- 1Centro Internacional de Entrenamiento e Investigaciones Médicas (CIDEIM), Cali, Colombia
- 2Universidad Icesi, Cali, Colombia
- 3Department of Environmental Health, Harvard T.H. Chan School of Public Health, Boston, MA, United States
- 4Faculty of Biology, Technion-Israel Institute of Technology, Haifa, Israel
- 5Department of Cell Biology and Molecular Genetics, University of Maryland, College Park, MD, United States
- 6Center for Bioinformatics and Computational Biology, University of Maryland, College Park, MD, United States
Host cell functions that participate in the pharmacokinetics and pharmacodynamics (PK/PD) of drugs against intracellular pathogen infections are critical for drug efficacy. In this study, we investigated whether macrophage mechanisms of xenobiotic detoxification contribute to the elimination of intracellular Leishmania upon exposure to pentavalent antimonials (SbV). Primary macrophages from patients with cutaneous leishmaniasis (CL) (n=6) were exposed ex vivo to L. V. panamensis infection and SbV, and transcriptomes were generated. Seven metallothionein (MT) genes, potent scavengers of heavy metals and central elements of the mammalian cell machinery for xenobiotic detoxification, were within the top 20 up-regulated genes. To functionally validate the participation of MTs in drug-mediated killing of intracellular Leishmania, tandem knockdown (KD) of MT2-A and MT1-E, MT1-F, and MT1-X was performed using a pan-MT shRNA approach in THP-1 cells. Parasite survival was unaffected in tandem-KD cells, as a consequence of strong transcriptional upregulation of MTs by infection and SbV, overcoming the KD effect. Gene silencing of the metal transcription factor-1 (MTF-1) abrogated expression of MT1 and MT2-A genes, but not ZnT-1. Upon exposure to SbV, intracellular survival of Leishmania in MTF-1KD cells was significantly enhanced. Results from this study highlight the participation of macrophage MTs in Sb-dependent parasite killing.
Introduction
Cutaneous leishmaniasis (CL) is caused by the intracellular protozoan parasite Leishmania, and affects more than 1.2 million people annually (Alvar et al., 2012). CL is endemic throughout Central and South America, where control remains dependent on chemotherapy with pentavalent antimonials (SbV). The high rates of treatment failure (as high as 30% in controlled clinical trials), toxicity, and the difficulties associated with access to these drugs limit this control strategy (Romero et al., 2001; Croft et al., 2006; Arevalo et al., 2007; Firdous et al., 2009; Oliveira et al., 2011; Monge-Maillo and López-Vélez, 2013; Sundar and Chakravarty, 2015). The direct activity of antimicrobials against intracellular pathogens is dependent on drug internalization into host cells and on host cell processes that mediate drug metabolism (activation/inactivation). This constitutes a challenge for the design of new drugs and the optimization of existing ones, because host cells act as an additional barrier for drug exposure of the intracellular microbe.
Antimony (Sb) is a metalloid closely related to arsenic (As). Sb exposure induces stress responses leading to activation of mechanisms of redox balance control and metal/xenobiotic detoxification (Cousins, 1994; Roesijadi, 2000; Lima et al., 2010; Coelho et al., 2014). Thus, factors mediating these responses could modulate Sb bioavailability within host cells, impacting the intracellular pharmacokinetics and pharmacodynamics (PK/PD) of these drugs. Illustrating this is evidence of the participation of macrophage ABC transporters in antileishmanial drug effects: Sb modulates the expression of macrophage ATP Binding Cassette (ABC) transporters (Gómez et al., 2014; Barrera et al., 2017). ABCC1, ABCB1 and ABCB5 have been shown to function as Sb efflux pumps in L. donovani (Mookerjee Basu et al., 2008) and L.V. braziliensis infected macrophages (Téllez et al., 2017), favoring intracellular parasite survival. ABCB6 can function as a plasma membrane Sb efflux transporter and as an intracellular Sb importer, potentially increasing drug concentrations within the phagolysosome, favoring intracellular L.V. panamensis killing (Gómez et al., 2014).
Expression of other metal stress responsive genes is also modulated upon Sb exposure (Gómez et al., 2014; Téllez et al., 2017). Among these is metallothionein 2A (MT2-A), a small cysteine-rich cytoplasmic protein involved in zinc homeostasis and the scavenging of metals and electrophilic molecules such as reactive oxygen species (ROS) and nitric oxide (NO) (Nielson et al., 1985; Thornalley and Vašák, 1985; Hamer, 1986; Andrews, 2000; Park et al., 2001; Vašák and Meloni, 2011). There are four MT families (MT1-4): MT1 and MT2 are ubiquitously expressed, whereas MT3 and MT4 are found in the central nervous system and in stratified squamous epithelium, respectively (Hamer, 1986; Namdarghanbari et al., 2011). MTs can bind toxic metals with high affinity such as Cd, Hg, Pd, Ag, As, and Sb (Nielson et al., 1985), resulting in toxic metal tolerance and detoxification (Satoh et al., 1997; Park et al., 2001; Namdarghanbari et al., 2011). This efficient metal scavenging function results from the high thiol content of MTs and the tight regulation of MTs gene expression, which can increase more than 100 fold under metal stress, reaching intracellular protein concentrations of the order of millimolar (Durnam and Palmiter, 1981; Hamer, 1986; Namdarghanbari et al., 2011).
Our group and others have provided evidence for the participation of host MTs in the Leishmania-macrophage interactions: a) Leishmania infection and SbV can strongly induce the expression of MT2-A in human macrophages (Gregory et al., 2008; Gómez et al., 2014; Fernandes et al., 2016); b) an inverse correlation of MT2-A gene expression and intracellular survival of Leishmania during in vitro SbV exposure has been reported (Gómez et al., 2014); c) an Sb-susceptible L. V. panamensis strain induced higher expression of macrophage MT2-A compared with its Sb-resistant counterart, suggesting strain-specific manipulation of MT2-A expression within macrophages (Barrera et al., 2017). However, the role of MTs in their response to antimony and their relationship to antileishmanial drug effects and intracellular parasite survival remains unknown. Based on the above, we sought to dissect and functionally validate the participation of MTs in the Sb-mediated killing of intracellular Leishmania.
Materials and methods
Ethics statement
This study was approved and monitored by the institutional review board for ethical conduct of research involving human subjects of the Centro Internacional de Entrenamiento e Investigaciones Médicas - CIDEIM, in accordance with national (resolution 008430, República de Colombia, Ministry of Health, 1993) and international (Declaration of Helsinki and amendments, World Medical Association, Fortaleza, Brazil, October 2013) guidelines. All individuals voluntarily participated in the study and written informed consent was obtained from each participant.
Reagents and chemicals
Additive-free meglumine antimoniate (MA) (Walter Reed 214975AK; lot no. BLO918690-278-1A1W601) was kindly provided by the Walter Reed Army Institute, Silver Spring, MD, USA. Phorbol-12-myristate 13-acetate was purchased from Sigma–Aldrich and zinc acetate dihydrate from J.T. Baker.
Subjects
Six adult patients, 18 to 65 years of age, with parasitological diagnosis of CL and time of lesion evolution <6 months, without apparent immune deficiencies (negative HIV test, no evidence of immunological disorder nor treatment with medication having immunomodulating effects), participated in this study. For in vitro primary macrophage differentiation, peripheral blood mononuclear cells (PBMCs) were obtained from study participants by separation using a Ficoll-Hypaque (Sigma-Aldrich) gradient.
