlois X, Fattaccini CM, Doyen C, Lombard MC, et al. 5-HT3 receptors in the rat central nervous system are mainly located on nerve fibers and terminals. Brain Res (1990) 612:289–98. doi:10.1016/0006-8993(93)91674-H
70. Leslie RA, Reynolds DJ, Andrews PL, Grahame-Smith DG, Davis CJ, Harvey JM. Evidence for presynaptic 5-hydroxytryptamine-3 recognition sites on vagal afferent terminals in the brainstem of the ferret. Neuroscience (1990) 38:667–73. doi:10.1016/0306-4522(90)90060-H
71. Pratt GD, Bowery NG. The 5-HT3 receptor ligand [3H]BRL 43694, binds to presynaptic sites in the nucleus tractus solitarius of the rat. Neuropharmacology (1989) 28:1367–76. doi:10.1016/0028-3908(89)90012-9
72. Wan S, Browning KN. Glucose increases synaptic transmission from vagal afferent central nerve terminals via modulation of 5-HT3 receptors. Am J Physiol Gastrointest Liver Physiol (2008) 295(5):G1050–7. doi:10.1152/ajpgi.90288.2008
73. Hillsley K, Grundy D. Sensitivity to 5-hydroxytryptamine in different afferent subpopulations within mesenteric nerves supplying the rat jejunum. J Physiol (1998) 509:717–27. doi:10.1111/j.1469-7793.1998.717bm.x
74. Li Y, Wu XY, Zhu JX, Owyang C. Intestinal serotonin acts as paracrine substance to mediate pancreatic secretion stimulated by luminal factors. Am J Physiol Gastrointest Liver Physiol (2001) 281:G916–23.
75. Raybould HE. Does your gut taste? Sensory transduction in the gastrointestinal tract. News Physiol Sci (1998) 13:275–80.
76. Raybould HE. Primary afferent response to signals in the intestinal lumen. J Physiol (2001) 530:343. doi:10.1111/j.1469-7793.2001.0343k.x
77. Raybould HE. Visceral perception: sensory transduction in visceral afferents and nutrients. Gut (2002) 251(Suppl 1):I11–4. doi:10.1136/gut.51.suppl_1.i11
78. Raybould HE, Glatzle J, Robin C, Meyer JH, Phan T, Wong H, et al. Expression of 5-HT3 receptors by extrinsic duodenal afferents contribute to intestinal inhibition of gastric emptying. Am J Physiol Gastrointest Liver Physiol (2003) 284:G367–72. doi:10.1152/ajpgi.00292.2001
79. Zhu JX, Wu XY, Owyang C, Li Y. Intestinal serotonin acts as a paracrine substance to mediate vagal signal transmission evoked by luminal factors in the rat. J Physiol (2001) 530:431–42. doi:10.1111/j.1469-7793.2001.0431k.x
80. Ruddick JP, Evans AK, Nutt DJ, Lightman SL, Rook GA, Lowry CA. Tryptophan metabolism in the central nervous system: medical implications. Expert Rev Mol Med (2006) 8:1–27. doi:10.1017/S1462399406000068
81. Yano JM, Yu K, Donaldson GP, Shastri GG, Ann P, Ma L, et al. Indigenous bacteria from the gut microbiota regulate host serotonin biosynthesis. Cell (2015) 161(2):264–76. doi:10.1016/j.cell.2015.02.047
82. Dahlstrom A, Fuxe K. Evidence for the existence of monoamine-containing neurons in the central nervous system. Acta Physiol Scand (1964) 62:1–55.
