- Department of Biochemistry and Microbiology, University of Victoria, Victoria, BC, Canada
Intestinal helminths have evolved an abundance of immunomodulatory mechanisms to ensure long-lived infections in mammalian hosts. To manipulate mammalian immune responses helminths can directly produce immunomodulatory molecules, but helminth infection can also elicit functional changes in the intestinal microbiome which can impact immune functioning. Here we examined how bile acids (BA)s, a group of host-produced, microbiota-modified immunomodulatory metabolites, were altered in abundance and composition during a murine small intestinal helminth infection. We found that murine helminth infection resulted in consistently reduced concentrations of specific taurine-conjugated primary BAs (T-α-MCA and T-CDCA) in the small intestinal luminal contents of mice. BA transporters facilitate the uptake of BAs from the small intestinal lumen, allowing BAs to engage with nuclear BA receptors, and helminth infected mice showed reduced expression of genes encoding basal BA transporters in the small intestine. Finally, we report that there is reduced signaling through the nuclear BA receptor FXR in both the proximal small intestine and ileum of mice during small intestinal helminth infection. Together, our data reveal disruptions to BA homeostasis and signaling in the small intestine during helminth infection. As BAs are known to impact many aspects of mucosal physiology and immunity, examining the functional consequences of BA disruptions during helminth infection will be an important avenue for future research.
1 Introduction
Soil-transmitted helminths are potent manipulators of the mammalian host immune system, and do so by dampening inflammation and inducing regulatory immune pathways in order to establish chronic infections within the gastrointestinal tract (Varyani et al., 2017). Identifying the molecules which mediate helminth-driven immunomodulation is of importance for helminth-infected people and livestock animals, but in addition, may have therapeutic applications for immune-driven inflammatory disorders including inflammatory bowel diseases (Ryan et al., 2020). The majority of efforts to date have concentrated on characterizing molecules directly produced by helminths in their excretory-secretory (ES) material, which has been shown to contain protein, small RNA, and metabolite components each with demonstrated immunomodulatory effects (Yeshi et al., 2022).
As well as ES material produced directly by helminths influencing immune functioning, there are profound changes to the structure and functional capabilities of the bacterial microbiota during helminth infection (Peachey et al., 2017; Llinás-Caballero and Caraballo, 2022). Together, this results in the helminth-infected gut harbouring a distinct concoction of potentially immunomodulatory molecules which differs to that present during homeostasis (Brosschot and Reynolds, 2018). Metabolites within this ‘helminth-modified’ intestinal environment have been shown to impact immune cell functions (Brosschot and Reynolds, 2018), although there is limited progress in both identifying the specific types of metabolites altered during intestinal helminth infection and examining the functional consequences of helminth-modified metabolites in vivo (Zaiss et al., 2015; Kennedy et al., 2021; Popple et al., 2021; Shute et al., 2021; Schälter et al., 2022). One group of metabolites that are candidates for contributing to helminth infection-induced immunomodulation are the bile acid (BA)s: a group of molecules deposited in the gut to aid with fat digestion, but which also have increasingly appreciated roles as immune signaling molecules (Chen et al., 2019). BAs can act directly on cell surface and nuclear receptors expressed by mucosal epithelial cells and leukocytes, thereby influencing mucosal immune cell functioning, intestinal motility, and intestinal inflammation (Chen et al., 2019). Two such BA receptors are the nuclear farnesoid X receptor (FXR) and the cell-surface receptor G protein-coupled bile acid receptor 1 (GPBAR1; also known as TGR5), which are expressed in the gut by epithelial cells and various innate immune cells (Chen et al., 2019).
Primary BAs are synthesized by mammals in the liver from cholesterol. Humans produce two primary BAs: cholic acid (CA) and chenodeoxycholic acid (CDCA), whereas mice produce these and an additional three primary BAs: α- and β- muricholic acid (α-/β-MCA) and ursodeoxycholic acid (UDCA) (Li and Dawson, 2019). In both species, primary BAs are conjugated with either glycine or taurine prior to being stored in the gallbladder and subsequently deposited in the duodenum; in mice, taurine conjugation dominates (Li and Dawson, 2019). Conjugation has two main consequences: it allows BAs to form micelles and solubilize dietary lipids, and it reduces the ability of BAs to freely diffuse across intestinal epithelial cell membranes (Dawson and Karpen, 2015). Instead, epithelial transporters are required for the active reuptake of conjugated BAs from the intestinal lumen, which occurs predominately in the ileum (Dawson and Karpen, 2015). Moreover, within the intestinal lumen, conjugated primary BAs can be modified by the bacterial microbiota (to create what are classed as secondary BAs) which has been proposed to reduce their toxicity towards the microbiota and/or to allow for their use by microbial species as an energy source. BAs are first deconjugated by bile salt hydrolases (BSH) encoded by many microbiota species, then they are further metabolized by the microbiota into a plethora of secondary BAs, a small fraction of which are ultimately excreted in feces (Wahlström et al., 2016). Those BAs that are re-absorbed from the intestinal tract recirculate in the blood to the liver, where they can be recycled and ultimately re-deposited in the duodenum as conjugated primary or secondary BAs. This system ensures that under homeostatic conditions the BA pool size is kept relatively constant, with fecal loss being balanced by new liver synthesis.
Here, we show that infection of mice with the small intestinal helminth Heligmosomoides polygyrus disrupts BA homeostasis, with a specific reduction in the concentrations of the taurine-conjugated (T-) primary BAs T-α-MCA and T-CDCA in the lumen of the proximal small intestine. Helminth infection further disrupted expression of BA transporter genes in the proximal small intestine and resulted in reduced signaling through the nuclear receptor FXR in both the proximal small intestine and ileum, thereby impacting a BA signaling pathway with established consequences for mucosal immunity (Chen et al., 2019).
2 Methods
2.1 Mice
All mouse experiments were conducted at the University of Victoria (UVic), approved by the UVic Animal Care Committee, and followed all Canadian Council on Animal Care guidelines (Animal Use Protocol #2021-008). C57BL/6J mice (stock #000664) were purchased from The Jackson Laboratory and either left to acclimatize for a week before being used in experiments, or were subsequently bred in-house and their offspring were used in experiments. Mice from different litters were split evenly between experimental groups, and were 6-11 weeks old at the start of experiments. Mice were housed in groups of 2 - 5 under specific pathogen-free conditions, with ad libitum access to food and water. Enrichment included in all cages included crinkle paper, nestlets, and a plastic hut. To avoid time of euthanasia/sample collection influencing experimental results, upon experimental endpoints, one mouse was euthanized from each experimental group sequentially until all euthanasias were complete. Mice were placed in an anaesthetic chamber and exposed to 5% inhaled isoflurane until they reached surgical plane anaesthesia, and then were euthanized by cervical dislocation.
2.2 Helminth infection
The life cycle of H. polygyrus bakeri was maintained as described previously (Johnston et al., 2015) in C57BL/6J mice. Mice were orally gavaged with 200 third stage H. polygyrus larvae. Naive control mice were orally gavaged with water that larvae had been stored in, which had been passed through a 40 μm cell strainer to remove larvae and visually checked under a microscope to ensure water was larvae-free. All mice were euthanized 14 days post-infection/mock infection. Feces were collected from all mice at the point of euthanasia to verify infection status through counting parasite eggs using a McMaster egg counting chamber. As expected, no eggs were detected in the feces of any naive mice, and the feces of all infected mice contained eggs, thus no data points were excluded due to the unexpected presence or lack of parasite eggs.