THP1 and primary macrophage differentiation
The human pro-monocytic cell line THP-1 and derived lines were maintained at 1 x 106 (Firdous et al., 2009) cells/mL in RPMI 1640 supplemented with 10% heat inactivated FBS, 100 μg/mL streptomycin, 100 U/mL penicillin, 5 µg/mL puromycin (only for maintenance of transfected cells lines), at 37°C and 5% CO2. THP-1 monocytes were differentiated with 250 ng/mL of PMA for 3 hours, washed twice with D-PBS and cultured 24h in 6 well plates. Human PBMC-derived monocytes were differentiated to macrophages by adherence to cell culture plastic-ware as previously described (Dohmen et al., 2016).
Parasites, infection and intracellular parasite survival assays
Antimony susceptible L. (V.) panamensis promastigotes (MHOM/CO/2002/3594) were kept at 25°C in RPMI supplemented with 10% heat-inactivated FBS, 100 μg/mL streptomycin, 100 U/mL penicillin. Primary human macrophages and differentiated THP-1 cells were infected with human AB+ serum-opsonized stationary phase promastigotes at 10:1 Leishmania-macrophage ratio for 2h, washed twice with D-PBS and incubated for 24h at 34°C, 5% CO2. After infection was established, cells were exposed for 24h or 48h to MA (8, 16, and 32 μg/mL), or left untreated as a control. Intracellular parasite survival was measured by RT-qPCR as previously described (Romero et al., 2010) (primers used in Supplementary Table S1).
RNA isolation, cDNA library preparation, and sequence analyses
Total RNA was isolated with Trizol from uninfected, infected, and drug-treated macrophages. RNA quality was assessed with an Agilent 2100 Bioanalyzer using RNAnano chips (Agilent). RNA Integrity Number (RIN) ≥ 7 was considered acceptable to continue with library construction. Poly-A enriched libraries were generated from macrophage RNA extracts using the Illumina TruSeq Standard mRNA preparation kit and checked via the Bioanalyzer and quantitative PCR (KAPA Biosystems). Paired-end reads (100 nt) were obtained using the Illumina HiSeq 1500 (BioProject ID PRJNA633893, Supplementary Table S2). Fastqc [Anon, (2020)] was used to evaluate sequencing quality; Trimmomatic (Bolger et al., 2014) filtered low-quality reads and trimmed bases when the mean quality score fell below a threshold phred score of 20. Reads were mapped against the human (Miga et al., 2014) (hg38) and L. V. panamensis (Aslett et al., 2010) genomes (v36ish) using tophat (Trapnell et al., 2012). HTSeq (Anders et al., 2015) was used to count reads mapping to each gene feature. The count tables were restricted to the set of protein coding genes and filtered to remove non-expressed and very weakly expressed genes. The remaining genes were assessed for significant outliers and batch effects by visualizations of normalized data. Library sizes and count densities were calculated on non-normalized data; pairwise correlation and distances, outlier detection, and principal component analysis (PCA) were performed on log2, cpm, quantile normalized data with and without accounting for batch in the model or surrogate estimation with sva (using svaseq or combat) (Leek et al., 2012). DESeq2 (Love et al., 2014) was used to perform differential expression analyses alongside a statistically uninformed basic method as a negative control (Supplementary Table S3). Differentially expressed genes were contrasted between control vs. infected and MA-treated primary human macrophages. Genes deemed significantly different according to DESeq2 (|log2FC| > 1.0 and a FDR adjusted p-value < 0.05) were passed to various ontology tools. Enrichment PPI network analysis was carried out using STRING 10.
Short hairpin RNA constructs
A lentivirus-based system was used for shRNA-mediated gene silencing in THP-1 monocytes as previously described (Zhou et al., 2012). At least two independent sets of oligonucleotide pairs for gene knockdown of human MTs and the transcription factor MTF-1 (Supplementary Table S4) were synthesized and cloned into the pLKO.1-TCR vector (Addgene, Cambridge, MA, USA); the same vector was also used as an empty vector control. Lentiviral particles were generated by co-transfection of endotoxin-free hairpin-containing pLKO.1-TCR, psPAX2 and MD2.G (Addgene) into HEK-293T cells. FuGENE HD (Roche) was used as the transfection reagent. Lentivirus-containing cell supernatant was collected 4 days after transfection and subsequently used to transduce THP-1 monocytes in medium containing 10 mg/mL polybrene in a proportion 1:1. Transduced cells were selected under puromycin pressure (5 µg/mL) for a minimum of 5 days. Gene knockdown was confirmed by RT–qPCR using SYBR green (Applied Biosystems) and TaqMan® Gene Expression Assays (Applied Biosystems, Supplementary Table 1). shRNA transduction was confirmed by DNA sequencing.
Cytotoxicity assays
PMA-differentiated THP-1 cells and derived cell lines were exposed to a dose range (8 μg/mL – 256 μg/mL) of MA for 72 hours. Cell viability was assessed by MTT assay (ATCC) (Supplementary Figure S1).
Statistical analysis
Based on the distribution of the data, differences in gene expression were tested with one-way analysis of variance and Tukey’s multiple comparisons test. Differences in variance for the remaining experiments were analyzed with unpaired t test. A significance level of p ≤ 0.05 was used for all statistical tests. Statistical analyses were performed using GraphPad Prism software (version 6).
Results
Metallothioneins are within the top 20 macrophages transcripts up-regulated by Leishmania infection and exposure to SbV
Peripheral blood mononuclear cell (PBMC)-derived macrophages from CL patients (n=6) were infected ex vivo with L.V. panamensis and exposed to SbV (32µg-Sb/mL, as meglumine antimoniate - MA). Following RNA-seq data collection, a total of 16,841 transcripts were detected. Principal Component Analysis (PCA) showed separation between uninfected/untreated control macrophages and those infected with L.V. panamensis and exposed to MA (Figure 1A). After filtering the differential expression (DE) data by |logFC| ≥ 2 and p ≤ 0.05, a set of 217 transcripts remained, of which 111 were up-regulated and 106 down-regulated (Supplementary Table 3). Interestingly, among the top twenty up-regulated transcripts, seven were metallothionein genes: MT1-E, MT1-F, MT1-G, MT1-H, MT1-M, MT1-X and MT2-A (Figure 1B; Supplementary Table 3).