83. Quigley EM. Probiotics in functional gastrointestinal disorders: what are the facts? Curr Opin Pharmacol (2008) 8:704–8. doi:10.1016/j.coph.2008.08.007
84. Lyte M, Li W, Opitz N, Gaykema R, Goehler LE. Induction of anxiety-like behavior in mice during the initial stages of infection with the agent of murine colonic hyperplasia Citrobacter rodentium. Physiol Behav (2006) 89:350–7. doi:10.1016/j.physbeh.2006.06.019
85. Wang H, Lee IS, Braun C, Enck P. Effect of probiotics on central nervous system functions in animals and humans: a systematic review. J Neurogastroenterol Motil (2016) 22(4):589–605. doi:10.5056/jnm16018
86. Sudo N, Chida Y, Aiba Y, Sonoda J, Oyama N, Yu XN, et al. Postnatal microbial colonization programs the hypothalamic-pituitary-adrenal system for stress response in mice. J Physiol (Lond) (2004) 558:263–75. doi:10.1113/jphysiol.2004.063388
87. Bravo JA, Forsythe P, Chew MV, Escaravage E, Savignac HM, Dinan TG, et al. Ingestion of Lactobacillus strain regulates emotional behavior and central GABA receptor expression in a mouse via the vagus nerve. Proc Natl Acad Sci U S A (2011) 108(38):16050–5. doi:10.1073/pnas.1102999108
88. Desbonnet L, Garrett L, Clarke G, Bienenstock J, Dinan TG. The probiotic Bifidobacteria infantis: an assessment of potential antidepressant properties in the rat. J Psychiatr Res (2008) 43:164–74. doi:10.1016/j.jpsychires.2008.03.009
89. Alenina N, Kikic D, Todiras M, Mosienko V, Qadri F, Plehm R, et al. Growth retardation and altered autonomic control in mice lacking brain serotonin. Proc Natl Acad Sci U S A (2009) 106(25):10332–7. doi:10.1073/pnas.0810793106
90. Gareau MG, Wine E, Rodrigues DM, Cho JH, Whary MT, Philpott DJ, et al. Bacterial infection causes stress-induced memory dysfunction in mice. Gut (2011) 60:307–17. doi:10.1136/gut.2009.202515
91. McVey Neufeld KA, Mao YK, Bienenstock J, Foster JA, Kunze WA. The microbiome is essential for normal gut intrinsic primary afferent neuron excitability in the mouse. Neurogastroenterol Motil (2013) 25(2):183–e88. doi:10.1111/nmo.12049
92. Heijtz RD, Wang S, Anuar F, Qian Y, Bjorkholm B, Samuelsson A, et al. Normal gut microbiota modulates brain development and behavior. Proc Natl Acad Sci U S A (2011) 108:3047–52. doi:10.1073/pnas.1010529108
93. Parracho HM, Bingham MO, Gibson GR, Mccartney AL. Differences between the gut microflora of children with autistic spectrum disorders and that of healthy children. J Med Microbiol (2005) 54:987–91. doi:10.1099/jmm.0.46101-0
94. Messaoudi M, Violle N, Bisson J, Desor D, Javelot H, Rugeot C. Beneficial psychological effects of a probiotic formulation (Lactobacillus helveticus R0052 and Bifidobacterium longum R0175) in healthy human volunteers. Gut Microbes (2011) 2(4):256–61. doi:10.4161/gmic.2.4.16108
95. Kiliaan AJ, Saunders PR, Bijlsma PB, Berin MC, Taminiau JA, Groot JA, et al. Stress stimulates transepithelial macromolecular uptake in rat jejunum. Am J Physiol (1998) 275:G1037–44.