2.3 Sample collection
Blood was collected via cardiac puncture, allowed to coagulate on ice, and spun to obtain sera which was stored at -80°C prior to metabolomic analysis. The proximal small intestine was defined as the first 6 cm following the pyloric sphincter (duodenum and proximal jejunum). The most distal 2 cm of the small intestine was collected for ileal sampling. The contents of the proximal small intestine and ileum were each squeezed out using tweezers. Proximal small intestine contents were flash-frozen with liquid nitrogen and stored at -80°C prior to metabolomic analysis. Proximal small intestinal and ileal tissues were collected into TRIzol™ (Thermo Fisher Scientific, Mississauga, Canada) and stored at -80°C prior to RNA extraction. Entire livers were collected into TRIzol™ and stored at -80°C prior to RNA extraction.
2.4 Bile acid quantification
Quantification of BAs was performed at the UVic Genome BC Proteomics Centre, Victoria, Canada, by liquid chromatography (LC)-multiple-reaction monitoring (MRM)/mass spectrometry (MS) using an Agilent 1290 ultra-high-performance liquid chromatography system coupled to a 6495B Agilent QQQ mass spectrometer. The MS instrument was operated in MRM mode with negative-ion detection. A Waters BEH C18 LC column (2.1*150 mm, 1.7 μm) was used, and the mobile phase was 0.01% formic acid in water and 0.01% formic acid in acetonitrile for binary-solvent gradient elution. Specific LC and MS operation parameters were as described previously (Han et al., 2015). Full details can be found in the Supplementary Material.
2.5 Gene expression analysis
RNA isolations were performed according to the TRIzol™ manufacturer’s instructions. A nanodrop was used to determine RNA concentrations and purity via 260/280 ratios. Agarose gel electrophoresis was then used to confirm RNA integrity through the presence of intact 28S and 18S rRNA bands. RNA concentrations were normalized prior to genomic DNA removal and conversion to cDNA, which were performed with the iScript™ gDNA Clear cDNA Synthesis Kit (Bio-Rad, Mississauga, Canada) according to manufacturer’s instructions. Quantitative PCR was performed using the Applied Biosystems™ PowerUp™ SYBR™ Green Master Mix (Thermo Fisher Scientific, Mississauga, Canada). Each sample was run in triplicate on a Roche LightCycler® 96 Instrument. Relative expression levels of target genes were determined using the ΔΔCq method, with Gapdh used as a housekeeping gene because its expression was not affected by H. polygyrus infection in any tissue examined (data not shown). Data points were excluded from further analyses if extracted RNA quality was poor.
Primer details and cycling conditions can be found in the Supplementary Material.
2.6 Statistical analyses
Metabolomics data were first analyzed en masse via a multiple t-test comparison between naive and infected mice for each individual BA species, as well as for broad groups of BAs and total BA levels (consistent standard deviations between samples not assumed, 5% false discovery rate (FDR), two-stage step-up method of Benjamini, Krieger, and Yekutieli). Where discoveries were made (q value ≤ 0.05), individual or groups of BAs were further statistically analyzed as indicated in Figure Legends. For moisture content and gene expression experiments, data were first analyzed for normality using a D’Agostino-Pearson omnibus normality test and a Shapiro-Wilk normality test, then unpaired t-tests or Mann-Whitney tests were used as appropriate and are indicated in Figure Legends. GraphPad Prism version 8 was used for statistical analyses.
3 Results and discussion
3.1 Small intestinal helminth infection disrupts the bile acid pool
To our knowledge, how intestinal helminth infection impacts BA homeostasis has not been previously investigated. We used targeted UPLC-MRM/MS to determine the concentration of ~80 individual BAs in the luminal contents of the murine proximal small intestine during infection with H. polygyrus, a strictly enteric helminth which establishes a chronic infection in the proximal small intestine. The size and composition of the BA pool is profoundly influenced by biological sex (Phelps et al., 2019), so we separated female and male mice for all analyses. We calculated BA concentrations normalized to wet sample weights (rather than dry sample weights), as this is more reflective of physiological BA concentrations in the intestinal lumen. When assessing the total BA pool size and the concentrations of the major groups of BAs, we did not find any consistent differences between naive and H. polygyrus-infected mice in either sex (Figure 1A; Supplementary Figure 1A). The most abundant major group of luminal BAs in the proximal small intestine was, as expected (Monte et al., 2009; Li and Dawson, 2019), the primary taurine-conjugated BAs (Figure 1A). Within this group, we found that the concentrations of T-α-MCA and T-CDCA were decreased during helminth infection in both female and male mice (Figure 1B). This was a consistent finding between repeat experiments using different batches of infectious H. polygyrus larvae (Figure 1C) which each resulted in robust infections as measured by parasite egg output in mouse feces (Supplementary Figure 1B). Concentrations of the secondary taurine-conjugated BAs taurodeoxycholic acid (T-DCA) and taurolithocholic acid (T-LCA) were also consistently decreased during H. polygyrus-infection (Figure 1D), as were concentrations of the less abundant glycine conjugates glycochenodeoxycholic acid (G-CDCA) and glycohyodeoxycholic acid (G-HDCA) in male and female mice (Supplementary Figures 1C, D). Aside from those BA indicated above, we did not find consistent statistical differences in the concentrations of any other BA species during helminth infection when we ran multiple t-test analyses for each of the ~80 detectable BA species, however there were fluctuations in the concentrations of other individual BAs in individual experiments during helminth infection (Supplementary Data Sheet 1).
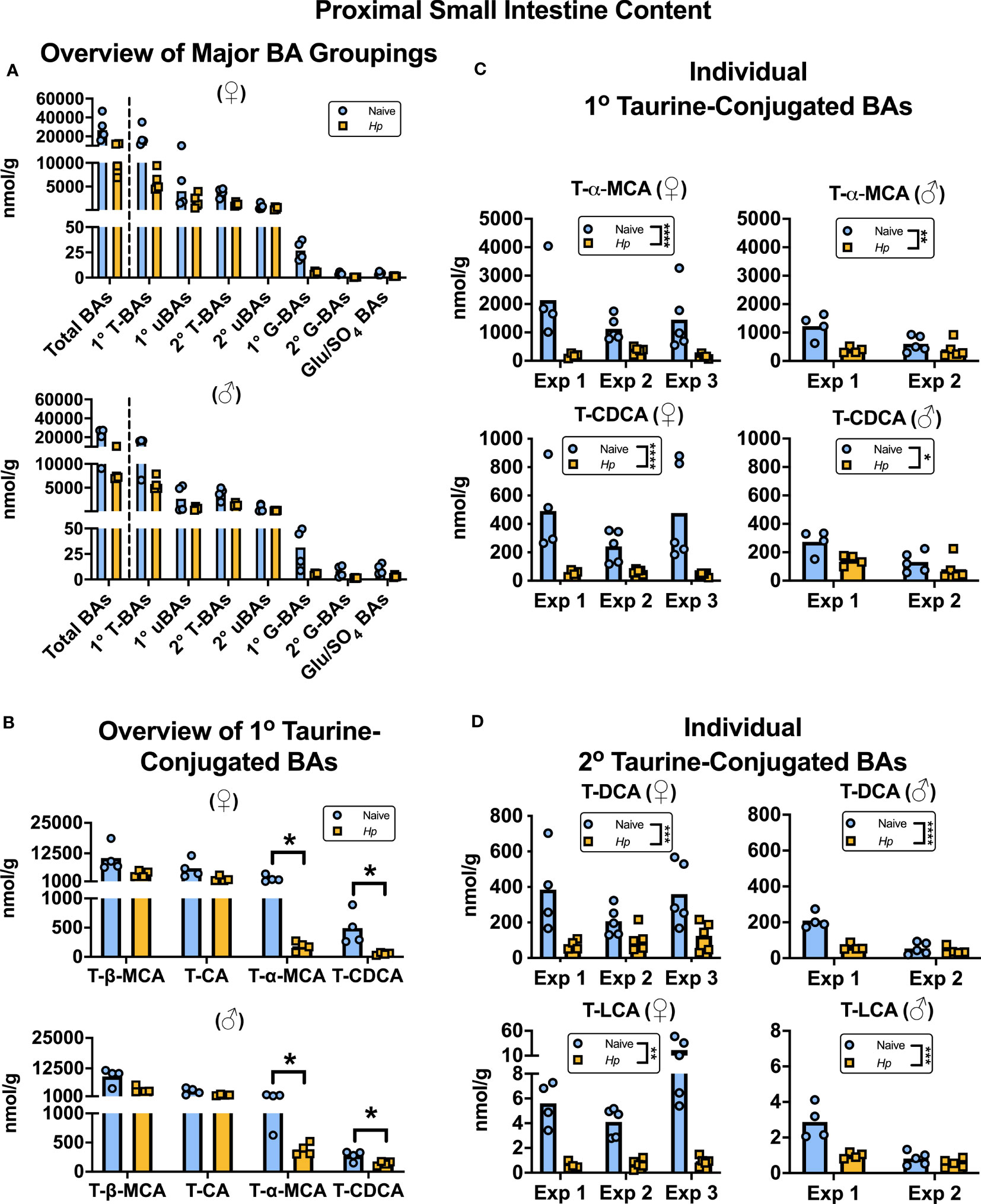