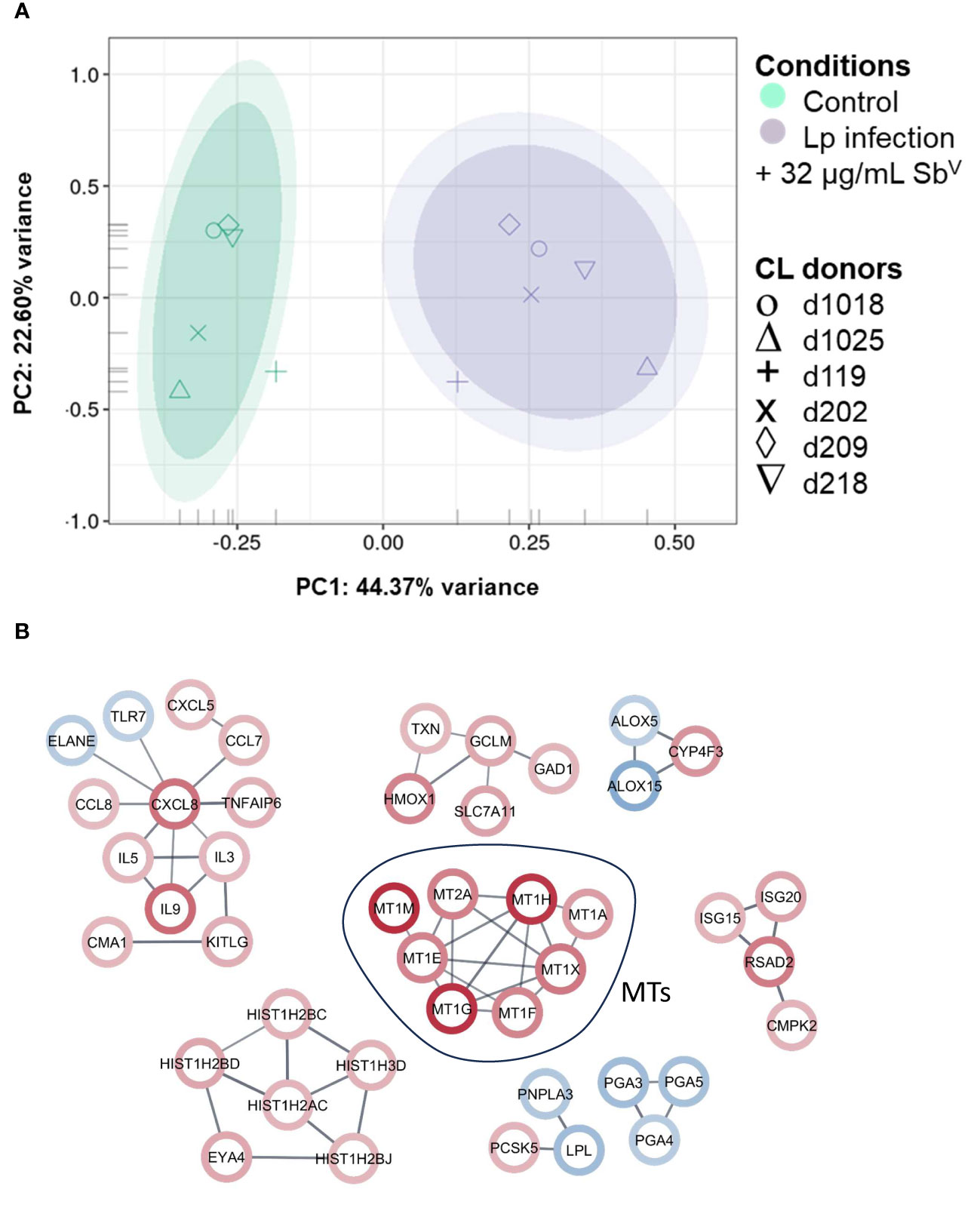
Figure 1 PCA plot and network analysis of macrophage transcriptomes. (A) PCA plot of RNA-seq data of primary macrophages from CL patients (n=6), which were infected in vitro with L.V. panamensis (Lp) and exposed to MA (32µg/mL SbV) for 24 h (purple) or were left uninfected and untreated as controls (green). Ovals represents confidence Interval-CI: 90% and 95% respectively. Each symbol represents each donor. (B) STRING network analysis with a |logFC|cutoff ≥ ± 2 and adjusted p value ≤ 0.05 (up-regulated genes: red circle; down-regulated genes: blue circle). Confidence of interaction set at 0.7.
Induction of MTs expression by zinc acetate enhances MA-mediated killing of Leishmania
To discern how MTs participate in the activity of antimonials, THP-1 cells were exposed to SbV and the expression of MT1-E, MT1-F, MT1-X, MT2-A, MT3 and MT4 was quantified (primer sequences available in Supplementary Table 1). Expression of MT1-X was the highest, peaking at 8-fold induction over untreated cells; followed by MT1-E, MT2-A and MT1-F (Figure 2A). MT3 and MT4 transcripts were not detected in macrophages.
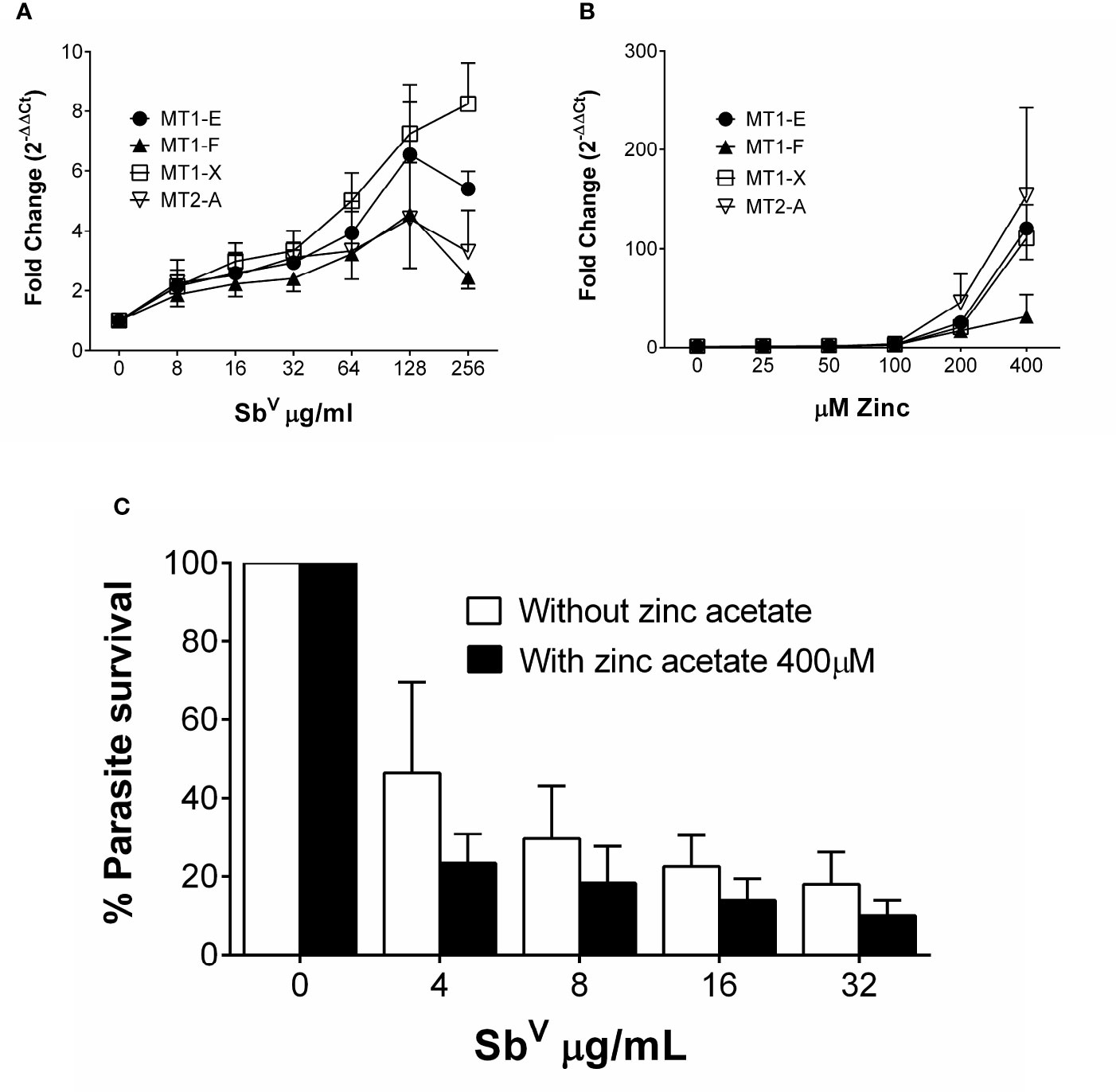
Figure 2 MTs are induced by zinc acetate and antimony. (A) Fold change gene expression of MTs in THP-1 cells exposed to sub-cytotoxic doses of SbV. (B) Fold change gene expression of MT1-E, 1-F, 1-X and MT2-A in THP-1 cells exposed to increasing doses of zinc acetate. (C) L.V. panamensis survival (%) in THP-1 cells pre-treated with 400 µM Zn-acetate for 24h and exposed to SbV (8, 16 and 32 µg/mL). THP-1 gene expression and parasite survival were quantified by RT-qPCR. Each experiment was run as 3 independent replicates. Data are shown as mean ± SD.