96. Bailey MT, Lubach GR, Coe CL. Prenatal stress alters bacterial colonization of the gut in infant monkeys. J Pediatr Gastroenterol Nutr (2004) 38:414–21. doi:10.1097/00005176-200404000-00009
97. Bailey MT, Coe CL. Maternal separation disrupts the integrity of the intestinal microflora in infant rhesus monkeys. Dev Psychobiol (1999) 35:146–55. doi:10.1002/(SICI)1098-2302(199909)35:2<146::AID-DEV7>3.0.CO;2-G
98. Blackwell CC, Moscovis SM, Gordon AE, Al Madani OM, Hall ST, Gleeson M, et al. Ethnicity, infection and sudden infant death syndrome. FEMS Immunol Med Microbiol (2004) 42(123):53–65. doi:10.1016/j.femsim.2004.06.007
99. Kinney HC, Randall LL, Sleeper LA, Willinger M, Belliveau RA, Zec N, et al. Serotonergic brainstem abnormalities in Northern Plains Indians with the sudden infant death syndrome. J Neuropathol Exp Neurol (2003) 62(11):1178–91. doi:10.1093/jnen/62.11.1178
100. Arumugam M, Raes J, Pelletier E, Le Paslier D, Yamada T, Mende DR, et al. Enterotypes of the human gut microbiome. Nature (2011) 473(7346):174–80. doi:10.1038/nature09944
101. O’Keefe SJ, Chung D, Mahmoud N, Sepulveda AR, Manafe M, Arch J, et al. Why do African Americans get more colon cancer than Native Africans. J Nutr (2007) 137(1 Suppl):175S–82S.
102. Stockwell EG, Swanson DA, Wicks JW. Economic status differences in infant mortality by cause of death. Public Health Rep (1988) 103:135–42.
103. Mello RM, Morais MB, Tahan S, Melli LC, Rodrigues MS, Mello CS, et al. Lactobacilli and bifidobacteria in the feces of schoolchildren of two different socioeconomic groups: children from a favela and children from a private school. J Pediatr (Rio J) (2009) 85(4):307–14. doi:10.1590/S0021-75572009000400007
104. Jones MD, Lucki I. Sex differences in the regulation of serotonergic transmission and behavior in 5-HT receptor knockout mice. Neuropsychopharmacology (2005) 30:1039–47. doi:10.1038/sj.npp.1300664
105. Maswood S, Truitt W, Hotema M, Caldarola-Pastuszka M, Uphouse L. Estrous cycle modulation of extracellular serotonin in mediobasal hypothalamus: role of the serotonin transporter and terminal autoreceptors. Brain Res (1999) 831:146–54. doi:10.1016/S0006-8993(99)01439-0
106. Bethea CL, Lu NZ, Gundlah C, Streicher JM. Diverse actions of ovarian steroids in the serotonin neural system. Front Neuroendocrinol (2002) 23:41–100. doi:10.1006/frne.2001.0225
107. Imwalle DB, Gustafsson JA, Rissman EF. Lack of functional estrogen receptor beta influences anxiety behavior and serotonin content in female mice. Physiol Behav (2005) 84:157–63. doi:10.1016/j.physbeh.2004.11.002
108. Kovacs A, Ben-Jacob N, Tayem H, Halperin E, Iraqi FA, Gophna U. Genotype is a stronger determinant than sex of the mouse gut microbiota. Microb Ecol (2011) 61:423–8. doi:10.1007/s00248-010-9787-2
109. Costello EK, Stagaman K, Dethlefsen L, Bohannan BJ, Relman DA. The application of ecological theory toward an understanding of the human microbiome. Science (2012) 336:1255–62. doi:10.1126/science.1224203
110. Freire AC, Basit AW, Choudhary R, Piong CW, Merchant HA. Does sex matter? The influence of gender on gastrointestinal physiology and drug delivery. Int J Pharm (2011) 415:15–28. doi:10.1016/j.ijpharm.2011.04.069