Figure 1 Heligmosomoides polygyrus (Hp) infection disrupts the bile acid (BA) composition of the proximal small intestinal lumen including a reduction in taurine-conjugated (Τ-)α-MCA and T-CDCA levels. Female (♀) and male (♂) mice were left naive or infected with Hp, then BA concentrations were determined and normalized to wet sample weights. (A) Total BA concentrations are presented (left side of the dotted line), as well as concentrations of the major groupings of BAs (right side of the dotted line; primary [1°] T-BAs, 1° unconjugated [u] BAs, secondary [2°] T-BAs, 2° uBAs, 1° glycine-conjugated [G-] BAs, 2° G-BAs, and gluconated or sulfated [Glu/SO4] BAs). Data shown are from one experiment with an n=4 in each experimental group and data from repeat experiments are presented in Supplemental Figure 1. (B) Concentrations of individual 1° T-BAs. Data shown are from one experiment with an n=4 in each experimental group and are representative of the results from three (♀ mice) or two (♂ mice) independent experiments. Although UDCA is a 1° BA in mice, in our assay T-UDCA was indistinguishable from the secondary 2° T-BA T-hyodeoxycholic acid and thus is not included in this graph. A multiple t-test was performed to assess how helminth infection impacted concentrations of all ~80 detectable BA species in our assay, and q values for those BAs presented in this graph are indicated. *q ≤ 0.05. (C, D) Concentrations of individual 1° T-BAs (C) and individual 2° T-BAs (D) across independent experiment (Exp)s, each with an n=4-5 in each experimental group. Two-way ANOVA tests were used to assess how Exp and infection status contributed to variation within data sets and the results of the impact of infection status are presented on graphs. *p ≤ 0.05, **p ≤ 0.01, ***p ≤ 0.001, ****p ≤ 0.0001. Each data point represents results from an individual mouse.
There are likely many contributing factors driving helminth infection-induced disruption to BA concentrations in the proximal small intestine. Firstly, the moisture content of the small intestinal luminal content was increased during H. polygyrus infection (Supplementary Figure 2A), which was expected due to the “weep and sweep” response during type 2-inducing infections (Varyani et al., 2017). For this reason, we also calculated BA concentrations relative to dry sample weights and found that when we did so, only T-α-MCA, T-CDCA, and T-LCA concentrations were still significantly decreased during helminth infection, and only in female mice (Supplementary Figures 2B, C). This suggests that the disruption to BA concentrations during helminth infection is partially, but not completely, due to the influx of fluids that occurs in response to intestinal helminth infections.
We next considered the possibility that host synthesis of BAs was disrupted during helminth infection. To test this, we measured the expression of six murine genes involved in the synthesis and conjugation of BAs (Cyp7a1, Cyp8b1, Akr1d1, Cyp27a1, Cyp2c70 and Baat) in the liver (Supplementary Figure 3). Of these genes, we only detected a modest downregulation of Akr1d1 expression during helminth infection, and only in female mice (Supplementary Figures 3B, C). This may be a slight contributing factor to the disruption of BA concentrations seen in female mice during H. polygyrus infection, as Akr1d1 knockout mice have a marked reduction in total acid levels including CDCA- and CA- derived BA species (Gathercole et al., 2022).
The bacterial microbiota is known to be disrupted by infection with intestinal helminths (including murine infection with H. polygyrus) (Reynolds et al., 2015; Rapin and Harris, 2018), and it is likely that microbiota perturbation also contributes to disruption of BA homeostasis during helminth infection. In the absence of a bacterial microbiota, the BA pool is dominated by conjugated primary BAs, demonstrating the importance of the bacterial microbiota for mediating the deconjugation and further modifications of BAs (Wahlström et al., 2016). Indeed, expression of BSH enzymes which catalyze the deconjugation of BAs is widespread amongst members of the small intestinal bacterial microbiota, with many species encoding multiple distinct BSH enzymes, each with varying degrees of specificity for different conjugated BA substrates (Foley et al., 2019). It is plausible that shifts in the small intestinal bacterial microbiota composition during H. polygyrus infection result in increased activity of BSH variants with specificity for T-α-MCA and T-CDCA, explaining the decreased concentrations of T-α-MCA and T-CDCA we observe in the proximal small intestine during helminth infection. Although we did not see a corresponding increase in the concentrations of the unconjugated forms of these BAs in the proximal small intestine contents (α-MCA and CDCA; Supplementary Data Sheet 1), it is possible that these metabolites and/or their secondary bile acid derivatives are rapidly removed from this sampling site, perhaps through transport, simple diffusion, or further metabolism by the microbiota. Bacterial microbiota fluctuations between experiments may also contribute to the experiment-to-experiment variability in the influence that H. polygyrus has on the overall BA pool size and major groupings that we report (Supplemental Figure 1A), as it was recently demonstrated that bacterial microbiota composition shifts during H. polygyrus infection vary based on both the starting microbiota composition of mice and on the batch of H. polygyrus larvae used for infections (Rapin et al., 2020). It remains to be explored whether H. polygyrus, or other intestinal helminth species, have the capacity to directly deconjugate BAs, and if so, if helminth-encoded BSH variants demonstrate substrate specificity for certain types of conjugated BAs.
3.2 Reduced expression of small intestinal bile acid transporters during helminth infection
With the knowledge that H. polygyrus infection resulted in reduced concentrations of T-α-MCA and T-CDCA in the lumen of the proximal small intestine, we next examined whether the capacity for BAs to be reabsorbed from the intestinal lumen was impacted by helminth infection. While unconjugated BAs are able to passively diffuse across the intestinal lumen to enter portal circulation, the major mechanism for the uptake of taurine-conjugated BAs is via active transport into enterocytes through the apical sodium-dependent BA transporter (ASBT), after which BAs are shuttled to the basolateral membrane and effluxed to the blood through the transporter complex organic solute transporter (OST)α-OSTβ (Dawson et al., 2009). We assessed expression levels of the genes encoding these BA transporters in the proximal small intestine, and while we found no significant differences in the expression levels of Slc10a2 (encodes ASBT) or Slc51a (encodes OSTα) during helminth infection, we report a significant, albeit modest, downregulation of one essential component of the basolateral transporter, Slc51b (encodes OSTβ), during helminth infection in both female and male mice (Figures 2A, B). While downregulation of Slc51a (encodes OSTα) during helminth infection did not reach statistical significance in the proximal small intestine, Slc51a was modestly but significantly downregulated during helminth infection in the ileum of female mice only (Figures 2C, D). Since expression of both OST-α and OST-β can be directly regulated by BAs signaling through BA receptors (Lu et al., 2022), reduced expression of these transporters may be a result of the disrupted luminal BA concentrations we detected during helminth infection, in an attempt to re-establish BA homeostasis.
