- 1Université Paris Saclay, CNRS UMR 9198-CEA, Institute for Integrative Biology of the Cell (I2BC), Gif sur Yvette, France
- 2School of Pharmacy and Pharmaceutical Sciences, Binghamton University, Binghamton, NY, United States
- 3Laboratoire de Biochimie et de Biologie Moléculaire, Faculté des Sciences et Technologies, Université d’Abomey Calavi, Cotonou, Benin
Rhoptries and micronemes are essential for host cell invasion and survival of all apicomplexan parasites, which are composed of numerous obligate intracellular protozoan pathogens including Plasmodium falciparum (malaria) and Toxoplasma gondii (toxoplasmosis) that infect humans and animals causing severe diseases. We identified Toxoplasma gondii TgSORT as an essential cargo receptor, which drives the transport of rhoptry (ROP) and microneme (MIC) proteins to ensure the biogenesis of these secretory organelles. The luminal domain of 752 amino acid long situated at the N-terminus end of TgSORT has been described to bind to MIC and ROP proteins. Here, we present an optimized protocol for expression of the entire luminal N-terminus of TgSORT (Tg-NSORT) in the yeast Pichia pastoris. Optimization of its coding sequence, cloning and transformation of the yeast P. pastoris allowed the secretion of Tg-NSORT. The protein was purified and further analyzed by negative staining electron microscopy. In addition, molecular modeling using AlphaFold identified key differences between the human and the T gondii sortilin. The structural features that are only present in T. gondii and other apicomplexan parasites were highlighted. Elucidating the roles of these specific structural features may be useful for designing new therapeutic agents against apicomplexan parasites
Introduction
Toxoplasma gondii is a single-celled obligate intracellular parasite responsible for toxoplasmosis. It is the leading cause of congenital neurological abnormalities and severe opportunistic infection in immunocompromised individuals (Pappas et al., 2009). This parasite belongs to the large phylum of Apicomplexa, which includes protozoan pathogens such as Babesia, Cryptosporidium, Cyclospora, Isospora and Plasmodium (Kim and Weiss, 2004). Apicomplexan parasites possess various but similar morphological features that constitute the hallmark of the phylum (Morrissette and Sibley, 2002). Among these different structures, the most remarkable is the presence of an apical complex composed of polar ring, conoid and unique secretory organelles named rhoptries and micronemes. During the invasion, micronemes (MICs) release their contents that are required for motility, host cell attachment and egress (Dubois and Soldati-Favre, 2019). MIC proteins (adhesins and escorters) form complexes that link host cell receptors to the glideosome (Opitz and Soldati, 2002). These complexes required for invasion are translocated backwards, allowing the parasite to propel itself into the host cell by membrane invagination. Subsequent proteolysis of these proteins is essential for cell invasion progression by enabling parasite migration into host cell (Brydges et al., 2006). MIC5 was the first microneme protein identified as not possessing an adhesive domain (Brydges et al., 2000). It acts as a regulator of the activity of the parasitic surface protease MPP2 (Brydges et al., 2006). Rhoptries (ROP) proteins are also involved in host cell entry and in the subversion of other host functions such as the control of nuclear transcription and immune responses (Boothroyd and Dubremetz, 2008). Among these proteins, ROP18 phosphorylates and inactivates a family of host immunity related GTPases (IRGs), preserving the parasite from lysis (Fentress and Sibley, 2011). ROP16 interferes with signal transduction in the host nucleus through phosphorylation of the activator STAT3/6 (Butcher et al., 2011). The key roles of rhoptries and micronemes make these organelles the pillars of the parasite survival. However, the formation of these vital secretory organelles depends on the presence of T. gondii Sortilin-like receptor (TgSORTLR), which is a type I transmembrane cargo transporter located in the post-Golgi and endosome related compartments. TgSORTLR is essential to the biogenesis of secretory organelles and in turn to motility, invasion and egress (Sloves et al., 2012). Furthermore, TgSORTLR is required for efficient host immune responses against infection (Sloves et al., 2015). The luminal domain of the receptor binds ROP and MIC proteins (Sloves et al., 2012) while the cytosolic tail recruits partners to enable anterograde and retrograde receptor transport (Sangaré et al., 2016), in a manner similar to sortilins of humans (Nielsen et al., 2001). Therefore, we now named this receptor TgSORT. Furthermore, homologues of TgSORT is present in all apicomplexan parasites whose genomes have been sequenced (VEuPathDB). For example, in the malaria P. falciparum, PfSORT determines transport of proteins to form rhoptries (Hallée et al., 2018a; Hallée et al., 2018b).
In humans, sortilins are multifunctional receptors whose structures are defined by the backbone of yeast VPS10 composed of ten β-propeller domains, two cystein bound domains, one transmembrane domain and C-terminus tail (Quistgaard et al., 2009). These sortilins function in a mannose-6-phosphate independent manner for the sorting of numerous enzymes from the endosomal system to the lysosomes. Expressed in a number of vertebrate tissues, notably brain, spinal cord, testis, and skeletal muscle, sortilins also function as a surface coreceptor for induction of neural apoptosis in the brain (Kim and Hempstead, 2009) and are linked to type 2 diabetes (Clee et al., 2006) and Alzheimer’s disease (Rogaeva et al., 2007). However, mice or yeast deficient in sortilin/VPS10 are viable and show relatively mild phenotypes (Marcusson et al., 1994; Jansen et al., 2007). In contrast, as demonstrated for T. gondii and P falciparum, this receptor is an essential factor that allows apicomplexan parasites to build the complex apical structure composed of the functional conoid containing rhoptries and micronemes (Sloves et al., 2012; Sakura et al., 2016; Sangaré et al., 2016; Hallée et al., 2018a; Hallée et al., 2018b). However, the percentage of identity between T. gondii sortilin and its human counterparts is only about 27%. Interestingly, four peptide insertions are present in the β-propeller domains B, H, 10CCb and the C-terminus tail in all apicomplexan parasite’s sortilins identified up-to-date (Sloves et al., 2012). The presence of conserved specific peptide insertions exclusively in sortilins of apicomplexan parasites suggests that they may have peculiar 3-D structures compared to the classical VPS10 backbone that can be exploited for future therapeutic interventions. In the present study, we established optimal conditions for expression of a secreted soluble N-terminus luminal end of TgSORT in the methylotrophic yeast Pichia pastoris for structural analysis including a powerful computational predictive program AlphaFold to propose a 3D-model of TgSORT.
Materials and methods
Strains, vectors and reagents
Pichiapink secreted protein kit (Thermofisher scientific) containing one, pPINKαHC vector, 5’α-factor primer, 3’CYC1 primer was used in this study. PichiaPink expression strains composed of 4 adenine auxotrophic Pichia pastoris strains were as follows: PichiaPink strain 1, a wild strain of genotype ade2, PichiaPink strain 2 (ade2, pep4), PichiaPink strain 3 (ade2, prb1) and PichiaPink strain 4 (ade2, pep4, prb1). Pichia Pink Media kit (Dextrose, Pichia Adenine Dropout Agar, yeast extract peptone dextrose, yeast extract peptone dextrose sorbitol, yeast extract peptone dextrose). Additional reagents included yeast Nitrogen Base (Thermofischer scientific), methanol (Millipore), biotin and sorbitol (Sigma Aldrich), glycerol, 1M potassium phosphate buffer. Rabbit anti-Flag antibodies (Sigma Aldrich) and rat anti-Tg-NSORT antibodies were produced in the laboratory as previously described (Sloves et al., 2012). The following media were also prepared and included YPD broth, YPD agar, YPDS yeast extract peptone dextrose sorbitol, PAD agar (Pichia Adenine Dropout Agar), BMGY and BMMY.
Pichia pastoris expression vector
The N-terminus luminal coding region of TgSORT (named here Tg-NSORT) from amino acid 37 to 789 (TgSORTLR ToxoDB accession Number TGME49_290160) was designed with Flag and 6xHistidine epitopes added to the C-terminus end. The synthesis and cloning of this Flag and 6xHis tagged TgSORT coding nucleotide sequence in pPINKαHC were performed by Genscript based on P. pastoris codon usage. This vector contained the Saccharomyces cerevisiae α-factor signal peptide added upstream to Tg-NSORT (N-terminus) to allow the traffic of expressed protein to the secretory pathway. The expression was under the control of the methanol-inducible promoter AOX1.