111. Koren O, Goodrich JK, Cullender TC, Spor A, Laitinen K, Bäckhed HK, et al. Host remodeling of the gut microbiome and metabolic changes during pregnancy. Cell (2012) 150:470–80. doi:10.1016/j.cell.2012.07.008
112. Markle JG, Frank DN, Mortin-Toth S, Robertson CE, Feazel LM, Rolle-Kampczyk U, et al. Sex differences in the gut microbiome drive hormone-dependent regulation of autoimmunity. Science (2013) 339:1084–8. doi:10.1126/science.1233521
113. Bolnick DI, Snowberg LK, Hirsch PE, Lauber CL, Org E, Parks B, et al. Individual diet has sex-dependent effects on vertebrate gut microbiota. Nat Commun (2014) 5:4500. doi:10.1038/ncomms5500
114. Opdal SH, Rognum TO. Gene variants predisposing to SIDS: current knowledge. Forensic Sci Med Pathol (2011) 7:26–36. doi:10.1007/s12024-010-9182-9
115. Rawls JF, Mahowald MA, Ley RE, Gordon JI. Reciprocal gut microbiota transplants from zebrafish and mice to germ-free recipients reveal host habitat selection. Cell (2006) 127:423–33. doi:10.1016/j.cell.2006.08.043
116. Toivanen P, Vaahtovuo J, Eerola E. Influence of major histocompatibility complex on bacterial composition of fecal flora. Infect Immun (2001) 69(4):2372–7. doi:10.1128/IAI.69.4.2372-2377.2001
117. Poetsch M, Czerwinski M, Wingenfeld L, Vennemann M, Bajanowski T. A common FMO3 polymorphism may amplify the effect of nicotine exposure in sudden infant death syndrome (SIDS). Int J Legal Med (2010) 124:301–6. doi:10.1007/s00414-010-0428-6
118. Naeye RL, Ladis B, Drage JS. Sudden infant death syndrome. A prospective study. Am J Dis Child (1976) 130:1207–10.
119. Machaalani R, Say M, Waters KA. Serotoninergic receptor 1A in the sudden infant death syndrome brainstem medulla and associations with clinical risk factors. Acta Neuropathol (2009) 117:257–65. doi:10.1007/s00401-008-0468-x
120. Say M, Machaalani R, Waters KA. Changes in serotoninergic receptors 1A and 2A in the piglet brainstem after intermittent hypercapnic hypoxia (IHH) and nicotine. Brain Res (2007) 1152:17–26. doi:10.1016/j.brainres.2007.03.037
121. Biedermann L, Zeitz J, Mwinyi J, Sutter-Minder E, Rehman A, Ott SJ, et al. Smoking cessation induces profound changes in the composition of the intestinal microbiota in humans. PLoS One (2013) 8(3):e59260. doi:10.1371/journal.pone.0059260
122. Carroll-Pankhurst C, Mortimer EA. Sudden infant death syndrome, bedsharing, parental weight, and age at death. Pediatrics (2001) 107:530–6. doi:10.1542/peds.107.3.530
123. Ley RE, Turnbaugh PJ, Klein S, Gordon JI. Microbial ecology: human gut microbes associated with obesity. Nature (2006) 21(444):1022–3. doi:10.1038/4441022a
124. Gohir W, Ratcliffe EM, Sloboda DM. Of the bugs that shape us: maternal obesity, the gut microbiome, and long-term disease risk. Pediatr Res (2015) 77:196–204. doi:10.1038/pr.2014.169
125. Sanghavi DM. Epidemiology of sudden infant death syndrome (SIDS) for Kentucky infants born in 1990: maternal, prenatal, and perinatal risk factors. J Ky Med Assoc (1995) 93(7):286–90.
126. Biasucci G, Benenati B, Morelli L, Bessi E, Boehm G. Cesarean delivery may affect the early biodiversity of intestinal bacteria. J Nutr (2008) 138(9):1796S–800S.