We evaluated the effect of maximal induction of MTs in intracellular Leishmania survival. THP-1 cells were exposed to a dose range of 25 µM to 400 µM Zn acetate for 24h, and peak expression of MTs (>100 fold) was observed with 400 µM Zn acetate (Namdarghanbari et al., 2011) (Figure 2B). Therefore, THP-1 cells were pre-treated for 24h with 400 µM Zn acetate, followed by L. V. panamensis infection for additional 24h, and exposed to increasing and non-cytotoxic concentrations of MA (Supplementary Figure 1). Pre-treatment with Zn acetate increased over 40% the Sb-dependent intracellular elimination of L.V. panamensis (Figure 2C), supporting the contribution of MTs to this phenotype.
Strong transcriptional up-regulation of MTs abrogates shRNA silencing of MT genes
An initial assessment of the participation of MT2-A gene silencing on intracellular survival of Leishmania did not show any significant effect (Supplementary Figure S2). This led us to hypothesize that a compensatory effect of other MTs maybe operating in our system. Taking advantage of the high sequence similarity of MT genes, an shRNA was constructed which targets the tandem knockdown -KD- (MT_tandemKD) of MT1 and MT2 family member genes (Figure 3A). Expression of MT1-E, 1F, 1X and MT2-A was efficiently silenced as shown in Figure 3B. To explore the phenotypic effects of MTs KD on intracellular parasite survival, MT_tandemKD cells were infected with L. V. panamensis and exposed to SbV. Despite effective KD of MT genes, intracellular parasite survival remained unchanged compared to empty-vector transfected control cells (Figure 3C). Considering that expression of MT genes is strongly induced by Leishmania and SbV, we questioned whether MTs knockdown was maintained during the experimental conditions (infection and drug exposure). Despite the efficient tandem KD of MTs at basal conditions, their strong transcriptional up-regulation during Leishmania and SbV exposure overcame the shRNA-silencing effect (Figure 3D), explaining why parasite survival was similar in MT_tandemKD and empty-vector control cells.
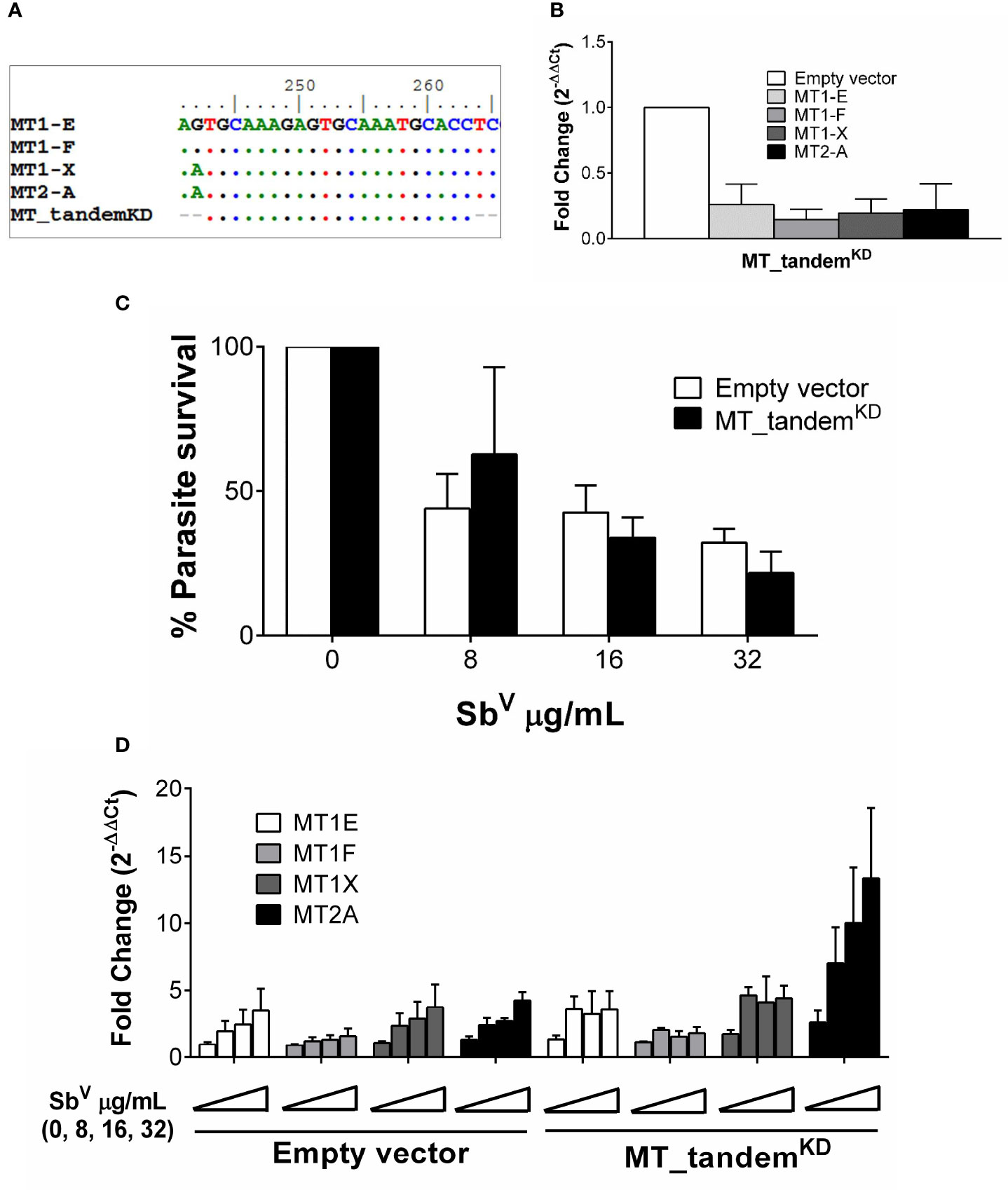
Figure 3 MTs tandem knockdown is abrogated by strong transcriptional up-regulation. (A) Sequence alignment of MT genes and MT_tandemKD oligo sequence showing the conserved region targeted for tandem shRNA. (B) Validation of the tandem KD shown by MTs gene expression in uninfected MT_tandemKD and empty-vector control cells. (C) Percentage of parasite survival in empty vector control and MT_tandemKD cells infected with L.V. panamensis and exposed to SbV (8, 16 and 32 µg/mL). (D) Fold change gene expression of MTs upon infection and exposure to SbV (8, 16 and 32 µg/mL) in empty vector control and MT_tandemKD cells. Gene expression and parasite survival were quantified by RT-qPCR.