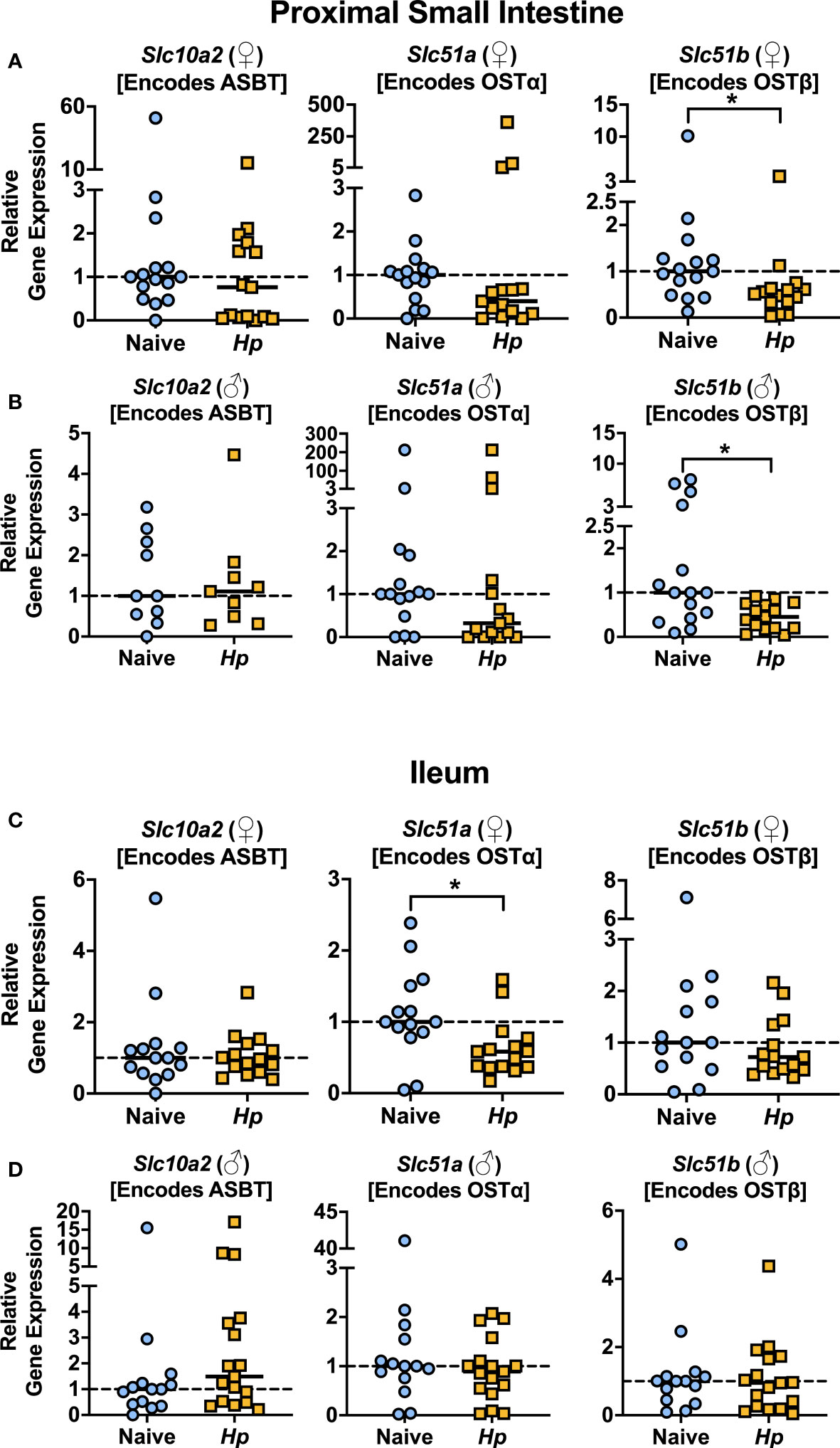
Figure 2 Basolateral bile acid transporter gene expression in the proximal small intestine is reduced during Heligmosomoides polygyrus (Hp) infection. (A, B) Expression levels of the indicated genes for female (♀; A) and male (♂; B) mice in the proximal small intestine. (C, D) Expression levels of the indicated genes for female (♀; C) and male (♂; D) mice in the ileum. Data shown for Slc10a2 from ♂ mice in the proximal small intestine are pooled from two independent experiments each with an n=4-5 in each experimental group (combined n=10 naive mice, n=9 infected mice); all other data shown are pooled from three independent experiments each with an n=4-6 in each experimental group (combined n=15 naive female mice, n=15 infected female mice, n=15 naive male mice, n=15 infected male mice). Statistical comparisons were made using unpaired t-tests if data were parametric, or Mann-Whitney tests if data were non-parametric. *p ≤ 0.05. Each data point represents results from an individual mouse.
We next looked in the serum to determine whether the impaired expression of OST-α-OST-β during helminth infection altered concentrations of circulating BA levels during infection but found no consistent disruptions (Supplementary Figure 4A), including no differences in circulating levels of any of the individual taurine-conjugated primary BAs in circulation during helminth infection (Supplementary Data Sheet 1). It is important to note that the expression of BA transporters is much lower in the proximal small intestine than in the ileum (Dawson et al., 2009) (Supplemental Figure 4B), and the ileum is known to be the main site of BA intestinal uptake into circulation (Dawson et al., 2009). That we only detected modest differences in BA transporter gene expression during H. polygyrus infection, especially in the ileum, might explain why we do not detect major differences in BA concentrations in circulation during helminth infection. Nevertheless, that we detected reduced BA transporter gene expression during helminth infection was suggestive of disrupted BA signaling, and so we next examined how signaling through BA receptors in the small intestine was impacted during helminth infection.
3.3 Helminth infection reduces signaling through FXR in the small intestine
The most well-characterized BA signaling receptors to date are the nuclear FXR and the cell-surface GPBAR1 (Ticho et al., 2019). FXR and GPBAR1 are both highly expressed by many intestinal cells, such as intestinal epithelial and endothelial cells, as well as by innate immune cells including dendritic cells, monocytes/macrophages, NK and NKT cells, and GPBAR1 specifically is also expressed by intestinal muscle and neuronal cells (Biagioli et al., 2021). We set out to determine whether intestinal helminth infection impacted the expression of genes encoding these receptors, and/or if signaling through these receptors was impacted by helminth infection in female and male mice. We did not detect any significant differences in expression of the genes encoding the receptors FXR (Nr1h4) or GPBAR1 (Gpbar1) themselves during helminth infection (Figure 3). T-CDCA and T-α-MCA, the two conjugated BAs that we found to be consistently and significantly decreased in concentration during H. polygyrus infection, are potent agonists and antagonists of FXR, respectively (Xiang et al., 2021). To determine the overall impact of helminth infection on signaling through FXR, we examined expression of genes known to be transcribed as a result of FXR activation: Fgf15 (encodes FGF15) and Nr0b2 (encodes SHP) (Chen et al., 2019). Detection of Fgf15 in the proximal small intestine was inconsistent due to low gene expression (data not shown), which is consistent with previous reports (Inagaki et al., 2005). However, we were able to quantify levels of Fgf15 expression in the ileum and found reduced expression levels during H. polygyrus infection in both female and male mice (Figure 4A). Further, expression levels of Nr0b2 were significantly reduced in the proximal small intestine of both female and male mice during helminth infection (Figure 4B). In the ileum expression of Nr0b2 in female mice was also reduced during helminth infection, with a trend for reduced Nr0b2 gene expression during helminth infection in male mice that did not reach statistical significance (Figure 4C). This decreased expression of key genes known to be transcribed downstream of FXR activation strongly indicates that FXR activation is downregulated in both the proximal small intestine (the site of H. polygyrus colonization) and in the ileum (the major site for BA transport across the intestinal epithelium, signaling, and interaction with immune cells). Reduced FXR activation during helminth infection may be a consequence of the reduced luminal concentrations of the FXR agonist T-CDCA in the small intestine we report during helminth infection, as T-CDCA is a potent FXR activator (Xiang et al., 2021).