127. Salminen S, Gibson GR, McCartney AL, Isolauri E. Influence of mode of delivery on gut microbiota composition in seven year old children. Gut (2004) 53(9):1388–9. doi:10.1136/gut.2004.041640
128. Hallstrom M, Eerola E, Vuento R, Janas M, Tammela O. Effects of mode of delivery and necrotising enterocolitis on the intestinal microflora in preterm infants. Eur J Clin Microbiol Infect Dis (2004) 23(6):463–70. doi:10.1007/s10096-004-1146-0
129. Gronlund MM, Lehtonen OP, Eerola E, Kero P. Fecal microflora in healthy infants born by different methods of delivery: permanent changes in intestinal flora after cesarean delivery. J Pediatr Gastroenterol Nutr (1999) 28(1):19–25. doi:10.1097/00005176-199901000-00007
130. Bettelheim KA, Breadon A, Faiers MC, O’Farrell SM, Shooter RA. The origin of O serotypes of Escherichia coli in babies after normal delivery. J Hyg (Lond) (1974) 72(1):67–70. doi:10.1017/S0022172400023226
131. Brook I, Barrett CT, Brinkman CR III, Martin WJ, Finegold SM. Aerobic and anaerobic bacterial flora of the maternal cervix and newborn gastric fluid and conjunctiva: a prospective study. Pediatrics (1979) 63(3):451–5.
132. Lennox-King SM, O’Farrell SM, Bettelheim KA, Shooter RA. Escherichia coli isolated from babies delivered by caesarean section and their environment. Infection (1976) 4(3):139–45. doi:10.1007/BF01638940
133. de La Cochetière MF, Rougé C, Darmaun D, Rozé JC, Potel G, Leguen CG. Intestinal microbiota in neonates and preterm infants: a review. Curr Pediatr Rev (2007) 3:21–34. doi:10.2174/157339607779941697
134. Osmond C, Murphy M. Seasonality in the sudden infant death syndrome. Paediatr Perinat Epidemiol (1988) 2:337–45. doi:10.1111/j.1365-3016.1988.tb00228.x
135. Hoffman HJ, Damus K, Hillman L, Krongrad E. Risk factors for SIDS. Results of the National Institute of Child Health and Human Development SIDS Cooperative Epidemiological Study. Ann N Y Acad Sci (1988) 533:13–30. doi:10.1111/j.1749-6632.1988.tb37230.x
136. Nelson AM, Walk ST, Taube S, Taniuchi M, Houpt ER, Wobus CE, et al. Disruption of the human gut microbiota following norovirus infection. PLoS One (2012) 7(10):e48224. doi:10.1371/journal.pone.0048224
137. Hunt CE. Small for gestational age infants and sudden infant death syndrome: a confluence of complex conditions. Arch Dis Child Fetal Neonatal Ed (2007) 92:F428–9. doi:10.1136/adc.2006.112243
138. Sakata H, Yoshioka H, Fujita K. Development of the intestinal flora in very low birth weight infants compared to normal full-term newborns. Eur J Pediatr (1985) 144(2):186–90.
139. Willinger M, Hoffman HJ, Hartford RB. Infant sleep position and risk for sudden infant death syndrome: report of meeting held January 13 and 14, 1994, National Institutes of Health, Bethesda, MD. Pediatrics (1994) 93:814–9.
140. Chong A, Murphy N, Matthews T. Effect of prone sleeping on circulatory control in infants. Arch Dis Child (2000) 82(3):253–6. doi:10.1136/adc.82.3.253
141. Ponsonby AL, Dwyer T, Gibbons LE, Cochrane JA, Wang YG. Factors potentiating the risk of sudden infant death syndrome associated with the prone position. N Engl J Med (1993) 329(6):377–82. doi:10.1056/NEJM199308053290601
142. McKenna JJ, McDade T. Why babies should never sleep alone: a review of the co-sleeping controversy in relation to SIDS, bedsharing and breast feeding. Paediatr Respir Rev (2005) 6:134–52. doi:10.1016/j.prrv.2005.03.006
143. Hauck FR, Thompson JM, Tanabe KO, Moon RY, Vennemann MM. Breastfeeding and reduced risk of sudden infant death syndrome: a meta-analysis. Pediatrics (2011) 128(1):103–10. doi:10.1542/peds.2010-3000
144. Horne RS, Parslow PM, Ferens D, Watts AM, Adamson TM. Comparison of evoked arousability in breast and formula fed infants. Arch Dis Child (2004) 89(1):22–5.