Expression of MTs is silenced by MTF-1KD and favors survival of intracellular Leishmania
Metal transcription factor-1 (MTF-1) is the principal transcription factor regulating expression of MTs genes during metal-induced stress (Heuchel et al., 1994). We explored whether knockdown of MTF-1 could limit the transcriptional induction of MT genes in our experimental conditions. Using shRNA, the steady-state level of MTF-1 was knocked down by 50% (Figure 4A). Expression of MTs and slc30a1 (ZnT1) in MTF-1KD cells was evaluated under basal conditions and upon infection with L.V. panamensis and exposure to SbV. A slight reduction (ranging from 5% to 40%) of MT1 and MT2 genes expression was observed in uninfected and unstimulated MTF-1KD cells (Figure 4B). However, induction of MT1-E, F, X and MT2-A expression was completely repressed in L.V. panamensis infected cells subsequently exposed to Sb (Figure 4C). In the case of slc30a1 expression, we observed no significant differences in its expression (Supplementary Figure S3), suggesting that the effect of MTF-1KD on MT expression responds from a more specific mechanisms triggered by the presence of xenobiotic metals such as aSb.
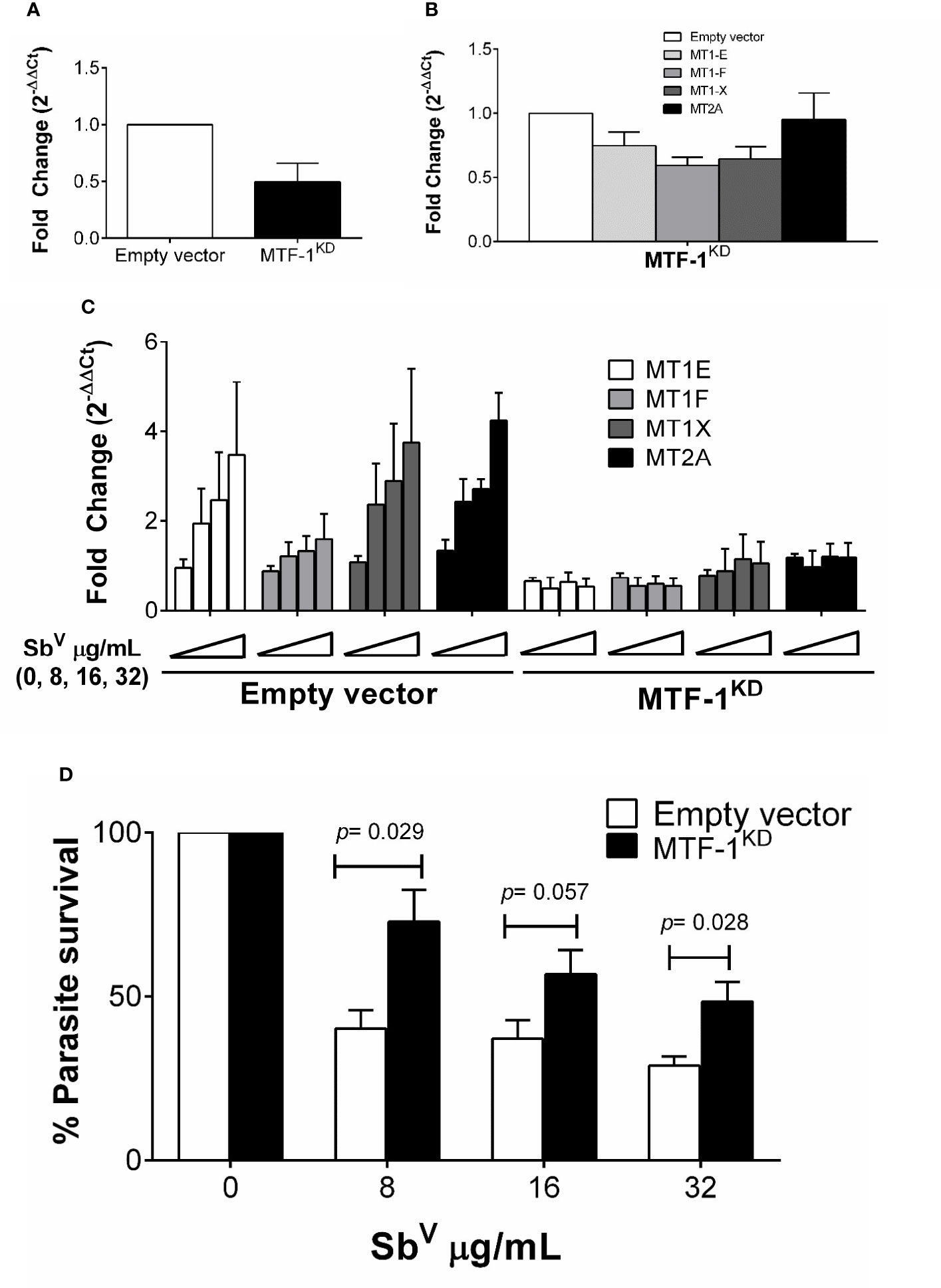
Figure 4 Induction of MTs is abolished by knockdown of MTF-1 favoring intracellular parasite survival. (A) Fold change gene expression of MTF-1 in empty vector control and MTF-1KD THP-1 cells. (B) Fold change gene expression of MTs in unstimulated or (C) infected and SbV (8, 16 and 32 µg/mL) exposed MTF-1KD cells. (D) Percentage of intracellular L.V. panamensis survival after SbV exposure. Gene expression and parasite survival were quantified by RT-qPCR. Each experiment was run in 3 independent replicates. Mann-Whitney test was used for statistical analysis. Data are presented as mean values ± SD.
MTF-1KD and empty vector control THP-1 cells were infected and exposed to SbV (8 - 32 µg/mL), and intracellular parasite survival measured by RT-qPCR. Sb-dependent parasite killing was efficient in empty-vector control cells, where parasite survival was below 50% for all SbV doses tested (Figure 4D). In contrast, Leishmania survival after drug exposure significantly increased in MTF-1KD cells compared to empty vector control cells (Figure 4D), and was above 50% for all evaluated doses and up to 75% in the 8 µg/mL SbV dose. These data suggest that MTF-1KD favors intracellular survival of Leishmania by impairing MTs gene expression.
Discussion
Metallothioneins were initially reported in the early ‘70s and characterized as cadmium binding proteins with a role in metal detoxification (Klaassen et al., 1999). Subsequent studies demonstrated that these proteins could also bind other metals including Sb (Nielson et al., 1985), leading to protection against metal-induced toxicity. Pentavalent antimonials continue to be first line treatment for leishmaniasis in many endemic countries (WHO, 2010). Despite more than 100 years of use, the mechanisms of action and of exposure of the intracellular parasite to the active drug (SbIII), remain poorly understood. Recently, the role of host cells in antimony metabolism and detoxification has gained increased attention due to their potential participation in treatment outcome, and as potential targets for optimization of drug exposure (Gómez et al., 2014; Barrera et al., 2017; Téllez et al., 2017). Here, we provide evidence for the participation of macrophage metallothioneins in the Sb-mediated killing of intracellular Leishmania.
Studies in MT1/MT2-null mice have demonstrated increased toxicity of cisplatinum, Cd, Hg, Cu, Zn and As -a metalloid closely related to Sb- (Masters et al., 1994; Satoh et al., 1997; Liu et al., 2000; Park et al., 2001; Eddins et al., 2008). Concurring with these findings, tolerance to metal-induced hepato- and nephrotoxicity has been demonstrated in transgenic mice overexpressing MTs (Iszard et al., 1995; Liu et al., 1995; Klaassen et al., 1999). Although the precise mechanism by which MTs facilitate Sb-dependent killing of Leishmania remains to be determined, the metal scavenging function of MTs could promote Sb accumulation within infected macrophages. Interestingly, it has been shown that MTs translocate to lysosomes (Moffatt and Denizeau, 1997; Klaassen et al., 1999; Sabolić et al., 2010), suggesting that MT-Sb complexes could be found within lysosomes. Leishmania resides in phagolysosomal compartments within host cells. Therefore, as a consequence of the biological process of phagosome-to-phagolysosome maturation, fusion of MT-Sb containing lysosomes with Leishmania-containing phagosomes could result in enhanced exposure of the intracellular parasite to the drug.