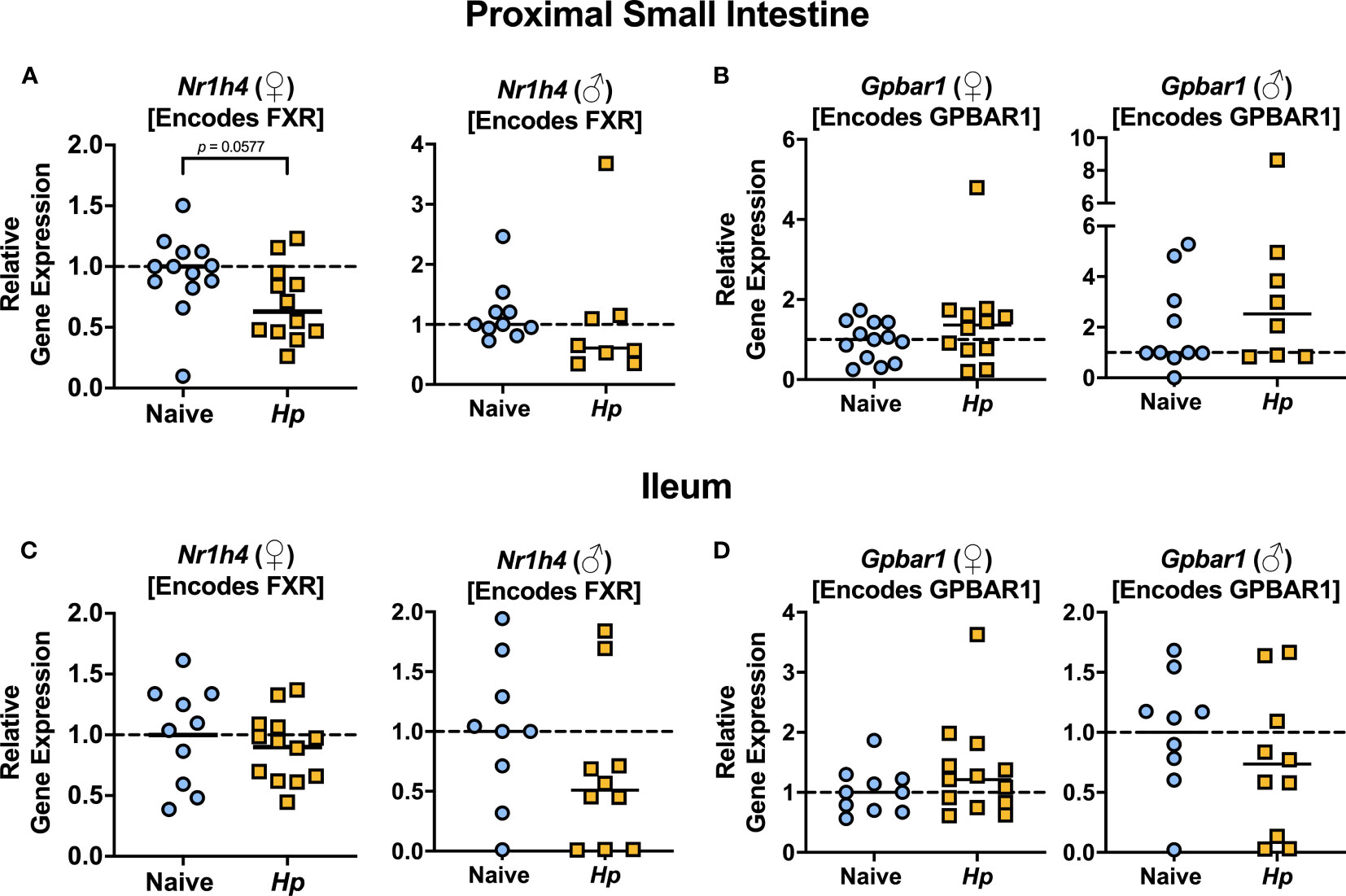
Figure 3 Heligmosomoides polygyrus (Hp) infection does not significantly alter expression of genes encoding BA receptors FXR or GPBAR1 in the small intestine. (A, B). Gene expression levels of Nr1h4 (A) and Gpbar1 (B) in the proximal small intestine. (C, D). Gene expression levels of Nr1h4 (C) and Gpbar1 (D) in the ileum. Data shown for female (♀) mice are pooled from three independent experiments each with an n=2-5 in each experimental group (combined n=13 naive mice, n=12 infected mice for proximal small intestine samples, and n=10 naive mice, n=13 infected mice for ileal samples) and data shown for male (♂) mice are pooled from two independent experiments each with an n=4-6 in each experimental group (combined n=10 for naive mice, n=8 for infected mice for proximal small intestine samples, and n=9 for naïve mice, n=10 for infected mice for ileal samples). Statistical comparisons were made using unpaired t-tests if data were parametric, or Mann-Whitney tests if data were non-parametric. P value was stated if under 0.1. Each data point represents results from an individual mouse.