Transformation of P. pastoris
Strains transformation was made according to Thermofisher scientific recommendations, which are based on modified protocols previously described (Wu and Letchworth, 2004). All cultures were carried out at 27°C, 300 rpm and centrifugations at 1500xg for 5 minutes. The different strains of Pichia pastoris were grown on YPD agar plates for 24 hours. Starter cultures were performed by incubating an isolated colony in 10 ml of YPD medium for 24 hours. Hundred ml of cultures were made from an OD600 of 0.2 to OD600 between 1.3 and 1.5. Pellet was recovered, washed twice in cold sterile water and then in ice-cold 1M sorbitol. Cells were permeabilized for 30 minutes at room temperature using a buffer composed of 100 mM lithium acetate, 10 mM DTT, 0.6 M sorbitol and 10 mM Tris-HCl pH 7.5. Three washes were performed with ice-cold 1M sorbitol and electro-competent yeast strains were transferred to 0.2-cm electroporation cuvette and 1 µg of linearized recombinant plasmid was added, and kept on ice for 5 minutes. Transfection was performed at 1500 V, 186 Ω and 25 µF using the BTX Electro Cell Manipulator 600. One ml of ice cold YPDS medium was immediately added and the mixture was kept for 2 hours at 27°C without shaking. Ten µl and 100 µl were spread on PAD plates and incubated at 27°C for 24-48 hours until formation of colonies. Positive clones were isolated and cultured for protein expression and purification as described below.
Recombinant Tg-NSORT protein expression
Protein expression was achieved according to the manufacturer’s protocol. All incubations were performed at 27°C under 250 rpm shaking. Pilot experiments were first performed to determine the optimal expression conditions. 10 ml of culture were carried out in BMGY for 24 hours in a 250 ml flask. Cultures were centrifuged at 1500 g for 5 minutes at room temperature and the pellet was resuspended in 1 ml of BMMY. Cells were again incubated overnight before starting the inductions. The four different strains have been tested as well as at range of concentrations of 0.5%, 1%, 2%, 3%, 4% and induction times of 6, 24, 48, 72 and 96 hours. At the end of induction periods, all supernatants were recovered by centrifugation for 10 minutes, analyzed by SDS-PAGE and Western blot using the rat anti Tg-NSORT antibodies. P. pastoris strain transformed with empty vector served as negative control.
After determining the best conditions for expression, a large-scale production was performed. Briefly, one liter of BMGY (Buffered Glycerol-complex Medium) culture medium was seeded by 25 ml of the 24-hour pre-culture of positive clones isolated above. When the OD reached between 2 and 6, the culture was centrifuged and the pellet was resuspended in 200 ml BMMY (Buffered Methanol-complex Medium) induction medium. After one day of incubation, inductions were performed every 24 hours. Supernatants were analyzed by SDS-PAGE and Western blots. We have also isolated Tg-NSORT from 3 liter of cultures.
Affinity column purification of Tg-NSORT
Two hundred ml of supernatant containing recombinant Tg-NSORT was concentrated to 5 ml using 30-kDa cutoff Millipore centrifugal filters. The concentrated sample was diluted 1:10 with 1X binding buffer (50 mM Tris pH 7.5, 250 mM NaCl and 5% glycerol) and then incubated on Nickel-NTA beads for 4 hours at 4°C with 1 mM PMSF and inhibitor cocktail. Three washes were performed with 50 mM Tris.HCl pH 7.5, 1 M NaCl and 5% glycerol buffer and three additional washes were done using 50 mM Tris.HCl pH 7.5, 150 mM NaCl and 5% glycerol. Recombinant Tg-NSORT was eluted twice with 200 mM of imidazole and eluates were concentrated in 1X PBS, 5% glycerol before size exclusion chromatography as described below.
SDS-PAGE
Sixteen µl of supernatant were mixed with 4 µl of 5X Laemmli buffer and heated at 100°C for five minutes. SDS-PAGE was performed in 12% gel under reducing conditions by sample migration at 30 V until its reached running gel then at 70 V and 120 V. Gels were stained with BIO-RAD Coomassie brilliant blue R-250 staining solution. The protein bands of interest were excised and processed for mass spectrometry.
In-gel digestion of protein and LC-MS/MS analysis
The protein bands corresponding to Tg-NSORT expressed and purified from culture supernatants of P. pastoris were washed three times with 120 μl solution of 25 mM ammonium bicarbonate (NH5CO3)/acetonitrile (50/50, v/v) for 10 min. Then, in-gel reduction and alkylation of protein disulfide bonds was performed with 100 μl of 10 mM of DTT for 50 min at 50 °C 199 μl of 50 mM iododacetamid was added and incubated for another 30 min at room temperature. After a washing step with 120 μl of 25 mM NH5CO3 and the dehydration step with 100 μl acetonitrile for 5 min, an in-gel digestion was performed on each sample with 0.07 μg of sequencing grade porcine trypsin (Promega, Madison, WI) for 16 h at 37 °C. The peptides were extracted from gel using a mixture of acetonitrile/25 mM of NH5CO3 (v/v) (60/40, v/v) containing 0.1% formic acid. The extracted peptides were then dried with vacuum centrifuge (Uniequip GmbH, Munich, Germany) and resuspended in 10 μl of water containing 0.1% formic acid. Aliquots of 3 μl from each sample was injected into the Ultimate 3,000 RSLC nano- System (Dionex, Thermo Scientific) connected to a Q-HF orbitrap mass spectrometer using a nano column (Acclaim PepMap RSLC, 15 cm × 75 μm inner diameter, C18, 2 μm, 100 Å; Dionex, Sunnyvale, CA). The elution was carried out by applying mixture of solvent A (HPLC grade water with 0.1% formic acid) and solvent B (HPLC grade acetonitrile with 0.1% formic acid) at the flow rate of 300 nL per min by applying a linear gradient from 2 to 40% solvent B over 38 min. After a washing step for 5 min with 70% solvent B, an equilibration step for 11 min with 2% solvent B were done. For ionization, a nanospray Flex Ion Source was used with a voltage set at 1.9 kV, and a capillary temperature at 275 °C. Full MS scans were acquired in the Orbitrap mass analyzer over an m/z range of 300-3,500 and a resolution of 70,000 at m/z 200. The target automatic gain control value of 1 × 106 was used with a maximum allowed injection time (Maximum IT) of 250 ms. For MS/MS, an isolation window of 2 m/z was utilized. Peptide fragmentation was performed using high-energy collision induced dissociation with a normalized collision energy set at 35. The tandem mass spectra were acquired over m/z 200-2,000 range at a resolution of 35,000 at m/z 200 and an automatic gain control of 2 × 105. The ion intensity selection threshold was 6.7 × 104, and the maximum injection time was 150 ms. All these systems were fully controlled by Thermo Xcalibur 3.0 (Thermo Fisher Scientific). All data files (*.raw) collected were processed with a specific workflow designed in Proteome Discoverer 1.4 (Thermo Fisher Scientific). MS/MS data was interpreted using two search engine Mascot (version 2.4.1, Matrix Science, London, UK) and Sequest HT (Thermo Fisher Scientific). Searches were performed against T. gondii (TGVEG, TGME49 and TGGT1 strains) protein sequences downloaded from www.toxodb.org. The Mascot ion score were >20 and Sequest HT XCorr >1.5. The decoy database search allowed us to control and to estimate the false positive identification rate.
Western blots
After SDS-PAGE, proteins were transferred on nitrocellulose membrane at 80 V for 1 hour. Membranes were blocked for 30 minutes at room temperature in 5% skim milk prepared in TNT (15 mM Tris-HCl pH8, 140 mM NaCl, 0.05% Tween-20). Incubation with antibodies was done for 1hour at room temperatures with 10 minutes washes for three times with TNT. Blotting membranes were developed with standard chemiluminescent solution (GE healthcare) and scanned using Vilber fusion FX 6.0 apparatus (France).
Size-exclusion chromatography of Tg-NSORT
Size-Exclusion Chromatography (SEC) using a 24 ml Superose 6 Increase 10/300 GL column in a buffer composed of 50 mM Tris-HCl pH 8, 150 mM NaCl and 1 mM EDTA, was used to purify the concentrated eluate of Tg-NSORT. A single peak corresponding to Tg-NSORT was observed at 17.5 ml by monitoring elution at 280 nm.