Pentavalent antimonials are pro-drugs which need to be reduced to the trivalent active form to exert their antileishmanial activity (SbV→SbIII). MTs have an important function in the cellular redox balance due to their high thiol content (Nielson et al., 1985; Thornalley and Vašák, 1985; Hamer, 1986; Andrews, 2000; Park et al., 2001; Vašák and Meloni, 2011). Under stress conditions, the strong up-regulation of MTs gene expression results in a redox capacity that can surpass that of GSH (Durnam and Palmiter, 1981; Hamer, 1986; Namdarghanbari et al., 2011). High GSH content has been shown to promote SbV to SbIII reduction in mammalian cells as well as in Leishmania (Miekeley et al., 2002; Dos Santos Ferreira et al., 2003). Thus, MTs could participate in the reduction SbV to SbIII favoring parasite elimination.
MTF-1 is the main transcription factor involved in MTs expression during metal stress responses, and partially during oxidative stress exposure/response (Dalton et al., 1999; Andrews, 2000; Pearce et al., 2000; St. Croix et al., 2002; Stitt et al., 2006). Our results and those of others demonstrate that MTF-1 knockout/knockdown efficiently abolishes cellular expression of MTs under metal and oxidative stress (Heuchel et al., 1994; Andrews, 2000; St. Croix et al., 2002). Although our data provide evidence that repression of MTs expression via MTF-1 gene knockdown promotes intracellular survival of Leishmania after exposure to Sb, we cannot rule out the intervention of other MTF-1 mediated mechanisms in the enhanced parasite survival, such as those involved in Zn transport (Lichtlen et al., 2001; Laity and Andrews, 2007; Troadec et al., 2010; Lichten et al., 2011; Kim et al., 2014). However, evaluation the effect of MTF-1 KD in the expression of slc30a1 (gene coding for the zinc transporter ZnT1) known to be modulated by MTF-1, did not result in significant expression differences in control and MTF-1KD cells when infected and exposed to Sb. We hypothesize that the specific effect observed over MT genes responds to a xenobiotic metal-specific response, which is supported by four MTF-1 binding sites in MT promoter regions, making this transcription factor the primary transcriptional regulator of MT genes. As was the case of ZnT1, and likely other genes where MTF-1 has some transcriptional participation, other transcription factors could be compensating for their expression in absence of MTF-1. However this remains to be experimentally demonstrated.
Our results support a dual role of MTs in toxic as well as therapeutic metal binding, highlighting the potential to harness host cell redox and metal detoxification systems to enhance drug bioavailability and exposure targeted to intracellular pathogens. These findings enlighten interesting drug-related homeostatic processes occurring during treatment of intracellular microbe infections, whereby the same mechanism that promotes host protection to drug-induced toxicity, in this case against Sb-induced stress, can enhance the antimicrobial activity of the drug.
Data availability statement
The original contributions presented in the study are publicly available. This data can be found here: https://www.ncbi.nlm.nih.gov/bioproject/?term=PRJNA633893.
Ethics statement
The studies involving humans were approved by Comité Institucional de Ética de Investigación en Humanos - CIEIH CIDEIM. The studies were conducted in accordance with the local legislation and institutional requirements. The participants provided their written informed consent to participate in this study.
Author contributions
All authors contributed equally to the design, discussion, review of results and approval of the final version of the manuscript. DV developed the entire experimental component. AB and NES performed RNA-seq and supported bioinformatics analysis. DG support the design and implementation of RNA interference assays using shRNA. All authors contributed to the article and approved the submitted version.
Funding
This work was supported in part by US National Institutes of Health (NIH) award numbers R01AI104823 and U19AI129910 (https://www.niaid.nih.gov/), and Wellcome Trust award 107595/Z/15/Z to MG. Ministerio de Ciencia, Tecnología e Innovación – Minciencias (code no. 222984368586). DV was supported by COLCIENCIAS DSc student award 647.
Acknowledgments
We gratefully acknowledge the patients and volunteers who participated in this study and the members of the Clinical Unit of CIDEIM in Cali and Tumaco for recruitment of participants and follow-up. This work was conducted in partial fulfillment of the requirements for the DSc degree in Biomedical Sciences of Universidad del Valle to DV.
Conflict of interest
The authors declare that the research was conducted in the absence of any commercial or financial relationships that could be construed as a potential conflict of interest.
Publisher’s note
All claims expressed in this article are solely those of the authors and do not necessarily represent those of their affiliated organizations, or those of the publisher, the editors and the reviewers. Any product that may be evaluated in this article, or claim that may be made by its manufacturer, is not guaranteed or endorsed by the publisher.
Supplementary material
The Supplementary Material for this article can be found online at: https://www.frontiersin.org/articles/10.3389/fpara.2023.1242727/full#supplementary-material
References
Alvar J., Vélez I. D., Bern C., Herrero M., Desjeux P., Cano. J., et al. (2012). Leishmaniasis worldwide and global estimates of its incidence. PloS One 7 (5), e35671. doi: 10.1371/journal.pone.0035671
Anders S., Pyl P. T., Huber W. (2015). HTSeq-A Python framework to work with high-throughput sequencing data. Bioinformatics 31, 166–169. doi: 10.1093/bioinformatics/btu638
Andrews G. K. (2000). Regulation of metallothionein gene expression by oxidative stress and metal ions. Biochem. Pharmacol. 59, 95–104. doi: 10.1016/S0006-2952(99)00301-9
Anon Babraham Bioinformatics - FastQC A Quality Control tool for High Throughput Sequence Data. Available at: https://www.bioinformatics.babraham.ac.uk/projects/fastqc/ 2020.