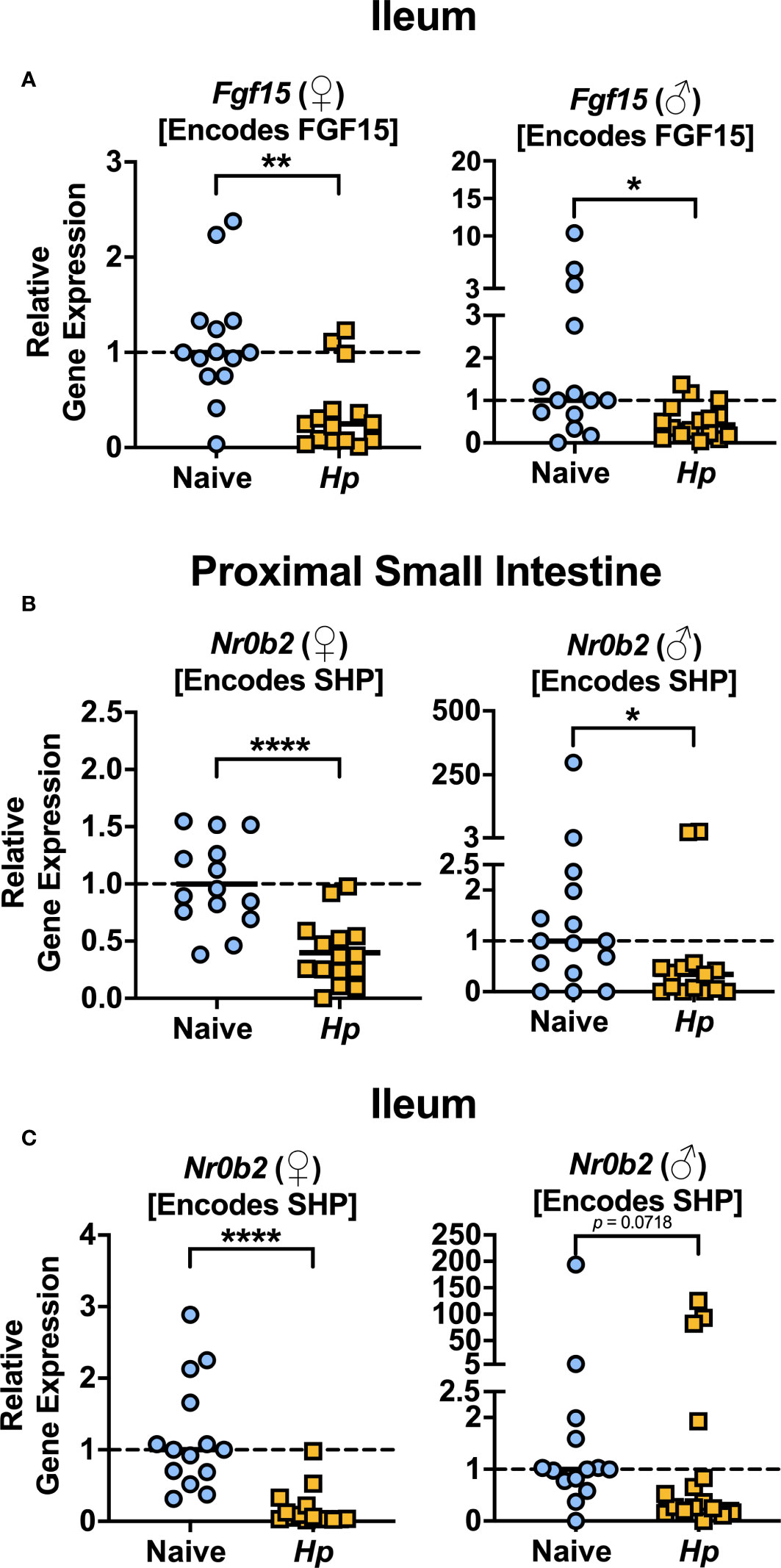
Figure 4 Heligmosomoides polygyrus (Hp) infection reduces signaling through FXR in the small intestine. (A) Gene expression levels of Fgf15 in the ileum were determined by quantitative PCR. (B, C) Gene expression levels of Nr0b2 in the proximal small intestine (B) and ileum (C) were determined quantitative PCR. Data shown are pooled from three independent experiments each with an n=3-6 in each experimental group (combined n=14 naive female (♀), n=15 infected female, n=15 naive male (♂), n=14 infected male proximal small intestine samples and n=14 naive female, n=15 infected female, n=14 naive male and n=16 infected male ileal samples. Statistical comparisons were made using unpaired t-tests if data were parametric, or Mann-Whitney tests if data were non-parametric. *p ≤ 0.05, **p ≤ 0.01, ****p ≤ 0.0001, and p value was stated if under 0.1. Each data point represents results from an individual mouse.
The consequences of an altered intestinal bile acid composition and reduced FXR activation in the small intestine during helminth infection will be important areas for future investigation. For example: could reduced FXR activation impact the chronicity of helminth infection? While the detection of bile has been implicated as a habitat selection cue for helminths such as H. polygyrus along the intestinal tract (Sukhdeo and Croll, 1981), and indeed microbiota depletion (which impacts the BA pool) results in altered H. polygyrus distribution along the intestinal tract (Moyat et al., 2022) to our knowledge, whether modified signaling through BA receptors impacts the host immune response during helminth has not yet been explored. It has been reported that FXR activation can inhibit intestinal IL-1β, IL-6, and TNF-α, and GPBAR1 activation can attenuate IL-12 and TNF-α production by dendritic cells (Fiorucci et al., 2021). There is evidence that some of these cytokines can reduce immunity to intestinal helminth infection: for example, IL-6 and IL-12 have each been shown to limit Th2 responses associated with H. polygyrus expulsion (Smith and Maizels, 2014; Coomes et al., 2015), and further, dietary delivery of BAs which activate FXR result in elevated intestinal type 2 responses which accelerated expulsion of Nippostrongylus brasiliensis (Arifuzzaman et al., 2022). Although we did not directly assess levels of GPBAR1 activation during helminth infection, we did find that levels of taurolithocholic acid (T-LCA), a potent agonist of GPBAR1 (Maruyama et al., 2002), were significantly reduced in the content of the proximal small intestine during H. polygyrus infection, which could in turn lead to reduced GPBAR1 activation and an increase in IL-12 production. It remains to be determined whether the reduced FXR signaling we observe in the small intestine during H. polygyrus infection is promoting helminth chronicity, perhaps through elevated IL-6/IL-12 levels or other mechanisms limiting the efficacy of type 2 responses.
The intestinal epithelium is comprised of diverse cell types including enterocytes, goblet cells, tuft cells, Paneth cells, M cells, and enteroendocrine cells. Several of these cell types have been shown to respond to and/or contribute to the host response against helminth infection (Baska and Norbury, 2022), and it will be important for future work to consider the impact of disrupted bile acid concentrations on the functioning of each of these cell types during helminth infection. Small intestinal tuft cells, for example, have a critical role in sensing helminth infection and stimulating type two innate lymphoid cell (ILC2) expansion through the production of IL-25, thus initiating a type 2 immune response which is critical for helminth expulsion (Faniyi et al., 2020). Tuft cells are particularly abundant in the mucosa of the gallbladder and extrahepatic bile ducts, and the abundance of these biliary tuft cells was recently shown to be negatively regulated by dietary administration of CA (O’Leary et al., 2022). While the abundance of small intestinal tuft cells was not impacted by CA-feeding in this report (O’Leary et al., 2022), it will be worth future work investigating how the helminth-modulated BA pool impacts tuft cell populations, given the importance of this cell type for anti-helminth immunity. Beyond helminth infection impacting signaling through FXR, helminth infection-induced shifts in the primary and secondary BA pool may impact signaling through other cell surface and nuclear receptors known to be responsive to BAs [recently reviewed in (Biagioli and Fiorucci, 2021)], with further potential consequences for intestinal physiology, motility and immunity. It will be important for future research to explore the broader consequences of disrupted BA homeostasis during helminth infection, for example, to determine whether BA disruptions contribute to the altered susceptibility to various enteric microbial infections that have been reported during intestinal helminth infections (Chen et al., 2005; Osborne et al., 2014; Reese et al., 2014; Su et al., 2014; Reynolds et al., 2017; Brosschot et al., 2021; Ingle et al., 2021).
Data availability statement
The original contributions presented in the study are included in the article/Supplementary Material. Further inquiries can be directed to the corresponding author.
Ethics statement
The animal study was reviewed and approved by University of Victoria's Animal Care Committee.
Author contributions
JL and TB designed experiments, performed experiments, analyzed and interpreted data, and wrote the manuscript. DG, CG, KL, and VP performed experiments and interpreted data. LR conceived the study, designed experiments, performed experiments, analyzed and interpreted data, and wrote the manuscript. All authors contributed to the article and approved the submitted version.
Funding
This work was supported by Canadian Institutes of Health Research Project Grants to LR (#156403 and #183831), and a Michael Smith Foundation for Health Research Scholar award to LR (SCH-2021-1582). This work was also supported by equipment grants to LR from the Canadian Foundation for Innovation (36419) and the British Columbia Knowledge Development Fund. These funding sources had no role in the design, analysis, or reporting of this study.