Negative staining of TgN-SORT
Samples were analyzed by conventional electron microscopy using the negative staining method. Three μL suspension (0.05 mg mL−1) were deposited on an airglow-discharged carbon-coated grid. Excess liquid was blotted, and the grid rinsed with 2% w/v aqueous uranyl acetate. The grids were visualized at 100 kV with a TECNAI Spirit (FEI) transmission electron microscope (ThermoFisher, New York NY, USA) equipped with a K2 Base 4k × 4k camera (Gatan, Pleasanton CA, USA). Final magnification was at 34.500 x, corresponding to a pixel size at the level of the specimen of 0.14 nm. Data were recorded under low-dose conditions (dose rate 20 e A−2).
Results
Optimizing conditions for Tg-NSORT expression
We have used four distinct yeast Pichia pastoris, which were mutants lacking respectively either one gene coding a first protease, a second protease or a double mutant lacking both proteases and wild type strains. The use of these mutants allows us to minimize the rate of degradation of the recombinant protein expressed in the yeast and to achieve an optimal expression in one of these four P. pastoris strains. Figure 1A depicted the plasmid that contains the coding DNA sequence of Tg-NSORT tagged to FLAG and 6XHis epitopes and used to transform these yeasts. Transformation of these P. pastoris strains resulted in good integration of the plasmids into the genome. Two positive colonies of each strain were shown to express a protein migrating at about 100 kDa band that corresponds to the expected size of Tg-NSORT (Figure 1B). However, different amounts of the 100-kDa protein were observed after Coomassie blue staining plus few additional smaller bands with one predominant band around 50 kDa (Figure 1B). We showed that the specific anti-TgSORT antibodies recognized this 50-kDa protein, suggesting it as a proteolytic cleavage product of the apparently intact and much stronger TgN-SORT band of 100-kDa size (Figure 1C). Based on the level and intactness of TgN-SORT, we selected clone 1 of Pichia pastoris mutant strain 4 for our studies (Figures 1B, C, see the red box). Using this Tg-NSORT clone 1, we established that the same level of the 100-kDa band was expressed regardless of the methanol concentrations, except that a slight decreased in intensity was observed at 4% methanol (Figures 1D, E). In addition, the amount of TgN-SORT produced in this clone also increased with time (Figures 1F, G). For our purposes, we picked out 2% of methanol induction for 72 hours as the optimal conditions at 27°C under constant shaking for efficient expression of Tg-NSORT in Pichia pastoris.
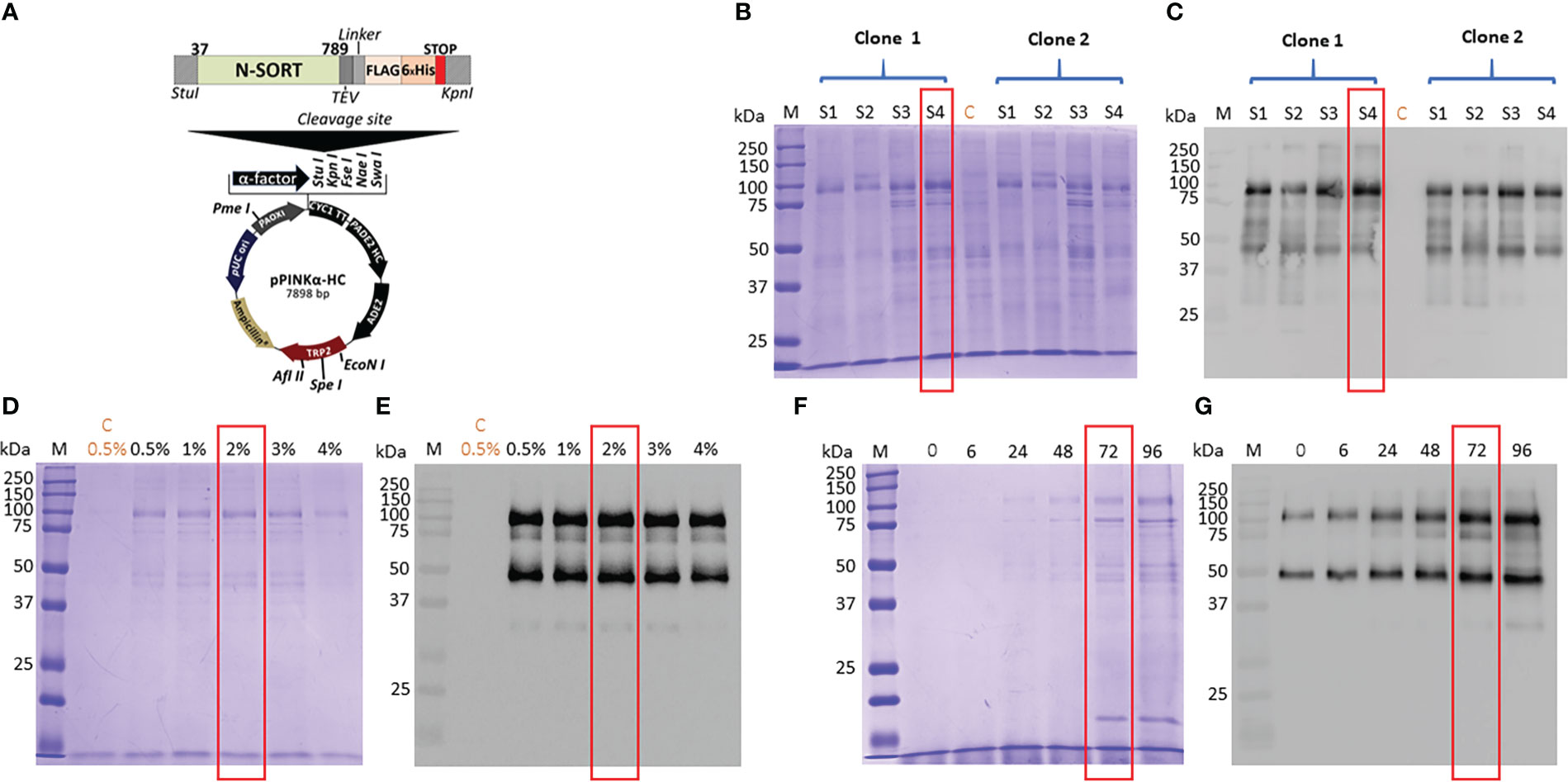
Figure 1 (A) Design of the expression vector of TgSORT in Pichia pastoris. (B) A pilot experiment of Tg-NSORT expression and transformants derived from Pichia pastoris S1, S2, S3 and S4-strains were analyzed by SDS-PAGE and Coomassie blue staining. (C) Western blot of this pilot experiment using anti-TgSORT antibodies; (D) The optimal methanol concentration for induction determined by Coomassie bue staining; (E) The corresponding blots of panel D; (F) Coomassie blue staining showing the optimal time (hours) for methanol induction; (G) The corresponding blot of panel (F) C means negative control.
Purification of secreted Tg-NSORT from Pichia pastoris
After, a small-scale purification of Tg-NSORT using Ni-NTA resin, we checked that the 100 kDa and 50 kDa bands were recognized by Western blots using anti-His and anti-Flag antibodies corresponding to the two-epitope tags placed at the C-terminus of Tg-NSORT (Figure 2A). The same bands stained by Coomassie blue after polyacrylamide gel electrophoresis were excised and processed for LC-MS/MS analysis. Sixty-three peptides covering 55% of the length of TgSORT were identified in the 100-kDa protein, indicating it is a genuine ectodomain of Tg-NSORT expressed and secreted from P. pastoris (Figure 2B). The nature of these peptides is described in Table 1, which also shows the sequence of 46 peptides detected by mass spectrometry in the 50-kDa region, suggesting it as a fragment product of Tg-NSORT. Altogether, these data demonstrate that Tg-NSORT was expressed and secreted by P. pastoris. After this verification, we embarked on a large-scale production and purification of Tg-NSORT. Figure 3 shows the quality of Tg-NSORT secreted by P. pastoris in one liter of culture medium, which was used to purify the protein by Ni+-NTA beads (Figures 3A, B). The highest amount of protein was obtained after 200 mM of imidazole elution (see E2) and this yielded to 0.5 mg of total Tg-NSORT protein with fewer degradation (Figures 3A, B). The increase of the volume of P. pastoris culture to three liters resulted in a higher quantity of protein that reached 2 mg of purified protein (Figures 3C, D). Next, we decided to improve the purity of Tg-NSORT by removing the smaller degration products seen in Figure 3 by size exclusion chromatography. In these gel filtration conditions, Tg-NSORT was eluted at 17.5 ml, which corresponds to a molecular mass between 44 and 158 kDa on this column (Figure 4A). SDS-PAGE revealed Tg-NSORT at about 100 kDa, but the proteolytic cleavage product of 50 kDa was still present after size exclusion chromatography, suggesting that may bind to the 100 kDa protein (Figure 4B). It is known that sortilins can dimerize and it is possible that this 50 kDa may strongly interact with the full-length ectodomain of 100 kDa, therefore it cannot be removed by size exclusion chromatography.