Arevalo J., Ramirez L., Adaui V., Zimic M., Tulliano G., Miranda-Verástegui C., et al. (2007). Influence of leishmania (Viannia) species on the response to antimonial treatment in patients with american tegumentary leishmaniasis. J. Infect. Dis. 195, 1846–1851. doi: 10.1086/518041
Aslett M., Aurrecoechea C., Berriman M., Brestelli J., Brunk B. P., Carrington M., et al. (2010). TriTrypDB: a functional genomic resource for the Trypanosomatidae. Nucleic Acids Res. 38, D457–D462. doi: 10.1093/nar/gkp851
Barrera M. C., Rojas L. J., Weiss A., Fernandez O., McMahon-Pratt D., Saravia N. G., et al. (2017). Profiling gene expression of antimony response genes in Leishmania (Viannia) panamensis and infected macrophages and its relationship with drug susceptibility. Acta Trop. 176, 355–363. doi: 10.1016/j.actatropica.2017.08.017
Bolger A. M., Lohse M., Usadel B. (2014). Trimmomatic: a flexible trimmer for Illumina sequence data. Bioinformatics 30, 2114–2120. doi: 10.1093/bioinformatics/btu170
Coelho D. R., Miranda E. S., Saint’Pierre T. D., Paumgartten F. J. R. (2014). Tissue distribution of residual antimony in rats treated with multiple doses of meglumine antimoniate. Mem Inst Oswaldo Cruz 109, 420–427. doi: 10.1590/0074-0276140030
Cousins R. J. (1994). Metal elements and gene expression. Annu. Rev. Nutr. 14, 449–469. doi: 10.1146/annurev.nu.14.070194.002313
Croft S. L., Sundar S., Fairlamb A. H. (2006). Drug resistance in leishmaniasis. Clin. Microbiol. Rev. 19, 111–126. doi: 10.1128/CMR.19.1.111-126.2006
Dalton T. P., Shertzer H. G., Puga A. (1999). Regulation of gene expression by reactive oxygen. Annu. Rev. Pharmacol. Toxicol. 39, 67–101. doi: 10.1146/annurev.pharmtox.39.1.67?url_ver=Z39.88-2003&rfr_dat=cr_pub=pubmed&rfr_id=ori:rid:crossref.org&journalCode=pharmtox
Dohmen L. C. T., Navas A., Vargas D. A., Gregory D. J, Kip A., Dorlo T. P. C., et al. (2016). Functional validation of ABCA3 as a miltefosine transporter in human macrophages: Impact on intracellular survival of Leishmania (viannia) panamensis. J. Biol. Chem. 291, 9638–9647. doi: 10.1074/jbc.M115.688168
Dos Santos Ferreira C., Silveira Martins P., Demicheli C., Brochu C., Ouellette M., Frézard F. (2003). Thiol-induced reduction of antimony(V) into antimony(III): A comparative study with trypanothione, cysteinyl-glycine, cysteine and glutathione. BioMetals 16, 441–446. doi: 10.1023/A:1022823605068
Durnam D. M., Palmiter R. D. (1981). Transcriptional regulation of the mouse metallothionein-I gene by heavy metals. J. Biol. Chem. 256, 5712–5716. doi: 10.1016/S0021-9258(19)69264-1
Eddins D., Petro A., Pollard N., Freedman J. H., Levin E. D. (2008). Mercury-induced cognitive impairment in metallothionein-1/2 null mice. Neurotoxicol Teratol 30, 88–95. doi: 10.1016/j.ntt.2007.12.005
Fernandes M. C., Dillon L. A. L., Belew A. T., Bravo H. C., Mosser D. M., El-Sayed N. M. (2016). Dual transcriptome profiling of leishmania -infected human macrophages reveals distinct reprogramming signatures. MBio 7, e00027–e00016. doi: 10.1128/mBio.00027-16
Firdous R., Yasinzai M., Ranja K. (2009). Efficacy of glucantime in the treatment of old world cutaneous leishmaniasis. Int. J. Dermatol. 48, 758–762. doi: 10.1111/j.1365-4632.2009.04072.x
Gómez M. A., Navas A., Márquez R., Rojas L. L., Vargas D. A., Blanco V. M., et al. (2014). Leishmania panamensis infection and antimonial drugs modulate expression of macrophage drug transporters and metabolizing enzymes: impact on intracellular parasite survival. J. Antimicrob. Chemother. 69, 139–149. doi: 10.1093/jac/dkt334
Gregory D. J., Sladek R., Olivier M., Matlashewski G. (2008). Comparison of the Effects of Leishmania major or Leishmania donovani Infection on Macrophage Gene Expression. Infect. Immun. 76, 1186–1192. doi: 10.1128/IAI.01320-07
Hamer D. H. (1986). Metallothionein. Annu. Rev. Biochem. 55, 913–951. doi: 10.1146/annurev.bi.55.070186.004405
Heuchel R., Radtke F., Georgiev O., Stark G., Aguet M., Schaffner W. (1994). The transcription factor MTF-1 is essential for basal and heavy metal-induced metallothionein gene expression. EMBO J. 13, 2870–2875. doi: 10.1002/j.1460-2075.1994.tb06581.x
Iszard M. B., Liu J., Liu Y. P., Dalton T., Andrews G. K., Palmiter R. D., et al. (1995). Characterization of metallothionein-I-transgenic mice. Toxicol. Appl. Pharmacol. 133, 305–312. doi: 10.1006/taap.1995.1155
Kim J. -H., Jeon J., Shin M., Won Y., Lee M., Kwak J. -S., et al. (2014). Regulation of the catabolic cascade in osteoarthritis by the zinc-ZIP8-MTF1 axis. Cell 156, 730–743. doi: 10.1016/j.cell.2014.01.007
Klaassen C. D., Liu J., Choudhuri S. (1999). METALLOTHIONEIN: an intracellular protein to protect against cadmium toxicity. Annu. Rev. Pharmacol. Toxicol. 39, 267–294. doi: 10.1146/annurev.pharmtox.39.1.267
Laity J. H., Andrews G. K. (2007). Understanding the mechanisms of zinc-sensing by metal-response element binding transcription factor-1 (MTF-1). Arch. Biochem. Biophys. 463, 201–210. doi: 10.1016/j.abb.2007.03.019
Leek J. T., Johnson W. E., Parker H. S., Jaffe A. E., Storey J. D. (2012). SVA detailed instruction. Bioinformatics 28, 882–883. doi: 10.1093/bioinformatics/bts034
Lichten L. A., Ryu M.-S., Guo L., Embury J., Cousins R. J. (2011). MTF-1-mediated repression of the zinc transporter zip10 is alleviated by zinc restriction bryk M, ed. PloS One 6, e21526. doi: 10.1371/journal.pone.0021526
Lichtlen P., Wang Y., Belser T., Georgiev O., Certa U., Sack R., et al. (2001). Target gene search for the metal-responsive transcription factor MTF-1. Nucleic Acids Res. 29, 1514–1523. doi: 10.1093/nar/29.7.1514
Lima M. I. S., Arruda V. O., Alves E. V. C., de Azevedo A. P. S., Monteiro S. G., Pereira S. R. F. (2010). Genotoxic effects of the antileishmanial drug glucantime®. Arch. Toxicol. 84, 227–232. doi: 10.1007/s00204-009-0485-0
Liu J., Liu Y., Goyer R. A., Achanzar W., Waalkes M. P. (2000). Metallothionein-I/II null mice are more sensitive than wild-type mice to the hepatotoxic and nephrotoxic effects of chronic oral or injected inorganic arsenicals. Toxicol. Sci. 55, 460–467. doi: 10.1093/toxsci/55.2.460
Liu Y. P., Liu J., Iszard M. B., Andrews G. K., Palmiter R. D., Klaassen C. D. (1995). Transgenic mice that overexpress metallothionein-I are protected from cadmium lethality and hepatotoxicity. Toxicol. Appl. Pharmacol. 135, 222–228. doi: 10.1006/taap.1995.1227
Love M. I., Huber W., Anders S. (2014). Moderated estimation of fold change and dispersion for RNA-seq data with DESeq2. Genome Biol. 15, 550. doi: 10.1186/s13059-014-0550-8
Masters B. A., Kelly E. J., Quaife C. J., Brinster R. L., Palmiter R. D. (1994). Targeted disruption of metallothionein I and II genes increases sensitivity to cadmium. Proc. Natl. Acad. Sci. 91, 584–588. doi: 10.1073/pnas.91.2.584