145. Ponsonby AL, Dwyer T, Gibbons LE, Cochrane JA, Jones ME, McCall MJ. Thermal environment and sudden infant death syndrome: case-control study. BMJ (1992) 304(6822):277–82. doi:10.1136/bmj.304.6822.277
146. Conn CA, Franklin B, Freter R, Kluger MJ. Role of Gram-negative and Gram-positive gastrointestinal flora in temperature regulation of mice. Am J Physiol (1991) 6(2):R1358–63.
147. Schmidt JP, Becker RE. Changes in the Intestinal Flora of Ground Squirrels during Periods of Hibernation. Fort Wainwright, Alaska: Arctic Aeromedical Laboratory, Aerospace Medical Division, Air Force Systems Command (1963).
148. Allen SD, Brock TD. The temperature optimum of the intestinal flora of the rat. Can J Microbiol (1968) 14(6):699–704. doi:10.1139/m68-116
149. Fuller A, Mitchell D. Oral antibiotics reduce body temperature of healthy rabbits in a thermoneutral environment. J Basic Clin Physiol Pharmacol (1999) 10(1):1–13. doi:10.1515/JBCPP.1999.10.1.1
150. Ollila P, Niemelä M, Uhari M, Larmas M. Risk factors for colonization of salivary lactobacilli and Candida in children. Acta Odontol Scand (1997) 55(1):9–13. doi:10.3109/00016359709091933
151. Sherburn RE, Jenkins RO. Aerial release of bacteria from cot mattress materials and the sudden infant death syndrome. J Appl Microbiol (2005) 98(2):293–8. doi:10.1111/j.1365-2672.2004.02456.x
152. Carpenter R, McGarvey C, Mitchell EA, Tappin DM, Vennemann MM, Smuk M, et al. Bed sharing when parents do not smoke: is there a risk of SIDS? An individual level analysis of five major case-control studies. BMJ Open (2013) 3(5):e002299. doi:10.1136/bmjopen-2012-002299
153. Lindberg E, Adlerberth I, Hesselmar B, Saalman R, Strannegård IL, Aberg N, et al. High rate of transfer of Staphylococcus aureus from parental skin to infant gut flora. J Clin Microbiol (2004) 42(2):530–4. doi:10.1128/JCM.42.2.530-534.2004
154. Weissbluth M. Infantile colic and near-miss sudden infant death syndrome. Med Hypotheses (1981) 7(9):1193–9. doi:10.1016/0306-9877(81)90062-1
155. Duffy LC. Interactions mediating bacterial translocation in the immature intestine. J Nutr (2000) 130(2S Suppl):432S–6S.
156. Edde L, Hipolito RB, Hwang FF, Headon DR, Shalwitz RA, Sherman MP. Lactoferrin protects neonatal rats from gut-related systemic infection. Am J Physiol Gastrointest Liver Physiol (2001) 281(5):G1140–50.