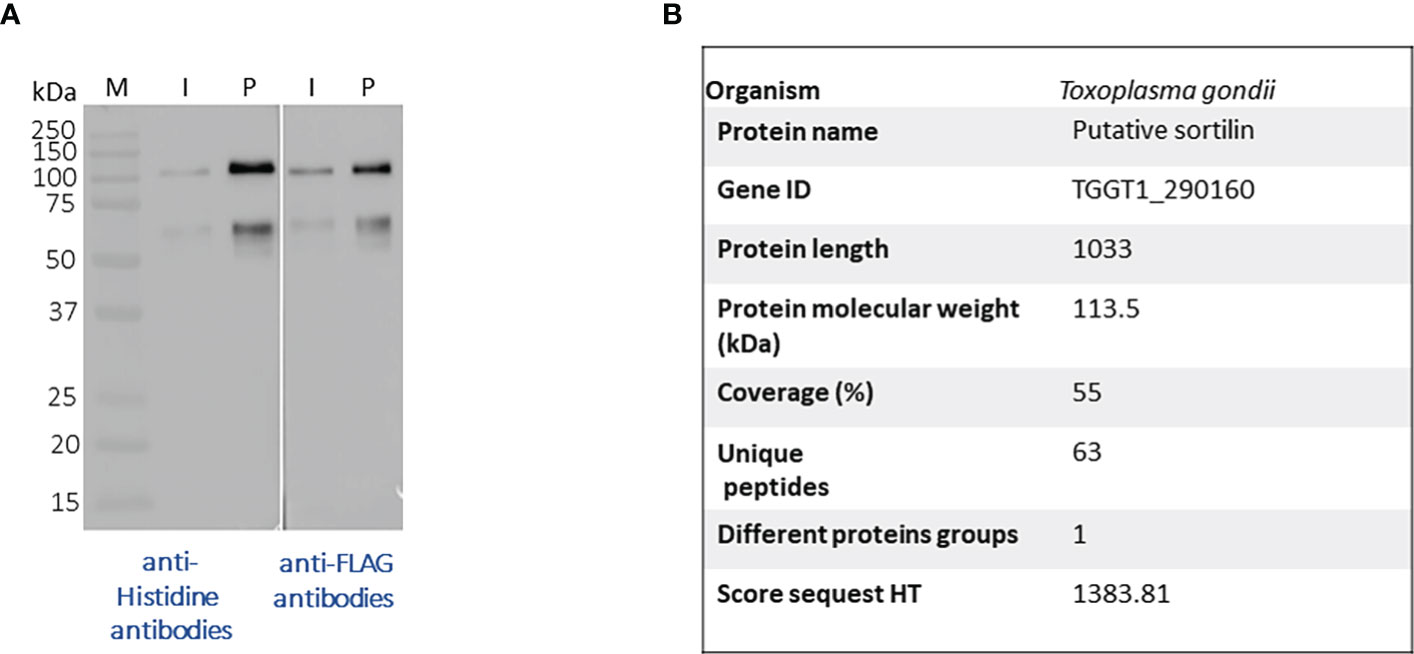
Figure 2 (A) Western blots after a small-scale purification of Tg-NSORT using Ni+NTA column and the culture supernatant of Pichia pastoris induced by 2% of methanol for 3 days. The blots were revealed with anti-Histidine and anti-Flag, two epitope tags at the C-terminus of Tg-NSORT. (B) The same material analyzed in panel A was staining by Coomassie blue and processed by mass spectrometry to confirm the 100-kDa band as Tg-NSORT.
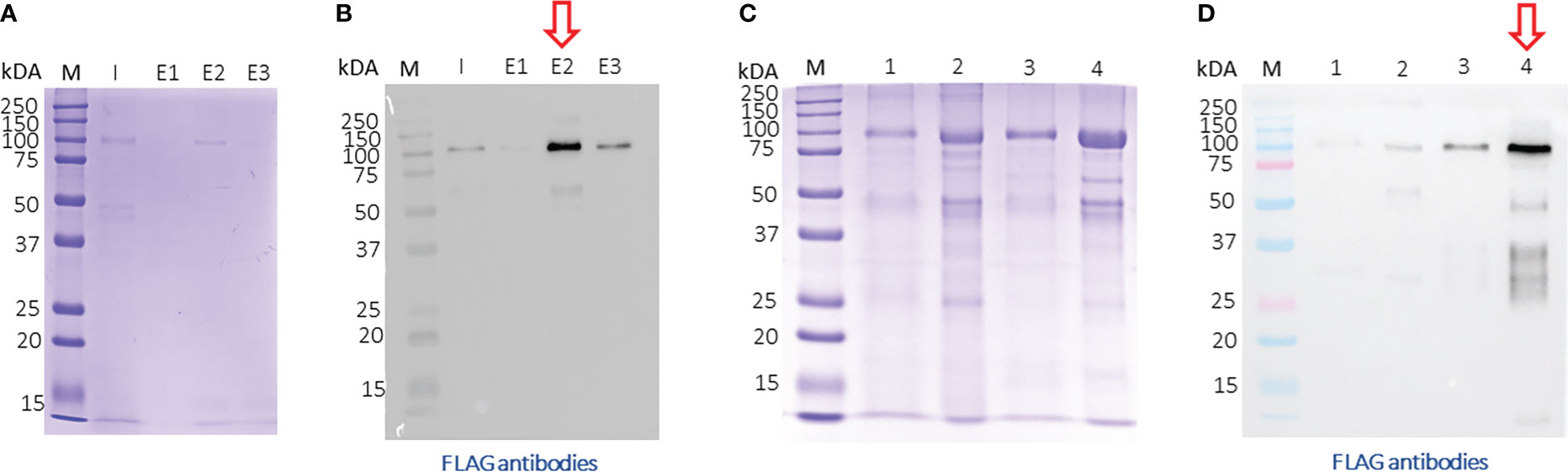
Figure 3 Large-scale purification of TgN-SORT by affinity chromatography (A) Coomassie blue staining and corresponding blot (B) after purification from one liter of Pichia pastoris transformant grown in complete medium culture; I: Input; E1: 1st eluate at 50 mM of imidazole; E2: 2nd eluate at 200 mM of imidazole; E3: 3rd eluate at 200 mM of imidazole; (C, D) the same experiment using three liters of culture medium containing secreted TgSORT by Pichia pastoris;1: Input 1X; 2: Input TgN-SORT 20X material; 3: 1X eluate of TgN-SORT; 4: 15X eluate of TgN-SORT.