Miekeley N., Mortari S., Schubach A. (2002). Monitoring of total antimony and its species by ICP-MS and on-line ion chromatography in biological samples from patients treated for leishmaniasis. Anal. Bioanal Chem. 372, 495–502. doi: 10.1007/s00216-001-1213-7
Miga K. H., Newton Y., Jain M., Altemose N., Willard H. F., Kent E. J. (2014). Centromere reference models for human chromosomes X and y satellite arrays. Genome Res. 24, 697–707. doi: 10.1101/gr.159624.113
Moffatt P., Denizeau F. (1997). Metallothionein in physiological and physiopathological processes. Drug Metab. Rev. 29, 261–307. doi: 10.3109/03602539709037585
Monge-Maillo B., López-Vélez R. (2013). Therapeutic options for old world cutaneous leishmaniasis and new world cutaneous and mucocutaneous leishmaniasis. Drugs 73, 1889–1920. doi: 10.1007/s40265-013-0132-1
Mookerjee Basu J., Mookerjee A., Banerjee R., Saha M., Singh S., Naskar K., et al. (2008). Inhibition of ABC transporters abolishes antimony resistance in leishmania infection. Antimicrob. Agents Chemother. 52, 1080–1093. doi: 10.1128/AAC.01196-07
Namdarghanbari M., Wobig W., Krezoski S., Tabatabai N. M., Petering D. H. (2011). Mammalian metallothionein in toxicology, cancer, and cancer chemotherapy. JBIC J. Biol. Inorg Chem. 16, 1087–1101. doi: 10.1007/s00775-011-0823-6
Nielson K. B., Atkin C. L., Winge D. R. (1985). Distinct metal-binding configurations in metallothionein. J. Biol. Chem. 260, 5342–5350. doi: 10.1021/bi00079a028
Oliveira L. F., Schubach A. O., Martins M. M., Passos S. L., Oliveira R. V., Marzochi M. C., et al. (2011). Systematic review of the adverse effects of cutaneous leishmaniasis treatment in the New World. Acta Trop. 118, 87–96. doi: 10.1016/j.actatropica.2011.02.007
Park J. D., Liu Y., Klaassen C. D. (2001). Protective effect of metallothionein against the toxicity of cadmium and other metals. Toxicology 163, 93–100. doi: 10.1016/S0300-483X(01)00375-4
Pearce L. L., Gandley R. E., Han W., Wasserloos K., Stitt M., Kanai A. J., et al. (2000). Role of metallothionein in nitric oxide signaling as revealed by a green fluorescent fusion protein. Proc. Natl. Acad. Sci. U.S.A. 97, 477–482. doi: 10.1073/pnas.97.1.477
Roesijadi G. (2000). Metal transfer as a mechanism for metallothionein-mediated metal detoxification. Cell Mol. Biol. (Noisy-le-grand) 46, 393–405.
Romero G. A. S., de Farias Guerra M. V., Paes M. G., Macedo V d. O. (2001). Comparison of Cutaneous Leishmaniasis Due to Leishmania (Viannia) Braziliensis and L. (V.) guyanensis in Brazil: Clinical Findings and Diagnostic Approach. Clin. Infect. Dis. 32, 1304–1312. doi: 10.1086/319990
Romero I., Téllez J., Suárez Y., Cardona M., Figueroa R., Zelazny A., et al. (2010). Viability and Burden of Leishmania in Extralesional Sites during Human Dermal Leishmaniasis Milon G, ed. PloS Negl. Trop. Dis. 4, e819. doi: 10.1371/journal.pntd.0000819
Sabolić I., Breljak D., Škarica M., Herak-Kramberger C. M. (2010). Role of metallothionein in cadmium traffic and toxicity in kidneys and other mammalian organs. BioMetals 23, 897–926. doi: 10.1007/s10534-010-9351-z
Satoh M., Aoki Y., Tohyama C. (1997). Protective role of metallothionein in renal toxicity of cisplatinum. Cancer Chemother. Pharmacol. 40, 358–362. doi: 10.1007/s002800050670
St. Croix C. M., Wasserloos K. J., Dineley K. E., Reynolds I. J., Levitan E. S., Pitt B. R. (2002). Nitric oxide-induced changes in intracellular zinc homeostasis are mediated by metallothionein/thionein. Am. J. Physiol. - Lung Cell Mol. Physiol. 282, L185–L192. doi: 10.1152/ajplung.00267.2001
Stitt M. S., Wasserloos K. J., Tang X., Liu X., Pitt B. R., St. Croix C. M. (2006). Nitric oxide-induced nuclear translocation of the metal responsive transcription factor, MTF-1 is mediated by zinc release from metallothionein. Vascul Pharmacol. 44, 149–155. doi: 10.1016/j.vph.2005.10.004
Sundar S., Chakravarty J. (2015). An update on pharmacotherapy for leishmaniasis. Expert Opin. Pharmacother. 16, 237–252. doi: 10.1517/14656566.2015.973850
Téllez J., Romero I., Soares M. J., Steindel M., Romanha A. J. (2017). Knockdown of host antioxidant defense genes enhances the effect of glucantime on intracellular leishmania Braziliensis in human macrophages. Antimicrob. Agents Chemother. 61, e02099–e02016. doi: 10.1128/AAC.02099-16
Thornalley P. J., Vašák M. (1985). Possible role for metallothionein in protection against radiation-induced oxidative stress. Kinetics and mechanism of its reaction with superoxide and hydroxyl radicals. Biochim. Biophys. Acta - Protein Struct. Mol. Enzymol. 827, 36–44. doi: 10.1016/0167-4838(85)90098-6
Trapnell C., Roberts A., Goff L., Pertea G., Kim D., Kelley D. R., et al. (2012). Differential gene and transcript expression analysis of RNA-seq experiments with TopHat and Cufflinks. Nat. Protoc. 7, 562–578. doi: 10.1038/nprot.2012.016
Troadec M.-B., Ward D. M., Lo E., Kaplan J., De Domenico I. (2010). Induction of FPN1 transcription by MTF-1 reveals a role for ferroportin in transition metal efflux. Blood 116, 4657–4664. doi: 10.1182/blood-2010-04-278614
Vašák M., Meloni G. (2011). Chemistry and biology of mammalian metallothioneins. J. Biol. Inorg Chem. 16, 1067–1078. doi: 10.1007/s00775-011-0799-2
WHO (2010). Control of the leishmaniases. World health organization, geneva. Tech Rep. Ser. 949, 22–26.
Zhou H., DeLoid G., Browning E., Gregory D. J., Tan F., Bedugnis A. S., et al. (2012). Genome-Wide RNAi Screen in IFN-γ-Treated Human Macrophages Identifies Genes Mediating Resistance to the Intracellular Pathogen Francisella tularensis Neyrolles O, ed. PloS One 7, e31752. doi: 10.1371/journal.pone.0031752
Keywords: metallothioneins, metal transcription factor-1 (MTF-1), leishmania, antimony, host-parasite interaction
Citation: Vargas DA, Gregory DJ, Koren RN, Zilberstein D, Belew AT, El-Sayed NM and Gómez MA (2023) Macrophage metallothioneins participate in the antileishmanial activity of antimonials. Front. Parasitol. 2:1242727. doi: 10.3389/fpara.2023.1242727
Received: 19 June 2023; Accepted: 12 September 2023;
Published: 04 October 2023.
Edited by:
Suzanne McDermott, Seattle Children’s Research Institute, United StatesCopyright © 2023 Vargas, Gregory, Koren, Zilberstein, Belew, El-Sayed and Gómez. This is an open-access article distributed under the terms of the Creative Commons Attribution License (CC BY). The use, distribution or reproduction in other forums is permitted, provided the original author(s) and the copyright owner(s) are credited and that the original publication in this journal is cited, in accordance with accepted academic practice. No use, distribution or reproduction is permitted which does not comply with these terms.
*Correspondence: María Adelaida Gómez, bWdvbWV6QGNpZGVpbS5vcmcuY28=; bWFyaWEuZ29tZXozNUB1LmljZXNpLmVkdS5jbw==