Acknowledgments
The Reynolds laboratory are members of the Host-Parasite Interactions Research Training Network (www.ucalgary.ca/host-parasite-interactions) and we thank this community for their support. We wish to acknowledge the UVic-Genome BC Proteomics Centre, Victoria, Canada, for performing the quantification of bile acid levels, with special thanks to Jun Han. We would like to thank Dr. Christopher J. Nelson for his technical expertise, and Dr. Haley Vecchiarelli and Richard Cartwright who each provided technical assistance during experiments. We would also like to thank the University of Victoria’s animal care team for providing excellent care of experimental animals used for this project.
Conflict of interest
The authors declare that the research was conducted in the absence of any commercial or financial relationships that could be construed as a potential conflict of interest.
Publisher’s note
All claims expressed in this article are solely those of the authors and do not necessarily represent those of their affiliated organizations, or those of the publisher, the editors and the reviewers. Any product that may be evaluated in this article, or claim that may be made by its manufacturer, is not guaranteed or endorsed by the publisher.
Supplementary material
The Supplementary Material for this article can be found online at: https://www.frontiersin.org/articles/10.3389/fpara.2023.1214136/full#supplementary-material
References
Arifuzzaman M., Won T. H., Li T. T., Yano H., Digumarthi S., Heras A. F., et al. (2022). Inulin fibre promotes microbiota-derived bile acids and type 2 inflammation. Nature 611, 578–584. doi: 10.1038/s41586-022-05380-y
Baska P., Norbury L. J. (2022). The role of the intestinal epithelium in the “Weep and sweep” response during gastro–intestinal helminth infections. Animals 12, 175. doi: 10.3390/ani12020175
Biagioli M., Fiorucci S. (2021). Bile acid activated receptors: integrating immune and metabolic regulation in non-alcoholic fatty liver disease. Liver Res. 5, 119–141. doi: 10.1016/j.livres.2021.08.003
Biagioli M., Marchianò S., Carino A., Di Giorgio C., Santucci L., Distrutti E., et al. (2021). Bile acids activated receptors in inflammatory bowel disease. Cells 10, 1281. doi: 10.3390/cells10061281
Brosschot T. P., Lawrence K. M., Moeller B. E., Kennedy M. H. E., Fitzpatrick R. D., Gauthier C. M., et al. (2021). Impaired host resistance to salmonella during helminth co-infection is restored by anthelmintic treatment prior to bacterial challenge. PloS Negl. Trop. Dis. 15, 1–16. doi: 10.1371/journal.pntd.0009052
Brosschot T. P., Reynolds L. A. (2018). The impact of a helminth modified microbiota on host immunity. Mucosal Immunol. 11, 1039–1046. doi: 10.1038/s41385-018-0008-5
Chen C. C., Louie S., McCormick B., Walker W. A., Shi H. N. (2005). Concurrent infection with an intestinal helminth parasite impairs host resistance to enteric citrobacter rodentium and enhances citrobacter-induced colitis in mice. Infect. Immun. 73, 5468–5481. doi: 10.1128/IAI.73.9.5468-5481.2005
Chen M. L., Takeda K., Sundrud M. S. (2019). Emerging roles of bile acids in mucosal immunity and inflammation. Mucosal Immunol. 12, 851–861. doi: 10.1038/s41385-019-0162-4
Coomes S. M., Pelly V. S., Kannan Y., Okoye I. S., Czieso S., Entwistle L. J., et al. (2015). IFNγ and IL-12 restrict Th2 responses during Helminth/Plasmodium Co-infection and promote IFNγ from Th2 cells. PloS Pathog. 11, e1004994. doi: 10.1371/journal.ppat.1004994
Dawson P. A., Karpen S. J. (2015). Intestinal transport and metabolism of bile acids. J. Lipid Res. 56, 1085–1099. doi: 10.1194/jlr.R054114
Dawson P. A., Lan T., Rao A. (2009). Thematic review series: bile acids. bile acid transporters. J. Lipid Res. 50, 2340–2357. doi: 10.1194/jlr.R900012-JLR200
Faniyi A. A., Wijanarko K. J., Tollitt J., Worthington J. J. (2020). Helminth sensing at the intestinal epithelial barrier–a taste of things to come. Front. Immunol. 11, 1489. doi: 10.3389/fimmu.2020.01489
Fiorucci S., Carino A., Baldoni M., Santucci L., Costanzi E., Graziosi L., et al. (2021). Bile acid signaling in inflammatory bowel diseases. Dig. Dis. Sci. 66, 674–693. doi: 10.1007/s10620-020-06715-3
Foley M. H., O’Flaherty S., Barrangou R., Theriot C. M. (2019). Bile salt hydrolases: gatekeepers of bile acid metabolism and host-microbiome crosstalk in the gastrointestinal tract. PloS Pathog. 15, e1007581. doi: 10.1371/journal.ppat.1007581
Gathercole L. L., Nikolaou N., Harris S. E., Arvaniti A., Poolman T. M., Hazlehurst J. M., et al. (2022). AKR1D1 knockout mice develop a sex-dependent metabolic phenotype. J. Endocrinol. 253, 97–113. doi: 10.1530/JOE-21-0280
Han J., Liu Y., Wang R., Yang J., Ling V., Borchers C. H. (2015). Metabolic profiling of bile acids in human and mouse blood by LC– MS/MS in combination with phospholipid-depletion solid-phase extraction. Anal. Chem. 87, 1127–1136. doi: 10.1021/ac503816u
Inagaki T., Choi M., Moschetta A., Peng L., Cummins C. L., McDonald J. G., et al. (2005). Fibroblast growth factor 15 functions as an enterohepatic signal to regulate bile acid homeostasis. Cell Metab. 2, 217–225. doi: 10.1016/j.cmet.2005.09.001
Ingle H., Hassan E., Gawron J., Mihi B., Li Y., Kennedy E. A., et al. (2021). Murine astrovirus tropism for goblet cells and enterocytes facilitates an IFN-λ response in vivo and in enteroid cultures. Mucosal Immunol. 14, 751–761. doi: 10.1038/s41385-021-00387-6
Johnston C. J. C., Robertson E., Harcus Y., Grainger J. R., Coakley G., Smyth D. J., et al. (2015). Cultivation of heligmosomoides polygyrus: an immunomodulatory nematode parasite and its secreted products. J. Vis. Exp. 2015, e52412. doi: 10.3791/52412
Kennedy M. H. E., Brosschot T. P., Lawrence K. M., FitzPatrick R. D., Lane J. M., Mariene G. M., et al. (2021). Small intestinal levels of the branched short-chain fatty acid isovalerate are elevated during infection with heligmosomoides polygyrus and can promote helminth fecundity. Infect. Immun. 89, e0022521. doi: 10.1128/IAI.00225-21
Li J., Dawson P. A. (2019). Animal models to study bile acid metabolism. biochim. biophys. Acta - Mol. Basis Dis. 1865, 895–911. doi: 10.1016/j.bbadis.2018.05.011
Llinás-Caballero K., Caraballo L. (2022) Helminths and bacterial microbiota: the interactions of two of humans’ “Old friends.” Int. J. Mol. Sci. 23, 13358 doi: 10.3390/ijms232113358
Lu Z. N., He H. W., Zhang N. (2022). Advances in understanding the regulatory mechanism of organic solute transporter α-β. Life Sci. 310, 121109. doi: 10.1016/j.lfs.2022.121109
Maruyama T., Miyamoto Y., Nakamura T., Tamai Y., Okada H., Sugiyama E., et al. (2002). Identification of membrane-type receptor for bile acids (M-BAR). Biochem. Biophys. Res. Commun. 298, 714–719. doi: 10.1016/S0006-291X(02)02550-0