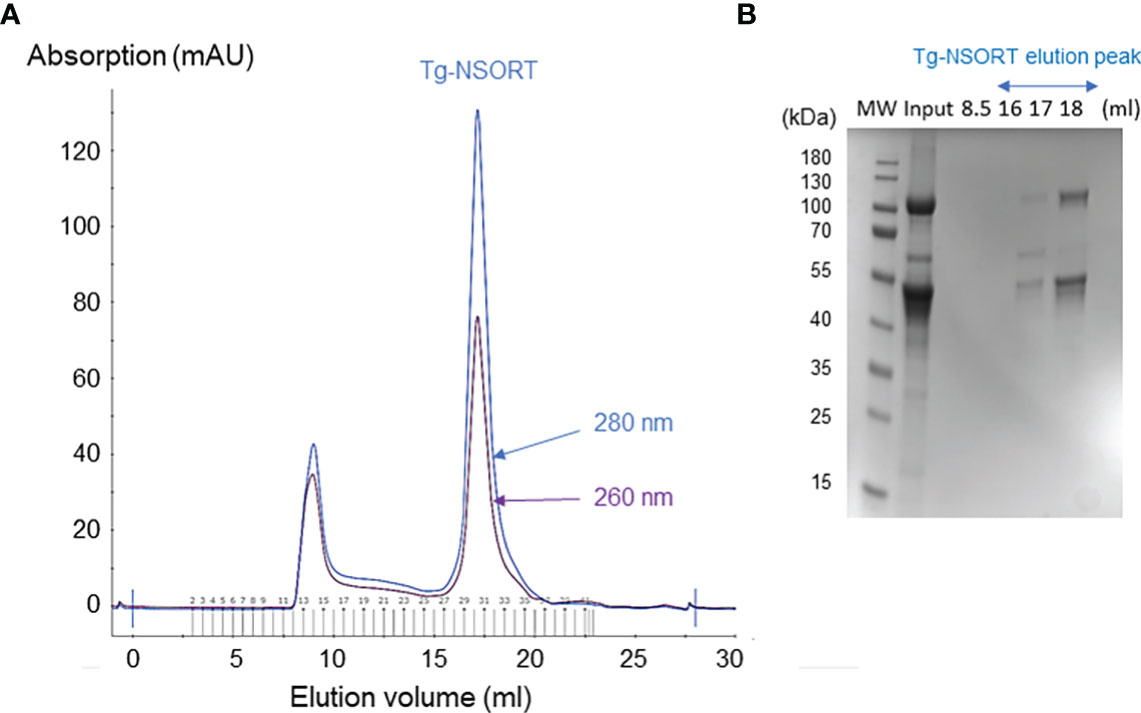
Figure 4 Size exclusion chromatography of Tg-NSORT. (A) Chromatogram recorded at 260 (purple) and 280 (blue) nm showing the peak corresponding to eluated Tg-NSORT. (B) SDS-PAGE and Coomassie blue staining of eluate containing Tg-NSORT.
Negative staining electron microscopy
We diluted the purified material from size chromatography down to 0.05 mg/ml concentration to analyze it by conventional Electron Microscopy (EM) using the negative staining method. We obtained EM micrographs showing homogeneous and well-dispersed particles, suggesting that a protein having a single conformation was present in our gel filtration samples (Figure 5A). Analysis of these micrographs revealed a ring-shaped protein structure with a diameter of about 8 nm that resembles that previously shown for human sortilin (Figure 5B). However, further attempts to crystallize Tg-NSORT or to analyze it by cryo-electron microscopy failed. Therefore, we used the recent AlphaFold2 program (Jumper et al., 2021) to calculate a model of Tg-NSORT.
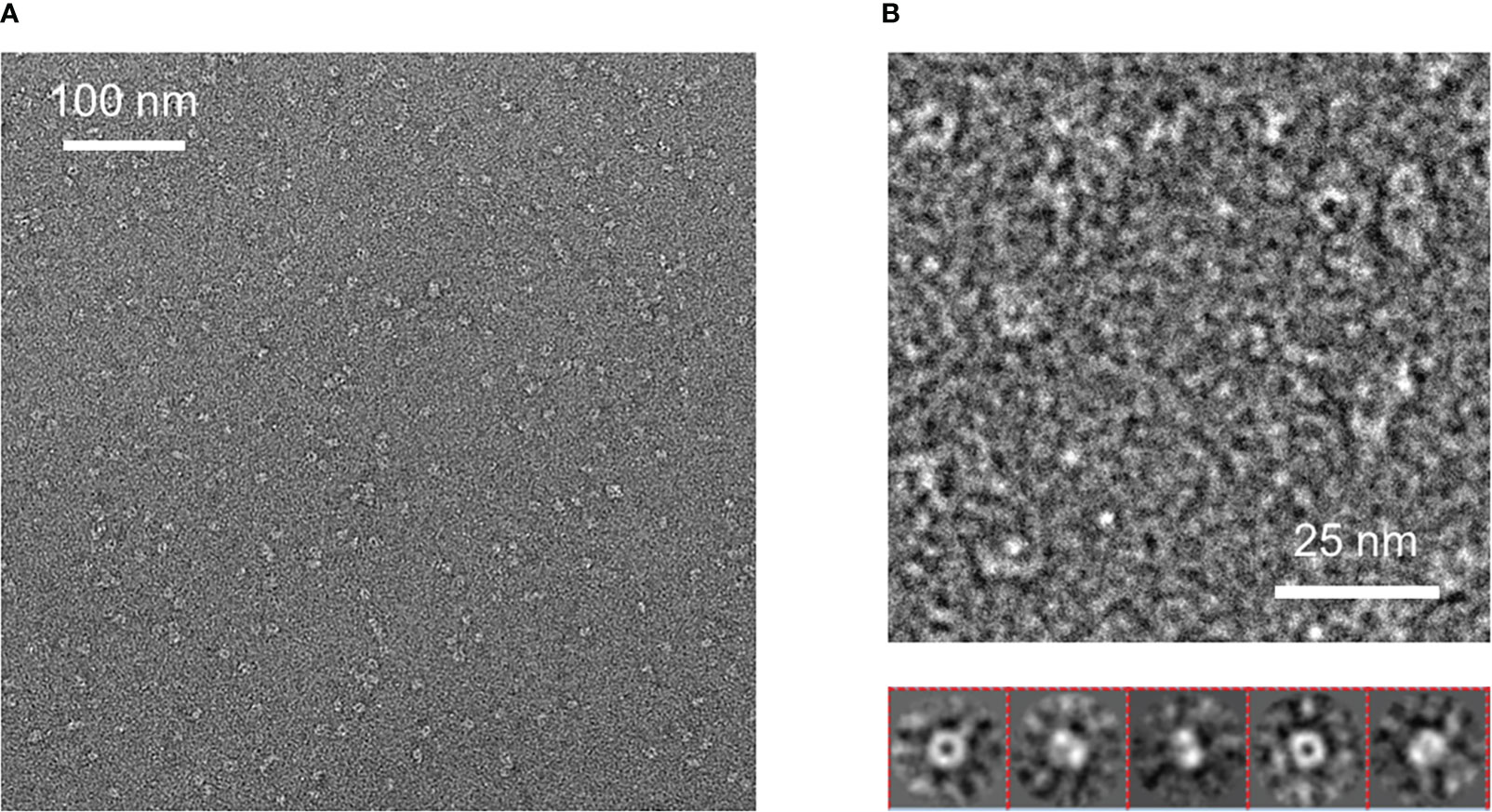
Figure 5 (A) Negative staining electron micrograph of Tg-NSORT purified by gel filtration. (B) Zoom on a micrograph and 2D classification of the particles picked on the micrographs.
AlphaFold analyses
We provided as an input for AlphaFold2 the sequence of Tg-NSORT. Using this recent program, we obtained a three-dimensional model of Tg-NSORT (Figure 6A), and in particular of the four parasite’s specific peptide insertions, which are organized as loops within TgSORT (Figures 6A, C). We also attempted to model the 3D structure of Tg-NSORT bound to its partners. AlphaFold2 predicted with a reasonable significant score (lDDT values for the residues of the disordered binding partners larger than 0.7) that Tg-NSORT interacts with different ROP proteins (ROP1, ROP5, ROP16) through specific motifs located in intrinsically disordered regions (IDRs) of ROPs proteins (see the model of Tg-NSORT bound to ROP1 in Figure 7A). The motif PPNAQELLPP of this IDR binds to the tunnel formed by the ten β-propeller domains of TgN-SORT. These motifs are found in the pro-peptide of the N-terminus of all ROP proteins. They all bind to the same site in Tg-NSORT within the inner tunnel of the protein. Such binding mode was already observed for neurotensin binding to human sortilin (Figure 7B; Quistgaard et al., 2009).