157. Moy J, Lee DJ, Harmon CM, Drongowski RA, Coran AG. Confirmation of translocated gastrointestinal bacteria in a neonatal model. J Surg Res (1999) 87(1):85–9. doi:10.1006/jsre.1999.5745
158. Seehofer D, Rayes N, Schiller R, Stockmann M, Müller AR, Schirmeier A, et al. Probiotics partly reverse increased bacterial translocation after simultaneous liver resection and colonic anastomosis in rats. J Surg Res (2004) 117(2):262–71. doi:10.1016/j.jss.2003.11.021
159. Yajima M, Nakayama M, Hatano S, Yamazaki K, Aoyama Y, Yajima T, et al. Bacterial translocation in neonatal rats: the relation between intestinal flora, translocated bacteria, and influence of milk. J Pediatr Gastroenterol Nutr (2001) 33(5):592–601. doi:10.1097/00005176-200111000-00015
160. Katayama M, Xu D, Specian RD, Deitch EA. Role of bacterial adherence and the mucus barrier on bacterial translocation: effects of protein malnutrition and endotoxin in rats. Ann Surg (1997) 225(3):317–26. doi:10.1097/00000658-199703000-00012
161. Clapp DW. Developmental regulation of the immune system. Semin Perinatol (2006) 30(2):69–72. doi:10.1053/j.semperi.2006.02.004
162. Lee DJ, Drongowski RA, Coran AG, Harmon CM. Evaluation of probiotic treatment in a neonatal animal model. Pediatr Surg Int (2000) 16(4):237–42. doi:10.1007/s003830050736
163. Mussi-Pinhata MM, Rego MA. [Immunological peculiarities of extremely preterm infants: a challenge for the prevention of nosocomial sepsis]. J Pediatr (Rio J) (2005) 81(1 Suppl):S59–68. doi:10.2223/1301
164. Zhang B, Ohtsuka Y, Fujii T, Baba H, Okada K, Shoji H, et al. Immunological development of preterm infants in early infancy. Clin Exp Immunol (2005) 140(1):92–6. doi:10.1111/j.1365-2249.2005.02741.x
165. Schwiertz A, Gruhl B, Lobnitz M, Michel P, Radke M, Blaut M. Development of the intestinal bacterial composition in hospitalized preterm infants in comparison with breast-fed, full-term infants. Pediatr Res (2003) 54(3):393–9. doi:10.1203/01.PDR.0000078274.74607.7A
166. Alm B, Norvenius SG, Wennergren G, Skjaerven R, Oyen N, Milerad J, et al. Changes in epidemiology of sudden infant death syndrome in Sweden 1973–1996. Arch Dis Child (2001) 84:24–30. doi:10.1136/adc.84.1.24
167. Getahun D, Amre K, Rhoads GG, demissie K. Maternal and obstetric risk factors for sudden infant death syndrome in the United States. Obstet Gynecol (2004) 103:646–52. doi:10.1097/01.AOG.0000117081.50852.04
168. Malloy MH, MacDorman M. Changes in the classification of sudden unexpected infant deaths: United States, 1992–2001. Pediatrics (2005) 115:1247–53. doi:10.1542/peds.2004-2188
169. D’Inca R, Kloareg M, Gras-Le Guen C, Le Huerou-Luron I. Intrauterine growth restriction modifies the developmental pattern of intestinal structure, transcriptomic profile, and bacterial colonization in neonatal pigs. J Nutr (2010) 140:925–31. doi:10.3945/jn.109.116822
Keywords: 5-HT, SIDS, gut–brain axis, gut flora, autoresuscitation
Citation: Praveen V and Praveen S (2017) Microbiome–Gut–Brain Axis: A Pathway for Improving Brainstem Serotonin Homeostasis and Successful Autoresuscitation in SIDS—A Novel Hypothesis. Front. Pediatr. 4:136. doi: 10.3389/fped.2016.00136
Received: 24 October 2016; Accepted: 30 November 2016;
Published: 06 January 2017
Edited by:
Frederick Robert Carrick, Bedfordshire Centre for Mental Health Research in Association with University of Cambridge, UKReviewed by:
Bernhard J. Mitterauer, Volitronics-Institute for Basic Research Psychopathology and Brain Philosophy, AustriaAnna Maria Lavezzi, University of Milan, Italy
Copyright: © 2017 Praveen and Praveen. This is an open-access article distributed under the terms of the Creative Commons Attribution License (CC BY). The use, distribution or reproduction in other forums is permitted, provided the original author(s) or licensor are credited and that the original publication in this journal is cited, in accordance with accepted academic practice. No use, distribution or reproduction is permitted which does not comply with these terms.
*Correspondence: Vijayakumar Praveen, ZHJwcmF2ZWVudmlqYXkmI3gwMDA0MDtob3RtYWlsLmNvbQ==