Monte M. J., Marin J. J. G., Antelo A., Vazquez-Tato J. (2009). Bile acids: chemistry, physiology, and pathophysiology. World J. Gastroenterol. 15, 804–816. doi: 10.3748/wjg.15.804
Moyat M., Lebon L., Perdijk O., Wickramasinghe L. C., Zaiss M. M., Mosconi I., et al. (2022). Microbial regulation of intestinal motility provides resistance against helminth infection. Mucosal Immunol. 15, 1283–1295. doi: 10.1038/s41385-022-00498-8
O’Leary C. E., Sbierski-Kind J., Kotas M. E., Wagner J. C., Liang H. E., Schroeder A. W., et al. (2022). Bile acid–sensitive tuft cells regulate biliary neutrophil influx. Sci. Immunol. 7, eabj1080. doi: 10.1126/sciimmunol.abj1080
Osborne L. C., Monticelli L. A., Nice T. J., Sutherland T. E., Siracusa M. C., Hepworth M. R., et al. (2014). Coinfection. virus-helminth coinfection reveals a microbiota-independent mechanism of immunomodulation. Science 345, 578–582. doi: 10.1126/science.1256942
Peachey L. E., Jenkins T. P., Cantacessi C. (2017). This gut ain’t big enough for both of us. or is it? helminth–microbiota interactions in veterinary species. Trends Parasitol. 33, 619–632. doi: 10.1016/j.pt.2017.04.004
Phelps T., Snyder E., Rodriguez E., Child H., Harvey P. (2019). The influence of biological sex and sex hormones on bile acid synthesis and cholesterol homeostasis. Biol. Sex Differ. 10, 1–12. doi: 10.1186/s13293-019-0265-3
Popple S. J., Burrows K., Mortha A., Osborne L. C. (2021). Remote regulation of type 2 immunity by intestinal parasites. Semin. Immunol. 53, 101530. doi: 10.1016/j.smim.2021.101530
Rapin A., Chuat A., Lebon L., Zaiss M. M., Marsland B. J., Harris N. L. (2020). Infection with a small intestinal helminth, heligmosomoides polygyrus bakeri, consistently alters microbial communities throughout the murine small and large intestine. Int. J. Parasitol. 50, 35–46. doi: 10.1016/j.ijpara.2019.09.005
Rapin A., Harris N. L. (2018). Helminth–bacterial interactions: cause and consequence. Trends Immunol. 39, 724–733. doi: 10.1016/j.it.2018.06.002
Reese T. A., Wakeman B. S., Choi H. S., Hufford M. M., Huang S. C., Zhang X., et al. (2014). Helminth infection reactivates latent γ-herpesvirus via cytokine competition at a viral promoter. Science 345, 573–577. doi: 10.1126/science.1254517
Reynolds L. A., Finlay B. B., Maizels R. M. (2015). Cohabitation in the intestine: interactions among helminth parasites, bacterial microbiota, and host immunity. J. Immunol. 195, 4059–4066. doi: 10.4049/jimmunol.1501432
Reynolds L. A., Redpath S. A., Yurist-Doutsch S., Gill N., Brown E. M., van der Heijden J., et al. (2017). Enteric helminths promote salmonella co-infection by altering the intestinal metabolome. J. Infect. Dis. 215, 1–17. doi: 10.1093/infdis/jix141
Ryan S. M., Eichenberger R. M., Ruscher R., Giacomin P. R., Loukas A. (2020). Harnessing helminth-driven immunoregulation in the search for novel therapeutic modalities. PloS Pathog. 16, e1008508. doi: 10.1371/journal.ppat.1008508
Schälter F., Frech M., Dürholz K., Lucas S., Sarter K., Lebon L., et al. (2022). Acetate, a metabolic product of heligmosomoides polygyrus, facilitates intestinal epithelial barrier breakdown in a FFAR2-dependent manner. Int. J. Parasitol. 52, 591–601. doi: 10.1016/j.ijpara.2022.04.004
Shute A., Callejas B. E., Li S. H., Wang A., Jayme T. S., Ohland C., et al. (2021). Cooperation between host immunity and the gut bacteria is essential for helminth-evoked suppression of colitis. Microbiome 9, 1–18. doi: 10.1186/s40168-021-01146-2
Smith K. A., Maizels R. M. (2014). IL-6 controls susceptibility to helminth infection by impeding Th2 responsiveness and altering the treg phenotype in vivo. Eur. J. Immunol. 44, 150–161. doi: 10.1002/eji.201343746
Su L., Su C., Qi Y., Yang G., Zhang M., Cherayil B. J., et al. (2014). Coinfection with an intestinal helminth impairs host innate immunity against salmonella enterica serovar typhimurium and exacerbates intestinal inflammation in mice. Infect. Immun. 82, 3855–3866. doi: 10.1128/IAI.02023-14
Sukhdeo M. V. K., Croll N. A. (1981). The location of parasites within their hosts: bile and the site selection behaviour of nematospiroides dubius. Int. J. Parasitol. 11, 157–162. doi: 10.1016/0020-7519(81)90079-5
Ticho A. L., Malhotra P., Dudeja P. K., Gill R. K., Alrefai W. A. (2019). Bile acid receptors and gastrointestinal functions. Liver Res. 3, 31–39. doi: 10.1016/j.livres.2019.01.001
Varyani F., Fleming J. O., Maizels R. M. (2017). Helminths in the gastrointestinal tract as modulators of immunity and pathology. Am. J. Physiol. - Gastrointest. Liver Physiol. 312, G537–G549. doi: 10.1152/ajpgi.00024.2017
Wahlström A., Sayin S. I., Marschall H. U., Bäckhed F. (2016). Intestinal crosstalk between bile acids and microbiota and its impact on host metabolism. Cell Metab. 24, 41–50. doi: 10.1016/j.cmet.2016.05.005
Xiang J., Zhang Z., Xie H., Zhang C., Bai Y., Cao H., et al. (2021). Effect of different bile acids on the intestine through enterohepatic circulation based on FXR. Gut Microbes 13, 1949095. doi: 10.1080/19490976.2021.1949095
Yeshi K., Ruscher R., Loukas A., Wangchuk P. (2022). Immunomodulatory and biological properties of helminth-derived small molecules: potential applications in diagnostics and therapeutics. Front. Parasitol. 1. doi: 10.3389/fpara.2022.984152
Keywords: helminths, Bile acids, FXR, Immunomodulation, small intestine, Metabolites
Citation: Lane JM, Brosschot TP, Gatti DM, Gauthier CM, Lawrence KM, Pluzhnikova V and Reynolds LA (2023) Chronic small intestinal helminth infection perturbs bile acid homeostasis and disrupts bile acid signaling in the murine small intestine. Front. Parasitol. 2:1214136. doi: 10.3389/fpara.2023.1214136
Received: 29 April 2023; Accepted: 13 June 2023;
Published: 06 July 2023.
Edited by:
Ulrike Kemmerling, University of Chile, ChileReviewed by:
Alba Cortés, University of Valencia, SpainAntonio Osuna, University of Granada, Spain
Rodolfo Paredes, Andres Bello University, Chile
Copyright © 2023 Lane, Brosschot, Gatti, Gauthier, Lawrence, Pluzhnikova and Reynolds. This is an open-access article distributed under the terms of the Creative Commons Attribution License (CC BY). The use, distribution or reproduction in other forums is permitted, provided the original author(s) and the copyright owner(s) are credited and that the original publication in this journal is cited, in accordance with accepted academic practice. No use, distribution or reproduction is permitted which does not comply with these terms.
*Correspondence: Lisa A. Reynolds, bGlzYXJleW5vbGRzQHV2aWMuY2E=
†Present address: Tara P. Brosschot, Université Paris Cité, Institut Cochin, INSERM U1016, CNRS UMR 8104, Paris, France