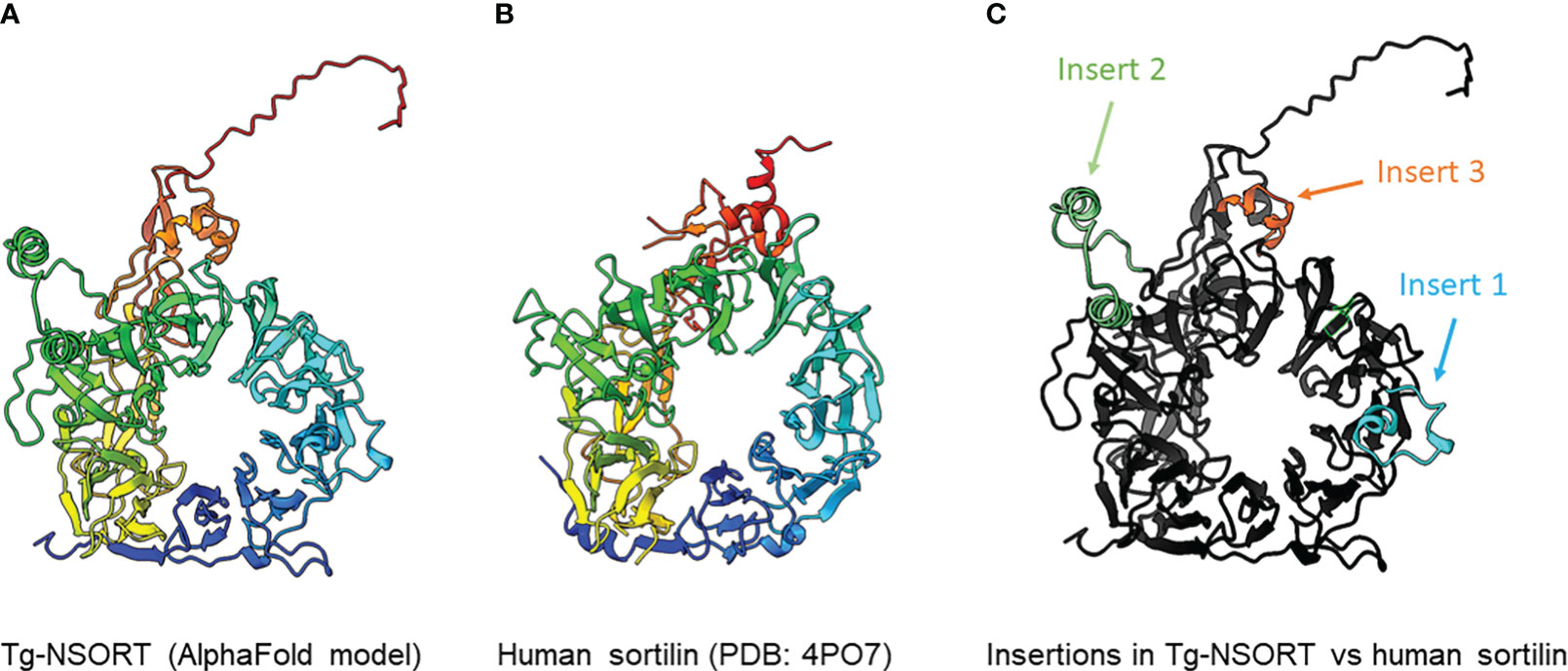
Figure 6 (A) 3D model of Tg-NSORT calculated using AlphaFold2. (B) Crystal structure of human sortilin. (C) Representation of the Tg-NSORT model, with the insertions specific to Apicomplexa colored in cyan (insert or loop 1), green (insert or loop 2) and orange (insert or loop 3). In panels (A) and (B), proteins are colored from blue (N-terminus) to red (C-terminus).
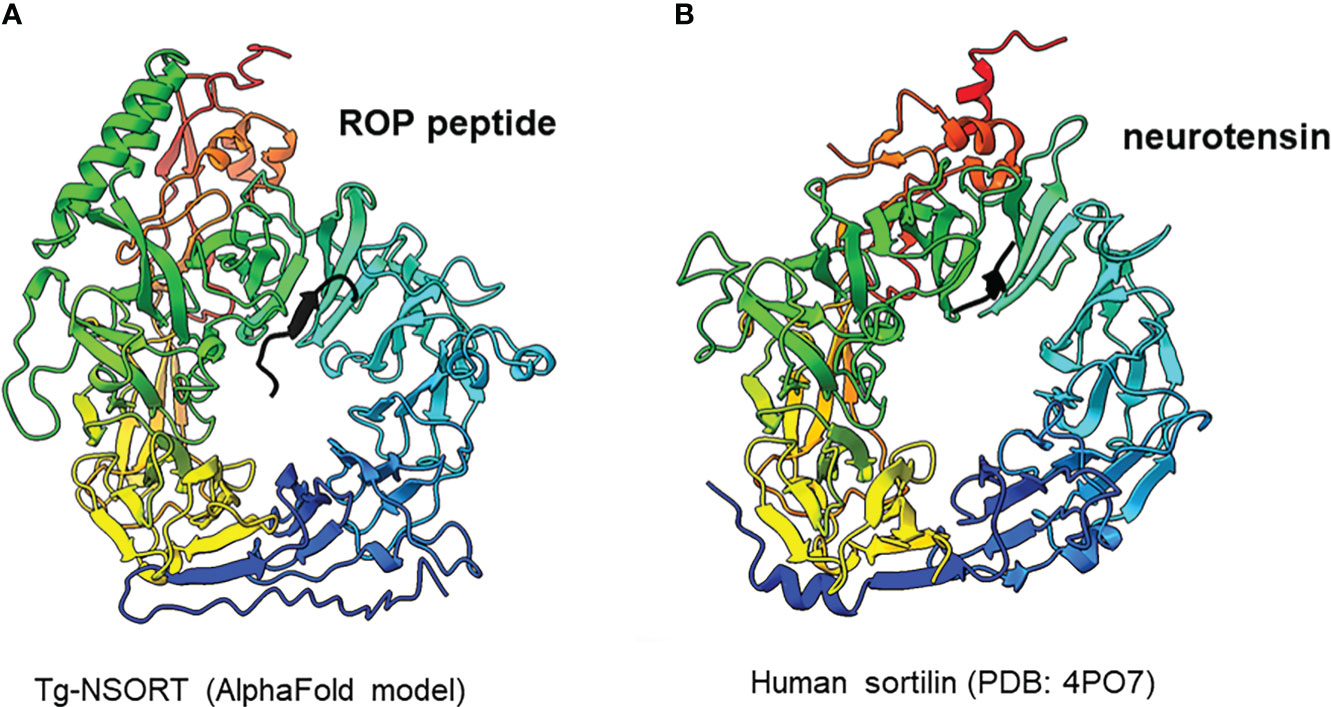
Figure 7 (A) Interaction between Tg-NSORT (from blue to red) and the intrinsically disordered region (IDR) of ROP1 (in black), as determined by AlphaFold2. The motif PPNAQELLPP of this IDR binds to the tunnel formed by the ten β-propeller domains of sortilin. (B) Interaction between human sortilin and the neurotensin peptide, as observed in the crystal structure referenced as 4PO7 in the PDB.
Discussion
Pichia pastoris system has a good record of accomplishment in expressing proteins from both prokaryotes and eukaryotes for the most part difficult to express in E. coli. Human Coagulation Factor XIIIa, which was insoluble after its complicated expression in E. coli (Nikolajsen et al., 2014) was successfully produced by Chang et al. (2011) using P. pastoris. As used in the present study, these authors also employed the methanol inducible AOX1 promoter to efficiently express the protein for which they evaluated different biological activities (Cheng et al., 2021). The same AOX1 promoter controls the expression of the human camel chymosin in P. pastoris (Wang et al., 2015). Proteins expression in P. pastoris depends on different parameters including pH of the culture medium, inducing agent and temperature. As for human coagulation factor XIIIa, the ectodomain of T. gondii TgSORT composed of the ten β-propeller and double 10 C-C bound domains is insoluble because it is completely directed into inclusion bodies when expressed in E. coli. Now, we have been quite successful in expressing the ectodomain of TgSORT, which is transported through the secretory pathway and can be recovered in the culture supernatant as several milligrams of proteins using P. pastoris. As this yeast is a eukaryotic model system; we assume that the parasite TgSORT will be well folded in addition to some post-translational modifications that may be necessary for future functional investigations. It should be noticed that, for example, T. gondii ROP2 protein has been already expressed in P. pastoris and the protein has been used for diagnosis (Chang et al., 2011). However, the level of ROP2 in P. pastoris appears weaker than what we have achieved in this study. After 72 hours of induction with 2% of methanol, we were able to obtain several milligrams of pure recombinant TgSORT. It is known that the experimental conditions are important for protein expression in this yeast. Unlike Cheng et al. (2021) who achieved expression of human coagulation factor XIIIa at 30°C with 1% methanol every 24h for 120h, Wang et al. (2015) induced expression of camel chymosin at pH 4.07 and even at lower temperature of 28°C after 8h. After evaluation of the different parameters, expression of human serum albumin was performed at 28°C for 24 hours in an acidic pH of 5.75 and the methanol concentration was set between 0.5 and 2% and for every 2 hours (Lau et al., 2012). In addition, of these conditions defined for human proteins expression in P. pastoris, other T. gondii proteins whose expression was difficult in E. coli were also produced in this yeast. TgGRA2 was expressed in P. pastoris at 28°C for 5 days under 0.5% final methanol every 24 hours (Zhou et al., 2007; Thiruvengadam et al., 2011; Lau et al., 2010a). GRA4 was also expressed under the same conditions but for 3 days (Lau et al., 2010b). In addition to these T. gondii cytosolic proteins, membrane proteins such as SAG1 and SAG2 were also expressed in P. pastoris at 30°C for 4 days under continuous 24 hours of induction using 0.5-1% methanol concentrations (Zhou et al., 2007; Lau and Fong, 2008; Thiruvengadam et al., 2011; Lau et al., 2010a). Methanol concentration generally varies between 0.5% and 2% and even very low amount can stimulate induction in a short time of culture as it represents an important source of carbon for the yeast. Its continuous addition in the culture favors a good yield of protein expression. We also noticed that even a minimal expression environment could be achieved if good aeration conditions of the cultures were established. Using P. pastoris, we obtained enough quantity of purified Tg-NSORT that was analyzed by negative-staining electron microscopy. The purified protein was homogenous, and we observed ring-shaped particles with a diameter of about 8 nm, reminiscent of the crystal structure of human sortilin (Figure 5B; Figures 6A, B) (Quistgaard et al., 2009). We were to obtain a higher atomic resolution structure of the luminal domain of Tg-NSORT. However, we were not able to obtain crystals of this parasite protein domain. Alternatively, we used the AlphaFold program for predicting the structure of Tg-NSORT. We observed that the resulting model exhibits a very large and poorly structured insertion when compared to human sortilin (insert 2; see Figure 6C). We concluded that the presence of this parasite’s specific loop hinders crystallization of Tg-NSORT. Our analysis also revealed the possible molecular bases of the interactions between TgSORT and ROP proteins. It indicated that the pro-peptide domain of ROP could bind to the tunnel of TgSORT in a manner similar to neurotensin with human sortilin. The specific motifs of ROP proteins are located in some intrinsically disordered regions (IDR) with all IDR tested binding to the same site of TgSORT. These IDR corresponds to the pro-peptide situated at the N-terminus of all ROP proteins, and it is normally cleaved off during the maturation of ROP proteins before they reached their destination (Bradley and Boothroyd, 1999; Bradley and Boothroyd, 2001; Hajagos et al., 2012).
In conclusion, Pichia pastoris represents a good organism for T. gondii protein expression. This expression system combines the advantages of prokaryotic system with those of eukaryotic model for use of minimal and inexpensive culture medium, fast and high growth rate, high productivity, extracellular expression, folding and post-translational modifications (Karbalei et al., 2020). Large-scale production in improved conditions can lead to high amounts of well-folded proteins that can be used for various applications. Collectively, our data provides the foundation of future and deeper structure-function studies of TgSORT, the key receptor required for host infection of apicomplexan parasites. The expression of large amount of soluble ectodomain of TgSORT provides an avenue for conducting detailed mechanistic studies with biochemical and chemical approaches to identify new and parasite-specific inhibitors.
Data availability statement
The original contributions presented in the study are included in the article/Supplementary Material. Further inquiries can be directed to the corresponding author.
Author contributions
The author(s) have made the following declarations about their contributions: Conceived and designed the experiments: SZ-J and ST. Performed the experiments: AH, RG, TA, A-AA, MO, and SZ-J. Contributed reagents/materials/analysis tools: SZ-J, YH, and ST. Performed data analysis: AH, CA, LF, and SZ-J. Wrote the paper: AH, SZ-J, and ST. YH provided mass spectrometry access and revised the proteomics data and the manuscript. All authors contributed to the article and approved the submitted version.
Funding
Financial support for this work was provided by the Agence Nationale de la Recherche grants N°ANR-19-CE44-0006. AH was supported by the International Campus France and African UEMOA fellowships. This work benefited from the CryoEM platform of I2BC, supported by the French Infrastructure for Integrated Structural Biology (FRISBI) [ANR-10-INSB-05-05].
Acknowledgments
We thank Dr. P. Legrand (Synchrotron Soleil) for the AlphaFold2 calculations.
Conflict of interest
The authors declare that the research was conducted in the absence of any commercial or financial relationships that could be construed as a potential conflict of interest.
Publisher’s note
All claims expressed in this article are solely those of the authors and do not necessarily represent those of their affiliated organizations, or those of the publisher, the editors and the reviewers. Any product that may be evaluated in this article, or claim that may be made by its manufacturer, is not guaranteed or endorsed by the publisher.
Supplementary material
The Supplementary Material for this article can be found online at: https://www.frontiersin.org/articles/10.3389/fpara.2023.1103772/full#supplementary-material
References
Boothroyd J. C., Dubremetz J.-F. (2008). Kiss and spit: the dual roles of Toxoplasma rhoptries. Nat. Rev. Microbiol. 6, 79–88. doi: 10.1038/nrmicro1800
Bradley P. J., Boothroyd J. C. (1999). Identification of the pro-mature processing site of Toxoplasma ROP1 by mass spectrometry. Mol. Biochem. Parasitol. 100, 103–109. doi: 10.1016/S0166-6851(99)00035-3
Bradley P. J., Boothroyd J. C. (2001). The pro region of Toxoplasma ROP1 is a rhoptry-targeting signal. Intern. J. Parasitol. 31, 1177–1186. doi: 10.1016/S0020-7519(01)00242-9
Brydges S. D., Sherman G. D., Nockemann S., Loyens A., Däubener W., Dubremetz J.-F., et al. (2000). Molecular characterization of TgMIC5, a proteolytically processed antigen secreted from the micronemes of Toxoplasma gondii. Mol. Biochem. Parasitol. 111, 51–66. doi: 10.1016/S0166-6851(00)00296-6
Brydges S. D., Zhou X. W., Huynh M.-H., Harper J. M., Mital J., Adjogble K. D. Z., et al. (2006). Targeted deletion of MIC5 enhances trimming proteolysis of Toxoplasma invasion proteins. Eukaryot Cell 5, 2174–2183. doi: 10.1128/EC.00163-06
Butcher B. A., Fox B. A., Rommereim L. M., Kim S. G., Maurer K. J., Yarovinsky F., et al. (2011). Toxoplasma gondii rhoptry kinase ROP16 activates STAT3 and STAT6 resulting in cytokine inhibition and arginase-1-dependent growth control. PloS Pathog. 7, e1002236. doi: 10.1371/journal.ppat.1002236
Chang P. Y., Fong M. Y., Nissapatorn V., Lau Y. L. (2011). Evaluation of Pichia pastoris-expressed recombinant rhoptry protein 2 of Toxoplasma gondii for its application in diagnosis of toxoplasmosis. Am. J. Trop. Med. Hyg. 85, 485–489. doi: 10.4269/ajtmh.2011.11-0351
Cheng L., Zhang T., Fei Y., Shen H., Huang H., Chen J., et al. (2021). Expression, purification and characterization of recombinant human coagulation factor XIIIa in pichia patoris. Protein Pept. Lett. 28, 55–62. doi: 10.2174/0929866527666200625203240
Clee S. M., Yandell B. S., Schueler K. M., Rabaglia M. E., Richards O. C., Raines S. M., et al. (2006). Positional cloning of Sorcs1, a type 2 diabetes quantitative trait locus. Nat. Genet. 38, 688–693. doi: 10.1038/ng1796
Dubois D. J., Soldati-Favre D. (2019). Biogenesis and secretion of micronemes in Toxoplasma gondii. Cell. Microbiol. 21, e13018. doi: 10.1111/cmi.13018
Fentress S. J., Sibley L. D. (2011). The secreted kinase ROP18 defends Toxoplasma’s border. BioEssays 33, 693–700. doi: 10.1002/bies.201100054
Hajagos B. E., Turetzky J. M., Peng E. D., Cheng S. J., Ryan C. M., Souda P., et al. (2012). Molecular dissection of novel trafficking and processing of theToxoplasma gondii rhoptry metalloprotease toxolysin-1. Traffic 13, 292–304. doi: 10.1111/j.1600-0854.2011.01308
Hallée S., Boddey J. A., Cowman A. F., Richard D. (2018a). Evidence that the plasmodium falciparum protein sortilin potentially acts as an escorter for the trafficking of the rhoptry-associated membrane antigen to the rhoptries. mSphere 3, e00551–e00517. doi: 10.1128/mSphere.00551-17
Hallée S., Counihan N. A., Matthews K., de Koning-Ward T. F., Richard D. (2018b). The malaria parasite plasmodium falciparum sortilin is essential for merozoite formation and apical complex biogenesis. Cell Microbiol. 20, e12844.
Jansen P., Giehl K., Nyengaard J. R., Teng K., Lioubinski O., Sjoegaard S. S., et al. (2007). Roles for the pro-neurotrophin receptor sortilin in neuronal development, aging and brain injury. Nat. Neurosci. 10, 1449–1457. doi: 10.1038/nn2000
Jumper J., Evans R., Pritzel A., Green T., Figurnov M., Ronneberger O., et al. (2021). Highly accurate protein structure prediction with AlphaFold. Nature 596, 583–589. doi: 10.1038/s41586-021-03819-2
Karbalei M., Rezaee S. A., Farsiani H. (2020). Pichia pastoris: a highly successful expression system for optimal synthesis of heterologous protein. J. Cell Physiol. 235, 5867–5881. doi: 10.1002/jcp.29583
Kim T., Hempstead B. L. (2009). NRH2 is a trafficking switch to regulate sortilin localization and permit proneurotrophin-induced cell death. EMBO J. 28, 1612–1623. doi: 10.1038/emboj.2009.118
Kim K., Weiss L. M. (2004). Toxoplasma gondii: The model apicomplexan. Int. J. Parasitol. 34, 423–432. doi: 10.1016/j.ijpara.2003.12.009
Lau Y. L., Fong M. Y. (2008). Toxoplasma gondii: Serological characterization and immunogenicity of recombinant surface antigen 2 (SAG2) expressed in the yeast Pichia pastoris. Exp. parasitology. 119, 373–378. doi: 10.1016/j.exppara.2008.03.016
Lau Y. L., Fong M. Y., Idris M. M., Ching X. T. (2012). Cloning and expression of toxoplasma gondii dense granular protein 2 (GRA2) gene by pichia pastoris. Southest Asian J. Trop. Med. Public Health 43, 10–16.
Lau Y. L., Hassan M. T., Thiruvengadam G., Idris M. M., Init I. (2010b). Cloning and expression of toxoplasma gondii dense granular protein 4 (GRA4) in pichia pastoris. Trop. Biomedicine 27 (3), 525–533.
Lau Y. L., Init I., Fong M. Y. (2010a). Optimization for high-level expression in Pichia pastoris and purification of truncated and full length recombinant SAG2 of Toxoplasma gondii for diagnostic use. Southest Asian J. Trop. Med. Public Health 41, 507–513.
Marcusson E. G., Horazdovsky B. F., Cereghino J. L., Gharakhanian E., Emr S. D. (1994). The sorting receptor for yeast vacuolar carboxypeptidase y is encoded by the VPS10 gene. Cell 77, 579–586. doi: 10.1016/0092-8674(94)90219-4
Morrissette N. S., Sibley L. D. (2002). Cytoskeleton of apicomplexan parasites. Microbiol. Mol. Biol. Rev. 66, 21–38. doi: 10.1128/MMBR.66.1.21-38.2002
Nielsen M. S., Madsen P., Christensen E. L., Nykjaer A., Gliemann J., Kasper D., et al. (2001). The sortilin cytoplasmic tail conveys golgi-endosome transport and binds the VHS domain of the GGA2 sorting protein. EMBO J. 20, 2180–2190. doi: 10.1093/emboj/20.9.2180
Nikolajsen C. L., Dyrlund T. F., Poulsen E. T., Enghild J. J., Scavenius C. (2014). Coagulation factor XIIIa substrates in human plasma. J. Biol. Chem. 289, 6526–6534. doi: 10.1074/jbc.M113.517904
Opitz C., Soldati D. (2002). ‘The glideosome’: a dynamic complex powering gliding motion and host cell invasion by Toxoplasma gondii. Mol. Microbiol. 45, 597–604. doi: 10.1046/j.1365-2958.2002.03056.x
Pappas G., Roussos N., Falagas M. E. (2009). Toxoplasmosis snapshots. global status of Toxoplasma gondii seroprevalence and implications for pregnancy and congenital toxoplasmisis. Int. J. Parasitol. 39, 1385–1394. doi: 10.1016/j.ijpara.2009.04.003
Quistgaard E. M., Madsen P., Groftehauge M K., Nissen P., Petersen C. M., Thirup S. S. (2009). Ligands bind to sortilin in the tunnel of a ten-bladed beta-propeller domain. Nat. Struct. Mol. Biol. 16, 96–98. doi: 10.1038/nsmb.1543
Rogaeva E., Meng Y., Lee J. H., Gu Y., Kawarai T., Zou F., et al. (2007). The neuronal sortilin-related receptor SORL1 is genetically associated with alzheimer’s disease. Nat. Genet. 39, 168–177. doi: 10.1038/ng1943
Sakura T., Sindikubwabo F., Oesterlin L. K., Bousquet H., Slomianny C., Hakimi M.-A., et al. (2016). A critical role for Toxoplasma gondii vacuolar protein sorting VpS9 in secretory organelles biogenesis and host infection. Sci. Rep. 6, 38842. doi: 10.1038/srep38842
Sangaré L. O., Alayi T. D., Hovasse A., Westermann B., Sindikubwabo F., Callebaut I., et al. (2016). Unconventional endosome-like compartment and retromer complex in Toxoplasma gondii govern parasite integrity and host infection. Nat. Commun. 7, 10191. doi: 10.1038/ncomms11191
Sloves P.-J., Delhaye S., Mouveaux T., Werkmeister E., Slomianny C., Hovasse A., et al. (2012). Toxoplasma sortilin-like receptor regulates protein transport and is essential for apical secretory organelle biogenesis and host infection. Cell Host Microbe 11, 515–527. doi: 10.1016/j.chom.2012.03.006
Sloves P.-J., Mouveaux T., Ait-Yahia S., Vorng H., Everaere L., Sangare L. O., et al. (2015). Apical organelle secretion by Toxoplasma controls innate and adaptive immunity and mediates long-term protection. J. Infect. Dis. 212, 1449–1458. doi: 10.1093/infdis/jiv250
Thiruvengadam G., Init I., Fong M. Y., Lau Y. L. (2011). Optimization of the expression of surface antigen SAG1/2 of Toxoplasma gondii in the yeast Pichia pastoris. Trop. Biomed. 28, 506–513.
Wang N., Wang K. Y., Li G., Guo W., Liu D. (2015). Expression and characterization of camel chymosin in Pichia pastoris. Protein Expr Purif. 111, 75–81. doi: 10.1016/j.pep.2015.03.012
Wu S., Letchworth G. J. (2004). High efficiency transformation by electroporation of Pichia pastoris pretreated with lithium acetate and dithiothreitol. BioTechniques 36, 152–154. doi: 10.2144/04361DD02
Keywords: Toxoplasma gondii, sortilin, Pichia pastoris, AlphaFold, 3D-models
Citation: Honfozo A, Ghouil R, Alayi TD, Ouldali M, Arteni A-A, Atindehou CM, Fanou LA, Hathout Y, Zinn-Justin S and Tomavo S (2023) The luminal domain of Toxoplasma gondii sortilin adopts a ring-shaped structure exhibiting motifs specific to apicomplexan parasites. Front. Parasitol. 2:1103772. doi: 10.3389/fpara.2023.1103772
Received: 21 November 2022; Accepted: 17 January 2023;
Published: 30 January 2023.
Edited by:
Mark C. Field, University of Dundee, United KingdomReviewed by:
Erica S. Martins-Duarte, Federal University of Minas Gerais, BrazilJoel Bryan Dacks, University of Alberta, Canada
Copyright © 2023 Honfozo, Ghouil, Alayi, Ouldali, Arteni, Atindehou, Fanou, Hathout, Zinn-Justin and Tomavo. This is an open-access article distributed under the terms of the Creative Commons Attribution License (CC BY). The use, distribution or reproduction in other forums is permitted, provided the original author(s) and the copyright owner(s) are credited and that the original publication in this journal is cited, in accordance with accepted academic practice. No use, distribution or reproduction is permitted which does not comply with these terms.
*Correspondence: Stanislas Tomavo, c3RhbmlzbGFzLnRvbWF2b0BpMmJjLnBhcmlzLXNhY2xheS5mcg==